- 1Normandy University, FR CNRS 3730 SCALE, UMR-I 02 INERIS-URCA-ULH Environmental Stress and Aquatic Biomonitoring (SEBIO), Université Le Havre Normandie, Le Havre, France
- 2Department of Biological Sciences, North Carolina State University, Raleigh, NC, United States
- 3School of Biological Sciences, Institute of Marine Sciences, University of Portsmouth, Portsmouth, United Kingdom
Crustaceans—and arthropods in general—exhibit many unique aspects to their physiology. These include the requirement to moult (ecdysis) in order to grow and reproduce, the ability to change color, and multiple strategies for sexual differentiation. Accordingly, the endocrine regulation of these processes involves hormones, receptors, and enzymes that differ from those utilized by vertebrates and other non-arthropod invertebrates. As a result, environmental chemicals known to disrupt endocrine processes in vertebrates are often not endocrine disruptors in crustaceans; while, chemicals that disrupt endocrine processes in crustaceans are often not endocrine disruptors in vertebrates. In this review, we present an overview of the evolution of the endocrine system of crustaceans, highlight endocrine endpoints known to be a target of disruption by chemicals, and identify other components of endocrine signaling that may prove to be targets of disruption. This review highlights that crustaceans need to be evaluated for endocrine disruption with consideration of their unique endocrine system and not with consideration of the endocrine system of vertebrates.
Introduction
Over the last two decades, both the scientific community and the lay public have become increasingly aware of the risk associated with endocrine disruption. Environmental authorities in Europe, the United States and many other countries are preoccupied with the identification of Endocrine Disrupting Chemicals (EDCs) and their effects on human health and wildlife. In 2012, a report on the state of endocrine disruption was composed under the auspices of the United Nations Environment Program (UNEP) and the World Health Organization (WHO). This report stated that in spite of numerous reports of reproductive disorders, skewed sex ratios and intersex phenomena, the understanding of endocrine disruption in invertebrates—including crustaceans—was limited (1). Since then some progress has been made, but knowledge about the effects of EDCs on the endocrine system of crustaceans remains scarce and concerns mainly pesticides designed to disrupt moulting in insects (2, 3). Among the vast array of chemical compounds listed on the Toxic Substances Control Act (TSCA) inventory (US EPA)—and many others not regulated under the TSCA—there will certainly be many other chemicals that have the potential to interfere with the crustacean endocrine system.
With about 68,000 extant species of crustaceans described—and still more to be discovered—these predominantly aquatic invertebrates form a large and very diverse arthropod taxon (4–6). As predators, scavengers, or filter feeders, they take important positions within the aquatic ecosystems at various levels of the food web. They also provide high-value fishery products and contribute with over 14 million tons—of which half are wild stock captures—to about 8% of the worldwide seafood resources (7).
This ecological and economical wealth is, however, endangered by habitat loss, climate change, pollution, overexploitation, invasive species and other anthropogenic stressors (8). In the early nineties, drastic declines of amphipods were reported in some of the Great Lakes (9, 10). Similarly, populations of the amphipod Gammarus lacustris significantly declined in the Selenga River delta, the main tributary to Lake Baikal (11). Although pollution, such as polychlorinated biphenyls (PCBs) or pulp mill effluents, respectively, were given consideration, the actual cause-effect relationships were complex and difficult to establish. Massive decreases in blue crab populations of Chesapeake Bay occurred in the nineties, where spawning stock abundance declined by about 80% (12). Similarly, catches of the edible crab, Cancer pagurus, in the English Channel were halved from 2012 to 2018 with no clear cause for this decline (13). Even if overfishing, increasing temperatures, and ocean acidification are contributing causes, reproduction impairment due to endocrine disruption could be adding to the declines, or hampering the recovery of stocks. A contribution of EDCs in these declines of marine and freshwater crustaceans is difficult to discern. Firstly, it is generally challenging to determine the quantitative contribution of individual challenges to population sustainability. Secondly, it is difficult to identify endocrine disruption without knowing how to measure it. In any case, no prominent example of endocrine disruption, comparable to imposex in prosobranch gastropods, is known for crustaceans. Examples of intersex that appear to be related to pollution have been reported for different crustacean species (14–19). But no cause-effect relationships have been established.
The assessment of endocrine disruption in crustaceans can be approached either from the perspective of the molecules suspected to cause endocrine disruption, i.e., effects of established EDCs, or from the perspective of the endocrine targets of EDCs, i.e., crustacean endocrinology. Environmental chemicals that have been classified as EDCs, are, for the most part, compounds that interfere with the vertebrate hormone system. Strenuous effort has been made to demonstrate possible endocrine disrupting effects of such EDCs in crustaceans (20–30). Although effects on growth and reproduction are often reported, most studies failed to demonstrate that endocrine mechanisms were involved. The generally high concentrations that are necessary to produce negative consequences for growth or reproduction suggest that the observed effects were merely a result of overt toxicity (e.g., (31, 32). More specific assays are being developed that use elements of the crustacean endocrine system, such as reporter assays for the ecdysteroid and methyl farnesoate receptors, measurement of 20-hydroxyecdysone (20E) titers, or assays for chitobiase activity (33–38). Nevertheless, establishing reliable testing protocols for endocrine disruption in crustaceans remains challenging and widely ignores the importance of neurohormonal regulation for the control of many physiological functions in crustaceans.
This review argues in favor of a more arthropod specific approach to endocrine disruption in crustaceans.
Evolution of the Invertebrate Endocrine System
Endocrine systems are key features of evolution reflecting metazoan diversification (39). Metazoan endocrine systems have evolved from a common bilaterian ancestor before the divergence of protostomes and deuterostomes more than 600 million years ago (40, 41). Specialized neurosecretory cells were already present in the pre-bilaterian cnidarians and neurohormonal signaling persists as a major endocrine component in both, the protostome and deuterostome lineages (42–44). The arthropods diverged within the protostome lineage some 500 million years ago (45). Unique aspects to the physiology of the arthropods, including crustaceans, required the development of unique endocrine pathways to regulate these physiological processes. For example, arthropods lost the capacity to synthesize cholesterol (41, 46). This loss of cholesterol synthesis may have limited opportunities for the evolution of steroid hormones, which utilize cholesterol as a precursor. However, this loss may have also promoted the evolution of methyl farnesoate (MF) in crustaceans and juvenile hormone in insects, which do not utilize cholesterol, into functional hormones (41). The divergence of the arthropod endocrine system has been deepened further by the evolution of an exoskeleton in arthropods, which requires moulting for growth and reproduction (47). Endocrine signaling processes were required to regulate moulting and coordinate this process with those operative in growth and reproduction.
While the endocrine systems of the two major arthropod taxons, crustaceans and insects, share many commonalities, divergences also occurred. For example, both groups possess the capacity to produce MF. However, insects possess a cytochrome P450 monooxygenase, which epoxidates MF to form juvenile hormone III (48). This latter hormone regulates many of the processes in insects that are under the control of methyl farnesoate in crustaceans. Crustaceans produce a family of hormones known as crustacean hyperglycemic hormones (CHHs) (49, 50). These hormones regulate a myriad of processes including aspects of moulting, reproduction, and energy generation. Only a single member of this family, the ion transport peptide, is known to exist in insects. This insect hormone controls ion transport (51, 52).
The presence or absence of nuclear receptors, i.e., ligand-regulated transcription factors, reflects the separation of steroid hormone signaling within the endocrine systems of crustaceans and vertebrates. The evolution of these receptors was shaped by whole genome duplications and losses combined with the evolution of neofunctionalization or subfunctionalization among duplicate receptors (39, 53–55). Importantly, arthropods lack receptors of the steroid receptor subfamily 3, which include the estrogen and androgen receptors. This may represent the loss of the progenitor of this subfamily (56, 57). However, subfamily 3 receptors are also absent in the ascidian Cionia intestinalis, a deuterostome invertebrate distantly related to vertebrates (55, 58). This latter observation suggests that subfamily 3 nuclear receptors evolved later in chordate evolution, long after protostomes and deuterostomes diverged (59, 60). This hypothesis is supported by the appearance of sex steroid receptors, including the estrogen receptor, in the cephalochordate Amphioxus (61, 62). The ecdysteroid receptor (EcR) of arthropods does not belong to the subfamily 3 nuclear receptors, but is an ortholog of the vertebrate liver X and farnesoid X receptors, which are members of the subfamily 1 nuclear receptors (63).
The loss and gain of functions and elements of the endocrine system, such as nuclear receptors or neuropeptide hormones in arthropods is likely to be related to the invention of a new body plan with an exoskeleton (64). The exoskeleton constrains growth and the impermeable cuticle limits gas and ion exchange with the environment. Moulting solves the first problem, while the second problem requires organs for gas (and ion) exchange, such as the gills in crustaceans or the tracheae in insects, both of which have similar organogenetic origins. Sanchez-Higueras et al. (65), demonstrated that trachea develop as serial homologues to the ectodermal prothoracic gland and the corpora allata, which have their counterparts in the Y-organ and the mandibular organ of crustaceans (see also (43)). These endocrine glands produce ecdysone and juvenile hormone/MF, respectively, i.e., insect/crustacean hormones related to moulting and metamorphosis. Hence, these findings link the evolution of arthropod hormones and endocrine organs with the evolutionary innovation of an exoskeleton. As a matter of consequence, the homology of endocrine organs and gills provides evidence for the integration of environmental cues into physiological responses, such as respiration, osmoregulation and growth by an endocrine system specific to arthropods.
Crustaceans and vertebrates share the principal concept of connecting neural, neurosecretory, and endocrine components (58). Yet, the endocrine systems of arthropods and vertebrates have evolved independently and differently since more than 540 million years ago when crustaceans and deuterostomes appeared in the early Cambrian (66, 67). As a matter of consequence, they have little in common. The fundamental difference between arthropod and vertebrate endocrine systems has implications for determining endocrine disruption. Exogenous substances that alter functions of the endocrine system are, for the most part, likely to be different for crustaceans and vertebrates. Substances designed to interfere with the insect endocrine system (i.e., insect growth regulating insecticides) by their design have significant potential to interfere with the crustacean endocrine system, but are much less likely to interfere with the endocrine system of vertebrates. Conversely, steroid hormone functional analogs are highly likely to disrupt endocrine regulated processes in vertebrates, but are much less likely to disrupt endocrine signaling in crustaceans. The most conserved elements associated with the endocrine regulation of physiological functions shared by both, arthropods and vertebrates are the upstream control of neurosecretory processes by biogenic amines. Besides their function as neurotransmitters and neuromodulators, biogenic amines, such as serotonin, can also serve as neurohormones circulating in the blood stream and affecting peripheral organs like ovaries.
Endocrine Regulation Of Moulting
The monophyly of arthropods is based on a sclerotized exoskeleton (68). To allow for growth, the cuticle has to be shed and renewed periodically. Moulting, i.e., ecdysis, is a distinctive characteristic of the crustacean life cycle (69), which involves a complex interplay of numerous neuropeptide and steroid hormones (Figure 1), thereby covering key features of the endocrine system of crustaceans. As such it is an endpoint par excellence for endocrine disruption in crustaceans. In many cases, moulting in crustaceans is also related to reproductive periods by alternating cycles of moulting and reproduction, as in Daphnia (80, 81), so that perturbations of ecdysis, may also affect reproductive phases.
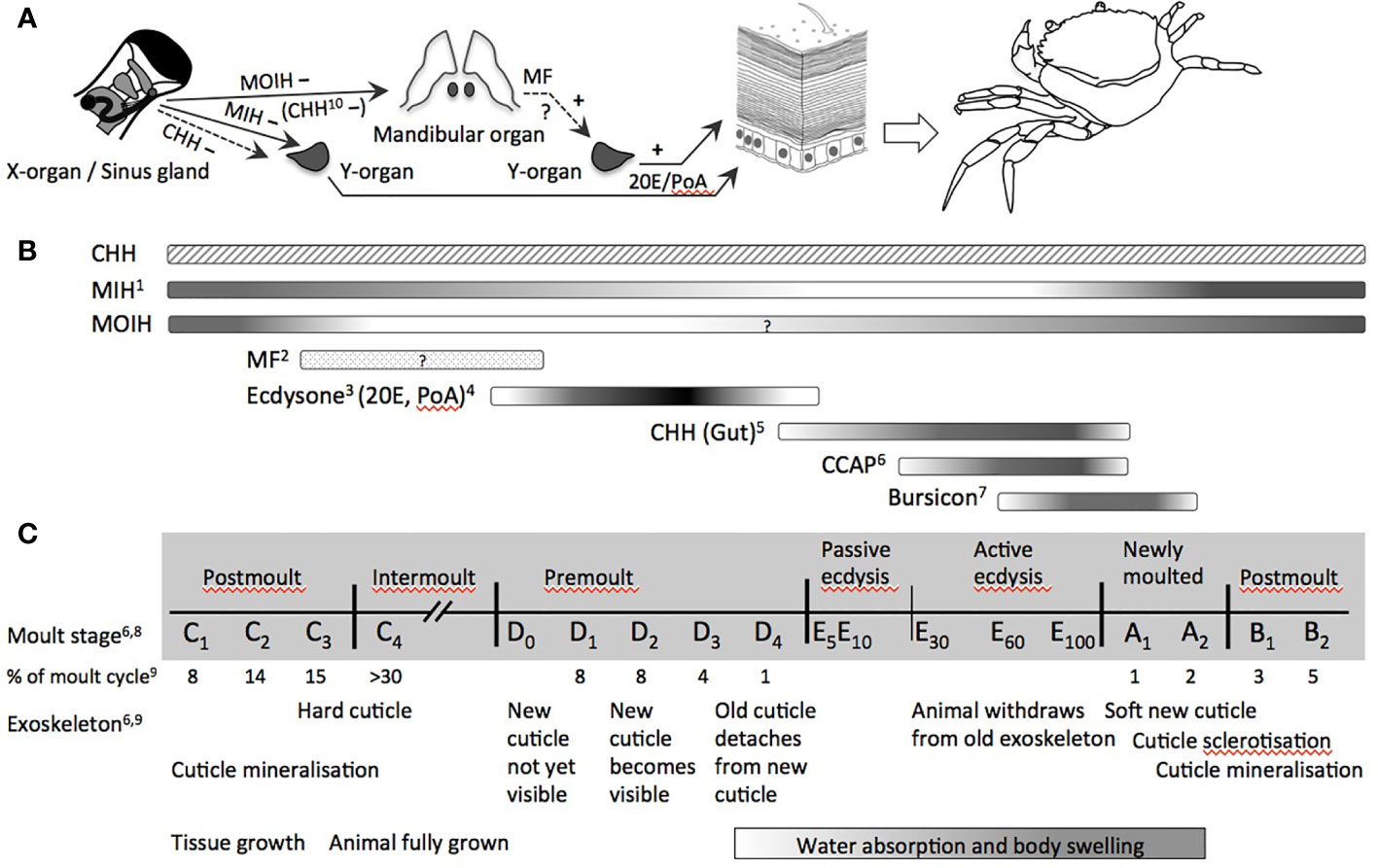
Figure 1 Endocrine control of moulting in decapod crustaceans (Malacostraca). (A) Organs directly involved in the control of moulting (release of CCAP and bursicon by the central nervous system and/or the pericardial organ as well as release of CHH by paraneurons of the fore- and hindgut not shown): MIH and CHH from the X-organ/sinus gland-complex negatively inhibit the synthesis of ecdysone by the moulting gland (Y-organ); MOIH (and in some species CHH) negatively inhibit the synthesis of MF, the stimulatory activity for ecdysteroid synthesis has been reported in several studies; ecdysteroids of the major forms 20E and PoA stimulate the epidermis via its corresponding EcR to decalcify and to lyse the membrane layer and the old cuticle by proteases, chitinase and chitobiase, and to synthesize material for the new cuticle. (B) Dynamic hemolymph titres of (neuro-)hormones involved in the control and/or physiological processes during moulting and ecdysis over an entire moult cycle. (C) Moult stages and main modifications of exoskeleton and growth (non-proportional presentation with respect to duration of each period). Abbreviations: CCAP, crustacean cardioactive peptide; CHH, crustacean hyperglycemic hormone; EcR, ecdysteroid receptor; MF, methyl farnesoate; MIH, moult-inhibiting hormone; MOIH, mandibular organ-inhibiting hormone; 20E, 20-hydroxyecdysone; PoA, ponasterone (A) 1 (70); 2 (71); 3 (72); 4 (73); 5 (74); 6 (75); 7 (76); 8 (77); 9 (78); 10 (79).
The crustacean moult cycle is divided into five major stages comprising numerous substages [Figure 1; (69, 82)]. During the intermoult stage (C4), the neuropeptide moult-inhibiting hormone (MIH) represses ecdysteroid synthesis. The proecdysial period begins with the apolysis of the old exoskeleton, during which the membrane layer and the endocuticle are degraded and their building materials are partially resorbed (D1–D3). Simultaneously, the new exoskeleton is synthesized. A surge of ecdysone during premoult triggers downstream events that lead to the extrication of the animal from its carapace (E). Despite the long history of endocrine research in crustaceans, the hormonal regulation of these events still lacks precise knowledge of some factors, such as homologs of ecdysis-triggering hormone (ETH) and eclosion hormone (EH). The function of these neuropeptides is well described for insects, but awaits further elucidation in crustaceans. More recent work also suggests a yet unknown role for corazonin in the control of moulting (83). Eventually, CHH and crustacean cardioactive peptide (CCAP) are tightly linked, respectively, with ecdysial water uptake and the onset of the behavioral motor program by which the animal extricates from its carapace. Tanning and sclerotization of the new cuticle are under the control of bursicon, another neuropeptide hormone. At large, the complex control of moulting described in the following, which involves many other hormones beyond MIH and ecdysone, provides various targets for endocrine disruption in crustaceans.
Neuro-Endocrine Regulation
Almost 70 years after Passano (84) proposed the existence of MIH secreted by the major neurohemal organ in decapod crustaceans, the X-organ/sinus gland (XO/SG) complex of the eyestalk (reviewed in (85, 86)) and the characterization of the respective neuropeptide by Webster and Keller in 1986 (87), the main features of neuroendocrine moult control are generally accepted for decapods. According to this regulatory model, the activity of the moulting gland (i.e., Y-organ) is inhibited by MIH during intermoult, thus subjecting ecdysteroid signaling to the negative control by neuropeptides from the XO/SG (Figure 1) (69, 72, 88–90). Evidence is provided by experiments removing eyestalks or XO/SGs, which results in shortened moult intervals and higher moult frequency (72). This inhibition of ecdysis in eyestalk/XO/SG-ablated animals can be restored with extracts from the SG. Dynamic variations of MIH during the moult cycle as well as decreases of MIH stored in the SGs and circulating MIH in the hemolymph during pro- and postecdysis further corroborated the role of MIH in neuro-endocrine regulation of ecdysis (70, 88, 90, 91). Furthermore, Techa and Chung (70) suggest a feedback control by which elevated ecdysteroid concentrations in the hemolymph stimulate mih-expression, but inhibit MIH-secretion. As a matter of consequence, high amounts of MIH are stored in the SG during ecdysis and released during post-moult, resulting in low ecdysteroid titers. Hence, any perturbation of synthesis, storage and release of MIH from the XO/SG could result in modifications of Y-organ inhibition, ecdysone synthesis and the resulting circulation of ecdysteroid levels (e.g., (92)).
MIH is not the only factor controlling ecdysteroid secretion from the Y-organ. During proecdysis, the Y-organ becomes progressively less sensitive toward MIH-mediated inhibition of ecdysteroid production (88, 89, 93, 94), most likely due to modifications in intracellular signaling cascades following binding of MIH to its G-protein coupled receptor. Little attention has been given to the role of CHH in the inhibition of ecdysone synthesis. Albeit, CHH is 10-fold less effective in inhibiting the Y-organ, its levels are roughly 10-fold higher than those of MIH. Chung and Webster (88), therefore, argued that CHH could have an equivalent role to MIH in the negative control of ecdysteroid synthesis. The situation is further complicated by the implication of other neurohormones in moulting (Figure 1). Notably, CCAP displays considerable dynamics during ecdysis (75) and increases massively during late ecdysis when the animals actively exuviate. Phlippen et al. (75) explain this surge of CCAP by its mytropic action, thus potentiating muscle contraction while the animals withdraw from their old exoskeleton, and by its effect on cardiovascular functions regulating hemolymph flow and pressure. Interestingly, CHH released from paraneurons of the fore- and hindgut rises about 100-fold during ecdysis, followed by the surge of CCAP, both reaching their maximum at late ecdysis and decreasing rapidly in newly moulted animals (74) (Figure 1). Injections of CHH at physiological concentrations were able to initiate ecdysis through swelling as a result of isosmotic water uptake by the hindgut, suggesting a role of CHH in ion and water uptake during moulting, similar to the function of the homologous ion transport peptides in the hindgut of insects (74). Eventually, bursicon, another neuropeptide hormone produced by the central nervous system overlapping with CCAP is massively released from the pericardial organ into the hemolymph when ecdysis is completed and remains significantly elevated during early postmoult (76) (Figure 1). Its consistent presence throughout the moult cycle at about one quarter of its concentration during ecdysis gives rise to further cuticle-associated functions of bursicon during moulting (95).
Interestingly, the nature of hindgut CHH, CCAP, and bursicon and their role in ecdysis bear resemblance to neuropeptides involved in the ecdysis of insects. Because any spontaneous release of, notably, CCAP could be excluded, the existence of yet non-identified ecdysis-inducing factors similar to ETH and EH of insects would complete the overall picture of ecdysis in crustaceans (75). Indeed, analyses of the Daphnia pulex genome, suggest that such factors may exist in crustaceans (96, 97). More recently, carcikinin, an ortholog of ETH was identified in Carcinus maenas (98) and ETH genes were found in several crustacean transcriptomes [see (99) for references]. Injection of ETH into the crayfish Cherax quadricarinatus prolonged the moult period, suggesting a role in the control of the moult cycle in decapod crustaceans (99). Similarly, EH was identified in the shrimp Exopalaemon carinicauda, and displayed highest expression levels during premoult, while gene-silencing lead to a delay in moulting and reduced rates of ecdysis (100). Furthermore, the virtually exclusive expression of a G-protein coupled receptor for corazonin in the Y-organ indicates a role for this neuropeptide in the control of ecdysis (83). Despite these promising results, the roles of ETH, EH, and corazonin in crustacean ecdysis await further characterization.
Ecdysteroids
Ecdysteroids are the predominant hormones responsible for moulting and other processes in crustaceans. Ecdysteroid metabolism is relatively well understood owing to advances in insect biochemistry and conserved pathways between insects and crustaceans. While crustacean ecdysteroids and their nuclear receptors are similar to those of insects, they differ in the number of hormones and in the number and structure of the receptor isoforms (101).
The concentrations of ecdysteroid hormones circulating within crustaceans vary during the moult cycle, and depending on the species, either gradually or quite rapidly spike to start a period of ecdysis or moulting (Figure 1) (102). Ecdysteroids and the enzymes responsible for breakdown of the chitin skeleton have been measured through various molecular and cellular assays targeting gene expression and/or enzyme synthesis, respectively.
Enzyme Synthesis and Inactivation
Ecdysteroid biosynthesis is divided into two stages (103). The first stage involves the conversion of cholesterol, derived from diet, to 5β-diketol and the second stage converts 5β-diketol to secreted products. Depending on the species, four major secreted products are ecdysone, 3-dehydroecdysone (3DE), 25-deoxyecdysone (25dE), and 3-dehydro-25-deoxyecdysone (3D25dE) (103). A large number of ecdysteroidogenic enzymes and associated genes have been identified in insects and other arthropods (104) with a large degree of conservation in the crustaceans (105, 106). For example, orthologs of nmg/sro, spo, phm, dib, sad, and shd have been identified in the D. pulex genome (46, 107, 108).
Both the increase and subsequent rapid decline in ecdysteroid titers are critical to moulting. The cytochrome P450 hydroxylase cyp18a1 is primarily responsible for the inactivation of the hormone, rendering it susceptible to further modification and elimination (103, 109).
The inhibition of cytochrome P450s by chemical compounds is a widespread mechanism (110), which could interfere with the multiple hydroxylation reactions catalyzed by the cytochrome P450s encoded by the “Halloween genes” (104). For instance, several classes of fungicides function by inhibiting cytochrome P450-mediated demethylation of sterols that are critical components of fungal cell membranes. Some of these compounds are also capable of inhibiting cytochrome P450s of non-target organisms that are involved in steroid biosynthesis. Exposure of Daphnia magna to the demethylase inhibiting fungicides fenarimol, pyrifenox, prochloraz, triadimefon, and propiconazole delayed moulting and/or caused developmental abnormalities in neonates (111, 112). Mechanistic studies revealed that fenarimol exposure reduced ecdysteroid levels in daphnids and that co-exposure with 20E protected against the delay in moulting and developmental abnormalities caused by this fungicide (113). These results are consistent with the hypothesis that fenarimol elicited toxicity by inhibiting cytochrome P450s involved in ecdysteroid synthesis.
Receptor-Mediated Activation
Ecdysteroid hormones, such as the major forms 20E and ponasterone A (PoA), bind to the ligand-binding domain of the EcR and activate the expression of primary early ecdysteroid responsive genes, such as E75 and E74 and early late genes such as HR3 and HR4 (114, 115) (see also reviews by (116, 117)). These transcriptional regulators drive the “late genes” responsible for metamorphosis, moulting, and/or ovarian development (114, 118–120). For ligand binding, the EcR forms a heterodimer with the retinoid-X-receptor (RXR), the ortholog of the insect ultraspiracle (121). The RXR contributes to DNA-binding and helps to stabilize the EcR-ligand binding pocket and allows for flexibility in ligand binding (122, 123). Unliganded EcR-RXR is a repressor of transcription (123).
An account of the transcriptional activities of some of these late genes during premoult and ecdysis is given by Li et al. (124). Notably, genes involved glucosamine synthesis, corresponding to the formation of material for a new cuticle, were upregulated during the premoult, whereas genes encoding chitin synthase and several chitinases were upregulated during ecdysis and postmoult stages. Consequently, enzymes such chitobiase (125), N-acetyl-β-glucosaminidase (126) or chitin synthase (127) can be used as biomarkers for EcR-induced late genes provided that the xenobiotic concentrations are low and act via EcR-signaling rather than inhibiting the enzyme itself or exerting non-specific effects on gene-expression. Furthermore, enzyme-expression and activity have to be put in the perspective of the precise moulting stage (127, 128).
Disruption of Ecdysteroid Signaling
Several targets exist at which environmental chemicals might interfere with ecdysteroid signaling. These include disruptions in ecdysteroid synthesis, ecdysteroid inactivation, and interactions with the EcR. The neuroendocrine regulation of ecdysteroid signaling also may provide targets of disruption, though few definitive examples of such disruption exist. Overall, the XO/SG-Y-organ-EcR axis of malacostracan crustaceans offers an endocrine signaling cascade similar to the mammalian hypothalamus-pituitary-gonadal-ER axis.
Modulation of Ecdysteroid Levels
Studies have reported on the ability of environmental chemicals to alter the expression of ecdysteroid biosynthetic or biotransformation enzymes. For example, exposure of the copepod Tigriopus japonicas to 20 mg·L–1 atrazine resulted in a reduction of mRNA transcripts for several enzymes involved in ecdysteroid biosynthesis and biotransformation (129). Unfortunately, impacts on ecdysteroid levels were not established. A generalized reduction in relevant gene expression levels suggest that atrazine may have disrupted common neuro-endocrine control of these genes, or that the high concentration of atrazine used resulted in overt toxicity presented as an overall decrease in transcription.
Exposure of the Chinese mitten crab, Eriocheir sinensis, to the pharmaceutical carbamazepine reduced hemolymph ecdysteroid levels and epidermal chitobiase activity. Carbamazepine exposure also increased chh and mih-expression; while, decreasing EcR and RXR mRNA levels. Taken together, these effects suggest that carbamazepine may have perturbed the neuroendocrine control of ecdysteroid synthesis resulting in a decrease in ecdysteroid levels and a down-regulation of ecdysteroid-regulated genes. These perturbations in the ecdysteroid-signaling pathway also resulted in delayed moulting (130).
Ecdysteroid Receptor Agonists/Antagonists
Plants produce compounds with ecdysteroidal activity, presumably to serve as an endocrine-disrupting defense against invading insects (e.g., (131)). Similarly, EcR-agonist activity has been exploited as a mode of action of some next-generation non-steroidal insecticides (132). The insecticidal EcR-agonist tebufenozide only weakly activated the D. magna EcR in a reporter two-hybrid assay (133). Similarly, tebufenozide, along with the related diacylhydrazines halofenozide and methoxyfenozide were weak agonists in a reporter assay containing the shrimp Neocaridina davidi-EcR (34). De Wilde et al. (33), however, could not confirm accommodation of tebufenozide into the ligand binding pocket of the shrimp Neomysis integer and found no effect of 100 µg tebufenozide·L–1 on nymphal development and moulting. Taken together, these results are consistent with the manufacturer’s report that the diacylhydrazine insecticides exhibit low specificity for EcRs of non-target arthropods (132). They may, however, displace 20E and/or PoA from the binding site by competitive binding (34).
A recent in silico study by (134) identified 274 potential non-steroidal EcR-ligands. Furthermore, the screening of 8795 compounds listed in the US EPA’s ToxCast chemical library revealed 34 potential agonists including the diacylhydrazines insecticides and numerous pharmaceuticals, such as non-steroidal anti-inflammatory drugs containing pyrazolone derivatives, or members of the amphenicol antibiotic family. Using the Drosophila melanogaster BII cell assay, Dinan et al. (135) detected no EcR-agonist activity among 80 environmental chemicals. Bisphenol A, diethylphthalate, some polycyclic aromatic hydrocarbons, naphthalenes, pesticides, and pharmaceuticals were weak antagonists in this assay. Notably, estradiol, progesterone, and testosterone as well as synthetic steroids neither displayed agonist, nor antagonist activity, except for two compounds, 4-androstene-3,17-dione and 17α-ethinylestradiol, which were weak antagonists.
Despite the lack of anti-ecdysteroidal activity associated with testosterone reported by Dinan et al. (135), weak EcR-antagonist activity of testosterone was reported in daphnids (136). Exposure of D. magna to micromolar concentrations of testosterone caused a concentration-dependent delay in moulting and an increase in developmental abnormalities among neonates. Co-exposure with 20E protected against this toxicity of testosterone. Testosterone did not lower endogenous 20E-levels, but rather appeared to antagonize the EcR, based upon competition assays between testosterone and 20E in ecdysone-responsive Drosophila Kc cells. While these results suggest that testosterone is anti-ecdysteroidogenic in daphnids, the results have little environmental relevance to environmental androgens due to the high concentrations required to elicit a response.
Gene Product Changes
Several studies have reported on the impacts of exposure to pollutants, pharmaceuticals, and vertebrate hormones on expression profiles of mRNAs or proteins along the ecdysteroid signaling pathway. Expression of ecr was elevated from exposure of prawns, Macrobrachium potiuna, to glyphosate-based herbicides, ethinylestradiol (estrogen), 4-hydroxytamoxifen (anti-estrogen), 17α-methyltestosterone (androgen), and cyproterone acetate (anti-androgen) (30, 137). Exposure of the intertidal mud crab Macrophthalmus japonicas to bisphenol A and di-(2-ethylhexyl) phthalate significantly elevated ecr expression levels (138). Studies, such as these are indicative of exposure to potential endocrine disrupting chemicals. However, whether the exposure actually results in apical disruption remains equivocal in the absence of demonstrated consequences of the molecular alterations.
Conversely, several studies have reported on effects of chemical exposure on apical endpoints, such as moulting. Zou (139) identified 33 compounds that have been shown to delay, impede, or advance moulting in crustaceans. While such studies inform on the toxicity of the chemicals to crustaceans, they do not provide insight on whether the effects elicited are actually due to endocrine disruption.
Neurohormone Activity Modulation
The negative control of ecdysteroid synthesis by MIH and by CHH is under the control of biogenic amines, notably, serotonin (5-hydroxytryptamine, 5-HT). Evidence suggests that the hyperglycemic action of 5-HT is due to the direct stimulation of CHH neurons (50, 140, 141). Indeed, 5-HT-immunopositive efferent axons to the medulla terminalis and the XO-neuropile have been demonstrated (142). The excitatory role of 5-HT on these XO-neurons was shown by Sáenz et al. (143). Therefore, serotoninergic stimulation of the release of neurohormones from the XO/SG-complex is likely to be a general phenomenon that applies to CHH as well as to MIH or mandibular organ inhibiting hormone (MOIH). The effects of fluoxetine on ecdysteroid levels in C. maenas demonstrated that fluoxetine, a selective serotonin reuptake inhibitor, significantly decreased 20E-levels at 0.5 and 0.75 µM after 8 and 4 h, respectively (92). Because, this effect was even more rapid and more pronounced with 0.5 µM 5-HT, but no effect of 5-HT or fluoxetine on 20E-levels could be observed in eyestalk-ablated animals, it was concluded that the mechanism leading to reduced 20E would be the inhibition of ecdysteroid synthesis by 5-HT-stimulated release of MIH. Interestingly, low ecdysteroid levels appeared to relate to an increased mih-expression in this study.
To date, only few studies examined the effects of pollutants on neuroendocrine processes that control moulting in crustaceans and only recently has MIH been proposed as a biomarker of endocrine disruption in crustaceans (30). This may be explained by the difficulties to quantify MIH-levels in the hemolymph, due to its 10 times lesser concentrations as compared to CHH and because of the pulsatory release of these neuropeptides from the SG (88, 144). Thus, mih-expression has been used to evaluate the effects of EDCs on the neuroendocrine regulation of moulting (30, 92, 137). Gismondi (30) and de Melo et al. (137) found an over-expression of mih in response to estrogen agonists and antagonists as well as antiandrogenic and androgenic compounds and a glyphosate-based herbicide. Because 20E equally increased mih-expression in the study of Gismondi (30), probably by the feedback control described by Techa and Chung (70), it was concluded that EDCs interfering with vertebrate steroid hormone signaling could affect mih-expression via an ecdysteroid related pathway, but without any further mechanistic explanation. The fact that very different compounds, which either activate or block the estrogen receptor or interact with other steroid receptors and even glyphosate all stimulated mih- as well as ecr-expression rather points to other, non-specific effects. Nevertheless, increased expression of mih may lead to an increased synthesis and release of this neuropeptide with the potential to modulate ecdysis.
Vertebrate-Type Sex Steroids
Whether or not crustaceans utilize estrogen, androgen, and progestogen signaling pathways has been debated for decades (145). Evidence in support of these signaling pathways is based largely upon observational studies; while, evidence against the existence of these signaling pathways is supported by genomic investigations and evolutionary biology. Interaction with the EcR is often cited as a mechanism by which vertebrate-type sex steroids function in crustaceans. However, as discussed above such interactions typically occur at high, non-physiologic levels.
Evidence in Support
Presence of the Hormones
The detection of vertebrate-type sex steroids in crustaceans was often cited in earlier literature as evidence that these hormones are of physiological significance in these organisms (146, 147). These include 17β-estradiol and testosterone in amphipods (148) and crayfish (149), pregnenolone in brine shrimp (150), and progesterone in shrimp (151). Several studies report that vertebrate-type steroid levels vary with the ovarian development cycle suggesting some functionality related to this process (discussed in (152)). However, the mere presence of a hormone in an organism does not indicate that the chemical possesses a signaling role in that organism. The hormone may be present as a consequence of dietary uptake or as a non-functional intermediate or metabolite of a biosynthetic pathway (153–156).
Responses to Exogenous Steroids
Many studies have demonstrated physiological responses of crustaceans to exogenously administered steroid hormones. For example, administration of 17β-estradiol advanced ovarian development (29) and stimulated vitellogenesis in female decapods (28). This compound also suppressed vitellogenesis-inhibiting hormone gene-expression, which was presumably responsible for the effects on ovarian development (28). While 17β-estradiol administration to crayfish stimulated vitellogenin-mRNA accumulation in the hepatopancreas, progesterone administration increased vitellogenin protein levels in the hemolymph of crayfish (157).
The provision of exogenous 17β-estradiol and progesterone support suggestions of a role for these hormones in crustacean reproduction. Some studies, however, indicate that estrogens downregulate monoamine oxidase activity (158–160) and may, therefore, increase 5-HT-levels that directly influence ovarian development. Furthermore, administration of testosterone has largely resulted in detrimental effects. Administration of testosterone to water fleas suppressed embryo development (136) and decreased lipid storage (161). These effects were attributed to the ability of testosterone to elicit anti-ecdysteroidal activity.
Responses to Endocrine Disruptors
Several investigators have reported on the negative effects of estrogenic and anti-androgenic compounds on crustaceans. Estrogenic compounds, such as diethylstilbestrol, endosulfan, Aroclor 1242, and diethylphthalate delayed moulting in D. magna (162, 163). Studies that have shown neuroendocrine disruption by some of these compounds [Table 1; (172, 173, 176–178)] may provide an explanation for these observations and would situate their effects upstream of ecdysteroid signaling.
The anti-androgen cyproterone acetate severely reduced growth of D. magna without eliciting any discernible effect on moult frequency (145). Effects of several anti-androgens (cyproterone acetate, linuron, vinclozolin, p,p’-DDE) on the reproductive system of copepods revealed varied effects, although consistent among the treatments were degeneration of spermatocytes and deformed spermatophores (163).
Evidence Against
Lack of a Critical Enzyme for Estrogen Biosynthesis
Aromatase (CYP19) is responsible for the metabolic conversion of androstenedione to estrone and testosterone to estradiol. It is thus critical to the synthesis of estrogens. CYP19 is a product of chordate evolution (187) and has not been detected among the protostome invertebrates. Notably, CYP19 is absent from the D. pulex genome (159, 188). While it is possible that estrogens are synthesized in crustaceans via an alternative metabolic pathway, we are aware of no support for this premise.
Lack of Sex Steroid Receptors
Arguably, the greatest evidence against a role of vertebrate-type sex steroids in crustaceans and other Ecdysozoans is the lack of sex steroid hormone receptors (see Section 2). Immunochemical studies have suggested the presence of estrogen receptor α in Gammarus fossarum (27), androgen and estrogen receptors in the mud crab (189), and progesterone and estrogen receptors in crayfish (190). However, immunochemical assays are prone to false positive results due to cross-reactivities or non-specific binding to abundant proteins (191). In the latter studies, putative estrogen receptor co-localized with the other receptor evaluated (progesterone receptor in crayfish and androgen receptor in crab) indicating that antibodies in the same studies may have all been binding to the same abundant protein. Further, results from these studies were inconsistent with estrogen receptor detected in the cytosol from crayfish and membranes of the crab.
We are aware of no reports of the identification of high-affinity sex-steroid binding proteins, indicative of receptors, in crustaceans. Importantly, no sex steroid receptor genes were found in the genome of D. pulex (57). Similarly, the sequenced genomes of the ecdyzoans D. melanogaster and Caenorhabditis elegans revealed no androgen, estrogen, or progesterone receptors [discussed in (27)]. The dominant consensus among researchers is that sex steroid receptors were lost in the lineage leading to the evolution of arthropods (54) and are not present in crustaceans.
High Exposure Concentrations of Hormones and EDCs Are Typically Required to Elicit a Response
Steroid, and similar acting, hormones regulate physiological processes such as development, growth, metabolism, and reproduction. Thus, the action of these chemicals, via receptor-mediated signal transduction, typically does not result in rapid, overt responses by the organism (e.g., acute responses). Rather, acute responses to these chemicals are largely the consequence of some ancillary response to high exposure concentrations of the chemical (e.g., membrane disruption). In contrast, receptor-mediated responses to the chemical present as long-term consequences, such as alterations in development or reproduction (e.g., chronic responses). These chronic responses are often, though not always, elicited at exposure concentrations significantly below those that elicit acute responses. The magnitude of the difference between concentrations of a chemical that elicit acute versus chronic responses is a function, in part, of the binding affinity of the agonist to responsive receptor protein. Hormones bind their receptors with high affinity and, thus, chronic responses to the hormone are typically elicited at concentrations orders-of-magnitude below concentrations that elicit acute toxicity (e.g., high acute/chronic ratio; see Table 2, 20E).
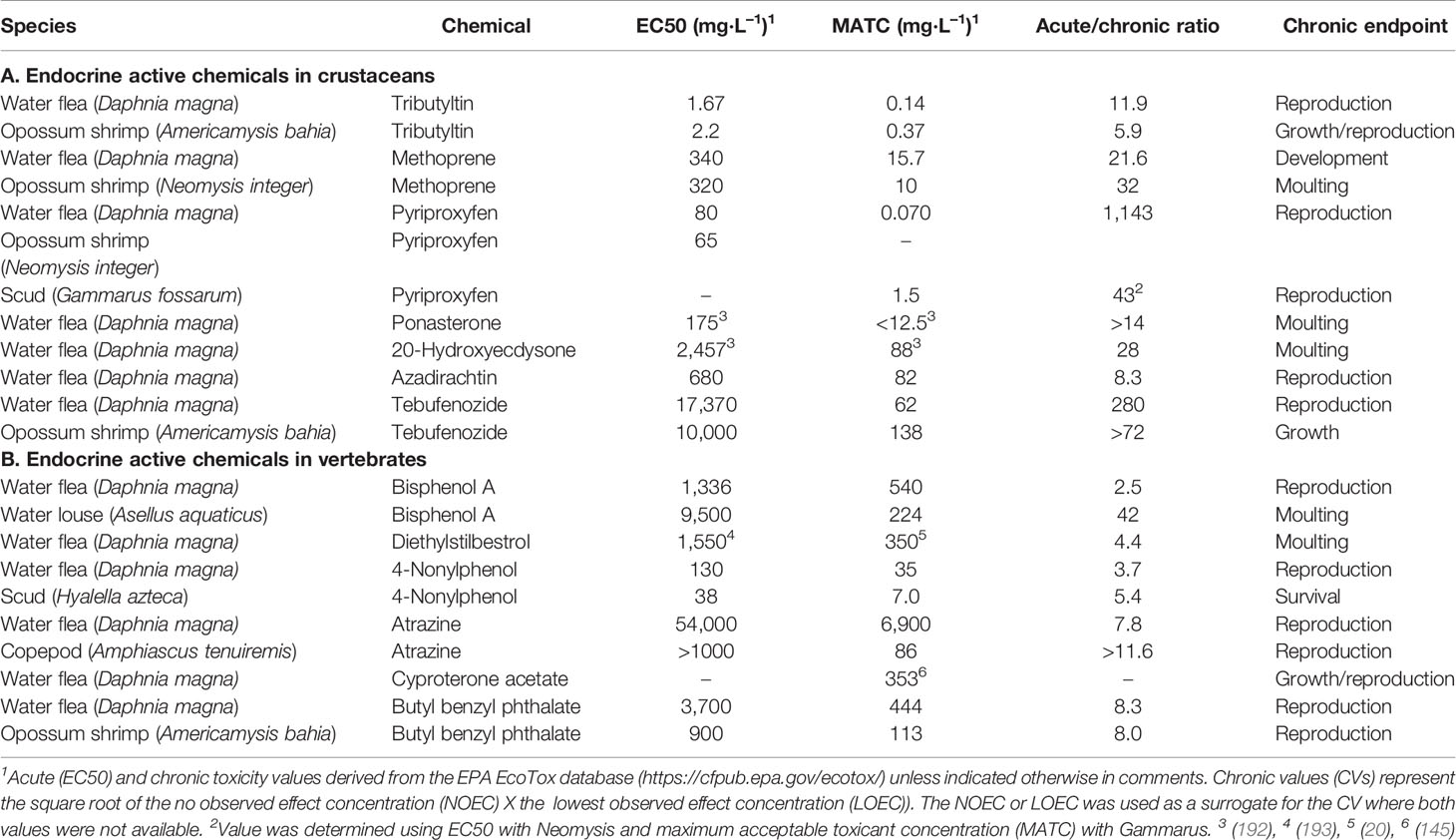
Table 2 Acute and chronic toxicity values for crustaceans exposed to (A) compounds that disrupt ecdysteroid or methyl farnesoate signaling and (B) compounds that disrupt estrogen or androgen signaling.
Compounds known to be endocrine active in crustaceans typically elicit an acute/chronic ratio of 10–1,000 (Table 2A). The chronic responses listed in Table 2A are due to disruption of ecdysteroid and MF-signaling. Compounds known to act in vertebrates via estrogen and androgen signaling pathways, typically elicit acute/chronic ratios in crustaceans of <10 (Table 2B). The latter suggests that chronic responses of crustaceans to these vertebrate EDCs are not elicited through interaction with a hormone receptor.
The strongest evidence for the susceptibility of crustaceans to vertebrate sex steroid agonists are those studies that have shown effects of 17β-estradiol on reproductive system development. Studies cited above reported stimulatory effects of 17β-estradiol on crustacean vitellogenesis (28, 157). However, 17β-estradiol treatments also have been shown to have a negative effect or no effect on crustacean vitellogenesis (194). Exposure of daphnids to the estrogens diethylstilbestrol and bisphenol A had no effect on vitellogenin mRNA levels (195). 17β-Estradiol has been shown to interact with the ecdysteroid receptor at sufficiently high concentrations (196), thus some effects of estrogen injection, the common mode of administration in these studies, may have been the consequence of low affinity interaction of the estrogenic hormone with the ecdysteroid receptor.
Endocrine Regulation of Color Change
Neuro-Endocrine Regulation
Active color changes are termed “morphological” in the case of slow color changes established over weeks and months, whereas the rapid type that can take place in minutes to hours is termed “physiological” (197, 198). In arthropods, coloration and reversible color change have evolved together with the development of an exoskeleton (197). Hence, color may be produced by pigments embedded in the pigmented layer of the endocuticle, or by pigment-containing cells in the epidermis (199). Accordingly, morphological color changes are related to moulting, notably, in terms of ontogenetic color changes (200, 201), whereas physiological color changes are produced by chromatophores.
Rapid color changes in most crustaceans rely on the dispersion and aggregation of pigments within stellate cells containing pigment granules. Generally, monochromatic chromatophores, i.e., black-brown melanophores, red erythrophores, yellow xanthophores, and white leucophores, are intimately arranged in clusters called chromatosomes so as to produce a wide variety of colors (197, 198, 202–204). The dispersion of pigment granules, i.e., their migration from the cell center into the ramifications of the chromatophores renders the coloration more intense, whereas the opposite is the case when the pigment granules aggregate within the center of the cell. Dispersion and aggregation of pigment granules can be completed within half an hour in Crangon up to 2 h in Carcinus or Macrobrachium (83, 205–208). Aggregation and dispersion are regulated by an antagonistic system of neuropeptide hormones composed of red pigment concentrating hormone (RPCH, or simply PCH) and pigment dispersing hormone (PDH) with its isoforms α- and β-PDH (198, 203). Notably, RPCH represents a highly conserved neuropeptide, which, in all decapods so far investigated, has an identical sequence (209, 210).
The antagonistic neurosecretory control of rapid color changes belongs to the best-studied hormonal systems in crustaceans [reviewed in (198, 202, 203)]. Indeed, RPCH and PDH have been the first neuropeptides to be characterized in crustaceans (211, 212). It has been established long ago that the eyestalk of decapod crustaceans is the source of hormones regulating color change in crustaceans (202). This was confirmed by Mangerich et al. (213), who localized the main perikarya of RPCH-secreting cells adjacent to or within the XO of C. maenas and Orconectes limosus, respectively. More recently, Alexander et al. (83) confirmed the presence of about 30 perikarya located in the XO, which project into the SG. A similar situation was shown for PDH, with the majority of PDH-perikarya located between the medulla interna and the medulla lateralis of the eyestalks of C. maenas and O. limosus (214), the axon terminals of which may project into the SG. Hence the major neurosecretory structures of RPCH and PDH are located within the eyestalk from where these neurohormones are released into hemolymph that transports them to the respective epithelial target cells. Because pigment dispersion and aggregation in eyestalk ablated animals could be observed, extra-eyestalk sources of RPCH and PDH have been considered. Indeed, RPCH-cells were found in small numbers in the brain, the thoracic ganglia and the circumoesophageal commissure as well as PDH-cells in the thoracic and connective ganglia (83, 213, 215). However RPCH and PDH of some of these cells may rather serve as a neurotransmitter instead of being implied in color change [e.g., (216)].
A model for signal transduction and intracellular signaling cascades upon binding of RPCH to a G-protein coupled receptor has been proposed for the freshwater shrimp Macrobrachium olfersii (207, 217). In this model, RPCH activates cyclic guanosine monophosphate (cGMP) and Ca2+ second messenger cascades, which in turn stimulate a protein kinase to phosphorylate a myosin II molecular motor. As a result, pigment aggregation is effectuated by the movement of pigment granules along actin filaments in the chromatophore [for details see (198, 218)]. More recently, highly specific RPCH receptors (RPCHR) have been cloned and functionally deorphanized in D. pulex and C. maenas (83, 210). The RPCHR of C. maenas bound RPCH at doses lower than 0.001 nM (EC50 0.02nM). A dose of as low as 0.1 pmol effectively induced pigment aggregation in erythrophores in vivo within 5 min and the effect was stronger and longer lasting when the concentrations of RPCH were increased to 1 and 10 pmol, respectively (83). The RPCHR of D. pulex, on the other hand, bound Daphnia-RPCH at an EC50 of 0.065 nM, but binding of crustacean RPCH was at least two orders of magnitude less efficient. Insect adipokinetic hormone did equally activate the Daphnia-RPCHR in a dose-dependent and only slightly less efficient manner than Daphnia-RPCH (210).
Potential Sites of Endocrine Disruption
The capacity to change color and to adapt to the surrounding luminance may be impaired by pollutants as different as metals, such cadmium or mercury (165), organic chlorine compounds, like PCBs and naphthalene (173, 177), or drugs that affect the levels of biogenic amines (179, 180, 219) (Table 1). In spite of the high concentrations that were employed in these studies, the authors could exclude toxicity and plausibly demonstrate that the respective compounds caused neuroendocrine disruption by affecting the neurotransmitters responsible for the release of, notably, PDH, or the synthesis of the latter. For instance, Aroclor 1242 appeared to reduce norepinephrine (NE) titers in the XO-neuropile (173), thereby reducing the dispersion of black pigment in the chromatophores. Similar observations were made for naphthalene (177). In both studies, the authors took care to verify that neither the chromatophores were affected, which were still able to respond to extracts from the eyestalk containing PDH, nor was the neural tissue damaged. In the case of cadmium, rather the amount of PDH stored in the SG was affected, putatively by inhibiting the synthesis of PDH (165). Fingerman et al. also showed that drugs that deplete monoamine levels, such as reserpine or bretylium, hamper pigment dispersion when fiddler crabs, Uca pugliator, were transferred from a white background with concentrated pigments to a black background, whereas fluoxetine enhanced pigment dispersion by increasing 5-HT-levels (179, 180, 219). Therefore, these early studies pointed to the possibility of psychoactive drugs targeting monoamine levels to interfere with the neurohormonal regulation of color change. This was confirmed by recent studies using more environmentally realistic concentrations of waterborne antidepressants (186). Color change in the sand shrimp, Crangon crangon, was affected by fluoxetine in the range of 10–1,000 ng·L−1 when exposed for 1 day or 1 week (186), suggesting enhanced dark adaptation following fluoxetine exposure (Table 1).
Endocrine Regulation of Sexual Differentiation
Vertebrates typically utilize a variety of genetic sex-determining strategies including sex-determining genes assembled on sex-chromosomes where females are the heterogametic sex, and sex-determining genes assembled on sex-chromosomes where males are the heterogametic sex (220), and autosomal sex-determining genes whose expression are environmentally controlled (221). Common to these sex-determining strategies, sex steroids (androgens, estrogens) are ultimately responsible for sexual differentiation. Indeed, androgens and estrogens from exogenous sources can sometimes circumvent genetic sex-determination (222).
Similarly, crustaceans possess diversity in sex determining mechanisms. Some decapods utilize sex chromosome where female are the heterogametic sex [Penaeus monodon (223); Penaeus japonicus (224)], while male are the heterogametic sex in others [Orchestia cavimana and Orchestia gammarellus, (225)]. Among branchiopods, clam shrimp Eulimnadia texana consists of monogametic males and heterogametic hermaphrodites (226), and brine shrimp Artemia franciscana consist of monogametic males and heterogametic females (227). In contrast, the female and male genomes of D. pulex are identical (228). A major distinction between vertebrates and crustaceans is that crustaceans have evolved strategies for sexual differentiation that do not involve steroidal androgens and estrogens.
Malacostracans
Evidence for an underlying genetic component to sex-determination in malacostracan crustaceans has come from a series of ablation/implantation experiments followed by cross breeding. The chromosomal system for these crustaceans is often referred to as ZW males and WW females. Using isopods (Armadillidium vulgare), Suzuki and Yamasaki (229) were able to transform males into functional females through the ablation of the androgenic gland (AG) and females into functional males through the implantation of the AG. The reciprocal crosses of “genetic” males with converted females and “genetic” females with converted females results in single sex broods. Similar experiments have been done with a variety of other crustaceans including prawns, crayfish, and hermit crabs (230–232). Further evidence of a genetic and potential chromosomal basis to sex-determination has come from breeding experiments with intersex crayfish. When intersex crayfish, Cherax quadricarinatus, that are functionally males were crossed with females the result was a 1:3 (male:female) sex ratio (232). Subsequent crossbreeding between female (WW) crayfish with normal males resulted in an all-female progeny 0:1 (male:female), leading the authors to conclude that the intersex specimens must have been genetic females (WZ). While the evidence for a genetic component to sex- determination remains strong, sex determination in Crustacea can also show degrees of plasticity. Therefore, it is most likely controlled also by epigenetic factors including environmental variables such as light and temperature (233, 234), parasites (235), and even diet (236).
Androgenic Gland Hormone
Male secondary sex characteristics in malacostracan crustaceans are under the control of androgenic gland hormone (AGH), which is produced by the ductless AG (237–239). The AG is usually situated on the paired testes or vas deferens in crustaceans. Its important role was discovered through a series of ablation and implantation experiments in the 1950’s (240–243). Removal of AG results in the cessation of spermatogenesis and the demasculinization of male secondary sexual characteristics [(244) and references within]. Complete andrectomy in some species leads to the conversion of testicular to ovarian tissues that have the capacity to accumulate yolk proteins (245). Similarly, implantation of AGs into female crustaceans results in the conversion from ovarian to testicular tissues and the development of male sexual characteristics. Suzuki (230) was also able to demonstrate through a series of these ablation and implantation experiments at different maturation stages within the isopods that AGH was a sex- differentiating, but not a sex-determining factor in these organisms.
First purifications by Hasegawa et al. and Martin et al. have identified the AGH (246–248). The full insulin like peptide structure, consisting of B chain, A chain, and C peptide and the gene sequence of AGH have been characterised in the late 1990s for the isopod A. vulgare (249–251). Immunohistochemistry has shown that antibodies raised from AGH-peptides display relatively strong species specificity (252), which is not surprising as sexual characteristics are under strong selection pressures. Unfortunately, this makes developing immuno-histochemistry based bioassays for endocrine disrupter studies more problematic. The cDNA sequence for the insulin-like androgenic gland (IAG) gene has now been reported by several species including crayfish, and several prawn/shrimp and crabs (253). This allows for RNA interference (RNAi) techniques to be used to demasculinize and sex reverse aquaculture species with an AG-specific IAG peptide-encoding transcript (254, 255).
Potential Targets of Endocrine Disruption
Currently, it is not known whether environmental pollutants can impact the development of the AG development, or the synthesis of AGH. A number of studies correlated pollutants with increased incidences of intersexuality in crustaceans or male crustaceans displaying certain degrees of feminization or de-masculinization (18). These phenotypic changes in field collected animals mirror the physiological changes caused by feminizing parasite infection, AG ablation or RNAi silencing the AG leading authors to hypothesize whether chemicals can directly or indirectly interfere with the AG or AGH (18, 244). These hypotheses require further testing. In the light of an endocrine axis between the XO/SG, the AG and the male reproductive system, which has been confirmed for decapods (256), a disruption of the neuroendocrine regulation of AGH synthesis and spermatogenesis is conceivable. Indeed, specific CHH-isoforms appear to regulate AGH-expression (257) and its has been demonstrated that metal and organic pollution has the potential to affect CHH-synthesis or -secretion (92, 164, 170–172) (Table 1).
Branchiopods
Sexual differentiation in branchiopods has been extensively studied in Daphnia. Daphnids do not possess sex chromosomes (258, 259) and sexual differentiation of offspring is regulated by environmental cues (32). Under environmental conditions that favor rapid population growth, daphnids reproduce parthenogenetically (diploid oocytes) with all offspring being largely female (260). Maternal organisms produce broods, often consisting of dozens of offspring, every few days. These female offspring then mature in a matter of days and begin producing broods of female offspring. As a result, the population expands at an exponential rate. Under conditions that foretell adversity to population sustainability (exhaustion of resources, impending temperature extremes associated with summer or winter), females introduce male offspring to the population. Males mate with females that are producing haploid oocytes (260). The resulting embryo has undergone genetic exchange, which helps to purge deleterious mutations (261). The embryo is in a resting state of diapause to wait out the period of adversity, and is encased in a protective ephippium, which withstands desiccation and freezing. The ephippium is also hydrophobic, which facilitates transport on transient biota (e.g., aquatic birds) or dispersal in air currents. This facilitates dispersal of the organisms to new habitats (262).
Environmental Regulation
The role of photoperiod and temperature in male sex differentiation of daphnids has been well characterized. Photoperiod and temperature function in concert to regulate sex ratios in D. pulex and D. magna populations (263). Under a long-day, summer-like photoperiod, daphnids produced only female offspring, regardless of temperature (range evaluated was 16-22°C). However, under a short-day, autumn-like photoperiod, daphnids became susceptible to temperature-dependent sex-determination. The different species exhibited different temperature optima for male sex-determination, probably relating to the geographic locations at which the populations used in the study were originally derived. Other environmental factors that have been implicated in male sex-determination include food restriction (264) and crowding (265).
Neuroendocrine Regulation
Exposure of daphnids to environmental conditions that stimulate the production of male offspring resulted in increased mRNA levels for various components of glutamate signaling based upon gene ontology (GO) terms (266). Further, Camp et al. (185) demonstrated that environmental stimulation of male sex determination resulted in increased mRNA levels of the subunit 2 of the N-methyl-D receptor (NMDAR) while having no effect on the NDMAR-a subunit. This change in the abundance of a single subunit of the receptor would result in alterations in subunit composition of the receptors, which has been shown to be responsible for plasticity in receptor function in vertebrates (267, 268). Conceivable, a reduction in the NMDAR-a/NMDAR-b subunit ratio may prompt glutamate signaling leading to male sex differentiation.
A role for the NMDAR in male sex-differentiation was further indicated by the observation that exposure of maternal daphnids to the NMDAR antagonists MK-801 and desipramine significantly increased the number of male offspring (185). Toyota et al. (266) also observed an effect of MK-801 on male sex differentiation; however, these investigators reported that the NMDAR antagonist suppressed male offspring production (266). Differences in results between these two research teams may reflect differences in experimental design. Where Camp et al. (185) reported the number of male and female offspring produced per female over six broods, Toyota et al. (266) reported the percentage of 30-day-old females that produced males, presumably in a single brood.
MK-801 also inhibits 5-HT, NE, and dopamine reuptake transporters, while desipramine inhibits noradrenergic reuptake transporters (269, 270). Therefore, Camp et al. (185) investigated the potential role of these neurotransmitter-signaling pathways in male sex differentiation. The mRNA levels of the serotonin reuptake transporter SERT-a and the α-adrenergic-like octopamine receptor OctaR-A were significantly elevated in daphnids reared upon a short-day photoperiod as compared to those reared under a long-day photoperiod. Two selective serotonin reuptake inhibitors fluoxetine hydrochloride and citalopram hydrobrimide increased offspring male sex determination, although the effect of fluoxetine hydrochloride was not statistically significant (p=0.08). These results suggest that in addition to glutamate signaling other neurotransmitters may be operative in male sex differentiation.
Methyl Farnesoate
Farnesyl units (C15), derived from acetate serve as building blocks for several important biomolecules, such as cholesterol and steroid hormones. In crustaceans, and other arthropods, farnesyl units also are used for the synthesis of farnesoic acid. Crustaceans utilize farnesoic acid as the substrate for MF. MF is a major sesquiterpenoid hormone in crustaceans, akin to juvenile hormone in insects (271).
Male-sex differentiation depends on MF (272, 273). During late stages of maturation MF programs oocytes to differentiate into males. In the absence of methyl farnesoate, offspring differentiate into females (272). MF is responsible for the induction of the doublesex gene (dsx1) during oocyte susceptibility to sex differentiation (274). The doublesex gene product is transcriptionally upregulated in males and is responsible for orchestrating male sex-differentiation (274, 275). Sexually dimorphic expression of the double sex gene also has been shown in other Branchiopod crustaceans including D. magna, Ceriodaphnia dubia, and Moina macrocopa (276).
Synthesis and Degradation
In insects, MF is a product of the mevalonate biosynthetic pathway (277), as is likely the case in crustaceans. Two enzymes along this pathway were identified in lobster that were induced commensurate with MF synthesis (278). 3-Hydroxy-3-methyl-glutaryl-coenzyme A reductase (HMGCR) activity increased within 24 h of eye-stalk ablation, which increased MF hemolymph levels, while farnesoic acid O-methyl transferase (FAMeT) activity was increased substantially two weeks after ablation. The reduction of HMGCR is the final step in the biosynthesis of mevalonate; while, methylation of farnesoic acid is the final step in the synthesis of MF. The authors surmised that increased production of mevalonate via heightened HMGCR reductase activity was responsible for the immediate increase in MF-production following eyestalk ablation, while prolonged increased synthesis of MF was due to elevation in FAMT activity.
In insects, MF is susceptible to metabolism and inactivation through ester hydrolysis and conjugation to polar molecules (279). These inactivation processes are operative in crustaceans as well (276, 280).
The location of synthesis in branchiopod crustaceans has not been established. In decapod crustaceans, MF is synthesized in the mandibular organ, which is under the negative control of mandibular organ inhibiting hormone (281, 282) (Figure 1). MF functions in some aspects of masculinization in decapods, such as the development of the male reproductive morphotype of the spider crab, Libinia emarginata. Abraded males (have not moulted in about a year or more) characteristically have high hemolymph MF-levels, large reproductive organs, and aggressive mating behavior (283).
Receptor-Mediated Activation
The regulatory activity of MF is mediated primarily through interaction with the bHLH-PAS protein methoprene-tolerant (MET) (284, 285). MET derives its name from the discovery that resistance of Drosophila to the insecticidal MF-analog, methoprene, was associated with a functional mutation in this gene (286). MF-activated MET recruits the bHLH-PAS protein, steroid receptor coactivator (SRC) (37). Together, this MET-SRC complex comprises the activated MF- receptor (MfR) in crustaceans.
Other suggested receptors for MF in crustaceans include the RXR and hormone receptor 97g (HR97g). RXR is a member of the nuclear receptor superfamily that has been identified in several crustacean species including the American lobster Homarus americanus (287), the fiddler crab Uca pugilator (288), the tropical land crab Gecarinus lateralis (289), the crayfish Procambarus clarkii (290), the amphipod G. fossarum (291) and the water fleas (D. magna, D. pulex) (58, 292). While MF was unable to activate daphnid RXR in a luciferase reporter assay, its co-administration with 20E to a reporter system consisting of RXR and EcR, resulted in activation greater than that observed with 20E alone (293). This apparent synergistic interaction between activated EcR and activated RXR was also observed in vivo using tributyltin as the RXR agonist (294). These results suggest that MF, through interaction with RXR, may function in concert with 20E to regulate crustacean moulting.
Nuclear receptor HR97g, isolated from D. pulex, was mildly activated by MF and the MF-analog pyriproxyfen in a luciferase reporter assay (295). This crustacean receptor was first identified in D. pulex (57), and has since been identified in the spiny lobster Panulirus ornatus (296). The physiological significance of the receptor as a ligand-activated regulator of crustacean physiology remains unknown.
Known Targets of Disruption
The MfR is the best-demonstrated target of disruption of this regulatory pathway by environmental chemicals. Compounds that elicit insecticidal activity as juvenile hormone analogs also typically bind and activate the MfR (37, 285). This activity is responsible for the high sensitivity of crustaceans to this class of insecticides (273, 297, 298). However, the MfR appears to have high ligand recognition specificity. We are aware of no demonstrations that compounds, other than juvenile hormone analogs, are capable of activating the MfR at environmentally relevant exposure levels (37).
Potential Targets of Disruption
Alterations in MF levels due to toxicant-mediated effects on biosynthetic or inactivating hormones is a plausible mechanism of endocrine disruption. The herbicide atrazine reportedly increased male offspring production in Daphnia pulicaria (299). However, atrazine did not interact with the MfR (37), suggesting that if atrazine did indeed activate this pathway, it may have been due atrazine increasing endogenous MF. Such an effect may have been the consequence of competition between atrazine and MF at a site of inactivation or elimination. Alternatively, atrazine disrupts endocrine function in mammals by increasing dopamine and reducing NE levels in the hypothalamus (300). These neuromediators are operative in the regulation of MF and sex determination in daphnids (185). Thus, disruption of these upstream signaling components may be responsible for the effects of atrazine on the MF-signaling pathway.
Future Directions of Research on Endocrine Disruption in Crustaceans
In 1998, a workshop was held in the Netherlands to address the issue of endocrine disruption in invertebrates sponsored by The Society of Environmental Toxicology and Chemistry (SETAC). The proceedings of this workshop were subsequently published (301) and made a number of recommendations. Recently, Ford and LeBlanc conducted a survey of experts to reflect on the progress made in endocrine disruptor studies with invertebrates over the past few decades (302). The majority of participants in that survey believed endocrine disruption was an issue for invertebrates that needed to be addressed, but were mixed over the relative progress that had been made. Strikingly, many of the recommendations provided in this recent survey mirrored those made back in 1998 indicating that the field had not significantly progressed.
A major impediment to advancing research on endocrine disruption in crustaceans has been attempts to detect endocrine effects using chemicals known to be endocrine disruptors in vertebrate species along with endpoints known to be indicative of endocrine disruption in vertebrates. This conclusion has recently been acknowledged by several researchers (303, 304). For example, many studies focus upon the effects of estrogens on crustaceans (18) and use biomarkers of feminization, such as vitellogenin induction, in crustaceans (305). Elevated vitellogenin levels following chemical exposure or in field-collected samples has often been interpreted as an estrogenic effect (306). However, unlike vertebrates, vitellogenin is not regulated by estrogens in crustaceans (195). Despite existing knowledge on the endocrine regulation of vitellogenesis in crustaceans, the precise molecular mechanisms by which vitellogenesis and ovarian maturation are controlled require further elucidation (307). Given the lack of clear understanding of seasonal or developmental fluctuations in normal vitellogenin levels and overall susceptibility of vitellogenin production to non-endocrine stressors, associations between altered vitellogenin levels and endocrine disruption in crustaceans are tenuous at best.
One area where progress has been made is the onset of more affordable “omic” technologies allowing for the high-throughput sequencing of genomes, transcriptomes, peptidomes, and metabolomes (19, 139, 304). These omics-techniques offer a rich opportunity for discovering conserved molecular and biochemical pathways, which can applied to the development of adverse outcome pathways (308). These in turn provide opportunities for further advancing our mechanistic understanding of crustacean endocrinology from which to develop appropriate biomarkers of disruption. Zou (139) in a recent review identified over 30 compounds that have either inhibited or stimulated moulting in crustaceans. However, in the absence of mechanistic linkages between exposure and effect, endocrine disruption cannot be invoked as responsible for these effects on moulting. The identification of appropriate biomarkers will facilitate establishing whether an effect on moulting, or some other endocrine-regulated process, is due to specific disruption of the endocrine system or due to some non-specific toxicity.
We hope to have advocated strongly that a “crab is not a fish” and therefore toxicity evaluations using crustaceans require appropriate endpoints to determine whether current and newly licensed chemical compounds might target endocrine processes in this ecologically important group. While significant progress has been made in our understanding of crustacean endocrinology, application of this knowledge to the study of endocrine disruption in crustaceans is lagging. Where, in the past, we were limited in our ability to develop the tools to confirm whether a substance was an endocrine disruptor, these limitations have been largely overcome with affordable omics-technologies. We now have the ability to develop high throughput screenings using key crustacean-relevant endocrine targets. Given the number of crustacean species incorporated into national toxicity programs, such tools are sorely needed.
Author Contributions
TK, GL, and AF have developed the concept of this review and have contributed to the writing. All authors contributed to the article and approved the submitted version.
Funding
This work was financially supported by the European Union through the Interreg France (Channel) England program. TK and AF worked on this article in the framework of the Project “Reduction of Pollution by endocrine disrupting compounds at source: innovative products for the commercial lab market” (RedPol; Interreg 5 a, # 185).
Conflict of Interest
The authors declare that the research was conducted in the absence of any commercial or financial relationships that could be construed as a potential conflict of interest.
Acknowledgments
We gratefully acknowledge H. Dircksen for encouraging us to write this article and T. Monsinjon for help with the bibliography.
Abbreviations
AG, androgenic gland; AGH, androgenic gland hormone; CCAP, crustacean cardioactive peptide; CHH, crustacean hyperglycemic hormone; Cyp19, aromatase; EcR, ecdysone receptor; EDC, endocrine disrupting chemical; EH, eclosion hormone; ETH, ecdysis triggering hormone; FAMeT, farnesoic-O-methyl transferase; HMGCR, 3-hydroxy-3methyl-glutaryl coenzyme A reductase; IAG, insulin-like androgenic gland factor; MET, methoprene tolerant; MF, methyl farnesoate; MfR, methyl farnesoate receptor; MIH, moult inhibiting hormone; NE, norepinephrine; NMDAR, N-methyl-D-aspartate receptor; PCBs, polychlorinated biphenyls; PDH, pigment dispersing hormone; PoA, ponasterone; RPCH, red pigment concentrating hormone; RPCHR, red pigment concentrating hormone receptor; RNAi, RNA interference; RXR, retinoid-X-receptor; SRC, steroid receptor coactivator; US EPA, United States Environmental Protection Agency; XO/SG, X-organ/sinus gland; 5-HT, 5-hydroxytryptamine, serotonin; 20E, 20-hydroxyecdysone.
References
1. Bergman Å, World Health Organization, United Nations Environment Programme. State of the science of endocrine disrupting chemicals - 2012: an assessment of the state of the science of endocrine disruptors prepared by a group of experts for the United Nations Environment Programme and World Health Organization. Genève; Nairobi: WHO; UNEP (2012).
2. Hutchinson TH. Small is useful in endocrine disrupter assessment—four key recommendations for aquatic invertebrate research. Ecotoxicology (2007) 16:231–8. doi: 10.1007/s10646-006-0107-z
3. Song Y, Villeneuve DL, Toyota K, Iguchi T, Tollefsen KE. Ecdysone receptor agonism leading to lethal molting disruption in arthropods: review and adverse outcome pathway development. Environ Sci Technol (2017) 51:4142–57. doi: 10.1021/acs.est.7b00480
4. Martin J, Davis G. Historical trends in crustacean systematics. Crustaceana (2006) 79:1347–68. doi: 10.1163/156854006779277321
6. Ahyong ST. “Evolution and radiation of crustacea,” In: Poore GC, Thiel M, editors. The Natural History of the Crustacea: Evolution and Biogeographyof the Crustacea. USA: Oxford University Press USA. (2020) 8:53–79.
7. Penn JW, Caputi N, de Lestang S, Johnston D, Kangas M, Bopp J. “Crustacean Fisheries,” In: Cochran JK, Bokuniewicz HJ, Yager PL, editors. Encyclopedia of Ocean Sciences (Third Edition). Oxford: Academic Press (2019). p. 324–37. doi: 10.1016/B978-0-12-409548-9.09577-4
8. Reid AJ, Carlson AK, Creed IF, Eliason EJ, Gell PA, Johnson PT. Emerging threats and persistent conservation challenges for freshwater diversity. Biol Rev (2019) 94:849–73. doi: 10.1111/brv.12480
9. Maity S, Jannasch A, Adamec J, Watkins JM, Nalepa T, Höök TO, et al. Elucidating causes of diporeia decline in the Great Lakes via metabolomics: Physiological responses after exposure to different stressors. Physiol Biochem Zool (2013) 86:213–23. doi: 10.1086/669132
10. Auer MT, Auer NA, Urban NR, Auer T. Distribution of the amphipod Diporeia in lake Superior: the ring of fire. J Gt Lakes Res (2013) 39:33–46. doi: 10.1016/j.jglr.2012.12.020
11. Matafonov DV, Bazova NV. Decline of Gammarus lacustris Sars (Crustacea: Amphipoda) population in the delta of the Selenga River. Biol Bull (2014) 41:168–75. doi: 10.1134/S1062359014020071
12. Lipcius RN. Stockhausen WT Concurrent decline of the spawning stock,recruitment, larval abundance, and size of the blue crab Callinectes sapidus inChesapeake Bay. Mar Ecol Prog Ser (2002) 226:45–61. doi:10.3354/meps226045
13. States Greffe. Draft sea fisheries (minimum size limits) (amendment n°7) (Jersey). Ministry of Environment, Sates of Jersey. (2019). https://statesassembly.gov.je/assemblypropositions/2019/p.41-2019.pdf.
14. Moore CG, Stevenson JM. The occurrence of intersexuality in harpacticoid copepods and its relationship with pollution. Mar Pollut Bull (1991) 22:72–4. doi: 10.1016/0025-326X(91)90139-J
15. Ford AT, Fernandes TF, Rider SA, Read PA, Robinson CD, Davies IM. Endocrine disruption in a marine amphipod? Field observations of intersexuality and de-masculinisation. Mar Environ Res (2004) 58:169–73. doi: 10.1016/j.marenvres.2004.03.013
16. Mazurová E, Hilscherová K, Šídlová-Štěpánková T, Köhler H-R, Triebskorn R, Jungmann D, et al. Chronic toxicity of contaminated sediments on reproduction and histopathology of the crustacean Gammarus fossarum and relationship with the chemical contamination and in vitro effects. J Soils Sediments (2010) 10:423–33. doi: 10.1007/s11368-009-0166-x
17. Stentiford GD. Histological intersex (ovotestis) in the European lobsterHomarus gammarus and a commentary on its potential mechanisticbasis. Dis Aquat Organ (2012)100:185–90. doi:10.3354/dao02455
18. Ford AT. Intersexuality in Crustacea: An environmental issue? Aquat Toxicol (2012) 108:125–9. doi: 10.1016/j.aquatox.2011.08.016
19. Short S, Yang G, Guler Y, Green Etxabe A, Kille P, Ford AT. Crustacean intersexuality is feminization without demasculinization: Implications for environmental toxicology. Environ Sci Technol (2014) 48:13520–9. doi: 10.1021/es5050503
20. Baldwin WS, Milam DL, Leblanc GA. Physiological and biochemical perturbations in Daphnia magna following exposure to the model environmental estrogen diethylstilbestrol. Environ Toxicol Chem (1995) 14:945–52. doi: 10.1002/etc.5620140604
21. Zou E, Fingerman M. Effects of estrogenic xenobiotics on molting of the water flea, Daphnia magna. Ecotoxicol Environ Saf (1997) 38:281–5. doi: 10.1006/eesa.1997.1589
22. Bechmann RK. Effect of the endocrine disrupter nonylphenol on the marine copepod Tisbe battagliai. Sci Total Environ (1999) 233:33–46. doi: 10.1016/S0048-9697(99)00177-1
23. Breitholtz M, Bengtsson B-E. Oestrogens have no hormonal effect on the development and reproduction of the harpacticoid copepod Nitocra spinipes. Mar Pollut Bull (2001) 42:879–86. doi: 10.1016/S0025-326X(01)00046-7
24. Vandenbergh GF, Adriaens D, Verslycke T, Janssen CR. Effects of 17α-ethinylestradiol on sexual development of the amphipod Hyalella azteca. Ecotoxicol Environ Saf (2003) 54:216–22. doi: 10.1016/S0147-6513(02)00030-1
25. Lye CM, Bentley MG, Clare AS, Sefton EM. Endocrine disruption in the shore crab Carcinusmaenas—a biomarker for benthic marine invertebrates? Mar EcolProg Ser (2005) 288:221–32.doi: 10.3354/meps288221
26. Gunamalai V, Kirubagaran R, Subramoniam T. Vertebrate steroids and the control of female reproduction in two decapod crustaceans, Emerita asiatica and Macrobrachium rosenbergii. Curr Sci (2006) 90:119–23.
27. Köhler H-R, Kloas W, Schirling M, Lutz I, Reye AL, Langen J-S, et al. Sex steroid receptor evolution and signalling in aquatic invertebrates. Ecotoxicology (2007) 16:131–43. doi: 10.1007/s10646-006-0111-3
28. Li GL, Chen HP, Deng SP, Ye M, Jiang S, Chan SF, et al. In vivo and in vitro inhibitory action of 17β-estradiol and environmental estrogen. Genet Mol Res (2015) 14:14056–65. doi: 10.4238/2015.October.29.25
29. Lu Y, Liu M, Gong J, Cheng Y, Wu X. Effect of exogenous estrogen on the ovarian development and gene expression in the female swimming crab Portunus trituberculatus (Miers, 1876) (Decapoda: Brachyura: Portunidae). J Crustac Biol (2018) 38:367–73. doi: 10.1093/jcbiol/ruy013
30. Gismondi E. Identification of molt-inhibiting hormone and ecdysteroid receptor cDNA sequences in Gammarus pulex, and variations after endocrine disruptor exposures. Ecotoxicol Environ Saf (2018) 158:9–17. doi: 10.1016/j.ecoenv.2018.04.017
31. Segner H, Caroll K, Fenske M, Janssen CR, Maack G, Pascoe D, et al. Identification of endocrine-disrupting effects in aquatic vertebrates and invertebrates: report from the European IDEA project. Ecotoxicol Environ Saf (2003) 54:302–14. doi: 10.1016/S0147-6513(02)00039-8
32. LeBlanc GA. Crustacean endocrine toxicology: a review. Ecotoxicology (2007) 16:61–81. doi: 10.1007/s10646-006-0115-z
33. De Wilde R, Swevers L, Soin T, Christiaens O, Rougé P, Cooreman K, et al. Cloning and functional analysis of the ecdysteroid receptor complex in the opossum shrimp Neomysis integer (Leach, 1814). Aquat Toxicol (2013) 130–131:31–40. doi: 10.1016/j.aquatox.2012.12.011
34. Chan YH, Chu KH, Chan KM. Ecdysteroid-mimicking compounds act as both agonists and antagonists to the crustacean ecdysone receptor. Chemosphere (2019) 237:124551. doi: 10.1016/j.chemosphere.2019.124551
35. Abe R, Watanabe H, Yamamuro M, Iguchi T, Tatarazako N. Establishment of a short-term, in vivo screening method for detecting chemicals with juvenile hormone activity using adult Daphnia magna. J Appl Toxicol (2015) 35:75–82. doi: 10.1002/jat.2989
36. Lafontaine A, Gismondi E, Boulangé-Lecomte C, Geraudie P, Dodet N, Caupos F, et al. Effects of chlordecone on 20-hydroxyecdysone concentration and chitobiase activity in a decapod crustacean, Macrobrachium rosenbergii. Aquat Toxicol (2016) 176:53–63. doi: 10.1016/j.aquatox.2016.04.006
37. Medlock Kakaley EK, Eytcheson SA, LeBlanc GA. Ligand-mediated receptor assembly as an end point for high-throughput chemical toxicity screening. Environ Sci Technol (2017) 51:9327–33. doi: 10.1021/acs.est.7b02882
38. Song Y, Evenseth LM, Iguchi T, Tollefsen KE. Release of chitobiase as an indicator of potential molting disruption in juvenile Daphnia magna exposed to the ecdysone receptor agonist 20-hydroxyecdysone. J Toxicol Environ Health A (2017) 80:954–62. doi: 10.1080/15287394.2017.1352215
39. Bonett RM. Analyzing endocrine system conservation and evolution. Gen Comp Endocrinol (2016) 234:3–9. doi: 10.1016/j.ygcen.2016.03.011
41. Loof AD, Lindemans M, Liu F, Groef BD, Schoofs L. Endocrine archeology: Do insects retain ancestrally inherited counterparts of the vertebrate releasing hormones GnRH, GHRH, TRH, and CRF? Gen Comp Endocrinol (2012) 177:18–27. doi: 10.1016/j.ygcen.2012.02.002
42. Marco HG. Unity and diversity in chemical signals of arthropods: the role of neuropeptides in crustaceans and insects. Int Congr Ser (2004) 1275:126–33. doi: 10.1016/j.ics.2004.08.053
43. Hartenstein V. The neuroendocrine system of invertebrates: a developmental and evolutionary perspective. J Endocrinol (2006) 190:555–70. doi: 10.1677/joe.1.06964
44. Elphick MR, Mirabeau O, Larhammar D. Evolution of neuropeptide signalling systems. J Exp Biol (2018) 221:jeb151092. doi: 10.1242/jeb.151092
45. Ayala FJ, Rzhetsky A, Ayala FJ. Origin of the metazoan phyla: Molecular clocks confirm paleontological estimates. Proc Natl Acad Sci (1998) 95:606. doi: 10.1073/pnas.95.2.606
46. Markov GV, Tavares R, Dauphin-Villemant C, Demeneix BA, Baker ME, Laudet V. Independent elaboration of steroid hormone signaling pathways in metazoans. Proc Natl Acad Sci USA (2009) 106:11913–8. doi: 10.1073/pnas.0812138106
47. Jegla T. Evidence for ecdysteroids as molting hormones in Chelicerata, Crustacea, and Myriapoda. In: Gupta A, editor. “Morphogenetic Hormones of Arthropods”. London: Rutgers Univ. Press (1990).
48. Defelipe LA, Dolghih E, Roitberg AE, Nouzova M, Mayoral JG, Noriega FG, et al. Juvenile hormone synthesis: “esterify then epoxidize” or “epoxidize then esterify”? Insights from the structural characterization of juvenile hormone acid methyltransferase. Insect Biochem Mol Biol (2011) 41:228–35. doi: 10.1016/j.ibmb.2010.12.008
49. Mercier J, Doucet D, Retnakaran A. Molecular physiology of crustacean and insect neuropeptides. J Pestic Sci (2007) 32:345–59. doi: 10.1584/jpestics.R07-04
50. Webster SG, Keller R, Dircksen H. The CHH-superfamily of multifunctional peptide hormones controlling crustacean metabolism, osmoregulation, moulting, and reproduction. Gen Comp Endocrinol (2012) 175:217–33. doi: 10.1016/j.ygcen.2011.11.035
51. Dircksen H. Insect ion transport peptides are derived from alternatively spliced genes and differentially expressed in the central and peripheral nervous system. J Exp Biol (2009) 212:401–12. doi: 10.1242/jeb.026112
52. Gáliková M, Dircksen H, Nässel DR. The thirsty fly: Ion transport peptide (ITP) is a novel endocrine regulator of water homeostasis in Drosophila. PloS Genet (2018) 14:e1007618. doi: 10.1371/journal.pgen.1007618
53. Sandve SR, Rohlfs RV, Hvidsten TR. Subfunctionalization versus neofunctionalization after whole-genome duplication. Nat Genet (2018) 50:908–9. doi: 10.1038/s41588-018-0162-4
54. Thornton JW, Need E, Crews D. Resurrecting the ancestral steroid receptor: ancient origin of estrogen signaling. Science (2003) 301:1714–7. doi: 10.1126/science.1086185
55. Escriva H, Bertrand S, Laudet V. The evolution of the nuclear receptor superfamily. Essays Biochem (2004) 40:11–26. doi: 10.1042/bse0400011
56. Bertrand S, Brunet FG, Escriva H, Parmentier G, Laudet V, Robinson-Rechavi M. Evolutionary genomics of nuclear receptors: from twenty-five ancestral genes to derived endocrine systems. Mol Biol Evol (2004) 21:1923–37. doi: 10.1093/molbev/msh200
57. Thomson SA, Baldwin WS, Wang YH, Kwon G, LeBlanc GA. Annotation, phylogenetics, and expression of the nuclear receptors in Daphnia pulex. BMC Genomics (2009) 10:500. doi: 10.1186/1471-2164-10-500
58. Campbell RK, Satoh N, Degnan BM. Piecing together evolution of the vertebrate endocrine system. Trends Genet (2004) 20:359–66. doi: 10.1016/j.tig.2004.06.005
59. Baker ME. Evolution of adrenal and sex steroid action in vertebrates: a ligand-based mechanism for complexity. BioEssays News Rev Mol Cell Dev Biol (2003) 25:396–400. doi: 10.1002/bies.10252
60. Baker ME. Trichoplax, the simplest known animal, contains an estrogen-related receptor but no estrogen receptor: Implications for estrogen receptor evolution. Biochem Biophys Res Commun (2008) 375:623–7. doi: 10.1016/j.bbrc.2008.08.047
61. Bridgham JT, Brown JE, Rodríguez-Marí A, Catchen JM, Thornton JW. Evolution of a new function by degenerative mutation in cephalochordate steroid receptors. PloS Genet (2008) 4:e1000191. doi: 10.1371/journal.pgen.1000191
62. Baker ME. Steroid receptors and vertebrate evolution. Mol Cell Endocrinol (2019) 496:110526. doi: 10.1016/j.mce.2019.110526
63. Bonneton F, Zelus D, Iwema T, Robinson-Rechavi M, Laudet V. Rapid divergence of the ecdysone receptor in Diptera and Lepidoptera suggests coevolution between ECR and USP-RXR. Mol Biol Evol (2003) 20:541–53. doi: 10.1093/molbev/msg054
64. Grillo M, Casanova J, Averof M. Development: a deep breath for endocrine organ evolution. Curr Biol (2014) 24:R38–40. doi: 10.1016/j.cub.2013.11.033
65. Sánchez-Higueras C, Sotillos S, Castelli-Gair Hombría J. Common origin of insect trachea and endocrine organs from a segmentally repeated precursor. Curr Biol (2014) 24:76–81. doi: 10.1016/j.cub.2013.11.010
66. Siveter DJ, Williams M, Waloszek D. A phosphatocopid crustacean with appendages from the lower Cambrian. Science (2001) 293:479–81. doi: 10.1126/science.1061697
67. Han J, Morris SC, Ou Q, Shu D, Huang H. Meiofaunal deuterostomes from the basal Cambrian of Shaanxi (China). Nature (2017) 542:228–31. doi: 10.1038/nature21072
68. Richter S, Wirkner CS. What the ur-crustacean lookedlike. Poore G, Thiel M (eds). Evolution and Biogeography (Volume 8 in the Series The Natural History of the Crustacea). Oxford University Press (2020). pp. 1–20.
69. Skinner DM, Graham DE, Holland CH, Mykles DL, Soumoff C, Yamaoka LH. “Control of molting incrustacea,” In: Wenner AM. Crustacean Issues 3: factors in adult growth. Rotterdam: A.A. Balkema Publishers (1985). p. 3–14. doi: 10.1201/9781315140681
70. Techa S, Chung JS. Ecdysteroids regulate the levels of Molt-Inhibiting Hormone (MIH) expression in the blue crab, Callinectes sapidus. PloS One (2015) 10:e0117278. doi: 10.1371/journal.pone.0117278
71. Nagaraju GPC, Reddy PR, Reddy PS. In vitro methyl farnesoate secretion by mandibular organs isolated from different molt and reproductive stages of the crab Oziotelphusa senex senex. Fish Sci (2006) 72:410–4. doi: 10.1111/j.1444-2906.2006.01164.x
72. Chang ES, Mykles DL. Regulation of crustacean molting: A review and our perspectives. Gen Comp Endocrinol (2011) 172:323–30. doi: 10.1016/j.ygcen.2011.04.003
73. Chung JS. Hemolymph ecdysteroids during the last three molt cycles of the blue crab, Callinectes sapidus: quantitative and qualitative analyses and regulation. Arch Insect Biochem Physiol (2010) 73:1–13. doi: 10.1002/arch.20327
74. Chung JS, Dircksen H. Webster SG. A remarkable, precisely timed release of hyperglycemic hormone from endocrine cells in the gut is associated with ecdysis in the crab Carcinus maenas. Proc Natl Acad Sci USA (1999) 96:13103–7. doi: 10.1073/pnas.96.23.13103
75. Phlippen MK, Webster SG, Chung JS, Dircksen H. Ecdysis of decapod crustaceans is associated with a dramatic release of crustacean cardioactive peptide into the haemolymph. J Exp Biol (2000) 203:521–36.
76. Webster SG, Wilcockson DC, Mrinalini, Sharp JH. Bursicon and neuropeptide cascades during the ecdysis program of the shore crab, Carcinus maenas. Gen Comp Endocrinol (2013) 182:54–64. doi: 10.1016/j.ygcen.2012.11.018
77. Drach P. Mue et cycle d’intermue chez les crustaces decapodes. Ann Inst Ocean Monaco (1939) 19:103–391.
78. Kuballa A, Elizur A. Novel molecular approach to study moulting in crustaceans. Bull-Fish Res Agency Jpn (2007) 20:53.
79. Liu L, Laufer H. Isolation and characterization of sinus gland neuropeptides with both mandibular organ inhibiting and hyperglycemic effects from the spider crab Libinia emarginata. Arch Insect Biochem Physiol (1996) 32:375–85. doi: 10.1002/(SICI)1520-6327(1996)32:3/4<375::AID-ARCH10>3.0.CO;2-9
80. Martin-Creuzburg D, Westerlund SA, Hoffmann KH. Ecdysteroid levels in Daphnia magna during a molt cycle: Determination by radioimmunoassay (RIA) and liquid chromatography–mass spectrometry (LC–MS). Gen Comp Endocrinol (2007) 151:66–71. doi: 10.1016/j.ygcen.2006.11.015
81. Sumiya E, Ogino Y, Miyakawa H, Hiruta C, Toyota K, Miyagawa S, et al. Roles of ecdysteroids for progression of reproductive cycle in the fresh water crustacean Daphnia magna. Front Zool (2014) 11:60. doi: 10.1186/s12983-014-0060-2
82. Drach P, Tchernigovtzeff C. Sur la méthode de détermination des stades d’intermue et son application générale aux crustacés. Vie Milieu (1967) 18A:595–610.
83. Alexander JL, Oliphant A, Wilcockson DC, Audsley N, Down RE, Lafont R, et al. Functional characterization and signaling systems of corazonin and red pigment concentrating hormone in the green shore crab, Carcinus maenas. Front Neurosci (2018) 11:752. doi: 10.3389/fnins.2017.00752
84. Passano L. Neurosecretory control of molting in crabs by the X-organ sinus gland complex. Physiol Comp Oecol (1953) 3:5–1.
85. Brown FA. Hormones in the crustacea: Their sources and activities. Q Rev Biol (1944) 19:32–46. doi: 10.1086/394686
86. Knowles SFGW, Carlisle DB. Endocrine control in the crustacea. Biol Rev (1956) 31:396–467. doi: 10.1111/j.1469-185X.1956.tb01556.x
87. Webster SG, Keller R. Purification, characterisation and amino acid composition of the putative moult-inhibiting hormone (MIH) of Carcinus maenas (Crustacea, Decapoda). J Comp Physiol B (1986) 156:617–24. doi: 10.1007/BF00692738
88. Chung JS, Webster SG. Moult cycle-related changes in biological activity of moult-inhibiting hormone (MIH) and crustacean hyperglycaemic hormone (CHH) in the crab, Carcinus maenas. From target to transcript. Eur J Biochem (2003) 270:3280–8. doi: 10.1046/j.1432-1033.2003.03720.x
89. Chung JS, Webster SG. Dynamics of in vivo release of molt-inhibiting hormone and crustacean hyperglycemic hormone in the shore crab, Carcinus maenas. Endocrinology (2005) 146:5545–51. doi: 10.1210/en.2005-0859
90. Nakatsuji T, Keino H, Tamura K, Yoshimura S, Kawakami T, Aimoto S, et al. Changes in the amounts of the Molt-Inhibiting Hormone in sinus glands during the molt cycle of the American crayfish, Procambarus clarkii. Zoolog Sci (2000) 17:1129–36. doi: 10.2108/zsj.17.1129
91. Watson RD, Lee KJ, Shihong Q, Ming L, Heidi RU, Robert DR, et al. Molecular cloning, expression, and tissue distribution of crustacean Molt-Inhibiting Hormone. Am Zool (2001) 41:407–17. doi: 10.1093/icb/41.3.407
92. Robert A, Monsinjon T, Delbecque J-P, Olivier S, Poret A, Foll FL, et al. Neuroendocrine disruption in the shore crab Carcinus maenas: Effects of serotonin and fluoxetine on chh- and mih-gene expression, glycaemia and ecdysteroid levels. Aquat Toxicol (2016) 175:192–204. doi: 10.1016/j.aquatox.2016.03.025
93. Sefiani M, Caer J-PL, Soyez D. Characterization of hyperglycemic and molt-inhibiting activity from sinus glands of the penaeid shrimp, Penaeus vannamei. Gen Comp Endocrinol (1996) 103:41–53. doi: 10.1006/gcen.1996.0092
94. Nakatsuji T, Sonobe H. Regulation of ecdysteroid secretion from the Y-organ by molt-inhibiting hormone in the American crayfish, Procambarus clarkii. Gen Comp Endocrinol (2004) 135:358–64. doi: 10.1016/j.ygcen.2003.11.001
95. Chung JS, Katayama H, Dircksen H. New functions of arthropod bursicon: inducing deposition and thickening of new cuticle and hemocyte granulation in the blue crab, Callinectes sapidus. PloS One (2012) 7(9):e46299. doi: 10.1371/journal.pone.0046299
96. Christie AE, McCoole MD, Harmon SM, Baer KN, Lenz PH. Genomic analyses of the Daphnia pulex peptidome. Gen Comp Endocrinol (2011) 171:131–50. doi: 10.1016/j.ygcen.2011.01.002
97. Dircksen H, Neupert S, Predel R, Verleyen P, Huybrechts J, Strauss J, et al. Genomics, transcriptomics, and peptidomics of Daphnia pulex neuropeptides and protein hormones. J Proteome Res (2011) 10:4478–504. doi: 10.1021/pr200284e
98. Oliphant A, Alexander JL, Swain MT, Webster SG, Wilcockson DC. Transcriptomic analysis of crustacean neuropeptide signaling during the moult cycle in the green shore crab, Carcinus maenas. BMC Genomics (2018) 19:711. doi: 10.1186/s12864-018-5057-3
99. Nhut TM, Mykles DL, Elizur A, Ventura T. Ecdysis triggering hormone modulates molt behaviour in the redclawcrayfish Cherax quadricarinatus, providing a mechanistic evidence for conservedfunction in molt regulation across Pancrustacea. Gen Comp Endocrinol (2020) 298:113556. 10.1016/j.ygcen.2020.113556
100. Zhou L, Li S, Wang Z, Li F, Xiang J. An eclosion hormone-like gene participates in the molting process of Palaemonid shrimp Exopalaemon carinicauda. Dev Genes Evol (2017) 227:189–99. 10.1007/s00427-017-0580-9
101. Hopkins PM. “Crustacean ecdysteroids and their receptors,” In: Smagghe G, editor. Ecdysone: Structures and Functions. Dordrecht: Springer Netherlands (2009). p. 73–97. doi: 10.1007/978-1-4020-9112-4_3
102. Soumoff C, Skinner DM. Ecdysteroid titers during the molt cycle of the blue crab resemble those of other crustacea. Biol Bull (1983) 165:321–9. doi: 10.2307/1541372
103. Mykles DL. Ecdysteroid metabolism in crustaceans. J Steroid Biochem Mol Biol (2011) 127:196–203. doi: 10.1016/j.jsbmb.2010.09.001
104. Schumann I, Kenny N, Hui J, Hering L, Mayer G. Halloween genes in panarthropods and the evolution of the early moulting pathway in ecdysozoa. R Soc Open Sci (2018) 5:180888. doi: 10.1098/rsos.180888
105. Niwa R, Niwa YS. Enzymes for ecdysteroid biosynthesis: their biological functions in insects and beyond. Biosci Biotechnol Biochem (2014) 78:1283–92. doi: 10.1080/09168451.2014.942250
106. Hosamani N, Reddy S, Reddy R. Crustacean molting: regulation and effects of environmentaltoxicants. J Mar Sci Res Dev (2017) 7:2. 10.4172/2155-9910.1000236
107. Rewitz KF, Gilbert LI. Daphnia Halloween genes that encode cytochrome P450s mediating the synthesis of the arthropod molting hormone: Evolutionary implications. BMC Evol Biol (2008) 8:60. doi: 10.1186/1471-2148-8-60
108. Niwa R, Namiki T, Ito K, Shimada-Niwa Y, Kiuchi M, Kawaoka S, et al. Non-molting glossy shroud encodes a short-chain dehydrogenase/reductase that functions in the ‘Black Box’ of the ecdysteroid biosynthesis pathway. Development (2010) 137:1991. doi: 10.1242/dev.045641
109. Sumiya E, Ogino Y, Toyota K, Miyakawa H, Miyagawa S, Iguchi T. Neverland regulates embryonic moltings through the regulation of ecdysteroid synthesis in the water flea Daphnia magna, and may thus act as a target for chemical disruption of molting. J Appl Toxicol (2016) 36:1476–85. doi: 10.1002/jat.3306
110. Fontana E, Dansette PM, Poli SM. Cytochrome p450 enzymes mechanism based inhibitors: common sub-structures and reactivity. Curr Drug Metab (2005) 6:413–54. doi: 10.2174/138920005774330639
111. Hassold E, Backhaus T. Chronic toxicity of five structurally diverse demethylase-inhibiting fungicides to the crustacean Daphnia magna: A comparative assessment. Environ Toxicol Chem (2009) 28:1218–26. doi: 10.1897/08-339.1
112. Kast-Hutcheson K, Rider CV, LeBlanc GA. The fungicide propiconazole interferes with embryonic development of the crustacean Daphnia magna. Environ Toxicol Chem (2001) 20:502–9. doi: 10.1002/etc.5620200308
113. Mu X, LeBlanc GA. Environmental antiecdysteroids alter embryo development in the crustacean Daphnia magna. J Exp Zool (2002) 292:287–92. doi: 10.1002/jez.10020
114. Bonneton F, Chaumot A, Laudet V. Annotation of Tribolium nuclear receptors reveals an increase in evolutionary rate of a network controlling the ecdysone cascade. Insect Biochem Mol Biol (2008) 38:416–29. doi: 10.1016/j.ibmb.2007.10.006
115. Hannas BR, LeBlanc GA. Expression and ecdysteroid responsiveness of the nuclear receptors HR3 and E75 in the crustacean Daphnia magna. Mol Cell Endocrinol (2010) 315:208–18. doi: 10.1016/j.mce.2009.07.013
116. Riddiford LM, Cherbas P, Truman JW. Ecdysone receptors and their biological actions. Vitam Horm (2000) 60:1–73. doi: 10.1016/S0083-6729(00)60016-X
117. Hill RJ, Billas IML, Bonneton F, Graham LD, Lawrence MC. Ecdysone receptors: From the Ashburner model to structural biology. Annu Rev Entomol (2013) 58:251–71. doi: 10.1146/annurev-ento-120811-153610
118. Baehrecke EH. Ecdysone signaling cascade and regulation of Drosophilametamorphosis. Arch Insect Biochem Physiol (1996) 33:231–44. doi: 10.1002/(SICI)1520-6327(1996)33:3/4<231::AID-ARCH5>3.0.CO;2-V
119. Belles X, Piulachs M-D. Ecdysone signalling and ovarian development in insects: from stem cells to ovarian follicle formation. Biochim Biophys Acta (2015) 1849:181–6. doi: 10.1016/j.bbagrm.2014.05.025
120. Gong J, Ye H, Xie Y, Yang Y, Huang H, Li S, et al. Ecdysone receptor in the mud crab Scylla paramamosain: a possible role in promoting ovarian development. J Endocrinol (2015) 224:273–87. doi: 10.1530/JOE-14-0526
121. Yao T-P, Forman BM, Jiang Z, Cherbas L, Chen J-D, McKeown M, et al. Functional ecdysone receptor is the product of EcR and Ultraspiraclegenes. Nature (1993) 366:476–9. doi: 10.1038/366476a0
122. Billas IML, Iwema T, Garnier J-M, Mitschler A, Rochel N, Moras D. Structural adaptability in the ligand-binding pocket of the ecdysone hormone receptor. Nature (2003) 426:91–6. doi: 10.1038/nature02112
123. Hu X, Cherbas L, Cherbas P. Transcription activation by the Ecdysone Receptor (EcR/USP): Identification of activation functions. Mol Endocrinol (2003) 17:716–31. doi: 10.1210/me.2002-0287
124. Li J, Sun J, Dong X, Geng X, Qiu G. Transcriptomic analysis of gills provides insights into the molecular basis of molting in Chinese mitten crab (Eriocheir sinensis). PeerJ (2019) 7:e7182–2. doi: 10.7717/peerj.7182
125. Zou E, Fingerman M. Chitobiase activity in the epidermis and hepatopancreas of the fiddler crab Uca pugilator during the molting cycle. Mar Biol (1999) 133:97–101. doi: 10.1007/s002270050447
126. Meng Y, Zou E. Impacts of molt-inhibiting organochlorine compounds on epidermal ecdysteroid signaling in the fiddler crab, Uca pugilator, in vitro. Comp Biochem Physiol Part C Toxicol Pharmacol (2009) 150:436–41. doi: 10.1016/j.cbpc.2009.06.009
127. Qian Z, He S, Liu T, Liu Y, Hou F, Liu Q, et al. Identification of ecdysteroid signaling late-response genes from different tissues of the Pacific white shrimp, Litopenaeus vannamei. Comp Biochem Physiol A Mol Integr Physiol (2014) 172:10–30. doi: 10.1016/j.cbpa.2014.02.011
128. Espie PJ, Roff JC. A biochemical index of duration of the molt cycle for planktonic crustacea based on the chitin-degrading enzyme, chitobiase. Limnol Oceanogr (1995) 40:1028–34. doi: 10.4319/lo.1995.40.6.1028
129. Yoon D-S, Park JC, Park HG, Lee J-S, Han J. Effects of atrazine on life parameters, oxidative stress, and ecdysteroid biosynthetic pathway in the marine copepod Tigriopus japonicus. Aquat Toxicol (2019) 213:105213. doi: 10.1016/j.aquatox.2019.05.015
130. Chen H, Gu X, Zeng Q, Mao Z, Liang X, Martyniuk CJ. Carbamazepine disrupts molting hormone signaling and inhibits molting and growth of Eriocheir sinensis at environmentally relevant concentrations. Aquat Toxicol (2019) 208:138–45. doi: 10.1016/j.aquatox.2019.01.010
131. Dinan L, Whiting P, Girault J-P, Lafont R, Dhadialla ST, Cress ED, et al. Cucurbitacins are insect steroid hormone antagonists acting at the ecdysteroid receptor. Biochem J (1997) 327:643–50. doi: 10.1042/bj3270643
132. Dhadialla TS, Carlson GR, Le DP. New insecticides with ecdysteroidal and juvenile hormone activity. Annu Rev Entomol (1998) 43:545–69. doi: 10.1146/annurev.ento.43.1.545
133. Kato Y, Kobayashi K, Oda S, Tatarazako N, Watanabe H, Iguchi T. Cloning and characterization of the ecdysone receptor and ultraspiracle protein from the water flea Daphnia magna. J Endocrinol (2007) 193:183–94. doi: 10.1677/JOE-06-0228
134. Mellor CL, Tollefsen KE, LaLone C, Cronin MTD, Firman JW. In silico identification of chemicals capable of binding to the ecdysone receptor. Environ Toxicol Chem (2020) 39:1438–50. doi: 10.1002/etc.4733
135. Dinan L, Bourne P, Whiting P, Dhadialla TS, Hutchinson TH. Screening of environmental contaminants for ecdysteroid agonist and antagonist activity using the Drosophila melanogaster BII cell in vitro assay. Environ Toxicol Chem (2001) 20:2038–46. doi: 10.1002/etc.5620200924
136. Mu X, LeBlanc GA. Developmental toxicity of testosterone in the crustacean Daphnia magna involves anti-ecdysteroidal activity. Gen Comp Endocrinol (2002) 129:127–33. doi: 10.1016/S0016-6480(02)00518-X
137. de Melo MS, Nazari EM, Joaquim-Justo C, Muller YMR, Gismondi E. Effects of low glyphosate-based herbicide concentrations on endocrine-related gene expression in the decapoda Macrobrachium potiuna. Environ Sci Pollut Res (2019) 26:21535–45. doi: 10.1007/s11356-019-05496-1
138. Park K, Kim W-S, Kwak I-S. Characterization and transcriptional response of ecdysone receptor gene in the mud crab Macrophthalmus japonicus: Effects of osmotic stress and endocrine disrupting chemicals. Ocean Sci J (2019) 54:611–20. doi: 10.1007/s12601-019-0029-3
139. Zou E. Invisible endocrine disruption and its mechanisms: A current review. Gen Comp Endocrinol (2020) 293:113470. doi: 10.1016/j.ygcen.2020.113470
140. Keller R, Beyer J. Zur hyperglykämischen Wirkung von Serotonin und Augenstielextrakt beim Flusskrebs Orconectes limosus. Z Vgl Physiol (1968)59:78–85. doi: 10.1007/BF00298812
141. Lee CY, Yang PF, Zou HS. Serotonergic regulation of crustacean hyperglycemic hormone secretion in the crayfish, Procambarus clarkii. Physiol Biochem Zool (2001) 74:376–82. doi: 10.1086/320430
142. Alvarado-Alvarez R, Aréchiga H, García U. Serotonin activates a Ca(2+)-dependent K(+) current in identified peptidergic neurons from the crayfish. J Exp Biol (2000) 203:715–23.
143. Sáenz F, García U, Aréchiga U. Modulation of electrical activity by 5-hydroxytryptamine in crayfish neurosecretory cells. J Exp Biol (1997) 200:3079–90.
144. Webster SG. High-affinity binding of putative moult-inhibiting hormone (MIH) and crustacean hyperglycaemic hormone (CHH) to membrane-bound receptors on the Y-organ of the shore crab Carcinus maenus. Proc R Soc Lond B Biol Sci (1993) 251:53–9. doi: 10.1098/rspb.1993.0008
145. LeBlanc GA, McLachlan JB. Molt-independent growth inhibition of Daphnia magna by a vertebrate antiandrogen. Environ Toxicol Chem (1999) 18:1450–5. doi: 10.1002/etc.5620180715
146. DeLoof A, DeClerk D, Andriesm JC, Dhainaut A. Vertebrate-type steroids in arthropods: Identification, concentrations and possible functions. In: Porchet M, editor. “Advances in Invertebrate Reproduction”, vol. 4. Amsterdam: Elsevier (1986).
147. Jeng SS, Wan WC-M, Chang CF. Existence of an estrogen-like compound in the ovary of the shrimp Parapenaeus fissurus. Gen Comp Endocrinol (1978) 36:211–4. doi: 10.1016/0016-6480(78)90024-2
148. Lewis SE, Freund JG, Riddell GE, Wankowski JL, Dick JTA, Baldridge MG. Interspecific comparison of estrogen and testosterone concentrations in three species of amphipods (Gammarus duebeni celticus, G. pseudolimnaeus, and G. pulex). J Crustac Biol (2015) 35:789–92. doi: 10.1163/1937240X-00002379
149. Mackevieciene G, Chibisova N. Concentration of steroid hormones at different stages of the intermoult cycle in the crayfish Astacus astacus and Pacifastacus leniusculus. Freshw Crayfish (1995) 10:267–73.
150. Novak F, Van Beek E, Lambert J, De Loof A. Pregnenolone and estradiol identification in the brine shrimp,Artemia sp., by means of gas chromatographical-mass spectrometricalanalysis. Comp Biochem Physiol Part B Comp Biochem (1990) 95:565–9. 10.1016/0305-0491(90)90022-L
151. Merlin JR, Mohanlal DL, Balasubramanian CP, Vijayan KK, Subramoniam T, Tomy S, et al. Vertebrate-type steroid profile in different tissues of wild and endocrinologically manipulated female brood stocks of Penaeus monodon. Curr Sci (2016) 111:1194–200.
152. Subramoniam T. Steroidal control of vitellogenesis in Crustacea: a newunderstanding for improving shrimp hatchery production. Proc Indian Natl SciAcad (2017) 83:595–610. 10.16943/ptinsa/2017/48969
153. Young NJ, Quinlan PT, Goad LJ. Progesterone metabolism in vitro in the decapod crustacean, Penaeus monodon. Gen Comp Endocrinol (1992) 87:300–11. doi: 10.1016/0016-6480(92)90035-I
154. Burns BG, Sangalang GB, Freeman HC, McMenemy M. Bioconversion of steroids by the testes of the American lobster, Homarus americanus, in vitro. Gen Comp Endocrinol (1984) 54:422–8. doi: 10.1016/0016-6480(84)90157-6
155. Baldwin WS, LeBlanc GA. In vivo biotransformation of testosterone by phase I and II detoxication enzymes and their modulation by 20-hydroxyecdysone in Daphnia magna. Aquat Toxicol (1994) 29:103–17. doi: 10.1016/0166-445X(94)90051-5
156. Depledge MH, Billinghurst Z. Ecological significance of endocrine disruption in marine invertebrates. Mar Pollut Bull (1999) 39:32–8. doi: 10.1016/S0025-326X(99)00115-0
157. Coccia E, Lisa ED, Cristo CD, Cosmo AD, Paolucci M. Effects of estradiol and progesterone on the reproduction of the freshwater crayfish Cherax albidus. Biol Bull (2010) 218:36–47. doi: 10.1086/BBLv218n1p36
158. Zhang Z, Chen K, Shih JC, Teng CT. Estrogen-related receptors-stimulated monoamine oxidase B promoter activity is down-regulated by estrogen receptors. Mol Endocrinol (2006) 20:1547–61. doi: 10.1210/me.2005-0252
159. Chakravorty SG, Halbreich U. The influence of estrogen on monoamine oxidase activity. Psychopharmacol Bull (1997) 33:229–33.
160. Ma Z-Q, Violani E, Villa F, Picotti GB, Maggi A. Estrogenic control of monoamine oxidase A activity in human neuroblastoma cells expressing physiological concentrations of estrogen receptor. Eur J Pharmacol (1995) 284:171–6. doi: 10.1016/0014-2999(95)00387-Z
161. Jordão R, Campos B, Piña B, Tauler R, Soares AMVM, Barata C. Mechanisms of action of compounds that enhance storage lipid accumulation in Daphnia magna. Environ Sci Technol (2016) 50:13565–73. doi: 10.1021/acs.est.6b04768
162. Zou E, Fingerman M. Synthetic estrogenic agents do not interfere with sex differentiation but do inhibit molting of the cladoceran Daphnia magna. Bull Environ Contam Toxicol (1997) 58:596–602. doi: 10.1007/s001289900376
163. Watermann BT, Albanis TA, Galassi S, Gnass K, Kusk KO, Sakkas VA, et al. Effects of anti-androgens cyproterone acetate, linuron, vinclozolin, and p,p’-DDE on the reproductive organs of the copepod Acartia tonsa. J Environ Sci Health Part A (2016) 51:1111–20. doi: 10.1080/10934529.2016.1199769
164. Reddy PS, Devi M, Sarojini R, Nagabhushanam R, Fingerman M. Cadmium chloride induced hyperglycemia in the red swamp crayfish, Procambarus clarkii: Possible role of crustacean hyperglycemic hormone. Comp Biochem Physiol C Pharmacol Toxicol Endocrinol (1994) 107:57–61. doi: 10.1016/1367-8280(94)90010-8
165. Reddy PS, Fingerman M. Effect of cadmium chloride on physiological color changes of thefiddler crab. Uca Pugilator Ecotoxicol Environ Saf (1995) 31:69–75. doi: 10.1006/eesa.1995.1045
166. Reddy PS, Nguyen LK, Obih P, Fingerman M. Effect of cadmium chloride on the distal retinal pigment cells of the fiddler crab, Uca pugilator. Bull Environ Contam Toxicol (1997) 58:504–10. doi: 10.1007/s001289900363
167. Reddy PS, Tuberty SR, Fingerman M. Effects of cadmium and mercury on ovarian maturation in the red swamp crayfish, Procambarus clarkii. Ecotoxicol Environ Saf (1997) 37:62–5. doi: 10.1006/eesa.1997.1523
168. Rodríguez EM, Greco LSL, Fingerman M. Inhibition of ovarian growth by cadmium in the fiddler crab, Uca pugilator (Decapoda, Ocypodidae). Ecotoxicol Environ Saf (2000) 46:202–6. doi: 10.1006/eesa.1999.1896
169. Medesani DA, Greco LSL, Rodrıguez EM. Interference of cadmium and copper with the endocrine control of ovarian growth, in the estuarine crab Chasmagnathus granulata. Aquat Toxicol (2004) 69:165–74. doi: 10.1016/j.aquatox.2004.05.003
170. Lorenzon S, Edomi P, Giulianini PG, Mettulio R, Ferrero EA. Variation of crustacean hyperglycemic hormone (cHH) level in the eyestalk and haemolymph of the shrimp Palaemon elegans following stress. J Exp Biol (2004) 207:4205–13. doi: 10.1242/jeb.01264
171. Lorenzon S, Edomi P, Giulianini PG, Mettulio R, Ferrero EA. Role of biogenic amines and cHH in the crustacean hyperglycemic stress response. J Exp Biol (2005) 208:3341–7. doi: 10.1242/jeb.01761
172. Fingerman M, Hanumate MM, Deshpande UD, Nagabhushanam R. Increase in the total reducing substances in the hemolymph of the freshwater crab, Barytelphusa guerini, produced by a pesticide (DDT) and an indolealkylamine (serotonin). Experientia (1981) 37:178–9. doi: 10.1007/BF01963219
173. Hanumante MM, Fingerman SW, Fingerman M. Antagonism of the inhibitory effect of the polychlorinated biphenyl preparation, Aroclor 1242, on color changes of the fiddler crab, Uca pugilator, by norepinephrine and drugs affecting noradrenergic neurotransmission. Bull Environ Contam Toxicol (1981) 26:479–84. doi: 10.1007/BF01622123
174. Reddy PS, Bhagyalakshmi A, Ramamurthi R. Effect of sumithion on ovarian growth of a fresh water rice field crab (Oziotelphusa senex senex Fabricius). Toxicol Lett (1983) 18:273–6. doi: 10.1016/0378-4274(83)90106-6
175. Bhagyalakshmi A, Reddy PS, Ramamurthi R. Changes in hemolymph glucose, hepatopancreas glycogen, total carbohydrates, phosphorylase and amino transferases of sumithion-stressed freshwater rice-field crab (Oziotelphusa senex senex). Toxicol Lett (1983) 18:277–84. doi: 10.1016/0378-4274(83)90107-8
176. Sarojini R, Nagabhushanam R, Fingerman M. A possible neurotransmitter-neuroendocrine mechanism in naphthalene-induced atresia of the ovary of the red swamp crayfish, Procambarus clarkii. Comp Biochem Physiol C Pharmacol Toxicol Endocrinol (1994) 108:33–8. doi: 10.1016/1367-8280(94)90085-X
177. Staub GC. Fingerman M. A mechanism of action for the inhibition of black pigment dispersion in the fiddler crab, Uca pugilator, by naphthalene. Comp Biochem Physiol Part C Comp Pharmacol (1984) 79:447–53. doi: 10.1016/0742-8413(84)90229-9
178. Staub GC, Fingerman M. Effect of naphthalene on color changes of the sand fiddler crab, Uca pugilator. Comp Biochem Physiol Part C Comp Pharmacol (1984) 77:7–12. doi: 10.1016/0742-8413(84)90123-3
179. Fingerman M, Hanumante MM, Fingerman SW. The effects of biogenic amines on color changes of the fiddler crab, Uca pugilator: Further evidence for roles of 5-hydroxytryptamine and dopamine as neurotransmitters triggering release of erythrophorotropic hormones. Comp Biochem Physiol Part C Comp Pharmacol (1981) 68:205–11. doi: 10.1016/0306-4492(81)90017-4
180. Hanumante MM, Fingerman M. Further evidence for norepinephrine as a neurotransmitter stimulating release of melanin-dispersing hormone in the fiddler crab, Uca pugilator: The changes in the melanophores of the crabs following reserpine, 6-hydroxydopamine and bretylium administration. Gen Pharmacol Vasc Syst (1982) 13:99–103. doi: 10.1016/0306-3623(82)90063-5
181. Sarojini R, Nagabhushanam R, Fingerman M. Evidence for opioid involvement in the regulation of ovarian maturation of the fiddler crab, Uca pugilator. Comp Biochem Physiol A Physiol (1995) 111:279–82. doi: 10.1016/0300-9629(94)00214-E
182. Sarojini R, Nagabhushanam R, Fingerman M. In vivo assessment of opioid agonists and antagonists on ovarian maturation in the red swamp crayfish, Procambarus clarkii. Comp Biochem Physiol C Pharmacol Toxicol Endocrinol (1996) 115:149–53. doi: 10.1016/S0742-8413(96)00108-9
183. Campos B, Rivetti C, Kress T, Barata C, Dircksen H. Depressing antidepressant: Fluoxetine affects serotonin neurons causing adverse reproductive responses in Daphnia magna. Environ Sci Technol (2016) 50:6000–7. doi: 10.1021/acs.est.6b00826
184. Robert A, Monsinjon T, Péden R, Rasoamampianina V, Le Mével J-C, Knigge T. In vivo effects of serotonin and fluoxetine on cardio-ventilatory functions in the shore crab Carcinus maenas (L. 1758). Aquat Toxicol (2019) 207:132–41. doi: 10.1016/j.aquatox.2018.12.004
185. Camp AA, Yun J, Chambers SA, Haeba MH, LeBlanc GA. Involvement of glutamate and serotonin transmitter systems in male sex determination in Daphnia pulex. J Insect Physiol (2020) 121:104015. doi: 10.1016/j.jinsphys.2020.104015
186. Ford AT, Feuerhelm E. Effects of the antidepressant fluoxetine on pigment dispersion in chromatophores of the common sand shrimp, Crangon crangon: repeated experiments paint an inconclusive picture. Ecotoxicology (2020) 29:1368–76. doi: 10.1007/s10646-020-02272-7
187. Nelson DR, Goldstone JV, Stegeman JJ. The cytochrome P450 genesis locus: the origin and evolution of animal cytochrome P450s. Philos Trans R Soc B Biol Sci (2013) 368:20120474. doi: 10.1098/rstb.2012.0474
188. Baldwin WS, Marko PB, Nelson DR. The cytochrome P450 (CYP) gene superfamily in Daphnia pulex. BMC Genomics (2009) 10:169. doi: 10.1186/1471-2164-10-169
189. Ye H, Huang H, Li S, Wang G. Immunorecognition of estrogen and androgen receptors in the brain and thoracic ganglion mass of mud crab, Scylla paramamosain. Prog Nat Sci (2008) 18:691–5. doi: 10.1016/j.pnsc.2007.12.012
190. Paolucci M, Cristo CD, Cosmo AD. Immunological evidence for progesterone and estradiol receptors in the freshwater crayfish Austropotamobius pallipes. Mol Reprod Dev (2002) 63:55–62. doi: 10.1002/mrd.10158
191. Gilda JE, Ghosh R, Cheah JX, West TM, Bodine SC, Gomes AV. Western blotting inaccuracies with unverified antibodies: Need for a Western Blotting Minimal Reporting Standard (WBMRS). PloS One (2015) 10:e0135392. doi: 10.1371/journal.pone.0135392
192. Baldwin WS, Bailey R, Long KE, Klaine S. Incomplete ecdysis is an indicator of ecdysteroid exposure in Daphnia magna. Environ Toxicol Chem (2001) 20:1564–9. doi: 10.1002/etc.5620200721
193. Brennan SJ, Brougham CA, Roche JJ, Fogarty AM. Multi-generational effects of four selected environmental oestrogens on Daphnia magna. Chemosphere (2006) 64:49–55. doi: 10.1016/j.chemosphere.2005.11.046
194. Tsukimura B. Crustacean vitellogenesis: Its role in oocyte development. Am Zool (2015) 41:465–76. doi: 10.1093/icb/41.3.465
195. Hannas BR, Wang YH, Thomson S, Kwon G, Li H, LeBlanc GA. Regulation and dysregulation of vitellogenin mRNA accumulation in daphnids (Daphnia magna). Aquat Toxicol (2011) 101:351–7. doi: 10.1016/j.aquatox.2010.11.006
196. Swetha CH, Girish BP, Reddy PS. Elucidation of the role of estradiol and progesterone in regulating reproduction in the edible crab, Oziothelphusa senex senex. RSC Adv (2016) 6:24959–67. doi: 10.1039/C5RA23637A
197. Umbers KDL, Fabricant SA, Gawryszewski FM, Seago AE, Herberstein ME. Reversible colour change in Arthropoda. Biol Rev Camb Philos Soc (2014) 89:820–48. doi: 10.1111/brv.12079
198. McNamara JC, Milograna SR. Adaptive color change and the molecular endocrinology of pigmenttranslocation in crustacean chromatophores. In: Chang ES, Thiel M (Eds). Nat Hist Crust Vol 4 (2015) 4:68–102. Physiology. Oxford University Press: Oxford, England ISBN: 9780199832415
199. Noël P, Chassard-Bouchaud C. “Chromatophores et pigmentation,” In: Traité de zoologie: anatomie, systématique, biologie: VII Crustacés: 1. Morphologie, physiologie, reproduction, systématique. Paris: Masson (1994). p. 145–60.
200. Detto T, Hemmi JM, Backwell PRY. Colouration and colour changes of the fiddler crab, Uca capricornis: a descriptive study. PloS One (2008) 3:e1629. doi: 10.1371/journal.pone.0001629
201. Wade NM, Melville-Smith R, Degnan BM, Hall MR. Control of shell colour changes in the lobster, Panulirus cygnus. J Exp Biol (2008) 211:1512–9. doi: 10.1242/jeb.012930
203. Rao KR. Crustacean pigmentary-effector hormones: Chemistry and functions of RPCH, PDH, and related peptides. Am Zool (2001) 41:364–79. doi: 10.1093/icb/41.3.364
204. Davie PJ, Guinot D, Ng PK. Anatomy and functional morphology ofBrachyura. In: Castro P, Davie PJF, Guinot D, Schram F, Von Vaupel Klein C, eds. Treatise on Zoology-Anatomy, Taxonomy, Biology. The Crustacea, Volume 9 Part C (2 vols). Leiden: E.J. Brill. p. 11–163.
206. Stevens M, Lown AE, Wood LE. Camouflage and individual variation in shore crabs (Carcinus maenas) from different habitats. PloS One (2014) 9:e115586. doi: 10.1371/journal.pone.0115586
207. Milograna SR, Bell FT, McNamara JC. Signal transduction, plasma membrane calcium movements, and pigment translocation in freshwater shrimp chromatophores. J Exp Zool (2010) 313A:605–17. doi: 10.1002/jez.633
208. Siegenthaler A, Mastin A, Dufaut C, Mondal D, Benvenuto C. Background matching in the brown shrimp Crangon crangon: adaptive camouflage and behavioural-plasticity. Sci Rep (2018) 8:3292. doi: 10.1038/s41598-018-21412-y
209. Gaus G, Kleinholz LH, Kegel G, Keller R. Isolation and characterization of red-pigment-concentrating hormone (RPCH) from six crustacean species. J Comp Physiol B (1990) 160:373–9. doi: 10.1007/BF01075668
210. Marco HG, Verlinden H, Vanden Broeck J, Gäde G. Characterisation and pharmacological analysis of a crustacean G protein-coupled receptor: the red pigment-concentrating hormone receptor of Daphnia pulex. Sci Rep (2017) 7:6851. doi: 10.1038/s41598-017-06805-9
211. Fernlund P, Josefsson L. Crustacean color-change hormone: Amino acid sequence and chemical synthesis. Science (1972) 177:173–5. doi: 10.1126/science.177.4044.173
212. Fernlund P. Structure of a light-adapting hormone from the shrimp, Pandalus borealis. Biochim Biophys Acta (1976) 439:17–25. doi: 10.1016/0005-2795(76)90155-0
213. Mangerich S, Keller R, Dircksen H. Immunocytochemical identification of structures containing putative red pigment-concentrating hormone in two species of decapod crustaceans. Cell Tissue Res (1986) 245:377–86. doi: 10.1007/BF00213945
214. Mangerich S, Keller R, Dircksen H, Rao KR, Riehm JP. Immunocytochemical localization of pigment-dispersing hormone (PDH) and its coexistence with FMRFamide-immunoreactive material in the eyestalks of the decapod crustaceans Carcinus maenas and Orconectes limosus. Cell Tissue Res (1987) 250:365–75. doi: 10.1007/BF00219081
215. Löhr J, Klein J, Webster S, Dircksen H. Quantification, immunoaffinity purification and sequence analysis of a pigment-dispersing hormone of the shore crab, Carcinus maenas (L.). Comp Biochem Physiol B (1993) 104:699—706. doi: 10.1016/0305-0491(93)90200-o
216. Sarojini R, Nagabhushanam R, Fingerman M. A neurotransmitter role for red-pigment-concentrating hormone in ovarian maturation in the red swamp crayfish Procambarus clarkii. J Exp Biol (1995) 198:1253–7.
217. Milograna SR, Bell FT, McNamara JC. Signaling events during cyclic guanosine monophosphate-regulated pigment aggregation in freshwater shrimp chromatophores. Biol Bull (2012) 223:178–91. doi: 10.1086/BBLv223n2p178
218. Milograna SR, Ribeiro MR, Bell FT, McNamara JC. Pigment translocation in caridean shrimp chromatophores: Receptor type, signal transduction, second messengers, and cross talk among multiple signaling cascades. J Exp Zool Part Ecol Genet Physiol (2016) 325:565–80. doi: 10.1002/jez.2052
219. Hanumante MM, Fingerman M. 5-Hydroxytryptaminergic control of red pigment-dispersing hormone release in the fiddler crab, Uca pugilator: Effects of a 5-hydroxytryptamine uptake inhibitor and a 5-hydroxytryptamine receptor blocker on 5-hydroxytryptamine-induced and iproniazid-induced red pigment dispersion. Gen Comp Endocrinol (1981) 45:395–401. doi: 10.1016/0016-6480(81)90080-0
220. Bull JJ. Sex determining mechanisms: An evolutionary perspective. Experientia (1985) 41:1285–96. doi: 10.1007/BF01952071
221. Hattori RS, Gould RJ, Fujioka T, Saito T, Kurita J, Strüssmann CA, et al. Temperature-dependent sex determination in Hd-rR medaka Oryzias latipes: Gender sensitivity, thermal threshold, critical period, and DMRT1 expression profile. Sex Dev (2007) 1:138–46. doi: 10.1159/000100035
222. Piferrer F. Endocrine sex control strategies for the feminization of teleost fish. Reprod Biotechnol Finfish Aquac (2001) 197:229–81. doi: 10.1016/S0044-8486(01)00589-0
223. Staelens J, Rombaut D, Vercauteren I, Argue B, Benzie J, Vuylsteke M. High-density linkage maps and sex-linked markers for the black tiger shrimp (Penaeus monodon). Genetics (2008) 179:917. doi: 10.1534/genetics.107.080150
224. Li Y, Dierens L, Byrne K, Miggiano E, Lehnert S, Preston N, et al. QTL detection of production traits for the Kuruma prawn Penaeus japonicus (Bate) using AFLP markers. Aquaculture (2006) 258:198–210. doi: 10.1016/j.aquaculture.2006.04.027
225. Juchault P, Rigaud T. Evidence for female heterogamety in two terrestrial crustaceans and the problem of sex chromosome evolution in isopods. Heredity (1995) 75:466–71. doi: 10.1038/hdy.1995.163
226. Weeks SC, Benvenuto C, Sanderson TF, Duff RJ. Sex chromosome evolution in the clam shrimp, Eulimnadia texana. J Evol Biol (2010) 23:1100–6. doi: 10.1111/j.1420-9101.2010.01963.x
227. De Vos S, Bossier P, Van Stappen G, Vercauteren I, Sorgeloos P, Vuylsteke M. A first AFLP-based genetic linkage map for brine shrimp Artemia franciscana and its application in mapping the sex locus. PloS One (2013) 8:e57585. doi: 10.1371/journal.pone.0057585
228. Kvist J, Athanàsio CG, Pfrender ME, Brown JB, Colbourne JK, Mirbahai L. A comprehensive epigenomic analysis of phenotypically distinguishable, genetically identical female and male Daphnia pulex. BMC Genomics (2020) 21:17. doi: 10.1186/s12864-019-6415-5
229. Suzuki S, Yamasaki K. Sex-reversal of male Armadillidium vulgare (Isopoda, Malacostraca, Crustacea) following andrectomy and partial gonadectomy. Gen Comp Endocrinol (1991) 83:375–8. doi: 10.1016/0016-6480(91)90142-S
230. Suzuki S. Androgenic gland hormone is a sex-reversing factor but cannot be a sex-determining factor in the female crustacean isopod Armadillidium vulgare. Gen Comp Endocrinol (1999) 115:370–8. doi: 10.1006/gcen.1999.7324
231. Aflalo ED, Hoang TTT, Nguyen VH, Lam Q, Nguyen DM, Trinh QS, et al. A novel two-step procedure for mass production of all-male populations of the giant freshwater prawn Macrobrachium rosenbergii. Aquaculture (2006) 256:468–78. doi: 10.1016/j.aquaculture.2006.01.035
232. Parnes S, Khalaila I, Hulata G, Sagi A. Sex determination in crayfish: are intersex Cherax quadricarinatus (Decapoda, Parastacidae) genetically females? Genet Res (2003) 82:107–16. doi: 10.1017/S0016672303006372
233. Dunn AM, Hogg JC, Kelly A, Hatcher MJ. Two cues for sex determination in Gammarus duebeni: Adaptive variation in environmental sex determination? Limnol Oceanogr (2005) 50:346–53. doi: 10.4319/lo.2005.50.1.0346
234. Guler Y, Short S, Green Etxabe A, Sherhod CM, Kille P, Ford AT. Impacts of a newly identified behaviour-altering trematode on its host amphipod: from the level of gene expression to population. Parasitology (2015) 142:1469–80. doi: 10.1017/S0031182015000918
235. Bulnheim H. Microsporidian infections of amphipods with special reference to host-parasite relationships: a review. Mar Fish Rev (1975) 37:39–45.
236. Zupo V, Messina P. How do dietary diatoms cause the sex reversal of the shrimp Hippolyte inermis Leach (Crustacea, Decapoda). Mar Biol (2007) 151:907–17. doi: 10.1007/s00227-006-0524-9
237. Charniaux-Cotton H. Discovery in an amphipod crustacean (Orchestia gammarella) of an endocrine gland responsible for the differentiation of primary and secondary male sex characteristics. C R Acad Sci Paris (1954) 239:780–2.
238. Charniaux-Cotton H. Vitellogenesis and its control in Malacostracan crustacea. Am Zool (1985) 25:197–206. doi: 10.1093/icb/25.1.197
239. Sagi A, Snir E, Khalaila I. Sexual differentiation in decapod crustaceans: role of the androgenic gland. Invertebr Reprod Dev (1997) 31:55–61. doi: 10.1080/07924259.1997.9672563
240. Charniaux-Cotton H. Surgical castration in an amphipod crustacean (Orchestia gammarella) and determination of the secondary sexual characteristics: first results. CR Acad Sci Paris (1952) 234:2570–2.
241. Charniaux-Cotton H. Etude du déterminisme des caractères sexuels secondaires par castration chirurgicale et implantation d’ovaire chez un Crustacé Amphipode (Orchestia gammarella). C R Acad Sci Paris (1953) 236:141–3.
242. Charniaux-Cotton H. Contrôle hormonal de la différenciation du sexe et de la reproduction chez les Crustacés supérieurs. Bull Soc Zool (1958) 82:314–36.
243. Charniaux-Cotton H, Payen G. Sexual differentiation. In: Bliss DE, Mantel LH, editors. The Biology of Crustacea, Volume 9. New York: Academic Press (1985).
244. Ford AT. Can you feminise a crustacean? Aquat Toxicol (2008) 88:316–21. doi: 10.1016/j.aquatox.2008.04.013
245. Barki A, Karplus I, Manor R, Sagi A. Intersexuality and behavior in crayfish: The de-masculinization effects of androgenic gland ablation. Horm Behav (2006) 50:322–31. doi: 10.1016/j.yhbeh.2006.03.017
246. Hasegawa Y, Haino-Fukushima K, Katakura Y. Isolation and properties of androgenic gland hormone from the terrestrial isopod, Armadillidium vulgare. Gen Comp Endocrinol (1987) 67:101–10. doi: 10.1016/0016-6480(87)90209-7
247. Martin G, Juchault P, Sorokine O, Van Dorsselaer A. Purification and characterization of androgenic hormone from the terrestrial isopod Armadillidium vulgare Latr. (Crustacea, Oniscidea). Gen Comp Endocrinol (1990) 80:349–54. doi: 10.1016/0016-6480(90)90183-M
248. Okuno A, Hasegawa Y, Nagasawa H. Purification and properties of androgenic gland hormone from the terrestrial isopod Armadillidium vulgare. Zool Sci (1997) 14:837–42. doi: 10.2108/zsj.14.837
249. Martin G, Sorokine O, Moniatte M, Van Dorsselaer A. The androgenic hormone of the crustacean isopod Armadillidium vulgare. Ann N Y Acad Sci (1998) 839:111–7. doi: 10.1111/j.1749-6632.1998.tb10741.x
250. Martin G, Sorokine O, Moniatte M, Bulet P, Hetru C, Van Dorsselaer A. The structure of a glycosylated protein hormone responsible for sex determination in the isopod, Armadillidium vulgare. Eur J Biochem (1999) 262:727–36. doi: 10.1046/j.1432-1327.1999.00442.x
251. Okuno A, Hasegawa Y, Ohira T, Katakura Y, Nagasawa H. Characterization and cDNA cloning of androgenic gland hormone of the terrestrial isopod Armadillidium vulgare. Biochem Biophys Res Commun (1999) 264:419–23. doi: 10.1006/bbrc.1999.1522
252. Martin G, Juchault P. Androgenic hormone specificity in terrestrial isopods (Oniscidea): Systematic involvements. J Crustac Biol (1999) 19:684–9. doi: 10.1163/193724099X00402
253. Guo Q, Li S, Lv X, Xiang J, Sagi A, Manor R, et al. A putative insulin-like androgenic gland hormone receptor gene specifically expressed in male chinese shrimp. Endocrinology (2018) 159:2173–85. doi: 10.1210/en.2017-03253
254. Ventura T, Manor R, Aflalo ED, Weil S, Raviv S, Glazer L, et al. Temporal silencing of an androgenic gland-specific insulin-like gene affecting phenotypical gender differences and spermatogenesis. Endocrinology (2009) 150:1278–86. doi: 10.1210/en.2008-0906
255. Ventura T, Manor R, Aflalo ED, Weil S, Rosen O, Sagi A. Timing sexual differentiation: Full functional sex reversal achieved through silencing of a single insulin-like gene in the prawn, Macrobrachium rosenbergii. Biol Reprod (2012) 86 86(3):90,1-6. doi: 10.1095/biolreprod.111.097261
256. Khalaila I, Manor R, Weil S, Granot Y, Keller R, Sagi A. The eyestalk–androgenic gland–testis endocrine axis in the crayfish Cherax quadricarinatus. Gen Comp Endocrinol (2002) 127:147–56. doi: 10.1016/S0016-6480(02)00031-X
257. Guo Q, Li S, Lv X, Xiang J, Manor R, Sagi A, et al. Sex-biased CHHs and their putative receptor regulate the expression of IAG gene in the shrimp Litopenaeus vannamei. Front Physiol (2019) 10:1525. doi: 10.3389/fphys.2019.01525
258. Cristescu MEA, Colbourne JK, Radivojac J. Lynch M. A microsatellite-based genetic linkage map of the waterflea, Daphnia pulex: On the prospect of crustacean genomics. Genomics (2006) 88:415–30. doi: 10.1016/j.ygeno.2006.03.007
259. Routtu J, Jansen B, Colson I, De Meester L, Ebert D. The first-generation Daphnia magna linkage map. BMC Genomics (2010) 11:508. doi: 10.1186/1471-2164-11-508
260. Hebert PDN. The adaptive significance of cyclomorphosis in Daphnia: more possibilities. Freshw Biol (1978) 8:313–20. doi: 10.1111/j.1365-2427.1978.tb01452.x
261. Paland S, Lynch M. Transitions to asexuality result in excess amino acid substitutions. Science (2006) 311:990–2. doi: 10.1126/science.1118152
262. Brendonck L, De Meester L. Egg banks in freshwater zooplankton: evolutionary and ecological archives in the sediment. Hydrobiologia (2003) 491:65–84. doi: 10.1023/A:1024454905119
263. Camp AA, Haeba MH, LeBlanc GA. Complementary roles of photoperiod and temperature in environmental sex determination in Daphnia spp. J Exp Biol (2019) 222:jeb195289. doi: 10.1242/jeb.195289
264. Kleiven OT, Larsson P, Hobæk A. Sexual reproduction in Daphnia magna requires three stimuli. Oikos (1992) 65:197–206. doi: 10.2307/3545010
265. Hobaek A, Larsson P. Sex Determination in Daphnia magna. Ecology (1990) 71:2255–68. doi: 10.2307/1938637
266. Toyota K, Miyakawa H, Yamaguchi K, Shigenobu S, Ogino Y, Tatarazako N, et al. NMDA receptor activation upstream of methyl farnesoate signaling for short day-induced male offspring production in the water flea, Daphnia pulex. BMC Genomics (2015) 16:186. doi: 10.1186/s12864-015-1392-9
267. Traynelis SF, Wollmuth LP, McBain CJ, Menniti FS, Vance KM, Ogden KK, et al. Glutamate Receptor Ion Channels: Structure, Regulation, and Function. Pharmacol Rev (2010) 62:405. doi: 10.1124/pr.109.002451
268. Paoletti P, Bellone C, Zhou Q. NMDA receptor subunit diversity: impact on receptor properties, synaptic plasticity and disease. Nat Rev Neurosci (2013) 14:383–400. doi: 10.1038/nrn3504
269. Nishimura M, Sato K, Okada T, Schloss P, Shimada S, Tohyama M. MK-801 blocks monoamine transporters expressed in HEK cells. FEBS Lett (1998) 423:376–80. doi: 10.1016/S0014-5793(98)00126-4
270. Brunello N, Mendlewicz J, Kasper S, Leonard B, Montgomery S, Nelson JC, et al. The role of noradrenaline and selective noradrenaline reuptake inhibition in depression. Eur Neuropsychopharmacol (2002) 12:461–75. doi: 10.1016/S0924-977X(02)00057-3
271. Hui JHL, Hayward A, Bendena WG, Takahashi T, Tobe SS. Evolution and functional divergence of enzymes involved in sesquiterpenoid hormone biosynthesis in crustaceans and insects. Peptides (2010) 31:451–5. doi: 10.1016/j.peptides.2009.10.003
272. Olmstead AW, LeBlanc GA. Juvenoid hormone methyl farnesoate is a sex determinant in the crustacean Daphnia magna. J Exp Zool (2002) 293:736–9. doi: 10.1002/jez.10162
273. Tatarazako N, Oda S, Watanabe H, Morita M, Iguchi T. Juvenile hormone agonists affect the occurrence of male Daphnia. Chemosphere (2003) 53:827–33. doi: 10.1016/S0045-6535(03)00761-6
274. Kato Y, Kobayashi K, Watanabe H, Iguchi T. Environmental sex determination in the branchiopod crustacean Daphnia magna: deep conservation of a Doublesex gene in the sex-determining pathway. PloS Genet (2011) 7:e1001345. doi: 10.1371/journal.pgen.1001345
275. Nong QD, Mohamad Ishak NS, Matsuura T, Kato Y, Watanabe H. Mapping the expression of the sex determining factor Doublesex1 in Daphnia magna using a knock-in reporter. Sci Rep (2017) 7:13521. doi: 10.1038/s41598-017-13730-4
276. Toyota K, Kato Y, Sato M, Sugiura N, Miyagawa S, Miyakawa H, et al. Molecular cloning of doublesex genes of four cladocera (water flea) species. BMC Genomics (2013) 14:239. doi: 10.1186/1471-2164-14-239
277. Bellés X, Martín D, Piulachs M-D. The mevalonate pathway and the synthesis of juvenile hormone in insects. Annu Rev Entomol (2004) 50:181–99. doi: 10.1146/annurev.ento.50.071803.130356
278. Li S, Friesen JA, Holford KC, Borst DW. Methyl farnesoate synthesis in the lobster mandibular organ: The roles of HMG-CoA reductase and farnesoic acid O-methyltransferase. Comp Biochem Physiol A Mol Integr Physiol (2010) 155:49–55. doi: 10.1016/j.cbpa.2009.09.016
279. White AF. Metabolism of the juvenile hormone analogue methyl farnesoate 10, 11-epoxide in two insect species. Life Sci (1972) 11:201–10. doi: 10.1016/0024-3205(72)90110-5
280. Homola E, Chang ES. Assay methods for methyl farnesoate esterases in crustaceans. Arch Insect Biochem Physiol (1997) 36:115–28. doi: 10.1002/(SICI)1520-6327(1997)36:2<115::AID-ARCH4>3.0.CO;2-U
281. Wainwright G, Webster SG, Wilkinson MC, Chung JS, Rees HH. Structure and significance of mandibular organ-inhibiting hormone in the crab, Cancer pagurus involvement in multihormonal regulation of growth and reproduction. J Biol Chem (1996) 271:12749–54. doi: 10.1042/bj3340651
282. Wainwright G, Webster SG, Rees HH. Neuropeptide regulation of biosynthesis of the juvenoid, methyl farnesoate, in the edible crab, Cancer pagurus. Biochem J (1998) 334:651–7. doi: 10.1042/bj3340651
283. Laufer H, Landau M, Homola E, Borst DW. Methyl farnesoate: Its site of synthesis and regulation of secretion in a juvenile crustacean. Insect Biochem (1987) 17:1129–31. doi: 10.1016/0020-1790(87)90134-X
284. LeBlanc GA, Wang YH, Holmes CN, Kwon G, Medlock EK. A transgenerational endocrine signaling pathway in Crustacea. PloS One (2013) 8:e61715. doi: 10.1371/journal.pone.0061715
285. Miyakawa H, Toyota K, Hirakawa I, Ogino Y, Miyagawa S, Oda S, et al. A mutation in the receptor Methoprene-tolerant alters juvenile hormone response in insects and crustaceans. Nat Commun (2013) 4:1856. doi: 10.1038/ncomms2868
286. Wilson TG. Fabian J. A Drosophila melanogaster mutant resistant to a chemical analog of juvenile hormone. Dev Biol (1986) 118:190–201. doi: 10.1016/0012-1606(86)90087-4
287. Tiu SH-K, Hult EF, Yagi KJ, Tobe SS. Farnesoic acid and methyl farnesoate production during lobster reproduction: Possible functional correlation with retinoid X receptor expression. Gen Comp Endocrinol (2012) 175:259–69. doi: 10.1016/j.ygcen.2011.11.016
288. Durica DS, Wu X, Anilkumar G, Hopkins PM, Chung AC-K. Characterization of crab EcR and RXR homologs and expression during limb regeneration and oocyte maturation. Mol Cell Endocrinol (2002) 189:59–76. doi: 10.1016/S0303-7207(01)00740-7
289. Kim H-W, Lee SG, Mykles DL. Ecdysteroid-responsive genes, RXR and E75, in the tropical land crab, Gecarcinus lateralis: Differential tissue expression of multiple RXR isoforms generated at three alternative splicing sites in the hinge and ligand-binding domains. Mol Cell Endocrinol (2005) 242:80–95. doi: 10.1016/j.mce.2005.08.001
290. Dai T-H, Sserwadda A, Song K, Zang Y-N, Shen H-S. Cloning and Expression of Ecdysone Receptor and Retinoid X Receptor from Procambarus clarkii: Induction by Eyestalk Ablation. Int J Mol Sci (2016) 17(10):1739. doi: 10.3390/ijms17101739
291. Gouveia D, Bonneton F, Almunia C, Armengaud J, Quéau H, Degli-Esposti D, et al. Identification, expression, and endocrine-disruption of three ecdysone-responsive genes in the sentinel species Gammarus fossarum. Sci Rep (2018) 8:3793. doi: 10.1038/s41598-018-22235-7
292. Wang YH, Wang G, LeBlanc GA. Cloning and characterization of the retinoid X receptor from a primitive crustacean Daphnia magna. Gen Comp Endocrinol (2007) 150:309–18. doi: 10.1016/j.ygcen.2006.08.002
293. Wang YH, LeBlanc GA. Interactions of methyl farnesoate and related compounds with a crustacean retinoid X receptor. Mol Cell Endocrinol (2009) 309:109–16. doi: 10.1016/j.mce.2009.05.016
294. Wang YH, Kwon G, Li H, LeBlanc GA. Tributyltin synergizes with 20-hydroxyecdysone to produce endocrine toxicity. Toxicol Sci (2011) 123:71–9. doi: 10.1093/toxsci/kfr154
295. Li Y, Ginjupalli GK, Baldwin WS. The HR97 (NR1L) group of nuclear receptors: A new group of nuclear receptors discovered in Daphnia species. Gen Comp Endocrinol (2014) 206:30–42. doi: 10.1016/j.ygcen.2014.07.022
296. Hyde CJ, Fitzgibbon QP, Elizur A, Smith GG, Ventura T. Transcriptional profiling of spiny lobster metamorphosis reveals three new additions to the nuclear receptor superfamily. BMC Genomics (2019) 20:531. doi: 10.1186/s12864-019-5925-5
297. Wang HY, Olmstead AW, Li H, LeBlanc GA. The screening of chemicals for juvenoid-related endocrine activity using the water flea Daphnia magna. Aquat Toxicol (2005) 74:193–204. doi: 10.1016/j.aquatox.2005.05.010
298. Olmstead AW, LeBlanc GA. Insecticidal juvenile hormone analogs stimulate the production of male offspring in the crustacean Daphnia magna. Environ Health Perspect (2003) 111:919–24. doi: 10.1289/ehp.5982
299. Dodson SI, Merritt CM, Shannahan J-P, Shults CM. Low exposure concentrations of atrazine increase male production in Daphnia pulicaria. Environ Toxicol Chem (1999) 18:1568–73. doi: 10.1002/etc.5620180732
300. Cooper RL, Stoker TE, Tyrey L, Goldman JM, McElroy WK. Atrazine disrupts the hypothalamic control of pituitary-ovarian function. Toxicol Sci (2000) 53:297–307. doi: 10.1093/toxsci/53.2.297
301. DeFur PL, Crane M, Ingersoll C, Tattersfield L. Endocrine disruption in invertebrates: endocrinology, testing, andassessment. Pensacola: SETAC technical publication series. SETAC press (1999).
302. Ford AT, LeBlanc GA. Endocrine disruption in invertebrates: A survey of research progress. Environ Sci Technol (2020) 54:13365–9. doi: 10.1021/acs.est.0c04226
303. Katsiadaki I. Are marine invertebrates really at risk from endocrine-disrupting chemicals? Curr Opin Environ Sci Health (2019) 11:37–42. doi: 10.1016/j.coesh.2019.06.005
304. Cuvillier-Hot V, Lenoir A. Invertebrates facing environmental contamination by endocrine disruptors: Novel evidences and recent insights. Mol Cell Endocrinol (2020) 504:110712. doi: 10.1016/j.mce.2020.110712
305. Short S, Yang G, Kille P, Ford AT. Vitellogenin is not an appropriate biomarker of feminisation in a Crustacean. Aquat Toxicol (2014) 153:89–97. doi: 10.1016/j.aquatox.2013.11.014
306. Matozzo V, Gagné F, Marin MG, Ricciardi F, Blaise C. Vitellogenin as a biomarker of exposure to estrogenic compounds in aquatic invertebrates: A review. Environ Int (2008) 34:531–45. doi: 10.1016/j.envint.2007.09.008
307. Jayasankar V, Tomy S, Wilder M. Insights on molecular mechanisms of ovarian development in decapod crustacea: Focus on vitellogenesis-stimulating factors and pathways. Front Endocrinol (2020) 11:577925. doi: 10.3389/fendo.2020.577925
Keywords: endocrine disruption, neuroendocrine disruption, ecdysteroid signaling, color change, sexual differentiation
Citation: Knigge T, LeBlanc GA and Ford AT (2021) A Crab Is Not a Fish: Unique Aspects of the Crustacean Endocrine System and Considerations for Endocrine Toxicology. Front. Endocrinol. 12:587608. doi: 10.3389/fendo.2021.587608
Received: 26 July 2020; Accepted: 25 January 2021;
Published: 02 March 2021.
Edited by:
Heinrich Dircksen, Stockholm University, SwedenReviewed by:
Taisen Iguchi, National Institute for Basic Biology, JapanLynn M. Riddiford, University of Washington, United States
Copyright © 2021 Knigge, LeBlanc and Ford. This is an open-access article distributed under the terms of the Creative Commons Attribution License (CC BY). The use, distribution or reproduction in other forums is permitted, provided the original author(s) and the copyright owner(s) are credited and that the original publication in this journal is cited, in accordance with accepted academic practice. No use, distribution or reproduction is permitted which does not comply with these terms.
*Correspondence: Thomas Knigge, dGhvbWFzLmtuaWdnZUB1bml2LWxlaGF2cmUuZnI=