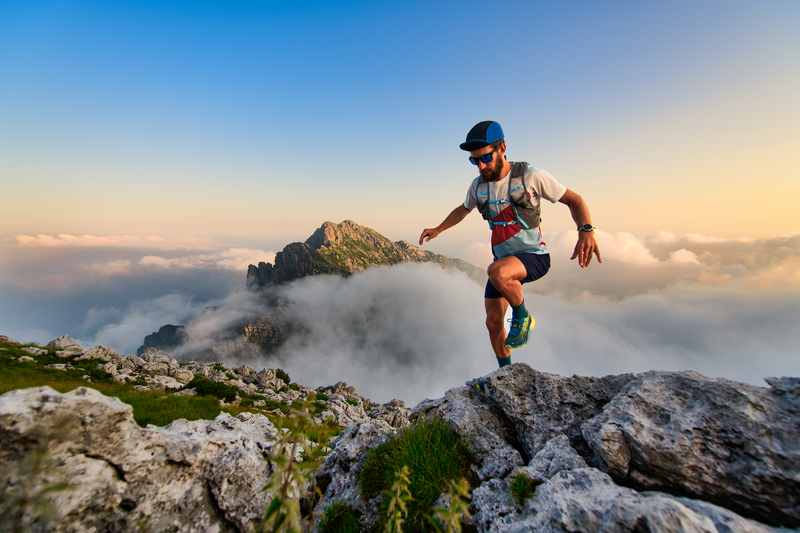
94% of researchers rate our articles as excellent or good
Learn more about the work of our research integrity team to safeguard the quality of each article we publish.
Find out more
REVIEW article
Front. Endocrinol. , 25 February 2021
Sec. Experimental Endocrinology
Volume 11 - 2020 | https://doi.org/10.3389/fendo.2020.619361
This article is part of the Research Topic Endocrine Disruptors in Aquatic Vertebrates View all 8 articles
Increasing industrial and agricultural activities have led to a disturbing increase of pollutant discharges into the environment. Most of these pollutants can induce short-term, sustained or delayed impacts on developmental, physiological, and behavioral processes that are often regulated by the endocrine system in vertebrates, including fish, thus they are termed endocrine-disrupting chemicals (EDCs). Physiological impacts resulting from the exposure of these vertebrates to EDCs include abnormalities in growth and reproductive development, as many of the prevalent chemicals are capable of binding the receptors to sex steroid hormones. The approaches employed to investigate the action and impact of EDCs is largely dependent on the specific life history and habitat of each species, and the type of chemical that organisms are exposed to. Aquatic vertebrates, such as fish, are among the first organisms to be affected by waterborne EDCs, an attribute that has justified their wide-spread use as sentinel species. Many fish species are exposed to these chemicals in the wild, for either short or prolonged periods as larvae, adults, or both, thus, studies are typically designed to focus on either acute or chronic exposure at distinct developmental stages. The aim of this review is to provide an overview of the approaches and experimental methods commonly used to characterize the effects of some of the environmentally prevalent and emerging EDCs, including 17 α-ethinylestradiol, nonylphenol, BPA, phthalates, and arsenic; and the pervasive and potential carriers of EDCs, microplastics, on reproduction and growth. In vivo and in vitro studies are designed and employed to elucidate the direct effects of EDCs at the organismal and cellular levels, respectively. In silico approaches, on the other hand, comprise computational methods that have been more recently applied with the potential to replace extensive in vitro screening of EDCs. These approaches are discussed in light of model species, age and duration of EDC exposure.
For more than a decade, a growing body of evidence has demonstrated that anthropogenically introduced compounds alter functions of the vertebrate endocrine system (1, 2). These compounds have been termed “endocrine-disrupting chemicals” (EDCs). EDCs, as defined by the U.S. Environmental Protection Agency (EPA), are “exogenous compounds that interfere with the processing and action of endogenous hormones involved in the maintenance of homeostasis and regulation of development (3). With the knowledge obtained from recent studies, the extent of our understanding of how these chemicals exert their endocrine interfering effects have expanded. From a physiological point of view, EDCs are defined as natural or synthetic compounds that disrupt the hormonal and homeostatic systems that control how organisms communicate with and respond to the environment (4). Based on the consensus statement of La Merrill et al. (5), the key characteristics of EDCs include: interaction with or activation of hormone receptors; antagonistic effect on hormone receptors; alterations of hormone receptor expression, hormone synthesis, signal transduction in hormone-responsive cells, hormone transport across cell membranes, hormone distribution or circulating hormone levels, hormone metabolism or clearance, fate of hormone-producing or hormone-responsive cells; and induction of epigenetic modifications in hormone-producing or hormone-responsive cells. Although, only mechanistic evidence for a chemical can unequivocally classify it as an EDC, it is sometimes difficult to exclude adverse endocrine effects that are correlational in nature, especially from in vivo studies, and confounding non-specific effects of a chemical on the endocrine system that are not directly related to the mode of action of EDCs as described above (i.e., cell toxicity, tissue injury, and compensatory effects). Known EDCs include plastic additives, plasticizers, industrial solvents/lubricants and their byproducts, dioxins, alkyl phenols, pesticides, pharmaceuticals, drugs, anabolic agents, and naturally occurring compounds. These and additional examples of EDCs and their effects have been comprehensively reviewed elsewhere (6–9). The growing concern for EDCs has been focused on their effects on wildlife and humans (10, 11). Data from human studies and rodent models show that EDCs are implicated in male and female reproductive development disorders (12–14), breast cancer (15), prostate cancer (16, 17), and obesity (18–20). Together, these studies not only underscore the significant public health concern and costs associated with EDCs (21), but also justify further in-depth investigation.
In aquatic environments, fish have been a reliable indicator of endocrine disruption, as they are among the first animals exposed to waterborne pollutants. In contrast to stable sexual determination in mammals, the process of sexual determination and differentiation in fish can be influenced by environmental factors (22–26). The developmental plasticity of sexual determination in fish make them particularly vulnerable to environmental EDCs that pollute waters they inhabit. Because hormone receptors play a major role in the regulation of growth and reproduction, EDCs that bind these receptors (4) and exert agonistic or antagonistic actions can result in abnormal endocrine functions (27). In rodents and amphibians, dichlorodiphenyldichloroethylene (DDE)/dichlorodiphenyltrichloroethane (DDT), diethylstilbestrol (DES), and bisphenol A (BPA) bind both androgen and estrogen receptors (28–31). DDE, DDT, kepone, methoxychlor, nonylphenol (NP), and polychlorinated biphenyls (PCB), were found to have affinity to the seven-transmembrane receptor, G-protein-coupled estrogen receptor (GPR30), in human embryonic kidney 293 (HEK293) cell lines (32). In mammals and zebrafish, BPA, phthalates, DDT, polybrominated diphenyl ethers (PBDE), and PCB can bind and alter the expression of peroxisome proliferator-activated receptor (PPAR), a protein which plays a critical role in regulating metabolism, affecting lipid metabolism and reproduction (33–35). In fish, EDCs are known to affect fertility, sexual maturation, somatic growth, stress responses and induce cellular damage, largely through alterations in the levels of hormones and their receptors (36–42). Some EDCs are reported to bioaccumulate in fish (43, 44) and shown to be transferred to offspring through the transfer of lipids from yolk to embryo (45–47). The pathways through which EDCs can impact the physiology of organisms are not only a result of their ability to bind receptors (5). The high sensitivity of larval fish to EDCs due to their developing endocrine system (48, 49) underscores the need to specifically address the effects of early-life exposures to environmental chemicals through targeted approaches. Chemicals found in municipal and industrial wastewater discharges are found to be the most common EDCs of concern for aquatic life (50, 51). The natural and synthetic steroid estrogens, 17β-estradiol (E2), and 17 α-ethinylestradiol (EE2), and alkyl phenols such as nonylphenol (NP) are among the most pervasive EDCs found in the aquatic environment (52–56). These chemicals are commonly found in sewage effluent discharged into rivers (57) and affect fish at concentrations, that range from 4.5 to 25 ng/L and 0.84 to 200 µg/L, respectively (58–61). EE2, a primary estrogen component of most oral contraceptive pills, is discharged through municipal wastewater. Because EE2 cannot be effectively removed by the treatment process, it contaminates other clean water sources that mix with treated water (62, 63). Nonylphenol ethoxylates (NPEs), widely used as surfactants in industrial processes and products, are also discharged through domestic and industrial wastewater (64, 65). NP, a degradation product of NPE, persists in the environment and has been determined to reduce fecundity and fertility in Japanese medaka (Oryzias latipes) (66), lower plasma testosterone (T) in male carp (Cyprinus carpio) (67), reduce male to female ratios in wild tilapia (Oreochromis niloticus) (68), and decrease in gonadosomatic index (percentage of gonad weight per body weight, GSI) and developmental delay in courtship behavior in male guppy (Poecilia reticulata) (69). Heavy metals have also been found to contaminate the environment (70) and act as endocrine disruptors (71–73). In particular, arsenic pollution has posed serious risk to aquatic organisms due to its widespread presence in aquatic environments (74–77). Arsenic has been shown to disrupt the endocrine system in rodents, chicken, and fish (78–81). BPA and phthalates, two of the most popular chemical building blocks in the plastics industry, are ubiquitous environmental pollutants (82, 83). BPA has been found to leach from dental sealants (84), tin cans (85), and food packaging (86, 87). Phthalates can leach from food packaging (87), alcoholic beverages (88), PVC flooring (89), personal care products (90), and medicinal products (91, 92). Thus, these chemicals can be easily released into water bodies that ultimately affect aquatic animals. A number of studies suggest that these plasticizers induce developmental impairment via thyroid and growth hormone axes, and impact reproduction in mammals and aquatic species (93). More recently, microplastic (MP) contamination has become a growing global concern (94). MPs are defined as plastic that is less than 5 mm in diameter (95) resulting from the breakdown of larger plastics that are degraded (96), or from manufactured plastic microbeads present in scrubbing agents and personal care products. MPs have been reported to be ubiquitously present in open oceans (95, 97, 98), estuaries (99), beaches, surface waters, and marine sediment (95, 100–102). Although there is no evidence that MPs directly act as EDCs on organisms, indirect effects found may be linked to chemicals, including plasticizers, that are adsorbed or leached from MPs. Nonetheless, the heightened concern over the effects of MPs as carriers of EDCs in fish still warrants thorough investigation of this emerging class of environmental contamination. Reports on the ingestion of MPs by aquatic animals, including fish (103–106), further drive efforts to understand the physiological effects of harmful chemicals leaching from MPs. Despite the plethora of known EDCs, we focus this review on the six categories of chemicals above to underscore the diversity of approaches employed to characterize their effects in fish.
While substantial progress has been made toward the assessment of the effects of EDCs in fish, a number of experimental considerations provide distinct paths for interpreting the mechanisms of action and physiological consequences of exposure to these chemicals. For example, exposing an adult fish to EDCs may have very different consequences compared with exposing fish at an early-life stage or at a developmentally critical stage. Moreover, the effects of EDCs may not be limited to the exposed individual but also extend to its progenies. Hence, approaches have ranged from in vivo assessments of the impact of EDCs during early-life and adult stages, along with long-term and transgenerational effects, to in vitro assays designed to characterize the direct effects of putative EDCs along with their affinity to hormone receptors, to in silico predictive models that integrate an array of pathways sensitive to EDCs. Some studies that have employed these different approaches are listed in Table 1 to provide a brief synopsis of the topics discussed in the following sections. Focusing on the disruption of growth and reproduction in fish, our aim here is to provide a brief overview of the approaches and experimental designs commonly employed to assess the effects of some of the pervasive and emerging EDCs, and to highlight the need of a strategy that considers combining the diverse approaches to study the adverse effects of EDCs on aquatic wildlife.
Table 1 List of studies used to analyze endocrine disrupting effects of NP, EE2, BPA, phthalates, arsenic, and microplastics with the corresponding approaches and endpoints.
Laboratory studies are used to confirm the correlational relationship between chemical pollutants in the environment and malformations or dysfunction observed in fish in the wild. The variety of approaches that are used reflects the diversity of fish species, their natural habitat, and the broad range of EDCs that they are exposed to. Each approach is most effective for addressing one particular question depending on the specific life history and habitat of each species, and the type of chemical fish are exposed to. These in vivo approaches employ a wide variety of experimental protocols, including exposure to chemicals presented in the water, those added to the diet, or compounds delivered through intraperitoneal injection. Exposure periods vary and concentrations of compounds used are either based on the levels found in the environment, those that elicit a physiological response or both. Fish models also differ among these studies, and can be generally subdivided by size into large and small fish species. Examples of the former include salmonids, tilapia, and bass, while the latter encompass zebrafish, medaka, and guppies. Larger fish models usually bear economic importance, include species of more relevance to aquaculture and conservation studies and can provide sufficient sampling of tissues for various types of analyses, but have longer life spans, require larger areas for rearing and trials and relatively higher maintenance costs. On the other hand, small fish models have a short life span, rapid development and reproductive rates, and low maintenance and husbandry costs, which provide versatility to their use as research models; their small size, however, limits the amount of various tissues and organs for analyses. Regardless of size class and experimental limitations, however, the rapid expansion in genomic approaches have allowed for the analysis of multiple fish species at various life-stages as models for investigating the effects of EDCs. This section will present various in vivo approaches for assessing the effects of EDCs on growth and reproduction taking into account differences between age, life stage, sex, exposure time and the fact that EDCs may affect not only the individual directly exposed to it, but also its progeny (4, 167–169). Among the commonly employed indicators for monitoring reproductive responses to EDC exposure in fish are GSI plasma sex steroid levels, steroidogenic enzymes, estrogen and androgen receptors (Er and Ar, respectively), and vitellogenin (VTG). VTG, an estrogen-dependent yolk protein precursor, is a common biomarker for exposure to estrogenic compounds. In normal conditions, VTG is present only in females, but males may express VTG in response to environmental estrogens and commercial diets containing estrogens (170). The actions of both natural steroid hormones and exogenous hormone mimics are mediated by steroid hormone receptors (171, 172). The synthesis of VTG is stimulated by activation of estrogen receptors (ERs) (173–176). Hence, VTG and ERs have been widely used in both in vivo and in vitro experimental designs to assess the effects of EDCs (172, 177–184). The growth hormone (GH)/insulin-like growth-factor (IGF) axis is the principal endocrine system regulating the growth and development of vertebrates, including teleosts (185, 186). GH stimulates the production of IGFs upon binding to its receptor, GHR; both GH and IGFs promote growth in target tissues (187–191). IGF binding proteins (IGFBPs), modulate the actions of IGFs by affecting their availability and activities (185, 192, 193). In addition to morphological indicators of growth such as length, weight and condition factor, the factors involved in the GH/IGF system can therefore be applied as molecular markers for growth and the assessment of the effects of EDCs on growth in fish. We divide this section into studies that examine the effects of EDCs on adult, subadult, larval stages, and across generations.
For decades, studies on the effects of EDCs have been geared toward determining the relationship between environmental chemical levels and the unnatural changes that lead to morphological abnormalities observed in wild fish (194–198). Many experimental approaches have focused on characterizing the effects of EDCs on sub-adult and adult fish. The large body mass of sub-adult and adult fish allows for the collection and analysis of numerous markers on several different tissues. On the other hand, adult fish being equipped with fully differentiated tissues and a well-developed endocrine system can more readily compensate from the effects of exogenous agents at environmental concentrations, thereby limiting the number of observable EDC effects.
Many studies have subjected adult fish to prolonged periods of EDCs exposure, while others have focused on short-term or acute exposure protocols. Since there are many definitions of chronic and acute exposure depending on the life cycle of the organism being studied, in this review, we define periods of acute exposure to EDCs as 1 week or less and sub-chronic/chronic exposure as more than 1 week to several months or years, regardless of the life-stage. In both paradigms, exposure to EDCs typically occur through waterborne exposure, diet or intraperitoneal injection. Physiological responses of fish may vary with different routes of exposure since this could affect bioaccumulation and/or bioavailability of contaminants. Bioaccumulation through these different routes may differ since the co-determinants for bioaccumulation are the various elimination mechanisms. For instance, accumulation of a waterborne contaminant by a non-dietary route can be alleviated by direct equilibrium exchange of the contaminants at the gill epithelium. Thus, dietary exposure may be quantitatively different from aqueous exposure (199). This difference, however, would still be life-stage dependent.
Acute exposure experiments evaluate EDCs that can elicit effects within a short period of time. This approach also allows investigators to obtain results expeditiously, thereby reducing cost of maintenance. The drawback of this method, however, is that it may overlook effects that are only apparent after a long period of exposure. Moreover, acute exposure of EDCs might not be suitable for adults of many larger fish species as they tend to have greater tolerance to chemical exposure, especially at low doses. Studies employing this approach are discussed below.
Because impairments in sexual development were among the first physiological effects to be observed as a result of exposure to EDCs, many studies on fish have focused on reproductive endpoints.
Experimental approaches employing acute exposure have been effective at detecting disruptive effects of NP and EE2 in adult fish. In reproductively mature male fathead minnows (Pimephales promelas) exposed to EE2 for 48 h, hepatic er alpha (erα) and vtg, and testicular ar and er were upregulated, while the testicular steroidogenic enzyme cytochrome P-45017 (cyp17) was downregulated (132). Aqueous exposure of gravid female zebrafish to EE2 elevated plasma VTG, reduced plasma E2 and T after 24 or 48 h, and affected the expression of genes involved in hormone metabolism, steroid binding, sterol metabolism, and cell growth in the liver after 24 and 168 h (124). A 1-week exposure to EE2 of sub-adult coho salmon (Oncorhynchus kisutch), increased hepatic vtg and pituitary luteinizing hormone (lh) β subunit mRNA, and induced gonadotropin-releasing hormone (gnrh) receptor contig in females (136). Adult male Swordtail fish (Xiphophorus helleri) exposed to NP and BPA for 3 days induced hepatic vtg mRNA expression and reproductive damage (112).
Assessments of acute exposure effects of EDCs in adult fish focus mainly on reproduction. Not many of these studies have focused on growth, however. Nile tilapia (Oreochromis niloticus) adult exposed to NP for 7 days exhibited anorexia (149). In gravid female zebrafish, gene ontology (GO) analysis showed that aqueous treatment with EE2 either downregulated or upregulated genes involved in the regulation of growth. Specifically, gene expression of igf2 decreased by 24 and 168 h, while igfbp1 was increased by 24 h and either decreased or increased by 168 h depending on the doses of EE2 (124). Genes involved in processes related to metabolism and lipid metabolism were downregulated by 168 h (124).
Generally, these acute exposure approaches on growth have mainly employed molecular marker targets, as changes in morphology or phenotype usually manifest after long periods of exposure.
Long-term exposure experiments represent a powerful tool to test EDCs that affect animals after a prolonged period. This approach better reflects the actual exposure of animals in the environment compared with acute exposure experiments.
Similar to acute exposure experiments, a large number of studies characterize the sub-chronic and chronic effects of EDCs on reproduction in adult fish. For instance, waterborne exposure of fish to NP or EE2 for 3 weeks increased plasma VTG and suppressed testicular growth in adult male rainbow trout, (Oncorhynchus mykiss) (109). In adult male and female Japanese medaka (Oryzias latipes), exposure of up to 6 weeks to EE2 or NP induced testis-ova in males, hepatic vtg and choriogenin (chg), ovarian development-related genes in testis, reduction in GSI in males, and decreased egg production and fertility (117, 134). Similarly, in sub-adult coho salmon, a 6-week exposure to EE2 increased hepatic vtg and pituitary lhß subunit mRNA, decreased follicle-stimulating hormone (fsh) β subunit mRNA, and induced gonadotropin synthesis and release-related contigs in females (136). A 3-week exposure of male and female Caspian brown trout (Salmo trutta caspius) to water containing NP resulted in increased levels of plasma E2 and decreased T and FSH levels in both sexes (165). Zebrafish exposed to EE2 for 3 weeks induced hepatic vtg and er mRNA expression (126). In a 60-day dietary exposure study, EE2-fed female largemouth bass (Micropterus salmoides) exhibited decreased plasma VTG, hepatosomatic index (HSI) and GSI, and increased hepatic and gonadal er, and gonadal aromatase (cyp19a) transcripts (138). Induction of forkhead box protein L2 (foxl2), a gene which play a role in ovarian differentiation and in maintenance of ovarian functions in mammals and fish (200–202), was observed after 14 and 28 days of exposure to BPA in females, and after 28 days in males of killifish (Goodea atripinnis) (162). Breeding groups of zebrafish treated with BPA for 15 days exhibited increased egg production, reduced fertilization success, alterations in levels of genes involved in reproductive function and epigenetic processes and reduction in global DNA methylation in both liver and gonad tissue (150). Sperm production, motility and velocity, 11-ketotestosterone (11-KT), and testicular steroidogenic acute regulatory protein (StAR) were reduced following a 15 or 30-day exposure to phthalate in mature male goldfish (Carassius auratus) (146). Female zebrafish exposed to phthalate for 3 weeks exhibited a decrease in ovulation, fecundity, and germinal vesicle breakdown; a decrease in ovarian lhr, progesterone receptors (mPRb), and cyclooxygenase (COX)-2 (ptgs2); and an increase in plasma VTG and ovarian bone morphogenetic protein-15 (BMP15) protein (130). In vivo studies testing the chronic effects of arsenic have also been effective at revealing disruption of reproduction in fish. In banded gourami (Colisa fasciatus), exposure to arsenic for 15 or 30 days, revealed decreased development of oocytes, decreased numbers and diameter of nucleoli, and increased numbers of atretic follicles in females (107). In a similar study, males were observed to have degenerative changes in the testicular lobules, reduction in the diameter of Leydig cells, and necrosis and pyknosis in testis (108). Female zebrafish fed with polychaete worms laden with metals including arsenic for 68 days showed a decrease in hepatic vtg transcripts, cumulative egg production, number of spawns, number of eggs per spawn, and % hatch rate (128).
Since it has only been recently that the risks of MP pollution and their associated chemicals have raised concern, there have been very limited studies on their effects, particularly on reproduction. In one of these studies, feeding adult Japanese medaka with diets containing 10% by weight marine-treated polyethylene virgin pellets for 2 months downregulated hepatic chg gene expression in males and hepatic vtg, chg and erα gene expression in females (142). Although this study only shows the effects of plastic treated with marine water, it suggests that MPs at environmentally relevant concentrations may indirectly alter endocrine system function in adult. A detailed overview of the studies conducted on the various effects of MPs on aquatic organisms can be found elsewhere (203).
While most research has focused on reproduction as an endpoint of EDC’s chronic effects, a limited number of studies have examined growth effects of selected EDCs in fish. Only few studies have been conducted so far on the effects of prolonged exposure to NP and EE2 on growth of sub-adult or adult fish. Nevertheless, some studies clearly showed effects of these EDCs on biomarkers of growth. For instance, in adult zebrafish exposed to EE2 for 3 weeks, hepatic igfbp1 mRNA was downregulated (126). In a chronic arsenic toxicity study in the euryhaline and warmwater fish, Mozambique tilapia (Oreochromis mossambicus), aqueous exposure to sodium arsenite for 4 weeks decreased the growth rate of males in a dose-dependent manner (121).
Overall, these chronic and sub-chronic in vivo exposure approaches are capable of detecting not only the changes in the expression of growth and reproductive genes and proteins, but also phenotypic alterations associated with the exposure of sub-adults and adults to EDCs.
Examining the effects of EDCs during early life stages allows for the detection of abnormalities across the lifespan of fish, helping to clarify the sensitivity to specific chemicals during larval stages and long-term developmental effects. In some early-life stage experimental approaches, for example, the long-lasting effects of EDCs can be examined either right after exposure and/or after a period of depuration, even after fish have reached an adult stage. An example of an early-life, in vivo approach illustrating the methodology used for exposing Mozambique tilapia fry to NP and E2 and sampling after depuration is shown in Figure 1. Approaches employing larval fish also reduce the experimental footprint, or the effort and costs associated with the study, as they usually require smaller rearing and exposure containers (Figure 1). The lower the experimental footprint, the lower the effort and costs to conduct the study. In some studies, even petri dishes have been used for exposure experiments (133, 143). For acute exposures, however, particularly during very early stages the small size of the fish will restrict the amount and type of tissue samples and thereby limit the number and specificity of targets that can be used for analyses. It can also be difficult, if not impossible, to identify and separate sexes at early stages of development. In some cases, the challenge of having a limited amount of tissue was resolved by using the whole animal (133, 139, 166), although with this approach it is not possible to specify the organ or tissue from which gene expression or protein levels originate. In this case, methods such as in situ hybridization can be carried out in parallel to determine the expression patterns in different organs. If the whole animal is used, however, it is not possible to conduct this analysis in the same fish from which gene expression was determined. Moreover, determining the effects of separate sexes can be achieved by allowing the fish to grow to a stage by which sex can be identified. The high mortality rates during early developmental stages in fishes (204) also present a further challenge to this experimental strategy.
Figure 1 Schematic illustration depicting an experimental design of an in vivo exposure study aiming to assess the long-term developmental effects of early-life exposure to EDCs in fish [adapted from Celino-Brady et al. (157), with author’s permission]. This can be customized for a wide variety of species and EDCs. Yolk-sac fry are first allowed to acclimate to the experimental tanks and are then exposed to chemicals after partial or full yolk-sac resorption. Following the exposure to EDCs, fish are reared in EDC-free water (depuration) before sampling. In this experimental set up, both header and fish tanks are lined with modified polytetrafluoroethylene (MPTFE) to prevent the leaching of chemicals from the plastic containers. E2, 17β-estradiol; NP, nonylphenol. Black squares = aeration, white rectangles = pipes.
Acute exposures in early-life stages like in adult stages can cut down experimental footprints. Conducting this approach during very early stages, however, would make the size of the fish as a drawback limiting the number and specificity of biomarkers that can be analyzed. Below are some of the representative studies that used this type of exposure method.
Relatively short-exposure periods (days) were conducted in salmonid species. After 3 or 6 days of exposure to NP, hepatic vtg and erα mRNA levels were increased, while brain gnrh2 mRNA were reduced in a dose-dependent manner in juvenile rainbow trout (125). Moreover, exposure to EE2 and NP for 4 days, elevated vtg transcripts in yolk-sac larvae, fry and smolts, and increased plasma VTG and erα mRNA levels in Atlantic salmon (Salmo salar) smolts (42, 139). An interesting experiment in which rainbow trout oocytes were exposed to BPA for 3 h in ovarian fluid so as to mimic maternal transfer, resulted in delay in hatching and yolk-absorption in embryo (129). Low levels of BPA exposure at 2 h post fertilization (hpf) to 120 hpf caused advanced hatching time, increased numbers of GNRH3 neurons and increased expression of reproduction-related genes such as kisspeptin1 (kiss1) kiss receptor, (kiss1r), gnrh3, lh, fsh, and er in zebrafish larvae (152). In fathead minnow, sex steroid hormones (E2 and T) either increased or decreased after 96 h exposure of eggs to different types of phthalates (137).
Acute exposure of Atlantic salmon smolts to EE2 or NP for 4 days also impacted growth. EE2 diminished plasma GH and IGF1 levels in parallel with reductions in hepatic ghr and igf1 (42). Smolt weights and plasma IGF-I levels were reduced by NP by 5 days of exposure (119). Rainbow trout embryo exposed to BPA for 3 h as oocytes exhibited growth impairment, increase in GH levels, and lower gene expression ghr, igfs and igf receptors (igfrs) (129). In a similar experiment, a decrease in the specific growth rate and food conversion ratio in rainbow trout larvae reared from BPA-treated eggs were observed. BPA also disrupted the mRNA levels of gh isoforms, ghr, igfs, igfr in a life stage-dependent manner (144). Zebrafish embryos exposed up to 120 hpf to arsenic had elevated GH levels, and reduced whole body ghr, igf2, igfbp3, igfbp2a, and igfbp5b (166). Although there is insufficient knowledge on the effects of MPs on growth, a one-week acute exposure study showed that growth was negatively affected by MPs in juvenile spiny chromis damselfish (Acanthochromis polyacanthus) (154). Nonetheless, the reduction in growth as a consequence of a reduction in feeding cannot be explicitly ruled out in this study. More studies designed to elucidate the direct effects and modes of action of MPs on growth are needed.
Chronic exposure approaches, although an effective tool for assessment of EDCs with delayed effects, have their own disadvantages if conducted during early stages of development. For example, prolonged exposure to chemicals can magnify the high mortality rates that are already a natural challenge during early-life development in fishes.
There have been more studies performed on the chronic early-life exposure effects of EDCs on the reproduction of fishes when compared with investigations of acute early-life effects. Long-term exposure (1 year) of rainbow trout to NP, from eyed-egg to the juvenile stage, resulted in the elevation of hepatic VTG expression and zona radiata protein (ZRP) (115). In a similar approach, fathead minnow embryos were continuously exposed to EE2 until swim-up stage, resulting in an increase in lh and vtg (133). Immature mixed sex Atlantic salmon injected with E2 or NP had higher HSI and elevated levels of plasma VTG by 30 days (110). In the same species, juvenile exposure to waterborne NP for 21 days induced plasma VTG in both males and females (36). Exposure of Atlantic salmon fry to EE2 or NP for 21 days resulted in increased hepatic vtg mRNA levels and decreased hepatic erα mRNA levels (42). In Mozambique tilapia, exposure of yolk-sac fry to waterborne NP for 21 days also resulted in alterations in reproductive-related genes. Specifically, a decrease in HSI, and stimulation of hepatic erα and erβ were observed in adult male fish 112 days after the end of the early-life exposure period (157). In Nile tilapia fry fed diets containing EE2 from 10–40 days post fertilization (dpf), sex ratio become skewed towards females (127). Three-month old goldfish subjected to aqueous exposure to BPA for 30 days demonstrated diminished ovarian maturation, decreased brain gnrh, fshβ and lhβ in females, and reduced GSI and testicular ar levels in male. Moreover, a decrease in 11-KT levels were also observed in BPA-exposed fish. The disruption of testicular development was not recovered after BPA withdrawal (161).
When fish are subjected to long-duration treatments with EDCs, their growth or growth-related physiological markers are also altered. Dietary exposure of Nile tilapia fry to EE2 from 10–40 dpf decreased serum IGF-I, hepatic igf1 mRNA, the number of hepatocytes expressing igf1, and pituitary gh by 75 dpf. Both body length and weight also decreased after 90 to 165 dpf (127). In Atlantic salmon, fry subjected to waterborne EE2 or NP exposure for 21 days had decreased hepatic ghr, igf1 and igf2 mRNA levels, reduced body mass and total length, and varying effects on various igfbp transcript levels (42). Intraperitoneal injection of NP or E2 within a 10-day period in Atlantic salmon smolts decreased both plasma IGF-I and GH levels (125). Following a 21-day exposure of Mozambique tilapia yolk-sac fry to waterborne NP, stimulation of hepatic igf1, igfbp1b, and igfbp2b in adult male fish exposed as yolk-sac fry was observed (157). Sixty-day exposure of juvenile swordtail fish to both NP and BPA significantly reduced growth (112). Continuous exposure of less than one-week-old guppy (Poecilia reticulata) to phthalate for 14–91 days reduced body weight and body length (131). In juvenile starry flounder (Platichthys stellatus), daily length and weight gain, and condition factor decreased following 2 and 4 weeks of aqueous exposure to arsenic (159).
While these studies subjected fish to relatively long-term exposures (several weeks), the main difference between experimental designs is that most of the studies analyzed the effects of EDCs immediately following exposure (36, 42, 115, 133), while some assessed the effects of EDCs after a period of depuration in which fish were no longer fed with EDC-containing feed or moved to EDC-free water (127, 157). Notwithstanding, all of these studies showed significant changes in biomarkers of growth and reproduction following exposure to EDCs. In summary, studies employing both acute and chronic exposure protocols on fish at early-life stages were effective at detecting various impacts of EDCs on growth and reproduction.
A number of studies in fish provide evidence of the disruptive effects of EDCs that span across parental and filial generations. Evidence from recent studies suggests that exposure to EDCs can directly impact not only the exposed individual, but also unexposed future progenies. This process is called transgenerational inheritance (167, 169). Transgenerational experiments only involve exposure of the parental or F0 generation to EDCs, with no exposure of subsequent generations. This can provide information on both direct and indirect consequences of EDC exposure. Furthermore, transgenerational effects are not limited to epigenetic changes such as DNA methylation, histone modification, and expression of non-coding RNAs (205) but also include the effects of the direct transfer of compounds via egg yolk and oocyte reserves. The main outcomes of determining the effects of parental exposure to EDCs on offspring have been highly variable and not strictly limited to endpoints of growth and reproduction. Our discussion focuses on the growth and reproduction-related biological markers and epigenetic changes. Moreover, only few studies employing a transgenerational approach have been conducted on the EDCs included in this review, hence this section is divided into acute and sub-chronic/chronic exposure.
Acute exposures to EDCs have been shown to affect filial generations in transgenerational studies. In Japanese medaka, aqueous EE2 and BPA treatments of late blastula stage for 7 days resulted in reduced fertilization rates in the F2 generation, and decreased embryo survival in F3 offspring (143). In vivo treatment of rainbow trout F0 oocyte with BPA for 3 h caused reduction in body mass in F1 generation. The long-term changes seen in the liver transcriptome of progenies raised from BPA-exposed eggs suggest epigenetic modifications (153).
In rainbow trout, male and female adults exposed to NP for 3 months induced an increase in plasma VTG in parental (F0) males, reduced hatching rates of eggs from F0 parents and elevated intersex frequency, and hormonal imbalance in male and female offspring (116). Exposure of adult Japanese medaka male and female pairs to NP for 21 days resulted in reduced egg production and fertility, elevated hepatic VTG levels in F0 males, and reduced hatchability and time to hatching of the F1 generation (66). In fathead minnow, rearing adult males and females in tanks with EE2 for 102 days caused reductions in F0 male survival and F1 juvenile production, reproductive failure or reduced reproductive capability in F1 adults and reduction in survival rates of F2 offspring (60). In inland silversides (Menidia beryllina), exposure to low levels of EE2 from 8 hpf to 21 days post hatch (dph), led to a female-biased sex ratio, higher number of atretic follicles, reduced 17β-hydroxysteroid dehydrogenases (17β-hsd), and increased brain cytochrome P450 aromatase (cyp19b) in the F0 generation. In the F1 generation, hatching success increased, while larval length, and egg production decreased. Larval survival rate was reduced and larval length increased in the F2 generation. Moreover, differential methylation of 17β-hsd and ar was found across generations (163). A similar study on the same species exposed to EE2 up to 21 dph, revealed changes in biological processes and pathways involved in growth, development and reproduction as assessed by GO and pathway analyses, and methylation of hormone receptors, steroidogenesis, and sexual development-related genes across generations (164). In fathead minnow, BPA inhibited body length and body weight in F0 males after up to 164 days of exposure. Plasma VTG levels in F0 parents were also elevated after 164 days of exposure. Egg production and hatchability were inhibited in the F1 generation after 164-days exposure (113). Furthermore, zebrafish chronically subjected to waterborne arsenic for 6 months had progeny whose body mass was lower than those from untreated parents (148). Marine medaka (Oryzias melastigma) exposed for 60-days to MPs showed a number of deleterious effecs including: 1) retardation of gonadal maturation, reduced plasma E2 and T, and decreased GSI of F0 female fish; 2) decrease in HSI and GSI in F0 males; 3) varying effects on gonadotropins and steroid synthesis-related genes in F0 parents; 4) increase in hepatic vtg and chg in F0 males; and 5) decrease in hatching rate and body length in progeny (160). As seen with other aforementioned MP studies, these effects may only be possible due to the leaching of plasticizers or to non-endocrine disrupting mechanisms, such as cell-toxicity, tissue-injury, or feeding inhibition.
The results from these transgenerational experiments show that acute EDC exposures are enough to induce transgenerational effects when parents are exposed at early stages. On the other hand, experiments carried out during adult stages may require longer exposure periods (weeks or months) to reveal transgenerational changes. Remarkably, these studies show that both F1 fish exposed to EDCs indirectly as primordial germ cells within F0 parents, and F2 animals not directly exposed to EDCs, exhibit physiological alterations induced by the parental EDC exposure.
While in vivo experimental approaches take into account all physiological processes and interactions occurring in the animal upon EDCs exposure, in vitro approaches, on the other hand, are helpful in determining the direct effects of chemicals on a specific tissue or cell type. Since in vitro studies are inherently more controlled and include fewer confounding factors, they are suitable to determine specific mechanisms of action of EDCs under near-ideal conditions. Experimental manipulation of cellular pathways such as addition of specific inhibitors or activators, can help pinpoint the specific molecular target/s of EDCs within the cell, a key step in categorizing an EDC. These in vitro culture systems (exemplified in Figure 2) can address the effects of a wide variety of EDCs on cells or organs when coupled with methods to measure morphological changes, disruption on cell development and hormone synthesis related to growth and reproduction. In contrast to in vivo approaches, in vitro experimental designs are more temporally constrained as most cell or organ incubations have limited viability. As such, exposure time varies in in vitro systems based on viability of cells and the amount of time required to induce an effect on specific markers or responsive elements. An overview of several in vitro assays for substances possessing endocrine activity in fish is also provided by Scholz et al. (207). Some in vitro approaches are routinely used for screening candidate EDCs and wastewater samples (208–216), and used as a guide for a higher tier EDC test (in vivo experiments and field studies) in a tiered strategy (Figure 3). These in vitro screening assays include cell proliferation assays (E-screen assays), yeast-based screens and reporter gene assays that utilize fish and human cell lines, including human breast cancer cells. Further information on this tiered approach is discussed elsewhere (217, 218). We divide this section into cell/organ culture experiments and binding assays. Below, in vitro studies designed to analyze the direct effects of EDCs on hormone synthesis, ER/er, VTG/vtg, and GH/IGF-related factors, and to validate the endocrine disrupting potency of complex environmental chemical mixtures are discussed.
Figure 2 Schematic illustration of a gonadal in vitro culture system that can be utilized for analyzing direct effects of a variety of compounds or hormones on germ cell growth and development, as well as gonadal gene expression in different teleost species [illustration modified from Miura and Miura (206), with author’s permission]. Testis or ovary are first cut into small fragments, and then placed on a nitrocellulose membrane set on top of agarose gel cylinders for long-term incubations, or directly placed in the wells for short-term incubations. Testicular or ovarian fragments are cultured in medium with or without EDCs, hormone, or steroid hormone precursors for up to 30 days. Endpoint analyses correspond to immunoassays, histology, immunohistochemistry, and gene expression assays. *Usually utilized for long culture periods.
Figure 3 Schematic illustration of an in vitro system used for screening of potential EDCs and testing for estrogenicity of waste water effluents in a tiered strategy. These screening assays utilize fish and human cell lines, yeast cells, and human breast cancer cells for the first tier. The results are then validated in the lab by in vivo testing, or further validated by examining phenotypic or genotypic changes in fish inhabiting contaminated areas.
A short incubation (18 h) of testicular fragments with arsenic, steroid hormone precursors, and human chorionic gonadotropin (hCG) suppressed hCG-induced synthesis of 11-KT and inhibited progesterone synthesis from pregnenolone (40). Bream (Abramis brama) and carp hepatocytes treated with EE2 for 4 days showed concentration-dependent induction of VTG (220). Primary hepatocyte cultures have also been employed to characterize the effects of EE2 on growth-related factors. In rainbow trout juveniles, for example, hepatocytes treated with EE2 for 48 h showed a concentration-dependent upregulation of er and vtg and downregulation of ghr1 and igfbp1 (145). Treatment of testicular fragments of Japanese eel (Anguilla japonica) with NP with or without 11-KT for 15 or 30 days stimulated early spermatogonial renewal, induced hypertrophy in Sertoli cells and decreased the number of germ cells (123). Using the same in vitro system, testicular fragments cultured with arsenic in the presence or absence of hCG for 6 or 15 days elicited a dose-dependent inhibition of hCG-induced germ cell proliferation and induced apoptosis and oxidative DNA damage in germ cells (40). Some studies have coupled in vitro cell screening assays with in vivo approaches to provide a more comprehensive understanding of the effects of EDCs or to validate EDC potencies of wastewater treatment plant effluents (WWTPE) in fish. E-screen assays which is based on proliferation of human breast cancer cells MCF-7 is used to analyze the estrogenicity of water, sediment and WWTPE. This in vitro assay supported the results of in vivo experiments using juvenile brown trout (Salmo trutta f. fario) (140). Rainbow trout and carp hepatocyte assays were used to examine induction of VTG by EE2 and other compounds to predict their estrogenicity supporting the in vivo life cycle tests using zebrafish (118).
An in vitro screening assay using a recombinant yeast system expressing rainbow trout ER and a 48 h trout hepatocyte culture showed ER affinity and induction of hepatocyte vtg by NP, respectively (111). In another in vitro assay, estrogenic environmental chemicals were screened using the rainbow trout gonad cell line, RTG-2, containing erα complementary DNA (rtErα cDNA). Results indicated that EE2, NP and sewage treatment effluent extract had estrogenic activity (114). Other in vitro screening assays for assessing estrogenic activity in sewage treatment effluents are described in Rutishauser et al. (219). Miyagawa et al. (141) developed customized in vitro ERα reporter gene assays using HEK293 cells as hosts for ERα of various fish species [medaka, common carp, goldfish guppy, stickleback (Gasterosteus aculeatus), bluegill (Lepomis macrochirus), fathead minnow, roach (Rutilus rutilus), and zebrafish] to analyze the ligand- and species-specificity for several estrogenic chemicals, including NP and BPA. NP and BPA induced the transactivation of ERα from all species of fish tested.
Several binding assays were also used in combination with in vivo experiments in a tiered approach. For instance, in zebrafish, in vitro screening assays using transfected human breast cancer cells (MVLN) containing an estrogen response element coupled to a luciferase reporter gene, and an estrogen-inducible expression system in yeast cells integrated with human estrogen receptor (YES screen) were used for dose-range and estrogenic potency studies to determine concentrations to be used in in vivo assays. EE2 and NP were found to be estrogenic in both in vitro assays and were able to induce plasma VTG and reduce the GSI in female zebrafish, in vivo (122). In another experiment, in vitro luciferase reporter assays employing human cell lines (HeLa-9903 and MDA-kb2) to detect ER or AR were used to analyze the endocrine activity of water, sediment, and WWTPE. The reproduction-disrupting effects revealed by these in vitro assays were subsequently corroborated by in vivo experiments using juvenile brown trout, thereby underscoring the power of high throughput in vitro approaches to narrow down the targets for endocrine disruption observed in field-exposed fish (140). In Segner et al. (118), the estrogenicity of compounds, including EE2, was tested by recombinant yeast ER assay, a carp hepatic ER competitive radioreceptor assay, and in vivo life cycle tests using zebrafish. In this study, EE2 estrogenicity was confirmed and the in vitro assay was found to be the best predictor of the in vivo test.
Unlike most in vivo approaches, these in vitro assays are usually rapid, cost-effective for the output of chemicals screened and can be performed under controlled environments, thereby eliminating the possibility of contamination by chemicals other than selected compounds to be tested. Some studies incorporate mammalian cell lines and luciferase reporter assays into in vitro approaches to evaluate dose responses of potential EDCs that can be used to test in fish models. Most of these in vitro tests have been designed to detect reproduction-related genes. For detecting growth-related genes, in vitro assays employing primary hepatocyte cultures have served as a good model for assessing the effects of EDCs on the GH/IGF system in fish (145). A combination of these in vitro tests provides a comprehensive perspective of the impact of EDCs on the growth and reproductive physiology of fish.
Computational methods used in drug development have been recently applied in EDCs research. These in silico approaches (illustrated in Figure 4) have the capacity to detect potential EDCs without the use of animals or cells, largely serving as an initial screening strategy that also attenuates the cost and time constraints of in vivo and in vitro approaches. So far, in fish, most computational models used structure–activity relationship methods which correlate the chemical structure of compounds with biological activity (Figure 4). The biological activity is determined through a number of molecular descriptors such as molecular weight, hydrophobicity, topology, or electronic properties (220). Based on these descriptors, the estrogenicity, androgenicity, lipophilicity, and LC50 are then predicted (Figure 4). Comprehensive descriptions of various in silico methods suitable for EDC screening and research have been recently reviewed (220–222). In this section we discuss in silico approaches that have been used in EDC studies, including quantitative structure property relationship (QSAR), structural alerts or pharmacophore modeling, and molecular docking. QSAR prediction models have been developed to predict a particular activity or property of the molecule (e.g., molecular weight) of interest. Pharmacophore modeling detect features within the binding cavity that would ensure interactions between the biological target (receptor) and the potential ligands based on receptor structures (223–225) or aligns a set of known ligands in the absence of receptor structure to observe common features that may predict biological activity (226). Molecular docking, on the other hand, involves prediction of the ligand conformation and its position and orientation within binding sites and assessment of the binding affinity (227). This approach is utilized as a model for predicting the interaction between a small molecule and a protein at the atomic level, allowing for characterization of the behavior of molecules in the binding site of target proteins (228). Nevertheless, in silico methods have been only recently applied to predicting the effects of EDCs in fish and studies are scarce.
Figure 4 Schematic illustration of a QSAR method for screening of EDCs [adapted from Bohlen et al. (156), with author’s permission]. This type of in silico approach used a structure-activity relationship correlating the chemical features of compounds with their biological activity. The resulting data can then be used to predict the estrogenic/androgenic potential, toxicity, and lipophilicity of a compound or a complex mixture.
Potential EDCs in fish were screened using data from structural alerts and in vitro and in vivo toxicological assays (151). Structural alerts represent potential estrogenic and androgenic endocrine activities based on in vitro studies and are used for indicating potential estrogenic and androgenic endocrine disruptors in fish. The model generated was then applied to a database of commercial substances to search for chemicals with estrogenic or androgenic effects. The combination of these methods was found to have a potential to further assess candidate estrogenic and androgenic EDCs. In largemouth bass, transcriptome profiling data was coupled with computational analysis to model the effects of chemicals in the ovary. First, a dynamic model representing the development of healthy ovaries from unexposed fish was constructed, and the responses of the transcriptomes from fish collected from polluted areas were mapped to enable the identification of clusters of genes affected at different stages of ovarian development. Then, using a robust database that provides information on chemical interaction with genes, proteins, and diseases (Comparative Toxicogenomic Database), potential chemicals associated with specific molecular responses on the ovary were identified (155). Another study, applied the QSAR method utilizing chemical features and mode of action to predict acute toxicity effects of EDCs in fish. This study, among other outcomes, determined that estrogen receptor binding affinity is dependent on the relationship between lipophilicity (expressed as octanol-water partition coefficient, Kow) and LC50, and that both EE2 and NP strongly bind to the ER (156).
A molecular docking approach is relevant for predicting the binding of EDCs to hormone receptors and binding proteins. It has been used to obtain insights on the molecular interactions of environmental xenoestrogens (229). In killifish, a molecular docking approach demonstrated that BPA interacted with residues in foxl2 (162). Chen et al. (158) evaluated the estrogenic potentials of NP and BPA through the use of molecular docking analysis in conjunction with a reduced life expectancy model in adult zebrafish. The analysis revealed an interaction potential of both NP and BPA to ERα. Another study employing a 3-D structure-based computational method, showed that the ligand binding domains of medaka ERs are similar in architecture and have conserved amino acid residues that interact with E2; the authors also found that NP and BPA form hydrogen bonds with medaka ER1 (147).
These and other emerging computer-assisted technologies shall allow for prediction of endocrine disrupting activities of chemicals with increasingly greater power and specificity and complement the toolbox of approaches used for screening EDCs, in vitro.
In this review, we provided an overview of studies employing different approaches to evaluate effects of select endocrine disrupting compounds. The results from these studies showed that common aquatic pollutants that are weakly estrogenic such as NP, can elicit long-term endocrine disrupting responses. Not only are EDCs long-lasting in contaminated environments due to their stable chemical structures, their effects can persist over the lifetime of an organism and in unexposed progenies of exposed parents.
In vivo approaches in various fish species are used to evaluate a wide range of consequences from exposure to EDCs including effects on body and organ weight, cell differentiation and growth, protein and gene expression and enzyme activities, among other endpoints. They tend to be, however, labor- and cost-intensive and unsuitable for large-scale screening. In vivo experiments can also provide more direct inferences to actual changes in biological activity but cannot, however, elucidate cellular mechanisms involved in EDCs exposure. By contrast, in vitro assays usually take into account a limited number of processes impacted by EDCs, but allow for assessment of the direct effects of chemicals on a specific tissue or cells with high screening throughput, though, testing a wide range of potential EDCs in vitro can be time consuming. Moreover, it is challenging to make direct inferences of biological activity at an organismal level from in vitro approaches. Last, in silico approaches offer an alternative strategy for rapid and robust screening while optimizing best practices for considering the use and care of animals in research, summarized by refinement, reduction and replacement (“3R’s”; 230). In silico approaches, however, still need further validation in vivo. Together, the results from various studies employing different approaches underscore the severity of EDC exposure in wild populations of fish. Alone or combined, in silico, in vitro and in vivo approaches shall continue to provide robust assessments of endocrine activity or adverse effects of EDCs on multiple physiological processes of aquatic organisms, including fish. Ultimately, collaborative efforts would enable the assessment of EDC’s effects by employing all three approaches to provide a more comprehensive understanding of the underlying processes involved in the exposure to EDCs or to evaluate potencies of wastewater effluents. The outcome of these comprehensive studies could in turn demonstrate the biological changes that impact the survival of aquatic organisms, thereby informing government agencies in making effective policy decisions.
FC-B and AS conceived the work. FC-B drafted the original manuscript and prepared the figures and table. FC-B, AS, and DL revised the manuscript. AS supervised the work. All authors contributed to the article and approved the submitted version.
This work was funded in part by grants from the National Science Foundation (IOS-1755016), the National Oceanic and Atmospheric Administration (NA18OAR4170347) to AS and DL, the National Institute of Diabetes and Digestive and Kidney Diseases (1R21DK111775-01), and the National Institute of Food and Agriculture (Hatch no. HAW02051-H) to AS.
The authors declare that the research was conducted in the absence of any commercial or financial relationships that could be construed as a potential conflict of interest.
1. Colborn T, Dumanoski D, Myers JP. Our Stolen Future: Are We Threatening Our Fertility, Intelligence, and Survival? A Scientific Detective Story. NewYork: Dutton (1996).
2. Oberdorster E, Cheek AO. Gender benders at the beach: endocrine disruption in marine and estuarine organisms. Environ Toxicol Chem (2001) 20(1):23–36. doi: 10.1002/etc.5620200103
3. Kavlock RJ, Daston GP, DeRosa C, Fenner-Crisp P, Gray LE, Kaattari S, et al. Research needs for the risk assessment of health and environmental effects of endocrine disruptors: a report of the U.S. EPA-sponsored workshop. Environ Health Perspect (1996) 104 Suppl 4:715–40. doi: 10.1289/ehp.96104s4715
4. Diamanti-Kandarakis E, Bourguignon JP, Giudice LC, Hauser R, Prins GS, Soto AM, et al. Endocrine-disrupting chemicals: an Endocrine Society scientific statement. Endocr Rev (2009) 30(4):293–342. doi: 10.1210/er.2009-0002
5. La Merrill MA, Vandenberg LN, Smith MT, Goodson W, Browne P, Patisaul HB, et al. Consensus on the key characteristics of endocrine-disrupting chemicals as a basis for hazard identification. Nat Rev Endocrinol (2020) 16(1):45–57. doi: 10.1038/s41574-019-0273-8
6. Tyler CR, Jobling S, Sumpter JP. Endocrine disruption in wildlife: a critical review of the evidence. Crit Rev Toxicol (1998) 28(4):319–61. doi: 10.1080/10408449891344236
7. Vos JG, Dybing E, Greim HA, Ladefoged O, Lambre C, Tarazona JV, et al. Health effects of endocrine-disrupting chemicals on wildlife, with special reference to the European situation. Crit Rev Toxicol (2000) 30(1):71–133. doi: 10.1080/10408440091159176
8. Darbre PD. Endocrine Disruptors and Obesity. Curr Obes Rep (2017) 6(1):18–27. doi: 10.1007/s13679-017-0240-4
9. Street ME, Angelini S, Bernasconi S, Burgio E, Cassio A, Catellani C, et al. Current Knowledge on Endocrine Disrupting Chemicals (EDCs) from Animal Biology to Humans, from Pregnancy to Adulthood: Highlights from a National Italian Meeting. Int J Mol Sci (2018) 19(6):1647. doi: 10.3390/ijms19061647
10. Colborn T, vom Saal FS, Soto AM. Developmental effects of endocrine-disrupting chemicals in wildlife and humans. Environ Health Perspect (1993) 101(5):378–84. doi: 10.1289/ehp.93101378
11. Sharpe RM, Skakkebaek NE. Are oestrogens involved in falling sperm counts and disorders of the male reproductive tract? Lancet (1993) 341(8857):1392–5. doi: 10.1016/0140-6736(93)90953-e
12. Bay K, Asklund C, Skakkebaek NE, Andersson AM. Testicular dysgenesis syndrome: possible role of endocrine disrupters. Best Pract Res Clin Endocrinol Metab (2006) 20(1):77–90. doi: 10.1016/j.beem.2005.09.004
13. Sharpe RM. Pathways of endocrine disruption during male sexual differentiation and masculinization. Best Pract Res Clin Endocrinol Metab (2006) 20(1):91–110. doi: 10.1016/j.beem.2005.09.005
14. Crain DA, Janssen SJ, Edwards TM, Heindel J, Ho SM, Hunt P, et al. Female reproductive disorders: the roles of endocrine-disrupting compounds and developmental timing. Fertil Steril (2008) 90(4):911–40. doi: 10.1016/j.fertnstert.2008.08.067
15. Darbre PD. Environmental oestrogens, cosmetics and breast cancer. Best Pract Res Clin Endocrinol Metab (2006) 20(1):121–43. doi: 10.1016/j.beem.2005.09.007
16. Alavanja MC, Samanic C, Dosemeci M, Lubin J, Tarone R, Lynch CF, et al. Use of agricultural pesticides and prostate cancer risk in the Agricultural Health Study cohort. Am J Epidemiol (2003) 157(9):800–14. doi: 10.1093/aje/kwg040
17. Mahajan R, Bonner MR, Hoppin JA, Alavanja MC. Phorate exposure and incidence of cancer in the agricultural health study. Environ Health Perspect (2006) 114(8):1205–9. doi: 10.1289/ehp.8911
18. Baillie-Hamilton PF. Chemical toxins: a hypothesis to explain the global obesity epidemic. J Altern Complement Med (2002) 8(2):185–92. doi: 10.1089/107555302317371479
19. Newbold RR, Padilla-Banks E, Snyder RJ, Jefferson WN. Developmental exposure to estrogenic compounds and obesity. Birth Defects Res A Clin Mol Teratol (2005) 73(7):478–80. doi: 10.1002/bdra.20147
20. Grun F, Watanabe H, Zamanian Z, Maeda L, Arima K, Cubacha R, et al. Endocrine-disrupting organotin compounds are potent inducers of adipogenesis in vertebrates. Mol Endocrinol (2006) 20(9):2141–55. doi: 10.1210/me.2005-0367
21. Bond GG, Dietrich DR. Human cost burden of exposure to endocrine disrupting chemicals. A critical review. Arch Toxicol (2017) 91(8):2745–62. doi: 10.1007/s00204-017-1985-y
22. Baroiller JF, Guiguen Y, Fostier A. Endocrine and environmental aspects of sex differentiation in fish. Cell Mol Life Sci (1999) 55:910–31. doi: 10.1007/s000180050344
23. Koopman P. The genetics and biology of vertebrate sex determination. Cell (2001) 105(7):843–7. doi: 10.1016/s0092-8674(01)00408-1
24. Munday PL, Buston PM, Warner RR. Diversity and flexibility of sex-change strategies in animals. Trends Ecol Evol (2006) 21(2):89–95. doi: 10.1016/j.tree.2005.10.020
25. Munday PL, White JW, Warner RR. A social basis for the development of primary males in a sex-changing fish. Proc Biol Sci (2006) 273(1603):2845–51. doi: 10.1098/rspb.2006.3666
26. Guerrero-Estévez S, Moreno-Mendoza N. Sexual determination and differentiation in teleost fish. Rev Fish Biol Fish (2010) 20(1):101–21. doi: 10.1007/s11160-009-9123-4
27. Zoeller RT, Brown TR, Doan LL, Gore AC, Skakkebaek NE, Soto AM, et al. Endocrine-disrupting chemicals and public health protection: a statement of principles from The Endocrine Society. Endocrinology (2012) 153(9):4097–110. doi: 10.1210/en.2012-1422
28. Kelce WR, Stone CR, Laws SC, Gray LE, Kemppainen JA, Wilson EM. Persistent DDT metabolite p,p’-DDE is a potent androgen receptor antagonist. Nature (1995) 375(6532):581–5. doi: 10.1038/375581a0
29. Kuiper GG, Carlsson B, Grandien K, Enmark E, Haggblad J, Nilsson S, et al. Comparison of the ligand binding specificity and transcript tissue distribution of estrogen receptors alpha and beta. Endocrinology (1997) 138(3):863–70. doi: 10.1210/endo.138.3.4979
30. Clark EJ, Norris DO, Jones RE. Interactions of gonadal steroids and pesticides (DDT, DDE) on gonaduct growth in larval tiger salamanders, Ambystoma tigrinum. Gen Comp Endocrinol (1998) 109(1):94–105. doi: 10.1006/gcen.1997.7013
31. Rasier G, Parent AS, Gerard A, Lebrethon MC, Bourguignon JP. Early maturation of gonadotropin-releasing hormone secretion and sexual precocity after exposure of infant female rats to estradiol or dichlorodiphenyltrichloroethane. Biol Reprod (2007) 77(4):734–42. doi: 10.1095/biolreprod.106.059303
32. Thomas P, Dong J. Binding and activation of the seven-transmembrane estrogen receptor GPR30 by environmental estrogens: a potential novel mechanism of endocrine disruption. J Steroid Biochem Mol Biol (2006) 102(1-5):175–9. doi: 10.1016/j.jsbmb.2006.09.017
33. Hurst CH, Waxman DJ. Activation of PPARalpha and PPARgamma by environmental phthalate monoesters. Toxicol Sci (2003) 74(2):297–308. doi: 10.1093/toxsci/kfg145
34. Lyche JL, Nourizadeh-Lillabadi R, Almaas C, Stavik B, Berg V, Skare JU, et al. Natural mixtures of persistent organic pollutants (POP) increase weight gain, advance puberty, and induce changes in gene expression associated with steroid hormones and obesity in female zebrafish. J Toxicol Environ Health A (2010) 73(15):1032–57. doi: 10.1080/15287394.2010.481618
35. Migliarini B, Piccinetti CC, Martella A, Maradonna F, Gioacchini G, Carnevali O. Perspectives on endocrine disruptor effects on metabolic sensors. Gen Comp Endocrinol (2011) 170(3):416–23. doi: 10.1016/j.ygcen.2010.11.025
36. Lerner DT, Bjornsson BT, McCormick SD. Aqueous exposure to 4-nonylphenol and 17beta-estradiol increases stress sensitivity and disrupts ion regulatory ability of juvenile Atlantic salmon. Environ Toxicol Chem (2007) 26(7):1433–40. doi: 10.1897/06-451R1.1
37. Lerner DT, Bjornsson BT, McCormick SD. Larval exposure to 4-nonylphenol and 17beta-estradiol affects physiological and behavioral development of seawater adaptation in Atlantic salmon smolts. Environ Sci Technol (2007) 41(12):4479–85. doi: 10.1021/es070202w
38. Ankley GT, Bencic DC, Breen MS, Collette TW, Conolly RB, Denslow ND, et al. Endocrine disrupting chemicals in fish: developing exposure indicators and predictive models of effects based on mechanism of action. Aquat Toxicol (2009) 92(3):168–78. doi: 10.1016/j.aquatox.2009.01.013
39. Bernanke J, Kohler HR. The impact of environmental chemicals on wildlife vertebrates. Rev Environ Contam Toxicol (2009) 198:1–47. doi: 10.1007/978-0-387-09647-6_1
40. Celino FT, Yamaguchi S, Miura C, Miura T. Arsenic inhibits in vitro spermatogenesis and induces germ cell apoptosis in Japanese eel (Anguilla japonica). Reproduction (2009) 138(2):279–87. doi: 10.1530/REP-09-0167
41. Bhandari RK, Deem SL, Holliday DK, Jandegian CM, Kassotis CD, Nagel SC, et al. Effects of the environmental estrogenic contaminants bisphenol A and 17alpha-ethinyl estradiol on sexual development and adult behaviors in aquatic wildlife species. Gen Comp Endocrinol (2015) 214:195–219. doi: 10.1016/j.ygcen.2014.09.014
42. Breves JP, Duffy TA, Einarsdottir IE, Bjornsson BT, McCormick SD. In vivo effects of 17alpha-ethinylestradiol, 17beta-estradiol and 4-nonylphenol on insulin-like growth-factor binding proteins (igfbps) in Atlantic salmon. Aquat Toxicol (2018) 203:28–39. doi: 10.1016/j.aquatox.2018.07.018
43. Geyer HJ, Rimkus GG, Scheunert I, Kaune A, Schramm KW, Kettrup A, et al. “Bioaccumulation and Occurrence of Endocrine-Disrupting Chemicals (EDCs), Persistent Organic Pollutants (POPs), and Other Organic Compounds in Fish and Other Organisms Including Humans,”. In: Beek B, Bohling S, editors. Bioaccumulation – New Aspects and Developments. Berlin: Springer (2000). p. 1–166. doi: 10.1007/10503050_1
44. Romero IC, Sutton T, Carr B, Quintana-Rizzo E, Ross SW, Hollander DJ, et al. Decadal Assessment of Polycyclic Aromatic Hydrocarbons in Mesopelagic Fishes from the Gulf of Mexico Reveals Exposure to Oil-Derived Sources. Environ Sci Technol (2018) 52(19):10985–96. doi: 10.1021/acs.est.8b02243
45. Nyholm JR, Norman A, Norrgren L, Haglund P, Andersson PL. Maternal transfer of brominated flame retardants in zebrafish (Danio rerio). Chemosphere (2008) 73(2):203–8. doi: 10.1016/j.chemosphere.2008.04.033
46. Freese M, Suhring R, Marohn L, Pohlmann JD, Wolschke H, Byer JD, et al. Maternal transfer of dioxin-like compounds in artificially matured European eels. Environ Pollut (2017) 227:348–56. doi: 10.1016/j.envpol.2017.04.096
47. Lubzens E, Bobe J, Young G, Sullivan CV. Maternal investment in fish oocytes and eggs: The molecular cargo and its contributions to fertility and early development. Aquaculture (2017) 472:107–43. doi: 10.1016/j.aquaculture.2016.10.029
48. Gray M, Niimi AJ, Metcalfe CD. Factors affecting the development of testis-ova in medaka, Oryzias latipes, exposed to octylphenol. Environ Toxicol Chem (1999) 18(8):1835–42. doi: 10.1002/etc.5620180834
49. Koger CS, Teh SJ, Hinton DE. Determining the sensitive developmental stages of intersex induction in medaka (Oryzias latipes) exposed to 17 beta-estradiol or testosterone. Mar Environ Res (2000) 50(1-5):201–6. doi: 10.1016/s0141-1136(00)00068-4
50. Kolpin DW, Furlong ET, Meyer MT, Thurman EM, Zaugg SD, Barber LB, et al. Pharmaceuticals, hormones, and other organic wastewater contaminants in U.S. streams, 1999-2000: a national reconnaissance. Environ Sci Technol (2002) 36(6):1202–11. doi: 10.1021/es011055j
51. Petrovic M, Sole M, Lopez de Alda MJ, Barcelo D. Endocrine disruptors in sewage treatment plants, receiving river waters, and sediments: integration of chemical analysis and biological effects on feral carp. Environ Toxicol Chem (2002) 21(10):2146–56. doi: 10.1002/etc.5620211018
52. Cailleaud K, Forget-Leray J, Souissi S, Lardy S, Augagneur S, Budzinski H. Seasonal variation of hydrophobic organic contaminant concentrations in the water-column of the Seine Estuary and their transfer to a planktonic species Eurytemora affinis (Calanoid, copepod). Part 2: Alkylphenol-polyethoxylates. Chemosphere (2007) 70(2):281–7. doi: 10.1016/j.chemosphere.2007.06.012
53. Peng X, Yu Y, Tang C, Tan J, Huang Q, Wang Z. Occurrence of steroid estrogens, endocrine-disrupting phenols, and acid pharmaceutical residues in urban riverine water of the Pearl River Delta, South China. Sci Total Environ (2008) 397(1-3):158–66. doi: 10.1016/j.scitotenv.2008.02.059
54. Brix R, Postigo C, Gonzalez S, Villagrasa M, Navarro A, Kuster M, et al. Analysis and occurrence of alkylphenolic compounds and estrogens in a European river basin and an evaluation of their importance as priority pollutants. Anal Bioanal Chem (2010) 396(3):1301–9. doi: 10.1007/s00216-009-3358-8
55. Aris AZ, Shamsuddin AS, Praveena SM. Occurrence of 17alpha-ethynylestradiol (EE2) in the environment and effect on exposed biota: a review. Environ Int (2014) 69:104–19. doi: 10.1016/j.envint.2014.04.011
56. Xu W, Yan W, Huang W, Miao L, Zhong L. Endocrine-disrupting chemicals in the Pearl River Delta and coastal environment: sources, transfer, and implications. Environ Geochem Health (2014) 36(6):1095–104. doi: 10.1007/s10653-014-9618-3
57. Cargouet M, Perdiz D, Mouatassim-Souali A, Tamisier-Karolak S, Levi Y. Assessment of river contamination by estrogenic compounds in Paris area (France). Sci Total Environ (2004) 324(1-3):55–66. doi: 10.1016/j.scitotenv.2003.10.035
58. Larsson DGJ, Adolfsson-Erici M, Parkkonen BJ, Pettersson M, Berg AH, Olsson PE, et al. Ethinyloestradiol — an undesired fish contraceptive? Aquat Toxicol (1999) 45:91–7. doi: 10.1016/S0166-445X(98)00112-X
59. Shved N, Berishvili G, Baroiller JF, Segner H, Reinecke M. Environmentally Relevant Concentrations of 17α-Ethinylestradiol (EE2) Interfere With the Growth Hormone (GH)/Insulin-Like Growth Factor (IGF)-I System in Developing Bony Fish. Toxicol Sci (2008) 106(1):93–102. doi: 10.1093/toxsci/kfn150
60. Schwindt AR, Winkelman DL, Keteles K, Murphy M, Vajda AM, Frid C. An environmental oestrogen disrupts fish population dynamics through direct and transgenerational effects on survival and fecundity. J Appl Ecol (2014) 51(3):582–91. doi: 10.1111/1365-2664.12237
61. Sun D, Chen Q, He N, Diao PP, Jia LX, Duan SS. Effect of environmentally-relevant concentrations of nonylphenol on sexual differentiation in zebrafish: a multi-generational study. Sci Rep (2017) 7(1):42907. doi: 10.1038/srep42907
62. Yin GG, Kookana RS, Ru YJ. Occurrence and fate of hormone steroids in the environment. Environ Int (2002) 28(6):545–51. doi: 10.1016/s0160-4120(02)00075-2
63. Zhang Z, Feng Y, Liu Y, Sun Q, Gao P, Ren N. Kinetic Degradation Model and Estrogenicity Changes of EE 2 (17α-Ethinylestradiol) in Aqueous Solution by UV and UV/H 2O 2 Technology. J Hazard Mater (2010) 181(1-3):1127–33. doi: 10.1016/j.jhazmat.2010.05.132
64. Servos MR, Maguire RJ, Bennie DT, Lee HB, Cureton PM, Davidson N, et al. An Ecological Risk Assessment of Nonylphenol and Its Ethoxylates in the Aquatic Environment. Hum Ecol Risk Assess (2003) 9(2):569–87. doi: 10.1080/713609923
65. Mao Z, Zheng XF, Zhang YQ, Tao XX, Li Y, Wang W. Occurrence and biodegradation of nonylphenol in the environment. Int J Mol Sci (2012) 13(1):491–505. doi: 10.3390/ijms13010491
66. Ishibashi H, Hirano M, Matsumura N, Watanabe N, Takao Y, Arizono K. Reproductive effects and bioconcentration of 4-nonylphenol in medaka fish (Oryzias latipes). Chemosphere (2006) 65(6):1019–26. doi: 10.1016/j.chemosphere.2006.03.034
67. Amaninejad P, Hosseinzadeh Sahafi H, Soltani M, Hosseini Shekarabi SP. Endocrine disrupting effects of 4-nonylphenol on plasma vitellogenin, reproductive system and histology in koi carp (Cyprinus carpio). Int Aquat Res (2018) 10(3):263–74. doi: 10.1007/s40071-018-0203-8
68. Chen WL, Gwo JC, Wang GS, Chen CY. Distribution of feminizing compounds in the aquatic environment and bioaccumulation in wild tilapia tissues. Environ Sci Pollut Res Int (2014) 21(19):11349–60. doi: 10.1007/s11356-014-3062-x
69. Cardinali M, Maradonna F, Olivotto I, Bortoluzzi G, Mosconi G, Polzonetti-Magni AM, et al. Temporary impairment of reproduction in freshwater teleost exposed to nonylphenol. Reprod Toxicol (2004) 18(4):597–604. doi: 10.1016/j.reprotox.2004.03.001
70. He ZL, Yang XE, Stoffella PJ. Trace elements in agroecosystems and impacts on the environment. J Trace Elem Med Biol (2005) 19(2-3):125–40. doi: 10.1016/j.jtemb.2005.02.010
71. Parizek J. The destructive effect of cadmium ion on testicular tissue and its prevention by zinc. J Endocrinol (1957) 15(1):56–63. doi: 10.1677/joe.0.0150056
72. Yamaguchi S, Miura C, Ito A, Agusa T, Iwata H, Tanabe S, et al. Effects of lead, molybdenum, rubidium, arsenic and organochlorines on spermatogenesis in fish: monitoring at Mekong Delta area and in vitro experiment. Aquat Toxicol (2007) 83(1):43–51. doi: 10.1016/j.aquatox.2007.03.010
73. Iavicoli I, Fontana L, Bergamaschi A. The effects of metals as endocrine disruptors. J Toxicol Environ Health B Crit Rev (2009) 12(3):206–23. doi: 10.1080/10937400902902062
74. Cullen WR, Reimer KJ. Arsenic speciation in the environment. Chem Rev (1989) 89:713–64. doi: 10.1021/cr00094a002
75. Alam MG, Allinson G, Stagnitti F, Tanaka A, Westbrooke M. Arsenic contamination in Bangladesh groundwater: a major environmental and social disaster. Int J Environ Health Res (2002) 12(3):235–53. doi: 10.1080/0960312021000000998
76. Kubota R, Kunito T, Tanabe S. Chemical speciation of arsenic in the livers of higher trophic marine animals. Mar Pollut Bull (2002) 45(1-12):218–23. doi: 10.1016/s0025-326x(02)00055-3
77. Mukherjee A, Sengupta MK, Hossain MA, Ahamed S, Das B, Nayak B, et al. Arsenic contamination in groundwater: a global perspective with emphasis on the Asian scenario. J Health Popul Nutr (2006) 24(2):142–63.
78. Kime D. Endocrine disruption in fish. Masachussettes: Kluwer Academic Publishers (1998). doi: 10.1007/978-1-4615-4943-7
79. Kaltreider RC, Davis AM, Lariviere JP, Hamilton JW. Arsenic alters the function of the glucocorticoid receptor as a transcription factor. Environ Health Perspect (2001) 109(3):245–51. doi: 10.1289/ehp.01109245
80. Bodwell JE, Kingsley LA, Hamilton JW. Arsenic at very low concentrations alters glucocorticoid receptor (GR)-mediated gene activation but not GR-mediated gene repression: complex dose-response effects are closely correlated with levels of activated GR and require a functional GR DNA binding domain. Chem Res Toxicol (2004) 17(8):1064–76. doi: 10.1021/tx0499113
81. Davey JC, Bodwell JE, Gosse JA, Hamilton JW. Arsenic as an endocrine disruptor: effects of arsenic on estrogen receptor-mediated gene expression in vivo and in cell culture. Toxicol Sci (2007) 98(1):75–86. doi: 10.1093/toxsci/kfm013
82. Centers for Disease Control and Prevention. Fourth Report on Human Exposure to Environmental Chemicals, Updated Tables Vol. Vol. 2. Atlanta, GA: U.S. Department of Health and Human Services, Centers for Disease Control and Prevention (2019).
83. Teuten EL, Saquing JM, Knappe DR, Barlaz MA, Jonsson S, Bjorn A, et al. Transport and release of chemicals from plastics to the environment and to wildlife. Philos Trans R Soc Lond B Biol Sci (2009) 364(1526):2027–45. doi: 10.1098/rstb.2008.0284
84. Olea N, Pulgar R, Pérez P, Olea-Serrano F, Rivas A, Novillo-Fertrell A, et al. Estrogenicity of resin-based composites and sealants used in dentistry. Environ Health Perspect (1996) 104:298–305. doi: 10.1289/ehp.96104298
85. Brotons JA, Olea-Serrano MF, Villalobos M, Pedraza V, Olea N. Xenoestrogens released from lacquer coatings in food cans. Environ Health Perspect (1995) 103:608–12. doi: 10.1289/ehp.95103608
86. Vandenberg LN, Hauser R, Marcus M, Olea N, Welshons WV. Human exposure to bisphenol A (BPA). Reprod Toxicol (2007) 24(2):139–77. doi: 10.1016/j.reprotox.2007.07.010
87. Fasano E, Bono-Blay F, Cirillo T, Montuori P, Lacorte S. Migration of phthalates, alkylphenols, bisphenol A and di(2-ethylhexyl)adipate from food packaging. Food Control (2012) 27(1):132–8. doi: 10.1016/j.foodcont.2012.03.005
88. Leitz J, Kuballa T, Rehm J, Lachenmeier DW. Chemical analysis and risk assessment of diethyl phthalate in alcoholic beverages with special regard to unrecorded alcohol. PloS One (2009) 4(12):e8127. doi: 10.1371/journal.pone.0008127
89. Carlstedt F, Jonsson BA, Bornehag CG. PVC flooring is related to human uptake of phthalates in infants. Indoor Air (2013) 23(1):32–9. doi: 10.1111/j.1600-0668.2012.00788.x
90. Sathyanarayana S, Karr CJ, Lozano P, Brown E, Calafat AM, Liu F, et al. Baby care products: possible sources of infant phthalate exposure. Pediatrics (2008) 121(2):e260–268. doi: 10.1542/peds.2006-3766
91. Hernandez-Diaz S, Mitchell AA, Kelley KE, Calafat AM, Hauser R. Medications as a potential source of exposure to phthalates in the U.S. population. Environ Health Perspect (2009) 117(2):185–9. doi: 10.1289/ehp.11766
92. Kelley KE, Hernandez-Diaz S, Chaplin EL, Hauser R, Mitchell AA. Identification of phthalates in medications and dietary supplement formulations in the United States and Canada. Environ Health Perspect (2012) 120(3):379–84. doi: 10.1289/ehp.1103998
93. Mathieu-Denoncourt J, Wallace SJ, de Solla SR, Langlois VS. Plasticizer endocrine disruption: Highlighting developmental and reproductive effects in mammals and non-mammalian aquatic species. Gen Comp Endocrinol (2015) 219:74–88. doi: 10.1016/j.ygcen.2014.11.003
94. Guzzetti E, Sureda A, Tejada S, Faggio C. Microplastic in marine organism: Environmental and toxicological effects. Environ Toxicol Pharmacol (2018) 64:164–71. doi: 10.1016/j.etap.2018.10.009
95. Thompson RC, Olsen Y, Mitchell RP, Davis A, Rowlan SJ, AW J, et al. Lost at sea: where is all the plastic? Science (2004) 304(5672):838. doi: 10.1126/science.1094559
96. Andrady AL. Microplastics in the marine environment. Mar Pollut Bull (2011) 62(8):1596–605. doi: 10.1016/j.marpolbul.2011.05.030
97. Cozar A, Echevarria F, Gonzalez-Gordillo JI, Irigoien X, Ubeda B, Hernandez-Leon S, et al. Plastic debris in the open ocean. Proc Natl Acad Sci USA (2014) 111(28):10239–44. doi: 10.1073/pnas.1314705111
98. Eriksen M, Lebreton LC, Carson HS, Thiel M, Moore CJ, Borerro JC, et al. Plastic Pollution in the World’s Oceans: More than 5 Trillion Plastic Pieces Weighing over 250,000 Tons Afloat at Sea. PloS One (2014) 9(12):e111913. doi: 10.1371/journal.pone.0111913
99. Zhao S, Zhu L, Wang T, Li D. Suspended microplastics in the surface water of the Yangtze Estuary System, China: first observations on occurrence, distribution. Mar Pollut Bull (2014) 86(1-2):562–8. doi: 10.1016/j.marpolbul.2014.06.032
100. Moore CJ, Moore SL, Leecaster MK, Weisberg SB. A comparison of plastic and plankton in the north Pacific central gyre. Mar Pollut Bull (2001) 42(12):1297–300. doi: 10.1016/s0025-326x(01)00114-x
101. Law KL, Moret-Ferguson S, Maximenko NA, Proskurowski G, Peacock EE, Hafner J, et al. Plastic accumulation in the North Atlantic subtropical gyre. Science (2010) 329(5996):1185–8. doi: 10.1126/science.1192321
102. Claessens M, De Meester S, Van Landuyt L, De Clerck K, Janssen CR. Occurrence and distribution of microplastics in marine sediments along the Belgian coast. Mar Pollut Bull (2011) 62(10):2199–204. doi: 10.1016/j.marpolbul.2011.06.030
103. Katsanevakis S. “Marine Debris, a Growing Problem: Sources, Distribution, Composition, and Impacts, “. In: Hofer TN, editor. Marine Pollution: New Research. New York: Nova Science Publishers (2008). p. 53–100.
104. Carlos de Sa L, Luis LG, Guilhermino L. Effects of microplastics on juveniles of the common goby (Pomatoschistus microps): confusion with prey, reduction of the predatory performance and efficiency, and possible influence of developmental conditions. Environ Pollut (2015) 196:359–62. doi: 10.1016/j.envpol.2014.10.026
105. Rummel CD, Loder MG, Fricke NF, Lang T, Griebeler EM, Janke M, et al. Plastic ingestion by pelagic and demersal fish from the North Sea and Baltic Sea. Mar Pollut Bull (2016) 102(1):134–41. doi: 10.1016/j.marpolbul.2015.11.043
106. Gove JM, Whitney JL, McManus MA, Lecky J, Carvalho FC, Lynch JM, et al. Prey-size plastics are invading larval fish nurseries. Proc Natl Acad Sci USA (2019) 116(48):24143–9. doi: 10.1073/pnas.1907496116
107. Shukla JP, Pandey K. Impaired ovarian functions in arsenic-treated freshwater fish, Colisa fasciatus (bl. and sch.). Toxicol Lett (1984) 20(1):1–3. doi: 10.1016/0378-4274(84)90175-9
108. Shukla JP, Pandey K. Impaired spermatogenesis in arsenic-treated freshwater fish, Colisa fasciatus (bl. and sch.). Toxicol Lett (1984) 21:191–5. doi: 10.1016/0378-4274(84)90205-4
109. Jobling S, Sumpter JP, Sheahan D, Osborne JA, Matthiessen P. nhibition of testicular growth in rainbow trout (Oncorhynchus mykiss) exposed to estrogenic alkylphenolic chemicals. Environ Toxicol Chem (1996) 15(2):194–202. doi: 10.1002/etc.5620150218
110. Madsen S, Mathiesen A, Korsgaard B. Effects of 17β-estradiol and 4-nonylphenol on smoltification and vitellogenesis in Atlantic salmon (Salmo salar). Fish Physiol Biochem (1997) 17:303–12. doi: 10.1023/A:1007754123787
111. Petit F, Le Goff P, Cravedi JP, Valotaire Y, Pakdel F. Two complementary bioassays for screening the estrogenic potency of xenobiotics: recombinant yeast for trout estrogen receptor and trout hepatocyte cultures. J Mol Endocrinol (1997) 19(3):321–35. doi: 10.1677/jme.0.0190321
112. Kwak HI, Bae MO, Lee MH, Lee YS, Lee BJ, Kang KS, et al. Effects of nonylphenol, bisphenol A, and their mixture on the viviparous swordtail fish (Xiphophorus helleri). Environ Toxicol Chem (2001) 20:787–95. doi: 10.1002/etc.5620200414
113. Sohoni P, Tyler CR, Hurd K, Caunter J, Hetheridge M, Williams T, et al. Reproductive effects of long-term exposure to Bisphenol A in the fathead minnow (Pimephales promelas). Environ Sci Technol (2001) 35(14):2917–25. doi: 10.1021/es000198n
114. Ackermann GE, Brombacher E, Fent K. Development of a fish reporter gene system for the assessment of estrogenic compounds and sewage treatment plant effluents. Environ Toxicol Chem (2002) 21(9):1864–75. doi: 10.1002/etc.5620210914
115. Ackermann GE, Schwaiger J, Negele RD, Fent K. Effects of long-term nonylphenol exposure on gonadal development and biomarkers of estrogenicity in juvenile rainbow trout Oncorhynchus mykiss. Aquat Toxicol (2002) 60(3-4):203–21. doi: 10.1016/s0166-445x(02)00003-6
116. Schwaiger J, Mallow U, Ferling H, Knoerr S, Braunbeck T, Kalbfus W, et al. How estrogenic is nonylphenol? A transgenerational study using rainbow trout (Oncorhynchus mykiss) as a test organism. Aquat Toxicol (2002) 59(3-4):177–89. doi: 10.1016/s0166-445x(01)00248-x
117. Kang IJ, Yokota H, Oshima Y, Tsuruda Y, Hano T, Maeda M, et al. Effects of 4-nonylphenol on reproduction of Japanese medaka, Oryzias latipes. Environ Toxicol Chem (2003) 22(10):2438–45. doi: 10.1897/02-225
118. Segner H, Navas JM, Schafers C, Wenzel A. Potencies of estrogenic compounds in in vitro screening assays and in life cycle tests with zebrafish in vivo. Ecotoxicol Environ Saf (2003) 54(3):315–22. doi: 10.1016/s0147-6513(02)00040-4
119. Arsenault JT, Fairchild WL, MacLatchy DL, Burridge L, Haya K, Brown SB. Effects of water-borne 4-nonylphenol and 17beta-estradiol exposures during parr-smolt transformation on growth and plasma IGF-I of Atlantic salmon (Salmo salar L.). Aquat Toxicol (2004) 66(3):255–65. doi: 10.1016/j.aquatox.2003.09.005
120. Rankouhi TR, Sanderson JT, van Holsteijn I, van Leeuwen C, Vethaak AD, van den Berg M. Effects of natural and synthetic estrogens and various environmental contaminants on vitellogenesis in fish primary hepatocytes: comparison of bream (Abramis brama) and carp (Cyprinus carpio). Toxicol Sci (2004) 81(1):90–102. doi: 10.1093/toxsci/kfh176
121. Tsai JW, Liao CM. Mode of Action and Growth Toxicity of Arsenic to Tilapia Oreochromis mossambicus Can Be Determined Bioenergetically. Arch Environ Contam Toxicol (2005) 50(1):144–52. doi: 10.1007/s00244-005-1054-z
122. Van den Belt K, Berckmans P, Vangenechten C, Verheyen R, Witters H. Comparative study on the in vitro/in vivo estrogenic potencies of 17beta-estradiol, estrone, 17alpha-ethynylestradiol and nonylphenol. Aquat Toxicol (2004) 66(2):183–95. doi: 10.1016/j.aquatox.2003.09.004
123. Miura C, Takahashi N, Michino F, Miura T. The effect of para-nonylphenol on Japanese eel (Anguilla japonica) spermatogenesis in vitro. Aquat Toxicol (2005) 71(2):133–41. doi: 10.1016/j.aquatox.2004.10.015
124. Hoffmann JL, Torontali SP, Thomason RG, Lee DM, Brill JL, Price BB, et al. Hepatic gene expression profiling using Genechips in zebrafish exposed to 17alpha-ethynylestradiol. Aquat Toxicol (2006) 79(3):233–46. doi: 10.1016/j.aquatox.2006.06.009
125. Vetillard A, Bailhache T. Effects of 4-n-nonylphenol and tamoxifen on salmon gonadotropin-releasing hormone, estrogen receptor, and vitellogenin gene expression in juvenile rainbow trout. Toxicol Sci (2006) 92(2):537–44. doi: 10.1093/toxsci/kfl015
126. Martyniuk CJ, Gerrie ER, Popesku JT, Ekker M, Trudeau VL. Microarray analysis in the zebrafish (Danio rerio) liver and telencephalon after exposure to low concentration of 17alpha-ethinylestradiol. Aquat Toxicol (2007) 84(1):38–49. doi: 10.1016/j.aquatox.2007.05.012
127. Shved N, Berishvili G, D’Cotta H, Baroiller JF, Segner H, Eppler E, et al. Ethinylestradiol differentially interferes with IGF-I in liver and extrahepatic sites during development of male and female bony fish. J Endocrinol (2007) 195(3):513–23. doi: 10.1677/JOE-07-0295
128. Boyle D, Brix KV, Amlund H, Lundebye AK, Hogstrand C, Bury NR. Natural arsenic contaminated diets perturb reproduction in fish. Environ Sci Technol (2008) 42(14):5354–60. doi: 10.1021/es800230w
129. Aluru N, Leatherland JF, Vijayan MM. Bisphenol A in oocytes leads to growth suppression and altered stress performance in juvenile rainbow trout. PloS One (2010) 5(5):e10741. doi: 10.1371/journal.pone.0010741
130. Carnevali O, Tosti L, Speciale C, Peng C, Zhu Y, Maradonna F. DEHP impairs zebrafish reproduction by affecting critical factors in oogenesis. PloS One (2010) 5(4):e10201. doi: 10.1371/journal.pone.0010201
131. Zanotelli VR, Neuhauss SC, Ehrengruber MU. Long-term exposure to bis(2-ethylhexyl)phthalate (DEHP) inhibits growth of guppy fish (Poecilia reticulata). J Appl Toxicol (2010) 30(1):29–33. doi: 10.1002/jat.1468
132. Garcia-Reyero N, Lavelle CM, Escalon BL, Martinovic D, Kroll KJ, Sorensen PW, et al. Behavioral and genomic impacts of a wastewater effluent on the fathead minnow. Aquat Toxicol (2011) 101(1):38–48. doi: 10.1016/j.aquatox.2010.08.014
133. Johns SM, Denslow ND, Kane MD, Watanabe KH, Orlando EF, Sepulveda MS. Effects of estrogens and antiestrogens on gene expression of fathead minnow (Pimephales promelas) early life stages. Environ Toxicol (2011) 26(2):195–206. doi: 10.1002/tox.20545
134. Hirakawa I, Miyagawa S, Katsu Y, Kagami Y, Tatarazako N, Kobayashi T, et al. Gene expression profiles in the testis associated with testis-ova in adult Japanese medaka (Oryzias latipes) exposed to 17alpha-ethinylestradiol. Chemosphere (2012) 87(7):668–74. doi: 10.1016/j.chemosphere.2011.12.047
135. Lerner DT, Sheridan MA, McCormick SD. Estrogenic compounds decrease growth hormone receptor abundance and alter osmoregulation in Atlantic salmon. Gen Comp Endocrinol (2012) 179(2):196–204. doi: 10.1016/j.ygcen.2012.08.001
136. Harding LB, Schultz IR, Goetz GW, Luckenbach JA, Young G, Goetz FW, et al. High-throughput sequencing and pathway analysis reveal alteration of the pituitary transcriptome by 17alpha-ethynylestradiol (EE2) in female coho salmon, Oncorhynchus kisutch. Aquat Toxicol (2013) 142-143:146–63. doi: 10.1016/j.aquatox.2013.07.020
137. Mankidy R, Wiseman S, Ma H, Giesy JP. Biological impact of phthalates. Toxicol Lett (2013) 217(1):50–8. doi: 10.1016/j.toxlet.2012.11.025
138. Colli-Dula RC, Martyniuk CJ, Kroll KJ, Prucha MS, Kozuch M, Barber DS, et al. Dietary exposure of 17-alpha ethinylestradiol modulates physiological endpoints and gene signaling pathways in female largemouth bass (Micropterus salmoides). Aquat Toxicol (2014) 156:148–60. doi: 10.1016/j.aquatox.2014.08.008
139. Duffy TA, Iwanowicz LR, McCormick SD. Comparative responses to endocrine disrupting compounds in early life stages of Atlantic salmon, Salmo salar. Aquat Toxicol (2014) 152:1–10. doi: 10.1016/j.aquatox.2014.03.015
140. Henneberg A, Bender K, Blaha L, Giebner S, Kuch B, Kohler HR, et al. Are in vitro methods for the detection of endocrine potentials in the aquatic environment predictive for in vivo effects? Outcomes of the Projects SchussenAktiv and SchussenAktivplus in the Lake Constance Area, Germany. PloS One (2014) 9(6):e98307. doi: 10.1371/journal.pone.0098307
141. Miyagawa S, Lange A, Hirakawa I, Tohyama S, Ogino Y, Mizutani T, et al. Differing species responsiveness of estrogenic contaminants in fish is conferred by the ligand binding domain of the estrogen receptor. Environ Sci Technol (2014) 48(9):5254–63. doi: 10.1021/es5002659
142. Rochman CM, Kurobe T, Flores I, Teh SJ. Early warning signs of endocrine disruption in adult fish from the ingestion of polyethylene with and without sorbed chemical pollutants from the marine environment. Sci Total Environ (2014) 493:656–61. doi: 10.1016/j.scitotenv.2014.06.051
143. Bhandari RK, vom Saal FS, Tillitt DE. Transgenerational effects from early developmental exposures to bisphenol A or 17alpha-ethinylestradiol in medaka, Oryzias latipes. Sci Rep (2015) 5:9303. doi: 10.1038/srep09303
144. Birceanu O, Servos MR, Vijayan MM. Bisphenol A accumulation in eggs disrupts the endocrine regulation of growth in rainbow trout larvae. Aquat Toxicol (2015) 161:51–60. doi: 10.1016/j.aquatox.2015.01.028
145. Hultman MT, Song Y, Tollefsen KE. 17alpha-Ethinylestradiol (EE2) effect on global gene expression in primary rainbow trout (Oncorhynchus mykiss) hepatocytes. Aquat Toxicol (2015) 169:90–104. doi: 10.1016/j.aquatox.2015.10.004
146. Golshan M, Hatef A, Socha M, Milla S, Butts IA, Carnevali O, et al. Di-(2-ethylhexyl)-phthalate disrupts pituitary and testicular hormonal functions to reduce sperm quality in mature goldfish. Aquat Toxicol (2015) 163:16–26. doi: 10.1016/j.aquatox.2015.03.017
147. Tohyama S, Miyagawa S, Lange A, Ogino Y, Mizutani T, Tatarazako N, et al. Understanding the molecular basis for differences in responses of fish estrogen receptor subtypes to environmental estrogens. Environ Sci Technol (2015) 49(12):7439–47. doi: 10.1021/acs.est.5b00704
148. Hallauer J, Geng X, Yang HC, Shen J, Tsai KJ, Liu Z. The Effect of Chronic Arsenic Exposure in Zebrafish. Zebrafish (2016) 13(5):405–12. doi: 10.1089/zeb.2016.1252
149. Ismail HT, Mahboub HH. Effect of acute exposure to nonylphenol on biochemical, hormonal, and hematological parameters and muscle tissues residues of Nile tilapia; Oreochromis niloticus. Vet World (2016) 9(6):616–25. doi: 10.14202/vetworld.2016.616-625
150. Laing LV, Viana J, Dempster EL, Trznadel M, Trunkfield LA, Uren Webster TM, et al. Bisphenol A causes reproductive toxicity, decreases dnmt1 transcription, and reduces global DNA methylation in breeding zebrafish (Danio rerio). Epigenetics (2016) 11(7):526–38. doi: 10.1080/15592294.2016.1182272
151. Nendza M, Wenzel A, Muller M, Lewin G, Simetska N, Stock F, et al. Screening for potential endocrine disruptors in fish: evidence from structural alerts and in vitro and in vivo toxicological assays. Environ Sci Eur (2016) 28(1):26. doi: 10.1186/s12302-016-0094-5
152. Qiu W, Zhao Y, Yang M, Farajzadeh M, Pan C, Wayne NL. Actions of Bisphenol A and Bisphenol S on the Reproductive Neuroendocrine System During Early Development in Zebrafish. Endocrinology (2016) 157(2):636–47. doi: 10.1210/en.2015-1785
153. Sadoul B, Birceanu O, Aluru N, Thomas JK, Vijayan MM. Bisphenol A in eggs causes development-specific liver molecular reprogramming in two generations of rainbow trout. Sci Rep (2017) 7(1):14131. doi: 10.1038/s41598-017-13301-7
154. Critchell K, Hoogenboom MO. Effects of microplastic exposure on the body condition and behaviour of planktivorous reef fish (Acanthochromis polyacanthus). PloS One (2018) 13(3):e0193308. doi: 10.1371/journal.pone.0193308
155. Basili D, Zhang JL, Herbert J, Kroll K, Denslow ND, Martyniuk CJ, et al. In Silico Computational Transcriptomics Reveals Novel Endocrine Disruptors in Largemouth Bass (Micropterus salmoides). Environ Sci Technol (2018) 52(13):7553–65. doi: 10.1021/acs.est.8b02805
156. Bohlen ML, Jeon HP, Kim YJ, Sung B. In Silico Modeling Method for Computational Aquatic Toxicology of Endocrine Disruptors: A Software-Based Approach Using QSAR Toolbox. J Vis Exp (2019) (150):e60054. doi: 10.3791/60054
157. Celino-Brady FT, Petro-Sakuma CK, Breves JP, Lerner DT, Seale AP. Early-life exposure to 17beta-estradiol and 4-nonylphenol impacts the growth hormone/insulin-like growth-factor system and estrogen receptors in Mozambique tilapia, Oreochromis mossambicus. Aquat Toxicol (2019) 217:105336. doi: 10.1016/j.aquatox.2019.105336
158. Chen H, Zhao L, Yu QJ. Determination and reduced life expectancy model and molecular docking analyses of estrogenic potentials of 17beta-estradiol, bisphenol A and nonylphenol on expression of vitellogenin gene (vtg1) in zebrafish. Chemosphere (2019) 221:727–34. doi: 10.1016/j.chemosphere.2019.01.093
159. Han JM, Park HJ, Kim JH, Jeong DS, Kang JC. Toxic effects of arsenic on growth, hematological parameters, and plasma components of starry flounder, Platichthys stellatus, at two water temperature conditions. Fish Aquat Sci (2019) 22(1):3. doi: 10.1186/s41240-019-0116-5
160. Wang J, Li Y, Lu L, Zheng M, Zhang X, Tian H, et al. Polystyrene microplastics cause tissue damages, sex-specific reproductive disruption and transgenerational effects in marine medaka (Oryzias melastigma). Environ Pollut (2019) 254(Pt B):113024. doi: 10.1016/j.envpol.2019.113024
161. Wang Q, Yang H, Yang M, Yu Y, Yan M, Zhou L, et al. Toxic effects of bisphenol A on goldfish gonad development and the possible pathway of BPA disturbance in female and male fish reproduction. Chemosphere (2019) 221:235–45. doi: 10.1016/j.chemosphere.2019.01.033
162. Cervantes-Camacho I, Guerrero-Estevez SM, Lopez MF, Alarcon-Hernandez E, Lopez-Lopez E. Effects of Bisphenol A on Foxl2 gene expression and DNA damage in adult viviparous fish Goodea atripinnis. J Toxicol Environ Health A (2020) 83(3):95–112. doi: 10.1080/15287394.2020.1730282
163. DeCourten BM, Forbes JP, Roark HK, Burns NP, Major KM, White JW, et al. Multigenerational and Transgenerational Effects of Environmentally Relevant Concentrations of Endocrine Disruptors in an Estuarine Fish Model. Environ Sci Technol (2020) 54(21):13849–60. doi: 10.1021/acs.est.0c02892
164. Major KM, DeCourten BM, Li J, Britton M, Settles ML, Mehinto AC, et al. Early Life Exposure to Environmentally Relevant Levels of Endocrine Disruptors Drive Multigenerational and Transgenerational Epigenetic Changes in a Fish Model. Front Mar Sci (2020) 7:471. doi: 10.3389/fmars.2020.00471
165. Shirdel I, Kalbassi MR, Esmaeilbeigi M, Tinoush B. Disruptive effects of nonylphenol on reproductive hormones, antioxidant enzymes, and histology of liver, kidney and gonads in Caspian trout smolts. Comp Biochem Physiol C Toxicol Pharmacol (2020) 232:108756. doi: 10.1016/j.cbpc.2020.108756
166. Wang L, Yan R, Yang Q, Li H, Zhang J, Shimoda Y, et al. Role of GH/IGF axis in arsenite-induced developmental toxicity in zebrafish embryos. Ecotoxicol Environ Saf (2020) 201:110820. doi: 10.1016/j.ecoenv.2020.110820
167. Crews D, Gore AC, Hsu TS, Dangleben NL, Spinetta M, Schallert T, et al. Transgenerational epigenetic imprints on mate preference. Proc Natl Acad Sci USA (2007) 104(14):5942–6. doi: 10.1073/pnas.0610410104
168. Rebuli ME, Patisaul HB. Assessment of sex specific endocrine disrupting effects in the prenatal and pre-pubertal rodent brain. J Steroid Biochem Mol Biol (2016) 160:148–59. doi: 10.1016/j.jsbmb.2015.08.021
169. Horan TS, Marre A, Hassold T, Lawson C, Hunt PA. Germline and reproductive tract effects intensify in male mice with successive generations of estrogenic exposure. PloS Genet (2017) 13(7):e1006885. doi: 10.1371/journal.pgen.1006885
170. Davis LK, Fox BK, Lim C, Hiramatsu N, Sullivan CV, Hirano T, et al. Induction of vitellogenin production in male tilapia (Oreochromis mossambicus) by commercial fish diets. Comp Biochem Physiol A Mol Integr Physiol (2009) 154(2):249–54. doi: 10.1016/j.cbpa.2009.06.009
171. Gross JM, Yee D. How does the estrogen receptor work? Breast Cancer Res (2002) 4(2):62–4. doi: 10.1186/bcr424
172. Park CB, Takemura A, Aluru N, Park YJ, Kim BH, Lee CH, et al. Tissue-specific suppression of estrogen, androgen and glucocorticoid receptor gene expression in feral vitellogenic male Mozambique tilapia. Chemosphere (2007) 69(1):32–40. doi: 10.1016/j.chemosphere.2007.04.072
173. Flouriot G, Pakdel F, Valotaire Y. Transcriptional and post-transcriptional regulation of rainbow trout estrogen receptor and vitellogenin gene expression. Mol Cell Endocrinol (1996) 124(1-2):173– 183. doi: 10.1016/s0303-7207(96)03960-3
174. Bowman CJ, Kroll KJ, Gross TG, Denslow ND. Estradiol-induced gene expression in largemouth bass (Micropterus salmoides). Mol Cell Endocrinol (2002) 196(1-2):67–77. doi: 10.1016/s0303-7207(02)00224-1
175. Jalabert B. Particularities of reproduction and oogenesis in teleost fish compared to mammals. Reprod Nutr Dev (2005) 45(3):261–79. doi: 10.1051/rnd:2005019
176. Nelson ER, Habibi HR. Functional significance of nuclear estrogen receptor subtypes in the liver of goldfish. Endocrinology (2010) 151(4):1668–76. doi: 10.1210/en.2009-1447
177. Jobling S, Sumpter JP. Detergent components in sewage effluent are weakly oestrogenic to fish: An in vitro study using rainbow trout (Oncorhynchus mykiss) hepatocytes. Aquat Toxicol (1993) 27(3-4):361–72. doi: 10.1016/0166-445x(93)90064-8
178. Sumpter JP Jobling S. Vitellogenesis as a Biomarker for Estrogenic Contamination of the Aquatic Environment. Environ Health Perspect (1995) 103(7):173–8. doi: 10.1289/ehp.95103s7173
179. Tyler CR, van der Eerden B, Jobling S, Panter G, Sumpter JP. Measurement of vitellogenin, a biomarker for exposure to oestrogenic chemicals, in a wide variety of cyprinid fish. J Comp Physiol B (1996) 166:418–26. doi: 10.1007/BF02337886
180. Panter GH, Thompson RS, Sumpter JP. Adverse reproductive effects in male fathead minnows (Pimephales promelas) exposed to environmentally relevant concentrations of the natural oestrogens, oestradiol and oestrone. Aquat Toxicol (1998) 42:243–53. doi: 10.1016/S0166-445X(98)00038-1
181. Tyler CR, Aerle RV, Hutchinson T, Maddix S, Trip H. An in vivo testing system for endocrine disruptors in fish early life stages using induction of vitellogenin. Environ Toxicol Chem (1999) 18:337–47. doi: 10.1002/etc.5620180234
182. Jones PD, De Coen WM, Tremblay L, Giesy JP. Vitellogenin as a biomarker for environmental estrogens. Water Sci Technol (2000) 42(7-8):1–14. doi: 10.2166/wst.2000.0546
183. Matozzo V, Gagne F, Marin MG, Ricciardi F, Blaise C. Vitellogenin as a biomarker of exposure to estrogenic compounds in aquatic invertebrates: a review. Environ Int (2008) 34(4):531–45. doi: 10.1016/j.envint.2007.09.008
184. Leet JK, Gall HE, Sepulveda MS. A review of studies on androgen and estrogen exposure in fish early life stages: effects on gene and hormonal control of sexual differentiation. J Appl Toxicol (2011) 31(5):379–98. doi: 10.1002/jat.1682
185. Duan C, Ren H, Gao S. Insulin-like growth factors (IGFs), IGF receptors, and IGF-binding proteins: roles in skeletal muscle growth and differentiation. Gen Comp Endocrinol (2010) 167(3):344–51. doi: 10.1016/j.ygcen.2010.04.009
186. Reindl KM, Sheridan MA. Peripheral regulation of the growth hormone-insulin-like growth factor system in fish and other vertebrates. Comp Biochem Physiol A Mol Integr Physiol (2012) 163(3-4):231–45. doi: 10.1016/j.cbpa.2012.08.003
187. Duan C. Nutritional and developmental regulation of insulin-like growth factors in fish. J Nutr (1998) 128(2 Suppl):306S–14S. doi: 10.1093/jn/128.2.306S
188. Butler AA, LeRoith D. Minireview: tissue-specific versus generalized gene targeting of the igf1 and igf1r genes and their roles in insulin-like growth factor physiology. Endocrinology (2001) 142(5):1685–8. doi: 10.1210/endo.142.5.8148
189. LeRoith D, Scavo L, Butler A. What is the role of circulating IGF-I? Trends Endocrinol Metab (2001) 12(2):48–52. doi: 10.1016/S1043-2760(00)00349-0
190. LeRoith D, Roberts CT Jr. The insulin-like growth factor system and cancer. Cancer Lett (2003) 195(2):127–37. doi: 10.1016/s0304-3835(03)00159-9
191. Fan Y, Menon RK, Cohen P, Hwang D, Clemens T, DiGirolamo DJ, et al. Liver-specific deletion of the growth hormone receptor reveals essential role of growth hormone signaling in hepatic lipid metabolism. J Biol Chem (2009) 284(30):19937–44. doi: 10.1074/jbc.M109.014308
192. Rajaram S, Baylink DJ, Mohan S. Insulin-like growth factor-binding proteins in serum and other biological fluids: regulation and functions. Endocr Rev (1997) 18(6):801–31. doi: 10.1210/edrv.18.6.0321
193. Duan C, Xu Q. Roles of insulin-like growth factor (IGF) binding proteins in regulating IGF actions. Gen Comp Endocrinol (2005) 142(1-2):44–52. doi: 10.1016/j.ygcen.2004.12.022
194. Overstreet RM. Aquatic pollution problems, Southeastern U.S. coasts: histopathological indicators. Aquat Toxicol (1988) 11(3-4):213–39. doi: 10.1016/0166-445x(88)90076-8
195. Ferreira M, Antunes P, Gil O, Vale C, Reis-Henriques MA. Organochlorine contaminants in flounder (Platichthys flesus) and mullet (Mugil cephalus) from Douro estuary, and their use as sentinel species for environmental monitoring. Aquat Toxicol (2004) 69(4):347–57. doi: 10.1016/j.aquatox.2004.06.005
196. Maes GE, Raeymaekers JA, Pampoulie C, Seynaeve A, Goemans G, Belpaire C, et al. The catadromous European eel Anguilla anguilla (L.) as a model for freshwater evolutionary ecotoxicology: relationship between heavy metal bioaccumulation, condition and genetic variability. Aquat Toxicol (2005) 73(1):99–114. doi: 10.1016/j.aquatox.2005.01.010
197. Palaniappan PL, Sabhanayakam S, Krishnakumar N, Vadivelu M. Morphological changes due to Lead exposure and the influence of DMSA on the gill tissues of the freshwater fish, Catla catla. Food Chem Toxicol (2008) 46(7):2440–4. doi: 10.1016/j.fct.2008.03.028
198. Sun PL, Hawkins WE, Overstreet RM, Brown-Peterson NJ. Morphological deformities as biomarkers in fish from contaminated rivers in Taiwan. Int J Environ Res Public Health (2009) 6(8):2307–31. doi: 10.3390/ijerph6082307
199. Streit B. Bioaccumulation of contaminants in fish. EXS (1998) 86:353–87. doi: 10.1007/978-3-0348-8853-0_12
200. Baron D, Batista F, Chaffaux S, Cocquet J, Cotinot C, Cribiu E, et al. Foxl2 gene and the development of the ovary: a story about goat, mouse, fish and woman. Reprod Nutr Dev (2005) 45:377–82. doi: 10.1051/rnd:2005028
201. Bertho S, Pasquier J, Pan Q, Le Trionnaire G, Bobe J, Postlethwait JH, et al. Foxl2 and its relatives are evolutionary conserved players in gonadal sex differentiation. Sex Dev (2016) 10:111–29. doi: 10.1159/000447611
202. Cocquet J, Pailhoux E, Jaubert F, Servel N, Xia X, Pannetier M, et al. Evolution and expression of FOXL2. J Med Genet (2002) 39(12):916–21. doi: 10.1136/jmg.39.12.916
203. Foley CJ, Feiner ZS, Malinich TD, Hook TO. A meta-analysis of the effects of exposure to microplastics on fish and aquatic invertebrates. Sci Total Environ (2018) 631-632:550–9. doi: 10.1016/j.scitotenv.2018.03.046
204. Sifa L, Mathias JA. The critical period of high mortality of larvae fish —A discussion based on current research. Chin J Oceanol Limnol (1987) 5:80–96. doi: 10.1007/BF02848526
205. Singh S, Li SS. Epigenetic effects of environmental chemicals bisphenol A and phthalates. Int J Mol Sci (2012) 13(8):10143–53. doi: 10.3390/ijms130810143
206. Miura T, Miura C. Japanese Eel: A Model for Analysis of Spermatogenesis. Zool Sci (2001) 18(8):1055–63. doi: 10.2108/zsj.18.1055
207. Scholz S, Renner P, Belanger SE, Busquet F, Davi R, Demeneix BA, et al. Alternatives to in vivo tests to detect endocrine disrupting chemicals (EDCs) in fish and amphibians–screening for estrogen, androgen and thyroid hormone disruption. Crit Rev Toxicol (2013) 43(1):45–72. doi: 10.3109/10408444.2012.737762
208. Klotz DM, Beckman BS, Hill SM, McLachlan JA, Walters MR, Arnold SF. Identification of environmental chemicals with estrogenic activity using a combination of in vitro assays. Environ Health Perspect (1996) 104(10):1084–9. doi: 10.1289/ehp.961041084
209. Routledge EJ, Sumpter JP. Estrogenic activity of surfactants and some of their degradation products assessed using a recombinant yeast screen. Environ Toxicol Chem (1996) 15(3):41–248. doi: 10.1002/etc.5620150303
210. Zacharewski T. In Vitro Bioassays for Assessing Estrogenic Substances. Environ Sci Technol (1997) 31(3):613–23. doi: 10.1021/es960530o
211. Andersen HR, Andersson AM, Arnold SF, Autrup H, Barfoed M, Beresford NA, et al. Comparison of short-term estrogenicity tests for identification of hormone-disrupting chemicals. Environ Health Perspect (1999) 107 Suppl 1:89–108. doi: 10.1289/ehp.99107s189
212. Balaguer P, Francois F, Comunale F, Fenet H, Boussioux AM, Pons M, et al. Reporter cell lines to study the estrogenic effects of xenoestrogens. Sci Total Environ (1999) 233(1-3):47–56. doi: 10.1016/s0048-9697(99)00178-3
213. Lascombe I, Beffa D, Ruegg U, Tarradellas J, Wahli W. Estrogenic activity assessment of environmental chemicals using in vitro assays: identification of two new estrogenic compounds. Environ Health Perspect (2000) 108(7):621–9. doi: 10.1289/ehp.00108621
214. Le Guevel R, Pakdel F. Assessment of oestrogenic potency of chemicals used as growth promoter by in-vitro methods. Hum Reprod (2001) 6(5):1030–6. doi: 10.1093/humrep/16.5.1030
215. Gutendorf B, Westendorf J. Comparison of an array of in vitro assays for the assessment of the estrogenic potential of natural and synthetic estrogens, phytoestrogens and xenoestrogens. Toxicology (2001) 166(1-2):79–89. doi: 10.1016/s0300-483x(01)00437-1
216. Murk AJ, Legler J, van Lipzig MM, Meerman JH, Belfroid AC, Spenkelink A, et al. Detection of estrogenic potency in wastewater and surface water with three in vitro bioassays. Environ Toxicol Chem (2002) 21(1):16–23. doi: 10.1002/etc.5620210103
217. Hutchinson TH, Brown R, Brugger KE, Campbell PM, Holt M, Lange R, et al. Ecological risk assessment of endocrine disruptors. Environ Health Perspect (2000) 108(11):1007–14. doi: 10.1289/ehp.001081007
218. Hutchinson TH, Ankley GT, Segner H, Tyler CR. Screening and testing for endocrine disruption in fish-biomarkers as “signposts,” not “traffic lights,” in risk assessment. Environ Health Perspect (2006) 114 Suppl 1:106–14. doi: 10.1289/ehp.8062
219. Rutishauser BV, Pesonen M, Escher BI, Ackermann GE, Aerni HR, Suter MJ, et al. Comparative analysis of estrogenic activity in sewage treatment plant effluents involving three in vitro assays and chemical analysis of steroids. Environ Toxicol Chem (2004) 23(4):857–64. doi: 10.1897/03-286
220. Schneider M, Pons JL, Labesse G, Bourguet W. In Silico Predictions of Endocrine Disruptors Properties. Endocrinology (2019) 160(11):2709–16. doi: 10.1210/en.2019-00382
221. Vuorinen A, Odermatt A, Schuster D. In silico methods in the discovery of endocrine disrupting chemicals. J Steroid Biochem Mol Biol (2013) 137:18–26. doi: 10.1016/j.jsbmb.2013.04.009
222. Coady KK, Biever RC, Denslow ND, Gross M, Guiney PD, Holbech H, et al. Current limitations and recommendations to improve testing for the environmental assessment of endocrine active substances. Integr Environ Assess Manag (2017) 13(2):302–16. doi: 10.1002/ieam.1862
223. Lyne PD. Structure-based virtual screening: an overview. Drug Discov Today (2002) 7(20):1047–55. doi: 10.1016/s1359-6446(02)02483-2
224. Cavasotto CN, Abagyan RA. Protein flexibility in ligand docking and virtual screening to protein kinases. J Mol Biol (2004) 337(1):209–25. doi: 10.1016/j.jmb.2004.01.003
225. Lewis SN, Garcia Z, Hontecillas R, Bassaganya-Riera J, Bevan DR. Pharmacophore modeling improves virtual screening for novel peroxisome proliferator-activated receptor-gamma ligands. J Comput Aided Mol Des (2015) 29(5):421–39. doi: 10.1007/s10822-015-9831-x
226. Stahura FL, Bajorath J. New methodologies for ligand-based virtual screening. Curr Pharm Des (2005) 11(9):1189–202. doi: 10.2174/1381612053507549
227. Meng XY, Zhang HX, Mezei M, Cui M. Molecular docking: a powerful approach for structure-based drug discovery. Curr Comput Aided Drug Des (2011) 7(2):146–57. doi: 10.2174/157340911795677602
228. McConkey BJ, Sobolev V Edelman M. The performance of current methods in ligand-protein docking. Curr Sci (2002) 83:845–55.
229. Sheikh IA, Tayubi IA, Ahmad E, Ganaie MA, Bajouh OS, AlBasri SF, et al. Computational insights into the molecular interactions of environmental xenoestrogens 4-tertoctylphenol, 4-nonylphenol, bisphenol A (BPA), and BPA metabolite, 4-methyl-2, 4-bis (4-hydroxyphenyl) pent-1-ene (MBP) with human sex hormone-binding globulin. Ecotoxicol Environ Saf (2017) 135:284–91. doi: 10.1016/j.ecoenv.2016.10.005
Keywords: arsenic, estrogenic endocrine-disrupting chemicals, growth, in silico, methods, microplastics, plasticizers, reproduction
Citation: Celino-Brady FT, Lerner DT and Seale AP (2021) Experimental Approaches for Characterizing the Endocrine-Disrupting Effects of Environmental Chemicals in Fish. Front. Endocrinol. 11:619361. doi: 10.3389/fendo.2020.619361
Received: 20 October 2020; Accepted: 30 December 2020;
Published: 25 February 2021.
Edited by:
Renata Guimarães Moreira, University of São Paulo, BrazilReviewed by:
Filipe Castro, University of Porto, PortugalCopyright © 2021 Celino-Brady, Lerner and Seale. This is an open-access article distributed under the terms of the Creative Commons Attribution License (CC BY). The use, distribution or reproduction in other forums is permitted, provided the original author(s) and the copyright owner(s) are credited and that the original publication in this journal is cited, in accordance with accepted academic practice. No use, distribution or reproduction is permitted which does not comply with these terms.
*Correspondence: Andre P. Seale, c2VhbGVAaGF3YWlpLmVkdQ==
Disclaimer: All claims expressed in this article are solely those of the authors and do not necessarily represent those of their affiliated organizations, or those of the publisher, the editors and the reviewers. Any product that may be evaluated in this article or claim that may be made by its manufacturer is not guaranteed or endorsed by the publisher.
Research integrity at Frontiers
Learn more about the work of our research integrity team to safeguard the quality of each article we publish.