- 1Molecular Physiology of Behavior Laboratory, Department of Molecular, Cellular and Developmental Neurobiology, Cajal Institute, Spanish National Research Council (CSIC), Madrid, Spain
- 2Sartorius Netherlands BV, Amersfoor, Netherlands
- 3Developmental Timing, Environment and Behaviors Laboratory, Institut Sophia Agrobiotech, Université Côte d’Azur-INRAE-CNRS-INSERM, Sophia Antipolis, France
Puberty and metamorphosis are two major developmental transitions linked to the reproductive maturation. In mammals and vertebrates, the central brain acts as a gatekeeper, timing the developmental transition through the activation of a neuroendocrine circuitry. In addition to reproduction, these neuroendocrine axes and the sustaining genetic network play additional roles in metabolism, sleep and behavior. Although neurohormonal axes regulating juvenile-adult transition have been classically considered the result of convergent evolution (i.e., analogous) between mammals and insects, recent findings challenge this idea, suggesting that at least some neuroendocrine circuits might be present in the common bilaterian ancestor Urbilateria. The initial signaling pathways that trigger the transition in different species appear to be of a single evolutionary origin and, consequently, many of the resulting functions are conserved with a few other molecular players being co-opted during evolution.
Introduction
Timing Metamorphosis as Compared to Puberty
Life is based on the transmission of genetic material across generations. In multicellular animals, the most frequent (and consequently the most successful) strategy is through sexual reproduction. It usually requires a juvenile-adult transition in order to reach sexual maturation. Both mammals and insects trigger this process through a complex, multi-step neurohormonal signaling that may show certain structural parallelisms (Figure 1) (1, 2). Indeed, the neuroendocrine axis is activated at the pre-pubertal and pre-metamorphic stages by the activity of a group of neurons, which transmitted the initial stimulus to the mammalian pituitary gland or the Drosophila PG (prothoracic gland). These neurons, named GnRHn (Gonadotropin Release Hormone-expressing neurons) in mammals and PTTHn (Prothoracicotropic Hormone-expressing neurons) in insects, project their axons out of the brain barrier towards the ME (median eminence) or the PG to secrete the GnRH and PTTH neuropeptides, respectively. GnRH activates its GPCR (G-protein-coupled receptor) named GnRHR in the anterior pituitary cells inducing the circulating secretion of FSH (Follicle-stimulating Hormone) and LH (Luteinizing Hormone), which stimulates the production of steroid hormones (estrogens) in the gonads. Instead, PTTH activates its RTK (receptor tyrosine kinase) TORSO in the PG, arousing the MAPK/ERK (mitogen-activated protein kinases/extracellular signal-regulated kinases) signaling pathway, which leads to the direct stimulation of a steroid hormone (ecdysone) (3, 4). Moreover, the awakening of PTTHn and GnRHn activity at pre-metamorphic or pre-pubertal timing depends on the insect AstA/AstAR1 (AllatostatinA) and the mammalian Kiss1/Kiss1R (kisspeptin1) GPCR-dependent systems (Figure 1). The general scheme is very similar in terms of the design of the molecular players but not in the evolutive history, with the exception of AstA/kiss1 (see below). In addition to the hierarchical regulation of both axes, the different clusters of neurohormone-expressing cells also act as regulatory hubs (1, 2). For instance, GnRHn activity is controlled not only by kiss1 but also by other neuropeptides like neurokinin-B, dynorphin, NPY and the neurotransmitter GABA, among others (1).
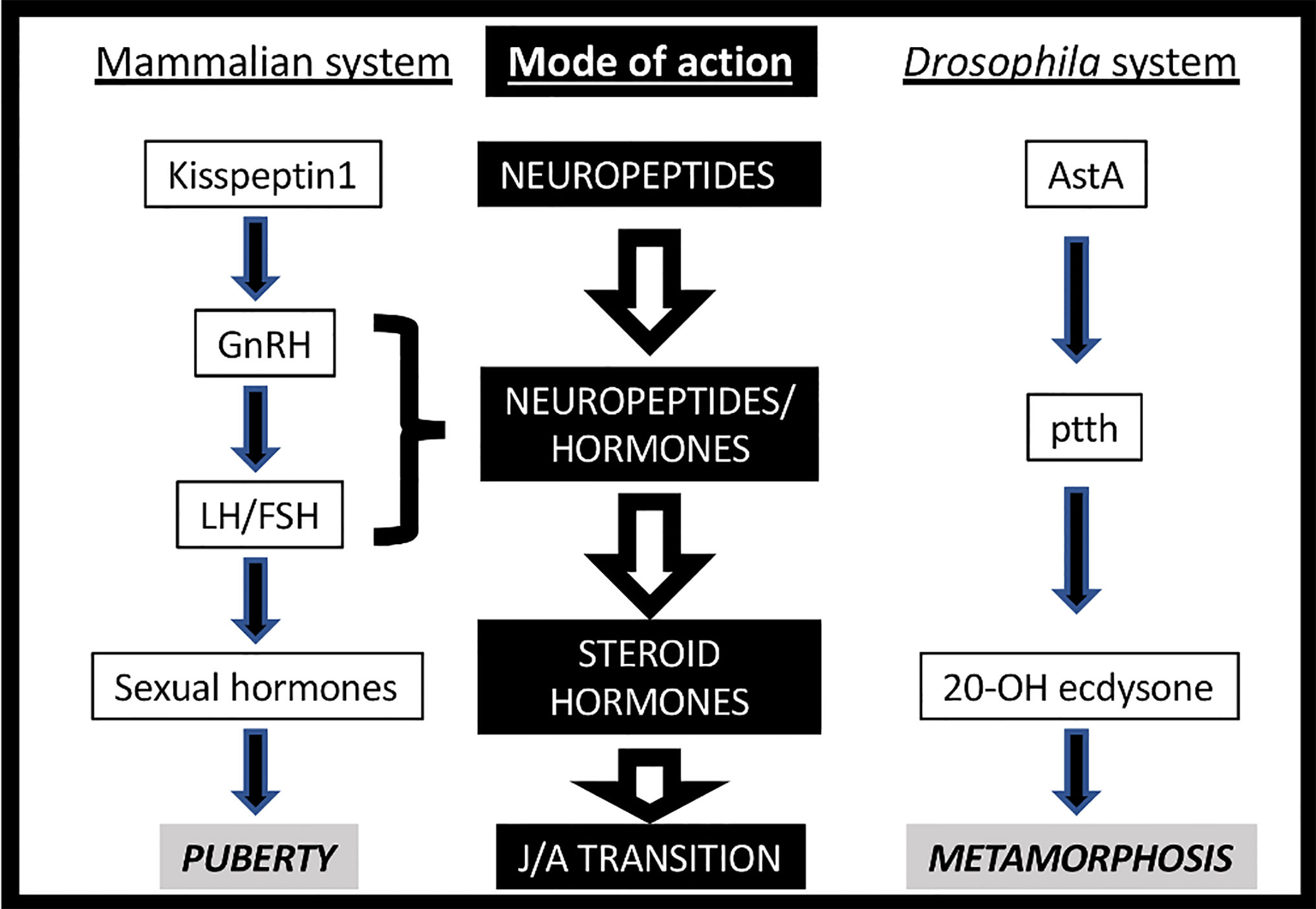
Figure 1 Neurohormonal axes triggering Juvenile-Adult (J/A) transition in mammals (left) and insects (right).
The complexity of the mammalian neurohormonal axis (also known as hypothalamic–pituitary–gonadal -HPG- or reproductive axis) is reflected in the imbricated architecture of the cellular circuitry in contrast with the apparent simple beauty of insect one, with fewer cells and genes. Nevertheless, new players have been found recently that added complexity and solved an apparent paradox: whereas the PG down-regulation of ecdysteroidogenic enzymes arrests the developmental transition (as shown by the absence of pupariation), removing or silencing ptth or torso only creates a delay in pupariation onset. In the last decade, several studies have pointed out the PG as another node (in addition to the brain), which also integrates signals to regulates ecdysteroidogenesis. Indeed, Cruz and colleagues recently described that prothoracic EGFR (Epidermal Growth Factor Receptor) stimulated by VEIN and SPITZ ligands activate MAPK/ERK pathway in the PG, as PTTH/TORSO signaling also does (5, 6). EGF signaling might compensate for ptth loss of function (LOF). EGFR LOF causes developmental arrest, resembling the lack of ecdysone (5). The autonomous PG expression of split and vein ligand genes depends on ecdysone signaling itself, suggesting that the EGFR pathway acts as an amplifying signal of the ecdysone production in the PG, a role previously described for ecdysone/EcR signaling (7, 8). Conversely, PTTHn are controlled by developmental and environmental inputs like growing tissue damages, photoperiod, over-crowding conditions, and ecdysone itself (2, 8). PTTHn also receive inputs from other neuronal circuits like the AstAn (9) and the corazonin (crz) neurons (10). Therefore, it is suggested that PTTH might be the link between the endocrine system and the internal/external milieu and other signals like EGF and ecdysone itself are required autonomously in the PG to ensure enough ecdysone surge to provoke metamorphosis (2).
Another enigma in the insect model comes from the difference in the observed developmental delay between the ptth null mutant (one day) and the PTTHn ablation (5 days). This suggests that PTTHn may cause electrical stimulation of the prothoracic gland to promote the release of ecdysone (11), or they may secrete another signal(s) that regulates ecdysone synthesis (12). Future studies are necessary to disclose the full PTTH neuronal function in timing the juvenile to adult transition.
The Neurohormonal Axis During Juvenile to Adult Transition and Fertility
Previous to adolescence and near to birth, the mammalian neurohormonal axis is active for a relatively short post-natal period known as mini-puberty (13, 14). This initial increase in the levels of sexual steroids is important for the correct development of gonads. Whether something similar may also happen in insects involving early pulses of ecdysone needs further investigation, although in larval stages three small ecdysone peaks of unknown function and non-related to ecdysis are described (15) After triggering puberty, the mammalian hypophysiotropic axis controls the overall pubertal process (somatic and sexual maturation) through steroid hormones (Figure 2). In Drosophila, the transition is commanded by ecdysone through the metamorphosis process. However, the role of PTTH in stimulating ecdysteroidogenesis during metamorphosis is not clear. ptth null mutant animals do not show any defect on the metamorphic process (12) although ptth transcription is highest at this stage (20 times higher than at larval or adult stages) (16). This result implies either that metamorphic PTTH does not stimulate ecdysteroidogenesis or, alternatively, that the role of PTTH in stimulating ecdysone synthesis during metamorphosis is compensated when absent (Figure 2). Moreover, crz or AstA silencing does not disturb metamorphic process itself. Therefore, whereas GnRH is essential for the pubertal process since its deficiency resulted in infertility and improper development of gonads, lack of AstA, crz, and ptth does not disrupt metamorphosis (like ecdysone absence does). This suggests the existence of another intrinsic/regulatory mechanism of ecdysone production in insects. Indeed, some hemimetabolous and ametabolous insects lack ptth, and the involvement of CRZ in their metamorphic process has not been described (17–19). Further studies should better clarify the existence of other ecdysone regulatory/intrinsic mechanism that might question the relevance of AstA, crz, and ptth signaling in timing Juvenile-to adult transition. Hence, the role of the PTTH-PG axis in the metamorphosis process itself remains controversial. Nevertheless, there are pieces of evidence indicating that the PTTH-PG axis is present during metamorphosis and regulates the rhythmicity of eclosion (i.e., pupal emergence) (20). PTTH acts as an intermediate player between the central clock, PDF-positive neurons, and the PG to produce ecdysone in a coordinated and timely manner to induce such rhythmic eclosion (20).
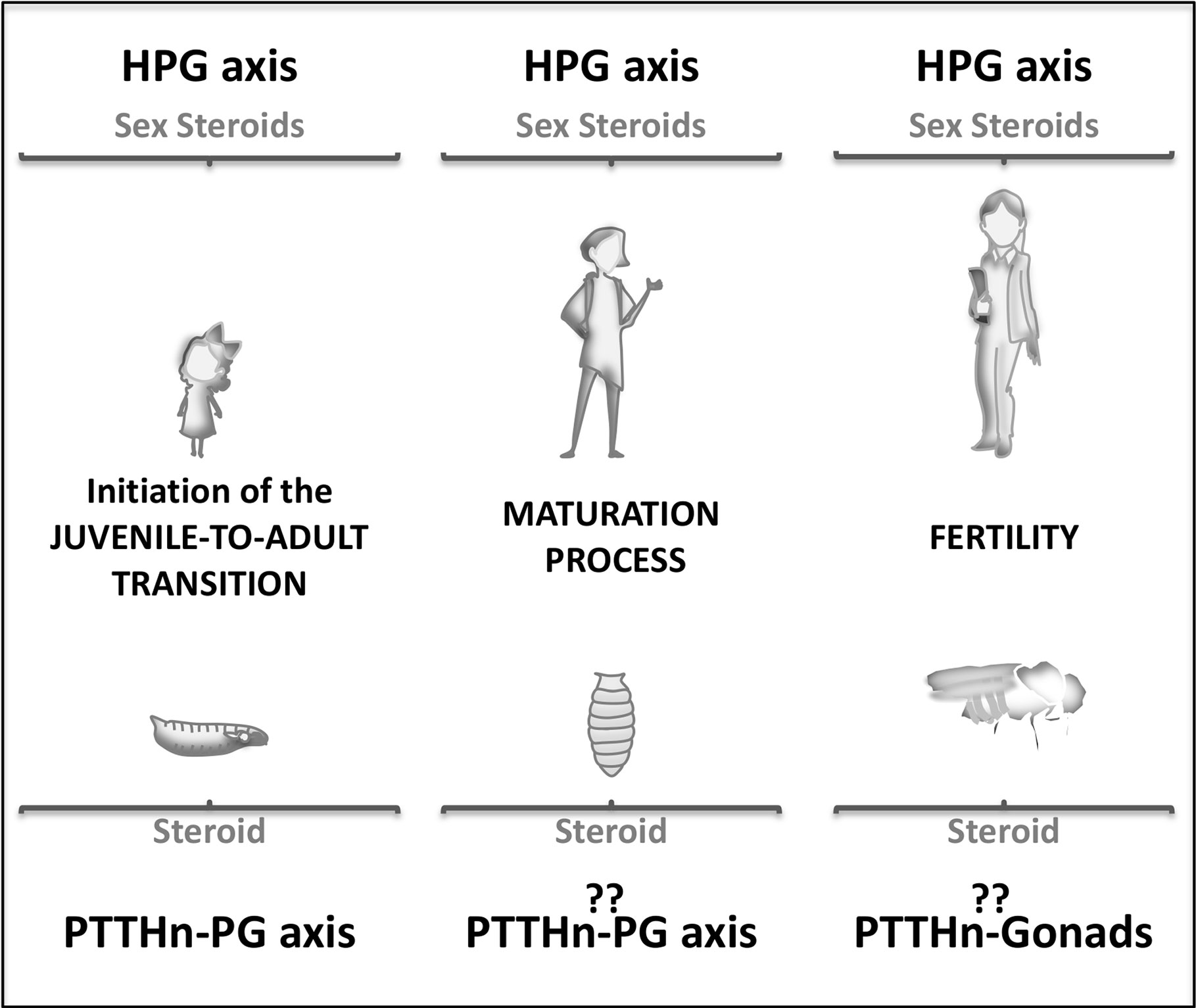
Figure 2 Schematic representation of the J/A neuroendocrine axis involvement during human (up) and Drosophila (down) lifespan. Juvenile stages (left), pubertal or metamorphic stage (center), and adult stage (right).
What is the role of these neurohormonal axes and their neuropeptide players in the adult stage? Are they still expressed and functional? In mammals, the pulsatile pre-pubertal secretion of GnRH enables the final maturation of a GnRH surge, which triggers the first ovulation (Figure 2) (21). Subsequently, the HPG axis regulates the ovulatory cycle in the female adult. In Drosophila, the fertility of adult females is also controlled by the ecdysone produced at the ovaries since the prothoracic gland degenerates by the end of metamorphosis (22, 23). In the adult brain, PTTH expresses in a group of neurons, but during metamorphosis, the larval brain undergoes extensive remodeling, and many new neurons are added. In this rewiring scenario, no precise analyses have been done to determine whether or not PTTH adult neurons are conserved from the juvenile larval stage. Moreover, adult PTTHn does not show projections towards the ovaries to directly control ovary ecdysteroidogenesis and reproduction (3). These observations may imply that the adult neuroendocrine axis is not identical to the larval one. Future research is needed to determine whether PTTH control adult ecdysteroidogenesis and reproduction as the mammalian neuroendocrine axis does.
Transitional Neurohormones in the Adult
In contrast with the reduced number of larval AstA-expressing neurons, adult AstA expresses in several neurons and endocrine cells (23). The existence of several AstA-producing cell populations suggests distinct AstA roles in adult physiology, including behavior. The most conserved and studied function of AstA/AstAR signaling during adulthood refers to feeding and hunger regulation (23, 24). The activity of AstA-expressing neurons provides a satiety signal and regulates systemic Insulin signaling. Lowering AstA levels in enteroendocrine secretory cells (EESs) from the gut induces early midgut senescence and shortens lifespan (24). In part, AstA mediates sugar-mediated satiety through the mushroom body, inhibiting dopaminergic input neurons that results in suppressed food search (25). This suppression of neuronal dopamine activity is essential to form long-term appetitive memory after sugar ingestion (26).
Sleep is also affected by AstA signaling in the Posterior Lateral Protocerebrum (PLP) cell cluster. These neurons are targets of the circadian PDF-expressing neurons, affecting sleep but not rhythmicity (23). AstA functionally links PLPs with an important sleep center such as the central complex through AstAR1, although the physical connection is still missing (27). Interestingly, input dopaminergic neurons of the mushroom body also play a role in sleep regulation, but a possible role of AstAn has not been explored yet (28). It is tempting to speculate that AstA acts as a single key molecule that can coordinate an energy-saving activity like a sleep state with general metabolic homeostasis regulation at several levels.
As discussed above, the insect AstA system is evolutionary related to the mammalian GAL and KISS1 system. The small neuropeptide GAL and its three described receptors are widely distributed in several neuronal populations of the central and peripheral nervous systems and in other tissues such as gastrointestinal tract (29). In contrast, Kiss1 expression is more restricted to particular neuronal populations of the hypothalamus (rostral periventricular area -R3PV- and arcuate nucleus -ARH-) and the amygdala (1). KISS1n are classically related to reproduction; however, their axons project to many other targets than solely GnRHn. Concerning feeding and metabolism, controversial data propose that the activation of GAL signaling has a transiently (up to 24 h) positive effect on sugar feeding ameliorating insulin resistance (29). A recent work implies the lateral hypothalamic subset of GALn in regulation of food reward, however the implication of GAL signaling is not studied (30). KISS1n themselves control feeding behavior, albeit independently from KISS1 signaling (31). A KISS1-related effect on metabolism has been reported in Kiss1R-deficient aged (but not young) mice, which are obese without increased feeding (32–34). Therefore, GAL and KISS1 are somehow connected to feeding and metabolism regulation, but they do not play the essential role that the AstA system does.
By comparison, the link between GAL signaling and sleep is well established. Preoptic GAL neuronal activity and expression are essential for sleep rebound after sleep deprivation (35). These results indicate that GALn, by means of GAL, is the output of the sleep homeostat in vertebrates. KISS1 function itself is not related to sleep regulation, although Kiss1n from the arcuate nucleus do play a key role in the control of circadian rhythms such as sleep, temperature, locomotor activity, and food consumption, probably downstream of the central clock (36). The most extensively established functions of GAL signaling are anxiety/depression and addiction, which are often co-morbid with addictive behaviors (37). Increased GAL signaling is associated with depression-like states. GALR 1 and 3 mediate this effect by interacting with the Serotonin receptor whereas GALR2 plays an opposite antidepressant and anxiolytic role (38). This differential effect may explain why increased GAL activity induces alcohol consumption, but it protects against other drugs of abuse (such as nicotine and opiates) (38). KISS1 signaling also has an impact on anxiety-like behaviors, which may be GnRH/estrogen-mediated or exclusively Kiss1R-mediated in the hippocampus (39). KISS1 regulates reproduction-related behaviors such as male sexual preference mediated by GnRH neurons, although a copulatory behavior like lordosis (the arching of female back in response to male copulation) is controlled by Kiss1n independently of KISS1 (40). A possible relation of AstA pathway with anxiety-like or addiction behaviors remains to be studied in Drosophila models of such behaviors. The only suggestive data is that high levels of AstA induce aggressiveness in Africanized honeybees (41).
In the adult brain, ecdysone regulates circadian rhythmicity through Let7 (although it is not clear where ecdysone is produced) (42). ptth mRNA is also detected in adult heads, with levels that change following a 24-h cycle (42). It has been proposed that PTTH controls adult ecdysone production participating in a regulatory feedback loop of the circadian clock although down-regulating ptth receptor torso does not produce any rhythmic alterations in the locomotion activity (20, 42), a classical readout of circadian rhythm in some insect and mammalian models. According to a ptth reporter that reflects faithfully larval and pupal expression, ptth expression in the adult is restricted to the Ellipsoid Body, a part of the Central Complex associated with sleep regulation (3, 27). This expression pattern is compatible with a role for ptth in sleep, although this possibility remains unexplored until now. In adult stages, PTTH behavioral function requires further studies, as well as its role in biological rhythms and steroid production.
Conversely, the adult role of CRZ has been studied in much more detail in regards to stress signaling metabolism and reproduction-related behaviors (43). crz is expressed in the somata of neurosecretory cells, among others. Down-regulation of crz in these cells or crzR in the IPCs (Insulin Producing Cells) prolongs survival in flies exposed to starving conditions, and also affect lipid and glucose metabolism (44). The knockdown of crzR in salivary glands and fat body (functionally equivalent to mammalian fat cells and liver) causes similar metabolic phenotypes, including increased triacylglyceride levels and feeding, after exposure to different stress conditions (45). Furthermore, in the marine bristle worm Platynereis dumerilii CRZ signaling coordinates metabolic state with the lunar phase in order to achieve the final size and induce sexual maturation (46). Intriguingly, crz- and crzR- expressing neurons in the abdominal ganglia regulate sperm transfer and copulation duration in males (47). The activation of CRZ signaling is sufficient to mimic ejaculation-caused reward, driving appetitive memories and reducing ethanol consumption (48). It was previously shown that CRZ regulates ethanol sensitivity and detoxification (43).
The presence of many GnRH neurons outside the hypothalamus in transparentized human fetuses, and the expression of GnRHR in several adult brain structures (which include the cortex, spinal cord, cerebellum, and hippocampus) suggest that GnRH signaling plays additional roles even after adolescence (49, 50). Most of them depend on its estrogenic activity, like fertility. Low GnRH levels and high GnIH (Gonadotropin Inhibitory Hormone, the main GnRH antagonist), correlate with the severity of human insomnia, probably through circadian estrogens regulation (51, 52). The role of estrogens in sleep and metabolism has been extensively studied and is described elsewhere (53). In addition, given the increasing evidence that GnRH signaling has neurotrophic, neuroprotective, and regenerative functions, it has been proposed that combined therapy of GnRH with Growth Hormone may be beneficial following neural damage (50). For instance, GnRH signaling in the female hippocampus regulates the synaptic plasticity through an estrogen-mediated mechanism (54).
Comparison of Neurohormonal Axes and Their Evolutionary Origin
One controversial issue is how similar the overall design of GnRHn and PTTHn neuroendocrine hubs is since both trigger very similar processes, puberty and metamorphosis, respectively. In humans, GnRHn unmyelinated projections have unipolar or bipolar morphology that functions as a dendrite and axon simultaneously, a structure named dendron (55). These projections travel to the ME of the pituitary gland covering distances over 1–3 mm (56). Once in the ME, GnRHn projections branch into multiple short axons with “specialized” neuroterminals boutons that target the blood vessels from the closed portal vasculature. There, GnRH is released to act on the anterior pituitary gland (57). In Drosophila, PTTHn projections also have a very simple unipolar morphology that functions simultaneously as a dendrite and axon and travels a long distance of up to 500 µm to reach the PG (3, 58). PTTHn projections branch into multiple short axons with “specialized” terminal boutons that target individual PG cells (12). GnRHn exhibit pulse and surge modes of activity to control fertility. Whereas the LH surge requires functional soma-proximal dendrites and distal dendron, the distal dendron integrates synaptic information to drive pulsatile GnRH secretion (59). Recent studies indicate the presence of regulatory mechanisms controlling GnRHn and PTTHn projections into the ME/PG, respectively (9, 60, 61). Actually, various genes involved in the Semaphorin signaling, like semaphorin-3A (Sema3A), neuropilin (Nrp1 or Nrp2) coreceptors, and its receptor plexin-A1 (PlxnA1), have been implicated in the guidance of GnRH neuronal migration, its survival and adult GnRHn plasticity affecting fertility (60, 62–66). Whereas PTTH neuronal development and migration towards the PG needs to be elucidated, a recent study suggests that PTTH neurons are also suffering from axon remodeling during the juvenile period (9). Drosophila Semaphorin signaling is well conserved, and it has also been implicated in axon guidance and synaptic plasticity (67). Interestingly, silencing the homologous of PlxnA1 (pLexB receptor) in the PG caused a delayed onset of metamorphosis, suggesting that a similar mechanism regulates PTTH neuronal growth (68). Further studies should investigate the involvement of Drosophila Semaphorin signaling pathway in PTTH neuroterminal remodeling, migration towards the PG or survival, expanding or not the similarities between PTTH and GnRH neuronal development and physiology.
Unlike Drosophila PTTHn, which directly innervate the prothoracic gland itself, Lepidoptera PTTHn release PTTH into the hemolymph from their specialized nerve endings in the corpus allatum (CA) (69). The CA is the endocrine organ that produces Juvenile hormone (JH), the primary negative regulator of PTTH in the Lepidoptera Manduca sexta. JH drops upon the organism attainment of a critical weight allowing PTTH titters to rise and induce metamorphosis (70). In Drosophila, the function of JH on ecdysone synthesis has been the center of controversies. Indeed, removal of total JH by CA ablation or the use of different null mutants does not advance metamorphosis as expected by releasing the potential JH inhibitory effect on ecdysteroidogenesis (71). However, the silencing of the JH receptor exclusively in the PG induces ecdysone biosynthesis and triggers the precocious initiation of pupariation (72, 73). It has been proposed that this difference reflects a secondary effect of JH in the Insulin-mediated growth pathway, which antagonizes the direct timing effect on the PG. Further studies are needed to better clarify this point. Differences in the neuroendocrine connectivity between Drosophila and Manduca might reflect species-specific variances in ecdysone regulation. Indeed, in other insects like Bombyx mori, PTTHn also innervates the CA, raising the possibility that PTTHn projections towards the CA is a general trait in insects (74). Furthermore, Drosophila PTTH functions as a neuropeptide on the PG, whereas Lepidoptera PTTH acts as a circulating hormone (3, 12, 69). Indeed, PTTH in Manduca sexta and Bombyx mori shows more similarities with the mammalian GnRH, which does not directly reach the hypophysis and acts as a secreted hormone into the circulating portal vessels.
Another pending question in the field is whether or not genes involved in the mammalian and insect neuroendocrine circuitry are evolutionary related, given the evolutionary distance (Figure 3A). Several phylogeny studies propose that the insect AstA system is evolutionary related to the mammalian GAL and KISS1, the mammalian gatekeeper of puberty (75–79). Indeed, gal and kiss emerged from the same ancestral gene precursor, and most likely this divergence occurs before protostome and deuterostome separation since kissR sequences have been found in protostomes such as annelids and mollusks (Figure 3A) (78–80). Based on neuropeptide receptor analysis, the AstA system was initially evolutionarily associated with GAL (Figure 3B) (75, 76). Functional studies in the Drosophila AstA system support this evolutionary relation since AstA participates in similar biological processes as GAL (sleep, food intake, metabolism, gut physiology, and others; see above). Nevertheless, more recent phylogeny and gene synteny studies on the receptors and mature peptides might indicate that AstAR and KISS1R emerged after GALR gene divergence from the common ancestral gene (Figure 3C) (77, 78). This model is also reinforced by our finding that AstA regulates the juvenile-to-adult transition, as Kiss1 does in mammals (9). Moreover, KISS signaling in the non-chordate deuterostome sea cucumber Apostichopus japonicus regulates both reproductive and non-reproductive (glucose metabolism) functions as the AstA system does in Drosophila, hinting to a possible dual ancestral role for KISS/AstA signaling (81). These data favor the hypothesis that AstA may be the true kiss1 orthologue while gal is a kiss1 paralog gene. Further studies are required to determine the precise mammalian AstA orthologous and paralogous conclusively.
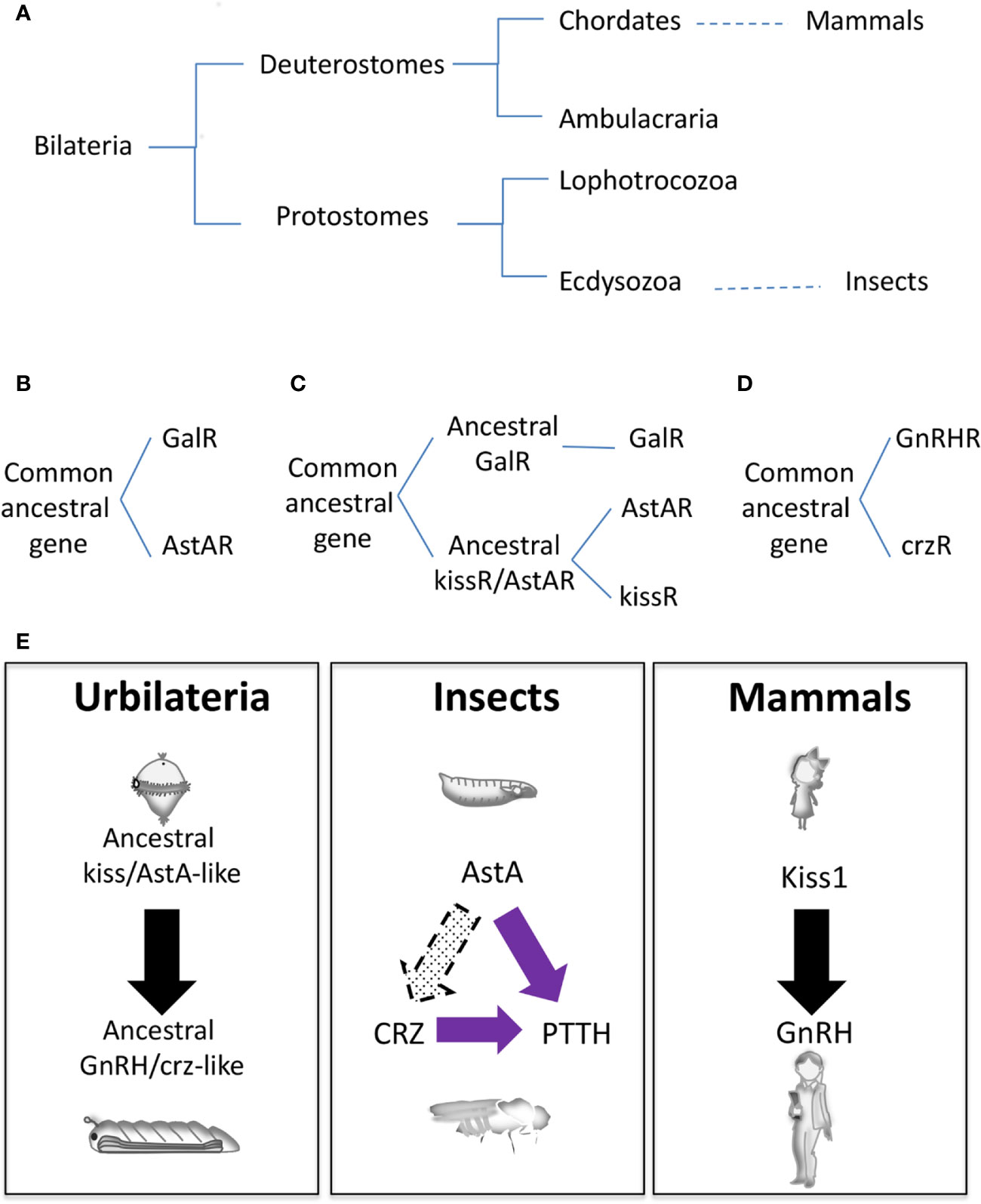
Figure 3 Evolutionary origins of the neuroendocrine gene network. (A) Animal phylogenetic diagram showing the position of mammals and insects. (B–D) Schematic representation of different hypothetical gene evolution for GalR, AstAR, kissR, GnRHR, and crzR. (E) Speculative neuroendocrine axis controlling J/A transition of Urbilateria (left) that has been evolutionary conserved in insects (center) and mammals (left).
Whereas AstA and Kiss1 are evolutionarily related, GnRH and ptth are non-homologous genes. PTTH, a cysteine knot protein, was the first hormone/neuropeptide identified in insects (82). It is part of the noggin/noggin-like family, an extracellular regulator of BMP (Bone Morphogenetic Protein) signaling first characterized in vertebrates (2). However, ptth has evolved from a noggin-like gene exclusively in insects being a common component of holometabolous, with no conservation in other insect species or in vertebrates (19). On the other hand, the PTTH receptor Torso (4) is an RTK, similar to the large numbers of RTKs present in animal genomes, making difficult its precise identification for evolutionary studies. However, some phylogenetic relationship analyses determine that torso appeared before the divergence of lophotrochozoans and ecdysozoans but not after the divergence of deuterostomes and protostomes (Figure 3A) (19).
GnRH neuropeptide and its receptor GnRHR do exist in Arthropoda, which include insects. Evolutionary analyses suggest that a common ancestral gene in the Bilateria family gave rise to GnRHR and crzR (corazonin receptor) through gene duplication; after that crzR was lost in the vertebrates (Figure 3D) (83, 84). The second gene duplication in Arthropoda from GnRHR gave rise to Adipokinetic Hormone (AKHR) and AKH/CRZ-related peptide Receptors (ACPR), the latter being lost in Drosophila (not shown). Functional experiments uncovered an essential role of AKH in energy/metabolism homeostasis, acting as the equivalent of the mammalian glucagon, and providing evidence that AKH does not function as the ortholog of GnRH (85). Accordingly, circuitry analyses in Drosophila demonstrated that AKH positive neurons do not show a direct innervation to the prothoracic gland as PTTH neurons do or as GnRH neurons connect with the pituitary gland (86). Interestingly, crz is expressed in a group of neurons (CRZn) that project towards both the PTTHn and PG. Early in development crzR is expressed and functional in PTTHn and not in the PG, thus regulating systemic growth PTTHn-mediated but not the metamorphosis timing (10). These observations may indicate that the CRZ-PTTH circuit is more likely a recent innovation in insects. Alternatively, a cross-talk between CRZ neurons and AstAn may exist, which would suggest a more persuasive homology between the mammalian and insect neuroendocrine systems than previously thought (10).
It is tempting to speculate that an ancestral common neurohormonal axis regulating metamorphosis existed in Urbilateria (the putative common ancestor of protostomes and deuterostomes), which would probably have an initial ciliated free-swimming (pelagic) larval phase (Figure 3E) (87). This axis would diverge to mammalian KISS1/GnRH and insect AstA/CRZ. Not surprisingly, non-chordate deuterostomes like sea cucumber and starfish have functional KISS and GnRH signaling, respectively (81, 88), and the metamorphosis of an ancient chordate like the Ascidian Ciona is triggered by GABA-induced GnRH release (89). In this scenario, PTTH/TORSO signaling might have been co-opted later on during insect evolution and somehow substituted the transitional developmental function of CRZ (Figure 3E). In contrast, CRZ kept its role in systemic growth and food intake during starvation-induced stress response (67). Supporting this hypothesis, in the diptera-like Oriental fruit fly (Batrocera dorsalis) CRZ signaling regulates juvenile-adult transition, although ptth/torso genes are also present in the genome with no discernable role on pupariation (90, 91). Also, the initiation of Manduca ecdysis depends on CRZ activity, and the role of ptth on Manduca pupariation is firmly established (82, 92). In summary, we propose that a functional neuroendocrine axis comprising ancestral KISS/GnRH-like signaling, which regulated juvenile-adult transition and probably also stress-related metabolism, existed in Urbilateria (Figure 3E). Despite posterior modifications in other functions and the incorporation of new genes, this surprising conservation among phyla would mean that separating immature juveniles and sexually mature adults might be a successful and most likely unique evolutionary event. It will be of interest to explore this hypothesis further.
Conclusions and Future Perspectives
The genetic network involved in the developmental switch display several functions in adulthood. While puberty and metamorphosis present common features regarding the central role of the brain controlling steroid production to initiate the developmental transition, the neuroendocrine axis looked simpler in insects than in mammals. The central neurons controlling gland hormone production involves GnRH and PTTH signaling pathways, with no sequence homology between GnRH/GnRHR and ptth/torso genes. These observations would suggest that both neurohormonal axes are just functionally analogous. However, we recently found that AstA is the Drosophila homolog for kisspeptin1, thus challenging this simple view. Moreover, a recent report on Drosophila crz together with novel evolutionary aspects of kiss-GnRH systems indicated that puberty and metamorphosis might be homologous processes. These data open the question of whether the neuroendocrine axis represents an evolutionary convergence or an evolutionary conserved mechanism timing Juvenile-to-adult transition between mammals and insects. Even if there are clear similarities between pubertal and metamorphic mechanism, several differences are also present.
In mammals, the adult HPG axis control steroid production to regulate fertility. In Drosophila, whereas it is not clear whether or not PTTH or CRZ regulate steroid production, steroid (ecdysone) does control adult fertility. The neuroendocrine gene network has also been found to regulate adult physiology and behavior both in mammals and Drosophila. GAL/KISS and AstA are involved in feeding/metabolism and sleep regulation, although with differential relevance. This may indicate not only the maintenance of putative ancestral metabolic roles but also the acquisition of novel functions. So the question is if we can model any dysregulations of the neuroendocrine axis or associated complex human pathologies (such as anxiety and addiction) in a simpler model system like Drosophila.
Future research might lead to a better understanding of the endocrine network evolution and confirm (or refute) the common origin of the neuroendocrine axis that controls the developmental transition to adulthood. Distinguishing similarities and differences in the juvenile to adult transition between mammals and invertebrates is an essential step in the process of using model organism to allows the identification of target elements to assay potential remedies to multifactorial ailments.
Author Contributions
CB: writing – original draft. BG-M: writing – original draft. DD: writing – original draft. NR: conceptualization, funding acquisition, project administration, writing – original draft. FM: conceptualization, funding acquisition, project administration, writing – original draft. All authors contributed to the article and approved the submitted version.
Funding
This work was supported by MICINN (Grant number PGC2018-094630-B-I00 to FM), Comunidad de Madrid (CB was a recipient of “Ayuda de Garantía Juvenil para la contratación de investigadores predoctorales” fellowship, grant number PEJD-2019-PRE/BMD-15940), CSIC (BG-M was a recipient of a JAE intro fellowship, grant number JAEINT_19_00602), Inserm (ATIP-Avenir program to NR) and the French National Research Agency -ANR- (“Investments for the Future” programs LABEX SIGNALIFE ANR-11-LABX-0028 and IDEX UCAJedi ANR-15-IDEX-01 to NR). FM is a recipient of a RyC-2014-14961 contract. MEFP (CGB is a recipient of a FPU predoctoral fellowship, grant number FPU19/04449), UAM (BG-M is a recipient of a predoctoral fellowship, grant number SFPI/2020/00878).
Conflict of Interest
DD was employed by company Sartorius Netherlands BV.
The remaining authors declare that the research was conducted in the absence of any commercial or financial relationships that could be construed as a potential conflict of interest.
Acknowledgments
The authors thank laboratory members for their insightful discussions and comments on the manuscript. We are especially grateful to Professor Alberto Ferrús, Dr. María Losada, and Dr. Sergio Casas-Tintó for reviewing the manuscript prior publication. We acknowledge support of the publication fee by the CSIC Open Access Publication Support Initiative through its Unit of Information Resources for Research (URICI).
References
1. Manfredi-Lozano M, Roa J, Tena-Sempere M. Connecting metabolism and gonadal function: Novel central neuropeptide pathways involved in the metabolic control of puberty and fertility. Front Neuroendocrinol (2018) 48:37–49. doi: 10.1016/j.yfrne.2017.07.008
2. Delanoue R, Romero NM. Growth and maturation in development: A fly’s perspective. Int J Mol Sci (2020) 21:1–12. doi: 10.3390/ijms21041260
3. McBrayer Z, Ono H, Shimell M, Parvy JP, Beckstead RB, Warren JT, et al. Prothoracicotropic Hormone Regulates Developmental Timing and Body Size in Drosophila. Dev Cell (2007) 13:857–71. doi: 10.1016/j.devcel.2007.11.003
4. Rewitz KF, Yamanaka N, Gilbert LI, O’Connor MB. The insect neuropeptide PTTH activates receptor tyrosine kinase torso to initiate metamorphosis. Science (80- ) (2009) 326:1403–5. doi: 10.1126/science.1176450
5. Cruz J, Martín D, Franch-Marro X. Egfr Signaling Is a Major Regulator of Ecdysone Biosynthesis in the Drosophila Prothoracic Gland. Curr Biol (2020) 30:1547–1554.e4. doi: 10.1016/j.cub.2020.01.092
6. Rewitz KF, O’Connor MB. Timing is everything: PTTH mediated DHR4 nucleocytoplasmic trafficking sets the tempo of drosophila steroid production. Front Endocrinol (Lausanne) (2011) 2(108):1–4. doi: 10.3389/fendo.2011.00108
7. Moeller ME, Thomas Danielsen E, Herder R, O’Connor MB, Rewitz KF. Dynamic feedback circuits function as a switch for shaping a maturation-inducing steroid pulse in Drosophila. Development (2013) 140:4730–9. doi: 10.1242/dev.099739
8. Christensen CF, Koyama T, Nagy S, Danielsen ET, Texada MJ, Halberg KA, et al. Ecdysone-dependent feedback regulation of prothoracicotropic hormone times developmental maturation. Development (2020) dev.188110. doi: 10.1242/dev.188110
9. Deveci D, Martin FA, Leopold P, Romero NM. AstA Signaling Functions as an Evolutionary Conserved Mechanism Timing Juvenile to Adult Transition. Curr Biol (2019) 29:813–22.e4. doi: 10.1016/j.cub.2019.01.053
10. Imura E, Shimada-Niwa Y, Nishimura T, Hückesfeld S, Schlegel P, Ohhara Y, et al. The Corazonin-PTTH Neuronal Axis Controls Systemic Body Growth by Regulating Basal Ecdysteroid Biosynthesis in Drosophila melanogaster. Curr Biol (2020) 30:2156–65.e5. doi: 10.1016/j.cub.2020.03.050
11. Yamanaka N, Marqués G, O’Connor MB. Vesicle-Mediated Steroid Hormone Secretion in Drosophila melanogaster. Cell (2015) 163:907–19. doi: 10.1016/j.cell.2015.10.022
12. Shimell MJ, Pan X, Martin FA, Ghosh AC, Leopold P, O’Connor MB, et al. Prothoracicotropic hormone modulates environmental adaptive plasticity through the control of developmental timing. Development (2018) 145:1–13. doi: 10.1242/dev.159699
13. Messina A, Langlet F, Chachlaki K, Roa J, Rasika S, Jouy N, et al. A microRNA switch regulates the rise in hypothalamic GnRH production before puberty. Nat Neurosci (2016) 19:835–44. doi: 10.1038/nn.4298
14. Kuiri-Hänninen T, Sankilampi U, Dunkel L. Activation of the hypothalamic-pituitary-gonadal axis in infancy: Minipuberty. Horm Res Paediatr (2014) 82:73–80. doi: 10.1159/000362414
15. Warren JT, Yerushalmi Y, Shimell MJ, O’Connor MB, Restifo LL, Gilbert LI. Discrete pulses of molting hormone, 20-hydroxyecdysone, during late larval development of Drosophila melanogaster: Correlations with changes in gene activity. Dev Dyn (2006) 235:315–26. doi: 10.1002/dvdy.20626
16. Brown JB, Boley N, Eisman R, May GE, Stoiber MH, Duff MO, et al. Diversity and dynamics of the Drosophila transcriptome. Nature (2014) 512:393–9. doi: 10.1038/nature12962
17. Boerjan B, Verleyen P, Huybrechts J, Schoofs L, De Loof A. In search for a common denominator for the diverse functions of arthropod corazonin: A role in the physiology of stress? Gen Comp Endocrinol (2010) 166(2):22–223. doi: 10.1016/j.ygcen.2009.09.004
18. Hamoudi Z, Lange AB, Orchard I. Identification and characterization of the Corazonin receptor and possible physiological roles of the Corazonin-signaling pathway in rhodnius prolixus. Front Neurosci (2016) 10:1–12. doi: 10.3389/fnins.2016.00357
19. Skelly J, Pushparajan C, Duncan EJ, Dearden PK. Evolution of the Torso activation cassette, a pathway required for terminal patterning and moulting. Insect Mol Biol (2019) 28:392–408. doi: 10.1111/imb.12560
20. Selcho M, Millán C, Palacios-Muñoz A, Ruf F, Ubillo L, Chen J, et al. Central and peripheral clocks are coupled by a neuropeptide pathway in Drosophila. Nat Commun (2017) 8:1–13. doi: 10.1038/ncomms15563
21. Liu X, Herbison AE. Kisspeptin regulation of neuronal activity throughout the central nervous system. Endocrinol Metab (2016) 31:193–205. doi: 10.3803/EnM.2016.31.2.193
22. Swevers L. An update on ecdysone signaling during insect oogenesis. Curr Opin Insect Sci (2019) 31:8–13. doi: 10.1016/j.cois.2018.07.003
23. Chen J, Reiher W, Hermann-Luibl C, Sellami A, Cognigni P, Kondo S, et al. Allatostatin A Signalling in Drosophila Regulates Feeding and Sleep and Is Modulated by PDF. PloS Genet (2016) 12(9):e1006346. doi: 10.1371/journal.pgen.1006346
24. Lin S, Senapati B, Tsao CH. Neural basis of hunger-driven behaviour in Drosophila. Open Biol (2019) 9:1–13. doi: 10.1098/rsob.180259
25. Tsao CH, Chen CC, Lin CH, Yang HY, Lin S. Drosophila mushroom bodies integrate hunger and satiety signals to control innate food-seeking behavior. Elife (2018) 7:1–35. doi: 10.7554/eLife.35264
26. Yamagata N, Hiroi M, Kondo S, Abe A, Tanimoto H. Suppression of Dopamine Neurons Mediates Reward. PloS Biol (2016) 14:1–16. doi: 10.1371/journal.pbio.1002586
27. Donlea JM, Pimentel D, Talbot CB, Kempf A, Omoto JJ, Hartenstein V, et al. Recurrent Circuitry for Balancing Sleep Need and Sleep. Neuron (2018) 97:378–89.e4. doi: 10.1016/j.neuron.2017.12.016
28. Sitaraman D, Aso Y, Rubin GM, Nitabach MN. Control of Sleep by Dopaminergic Inputs to the Drosophila Mushroom Body. Front Neural Circuits (2015) 9:73. doi: 10.3389/fncir.2015.00073
29. Fang P, Yu M, Shi M, Bo P, Zhang Z. Galanin peptide family regulation of glucose metabolism. Front Neuroendocrinol (2020) 56:1–8. doi: 10.1016/j.yfrne.2019.100801
30. Qualls-Creekmore E, Yu S, Francois M, Hoang J, Huesing C, Bruce-Keller A, et al. Galanin-expressing GABA neurons in the lateral hypothalamus modulate food reward and noncompulsive locomotion. J Neurosci (2017) 37:6053–65. doi: 10.1523/JNEUROSCI.0155-17.2017
31. Qiu J, Rivera HM, Bosch MA, Padilla SL, Stincic TL, Palmiter RD, et al. Estrogenic-dependent glutamatergic neurotransmission from kisspeptin neurons governs feeding circuits in females. Elife (2018) 7:1–34. doi: 10.7554/eLife.35656
32. Tolson KP, Garcia C, Delgado I, Marooki N, Kauffman AS. Metabolism and energy expenditure, but not feeding or glucose tolerance, are impaired in young kiss1r ko female mice. Endocrinology (2016) 157:4192–9. doi: 10.1210/en.2016-1501
33. Tolson KP, Marooki N, Wolfe A, Smith JT, Kauffman AS. Cre/lox generation of a novel whole-body Kiss1r KO mouse line recapitulates a hypogonadal, obese, and metabolically-impaired phenotype. Mol Cell Endocrinol (2019) 498:1–8. doi: 10.1016/j.mce.2019.110559
34. Manfredi-Lozano M, Roa J, Ruiz-Pino F, Piet R, Garcia-Galiano D, Pineda R, et al. Defining a novel leptin–melanocortin–kisspeptin pathway involved in the metabolic control of puberty. Mol Metab (2016) 5:844–57. doi: 10.1016/j.molmet.2016.08.003
35. Ma Y, Miracca G, Yu X, Harding EC, Miao A, Yustos R, et al. Galanin Neurons Unite Sleep Homeostasis and α2-Adrenergic Sedation. Curr Biol (2019) 29:3315–3322.e3. doi: 10.1016/j.cub.2019.07.087
36. Padilla SL, Perez JG, Ben-Hamo M, Johnson CW, Sanchez REA, Bussi IL, et al. Kisspeptin Neurons in the Arcuate Nucleus of the Hypothalamus Orchestrate Circadian Rhythms and Metabolism. Curr Biol (2019) 29:592–604.e4. doi: 10.1016/j.cub.2019.01.022
37. Koob GF, Le Moal M. Addiction and the Brain Antireward System. Annu Rev Psychol (2008) 59:29–53. doi: 10.1146/annurev.psych.59.103006.093548
38. Genders SG, Scheller KJ, Djouma E. Neuropeptide modulation of addiction: Focus on galanin. Neurosci Biobehav Rev (2020) 110:133–49. doi: 10.1016/j.neubiorev.2018.06.021
39. Delmas S, Porteous R, Bergin DH, Herbison AE. Altered aspects of anxiety-related behavior in kisspeptin receptor-deleted male mice. Sci Rep (2018) 8:1–7. doi: 10.1038/s41598-018-21042-4
40. Hellier V, Brock O, Candlish M, Desroziers E, Aoki M, Mayer C, et al. Female sexual behavior in mice is controlled by kisspeptin neurons. Nat Commun (2018) 9:1–12. doi: 10.1038/s41467-017-02797-2
41. Pratavieira M, Menegasso ARDS, Esteves FG, Sato KU, Malaspina O, Palma MS. MALDI Imaging Analysis of Neuropeptides in Africanized Honeybee (Apis mellifera) Brain: Effect of Aggressiveness. J Proteome Res (2018) 17:2358–69. doi: 10.1021/acs.jproteome.8b00098
42. Chen W, Liu Z, Li T, Zhang R, Xue Y, Zhong Y, et al. Regulation of Drosophila circadian rhythms by miRNA let-7 is mediated by a regulatory cycle. Nat Commun (2014) 5:1–13. doi: 10.1038/ncomms6549
43. Nässel DR, Zandawala M. Recent advances in neuropeptide signaling in Drosophila, from genes to physiology and behavior. Prog Neurobiol (2019) 179:101607. doi: 10.1016/j.pneurobio.2019.02.003
44. Kapan N, Lushchak OV, Luo J, Nässel DR. Identified peptidergic neurons in the Drosophila brain regulate insulin-producing cells, stress responses and metabolism by coexpressed short neuropeptide F and corazonin. Cell Mol Life Sci (2012) 69:4051–66. doi: 10.1007/s00018-012-1097-z
45. Kubrak OI, Lushchak OV, Zandawala M, Nässel DR. Systemic corazonin signalling modulates stress responses and metabolism in Drosophila. Open Biol (2016) 6:160152. doi: 10.1098/rsob.160152
46. Andreatta G, Broyart C, Borghgraef C, Vadiwala K, Kozin V, Polo A, et al. Corazonin signaling integrates energy homeostasis and lunar phase to regulate aspects of growth and sexual maturation in Platynereis. Proc Natl Acad Sci USA (2020) 117:1097–106. doi: 10.1073/pnas.1910262116
47. Tayler TD, Pacheco DA, Hergarden AC, Murthy M, Anderson DJ. A neuropeptide circuit that coordinates sperm transfer and copulation duration in Drosophila. Proc Natl Acad Sci USA (2012) 109:20697–702. doi: 10.1073/pnas.1218246109
48. Zer-Krispil S, Zak H, Shao L, Ben-Shaanan S, Tordjman L, Bentzur A, et al. Ejaculation Induced by the Activation of Crz Neurons Is Rewarding to Drosophila Males. Curr Biol (2018) 28:1445–52.e3. doi: 10.1016/j.cub.2018.03.039
49. Casoni F, Malone SA, Belle M, Luzzati F, Collier F, Allet C, et al. Development of the neurons controlling fertility in humans: New insights from 3D imaging and transparent fetal brains. Dev (2016) 143:3969–81. doi: 10.1242/dev.139444
50. Martínez-Moreno CG, Calderón-Vallejo D, Harvey S, Arámburo C, Quintanar JL. Growth hormone (GH) and gonadotropin-releasing hormone (GnRH) in the central nervous system: A potential neurological combinatory therapy? Int J Mol Sci (2018) 19:1–21. doi: 10.3390/ijms19020375
51. Xia L, Chen GH, Li ZH, Jiang S, Shen J. Alterations in Hypothalamus-Pituitary-Adrenal/Thyroid Axes and Gonadotropin-Releasing Hormone in the Patients with Primary Insomnia: A Clinical Research. PloS One (2013) 8:1–21. doi: 10.1371/journal.pone.0071065
52. Joffe H, Crawford S, Economou N, Kim S, Regan S, Hall JE, et al. A Gonadotropin-Releasing Hormone Agonist Model Demonstrates That Nocturnal Hot Flashes Interrupt Objective Sleep. Sleep (2013) 36:1977–85. doi: 10.5665/sleep.3244
53. López M, Tena-Sempere M. Estrogens and the control of energy homeostasis: A brain perspective. Trends Endocrinol Metab (2015) 26:411–21. doi: 10.1016/j.tem.2015.06.003
54. Fester L, Rune GM. Sexual neurosteroids and synaptic plasticity in the hippocampus. Brain Res (2015) 1621:162–9. doi: 10.1016/j.brainres.2014.10.033
55. Herde MK, Iremonger KJ, Constantin S, Herbison AE. GnRH neurons elaborate a long-range projection with shared axonal and dendritic functions. J Neurosci (2013) 33:12689–97. doi: 10.1523/JNEUROSCI.0579-13.2013
56. Iremonger KJ, Herbison AE. Multitasking in gonadotropin-releasing hormone neuron dendrites. Neuroendocrinology (2015) 102:1–7. doi: 10.1159/000368364
57. Clasadonte J, Prevot V. The special relationship: Glia-neuron interactions in the neuroendocrine hypothalamus. Nat Rev Endocrinol (2018) 14:25–44. doi: 10.1038/nrendo.2017.124
58. Siegmund T, Korge G. Innervation of the ring gland of Drosophila melanogaster. J Comp Neurol (2001) 431:481–91. doi: 10.1002/1096-9861(20010319)431:4<481::AID-CNE1084>3.0.CO;2-7
59. Wang L, Guo W, Shen X, Yeo S, Long H, Wang Z, et al. Different dendritic domains of the GnRH neuron underlie the pulse and surge modes of GnRH secretion in female mice. Elife (2020) 9:1–19. doi: 10.7554/eLife.53945
60. Giacobini P, Parkash J, Campagne C, Messina A, Casoni F, Vanacker C, et al. Brain Endothelial Cells Control Fertility through Ovarian-Steroid–Dependent Release of Semaphorin 3A. PloS Biol (2014) 12:e1001808. doi: 10.1371/journal.pbio.1001808
61. Anbalagan S, Gordon L, Blechman J, Leshkowitz D, Stainier YR, Correspondence GL. Pituicyte Cues Regulate the Development of Permeable Neuro-Vascular Interfaces. Dev Cell (2018) 47:711–26.e5. doi: 10.1016/j.devcel.2018.10.017
62. Hanchate NK, Giacobini P, Lhuillier P, Parkash J, Espy C, Fouveaut C, et al. SEMA3A, a Gene Involved in Axonal Pathfinding, Is Mutated in Patients with Kallmann Syndrome. PloS Genet (2012) 47:711–26. doi: 10.1371/journal.pgen.1002896
63. Marcos S, Monnier C, Rovira X, Fouveaut C, Pitteloud N, Ango F, et al. Defective signaling through plexin-A1 compromises the development of the peripheral olfactory system and neuroendocrine reproductive axis in mice. Hum Mol Genet (2017) 26(11):2006–17. doi: 10.1093/hmg/ddx080
64. Cariboni A, André V, Chauvet S, Cassatella D, Davidson K, Caramello A, et al. Dysfunctional SEMA3E signaling underlies gonadotropin-releasing hormone neuron deficiency in Kallmann syndrome. J Clin Invest (2015) 125(6):2413–28. doi: 10.1172/JCI78448
65. Oleari R, Andrè V, Lettieri A, Tahir S, Roth L, Paganoni A, et al. A novel SEMA3G mutation in two siblings affected by syndromic GnRH deficiency. Neuroendocrinology (2020) 1–21. doi: 10.1159/000508375
66. Vanacker C, Trova S, Shruti S, Casoni F, Messina A, Croizier S, et al. Neuropilin-1 expression in GnRH neurons regulates prepubertal weight gain and sexual attraction. EMBO J (2020) 39(19):1–17. doi: 10.15252/embj.2020104633
67. Battistini C, Tamagnone L. Transmembrane semaphorins, forward and reverse signaling: Have a look both ways. Cell Mol Life Sci (2016) 73:1609–22. doi: 10.1007/s00018-016-2137-x
68. Danielsen ET, Moeller ME, Yamanaka N, Ou Q, Laursen JM, Soenderholm C, et al. A Drosophila Genome-Wide Screen Identifies Regulators of Steroid Hormone Production and Developmental Timing. Dev Cell (2016) 37:558–70. doi: 10.1016/j.devcel.2016.05.015
69. Da DJ, Mizoguchi A, Satake S, Ishizaki H, Gilbert LI. Developmental changes in the prothoracicotropic hormone content of the Bombyx mori brain - Retrocerebral complex and hemolymph: Analysis by immunogold electron microscopy, quantitative image analysis, and time-resolved fluoroimmunoassay. Dev Biol (1995) 171:212–23. doi: 10.1006/dbio.1995.1272
70. Riddiford LM. Hormonal regulation of gene expression during lepidopteran development. In: Goldsmith M, Wilkins A, editors. Molecular Model Systems in the Lepi- doptera. New York: Cambridge (1995).
71. Mirth CK, Shingleton AW. The roles of juvenile hormone, insulin/target of rapamycin, and ecydsone signaling in regulating body size in Drosophila. Commun Integr Biol (2014) 7:1–3. doi: 10.4161/cib.29240
72. Zhang T, Song W, Li Z, Qian W, Wei L, Yang Y, et al. Krüppel homolog 1 represses insect ecdysone biosynthesis by directly inhibiting the transcription of steroidogenic enzymes. Proc Natl Acad Sci USA (2018) 115(15):3960–65. doi: 10.1073/pnas.1800435115
73. Liu S, Li K, Gao Y, Liu X, Chen W, Ge W, et al. Antagonistic actions of juvenile hormone and 20-hydroxyecdysone within the ring gland determine developmental transitions in Drosophila. Proc Natl Acad Sci USA (2018) 115:139–44. doi: 10.1073/pnas.1716897115
74. Truman JW. Steroid hormone secretion in insects comes of age. Proc Natl Acad Sci U S A (2006) 103(24):8909–10. doi: 10.1073/pnas.0603252103
75. Jékely G. Global view of the evolution and diversity of metazoan neuropeptide signaling. Proc Natl Acad Sci USA (2013) 110:8702–7. doi: 10.1073/pnas.1221833110
76. Mirabeau O, Joly JS. Molecular evolution of peptidergic signaling systems in bilaterians. Proc Natl Acad Sci USA (2013) 110:E2028–37. doi: 10.1073/pnas.1219956110
77. Felix RC, Trindade M, Pires IRP, Fonseca VG, Martins RS, Silveira H, et al. Unravelling the Evolution of the Allatostatin-Type A, KISS and Galanin Peptide-Receptor Gene Families in Bilaterians: Insights from Anopheles Mosquitoes. PloS One (2015) 10:e0130347. doi: 10.1371/journal.pone.0130347
78. Cardoso JCR, Félix RC, Bjärnmark N, Power DM. Allatostatin-type A, kisspeptin and galanin GPCRs and putative ligands as candidate regulatory factors of mantle function. Mar Genomics (2016) 27:25–35. doi: 10.1016/j.margen.2015.12.003
79. Elphick MR, Mirabeau O, Larhammar D. Evolution of neuropeptide signalling systems. J Exp Biol (2018) 221:jeb151092. doi: 10.1242/jeb.151092
80. Kim DK, Yun S, Son GH, Hwang JI, Park CR, Il KJ, et al. Coevolution of the spexin/galanin/kisspeptin family: Spexin activates galanin receptor type II and III. Endocrinology (2014) 155:1864–73. doi: 10.1210/en.2013-2106
81. Wang T, Cao Z, Shen Z, Yang J, Chen X, Yang Z, et al. Existence and functions of a kisspeptin neuropeptide signaling system in a non-chordate deuterostome species. Elife (2020) 9:1–28. doi: 10.7554/eLife.53370
82. Nagasawa H, Guo F, Zhong XC, Xia BY, Wang ZS, Qui XJ, et al. Large-scale purification of prothoracicotropic hormone of the silkworm (Bombyx mori) - PubMed. Sci Sin (1980) 23(8):1053–60.
83. Tian S, Zandawala M, Beets I, Baytemur E, Slade SE, Scrivens JH, et al. Urbilaterian origin of paralogous GnRH and corazonin neuropeptide signalling pathways. Sci Rep (2016) 6:1–7. doi: 10.1038/srep28788
84. Zandawala M, Moghul I, Guerra LAY, Delroisse J, Abylkassimova N, Hugall AF, et al. Discovery of novel representatives of bilaterian neuropeptide families and reconstruction of neuropeptide precursor evolution in ophiuroid echinoderms. Open Biol (2017) 7:1–20. doi: 10.1098/rsob.170129
85. Bharucha KN, Tarr P, Zipursky SL. A glucagon-like endocrine pathway in Drosophila modulates both lipid and carbohydrate homeostasis. J Exp Biol (2008) 211:3103–10. doi: 10.1242/jeb.016451
86. Nässel DR. Substrates for neuronal cotransmission with neuropeptides and small molecule neurotransmitters in drosophila. Front Cell Neurosci (2018) 12:83. doi: 10.3389/fncel.2018.00083
87. De Robertis EM. Evo-Devo: Variations on Ancestral Themes. Cell (2008) 132:185–95. doi: 10.1016/j.cell.2008.01.003
88. Tian S, Egertová M, Elphick MR. Functional characterization of paralogous gonadotropin-releasing hormone-type and corazonin-type neuropeptides in an echinoderm. Front Endocrinol (Lausanne) (2017) 8(259):1–24. doi: 10.3389/fendo.2017.00259
89. Hozumi A, Matsunobu S, Mita K, Treen N, Sugihara T, Horie T, et al. GABA-Induced GnRH Release Triggers Chordate Metamorphosis. Curr Biol (2020) 30:1555–61.e4. doi: 10.1016/j.cub.2020.02.003
90. Hou Q-L, Jiang H-B, Gui S-H, Chen E-H, Wei D-D, Li H-M, et al. A Role of Corazonin Receptor in Larval-Pupal Transition and Pupariation in the Oriental Fruit Fly Bactrocera dorsalis (Hendel) (Diptera: Tephritidae). Front Physiol (2017) 8:77. doi: 10.3389/fphys.2017.00077
91. Dong Y, Desneux N, Lei C, Niu C. Transcriptome characterization analysis of Bactrocera minax and new insights into its pupal diapause development with gene expression analysis. Int J Biol Sci (2014) 10:1051–63. doi: 10.7150/ijbs.9438
Keywords: metamorphosis, puberty, Urbilateria, Drosophila, sleep, juvenile-adult transition, neuro-hormonal regulation, neuroendocrine axis
Citation: Barredo CG, Gil-Marti B, Deveci D, Romero NM and Martin FA (2021) Timing the Juvenile-Adult Neurohormonal Transition: Functions and Evolution. Front. Endocrinol. 11:602285. doi: 10.3389/fendo.2020.602285
Received: 02 September 2020; Accepted: 28 December 2020;
Published: 12 February 2021.
Edited by:
Susan Zup, University of Massachusetts Boston, United StatesReviewed by:
Vincent Prevot, Institut National de la Santé et de la Recherche Médicale (INSERM), FranceXavier Franch-Marro, Institute of Evolutionary Evolution CSIC-UPF, Spain
Copyright © 2021 Barredo, Gil-Marti, Deveci, Romero and Martin. This is an open-access article distributed under the terms of the Creative Commons Attribution License (CC BY). The use, distribution or reproduction in other forums is permitted, provided the original author(s) and the copyright owner(s) are credited and that the original publication in this journal is cited, in accordance with accepted academic practice. No use, distribution or reproduction is permitted which does not comply with these terms.
*Correspondence: Nuria M. Romero, TnVyaWEuUk9NRVJPQHVuaXYtY290ZWRhenVyLmZy; Francisco A. Martin, ZmFtYXJ0aW5AY2FqYWwuY3NpYy5lcw==
†These authors have contributed equally to this work