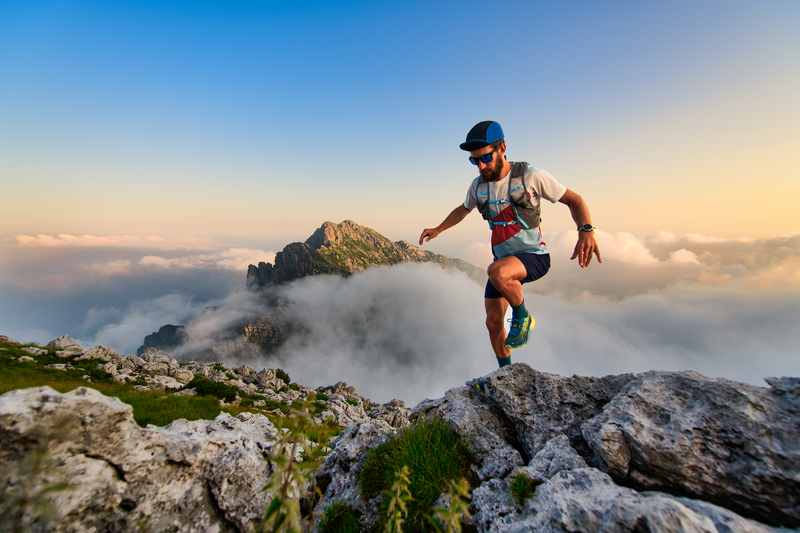
95% of researchers rate our articles as excellent or good
Learn more about the work of our research integrity team to safeguard the quality of each article we publish.
Find out more
REVIEW article
Front. Endocrinol. , 04 November 2020
Sec. Neuroendocrine Science
Volume 11 - 2020 | https://doi.org/10.3389/fendo.2020.566026
This article is part of the Research Topic Involvement of Bioactive Peptides in the Control of Cell Survival, Proliferation and Plasticity in Physiological and Pathological Conditions View all 16 articles
Octadecaneuropeptide (ODN) and its precursor diazepam-binding inhibitor (DBI) are peptides belonging to the family of endozepines. Endozepines are exclusively produced by astroglial cells in the central nervous system of mammals, and their release is regulated by stress signals and neuroactive compounds. There is now compelling evidence that the gliopeptide ODN protects cultured neurons and astrocytes from apoptotic cell death induced by various neurotoxic agents. In vivo, ODN causes a very strong neuroprotective action against neuronal degeneration in a mouse model of Parkinson's disease. The neuroprotective activity of ODN is based on its capacity to reduce inflammation, apoptosis, and oxidative stress. The protective effects of ODN are mediated through its metabotropic receptor. This receptor activates a transduction cascade of second messengers to stimulate protein kinase A (PKA), protein kinase C (PKC), and mitogen-activated protein kinase (MAPK)-extracellular signal-regulated kinase (ERK) signaling pathways, which in turn inhibits the expression of proapoptotic factor Bax and the mitochondrial apoptotic pathway. In N2a cells, ODN also promotes survival and stimulates neurite outgrowth. During the ODN-induced neuronal differentiation process, numerous mitochondria and peroxisomes are identified in the neurites and an increase in the amount of cholesterol and fatty acids is observed. The antiapoptotic and neurotrophic properties of ODN, including its antioxidant, antiapoptotic, and pro-differentiating effects, suggest that this gliopeptide and some of its selective and stable derivatives may have therapeutic value for the treatment of some neurodegenerative diseases.
The existence of binding sites for benzodiazepines (BZs), the most widely prescribed and therapeutically used drugs for their anxiolytic, sedative, and muscle relaxant properties, has prompted several teams to search for endogenous ligands of the BZ receptors. Thus, the team of Erminio Costa has isolated from rat brain extracts an 11-kDa polypeptide able to competitively displace tritiated diazepam on synaptosomes, which has been called diazepam binding inhibitor (DBI) (1). Two major compounds, generated from DBI, have been identified in rat brains, the triakontatetraneuropeptide (TTN, DBI17−50) and the octadecaneuropeptide (ODN, DBI33−50). DBI and its processing products, collectively grouped under the generic term of endozepines (2), are currently considered as the endogenous ligands of BZ receptors. DBI is also known as an acyl-coenzyme A-binding protein (ACBP) due to its ability to bind acyl-coenzyme A esters and to stimulate fatty acid synthesis in different cell types (3).
In this review, which will only refer to endozepines as natural ligands of BZ receptors, the acronym DBI will be used. In addition, we will focus on ODN, which is the major form of endozepines produced in the brain and whose structure has been well-preserved during evolution, suggesting that it exerts important biological functions in the central nervous system (CNS). Supporting this hypothesis, high concentrations of DBI mRNA and DBI-derived peptides are detected in the rat brain during ontogenesis. Furthermore, it has been reported that in the cerebellar cortex, ODN is expressed by Bergmann glia, which controls cerebellar granule neuron migration (4), indicating that ODN may act as a neurotrophic factor during brain development.
Cloning and characterization of cDNAs encoding DBI were first performed from a rat brain cDNA library (5). Since then, DBI has been cloned from other tissues (adrenal, liver, and testis) and from various animal species, including human (6), cattle (7), frog (8), and carp (9). The cDNA encoding DBI has also been cloned in invertebrate species such as the fruit fly Drosophila melanogaster (10), the butterfly Bombyx mori (11), and the tobacco maggot Manduca sexta (12), different plant species such as Arabidopsis thaliana (13), and unicellular organisms such as the yeast Saccharomyces cerevisiae (14) and the bacteria Escherichia coli (15, 16).
Southern blot analysis of genomic DNA from human (6), rat (5), and mouse (17) revealed the existence of several genes encoding DBI, but only one of which is functional. In human, the active gene is located on the q12-21 region of the long arm of chromosome 2 (18) and the pseudogenes are located on chromosomes 5, 6, 11, and 14 (19). The human and murine genes are organized into four exons (E1–E4), coding for regions 1–2, 3–41, 42–62, and 63–86 of the DBI, respectively (20, 21), while in Drosophila, there are only three exons (10). In human and murine adipocytes, a novel exon, named E1c, has been identified in the DBI gene, which codes with the three other exons for a longer transcript. Genomic DNA analysis shows that the E1c exon is located in the intron between exons E1 and E2, and that its expression would be under the control of a second promoter located in intron 1 (22, 23). These data reinforce the work of Helledie et al. (24), which showed that the DBI gene, cloned from human and murine adipocytes, contains a functional consensus sequence on intron 1, the peroxisome proliferator-activated receptor (PPAR)-response element (PPRE), capable of binding the transcription factors PPARγ and retinoid X receptor α. All these data indicate that the expression of the DBI gene is under the control of two promoter regions (P1 and P2) located upstream and downstream of the E1 exon initially described in human and rat (Figure 1).
Figure 1. Schematic representation of the organization of the diazepam-binding inhibitor (DBI) gene in humans. The exons (E) are numbered from 1 to 4. Alternative splicing of exon 1, which is composed of three sub-exons E1a, E1b, E1c, generates DBI 1a (86 amino acids), 1b (105 amino acids), and 1c (87 amino acids). The letters in the blue rectangles correspond to the amino acid sequence of the regions translated by E1a, E1b, and E1c. The P1 promoter region controls the expression of DBI 1a and 1b. The P1 and P2 promoters control the expression of DBI 1c. The green arrows correspond to the sites of transcriptional initiation from promoters P1 and P2. The regions that are common to the three DBI isoforms are represented by rectangles in purple. The basic residues are noted in red, and the underlined amino acids correspond to the sequences of triakontatetraneuropeptide (TTN) (DBI17−50) and octadecaneuropeptide (ODN) (DBI33−50). NFY, nuclear transcription factor Y; SP1, transcription factor specificity protein 1; SRE, sterol response element; PPRE, peroxisome proliferator-response element.
The absence of TATA and CCAAT boxes, the presence of several element initiators of transcription, and the high content of nucleotide acids C and G in a promoter are often considered as the hallmarks of a domestic gene. Nevertheless, the expression level of DBI gene is regulated in different cell types (25). In addition, the P1 and P2 regions of the DBI gene have several consensus sequences for transcription factors including a glucocorticoid-response element (GRE), a CAAT-binding transcription factor/nuclear factor 1 (CTF/NF1), a hepatocyte nuclear factor (HNF), a sterol regulatory element-like sequence (SRE), and a PPAR sequence. Moreover, it has been reported that glucose and hormones, such as insulin or androgens, regulate the expression of the DBI gene (21, 26–28). Several research groups have also shown a modification of the levels of DBI gene expression in the brain in various experimental conditions. For instance, a decrease in DBI mRNA levels is observed after starvation (29) or castration (30) in rat, while an increase of DBI expression is shown after high-frequency vestibular stimulation in rabbits (31). Drug addiction (alcohol, morphine, and nicotine) also leads to an increase in DBI gene expression in the rodent brain (32, 33), and abrupt interruption of the consumption of these substances further increases DBI mRNA levels (33). In vitro studies on cultured astrocytes have shown that DBI gene expression is stimulated by different cell stress inducers such as peptide β-amyloid (34) and hydrogen peroxide (H2O2) (35). All these observations reveal that the DBI gene cannot be considered as a “housekeeping gene.”
In all vertebrate species studied, endozepines are present both in the CNS and in many peripheral organs and tissues (25, 36). Although DBI and its derivatives are found in the gonads (126 pM), duodenum (100 pM), kidneys (73 pM), heart (30 pM), liver (22 pM), skeletal muscle (18 pM), adrenals (15 pM), lungs (13 pM), and spleen (11 pM) (25), the highest concentrations are found in rat brain (10–50 μM). Peptides of the ODN family are widely distributed in the brain, with the highest levels measured in the olfactory bulb, hypothalamus, hippocampus, cerebellum, striatum, cerebral cortex, and circumventricular organs (Table 1).
Table 1. Octadecaneuropeptide-like immunoreactivity (ODN-LI) content in different rat brain structures.
A limited number of immunocytochemical studies using antibodies against DBI show a localization of endozepines in neurons (37, 38). However, the majority of the data in the literature indicate that endozepines are primarily expressed in glial cells (25). For example, ODN- and/or DBI-like immunoreactivities are detected in astrocytes in many brain areas including the cerebral cortex, hippocampus, amygdala, and olfactory bulb (39, 40), in the ependymocytes bordering the cerebral ventricles (39, 41), in the tanycytes of the median eminence (39, 42), in Bergmann cells from the cerebellum (40), and in Gomori-positive astrocytes from the arcuate nucleus (43). Similarly, in the peripheral nervous system, immunostaining for ODN and/or DBI is associated with Schwann cells (44). Finally, in the retina, endozepines are exclusively expressed by specialized glial cells, the Müller cells (45).
Ultrastructural studies revealed that DBI immunoreactivity is diffused throughout the cytoplasm (39), excluding its packaging in secretory vesicles. This is consistent with the absence of signal peptide in the primary sequence of DBI that could direct the protein into the endoplasmic reticulum/Golgi system. Nevertheless, mass spectrometry analysis of rat astroglial cell secretome revealed the presence of DBI in incubation media (46). The endozepine secretion is insensitive to brefeldin A, an inhibitor of Golgi vesicular transport (46), and can be stimulated by induction of autophagy by rapamycin (47). These data indicate that DBI and its derived peptides may be released by a mechanism independent of the conventional vesicular exocytosis pathway. The translocation of endozepines to the extracellular space could be provided by carriers called ATP-binding cassettes (ABCs), as demonstrated for the release of interleukin (IL)-1β by astrocytes (48) and annexin 1 by follicle-stellar cells (49), two proteins whose precursors are devoid of a signal peptide sequence. Consistent with this hypothesis, a site-directed mutagenesis study conducted with the amoeba Dictyostelium discoideum has shown the existence of a direct interaction between DBI and ABC-serine protease transporter (TagA) (50).
Although the mechanism of secretion of ODN and other DBI-derived peptides is not yet clearly elucidated, the secretion of endozepines is modulated in different pathophysiological situations and regulated by many factors. An assay using antibodies against ODN shows that the level of ODN-like immunoreactivity (ODN-LI) is increased in the plasma of septic shock patients (51). In vitro studies reveal that the release of ODN-LI is positively regulated by numerous neuroactive compounds, including neuropeptides [pituitary adenylate cyclase-activating polypeptide (PACAP) (52), urotensin-II (UII), UII-related peptide (53), and peptide β-amyloid (54)], steroid hormones [cortisol, pregnenolone, or progesterone (55)], cytokines [tumor necrosis factor-α and IL-1β (56)], or elevated extracellular potassium concentrations (57), and conversely repressed by the neuropeptide somatostatin (58) and the neurotransmitter gamma aminobutyric acid (GABA) (59). Pharmacological studies indicate that the release of ODN-LI from astroglial and retinal cells is mediated by ABC transporter activity through a mechanism that is dependent on the phosphorylation by protein kinases A and C (PKA and PKC) (52, 54, 60) (Figure 2), and blockage of the phosphorylation of ABC transporter inhibits the basal release of ODN-LI by about 50%. A phosphorylated form of ODN is also released by astrocytes but appears less active than the non-phosphorylated peptide, suggesting the existence of a posttranslational regulation mechanism (61).
Figure 2. Intracellular pathways involved in the regulation of the release of diazepam-binding inhibitor (DBI)-related peptides from cultured astroglial cells. Activation of cAMP/protein kinase A (PKA) and Diacylglycerol (DAG)/protein kinase C (PKC) cascades stimulates the release of DBI-related peptides through phosphorylation, at consensus sites, of transmembrane ATP-binding cassette transporters (ABC-Ts). The production of the octadecaneuropeptide (ODN), which occurs extracellularly, is finely regulated (positively or negatively) by various neuronal mediators, including classical neurotransmitters and neuropeptides, and under cerebral pathologies and oxidative injuries.
In culture media from astrocytes exposed to moderate oxidative stress, the quantity of authentic ODN is significantly higher than that detected in culture media from control astrocytes (35). Clinical studies have shown that the levels of endozepines, ODN, and DBI, increase in the plasma or cerebrospinal fluid (CSF) of patients suffering from pathological disorders related to oxidative stress, such as systemic inflammation, hepatic encephalopathy, and neurodegenerative diseases (25). Such an increase in ODN release could be responsive for the stimulation of antioxidative defenses (induction of Mn-superoxide dismutases, catalase, glutathione peroxidase-1, sulfiredoxin-1 gene transcription, and glutathione biosynthesis) in glia and neurons (62, 63) in agreement with the emerging concept indicating that ODN acts as a potential cytoprotective actor preventing the deleterious action of oxidative insults.
As endogenous ligands of BZ receptors, ODN interacts with the central-type BZ receptors, which is an integrated component of the GABA-A receptor (GABA-A-R)/Cl− channel complex (64, 65). Like DBI, ODN has been shown to displace diazepam and modulate GABAergic transmission via an allosteric reaction (1). Electrophysiology and cell functional studies indicate that ODN may act as an agonist, i.e., a positive allosteric modulator (PAM) promoting the action of GABA, or as an inverse agonist, i.e., a negative allosteric modulator (NAM) reducing the action of GABA, depending on the subunit composition of the GABA-A-R (57, 66). Studies conducted on cultured astrocytes have demonstrated that ODN is also the endogenous ligand of a G protein-coupled receptor, which is functionally and pharmacologically different from the conventional BZ receptors (67, 68). In particular, ODN stimulates the metabolism of polyphosphoinositides (PIPs) in rat astrocytes and cerebellar granule neurons via a phospholipase C (PLC) coupled to a G protein sensitive to pertussis toxin (63, 69). In addition, in cultured astrocytes, ODN increases the intracellular concentration of calcium from intracellular pools (68, 70). In the same cell type, ODN stimulates the formation of cAMP through activation of adenylyl cyclase (AC) activity (61, 71). The ability of ODN to stimulate phosphorylation of extracellular signal-regulated kinase 1 and 2 (ERK 1 and 2) is also found in both astrocytes and cerebellar granule neurons (63, 71). These data collectively indicate that ODN is the natural and specific ligand of a Gi/0 or GS protein-coupled receptor, which activates complementary transduction mechanisms depending on the cell type.
The structure–activity studies of ODN in relation to its ability to activate the metabotropic receptor expressed in rat astrocytes show that the C-terminal octapeptide of ODN (OP, ODN11−18) is the shortest biologically active fragment of the peptide (72). OP is able to mimic the effects of ODN on calcium mobilization and cAMP level increase observed in astrocytes (71, 72). Conversely, OP has no effect on the binding of [3H]flumazenil in cerebellar granular cells or of [3H]PK11195 in rat astrocytes (73). The effects of ODN on the activation of the cAMP/PKA, PIPs/calcium/PKC, and MAPK-ERK transduction pathways in astrocytes and neurons are completely suppressed by a specific antagonist of this receptor, the cyclo(1−8)[DLeu5]OP (63, 68, 72).
ODN, via activation of the central-type BZ receptors, stimulates the incorporation of [3H]thymidine into cultured rat astrocytes (74). Consistent with this proliferative effect of ODN, in vivo studies have demonstrated that ODN promotes the proliferation of neuronal progenitor stem cells from the germinative sub-ventricular zone in adult rat (75). Conversely, inhibition of DBI gene transcription by shRNA transfection in vivo reduces the number of proliferating cells and the number of neurons newly formed at the level of olfactory bulbs but does not affect stem cell survival (75). Furthermore, the fact that ODN can inhibit neuronal cell death and stimulate neurogenesis suggests that it could also play a key role during brain development. In support of this hypothesis, it has been shown that, in the cerebellar cortex, endozepines are exclusively expressed by Bergmann glia (2, 4), which control cerebellar granule neuron migration. Taken together, these data indicate that ODN may exert a neurotrophic effect and reinforce the notion that ODN may promote cell proliferation, survival, and/or differentiation.
ODN has been shown to rescue neurons and glial cells from neurotoxicity induced by several substances such as H2O2 (35, 71, 76, 77), 6-hydroxydopamine (6-OHDA) (62, 63), and 1-methyl-4-phenyl-1,2,3,6-tetrahydropyridine (MPTP) (35). Indeed, exposure of cultured granule neurons and N2a neuronal cell line to very low concentrations (in the subpicomolar range) of ODN totally abolishes the deleterious effects of 6-OHDA (63) and H2O2 (76– 78). ODN also exerts a strong protective effect against oxidative stress-induced apoptosis on cultured astrocytes (62, 78, 79). ODN acts by preventing (i) the accumulation and overproduction of intracellular reactive oxygen species (ROS), (ii) the depletion of glutathione (GSH) levels, and (iii) the decrease of the expression and activity of the antioxidant enzymes provoked by oxidative stress (79). Furthermore, ODN prevents apoptotic cell death by inhibiting (i) the overexpression of the proapoptotic protein Bax as well as the repression of the antiapoptotic protein Bcl-2 and (ii) the drop of the mitochondrial membrane potential responsible for the stimulation of caspase-3 activity. Treatment of neuroblastoma cell lines and cerebellar granule neurons with astrocyte-conditioned medium significantly promotes neuron survival under oxidative injury induced by 6-OHDA (80) and H2O2 (76, 81). Treatment of cerebellar granule neurons with ODN metabotropic receptor antagonist greatly attenuated the protective action of astrocyte-conditioned medium. Quantitative measurement of ODN by mass spectrometry indicates that the amount of ODN present in glial conditioned medium is in the same range of concentration as the one necessary for the neuroprotective action of the peptide on granular neurons against apoptotic cell death induced by oxidative damage, suggesting a neuroprotective effect of the endogenous gliopeptide (76).
Some behavioral studies have shown that in the same way as ODN, intracerebroventricular administration of TTN induces proconflict- and anxiety-related behavior. At the cellular level, TTN also promotes proliferation and intracellular calcium increase in glial cells (70, 74). However, the potential neuroprotective and/or neurotrophic activity of TTN has never been reported.
The neuroprotective activity of ODN has also been observed in vivo, in an MPTP mouse model of Parkinson's disease (35). A single intracerebroventricular injection of 10 ng ODN, 1 h after the last administration of MPTP, is sufficient to prevent the loss of dopaminergic neurons in the substantia nigra pars compacta (SNpc) induced by the toxin and to block the degeneration of nerve fibers in the striatum (35). ODN-mediated neuroprotection is associated with a reduction of the number of glial fibrillary acidic protein-positive reactive astrocytes and a strong inhibition of the expression of pro-inflammatory genes induced by MPTP in the SNpc. Moreover, ODN blocks the inhibition of the antiapoptotic gene Bcl-2 and the stimulation of the proapoptotic genes Bax and caspase-3 (35). ODN also prevents the accumulation of ROS and lipid oxidation products both in the SNpc and the striatum. Furthermore, DBI−/− mice exhibit more vulnerability to MPTP injection than wild-type animals (DBI+/+). Thus, ODN-knockout (KO) mice are more sensitive to MPTP-induced inflammatory and oxidative brain damages, suggesting that the endogenous ODN may also be neuroprotective (35). These data indicate that the induction of ODN production in pathological conditions, similar to the one observed at the early stages of neurodegenerative processes, may correspond to a compensatory mechanism, initiated by reactive astrocytes, to reduce their sensitivity to oxidative aggression and to limit the progression of brain damages.
Altogether, these results demonstrate that, based on its antioxidative (77, 82), anti-inflammatory (35), and antiapoptotic effects (63, 71), the gliopeptide ODN, which acts as a potent neuroprotective agent, could lead to the development of effective therapeutic agents for the treatment of cerebral injuries involving oxidative neurodegeneration. It should nevertheless be pointed out that in the acute phase of stroke, ODN, through its allosteric modulation of GABA A receptor, boosts the excitability of cortical neurons and as a consequence increases neuronal damages (83). Consistent with this observation, DBI−/− mice exhibit increased infarct volume compared to wild-type animals. However, when administered in the subacute period after stroke, ODN improves functional recovery, showing that if provided at the right time, ODN can also be of interest for the treatment of stroke patients.
There is evidence indicating that neuropeptides, such as PACAP (84, 85), brain-derived neurotrophic factor (BDNF), and nerve growth factor (NGF) (86), promote both neuronal survival and differentiation, suggesting that the gliopeptide ODN, which protects neurons and astrocytes from apoptosis (25, 80), could also have a differentiating activity. We have thus tested whether ODN can have a neurotrophic property on murine N2a cells and stimulate neurite (axons and dendrites) outgrowth, in indded, at very low concentrations (in the fentomolar range, 10−14 M), ODN exerts a protective action on H2O2-induced N2a cell apoptosis and induces cell differentiation in the presence or absence of fetal bovine serum (87) (Figure 3). The cytoprotective effects of ODN on N2a cells (capacity to prevent damages induced by oxidative stress) are observed at similar concentrations to those inducing neuronal differentiation (35, 63), which leads us to propose that ODN can be considered as a potent neurotrophic factor. It is noteworthy that no cytotoxic effect (inhibition of cell growth and cell adhesion, loss of transmembrane mitochondria potential, ROS overproduction, loss of lysosomal integrity, or cell death induction) is observed with ODN in the concentration range inducing neurite outgrowth (87). During neuronal differentiation, cells also acquire excitability and express genes with functional identity (88).
Figure 3. Schematic representation of transduction pathways involved in the neuroprotective effects of octadecaneuropeptide (ODN). ODN, through activation of its metabotropic receptor and the AC–protein kinase A (PKA), phospholipase C (PLC)–protein kinase C (PKC), and mitogen-activated protein (MAP)-kinases/extracellular signal-regulated kinase (ERK) signaling pathways, stimulates superoxide dismutase (SOD), and catalase (CAT) activities and glutathione (GSH) cellular contents, which prevent the drop of the mitochondrial membrane potential (Ψ) and activation of caspase-3 (Casp-3) induced by hydrogen peroxide (H2O2) or 6-hydroxydopamine (6-OHDA) in cultured neurons and astrocytes. ODN also abolished neurotoxin-induced overproduction of reactive oxygen species (ROS) and blocked oxidative damage of cell molecules, i.e., formation and accumulation of lipid oxidation products [malondialdehydes (MDAs)] and protein carbonyl compounds (PCs). Concomitantly, in cultured astrocytes, ODN stimulates Mn-SOD, CAT, glutathione peroxidase-1 (GPx), and sulfiredoxin-1 (Srxn) gene transcription and rescues 6-OHDA-associated reduced expression of endogenous antioxidant enzymes. In 1-methyl-4-phenyl-1,2,3,6-tetrahydropyridine (MPTP)-treated mice, ODN prevents the degeneration of dopaminergic neurons in the substantia nigra pars compacta, abrogates the effect of toxin on inhibition of tyrosine hydoxylase (TH) gene transcription, and blocks the stimulation of the expression of pro-inflammatory genes, such as interleukins (IL) 1 and 6, tumor necrosis factor-α (TNF-α), and the proapoptotic gene caspase-3. Taken together, these results show that the gliopeptide ODN exerts a potent neuroprotective effect through mechanisms inhibiting oxidative stress, neuroinflammation, and apoptosis.
To define the signaling pathway associated with the differentiating effect of ODN on N2a cells, inhibitors that are linked to the pathways involved in the cytoprotective action of ODN, such as the PKA inhibitor H89, the PLC inhibitor U73122, the PKC inhibitor chelerythrine, and the MEK inhibitor U0126, were used. All these molecules were able to block ODN differentiating activity (79, 87), indicating that ODN's cytoprotective and differentiating activities use common signaling pathways. The MEK–ERK pathway may be activated by both PKA and PKC (63, 71). Some other pathways such as the phosphoinositide 3-kinase (PI3K)/Akt involved in cell differentiation and activated by other neurotrophic factors could also contribute to ODN-induced N2a cell differentiation.
Since the growth of neurites (dendrites and/or neurons) requires significant energy and lipid synthesis, the effect of ODN on mitochondria and peroxisomes was investigated (89–91). In the presence of ODN, topographical changes, especially regarding mitochondria distribution in the N2a cells, are observed not only in the soma but also in neurites (dendrites and/or axons) (Figure 4). It is well-established that mitochondria are capable of producing fatty acids and cholesterol, which are essential for neurite outgrowth, and that peroxisome also contributes to cholesterol biogenesis via the production of acetyl-CoA (92, 93). Noteworthy, higher levels of cholesterol and its precursors (lanosterol, desmosterol, and lathosterol) are detected in cells treated with ODN (10−14 M) (87). It should be noted that there is a preference for the Bloch pathway for the cholesterol biogenesis as shown by the increased levels of lathosterol and desmosterol after treatment with ODN (87). Altogether, these data provide some of the mechanisms involved in the differentiation of the neuronal cell line N2a by ODN.
Figure 4. Induction of neuronal differentiation in octadecaneuropeptide (ODN)-treated N2a cells. Murine neuronal N2a cells were treated with ODN (at 10−14 M for 48 h). (A,B) Undifferentiated [control, (A)] and differentiated cells (characterized by morphological aspects based on neurite outgrowth, (B) cells were observed by phase contrast microscopy. Due to the presence of numerous mitochondria and peroxisomes in neurites, these later can be considered functional. (C,D) The mitochondria were detected with Mitotracker Red (red fluorescence) and the peroxisomes by indirect immunofluorescence after staining with an antibody raised against the ABCD3 peroxisomal transporter (green fluorescence) in control (C) and ODN-treated cells (D). The nuclei were counterstained with Hoechst 33342; the images were acquired under a fluorescent microscope coupled with an Apotome (Zeiss). The green spots point toward peroxisomes, and red spots point toward mitochondria. (E–H) Visualization by transmission electron microscopy of mitochondria (red arrows) and peroxisomes (yellow arrows) in control (E,G) and differentiated N2a cells [treated with ODN, (F,H)].
Pharmacological studies have shown that central BZ receptor agonists are unable to mimic the protective effects of ODN (25). Similarly, central-type BZ receptors and translocator protein (TSPO) antagonists fail to suppress ODN-induced protection of neurons and glial cells from injuries, indicating that “classical” BZ receptors are not involved in the protective activity of ODN. However, OP and cyclo(1−8)OP, two agonists of the ODN metabotropic receptor, exhibit a protective activity when used in the same range of concentration as ODN. In addition, cyclo(1−8)[DLeu5]OP, a potent antagonist of this metabotropic receptor, blocks the protective effects of ODN on neurons and astrocytes (62, 63, 71, 82).
Downstream of the receptor, several transduction pathways can mediate the protective effect of ODN according to the cell type. In astrocytes, activation of ODN metabotropic receptor implies stimulation of the activity of the AC/cAMP/PKA pathway (71). The neuroprotective effect of ODN against 6-OHDA-induced cerebellar granule cell death involves activation of the PLC/IP3/PKC pathway (63). Downstream to PKA and PKC activations, ODN stimulates the phosphorylation of the ERK1 and ERK2 proteins in astrocytes and neurons (79). The phosphorylation of ERK1 and ERK2 in turn stimulates the expression of the Bcl-2 protein and concomitantly inhibits the expression of Bax, thereby preventing mitochondrial dysfunction and blocking cell death via the intrinsic apoptotic pathway (84, 94). The stimulating effect of ODN on the expression of antioxidant enzyme genes and their enzymatic activities is dependent on the activity of the cAMP/PKA transduction pathway as well as that of MAPK–ERKs in astrocytes (62, 77, 82). ODN promotes cerebellar granule cell survival from apoptosis through the PKC pathway (63), while another neuropeptide, PACAP, protects the same neurons from apoptosis through the PKA pathway (95). It would thus now be of interest to investigate if combining the two molecules leads to an enhanced and/or prolonged antiapoptotic effect.
Clinical studies have revealed that endozepine levels are significantly increased in the CSF of patients suffering from neurological disorders such as epilepsy, hepatic encephalopathy (96, 97), or Alzheimer's and Parkinson's diseases (98). Mass spectrometry, radioimmunoassay (RIA), and q-RT PCR analyses reveal that moderate oxidative stress induces stimulation of the endogenous production of ODN, as well as the expression of the ODN precursor gene DBI from cultured astrocytes (76). Furthermore, induction of endogenous ODN production is responsible for (i) the stimulation of the expression and activity of antioxidant enzymes and thus rapid resorption of ROS, (ii) the protection of bio-macromolecules from oxidative damages, and (iii) the prevention of astrocytes and neuron cell death induced by oxidative stress. In contrast, inhibition of ODN release by the PKA inhibitor H89 or blockage of the effects of ODN with an antagonist of its metabotropic receptor, the cyclo(1−8)[DLeu5]OP, exacerbates damages induced by oxidative stress on astroglial cell viability (76). Consistently, knockdown of ODN precursor expression, by DBI siRNA, induces morphological alterations with loss of membrane integrity and the formation of apoptotic bodies and increases the vulnerability of oxidative stress inducing cell death (76). It has also been shown, by using DBI-KO animals, that ODN precursor deficiency increases brain sensitivity to MPTP toxicity, highlighting the neuroprotective role of endogenous ODN against neuronal degeneration (35). Taken as a whole, these data indicate that the induction of ODN production in pathological conditions reproducing early stages of neurodegenerative processes may represent a compensatory mechanism, initiated by reactive astrocytes, to reduce their sensitivity, as well as that of their surrounding neurons, to oxidative aggression and to limit the progression of the neurodegeneration process in some neurological disorders.
OM-K, AN, GL, JL, M-CT, and DV provided valuable editorial comments and relevant bibliographic references. YH, SB, IG, and DV provided valuable editorial comments. OM-K wrote the manuscript with the contribution of AN, GL, and DV. TG, JC, and BL contributed to the review and editing revised version. All authors contributed to the article and approved the submitted version.
This work was supported by a France–Tunisia CMCU-Campus France/PHC Utique 20G0826/44306YD exchange program (to OM-K and DV), LR18ES03, EA7270, and INSERM (U1239). Funders had no role in the study design, data collection and analysis, decision to publish, or preparation of the manuscript.
The authors declare that the research was conducted in the absence of any commercial or financial relationships that could be construed as a potential conflict of interest.
1. Guidotti A, Forchetti CM, Corda MG, Konkel D, Bennett CD, Costa E. Isolation, characterization, and purification to homogeneity of an endogenous polypeptide with agonistic action on benzodiazepine receptors. Proc Natl Acad Sci USA. (1983) 80:3531–5. doi: 10.1073/pnas.80.11.3531
2. Tonon MC, Leprince J, Gandolfo P, Compère V, Pelletier G, Malagon MM, et al. Endozepines. In Kastin AJ, editor. Handbook of Biologically Active Peptides. New York, NY: Elsevier (2006). p. 813–9. doi: 10.1016/B978-012369442-3/50114-8
3. Knudsen J, Mandrup S, Rasmussen JT, Andreasen PH, Poulsen F, Kristiansen K. The function of acyl-CoA-binding protein (ACBP)/diazepam binding inhibitor (DBI). Mol Cell Biochem. (1993) 123:129–38. doi: 10.1007/978-1-4615-3096-1_17
4. Podkletnova I, Rothstein JD, Helen P, Alho H. Microglial response to the neurotoxicity of 6-hydroxydopamine in neonatal rat cerebellum. Int J Dev Neurosci. (2001) 19:47–52. doi: 10.1016/S0736-5748(00)00069-1
5. Mocchetti I, Einstein R, Brosius J. Putative diazepam binding inhibitor peptide: cDNA clones from rat. Proc Natl Acad Sci USA. (1986) 83:7221–5. doi: 10.1073/pnas.83.19.7221
6. Gray PW, Glaister D, Seeburg PH, Guidotti A, Costa E. Cloning and expression of cDNA for human diazepam binding inhibitor, a natural ligand of an allosteric regulatory site of the gamma-aminobutyric acid type A receptor. Proc Natl Acad Sci USA. (1986) 83:7547–51. doi: 10.1073/pnas.83.19.7547
7. Mogensen IB, Schulenberg H, Hansen HO, Spener F, Knudsen J. A novel acyl-CoA-binding protein from bovine liver. Effect on fatty acid synthesis. Biochem J. (1987) 241:189–92. doi: 10.1042/bj2410189
8. Lihrmann I, Plaquevent JC, Tostivint H, Raijmakers R, Tonon MC, Conlon JM, et al. Frog diazepam-binding inhibitor: peptide sequence, cDNA cloning, and expression in the brain. Proc Natl Acad Sci USA. (1994) 91:6899–903. doi: 10.1073/pnas.91.15.6899
9. Chang JL, Tsai HJ. Carp cDNA sequence encoding a putative diazepam-binding inhibitor/endozepine/acyl-CoA-binding protein. Biochim Biophys Acta. (1996) 1298:9–11. doi: 10.1016/S0167-4838(96)00164-1
10. Kolmer M, Roos C, Tirronen M, Myohanen S, Alho H. Tissue-specific expression of the diazepam-binding inhibitor in Drosophila melanogaster: cloning, structure, and localization of the gene. Mol Cell Biol. (1994) 14:6983–95. doi: 10.1128/MCB.14.10.6983
11. Matsumoto S, Yoshiga T, Yokoyama N, Iwanaga M, Koshiba S, Kigawa T, et al. Characterization of acyl-CoA-binding protein (ACBP) in the pheromone gland of the silkworm, Bombyx mori. Insect Biochem Mol Biol. (2001) 31:603–9. doi: 10.1016/S0965-1748(00)00165-X
12. Snyder MJ, Feyereisen R. A diazepam binding inhibitor (DBI) homolog from the tobacco hornworm, Manduca sexta. Mol Cell Endocrinol. (1993) 94:R1–4. doi: 10.1016/0303-7207(93)90064-Q
13. Guerrero C, Martin-Rufian M, Reina JJ, Heredia A. Isolation and characterization of a cDNA encoding a membrane bound acyl-CoA binding protein from agave Americana L. epidermis. Plant Physiol Biochem. (2006) 44:85–90. doi: 10.1016/j.plaphy.2006.01.002
14. Rose TM, Schultz ER, Todaro GJ. Molecular cloning of the gene for the yeast homolog (ACB) of diazepam binding inhibitor/endozepine/acyl-CoA-binding protein. Proc Natl Acad Sci USA. (1992) 89:11287–91. doi: 10.1073/pnas.89.23.11287
15. Burton M, Rose TM, Faergeman NJ, Knudsen J. Evolution of the acyl-CoA binding protein (ACBP). Biochem J. (2005) 392:299–307. doi: 10.1042/BJ20050664
16. Faergeman NJ, Wadum M, Feddersen S, Burton M, Kragelund BB, Knudsen J. Acyl-CoA binding proteins; structural and functional conservation over 2000 MYA. Mol Cell Biochem. (2007) 299:55–65. doi: 10.1007/s11010-005-9040-3
17. Owens GP, Sinha AK, Sikela JM, Hahn WE. Sequence and expression of the murine diazepam binding inhibitor. Brain Res Mol Brain Res. (1989) 6:101–8. doi: 10.1016/0169-328X(89)90043-0
18. DeBernardi MA, Crowe RR, Mocchetti I, Shows TB, Eddy RL, Costa E. Chromosomal localization of the human diazepam binding inhibitor gene. Proc Natl Acad Sci USA. (1988) 85:6561–5. doi: 10.1073/pnas.85.17.6561
19. Gersuk VH, Rose TM, Todaro GJ. Molecular cloning and chromosomal localization of a pseudogene related to the human acyl-CoA binding protein/diazepam binding inhibitor. Genomics. (1995) 25:469–76. doi: 10.1016/0888-7543(95)80047-P
20. Mandrup S, Hummel R, Ravn S, Jensen G, Andreasen PH, Gregersen N, et al. Acyl-CoA-binding protein/diazepam-binding inhibitor gene and pseudogenes. A typical housekeeping gene family. J Mol Biol. (1992) 228:1011–22. doi: 10.1016/0022-2836(92)90888-Q
21. Swinnen JV, Esquenet M, Rosseels J, Claessens F, Rombauts W, Heyns W, et al. A human gene encoding diazepam-binding inhibitor/acy1-CoA-binding protein: transcription and hormonal regulation in the androgen-sensitive human prostatic adenocarcinoma cell line LNCaP. DNA Cell Biol. (1996) 15:197–208. doi: 10.1089/dna.1996.15.197
22. Nitz I, Doring F, Schrezenmeir J, Burwinkel B. Identification of new acyl-CoA binding protein transcripts in human and mouse. Int J Biochem Cell Biol. (2005) 37:2395–405. doi: 10.1016/j.biocel.2005.06.008
23. Nitz I, Kruse ML, Klapper M, Doring F. Specific regulation of low-abundance transcript variants encoding human Acyl-CoA binding protein (ACBP) isoforms. J Cell Mol Med. (2011) 15:909–27. doi: 10.1111/j.1582-4934.2010.01055.x
24. Helledie T, Grontved L, Jensen SS, Kiilerich P, Rietveld L, Albrektsen T, et al. The gene encoding the Acyl-CoA-binding protein is activated by peroxisome proliferator-activated receptor gamma through an intronic response element functionally conserved between humans and rodents. J Biol Chem. (2002) 277:26821–30. doi: 10.1074/jbc.M111295200
25. Tonon MC, Vaudry H, Chuquet J, Guillebaud F, Fan J, Masmoudi-Kouki O, et al. Endozepines and their receptors: structure, functions and pathophysiological significance. Pharmacol Ther. (2020) 208:107386. doi: 10.1016/j.pharmthera.2019.06.008
26. Hansen HO, Andreasen PH, Mandrup S, Kristiansen K, Knudsen J. Induction of acyl-CoA-binding protein and its mRNA in 3T3-L1 cells by insulin during preadipocyte-to-adipocyte differentiation. Biochem J. (1991) 277:341–4. doi: 10.1042/bj2770341
27. Rosen MB, Wilson VS, Schmid JE, Gray LE. Gene expression analysis in the ventral prostate of rats exposed to vinclozolin or procymidone. Reprod Toxicol. (2005) 19:367–79. doi: 10.1016/j.reprotox.2004.10.005
28. Sandberg MB, Bloksgaard M, Duran-Sandoval D, Duval C, Staels B, Mandrup S. The gene encoding acyl-CoA-binding protein is subject to metabolic regulation by both sterol regulatory element-binding protein and peroxisome proliferator-activated receptor alpha in hepatocytes. J Biol Chem. (2005) 280:5258–66. doi: 10.1074/jbc.M407515200
29. Bhuiyan J, Pritchard PH, Pande SV, Seccombe DW. Effects of high-fat diet and fasting on levels of acyl-coenzyme A binding protein in liver, kidney, and heart of rat. Metabolism. (1995) 44:1185–9. doi: 10.1016/0026-0495(95)90013-6
30. Ettinger SL, Sobel R, Whitmore TG, Akbari M, Bradley DR, Gleave ME, et al. Dysregulation of sterol response element-binding proteins and downstream effectors in prostate cancer during progression to androgen independence. Cancer Res. (2004) 64:2212–21. doi: 10.1158/0008-5472.CAN-2148-2
31. Barmack NH, Bilderback TR, Liu H, Qian Z, Yakhnitsa V. Activity-dependent expression of acyl-coenzyme a-binding protein in retinal muller glial cells evoked by optokinetic stimulation. J Neurosci. (2004) 24:1023–33. doi: 10.1523/JNEUROSCI.3936-03.2004
32. Katsura M, Ohkuma S. Functional proteins involved in regulation of intracellular Ca(2+) for drug development: chronic nicotine treatment upregulates L-type high voltage-gated calcium channels. J Pharmacol Sci. (2005) 97:344–7. doi: 10.1254/jphs.FMJ04007X3
33. Katsura M, Shuto K, Mohri Y, Tsujimura A, Shibata D, Tachi M, et al. Continuous exposure to nitric oxide enhances diazepam binding inhibitor mRNA expression in mouse cerebral cortical neurons. Brain Res Mol Brain Res. (2004) 124:29–39. doi: 10.1016/j.molbrainres.2004.02.008
34. Tokay T, Masmoudi O, Gandolfo P, Leprince J, Pelletier G, Vaudry H, et al. Beta-amyloid peptides stimulate endozepine biosynthesis in cultured rat astrocytes. J Neurochem. (2005) 94:607–16. doi: 10.1111/j.1471-4159.2005.03102.x
35. Bahdoudi S, Ghouili I, Hmiden M, do Rego JL, Lefranc B, Leprince J, et al. Neuroprotective effects of the gliopeptide ODN in an in vivo model of Parkinson's disease. Cell Mol Life Sci. (2018) 75:2075–91. doi: 10.1007/s00018-017-2727-2
36. Tonon MC, Leprince J, Morin F, Gandolfo P, Compère V, Pelletier G, et al. Endozepines. In: Kastin AJ, editor. Handbook of Biologically Active Peptides. Oxford, UK: Elsevier (2013). p. 760–5. doi: 10.1016/B978-0-12-385095-9.00102-0
37. Alho H, Costa E, Ferrero P, Fujimoto M, Cosenza-Murphy D, Guidotti A. Diazepam-binding inhibitor: a neuropeptide located in selected neuronal populations of rat brain. Science. (1985) 229:179–82. doi: 10.1126/science.3892688
38. Christian CA, Herbert AG, Holt RL, Peng K, Sherwood KD, Pangratz-Fuehrer S, et al. Endogenous positive allosteric modulation of GABA(A) receptors by diazepam binding inhibitor. Neuron. (2013) 78:1063–74. doi: 10.1016/j.neuron.2013.04.026
39. Tonon MC, Desy L, Nicolas P, Vaudry H, Pelletier G. Immunocytochemical localization of the endogenous benzodiazepine ligand octadecaneuropeptide (ODN) in the rat brain. Neuropeptides. (1990) 15:17–24. doi: 10.1016/0143-4179(90)90155-R
40. Yanase H, Shimizu H, Yamada K, Iwanaga T. Cellular localization of the diazepam binding inhibitor in glial cells with special reference to its coexistence with brain-type fatty acid binding protein. Arch Histol Cytol. (2002) 65:27–36. doi: 10.1679/aohc.65.27
41. Lanfray D, Arthaud S, Ouellet J, Compere V, Do Rego JL, Leprince J, et al. Gliotransmission and brain glucose sensing: critical role of endozepines. Diabetes. (2013) 62:801–10. doi: 10.2337/db11-0785
42. Malagon M, Vaudry H, Van Strien F, Pelletier G, Gracia-Navarro F, Tonon MC. Ontogeny of diazepam-binding inhibitor-related peptides (endozepines) in the rat brain. Neuroscience. (1993) 57:777–86. doi: 10.1016/0306-4522(93)90023-9
43. Young JK. Immunoreactivity for diazepam binding inhibitor in gomori-positive astrocytes. Regul Pept. (1994) 50:159–65. doi: 10.1016/0167-0115(94)90031-0
44. Lacor P, Gandolfo P, Tonon MC, Brault E, Dalibert I, Schumacher M, et al. Regulation of the expression of peripheral benzodiazepine receptors and their endogenous ligands during rat sciatic nerve degeneration and regeneration: a role for PBR in neurosteroidogenesis. Brain Res. (1999) 815:70–80. doi: 10.1016/S0006-8993(98)01105-6
45. Roesch K, Jadhav AP, Trimarchi JM, Stadler MB, Roska B, Sun BB, et al. The transcriptome of retinal Muller glial cells. J Comp Neurol. (2008) 509:225–38. doi: 10.1002/cne.21730
46. Lafon-Cazal M, Adjali O, Galeotti N, Poncet J, Jouin P, Homburger V, et al. Proteomic analysis of astrocytic secretion in the mouse. Comparison with the cerebrospinal fluid proteome. J Biol Chem. (2003) 278:24438–48. doi: 10.1074/jbc.M211980200
47. Galluzzi L, Pietrocola F, Levine B, Kroemer G. Metabolic control of autophagy. Cell. (2014) 159:1263–76. doi: 10.1016/j.cell.2014.11.006
48. Hamon Y, Luciani MF, Becq F, Verrier B, Rubartelli A, Chimini G. Interleukin-1beta secretion is impaired by inhibitors of the Atp binding cassette transporter, ABC1. Blood. (1997) 90:2911–5. doi: 10.1182/blood.V90.8.2911
49. Omer S, Meredith D, Morris JF, Christian HC. Evidence for the role of adenosine 5'-triphosphate-binding cassette (ABC)-A1 in the externalization of annexin 1 from pituitary folliculostellate cells and ABCA1-transfected cell models. Endocrinology. (2006) 147:3219–27. doi: 10.1210/en.2006-0099
50. Cabral M, Anjard C, Loomis WF, Kuspa A. Genetic evidence that the acyl coenzyme A binding protein AcbA and the serine protease/ABC transporter TagA function together in Dictyostelium discoideum cell differentiation. Eukaryot Cell. (2006) 5:2024–32. doi: 10.1128/EC.00287-05
51. Clavier T, Tonon MC, Foutel A, Besnier E, Lefevre-Scelles A, Morin F, et al. Increased plasma levels of endozepines, endogenous ligands of benzodiazepine receptors, during systemic inflammation: a prospective observational study. Crit Care. (2014) 18:633. doi: 10.1186/s13054-014-0633-7
52. Masmoudi O, Gandolfo P, Leprince J, Vaudry D, Fournier A, Patte-Mensah C, et al. Pituitary adenylate cyclase-activating polypeptide (PACAP) stimulates endozepine release from cultured rat astrocytes via a PKA-dependent mechanism. FASEB J. (2003) 17:17–27. doi: 10.1096/fj.02-0317com
53. Jarry M, Diallo M, Lecointre C, Desrues L, Tokay T, Chatenet D, et al. The vasoactive peptides urotensin II and urotensin II-related peptide regulate astrocyte activity through common and distinct mechanisms: involvement in cell proliferation. Biochem J. (2010) 428:113–24. doi: 10.1042/BJ20090867
54. Tokay T, Hachem R, Masmoudi-Kouki O, Gandolfo P, Desrues L, Leprince J, et al. Beta-amyloid peptide stimulates endozepine release in cultured rat astrocytes through activation of N-formyl peptide receptors. Glia. (2008) 56:1380–9. doi: 10.1002/glia.20705
55. Loomis WF, Behrens MM, Williams ME, Anjard C. Pregnenolone sulfate and cortisol induce secretion of acyl-CoA-binding protein and its conversion into endozepines from astrocytes. J Biol Chem. (2010) 285:21359–65. doi: 10.1074/jbc.M110.105858
56. Clavier T, Besnier E, Lefevre-Scelles A, Lanfray D, Masmoudi O, Pelletier G, et al. Increased hypothalamic levels of endozepines, endogenous ligands of benzodiazepine receptors, in a rat model of sepsis. Shock. (2016) 45:653–9. doi: 10.1097/SHK.0000000000000560
57. Farzampour Z, Reimer RJ, Huguenard J. Endozepines. Adv Pharmacol. (2015) 72:147–64. doi: 10.1016/bs.apha.2014.10.005
58. Masmoudi O, Gandolfo P, Tokay T, Leprince J, Ravni A, Vaudry H, et al. Somatostatin down-regulates the expression and release of endozepines from cultured rat astrocytes via distinct receptor subtypes. J Neurochem. (2005) 94:561–71. doi: 10.1111/j.1471-4159.2005.03076.x
59. Patte C, Gandolfo P, Leprince J, Thoumas JL, Fontaine M, Vaudry H, et al. GABA inhibits endozepine release from cultured rat astrocytes. Glia. (1999) 25:404–11. doi: 10.1002/(SICI)1098-1136(19990215)25:4<404::AID-GLIA9>3.0.CO;2-Q
60. Qian Z, Bilderback TR, Barmack NH. Acyl coenzyme A-binding protein (ACBP) is phosphorylated and secreted by retinal Muller astrocytes following protein kinase C activation. J Neurochem. (2008) 105:1287–99. doi: 10.1111/j.1471-4159.2008.05229.x
61. Gach K, Belkacemi O, Lefranc B, Perlikowski P, Masson J, Walet-Balieu ML, et al. Detection, characterization and biological activities of [bisphospho-Thr3,9]ODN, an endogenous molecular form of ODN released by astrocytes. Neuroscience. (2015) 290:472–84. doi: 10.1016/j.neuroscience.2015.01.045
62. Kaddour H, Hamdi Y, Amri F, Bahdoudi S, Bouannee I, Leprince J, et al. Antioxidant and anti-apoptotic activity of octadecaneuropeptide against 6-OHDA toxicity in cultured rat astrocytes. J Mol Neurosci. (2019) 69:1–16. doi: 10.1007/s12031-018-1181-4
63. Kaddour H, Hamdi Y, Vaudry D, Basille M, Desrues L, Leprince J, et al. The octadecaneuropeptide ODN prevents 6-hydroxydopamine-induced apoptosis of cerebellar granule neurons through a PKC-MAPK-dependent pathway. J Neurochem. (2013) 125:620–33. doi: 10.1111/jnc.12140
64. Costa E, Corda MG, Guidotti A. On a brain polypeptide functioning as a putative effector for the recognition sites of benzodiazepine and beta-carboline derivatives. Neuropharmacology. (1983) 22:1481–92. doi: 10.1016/0028-3908(83)90116-8
65. Ferrero P, Conti-Tronconi B, Guidotti A. DBI, an anxiogenic neuropeptide found in human brain. Adv Biochem Psychopharmacol. (1986) 41:177–85.
66. Bormann J. Electrophysiological characterization of diazepam binding inhibitor (DBI) on GABAA receptors. Neuropharmacology. (1991) 30:1387–9. doi: 10.1016/S0028-3908(11)80006-7
67. Cosentino M, Marino F, Cattaneo S, Di Grazia L, Francioli C, Fietta AM, et al. Diazepam-binding inhibitor-derived peptides induce intracellular calcium changes and modulate human neutrophil function. J Leukoc Biol. (2000) 67:637–43. doi: 10.1002/jlb.67.5.637
68. Leprince J, Oulyadi H, Vaudry D, Masmoudi O, Gandolfo P, Patte C, et al. Synthesis, conformational analysis and biological activity of cyclic analogs of the octadecaneuropeptide ODN. Design of a potent endozepine antagonist. Eur J Biochem. (2001) 268:6045–57. doi: 10.1046/j.0014-2956.2001.02533.x
69. Patte C, Vaudry H, Desrues L, Gandolfo P, Strijdveen I, Lamacz M, et al. The endozepine ODN stimulates polyphosphoinositide metabolism in rat astrocytes. FEBS Lett. (1995) 362:106–10. doi: 10.1016/0014-5793(95)00209-R
70. Gandolfo P, Patte C, Leprince J, Thoumas JL, Vaudry H, Tonon MC. The stimulatory effect of the octadecaneuropeptide (ODN) on cytosolic Ca2+ in rat astrocytes is not mediated through classical benzodiazepine receptors. Eur J Pharmacol. (1997) 322:275–81. doi: 10.1016/S0014-2999(97)00012-5
71. Hamdi Y, Kaddour H, Vaudry D, Bahdoudi S, Douiri S, Leprince J, et al. The octadecaneuropeptide ODN protects astrocytes against hydrogen peroxide-induced apoptosis via a PKA/MAPK-dependent mechanism. PLoS ONE. (2012) 7:e42498. doi: 10.1371/journal.pone.0042498
72. Leprince J, Gandolfo P, Thoumas JL, Patte C, Fauchere JL, Vaudry H, et al. Structure-activity relationships of a series of analogues of the octadecaneuropeptide ODN on calcium mobilization in rat astrocytes. J Med Chem. (1998) 41:4433–8. doi: 10.1021/jm980275d
73. Alho H, Bovolin P, Jenkins D, Guidotti A, Costa E. Cellular and subcellular localization of an octadecaneuropeptide derived from diazepam binding inhibitor: immunohistochemical studies in the rat brain. J Chem Neuroanat. (1989) 2:301–18. doi: 10.1007/BF00972211
74. Gandolfo P, Patte C, Thoumas JL, Leprince J, Vaudry H, Tonon MC. The endozepine ODN stimulates [3H]thymidine incorporation in cultured rat astrocytes. Neuropharmacology. (1999) 38:725–32. doi: 10.1016/S0028-3908(98)00231-7
75. Alfonso J, Le Magueresse C, Zuccotti A, Khodosevich K, Monyer H. Diazepam binding inhibitor promotes progenitor proliferation in the postnatal SVZ by reducing GABA signaling. Cell Stem Cell. (2012) 10:76–87. doi: 10.1016/j.stem.2011.11.011
76. Ghouili I, Bahdoudi S, Morin F, Amri F, Hamdi Y, Coly PM, et al. Endogenous expression of ODN-related peptides in astrocytes contributes to cell protection against oxidative stress: astrocyte-neuron crosstalk relevance for neuronal survival. Mol Neurobiol. (2018) 55:4596–611. doi: 10.1007/s12035-017-0630-3
77. Hamdi Y, Kaddour H, Vaudry D, Douiri S, Bahdoudi S, Leprince J, et al. The stimulatory effect of the octadecaneuropeptide ODN on astroglial antioxidant enzyme systems is mediated through a GPCR. Front Endocrinol. (2012) 3:138. doi: 10.3389/fendo.2012.00138
78. Namsi A, Nury T, Hamdouni H, Yammine A, Vejux A, Vervandier-Fasseur D, et al. Induction of neuronal differentiation of murine N2a cells by two polyphenols present in the mediterranean diet mimicking neurotrophins activities: resveratrol and apigenin. Diseases. (2018) 6:67. doi: 10.3390/diseases6030067
79. Masmoudi-Kouki O, Hamdi Y, Ghouili I, Bahdoudi S, Kaddour H, Leprince J, et al. Neuroprotection with the endozepine octadecaneuropeptide, ODN. Curr Pharm Des. (2018) 24:3918–25. doi: 10.2174/1381612824666181112111746
80. Murakami S, Miyazaki I, Asanuma M. Neuroprotective effect of fermented papaya preparation by activation of Nrf2 pathway in astrocytes. Nutr Neurosci. (2018) 21:176–84. doi: 10.1080/1028415X.2016.1253171
81. Douiri S, Bahdoudi S, Hamdi Y, Cubi R, Basille M, Fournier A, et al. Involvement of endogenous antioxidant systems in the protective activity of pituitary adenylate cyclase-activating polypeptide against hydrogen peroxide-induced oxidative damages in cultured rat astrocytes. J Neurochem. (2016) 137:913–30. doi: 10.1111/jnc.13614
82. Hamdi Y, Kaddour H, Vaudry D, Leprince J, Zarrouk A, Hammami M, et al. Octadecaneuropeptide ODN prevents hydrogen peroxide-induced oxidative damage of biomolecules in cultured rat astrocytes. Peptides. (2015) 71:56–65. doi: 10.1016/j.peptides.2015.06.010
83. Lamtahri R, Hazime M, Gowing EK, Nagaraja RY, Maucotel J, Quilichini P, et al. The gliopeptide ODN, a ligand for the benzodiazepine site of GABAA receptors, boosts functional recovery after stroke. bioRxiv [Preprint]. (2020). doi: 10.1101/2020.03.05.977934
84. Bonaventura G, Iemmolo R, D'Amico AG, La Cognata V, Costanzo E, Zappia M, et al. PACAP and PAC1R are differentially expressed in motor cortex of amyotrophic lateral sclerosis patients and support survival of iPSC-derived motor neurons. J Cell Physiol. (2018) 233:3343–51. doi: 10.1002/jcp.26182
85. Seaborn T, Masmoudi-Kouli O, Fournier A, Vaudry H, Vaudry D. Protective effects of pituitary adenylate cyclase-activating polypeptide (PACAP) against apoptosis. Curr Pharm Des. (2011) 17:204–14. doi: 10.2174/138161211795049679
86. Sampaio TB, Savall AS, Gutierrez MEZ, Pinton S. Neurotrophic factors in Alzheimer's and Parkinson's diseases: implications for pathogenesis and therapy. Neural Regen Res. (2017) 12:549–57. doi: 10.4103/1673-5374.205084
87. Namsi A, Nury T, Khan AS, Leprince J, Vaudry D, Caccia C, et al. Octadecaneuropeptide (ODN) induces N2a cells differentiation through a PKA/PLC/PKC/MEK/ERK-dependent pathway: incidence on peroxisome, mitochondria, and lipid profiles. Molecules. (2019) 24:E3310. doi: 10.3390/molecules24183310
88. Ravni A, Vaudry D, Gerdin MJ, Eiden MV, Falluel-Morel A, Gonzalez BJ, et al. A cAMP-dependent, protein kinase A-independent signaling pathway mediating neuritogenesis through Egr1 in PC12 cells. Mol Pharmacol. (2008) 73:1688–708. doi: 10.1124/mol.107.044792
89. Noh KT, Son KH, Jung ID, Kang HK, Hwang SA, Lee WS, et al. Protein kinase C delta (PKC delta)-extracellular signal-regulated kinase 1/2 (ERK1/2) signaling cascade regulates glycogen synthase kinase-3 (GSK-3) inhibition-mediated interleukin-10 (IL-10) expression in lipopolysaccharide (LPS)-induced endotoxemia. J Biol Chem. (2012) 287:14226–33. doi: 10.1074/jbc.M111.308841
90. Tsui-Pierchala BA, Encinas M, Milbrandt J, Johnson EM Jr. Lipid rafts in neuronal signaling and function. Trends Neurosci. (2002) 25:412–7. doi: 10.1016/S0166-2236(02)02215-4
91. Knobloch M. The role of lipid metabolism for neural stem cell regulation. Brain Plast. (2017) 3:61–71. doi: 10.3233/BPL-160035
92. Knobloch M, Pilz GA, Ghesquiere B, Kovacs WJ, Wegleiter T, Moore DL, et al. A fatty acid oxidation-dependent metabolic shift regulates adult neural stem cell activity. Cell Rep. (2017) 20:2144–55. doi: 10.1016/j.celrep.2017.08.029
93. Zarrouk A, Nury T, Karym EM, Vejux A, Sghaier R, Gondcaille C, et al. Attenuation of 7-ketocholesterol-induced overproduction of reactive oxygen species, apoptosis, and autophagy by dimethyl fumarate on 158N murine oligodendrocytes. J Steroid Biochem Mol Biol. (2017) 169:29–38. doi: 10.1016/j.jsbmb.2016.02.024
94. Amri F, Ghouili I, Tonon MC, Amri M, Masmoudi-Kouki O. Hemoglobin-improved protection in cultured cerebral corticalastroglial Ccells: inhibition of oxidative stress and caspase activation. Front Endocrinol (Lausanne). (2017) 8:67. doi: 10.3389/fendo.2017.00067
95. Vaudry D, Gonzalez BJ, Basille M, Anouar Y, Fournier A, Vaudry H. Pituitary adenylate cyclase-activating polypeptide stimulates both c-fos gene expression and cell survival in rat cerebellar granule neurons through activation of the protein kinase A pathway. Neuroscience. (1998) 84:801–12. doi: 10.1016/S0306-4522(97)00545-9
96. Barbaccia ML, Costa E, Ferrero P, Guidotti A, Roy A, Sunderland T, et al. Diazepam-binding inhibitor. A brain neuropeptide present in human spinal fluid: studies in depression, schizophrenia, and Alzheimer's disease. Arch Gen Psychiatry. (1986) 43:1143–7. doi: 10.1001/archpsyc.1986.01800120029007
97. Rothstein JD, McKhann G, Guarneri P, Barbaccia ML, Guidotti A, Costa E. Cerebrospinal fluid content of diazepam binding inhibitor in chronic hepatic encephalopathy. Ann Neurol. (1989) 26:57–62. doi: 10.1002/ana.410260109
Keywords: gliopeptide ODN, neurodegeneration, cell protection, cell differentiation, oxidative stress
Citation: Masmoudi-Kouki O, Namsi A, Hamdi Y, Bahdoudi S, Ghouili I, Chuquet J, Leprince J, Lefranc B, Ghrairi T, Tonon M-C, Lizard G and Vaudry D (2020) Cytoprotective and Neurotrophic Effects of Octadecaneuropeptide (ODN) in in vitro and in vivo Models of Neurodegenerative Diseases. Front. Endocrinol. 11:566026. doi: 10.3389/fendo.2020.566026
Received: 26 May 2020; Accepted: 28 August 2020;
Published: 04 November 2020.
Edited by:
Jacques Epelbaum, Institut National de la Santé et de la Recherche Médicale (INSERM), FranceReviewed by:
Arturo Ortega, Center for Research and Advanced Studies, National Polytechnic Institute of Mexico (CINVESTAV), MexicoCopyright © 2020 Masmoudi-Kouki, Namsi, Hamdi, Bahdoudi, Ghouili, Chuquet, Leprince, Lefranc, Ghrairi, Tonon, Lizard and Vaudry. This is an open-access article distributed under the terms of the Creative Commons Attribution License (CC BY). The use, distribution or reproduction in other forums is permitted, provided the original author(s) and the copyright owner(s) are credited and that the original publication in this journal is cited, in accordance with accepted academic practice. No use, distribution or reproduction is permitted which does not comply with these terms.
*Correspondence: Olfa Masmoudi-Kouki, T2xmYS5tYXNtb3VkaUBmc3QudXRtLnRu
Disclaimer: All claims expressed in this article are solely those of the authors and do not necessarily represent those of their affiliated organizations, or those of the publisher, the editors and the reviewers. Any product that may be evaluated in this article or claim that may be made by its manufacturer is not guaranteed or endorsed by the publisher.
Research integrity at Frontiers
Learn more about the work of our research integrity team to safeguard the quality of each article we publish.