- Laboratory of Behavioral and Developmental Genetics, Department of Human Genetics, KU Leuven, Leuven, Belgium
The insulin-producing cells (IPCs), a group of 14 neurons in the Drosophila brain, regulate numerous processes, including energy homeostasis, lifespan, stress response, fecundity, and various behaviors, such as foraging and sleep. Despite their importance, little is known about the development and the factors that regulate morphological and functional differentiation of IPCs. In this study, we describe the use of a new transgenic reporter to characterize the role of the Drosophila L1-CAM homolog Neuroglian (Nrg), and the transmembrane Semaphorin-1a (Sema-1a) and its receptor Plexin A (PlexA) in the differentiation of the insulin-producing neurons. Loss of Nrg results in defasciculation and abnormal neurite branching, including ectopic neurites in the IPC neurons. Cell-type specific RNAi knockdown experiments reveal that Nrg, Sema-1a and PlexA are required in IPCs and glia to control normal morphological differentiation of IPCs albeit with a stronger contribution of Nrg and Sema-1a in glia and of PlexA in the IPCs. These observations provide new insights into the development of the IPC neurons and identify a novel role for Sema-1a in glia. In addition, we show that Nrg, Sema-1a and PlexA in glia and IPCs not only regulate morphological but also functional differentiation of the IPCs and that the functional deficits are likely independent of the morphological phenotypes. The requirements of nrg, Sema-1a, and PlexA in IPC development and the expression of their vertebrate counterparts in the hypothalamic-pituitary axis, suggest that these functions may be evolutionarily conserved in the establishment of vertebrate endocrine systems.
Introduction
The insulin-producing cells (IPCs) are a cluster of 14 neurosecretory neurons located in the pars intercerebralis of the Drosophila brain. The IPCs are the major source of the Drosophila insulin-like peptides (Dilps). They express Dilp2, -3, and -5, which are secreted into the circulatory hemolymph via contacts on the aorta (1). Loss of IPCs or IPC function results in insulin/insulin-like growth factor signaling defects, which in turn affect numerous processes, including energy homeostasis, growth and body size, stress resistance, lifespan, fecundity, and behavior (2, 3)
Despite the importance of the IPC neurons, very little is known about their development. While the lineage of the IPCs and the factors and pathways contributing to their specification have been well-described (4, 5), the factors that regulate the morphological and functional differentiation of the IPCs remain almost completely unknown. We have previously demonstrated that the transcription factor Eyeless is required both for the proper differentiation of the IPC neurons as well as the expression of dilp5 (6). It was later shown that the transcription factor Dachshund also regulates dilp5 transcription via an interaction with Eyeless, but no defects in IPC differentiation were reported in dachshund loss-of-function animals (7). A study to identify factors contributing to insulin-like peptide production identified a role for Unc-104 and Rab1 in IPC development, where loss of either protein resulted in larvae with reduced IPC cell number and aberrant IPC morphology (8).
Important classes of molecules in the regulation of neuronal morphogenesis are cell-adhesion molecules (CAMs) and axon guidance molecules (9–11). Glia are closely associated with neurons and exercise significant control over their development, yet our knowledge of the mechanistic basis thereof is far from complete (12, 13). The L1-type cell-adhesion molecules are a family of evolutionarily conserved CAMs important for axonal pathfinding and synaptogenesis during nervous system development in vertebrates and invertebrates (10, 14). Mutations in these genes lead to severe neural defects in various species (15–17). In Drosophila, the L1-CAM homolog Neuroglian (Nrg) is expressed in neuronal and glial cells. Elav-dependent differential splicing results in the neuron-specific isoform Nrg-180, while the more broadly expressed Nrg-167 isoform is found in glial cells (18–21). We and others have previously shown that nrg and Semaphorin-1a (Sema-1a) interact genetically (22, 23). Sema-1a, a transmembrane glycoprotein, and its receptor Plexin A (PlexA) act in pathfinding, where both Sema-1a and PlexA can serve as receptor (24–32).
Here we show that Nrg, Sema-1a, and PlexA are required for IPC differentiation. nrg loss-of-function results in IPCs exhibiting defasciculation in the median bundle, neurite pathfinding defects resulting in ectopic branches, and aberrant outgrowth of the lateral and subesophageal neurites. We also show that the ectopic branches are the result of loss of Neuroglian function in glia, and that Sema-1a and its receptor PlexA are also required in this process of neuritogenesis. In addition, we find evidence for a possible role of Nrg in the recruitment or organization of synaptic protein complexes in IPCs. Furthermore, we demonstrate that Nrg, Sema-1a, and PlexA not only regulate morphological but also physiological differentiation of IPCs.
Materials and Methods
Transgenes and Drosophila Stocks
The following fly stocks were used: Canton-S (CanS, wildtype), nrg849 and nrg892 (23, 33), nrgGFP [also known as nrgG00305, Bloomington Stock Center (34, 35)], nrg3 (36), nrg1 [Bloomington Stock Center (36)], UAS-nrg RNAi [Vienna Drosophila RNAi Center, VDRC #6688; validated in (23)], UAS-Nrg-180 and UAS-Nrg-167 (23, 37), 30B07-Gal4 [Bloomington Stock Center (38)], Dilp2-Gal4 (1), repo-Gal4 (39), UAS-DenMark (40), UAS-mCD8-GFP (Bloomington Stock Center), PlexAGFP [PlexAYD0269 (41)], Sema-1aGFP [Sema-1aCA07125 (42)], UAS-Sema-1a (gift from Corey Goodman), UAS-Sema-1a RNAi [Bloomington Stock Center, TRiP.HMS01307; validated in (43)], UAS-PlexA RNAi [Bloomington Stock Center, TRiP.HM05221; validated in (43)], UAS-mCherry RNAi (Bloomington Stock Center, TRiP VALIUM20-mCherry, attP2) and Dilp2-DenMark (this study).
Dilp2-DenMark (D2:DM), in which DenMark (40) was expressed under the direct control of the dilp2 enhancer (1) was used to visualize the IPC neurons and their neurites. The p{Dilp2-DenMark} construct was generated by digesting pPTGAL (44) with PstI and removing the portion of the plasmid containing the Gal4 and the polyadenylation sequences. The dilp2 enhancer, which was generated by PCR amplification of wildtype genomic DNA using the dilp2-enhancer-F and dilp2-enhancer-R primers, was then cloned into the StuI and KpnI sites within the multiple cloning site of the truncated pPTGAL. Next, the PstI fragment containing the multiple cloning site and the polyadenylation sequence from pUAST (45) was cloned into the PstI site approximately 150 bp downstream of the previously cloned dilp2 enhancer, creating p{Dilp2}. To generate the p{Dilp2-DenMark} construct, DenMark cDNA was obtained by PCR amplification of p{UAS-DenMark} (gift from Bassem Hassan) using the DenMark-F and DenMark-R primers and cloned into the BglII and XbaI sites of p{Dilp2}.
The sequences of the PCR primers used for cloning are as follows:
dilp2-enhancer-F: 5’- CTAAGGCCTCCAACACACACACATTCATTACCCA;
dilp2-enhancer-R: 5’- CTAGGTACCTGGTTATGGGTTTACTGCTTAGGTTG;
DenMark-F: 5’- CTAAGATCTGCCACCATGCCGGGGCCTTCGCCAGGGCT;
DenMark-R: 5’- CTATCTAGATTATCAGGAAGATGTCAGCTGGATAG
Immunohistochemistry
Whole-mount larval and adult brain immunohistochemistry was performed as previously described (6, 46).
The following primary antibodies were used: mouse anti-GFP (Roche) was used at 1:500, mouse anti-GFP monoclonal antibody 8H11 (Developmental Studies Hybridoma Bank) was used at 1:200, rabbit anti-dsRed (Clontech) was used at 1:500, rabbit anti-Dilp2 (1) was used at 1:1000, rabbit anti-Dilp3 (46) was used at 1:500, rabbit anti-Dilp5 (46) was used at 1:200. Secondary antibodies conjugated to either FITC or Cy3 were used at 1:200 (Jackson ImmunoResearch).
Quantification of the large DenMark-positive densities in the subesophageal region of the adult insulin-producing neurons was obtained by the analysis of confocal stacks with ImageJ (47) and Imaris software (version 6.1.0; Olympus). Images of the immunostained brains were scanned in a single sitting using the same confocal settings for both the nrg849 and the wildtype brains. The intensity of the DenMark signals was measured from a defined region at the level of the suboesophageal ganglion (SOG; area of defined region was kept constant for all samples).
Quantification of Dilp peptide levels in IPCs was done based on fluorescence intensity as described (46).
Determination of Body Weight
Body weight was determined by weighing individual 3-day-old males. Flies were anaesthetized and collected with chloroform instead of CO2 to avoid desiccation. The nrg849 stock was isogenized by outcrossing for three generations to CanS.
Stress Resistance
For both starvation resistance and oxidative stress resistance, 3-5 day-old adult nrg849 and CanS flies or Dilp2-Gal4/+; UAS-nrgRNAi/+ and CyO/+; UAS-nrgRNAi/+ sibling controls were used. For the additional starvation resistance experiments, repo-Gal4 was also used, as well as Sema-1aRNAi and PlexARNAi. For these two RNAis, a neutral mCherry RNAi that was generated in the same vector and inserted into the same genomic site was used as a control. For starvation resistance, flies were kept on 2% agar, and dead flies were scored multiple times per day. For the oxidative stress resistance experiments, flies were kept on 2% agar, 5% sucrose, 20 mM paraquat and scored in bins of 30 minutes using Drosophila Activity Monitors (Trikinetics, Waltham, MA, USA). For both stress resistance experiments, flies were housed in an incubator at 25°C on a 12/12-hour on/off light cycle. Resistance to starvation or oxidative stress was defined as the time of death after initiation of food deprivation or paraquat exposure, respectively.
qRT-PCR Analysis
Sample preparation and qRT-PCR analysis was done as described (46). Briefly, total RNA was extracted from adult heads with TRI reagent (Invitrogen) and reverse transcribed with Transcriptor First Strand Synthesis kit (Roche). qRT-PCR was done using SYBR Green Detection on a StepOnePlus™ Real-Time PCR System. The geometric means of RpS13 and Rp49 were used for normalization. Relative expression levels were calculated with the ΔΔCt method (48). qRT-PCR primer sequences are:
dilp2: Forward GCGAGGAGTATAATCCCGTGAT, Reverse GGATTGAGGGCGTCCAGAT;
dilp3: Forward GCAATGACCAAGAGAACTTTGGA, Reverse GCAGGGAACGGTCTTCGA
dilp5: Forward GAGGCACCTTGGGCCTATTC, Reverse CATGTGGTGAGATTCGGAGCTA
Nrg: Forward ACGATGCACAGGATCAAGGG, Reverse ATCTAAGCCACCGACCTCCT
Sema1a: Forward ACTTTGAAGATATAATCAACGCCCA, Reverse AGCCTGTAAAGAAGCCGACC
PlexA: Forward GGAAAACAAACAGTTTACATGATGG, Reverse TGCGTTGGTGTCATTCTTGC
Dsk: Forward CTGGGCATTAGCCTTCTGCT, Reverse CCTGTAGTCGCCGATCATCC
Rp49: Forward GCCCAAGGGTATCGACAACA, Reverse CTTGCGCTTCTTGGAGGAGA
RpS13: Forward GGGTCTGAAGCCCGACATT, Reverse GGCGACGGCCTTCTTGAT
Statistical analysis
All statistics were conducted using GraphPad Prism 9.1.1. For assays comparing means, unpaired T-tests for pairwise comparisons were used. Non-parametric results were analyzed using the Mann Whitney U test. For lifespan analysis under oxidative or starvation stress, median lifespan was compared with a log-rank test. For all statistical analyses, we assumed a significance level of 0.05.
Results
Neuroglian Is Required for Function and Differentiation of the Insulin-Producing Neurons
The insulin-producing cells (IPCs) are a cluster of neurons in the pars intercerebralis of the brain that express Dilps (1, 49, 50). Given that the Nrg enhancer reporters 29H05 and 30B07 drive strong expression in the pars intercerebralis (unpublished observations), we examined whether any of the labelled cells were IPCs. Immunohistochemical analysis revealed that the IPC marker Dilp2 is co-expressed with the 30B07 enhancer reporter (Figure 1A). To further validate this finding, we looked for endogenous Nrg expression in the IPCs. Using a GFP-tagged allele of nrg (nrgGFP) (34, 35), we observed clear coexpression of Dilp2 and Neuroglian, confirming that Nrg is expressed in the IPCs (Figures 1B, C).
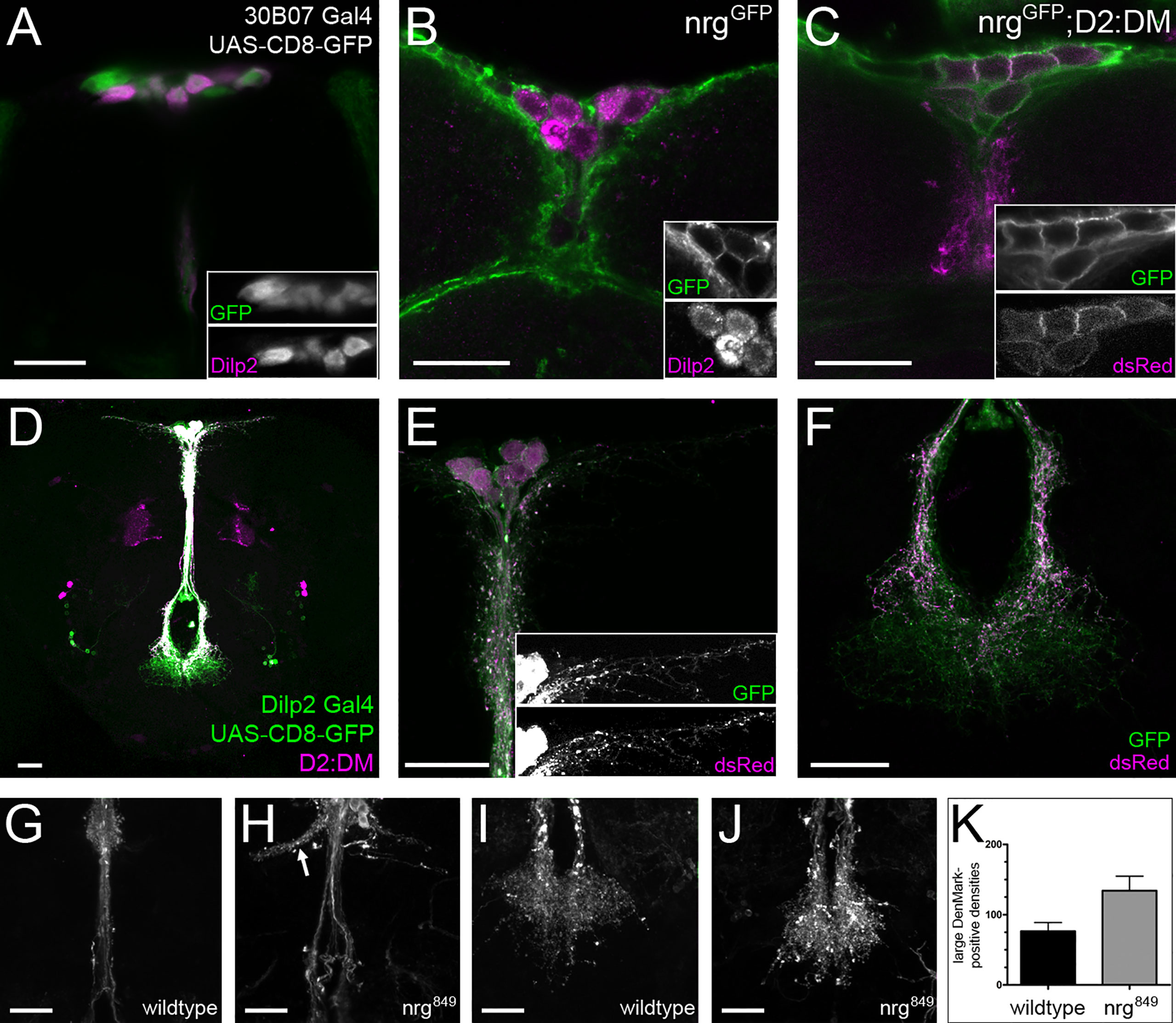
Figure 1 nrg is expressed in insulin-producing cells and required for their differentiation. (A) The Nrg Gal4 reporter 30B07 is expressed in the IPCs, as shown with double-labeling with anti-GFP (green) and anti-Dilp2 (magenta) (B, C) Expression of Nrg (α-GFP, green) can be seen in nrgGFP brains in the IPCs, labeled with Dilp2 (magenta) as well as with the D2:DM IPC marker (α-dsRed, magenta). (D–F) The D2:DM marker (α-dsRed, magenta) is coexpressed in the IPCs with Dilp2 Gal4 > UAS-CD8-GFP (α-GFP, green). (G, H) The IPCs of nrg849 mutants display defasciculation and ectopic branching phenotypes (marked by the arrow) as well as (I-K) a significant increase in the number of large DenMark-positive densities (unpaired t-test: P=0.027; wildtype, mean = 76.36 ± 12.61, n = 11; nrg849, mean = 134.1 ± 20.66, n = 11). Adult brains were used in all panels. Scale bars = 20μm.
To investigate whether nrg is required for normal IPC development, we generated an IPC marker (named D2:DM for Dilp2:DenMark) in which the expression of the somatodendritic marker DenMark is under the direct control of the dilp2 promoter (1, 40). D2:DM is Gal4-independent, thus enabling us to drive RNAi and rescue constructs in varying cell types without affecting the IPC marker. We observed that the D2:DM marker labels the complete IPC neuron (Figures 1C–F) despite DenMark being a selective marker for the somatodendritic compartment of neurons. This indicates that the IPCs dendritic and axonal compartments are not strictly delineated but rather that axoaxonal synapses are formed on the IPCs. We examined the IPCs in nrg849 mutants, a homozygous-viable hypomorph, revealing multiple abnormalities in IPC morphology. One of the strongest phenotypes observed in nrg mutants was the strong defasciculation of the neurite projections along the median bundle (Figures 1G, H). Additionally, in nrg mutants we observe ectopic branches originating from the median bundle, with the ectopic neurites projecting alongside the superior arch commissure (51, 52). These branches form horn-like projections containing a variable number of loosely-associated neurites, which project away from the midline (Figures 1G, H). Neurite complexity is also affected in nrg mutants, as there are fewer lateral neurites on either side of the soma (Figure 3B). In addition, a 75% increase in the number of large DenMark-positive densities within the neurites of the suboesophageal projections is observed (Figures 1I–K), suggesting that the distribution of dendritic components and/or synapse formation and remodeling may be altered in nrg mutants.
No effect on body size of nrg849 mutants was observed (Figure 2A), suggesting that the transport and secretion of Dilps, and most prominently Dilp2, to the aorta, where the Dilps are secreted into the circulating hemolymph (1), is not significantly affected. However, we found that nrg849 mutants display other phenotypes associated with insulin signaling in the IPCs, such as enhanced resistance to stress (2, 53). nrg849 mutants show increased resistance to both oxidative stress and starvation (Figures 2B, C). Furthermore, these phenotypes persist in flies in which nrg is knocked-down specifically in the IPCs (Figures 2D and 9A), consistent with Nrg being required cell-autonomously in the IPCs for normal function.
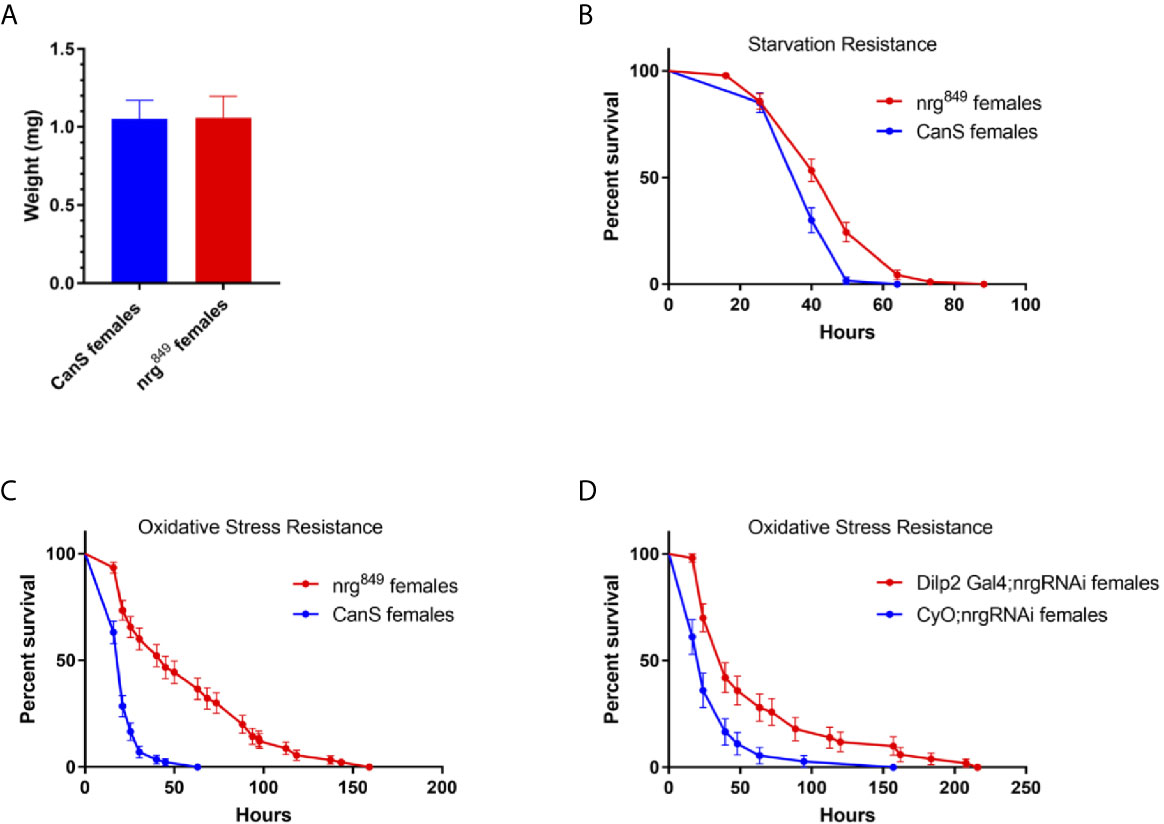
Figure 2 Loss of Nrg results in phenotypes associated with disrupted insulin signaling. (A) While there is no difference in nrg849 animal size (P=0.790, Mann Whitney U test), nrg849 mutants are more resistant to (B) starvation (P=0.0005, log-rank test; wildtype median survival = 40 hours, nrg849 median survival = 49.75 hours) and (C) oxidative stress (P < 0.0001, log-rank test; wildtype median survival = 21 hours, nrg849 median survival = 45 hours). Similarly, IPC-specific knockdown of nrg also results in increased resistance to (D) oxidative stress (P < 0.0001, log-rank test; wildtype median survival = 24 hours, Dilp2 Gal4:nrg RNAi median survival = 39.5 hours).
To investigate whether the phenotypes observed in nrg849 mutants are also seen with other alleles, we analyzed IPC morphology in adults of other allelic combinations of nrg, using alleles nrg892, nrgGFP, nrg3, and nrg1. nrg892 and nrgGFP are hypomorphic alleles, nrg3 is a temperature-sensitive allele that behaves as a functional null allele at restrictive temperature, and nrg1 is a homozygous lethal null allele. All allelic combinations examined showed phenotypes similar to what we observed in nrg849, albeit with variable penetrance (Figure 3 and Table 1). Frequency of ectopic branching was lower in nrg892 and nrg3 hemizygous males (the nrg locus is on the X chromosome), as well as in nrg849/nrg3 females. The observed reduction of lateral neurites was also less penetrant in the three transheterozygous conditions (Table 1).
We next examined the development of the IPC neurons throughout late larval and pupal stages to better understand the developmental origin of the nrg phenotype. In wildtype larvae, there are two types of prominent IPC neurites: those that project contralaterally and leave the brain to innervate the corpora cardiaca, and those that project ipsilaterally to the subesophageal ganglion (8). While in the larval stage the projections to the corpora cardiaca are outside the brain, these two groups of projections will eventually both project along the median bundle in the adult until they approach the esophageal foramen. In 25% pupae the median bundle has fully extended into the subesophageal ganglion, but the distal neurites of the median bundle projection from each hemisphere are not yet organized into the uniform fan-shape seen in the adult until the end of the pupal stage (see Figures 1F, I). In 50% pupae, the cell bodies from the two hemispheres are clustered at the midline and the median bundle fascicles dorsal of the esophagus have completely merged into one median neuropil. Refinement of the median bundle continues into later pupal stages. In nrg849 mutants, a median bundle defasciculation phenotype can be observed from the 25% pupal stage onwards. Elongation of the lateral neurites also begins in early pupae, where proximoventrally of the IPC soma the projections extend laterally from the neuronal fascicle (Figure 4, 25% pupa). These neurites also continue to extend and become more elaborate throughout pupal development. Additionally, the clustering of the neurons and the merger of the neurite projections from the two hemispheres is affected in nrg849 animals. Abnormal clustering of cell bodies was seen consistently in adult brains of nrg849 mutant animals (Figure 4, Adult). Ectopic neurites are first observed in 75% pupae, and therefore are formed in a later stage of development when all the neurites of a wildtype IPC neuron have already been formed and are in an advanced stage of development (Figure 4, 75% pupa). Since no comparable neurite formation is seen during wildtype IPC development, we conclude that this phenotype is the result of ectopic neurite formation, rather than a failure to prune neurites transiently found during development.
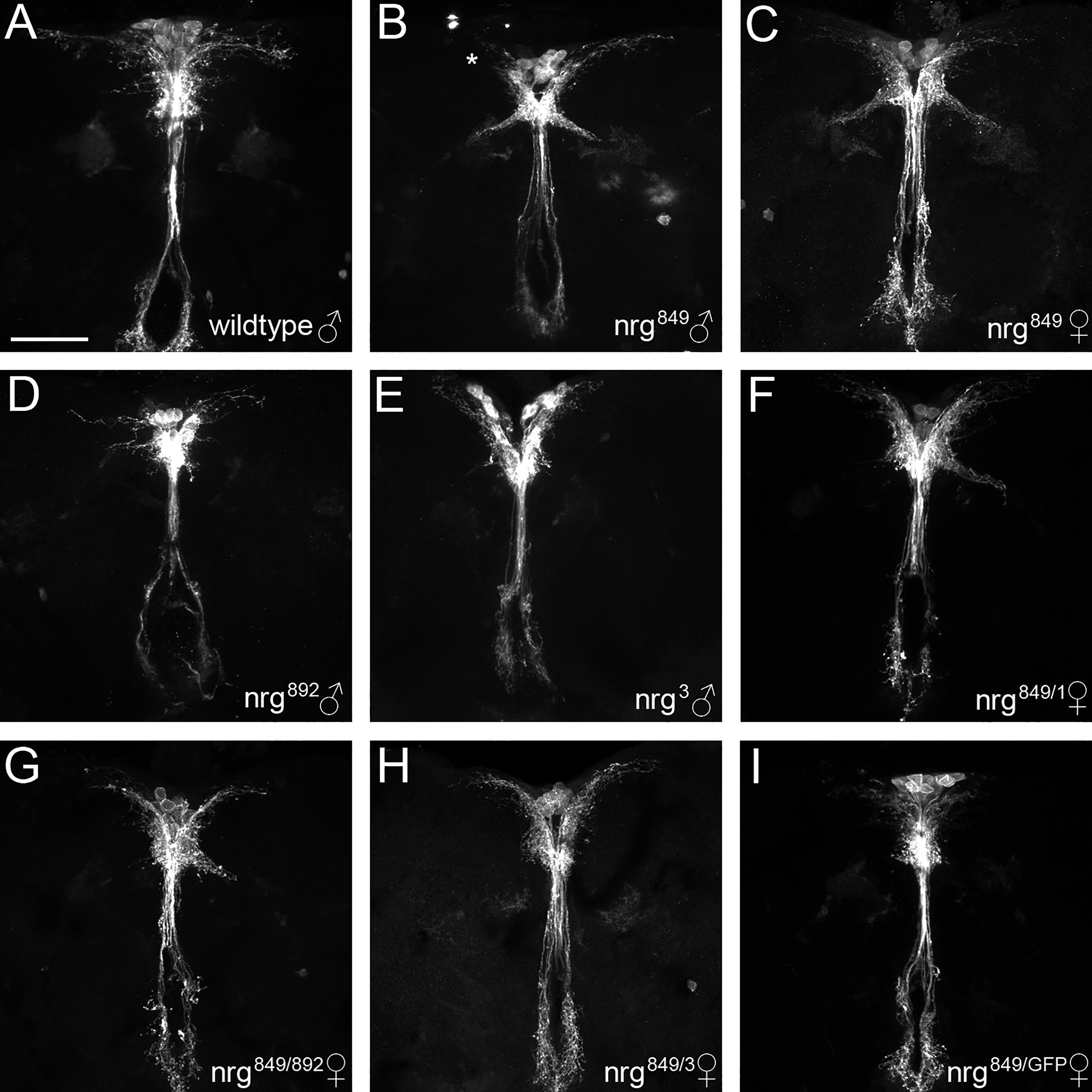
Figure 3 Complementation analysis of nrg alleles in IPC neuron development. Compared to (A) wildtype adult IPCs, (B, C) nrg849 mutants display ectopic branches, median bundle defasciculation, and loss of lateral neurite complexity (asterisk in B). (D-I) Similar phenotypes can be seen in nrg892 and nrg3 hemizygous males, and nrg849/1, nrg849/892, nrg849/3, and nrg849/GFP transheterozygous females. Flies with the temperature-sensitive nrg3 allele were raised at the permissive temperature of 18°C and shifted to the restrictive temperature of 29°C as wandering 3rd instar larvae. IPCs were visualized using Dilp2-GAL4 >GFP-CD8. Scale bar = 50μm.
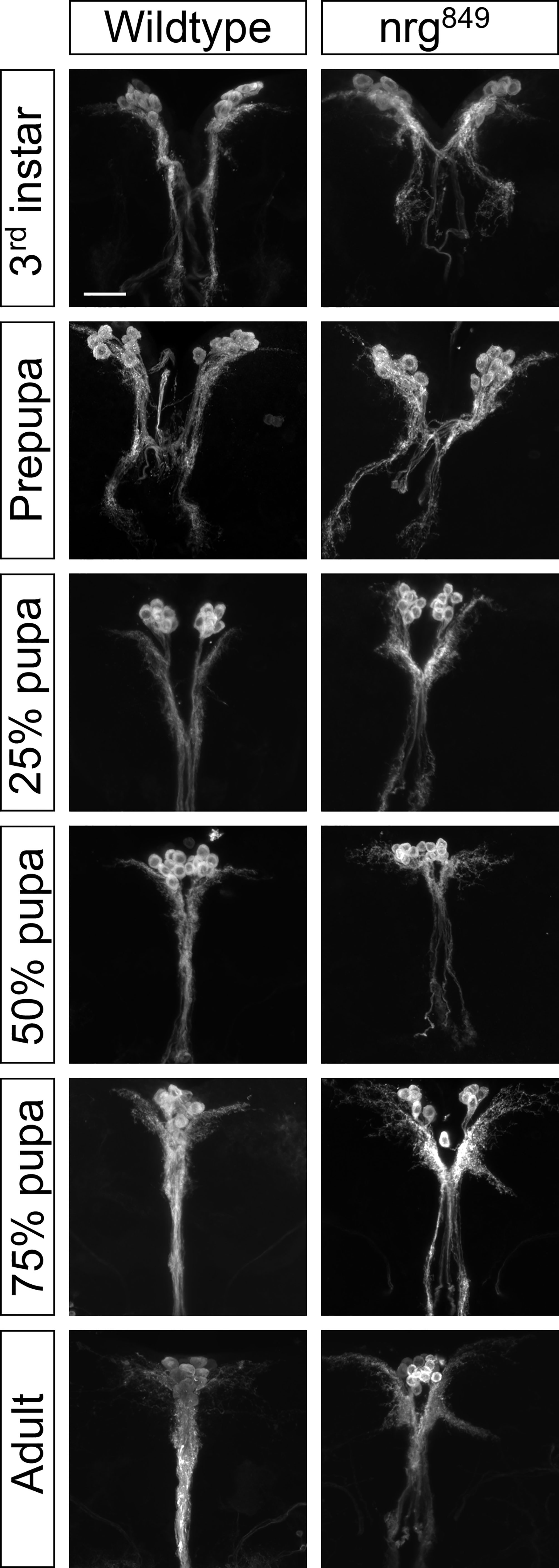
Figure 4 IPC development was studied by imaging the neurons at different stages throughout development (wandering 3rd instar larvae, prepupa, 25% pupa (24 hours after puparium formation (APF)), 50% pupa (48 hours APF), 75% pupa (72 hours APF), and 3-5 day old adults) of flies in which the IPC neurons were labeled via UAS-CD8-GFP driven by Dilp2 Gal4. Scale bar = 25μm.
Loss of Glial Neuroglian Leads to Ectopic Branches
To further investigate the ectopic branching phenotype, we sought to address whether these defects in IPC differentiation were due to a cell-autonomous requirement of Nrg within the IPC neurons themselves. We specifically knocked down nrg levels in the IPCs using Dilp2-Gal4 to drive a previously characterized nrg RNAi line that effectively phenocopies loss-of-function mutations (23). Specificity of Dilp2-Gal4 and of efficiency of the Nrg-RNAi was verified using a combination of immunohistochemistry and qRT-PCR (see Supplemental Figures S1 and S2). IPC-specific knockdown of nrg did not reproduce any of the ectopic branching phenotypes observed in nrg849 mutants and resulted only in subtle neurite phenotypes (Figures 5A, C and Table 2). However, in 33% of the brains examined, single ectopic pioneer neurites could be seen (Figure 5I). Additionally, neither the IPC-specific expression of Nrg-180 nor Nrg-167 rescued either the ectopic branching or the defasciculation phenotypes in nrg849 mutants (Figure 6C and data not shown). Nrg-RNAi mediated knockdown using Dilp2-Gal4 had no impact on IPC cell number (see Supplemental Figure S3).
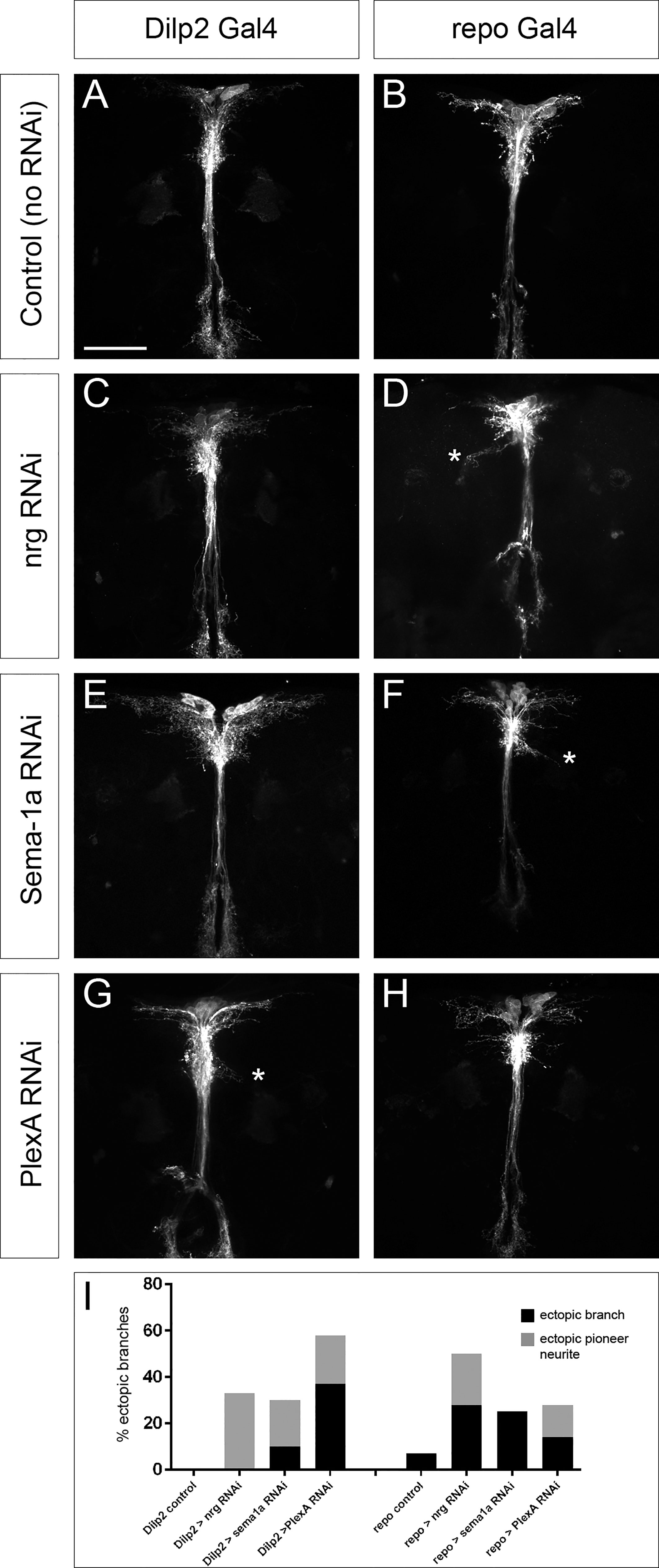
Figure 5 Cell- and non-cell autonomous contributions of nrg, Sema-1a, and PlexA to the IPC neuronal differentiation phenotype. Cell-type specific RNAi knockdowns were performed and compared to (A, B) control brains with the Gal4 driver alone. (C) Brains in which nrg was knocked down in the IPCs do not exhibit any major phenotypes, while (D) nrg knockdown in glia results in ectopic branching as well as a reduction in lateral neurite complexity. (E) IPC knockdown of Sema-1a results primarily in an increase in lateral neurite complexity, but also occasionally in mild ectopic branch phenotypes (not shown; see Table 2). (F) Sema-1a knockdown in glial cells results in more robust ectopic branches. (G) Knockdown of the Sema-1a receptor PlexA in IPC neurons gives rise to ectopic branches with high penetrance, while (H) knockdown of PlexA in glia results in ectopic branches much less frequently. (I) Graph showing the frequency of ectopic branching phenotypes (both ‘mature’ ectopic branches as well as immature single-neurite pioneers) in the different RNAi knockdown conditions. Ectopic branches in (D, F, G) are marked with an asterisk. Scale bar = 50μm.
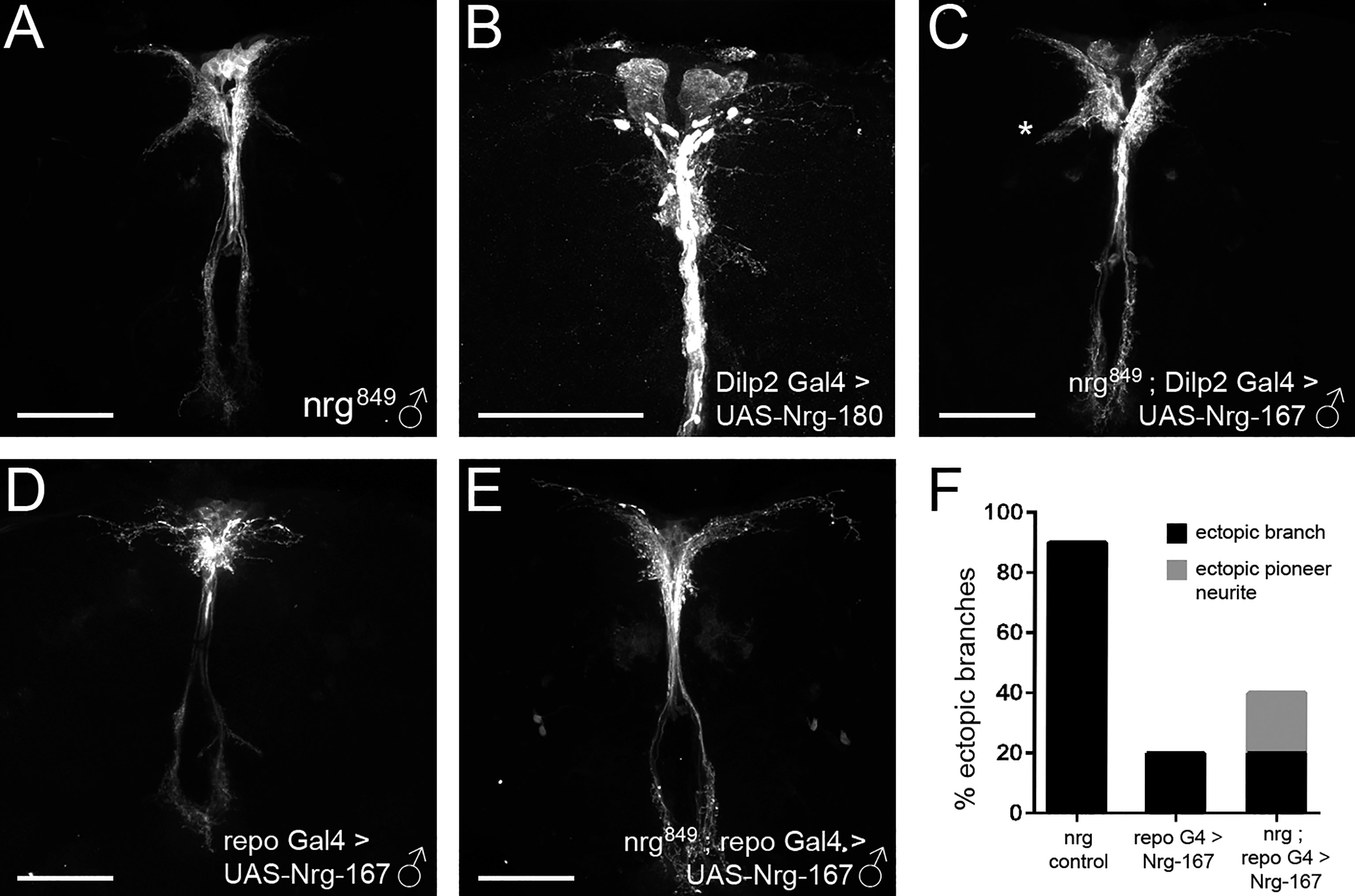
Figure 6 Glial-specific expression of Nrg rescues the ectopic branching phenotype of nrg849 mutants. Attempts to rescue the (A) ectopic branch phenotype of nrg849 hemizygous males with (C) IPC-specific expression of Nrg failed to rescue the ectopic branching (marked by asterisk). However, (E, F) restoration of Nrg-167 expression in glia was sufficient to rescue the ectopic branching phenotype in nrg849 hemizygous males. Overexpression of Nrg in (B) IPC neurons or (D) glia results in decreases in lateral neurite complexity as well as a redistribution of DenMark. Scale bars = 50μm.
Given our results demonstrating that the observed nrg849 IPC morphological defects are largely non-autonomous and the previously described link between Nrg-mediated neuron-glia interactions and axon sprouting (35, 54), we investigated whether glia-expressed Nrg had any role in IPC differentiation. Using the repo-Gal4 driver to express nrg RNAi, we specifically knocked down nrg in glial cells. Specificity of Repo-Gal4 was verified by means of immunohistochemistry (see Supplemental Figure S1). Glial loss of nrg results in IPCs with ectopic branches, median bundle defasciculation, and aberrant neurite arborization (Figures 5B, D and Table 2). To further test the requirement of glia-expressed Nrg in IPC ectopic branch formation, we attempted to rescue the ectopic branching phenotype seen in nrg849 hemizygous males by expressing the Nrg-167 isoform with repo-Gal4. Expression of Nrg-167 in glia was sufficient to rescue the ectopic branching phenotype, further demonstrating that Nrg expression in the glial cells surrounding the IPC neurons plays a crucial role in regulating IPC neurite branching (Figures 6A, E, F).
In the course of the rescue experiments, we observed that overexpression of the Nrg-180 isoform in wildtype IPCs resulted in a dramatic decrease in lateral neurite branching (Figure 6B). Furthermore, there is a clear redistribution of the dendritic DenMark marker in the lateral neurites, where DenMark is predominantly localized proximoventrally of the IPC soma instead of the more diffuse localization observed in wildtype (Figure 6B). Similar to the overexpression phenotypes seen in the IPCs, overexpression of Nrg-167 in glia resulted in IPC neurons with reduced lateral neurite complexity as well as aberrant DenMark localization (Figure 6D).
Disruption of Semaphorin-1a/PlexinA Signaling Between IPCs and Glia Results in Ectopic Branches
It has been shown that Nrg-mediated axon guidance can be influenced by Sema-1a (23, 54). To determine whether Sema-1a/PlexA signaling might also play a role in IPC neurite pathfinding, we first examined whether they are expressed in the IPCs. Using GFP-tagged alleles, we observed that, like Nrg, both Sema-1a and PlexA are broadly expressed in the pars intercerebralis, with expression in the IPCs as well as in neighboring cells (Figures 7A–C). To investigate the role of these molecules in IPC differentiation, we performed targeted knockdown experiments in which an RNAi against either Sema-1a or PlexA was specifically expressed in the IPC neurons or in glia. Efficiency of RNAi-mediated knockdown was determined using qRT-PCR (see Supplemental Figure S2). As with nrg RNAi, all RNAi-Gal4 combinations resulted in IPCs that failed to differentiate properly, suggesting that these three molecules are required in both cell types, consistent with their strong expression in glia and neurons (55). However, IPC knockdown of PlexA and glial knockdown of Sema-1a resulted in phenotypes much stronger than the other two conditions, indicating that Sema-1a is primarily required in glia, while PlexA is primarily required in the IPC neurons for their proper differentiation (Figures 5E–I and Table 2). Interestingly, while the ectopic branch phenotype was stronger when Sema-1a was knocked down in glial cells, IPC-specific knockdown resulted in an increase of lateral neurite complexity (Figure 5E and Table 2). Sema1-a and PlexA-RNAi mediated knockdown using Dilp2-Gal4 had no impact on IPC cell number (see Supplemental Figure S3).
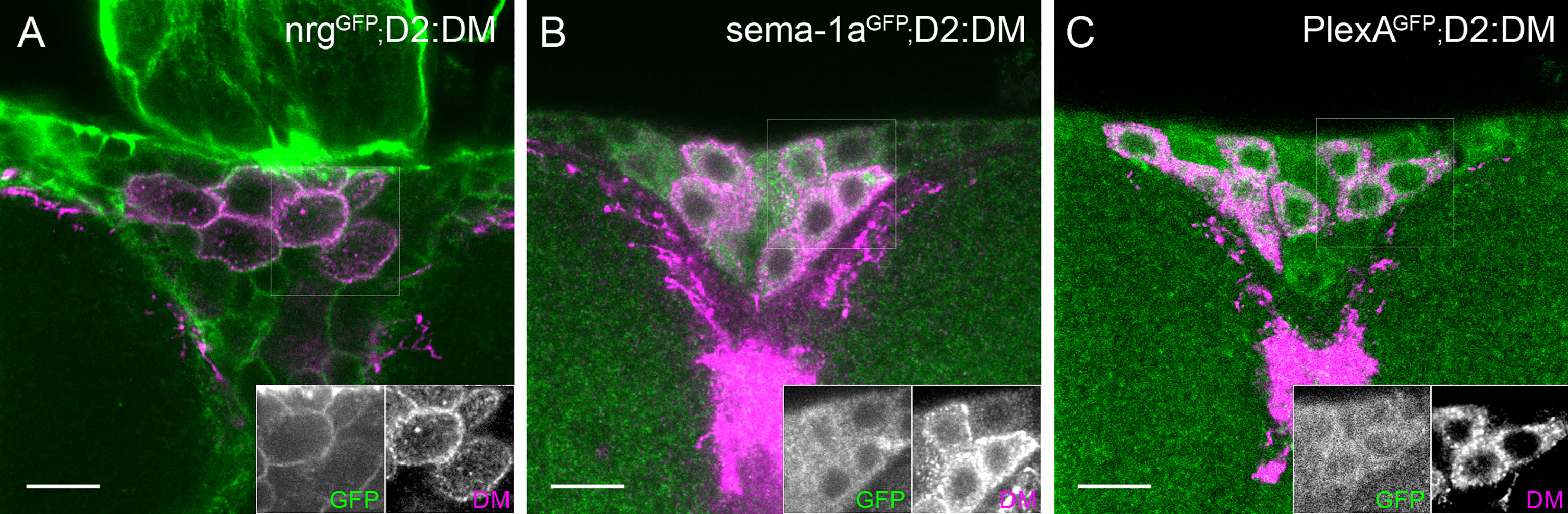
Figure 7 Expression of the signaling molecules Sema-1a and PlexA in the IPCs. Similar to Nrg (A), both Sema-1a (B) and PlexA (C) are expressed in the IPCs as well as in neighboring cells.
Nrg, Sema-1a, and PlexA Influence Stress Resistance
In order to better understand how loss of nrg in IPCs may affect stress resistance (Figures 2B–E), we analyzed how expression of dilp2, dilp3, and dilp5, the three IPC-expressed dilps, dsk, which encodes an IPC-expressed peptide involved in feeding behavior (56), as well as nrg, Sema-1a, and PlexA are affected in adult female nrg849 hypomorphs (Figure 8). In nrg females, dilp3 expression is significantly reduced to 28% of wildtype levels, and dsk expression is increased by 53% (Figures 8B, D). We also found dilp2 expression to be elevated by 72%, which is borderline statistically significant (p=0.053; Figure 8A). No significant changes in the expression of nrg, Sema-1a, or PlexA were observed (Figures 8E–G). Similarly, Dilp3 peptide levels in the soma of adult female nrg849 mutants are reduced by 43%, while levels of Dilp2 and Dilp5 peptide are not altered (Figures 8H–J).
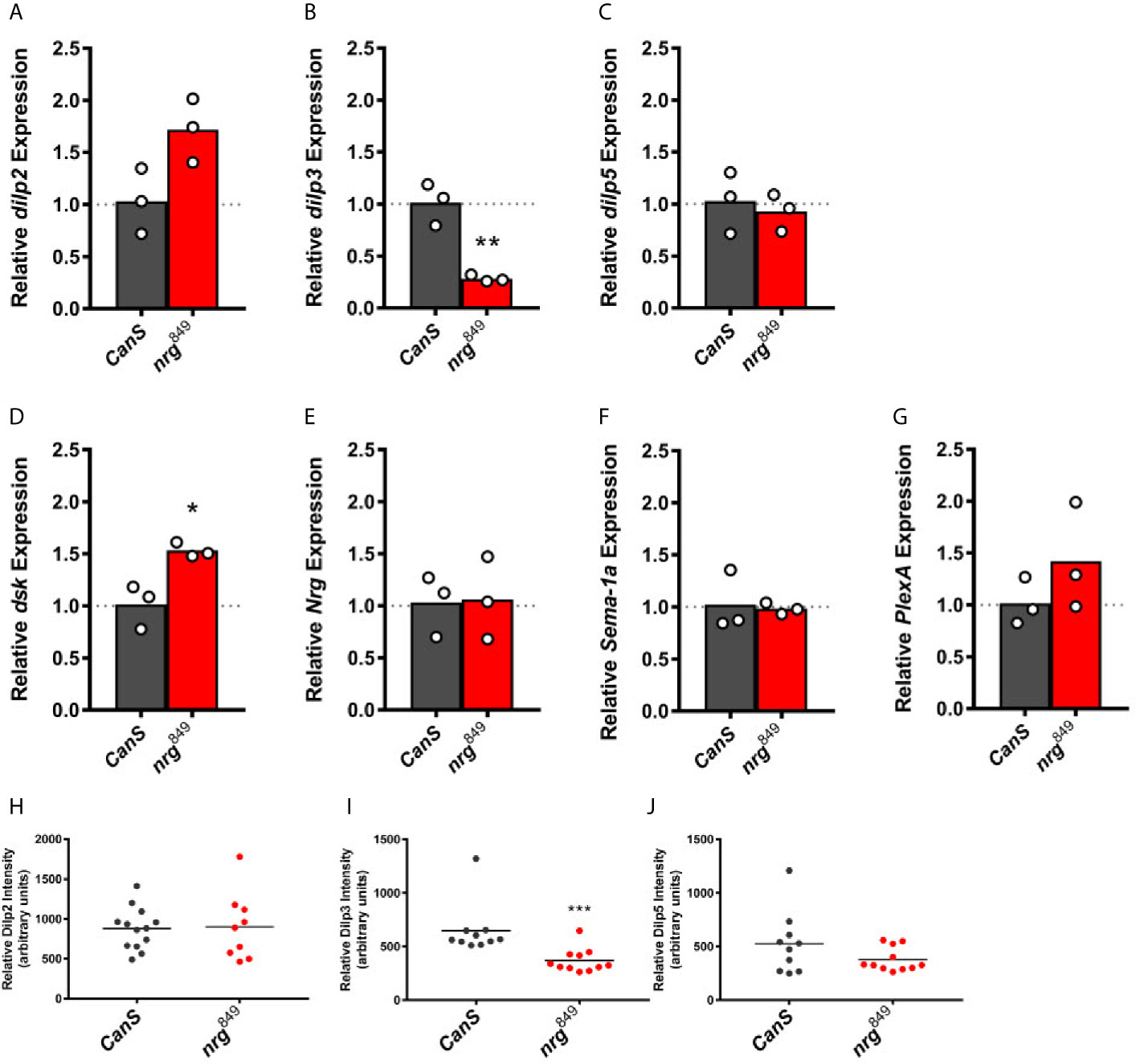
Figure 8 Expression analysis of the IPC-expressed dilps, dsk, nrg, Sema-1a, and PlexA in nrg849 adult female heads. At the transcript level, expression of dilp2 (A) is elevated 72% (unpaired t-test: P = 0.0530), dilp3 (B) is reduced to 28% of wildtype levels (**P = 0.0034), dilp5 (C) is unchanged, dsk (D) is elevated 53% (unpaired t-test: *P = 0.0159), and expression of nrg (E), Sema-1a (F), and PlexA (G) are unaffected. At the peptide level, revealed by immunohistochemistry, Dilp2 levels (H) are unchanged, Dilp3 peptide levels (I) are reduced by 43% (unpaired t-test: ***P = 0.0003), and Dilp5 levels (J) are unaffected.
We then expanded the cell-type specific RNAi knockdown experiment to downregulate nrg, Sema-1a, and PlexA in IPCs (Dilp2-Gal4) and glia (repo-Gal4) and investigated the impact on starvation resistance in adult females. Knockdown of nrg in either IPCs or glia results in starvation resistance phenotypes similar to what we observed in nrg849 animals, demonstrating that nrg is required in IPCs and glia for this phenotype associated with disrupted insulin signaling (Figures 9A, B). Knockdown of Sema-1a in the IPCs did not have an effect on starvation resistance, while Sema-1a knockdown in glial cells did significantly increase starvation resistance (Figures 9C, D). Conversely, knockdown of the PlexA receptor in the IPCs resulted in an 11% increase in starvation resistance, while knockdown of the receptor in glia had no effect on the phenotype (Figures 9E, F).
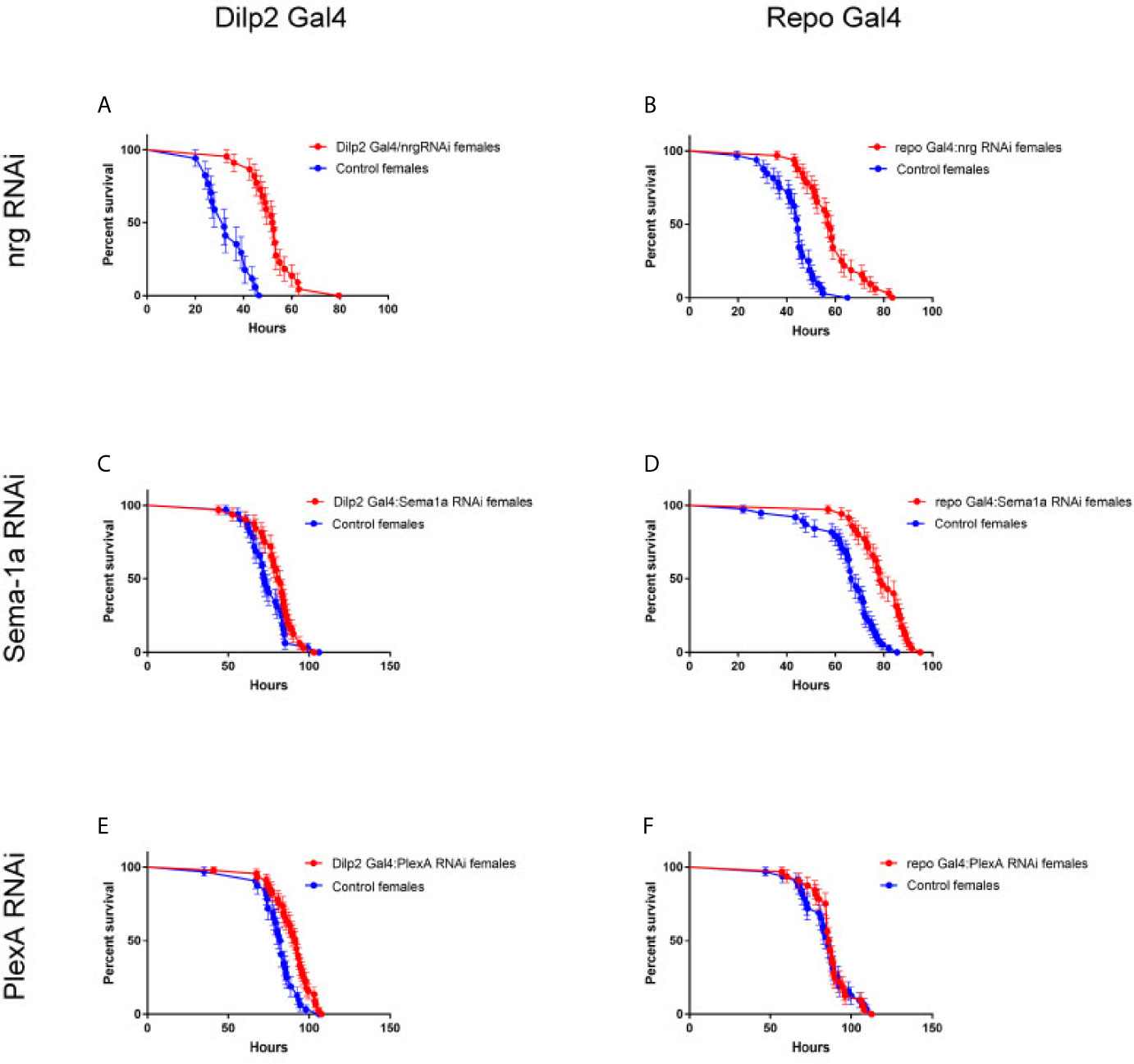
Figure 9 Cell-type specific loss of nrg, Sema-1a, and PlexA gives rise to starvation stress survival phenotypes. (A) Knockdown of nrg in IPCs with Dilp2 Gal4 results in increased starvation resistance (IPCs: P<0.0001, log-rank test; control median survival = 32 hours, RNAi median survival = 52.25 hours; (B) and in Glia: P<0.0001, log-rank test; control median survival = 44.5 hours, RNAi median survival = 57.5 hours). Knockdown of Sema-1a in IPCs (C) does not significantly affect survival (P=0.1120, log-rank test; control median survival = 72.25 hours, RNAi median survival = 81.25 hours), while knockdown of Sema-1a in glia (D) extends survival by 16% (P<0.0001, log-rank test; control median survival = 67.5 hours, RNAi median survival = 78.5 hours). The opposite is seen with PlexA, where knockdown of PlexA in IPCs (E) does extend survival by 11% (P=0.0014, log-rank test; control median survival = 82.25 hours, RNAi median survival = 91.5 hours), while knockdown of PlexA in glia (F) does not affect survival (P=0.7509, log-rank test; control median survival = 85.5 hours, RNAi median survival = 86.25 hours).
Discussion
Here we identify the requirement of three genes, nrg, Sema-1a, and PlexA in the morphological and functional differentiation of IPC neurons, and identify glia as a source of morphoregulators controlling neuritogenesis of the IPCs. When transcript levels of either nrg or Sema-1a were reduced in glia, ectopic neurite branches formed on the IPC neurons. This phenotype was reproduced when PlexA, which encodes the Sema-1a receptor, was knocked down in the IPCs. We also describe a role of Nrg in synaptic organization of the IPCs.
The cell adhesion molecule Neuroglian has been shown to be a key factor in nervous system development, where it is expressed both in neurons and glia. nrg is required for IPC development and differentiation, as observed by the severe defasciculation and misbranching phenotypes caused by loss of Nrg function. Furthermore, we show that Nrg is predominantly required in glia for proper IPC neurite formation. When nrg was knocked down in glia, we observed the formation of ectopic branches as well as a loss of neurite complexity in the IPC complex, whereas IPC-specific knockdown of nrg only produced single pioneer neurites. This suggests that while cell-autonomous Nrg may be necessary for the initial stages of ectopic branch formation, glia-encoded Nrg has a repulsive function in preventing additional neurites from fasciculating onto the pioneer neurites. It is also noteworthy that the developmental stage in which we first observe ectopic branches in nrg mutants (75% pupa), corresponds with the CNS-wide assembly of adult neural circuits, a process that is dependent on glia (57). Glia expression of Nrg-167 was able to rescue the ectopic branching phenotype, demonstrating that the glia-encoded Nrg is sufficient to control neurite outgrowth. This is reminiscent of embryonic CNS development, where glia are not absolutely required for pioneer neurite formation, but are necessary for the ensuing fasciculation of follower neurites onto the pioneer (58). Interestingly, ectopic branches have also been observed in the dendritic arborization (da) neurons of nrg2 mutants (35). While expression of the Nrg-167 isoform in da neurons alone failed to rescue the ectopic branching phenotype, expression of Nrg-167 in both da neurons and glia was able to completely rescue the ectopic branching phenotype (35). Although a rescue of the ectopic da branching was not demonstrated by driving Nrg in glia alone, this data supports the model that glia-encoded Nrg acts as a suppressor of neurite sprouting.
In vertebrates, L1-CAM, in a receptor complex with Plexin-A1 and Neuropilin-1, interacts with Semaphorin3A to influence neurite outgrowth and growth cone stability in neuronal cell culture experiments (59–62). In Drosophila, Sema-1a has been shown to interact genetically with Nrg (22, 23). In addition to identifying a role for Nrg in IPC differentiation, we demonstrated that Sema-1a and its receptor, PlexA, also are required in IPC differentiation. To our knowledge, this is the first time Sema-1a has been shown to be required in glia to control neurite outgrowth. In vertebrate models, both Sema3A and Sema6D have been shown to be required in glia in the regulation of axonal outgrowth in motor neurons and retinal axons, respectively, presumably via PlexA expressed in the developing neuron (63, 64). Interestingly, in the retinal axon model, glial expression of the L1-related Nr-CAM was also required to mediate the effects of Semaphorin (63) Our data suggest that this combination of glia-expressed L1- (or L1-related) CAM and Semaphorin and neuronal PlexA in regulation of neurite outgrowth is evolutionarily conserved.
Despite the major morphological defects observed in the IPCs of nrg mutants, at least one essential function of these neurons in insulin signaling appears to be unaffected, as we did not observe any growth defects in nrg mutant animals. This observation, as well as the lack of more overt morphological phenotypes upon IPC-specific nrg knockdown, could to an extent be due to compensatory effects by other IPC-expressed CAMs, such as Fasciclin 2, which is also expressed in the IPCs (unpublished data and 8) and previously shown to be functionally interchangeable in some axons (65). However, we cannot rule out the possibility that loss of IPC-specific nrg results in subtle defects affecting signaling, connectivity, or ultrastructural morphology. Our observations that loss of nrg in the mutant as well as in cell-type specific knockdown in both IPCs and glia results in stress resistance phenotypes suggests the existence of one or more of these underlying defects. Loss of nrg leads to a strong reduction of Dilp3 (observed at both the mRNA and peptide levels) and an increase in dsk expression. While both gene products have clear roles in metabolism, it seems unlikely that they can explain the observed nrg phenotypes. Loss of dilp3 alone has been shown not to have any significant effect on stress resistance, and knockdown of dsk has been shown to increase stress resistance (56, 66). It is likely that both gene products, together with Nrg, Sema-1a, PlexA, and other factors all contribute to the phenotype. That loss of Sema-1a in glia and PlexA in the IPCs also gives rise to altered stress resistance demonstrates that these molecules are also involved in the regulation of IPC function. These phenotypes, taken together with the glia-regulated neurite outgrowth phenotypes, demonstrate a requirement for Nrg in both the IPCs and glia for the proper development and function of these neurons. Furthermore, a comparison of the stress resistance with the neurite outgrowth phenotypes from the cell-type specific RNAi knockdown experiments shows a prominent need for Nrg in both cell types, while the Sema-1a ligand is required in glia, and PlexA, the Sema-1a receptor, in the IPCs. These data suggest that Nrg and Sema-1a in glia work jointly to coordinate IPC neurite outgrowth and function, and this together with IPC-expressed PlexA.
We observed increased numbers of large DenMark-positive densities in the SOG neurites of nrg849 mutants, as well as aberrant localization of DenMark upon overexpression of Nrg-180. Since DenMark is enriched at postsynaptic sites (40), and Nrg has been implicated in synapse organization (22, 54, 67), the observed alterations in DenMark-positive structures suggest that Nrg has an active role in the distribution of synaptic components and/or the establishment or maintenance of synapses in the IPC neurons, similar to what has been shown in other neuronal types (54). The observed redistribution of DenMark in our overexpression experiments (see Figures 6B, D), on the one hand, is likely an ankyrin-independent gain-of-function phenotype, similar to what has been observed in wing neurons (37). This hypothesis is supported by the observation that expression of the GPI-anchored isoform of Nrg, which lacks the intracellular ankyrin-binding domain, gives a very similar phenotype in the IPCs (data not shown). On the other hand, the increased number of large DenMark-positive densities in the neurites of nrg849 mutants (see Figures 1I–K), is a loss-of-function phenotype and suggests that the organization of the cytoskeleton is altered at these synapses. Aggregated Nrg interacts with Ankyrins, leading to the assembly of the spectrin cytoskeleton (68–70). Intriguingly, when components of the spectrin scaffold are removed postsynaptically, significantly larger active zones are also seen at the larval neuromuscular junction (71). Given the similarity between our observations in the SOG, the data of (71), and the well-established link between Nrg, Ankyrin and the spectrin cytoskeleton, we hypothesize that the increased number of large DenMark-positive densities in the SOG is a result of disrupted spectrin-mediated synapse development. Alternatively, the SOG phenotype may be the result of an Ankyrin-independent mechanism, as was proposed for the observed synaptic defects in the giant fiber of nrg849 mutants (54). Our data with DenMark demonstrate that the localization of synaptic proteins is also likely to be altered in nrg mutants.
It has been postulated that the neurosecretory cells in the pars intercerebralis and the ring gland in insects and the endocrine system of vertebrates are derived from a common ancestral system, as they both are derived from anterior neuroectoderm and oral ectoderm and are specified by the same transcription factors (4). Our data builds upon this idea and suggests that L1/Semaphorin/Plexin A-signaling may also have a role in the development as well as the function of vertebrate endocrine systems. In vertebrates, weak expression of L1-CAM is found in adult islets of Langerhans, where the endocrine cells of the pancreas are found, and a single nucleotide polymorphism near the CHL1 locus, another L1 homolog, has been associated with insulin resistance (72, 73). L1-CAM is broadly expressed in neurons of the CNS, and has enriched expression in the hypothalamus, including the supraoptic tract nucleus and in the paraventricular nucleus (PVN), both of which project to the neurohypophysis, and the thalamus, with particularly high expression in the midline thalamic nuclei, which includes the paraventricular thoracic nucleus (tPVN) (74). Semaphorins and Plexins are also found in the hypothalamic-pituitary axis (75). Furthermore, while expression of L1-CAM in the CNS was thought to only be found in neurons, expression in pituicytes, a specialized set of glial cells in the hypophysis, is detected from the moment that the neurohypophysis is innervated (72, 76). These pituicytes interact with the neurosecretory axons of the neurohypophysis and may even play an active role in controlling neurosecretion (77). The PVN has been shown to control the ingestion of carbohydrates, and this role in modulating feeding behavior is mediated by Neuropeptide Y (78, 79). The tPVN also plays a role in feeding behavior, where it can anticipate feeding (80). Interestingly, dilp expression is stimulated by sNPF, an ortholog of NPY, in the IPCs, which express the sNPF receptor and seem to contact sNPF-expressing neurons (81, 82). In line with the PVN and tPVN, the IPCs have also been shown to influence feeding behavior (83). These data suggest that L1-type cell adhesion molecules may be required in these processes. In summary, we have shown that Nrg, Sema-1a and PlexA are required in IPCs and glia to control morphological and functional differentiation of IPCs.
Data Availability Statement
The original contributions presented in the study are included in the article/Supplementary Material. Further inquiries can be directed to the corresponding author.
Author Contributions
JC and PC conceived research project and designed experiments. JC, KB, MW, and VV performed experiments. JC, MW, and PC interpreted data and wrote manuscript. JC, KB, MW, VV and PC revised the manuscript. All authors contributed to the article and approved the submitted version.
Funding
This work was supported by FWO grants G065408.N10 and G078914N and by KULeuven grant C14/17/099.
Conflict of Interest
The authors declare that the research was conducted in the absence of any commercial or financial relationships that could be construed as a potential conflict of interest.
Acknowledgments
We thank Bassem Hassan for the DenMark cDNA, Jan Veenstra for the Dilp2 antibody, members of the Drosophila community for sharing fly stocks, and Lies Vanden Broeck and Liesbeth Zwarts for help with data analysis and all members of the laboratory for critical reading of the manuscript.
Supplementary Material
The Supplementary Material for this article can be found online at: https://www.frontiersin.org/articles/10.3389/fendo.2021.600251/full#supplementary-material
Abbreviations
IPC, insulin-producing cell; Nrg, neuroglian; Sema-1a, semaphorin-1a; PlexA, plexin A; Dilp, Drosophila insulin-like peptide.
References
1. Rulifson EJ, Kim SK, Nusse R. Ablation of Insulin-Producing Neurons in Flies: Growth and Diabetic Phenotypes. Science (2002) 296:1118–20. doi: 10.1126/science.1070058
2. Nässel DR, Kubrak OI, Liu Y, Luo J, Lushchak OV. Factors That Regulate Insulin Producing Cells and Their Output in Drosophila. Front Physiol (2013) 4:252. doi: 10.3389/fphys.2013.00252
3. Nässel DR, Liu Y, Luo J. Insulin/IGF Signaling and Its Regulation in Drosophila. Gen Comp Endocrinol (2015) 221:255–66. doi: 10.1016/j.ygcen.2014.11.021
4. Wang S, Tulina N, Carlin DL, Rulifson EJ. The Origin of Islet-Like Cells in Drosophila Identifies Parallels to the Vertebrate Endocrine Axis. Proc Natl Acad Sci USA (2007) 104:19873–78. doi: 10.1073/pnas.0707465104
5. Hwang HJ, Rulifson E. Serial Specification of Diverse Neuroblast Identities From a Neurogenic Placode by Notch and Egfr Signaling. Development (2011) 138:2883–93. doi: 10.1242/dev.055681
6. Clements J, Hens K, Francis C, Schellens A, Callaerts P. Conserved Role for the Drosophila Pax6 Homolog Eyeless in Differentiation and Function of Insulin-Producing Neurons. Proc Natl Acad Sci USA (2008) 105:16183–8. doi: 10.1073/pnas.0708330105
7. Okamoto N, Nishimori Y, Nishimura T. Conserved Role for the Dachshund Protein With Drosophila Pax6 Homolog Eyeless in Insulin Expression. Proc Natl Acad Sci USA (2012) 109:2406–11. doi: 10.1073/pnas.1116050109
8. Cao J, Ni J, Ma W, Shiu V, Milla LA, Park S, et al. Insight Into Insulin Secretion From Transcriptome and Genetic Analysis of Insulin-Producing Cells of Drosophila. Genetics (2014) 197:175–92. doi: 10.1534/genetics.113.160663
9. Seiradake E, Jones EY, Klein R. Structural Perspectives on Axon Guidance. Annu Rev Cell Dev Biol (2016) 32:577–608. doi: 10.1146/annurev-cellbio-111315-125008
10. Sytnyk V, Leshchyns’ka I, Schachner L. Neural Cell Adhesion Molecules of the Immunoglobulin Superfamily Regulate Synapse Formation, Maintenance, and Function. Trends Neurosci (2017) 40:295–308. doi: 10.1016/j.tins.2017.03.003
11. Bellon A, Mann F. Keeping Up With Advances in Axon Guidance. Curr Opin Neurobiol (2018) 53:183–91. doi: 10.1016/j.conb.2018.09.004
12. Learte AR, Hidalgo A. The Role of Glial Cells in Axon Guidance, Fasciculation and Targeting. Adv Exp Med Biol (2007) 621:156–66. doi: 10.1007/978-0-387-76715-4_12
13. Rigby MJ, Gomez TM, Puglielli L. Glial Cell-Axonal Growth Cone Interactions in Neurodevelopment and Regeneration. Front Neurosci (2020) 14:203. doi: 10.3389/fnins.2020.00203
14. Hortsch M. Structural and Functional Evolution of the L1 Family: Are Four Adhesion Molecules Better Than One? Mol Cell Neurosci (2000) 15:1–10. doi: 10.1006/mcne.1999.0809
15. Fransen E, Lemmon V, Van Camp G, Vits L, Coucke P, Willems PJ. CRASH Syndrome: Clinical Spectrum of Corpus Callosum Hypoplasia, Retardation, Adducted Thumbs, Spastic Paraparesis and Hydrocephalus Due to Mutations in One Single Gene, L1. Eur J Hum Genet (1995) 3:273–84. doi: 10.1159/000472311
16. Dahme M, Bartsch U, Martini R, Anliker B, Schachner M, Mantei N. Disruption of the Mouse L1 Gene Leads to Malformations of the Nervous System. Nat Genet (1997) 17:346–9. doi: 10.1038/ng1197-346
17. Demyanenko GP, Tsai AY, Maness PF. Abnormalities in Neuronal Process Extension, Hippocampal Development, and the Ventricular System of L1 Knockout Mice. J Neurosci (1999) 19:4907–20. doi: 10.1523/JNEUROSCI.19-12-04907.1999
18. Bieber AJ, Snow PM, Hortsch M, Patel NH, Jacobs JR, Traquina ZR, et al. Drosophila Neuroglian: A Member of the Immunoglobulin Superfamily With Extensive Homology to the Vertebrate Neural Adhesion Molecule L1. Cell (1989) 59:447–60. doi: 10.1016/0092-8674(89)90029-9
19. Hortsch M, Bieber AJ, Patel NH, Goodman CS. Differential Splicing Generates a Nervous System-Specific Form of Drosophila Neuroglian. Neuron (1990) 4:697–709. doi: 10.1016/0896-6273(90)90196-M
20. Koushika SP, Lisbon MJ, White K. ELAV, a Drosophila Neuron-Specific Protein, Mediates the Generation of an Alternatively Spliced Neural Protein Isoform. Curr Biol (1996) 6:1634–41. doi: 10.1016/S0960-9822(02)70787-2
21. Lisbin MJ, Qiu J, White K. The Neuron-Specific RNA-Binding Protein ELAV Regulates Neuroglian Alternative Splicing in Neurons and Binds Directly to its pre-mRNA. Genes Dev (2001) 15:2546–61. doi: 10.1101/gad.903101
22. Godenschwege TA, Murphey RK. Genetic Interaction of Neuroglian and Semaphorin1a During Guidance and Synapse Formation. J Neurogenet (2009) 23:147–55. doi: 10.1080/01677060802441380
23. Goossens T, Kang YY, Wuytens G, Zimmermann P, Callaerts-Végh Z, Pollarolo G, et al. The Drosophila L1CAM Homolog Neuroglian Signals Through Distinct Pathways to Control Different Aspects of Mushroom Body Axon Development. Development (2011) 138:1595–605. doi: 10.1242/dev.052787
24. Winberg ML, Noordermeer JN, Tamagnone L, Comoglio PM, Spriggs MK, Tessier-Lavigne M, et al. Plexin A Is a Neuronal Semaphorin Receptor That Controls Axon Guidance. Cell (1998) 95:903–16. doi: 10.1016/S0092-8674(00)81715-8
25. Yu HH, Araj HH, Ralls SA, Kolodkin AL. The Transmembrane Semaphorin Sema I is Required in Drosophila for Embryonic Motor and CNS Axon Guidance. Neuron (1998) 20:207–20. doi: 10.1016/S0896-6273(00)80450-X
26. Godenschwege TA, Hu H, Shan-Crofts X, Goodman CS, Murphey RK. Bi-Directional Signaling by Semaphorin 1a During Central Synapse Formation in. Drosophila Nat Neurosci (2002) 5:1294–301. doi: 10.1038/nn976
27. Kruger RP, Aurandt J, Guan KL. Semaphorins Command Cells to Move. Nat Rev Mol Cell Biol (2005) 6:789–800. doi: 10.1038/nrm1740
28. Cafferty P, Yu L, Long H, Rao Y. Semaphorin-1a Functions as a Guidance Receptor in the Drosophila Visual System. J Neurosci (2006) 26:3999–4003. doi: 10.1523/JNEUROSCI.3845-05.2006
29. Lattemann M, Zierau A, Schulte C, Seidl S, Kuhlmann B. And Hummel, T. Semaphorin-1a Controls Receptor Neuron-Specific Axonal Convergence in the Primary Olfactory Center of Drosophila. Neuron (2007) 53:169–84. doi: 10.1016/j.neuron.2006.12.024
30. Sweeney LB, Couto A, Chou Y-H, Berdnik D, Dickson BJ, Luo L, et al. Temporal Target Restriction of Olfactory Receptor Neurons by Semaphorin-1a/PlexinA-Mediated Axon-Axon Interactions. Neuron (2007) 53:185–200. doi: 10.1016/j.neuron.2006.12.022
31. Yu L, Zhou Y, Cheng S, Rao Y. Plexin A-Semaphorin-1a Reverse Signaling Regulates Photoreceptor Axon Guidance in Drosophila. J Neurosci (2010) 30:12151–6. doi: 10.1523/JNEUROSCI.1494-10.2010
32. Jongbloets BC, Pasterkamp RJ. Semaphorin Signalling During Development. Development (2014) 141:3292–7. doi: 10.1242/dev.105544
33. Strauss R, Heisenberg M. A Higher Control Center of Locomotor Behavior in the Drosophila Brain. J Neurosci (1993) 13:1852–61. doi: 10.1523/JNEUROSCI.13-05-01852.1993
34. Morin X, Daneman R, Zavortink M, Chia W. A Protein Trap Strategy to Detect GFP-Tagged Proteins Expressed From Their Endogenous Loci in Drosophila. Proc Natl Acad Sci USA (2001) 98:15050–5. doi: 10.1073/pnas.261408198
35. Yamamoto M, Ueda R, Takahashi K, Saigo K, Uemura T. Control of Axonal Sprouting and Dendrite Branching by the Nrg-Ank Complex at the Neuron-Glia Interface. Curr Biol (2006) 16:1678–83. doi: 10.1016/j.cub.2006.06.061
36. Hall SG, Bieber AJ. Mutations in the Drosophila Neuroglian Cell Adhesion Molecule Affect Motor Neuron Pathfinding and Peripheral Nervous System Patterning. J Neurobiol (1997) 32:325–40. doi: 10.1002/(SICI)1097-4695(199703)32:3<325::AID-NEU6>3.0.CO;2-9
37. Islam R, Kristiansen LV, Romani S, Garcia-Alonso L, Hortsch M. Activation of EGF Receptor Kinase by L1-Mediated Homophilic Cell Interactions. Mol Biol Cell (2004) 15:2003–12. doi: 10.1091/mbc.e03-05-0333
38. Pfeiffer BD, Jenett A, Hammonds AS, Ngo TT, Misra S, Murphy C, et al. Tools for Neuroanatomy and Neurogenetics in Drosophila. Proc Natl Acad Sci USA (2008) 105:9715–20. doi: 10.1073/pnas.0803697105
39. Lee BP, Jones BW. Transcriptional Regulation of the Drosophila Glial Gene Repo. Mech Dev (2005) 122:849–62. doi: 10.1016/j.mod.2005.01.002
40. Nicolaï LJ, Ramaekers A, Raemaekers T, Drozdzecki A, Mauss AS, Yan J, et al. Genetically Encoded Dendritic Marker Sheds Light on Neuronal Connectivity in Drosophila. Proc Natl Acad Sci USA (2010) 107:20553–8. doi: 10.1073/pnas.1010198107
41. Kelso RJ, Buszczak M, Quiñones AT, Castiblanco C, Mazzalupo S, Cooley L. Flytrap, a Database Documenting a GFP Protein-Trap Insertion Screen in Drosophila Melanogaster. Nucleic Acids Res (2004) 32:D418–20. doi: 10.1093/nar/gkh014
42. Buszczak M, Paterno S, Lighthouse D, Bachman J, Planck J, Owen S, et al. The Carnegie Protein Trap Library: A Versatile Tool for Drosophila Developmental Studies. Genetics (2007) 175:1505–31. doi: 10.1534/genetics.106.065961
43. Yoo SK, Pascoe HG, Pereira T, Kondo S, Jacinto A, Zhang X, et al. Plexins Function in Epithelial Repair in Both Drosophila and Zebrafish. Nat Commun (2016) 7:12282. doi: 10.1038/ncomms12282
44. Sharma Y, Cheung U, Larsen EW, Eberl DF. pPTGAL, a Convenient Gal4 P-Element Vector for Testing Expression of Enhancer Fragments in Drosophila. Genesis (2002) 34:115–8. doi: 10.1002/gene.10127
45. Brand AH, Perrimon N. Targeted Gene Expression as a Means of Altering Cell Fates and Generating Dominant Phenotypes. Development (1993) 118:401–15. doi: 10.1242/dev.118.2.401
46. Buhler K, Clements J, Winant M, Bolckmans L, Vulsteke V, Callaerts P. Growth Control Through Regulation of Insulin Signaling by Nutrition-Activated Steroid Hormone in Drosophila. Development (2018) 145(21):dev165654. doi: 10.1242/dev.165654
48. Schmittgen TD, Livak KJ. Analyzing Real-Time PCR Data by the Comparative C(T) Method. Nat Protoc (2008) 3:1101–8. doi: 10.1038/nprot.2008.73
49. Brogiolo W, Stocker H, Ikeya T, Rintelen F, Fernandez R, Hafen E. An Evolutionarily Conserved Function of the Drosophila Insulin Receptor and Insulin-Like Peptides in Growth Control. Curr Biol (2001) 11:213–21. doi: 10.1016/S0960-9822(01)00068-9
50. Ikeya T, Galic M, Belawat P, Nairz K, Hafen E. Nutrient-Dependent Expression of Insulin-Like Peptides From Neuroendocrine Cells in the CNS Contributes to Growth Regulation in Drosophila. Curr Biol (2002) 12:1293–300. doi: 10.1016/S0960-9822(02)01043-6
51. Lovick JK, Ngo KT, Omoto JJ, Wong DC, Nguyen JD, Hartenstein V. Postembryonic Lineages of the Drosophila Brain: I. Development of the Lineage-Associated Fiber Tracts. Dev Biol (2013) 384:228–57. doi: 10.1016/j.ydbio.2013.07.008
52. Ito K, Shinomiya K, Ito M, Armstrong JD, Boyan G, Hartenstein V, et al. A Systematic Nomenclature for the Insect Brain. Neuron (2014) 81:755–65. doi: 10.1016/j.neuron.2013.12.017
53. Broughton SJ, Piper MDW, Ikeya T, Bass TM, Jacobson J, Driege Y, et al. Longer Lifespan, Altered Metabolism, and Stress Resistance in Drosophila From Ablation of Cells Making Insulin-Like Ligands. Proc Natl Acad Sci USA (2005) 102:3105–10. doi: 10.1073/pnas.0405775102
54. Godenschwege TA, Kristiansen LV, Uthaman SB, Hortsch M, Murphey RK. A Conserved Role for Drosophila Neuroglian and Human L1-CAM in Central-Synapse Formation. Curr Biol (2006) 16:12–23. doi: 10.1016/j.cub.2005.11.062
55. DeSalvo MK, Hindle SJ, Rusan ZM, Orng S, Eddison M, Halliwill K, et al. The Drosophila Surface Glia Transcriptome: Evolutionary Conserved Blood-Brain Barrier Processes. Front Neurosci (2014) 8:346. doi: 10.3389/fnins.2014.00346
56. Söderberg JA, Carlsson MA, Nässel DR. Insulin-Producing Cells in the Drosophila Brain Also Express Satiety-Inducing Cholecystokinin-Like Peptide, Drosulfakinin. Front Endocrinol (2012) 3:109. doi: 10.3389/fendo.2012.00109
57. Muthukumar AK, Stork T, Freeman MR. Activity-Dependent Regulation of Astrocyte GAT Levels During Synaptogenesis. Nat Neurosci (2014) 17:1340–50. doi: 10.1038/nn.3791
58. Hidalgo A, Booth GE. Glia Dictate Pioneer Axon Trajectories in the Drosophila Embryonic CNS. Development (2000) 127:393–402. doi: 10.1242/dev.127.2.393
59. Castellani V, Chédotal A, Schachner M, Faivre-Sarrailh C, Rougon G. Analysis of the L1-Deficient Mouse Phenotype Reveals Cross-Talk Between Sema3A and L1 Signaling Pathways in Axonal Guidance. Neuron (2000) 27:237–49. doi: 10.1016/S0896-6273(00)00033-7
60. Castellani V, De Angelis E, Kenwrick S, Rougon G. Cis and Trans Interactions of L1 With Neuropilin-1 Control Axonal Responses to Semaphorin 3A. EMBO J (2002) 21:6348–57. doi: 10.1093/emboj/cdf645
61. Castellani V, Falk J, Rougon G. Semaphorin3A-Induced Receptor Endocytosis During Axon Guidance Responses Is Mediated by L1 CAM. Mol Cell Neurosci (2004) 26:89–100. doi: 10.1016/j.mcn.2004.01.010
62. Bechara A, Nawabi H, Moret F, Yaron A, Weaver E, Bozon M, et al. FAK-MAPK-Dependent Adhesion Disassembly Downstream of L1 Contributes to Semaphorin3A-Induced Collapse. EMBO J (2008) 27:1549–62. doi: 10.1038/emboj.2008.86
63. Kuwajima T, Yoshida Y, Takegahara N, Petros TJ, Kumanogoh A, Jessell TM, et al. Optic Chiasm Presentation of Semaphorin6D in the Context of Plexin-A1 and Nr CAM Promotes Retinal Axon Midline Crossing. Neuron (2012) 74:676–90. doi: 10.1016/j.neuron.2012.03.025
64. Molofsky AV, Kelley KW, Tsai H-H, Redmond SA, Chang SM, Madireddy L, et al. Astrocyte-Encoded Positional Cues Maintain Sensorimotor Circuit Integrity. Nature (2014) 509:189–94. doi: 10.1038/nature13161
65. Kristiansen LV, Velasquez E, Romani S, Baars S, Berezin V, Bock E, et al. Genetic Analysis of an Overlapping Functional Requirement for L1- and NCAM-Type Proteins During Sensory Axon Guidance in Drosophila. Mol Cell Neurosci (2005) 28:141–52. doi: 10.1016/j.mcn.2004.09.003
66. Grönke S, Clarke DF, Broughton S, Andrews TD, Partridge L. Molecular Evolution and Functional Characterization of Drosophila Insulin-Like Peptides. PloS Genet (2010) 6:e1000857. doi: 10.1371/journal.pgen.1000857
67. Enneking E-M, Kudumala SR, Moreno E, Stephan R, Boerner J, Godenschwege TA, et al. Transynaptic Coordination of Synaptic Growth, Function, and Stability by the L1-Type CAM Neuroglian. PloS Biol (2013) 11:e1001537. doi: 10.1371/journal.pbio.1001537
68. Dubreuil RR, MacVicar G, Dissanayake S, Liu C, Homer D, Hortsch M. Neuroglian-Mediated Cell Adhesion Induces Assembly of the Membrane Skeleton at Cell Contact Sites. J Cell Biol (1996) 133:647–55. doi: 10.1083/jcb.133.3.647
69. Jefford G, Dubreuil RR. Receptor Clustering Drives Polarized Assembly of Ankyrin. J Biol Chem (2000) 275:27726–32. doi: 10.1074/jbc.M004959200
70. Hortsch M, Nagaraj K, Godenschwege TA. The Interaction Between L1-Type Proteins and Ankyrins - a Master Switch for L1-Type CAM Function. Cell Mol Biol Lett (2009) 14:57–69. doi: 10.2478/s11658-008-0035-4
71. Pielage J, Fetter RD, Davis GW. A Postsynaptic Spectrin Scaffold Defines Active Zone Size, Spacing, and Efficacy at the Drosophila Neuromuscular Junction. J Cell Biol (2006) 175:491–503. doi: 10.1083/jcb.200607036
72. Grant NJ, Leon C, Aunis D, Langley K. Cellular Localization of the Neural Cell Adhesion Molecule L1 in Adult Rat Neuroendocrine and Endocrine Tissues: Comparisons With NCAM. J Comp Neurol (1992) 325:548–58. doi: 10.1002/cne.903250407
73. Manning AK, Hivert MF, Scott RA, Grimsby JL, Bouatia-Naji N, Chen H, et al. A Genome-Wide Approach Accounting for Body Mass Index Identifies Genetic Variants Influencing Fasting Glycemic Traits and Insulin Resistance. Nat Genet (2012) 44:659–69. doi: 10.1038/ng.2274
74. Horinouchi K, Nakamura Y, Yamanaka H, Watabe T, Shiosaka S. Distribution of L1cam mRNA in the Adult Mouse Brain: In Situ Hybridization and Northern Blot Analyses. J Comp Neurol (2005) 482:386–404. doi: 10.1002/cne.20398
75. Giacobini P, Prevot V. Semaphorins in the Development, Homeostasis and Disease of Hormone Systems. Semin Cell Dev Biol (2013) 24:190–8. doi: 10.1016/j.semcdb.2012.11.005
76. Berardi M, Hindelang C, Félix JM, Stoeckel ME. L1 and Laminin: Their Expression During Rat Hypophysis Ontogenesis and in Adult Neurohemal Areas. Int J Dev Neurosci (1999) 17:121–30. doi: 10.1016/S0736-5748(98)00067-7
77. Hatton GI, Perlmutter LS, Salm AK, Tweedle CD. Dynamic Neuronal-Glial Interactions in Hypothalamus and Pituitary: Implications for Control of Hormone Synthesis and Release. Peptides (1984) 5:121–38. doi: 10.1016/0196-9781(84)90271-7
78. Stanley BG, Leibowitz SF. Neuropeptide Y: Stimulation of Feeding and Drinking by Injection Into the Paraventricular Nucleus. Life Sci (1984) 35:2635–42. doi: 10.1016/0024-3205(84)90032-8
79. Leibowitz SF, Weiss GF, Yee F, Tretter JB. Noradrenergic Innervations of the Paraventricular Nucleus: Specific Role in Control of Carbohydrate Ingestion. Brain Res Bull (1985) 14:561–7. doi: 10.1016/0361-9230(85)90105-4
80. Nakahara K, Fukui K, Murakami N. Involvement of Thalamic Paraventricular Nucleus in the Anticipatory Reaction Under Food Restriction in Rat. J Vet Med Sci (2004) 66:1297–300. doi: 10.1292/jvms.66.1297
81. Lee K-S, Kwon O-Y, Lee JH, Kwon K, Min K-J, Jung S-A, et al. Drosophila Short Neuropeptide F Signalling Regulates Growth by ERK-Mediated Insulin Signalling. Nat Cell Biol (2008) 10:468–75. doi: 10.1038/ncb1710
82. Nässel DR, Enell LE, Santos JG, Wegener C, Johard HA. A Large Population of Diverse Neurons in the Drosophila Central Nervous System Expresses Short Neuropeptide F, Suggesting Multiple Distributed Peptide Functions. BMC Neurosci (2008) 9:90. doi: 10.1186/1471-2202-9-90
Keywords: Drosophila, insulin-producing cells, glia, morphological and functional differentiation, neuron, cell adhesion molecules, axon guidance molecules, stress resistance
Citation: Clements J, Buhler K, Winant M, Vulsteke V and Callaerts P (2021) Glial and Neuronal Neuroglian, Semaphorin-1a and Plexin A Regulate Morphological and Functional Differentiation of Drosophila Insulin-Producing Cells. Front. Endocrinol. 12:600251. doi: 10.3389/fendo.2021.600251
Received: 09 September 2020; Accepted: 11 June 2021;
Published: 01 July 2021.
Edited by:
Sebastien G. Bouret, U1172 Centre de Recherche Jean Pierre Aubert (INSERM), FranceReviewed by:
Xi Gu, Southern Medical University, ChinaThomas Worzfeld, University of Marburg, Germany
Copyright © 2021 Clements, Buhler, Winant, Vulsteke and Callaerts. This is an open-access article distributed under the terms of the Creative Commons Attribution License (CC BY). The use, distribution or reproduction in other forums is permitted, provided the original author(s) and the copyright owner(s) are credited and that the original publication in this journal is cited, in accordance with accepted academic practice. No use, distribution or reproduction is permitted which does not comply with these terms.
*Correspondence: Patrick Callaerts, cGF0cmljay5jYWxsYWVydHNAa3VsZXV2ZW4uYmU=