- Laboratory of Immunology and Inflammation, Department of Cell Biology, University of Brasilia, Brasilia, Brazil
Obesity is a multifactorial and complex condition that is characterized by abnormal and excessive white adipose tissue accumulation, which can lead to the development of metabolic diseases, such as type 2 diabetes mellitus, nonalcoholic fatty liver disease, cardiovascular diseases, and several types of cancer. Obesity is characterized by excessive adipose tissue accumulation and associated with alterations in immunity, displaying a chronic low-grade inflammation profile. Adipose tissue is a dynamic and complex endocrine organ composed not only by adipocytes, but several immunological cells, which can secrete hormones, cytokines and many other factors capable of regulating metabolic homeostasis and several critical biological pathways. Remarkably, adipose tissue is a major source of circulating microRNAs (miRNAs), recently described as a novel form of adipokines. Several adipose tissue–derived miRNAs are deeply associated with adipocytes differentiation and have been identified with an essential role in obesity-associated inflammation, insulin resistance, and tumor microenvironment. During obesity, adipose tissue can completely change the profile of the secreted miRNAs, influencing circulating miRNAs and impacting the development of different pathological conditions, such as obesity, metabolic syndrome, and cancer. In this review, we discuss how miRNAs can act as epigenetic regulators affecting adipogenesis, adipocyte differentiation, lipid metabolism, browning of the white adipose tissue, glucose homeostasis, and insulin resistance, impacting deeply obesity and metabolic diseases. Moreover, we characterize how miRNAs can often act as oncogenic and tumor suppressor molecules, significantly modulating cancer establishment and progression. Furthermore, we highlight in this manuscript how adipose tissue–derived miRNAs can function as important new therapeutic targets.
Introduction
The worldwide statistics of overweight and obesity are considered a threat and contribute to a set of risk factors that enhances the development of several other diseases, such as type 2 diabetes, cardiovascular diseases, and cancer (1). Obesity is considered a low-grade chronic inflammation disease, which can lead to increased insulin resistance, altered preadipocyte differentiation, pre-existing adipocyte hypertrophy, macrophages infiltration and polarization, and the release of inflammatory cytokines (2).
Obesity is characterized by a dysfunction in adipose tissue homeostasis, with triglycerides accumulation and prominent adipocyte hypertrophy, resulting in hypoxia, adipocyte cell death, and free fatty acids released. There is also an intense inflammatory profile in adipose tissue from obese people due to the excessive macrophages and other immune cells infiltration to this tissue (3), which also contribute to the adipocyte cell death and free fatty acids release. Both adipocytes and immune cells are relevant sources of cytokines and chemokines secretion in adipose tissue. During obesity, the adipose tissue increases in mass and quantity, with a significant change in its metabolic and immunological profile, followed by adipocyte lytic cell death. This adipocyte death triggers free fatty acids and several metabolites release, thus pivoting the immune repertoire toward a type 1 (pro-inflammatory) state and establishing a microenvironment that supports oxidative stress, free radicals generation associated with DNA damage and mutations. These parameters can directly contribute to turning this microenvironment into a pro-carcinogenic model. Moreover, an increased free fatty acids release in adipose tissue during obesity can provide a direct faster source of energy for cancer cells, also remodeling the immune cell landscape and facilitating tumor establishment and development (4, 5). In addition, several inflammatory genes upregulation in immune cells can drive the inflammatory response itself in adipose tissue, contributing to the insulin resistance and glucose intolerance (6), critical factors associated with metabolic syndrome. Therefore, those metabolic and inflammatory changes in adipose tissue can disrupt physiological homeostasis both within local tissues and systemically, impacting directly the obesity development, as well as the initiation and progression of cancer and metabolic syndrome, creating positive feedback loops among them. Metabolic syndrome is defined as a cluster of metabolic disorders components, including obesity, cardiometabolic alterations, associated with other diseases such as type 2 diabetes, hypertension, and dyslipidemia with dysregulated levels of non-esterified fatty acids (NEFA) and decreased levels of high-density lipoprotein (HDL) (7). Metabolic syndrome leads to a higher secretion of inflammatory mediators, such as leptin, tumor necrosis factor alpha (TNF-α), monocyte chemoattractant protein-1 (MCP-1), resistin, and interleukin 6 (IL-6) (8). This prevalent inflammatory profile described in metabolic syndrome does not result from tissue injury, infection, or an autoimmune response, but instead, a meta-inflammation (9). Meta-inflammation is a chronic state of inflammation characterized by dysregulation of proinflammatory cytokines and chemokines, and mediated by macrophages (10). Recent studies have highlighted the importance of adipose tissue resident macrophages as a principal source for this inflammatory signature supporting meta-inflammation and miRNAs are key molecules in these signaling pathways (11).
Adipose Tissue and Macrophages
Adipose tissue can be classified into white, brown, beige and pink adipose tissues, which display different regulatory, morphological and functional characteristics of their adipocyte and immune cells (12). White adipose tissue (WAT) acts as a reservoir for energy storage and secretes paracrine factors that regulate other metabolic tissues. WAT is significantly expanded during obesity triggering several harmful effects, including inflammation, altered adipokines secretion, fibrosis, hypoxia, and mitochondrial dysfunction (13). Brown adipocytes precursors can transdifferentiate into white or beige, as wells as precursors of white adipocytes can differentiate into brown and beige (14). The brown adipose tissue (BAT) is related to a higher thermogenic activity, supporting non-shivering thermogenesis, and recently identified and characterized by proteomics-based analysis, secreted factors from BAT, known as batokines, targeting immune cells, regulating glucose homeostasis and insulin sensitivity, angiogenesis, and neurite outgrowth (15, 16). Recent studies have shown the role of miRNA in adipocyte development, metabolic functions, proliferation, and differentiation (17). The beige adipose tissue is an intermediate phenotype of WAT to BAT, which when stimulated by cold exposure or β-3 adrenergic agonists may lead to increased BAT markers expression, such as the uncoupling protein 1 (UCP-1), PGC-1α, PGC-1β, CIDEA, PPARγ, and PRDM16 (18). A forth type of adipose tissue, is the pink adipose tissue mainly composed by pink adipocytes (19, 20), which are milk-secreting alveolar cells that can arise from transdifferentiation of white adipocytes during pregnancy and lactation.
Macrophages can function as key sentinel orchestrators of the immune activity in adipose tissues. Adipose tissue resident macrophages have an important role mediating meta-inflammation, secreting inflammatory mediators such as chemokines, activating the recruitment of more immune cells to the adipose tissues (21, 22). Among all immune cells within the adipose tissue, macrophages contributed predominantly to the activation of inflammatory pathways within adipose tissues during the establishment of obesity and other metabolic diseases (23).
The infiltration of macrophages in adipose tissue, surrounding dead adipocytes can form the crown-like structures (CLSs), which are directly related to increased tissue inflammation and release of free fatty acids and other pro-inflammatory molecules. In obesity, there is an exacerbated presence of CLS in WAT (24). It has been described that CLS can promote the survival and proliferation of breast cancer in patients with obesity, influencing the tumor microenvironment (25).
The exposure of adipose tissue macrophages to different pro- or anti-inflammatory cytokines can lead to two polarization profiles, M1 (pro-inflammatory) and M2 (anti-inflammatory), respectively (26). The M1 profile is induced by interferon gamma (IFN-γ), microbial products such as LPS, and tumor necrosis factor alpha (TNFα). It is characterized by the release of increased levels of reactive oxygen species, interleukins IL-12, IL-10, and IL-23 (27). The M2 phenotype is triggered by glucocorticoid hormones and interleukins IL-4, IL-13, IL-10, and increased expression of arginase 1, also related to cell growth and proliferation (28). Lumen and colleagues analyzed white adipose tissue macrophages from lean and obese mice, showing that the M1 macrophages were prevalent in adipose tissue from obese mice. Moreover, in adipose tissue from lean mice, macrophages mostly polarized to M2 profile and presented a higher expression of anti-inflammatory proteins, such as arginase (29).
Both adipocytes and macrophages within the adipose tissue can secrete miRNAs, developing an important role between adipocytes and macrophages communication, as well as impacting on macrophages polarization and the inflammatory status of adipose tissue, as summarized in Figure 1. The polarization of macrophages to M1 or M2 phenotypes can be also regulated by miRNAs. Upregulation of miRNA-181a, miR-155-5p, and miR-451 is related to M1 polarization, contributing to the inflammatory status of adipose tissue (30). Adipose tissue macrophages could also regulate obesity-induced inflammation by downregulating miR-30 in high fat diet-induced obese mice, promoting the Notch1 signaling and M1 phenotype switch (31). Increased release of miR-155 by adipose tissue macrophages in obese mice is associated with insulin resistance and PPARγ modulation (32). Moreover, analysis from adipose tissue from knockout mice lacking miRNA-181, showed an increased expression of genes associated with M2 macrophages polarization as Ym1, Realm, and Arg1, while the genes related to pro-inflammatory M1 macrophage polarization were reduced (33). Therefore, miRNAs are key players in the adipocytes and immune cells crosstalk in adipose tissue, contributing significantly to the inflammatory status of this microenvironment.
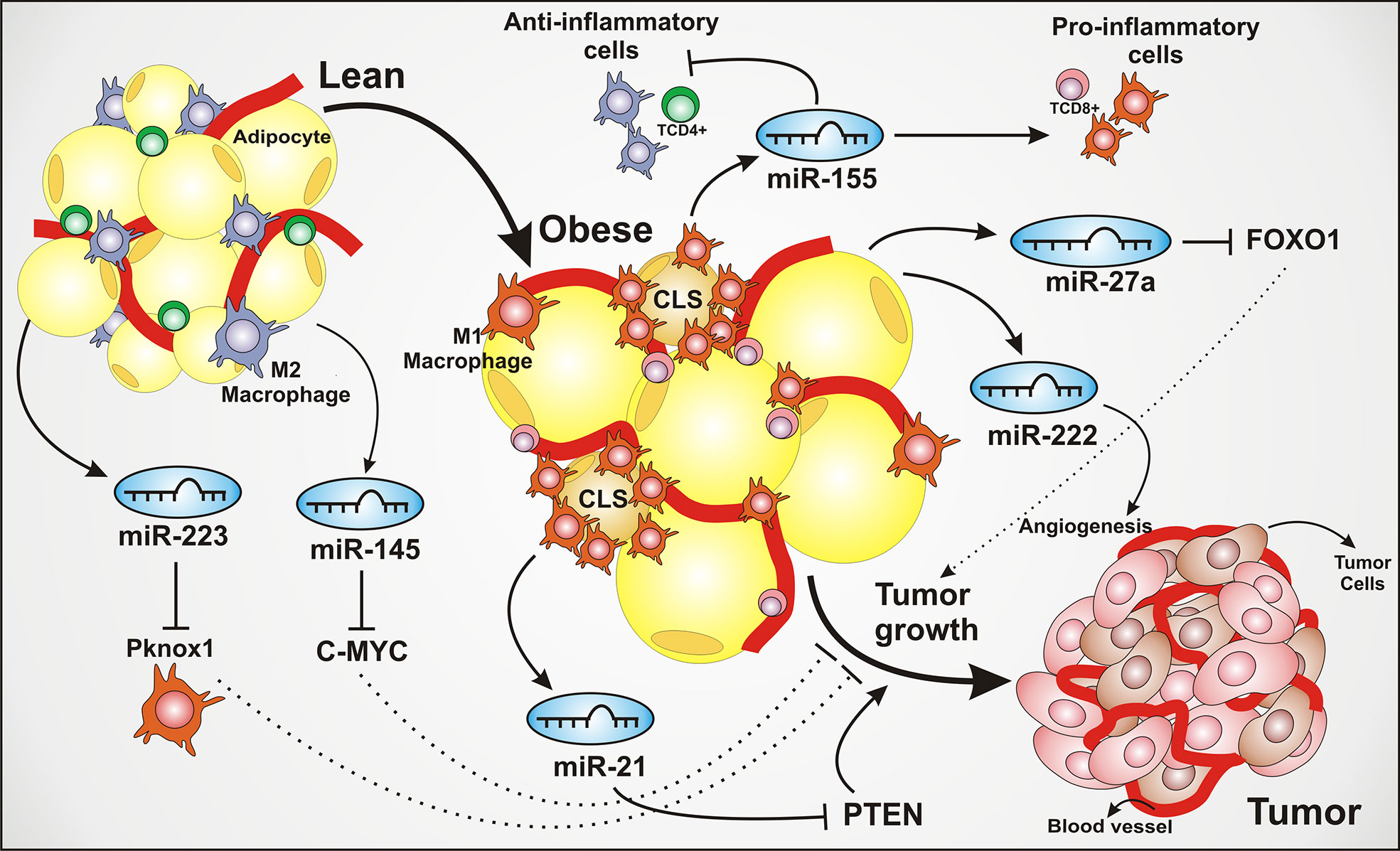
Figure 1 Adipose tissue–derived miRNAs modulate inflammation and tumor microenvironment. The adipose tissue produces and secretes several miRNAs that dynamically regulate the tissue homeostasis. These miRNAs can be secreted by both adipose tissue and macrophages, playing a crucial role impacting on inflammatory pathways, angiogenesis, cell growth, cell proliferation, and contributing to tumor development. The lean adipose tissue is characterized by an infiltrate of M2 polarized macrophages and TCD4+ lymphocytes. The secretion of miR-223, suppressing PBX/Knotted 1 Homeobox 1 (Pknox1), is shown to inhibit the activation of pro-inflammatory M1 macrophages, leading to an anti-inflammatory environment. The miR-145 exerts a tumor suppressor role, associated to the inhibition of oncogenic transcriptional factor C-MYC. The transition from lean to obese adipose tissue is characterized by the adipocytes hypertrophy, an increase of blood vessels and pro-inflammatory cell recruitment and polarizing as a common feature of obesity. Regarding the inflammatory recruitment, the infiltration of pro-inflammatory macrophages around the dying adipocytes is strongly present, forming structures known as CLS (crown-like structures), associated with the secretion of multiple inflammatory factors and considered an aggressiveness marker in breast cancer. The miR-21 is depicted being secreted by adipose tissue macrophages, also known as an oncomiR or oncogenic, through PTEN silencing, thus leading to the activation of tumor growth and proliferation pathways. The miR-155 contributes to the activation of the inflammatory response. The miR-222 is associated with angiogenesis, one of the tumor development hallmarks. The oncogenic miR-27a can lead to the inhibition of Forkhead box transcription factor O1 (FOXO1), an antitumoral multifunctional transcription factor, regulating genes linked to cell cycle arrest and apoptosis.
The Biogenesis of miRNAs
The miRNAs are small non-coding RNAs consisting of 19-23 nucleotides that regulate several biological processes mostly through post-transcriptional gene silencing mechanism and RNA interference. miRNAs suppress post-transcriptional activity, acting on several genes and many of these non-coding nucleotides are related to adipocyte development, lipolysis, insulin sensitivity, glucose uptake (34), and multiple cytokines release (35). It is known that hundreds of miRNAs can regulate up to 30% to 80% of genes encoded in the human genome, which each miRNA can target more than one hundred genes, undertaking gene regulation (36) A single miRNA may have multiple target sites in the 3’UTR region of a specific mRNA (messenger RNA) (36). The biogenesis of the miRNA can be distinguished into a canonical and non-canonical pathway.
The canonical transcriptional pathway begins with the RNA polymerase II/III leading to a long primary miRNA transcript, known as pri-miRNA, which has a 5’ cap and a poly A tail (37). The pri-miRNAs are transcribed and processed into pre-miRNAs by a complex consisting of an RNA-binding protein called DGCR8 (DiGeorge Syndrome Critical Region 8) and a ribonuclease III, Drosha, while DGCR8 recognizes the N6-methyladenylated GGAC and other motifs in the pri-miRNA are recognized by DGCR8. The DROSHA cleaves the duplex of pri-miRNA in the structure of the hairpin, resulting in the pre-miRNA. The pre-miRNA is transported into the cytoplasm by exportin 5 (XPO5)/RanGTP and then processed by Dicer RNase III endonuclease (38). The miRNA biogenesis and the impact of miRNAs on metabolic syndrome, obesity and cancer are summarized in Figure 2. The removal of the terminal loop leads to the mature miRNA duplex, which in turn is incorporated into a multimeric complex called RISC, including the Argonaut proteins as the main components, in an ATP-dependent manner.
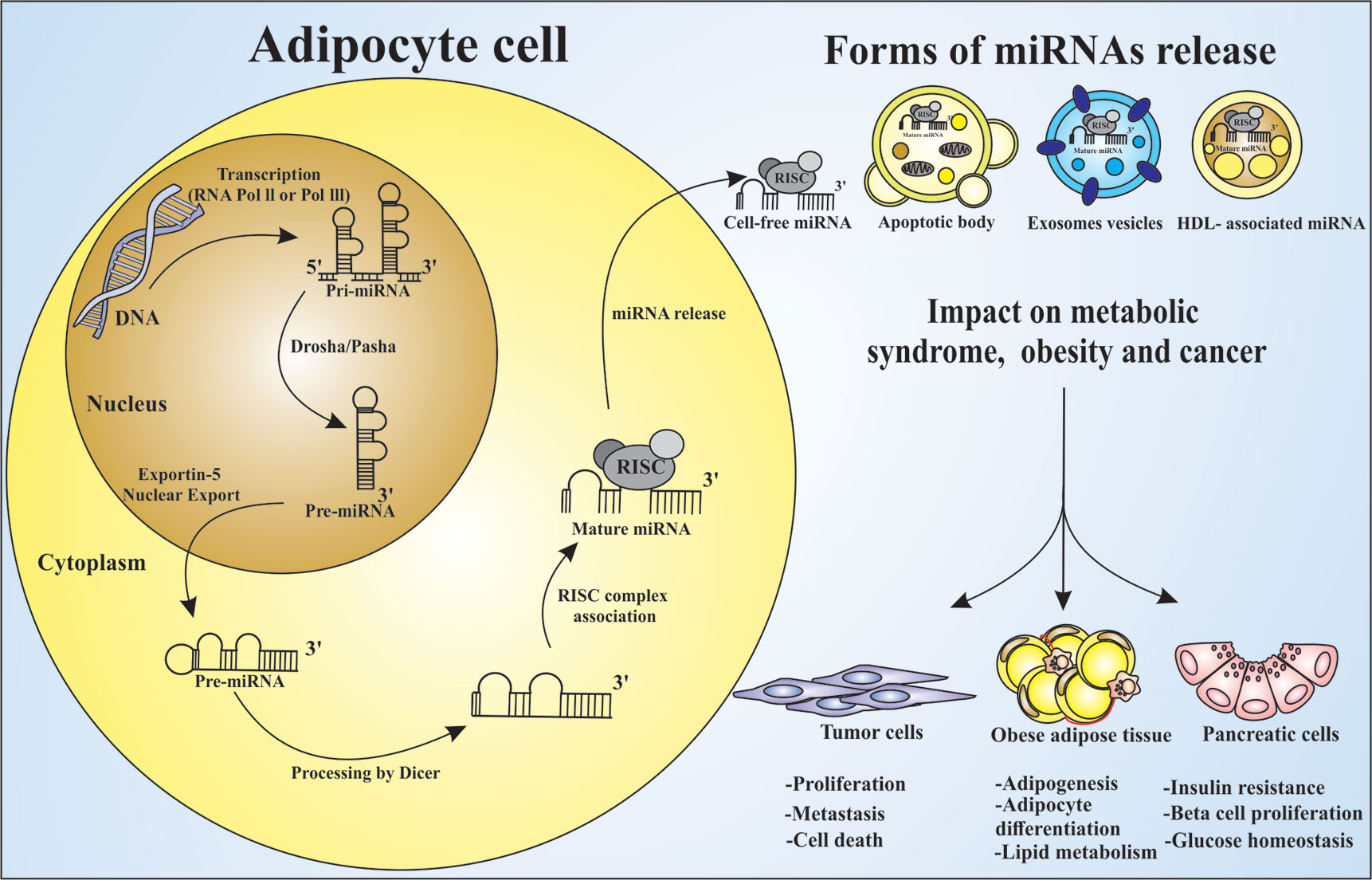
Figure 2 miRNAs processing and releasing by adipocytes and their influence on metabolic syndrome, obesity and cancer. Adipocytes are the main source of circulating miRNAs in mice and human. The process of miRNA maturation begins in the nucleus with the gene transcription by RNA polymerase II or III in pri-miRNA. Following the maturation process, the enzyme DROSHA and its Pasha cofactor leads to the pre-miRNA, which are exported to the cytosol by the Exportin-5 protein, and further processed by Dicer enzyme, leading to the mature miRNA. It is taken into the RISC silencing complex and then become an active post-transcriptional regulator. This may occur by cell free miRNA release, associated with apoptotic body, exosomes, or HDL. miRNAs can act influencing different types of cells. Circulating miRNAs are able to control: (I) the expression of several genes related to adipogenesis, (II) the adipocyte differentiation and lipid metabolism impacting obesity, (III) the expression of membrane proteins responsible for the glucose homeostasis and insulin resistance, (IV) the metabolic diseases development, and (V) the cancer establishment and progression as oncomiR molecules.
In the canonical pathway, miRNAs are generated by transcriptional units of protein-coding, while in the non-canonical pathway they are generated from non-protein-coding transcriptional units. Another critical difference between these two pathways is that in a canonical way the intronic miRNAs are Drosha-dependent and then processed with protein-coding transcripts in the nucleus. The pre-miRNA undergoes splicing producing the mature mRNA which directs to protein synthesis. In the non-canonical pathway, small introns of RNA, called mirtrons, are not processed by Drosha (39).
miRNAs show an essential role in gene expression, through mRNA degradation, deadenylation, or inhibiting the protein translational (40). Recent studies have shown that inhibition of Drosha and Dicer enzymes, critical in miRNA biogenesis, corroborated to the blockage of adipocytes differentiation and adipogenesis. Adipocytes are the major site of exosomal miRNAs, acting on cell to cell communication, as biomarkers in several diseases, including type 2 diabetes and cancer (41). The Dicer enzyme processes miRNA in the cytoplasm, a crucial stage of the miRNA biogenesis. The silencing of Dicer enzyme in adipose tissue, in a mice model, exhibited abnormal fat accumulation in the interscapular region and intra-abdominal, whitening of brown adipocytes, lipodystrophy, dyslipidemia, and insulin resistance (42). Lipodystrophy, characterized by lipoatrophy, loss of adipose tissue or abnormal distribution in specific regions anatomically, is a clinical manifestation of metabolic syndrome, as well as increased visceral fat accumulation and dyslipidemia (43).
The Role of miRNAs in White and Brown Adipose Tissue
Several works have shown the role of miRNAs in the development of metabolic disease, glucose homeostasis, and progression of obesity (44–47). miRNAs can exhibit a crucial impact on adipose tissues (Table 1) and influence the phenotype and function of white and brown adipocyte (75). miRNAs can function both as activators and inhibitors of brown adipogenesis and are crucial for adipose tissue plasticity and dynamics of adipocytes differentiation (76, 77). The absence of miRNAs can impact the differentiation of white adipocyte into brown adipocyte dynamics and can be involved in the recruitment and activation of thermogenic adipocytes (75). There is a strong correlation between miRNAs and the regulation of browning of the WAT.
Adipose tissue–specific DGCR8 knockout mice presented enlarged, but pale interscapular brown fat with decreased expression of characteristic genes of brown fat and intolerant to cold exposure (78). miR-182 and miR-203 were identified as key regulators of the brown adipocyte development (78). The recruitment and activation of constitutive BAT in humans, as well as the transdifferentiation of visceral and subcutaneous WAT into brown adipocytes appear as a new strategy for obesity treatment (79). BAT associated thermogenesis increases caloric burning, contributing to accelerating weight loss. That highlights the perspectives of these new targets to restore the normality of energy homeostasis and to struggle the progression of obesity by modulating the plasticity of adipose tissue, mainly visceral WAT (80). Since miRNAs are deeply associated with adipocytes differentiation and obesity, circulating miRNAs are very attractive biomarkers and therapeutic tools for obesity prevention and treatment (46).
The miRNA cluster miR-193b and miR-365 may display a regulation of brown fat fate through myogenesis and adipogenesis. Sun and colleagues compared the genome-wide miRNA profile expression of the epididymal WAT, skeletal muscle and interscapular BAT. The WAT samples presented an upregulation of miR-193b and miR-365 during adipogenesis, and a downregulation of up to 30% in BAT of ob/ob mice (metabolic impairment). The authors showed that miR-193b induced myoblasts to differentiate into brown adipocytes, and demonstrated that miR-193b and miR-365 365 can serve as essential regulators for brown fat differentiation, in part by repressing myogenesis (48).
The miR-103 expression could be related to impaired insulin sensitivity and glucose uptake, possibly an attractive pharmacological target in obesity and diabetes, promoting cell adipogenesis by targeting MEF2D and activating AKT/mTOR signaling pathway (81). The miR-103/107 also trigger endoplasmic reticulum (ER) stress-mediated apoptosis by targeting the Wnt3a/β-catenin/ATF6 signaling pathway in preadipocytes impairing adipogenesis (82). Overexpression of miR-103/107 was observed in obese mice and resulted in augmented accumulation of triglycerides and impaired regulation of adipogenic genes, while silencing of miR-103/107, improved glucose homeostasis and insulin sensitivity (51). Caveolin-1 could explain one of the mechanisms discussed for negative regulation regarding insulin sensitivity of miRNA-103. The miR-103 targets caveolin-1 and diminishes the expression of insulin receptors in the caveolae-enriched plasma membrane (51).
miRNAs also display a master role in brown adipogenesis of white fat progenitor cells, as reported for miR-196 in WAT. This miRNA suppresses the transcriptional factor HOXC8, avoiding the repression of its target C/EBPβ (CCAAT/enhancer binding protein β), considered a key regulator of BAT gene (49). Moreover, cold stimulus and β-3 adrenergic activation in white adipocytes can activate miR-196 in WAT from mice (49).
miR-133 is involved in BAT activation. miR-133 directly targets and negatively regulates PRDM16. In addition, inhibition of miR-133 can promote differentiation of precursors from BAT to mature brown adipocytes and increased mitochondrial activity in response to cold exposure (83). The upregulation of PRDM16 is linked to a decrease of miR-133a expression and browning of WAT enhancement (53), suggesting that pharmacological strategies to inhibit miR-133 could be very prominent to promote browning of the WAT. Likewise, miR-155 is enriched in BAT but efficiently inhibits adipogenic differentiation and development of the thermogenic program in brown adipocytes. Moreover, miR-155–null mice exhibit enhanced BAT function, while overexpressing miR-155 in mice induced a diminished browning markers expression, such as UCP1, BAT mass reduction, and a total impairment of BAT function (84).
miR-27 can negatively regulate brown and white adipogenesis both in mice (55, 85, 86) and humans (87, 88). miR-27 is reported to promote adipogenesis of WAT, targeting the 3’UTR region of PPARγ (Peroxisome Proliferator-Activated Receptor gamma) (17). The cold exposure can downregulate miR-27 expression in murine BAT and subcutaneous WAT (55). The miR-27 can also reduce the expression of brown markers genes, such as PRDM16, PPARα, CREB (Cyclic AMP Response Element Binding Protein), and PGC1β (Peroxisome Proliferator-Activated Receptor Gamma Co-activator 1 β). Moreover, the overexpression of miRNA-27 is also related to a higher risk of gastric cancer, promoting a transformation of cancer-associated fibroblasts (54).
miR-26a and miR-26b can trigger adipocytes differentiation, both in human and mice studies. The overexpression of miR-26a and miR-26b promote adipocyte differentiation of human multipotent adipose derived-stem cells (56). miR-26b also modulates insulin-stimulated AKT activation via inhibition of its target gene, PTEN (Phosphatase and tensin homolog), and significantly increases insulin sensitivity via the PTEN/PI3K/AKT pathway (57). Moreover, overexpression of miR-26b significantly increases the mRNA expression of the adipogenic markers, PPARγ, fatty acid synthase (FAS), CCAAT/enhancer-binding protein alpha (C/EBPα), and lipoprotein lipase (89).
The miR-143, miR-145, and miR-130 also exert a role in adipogenesis. The miR-130 inhibits PPARγ expression (90). The liver analysis from obese mice shows an increased expression of miR-143 and miR-145, also an association of these miRNAs expression and changes of insulin-stimulated AKT signaling pathway. The knockout mice for miR-143 and miR-145 did not develop obesity in insulin resistance conditions (58).
miRNAs are differentially expressed in white and brown adipocytes and may be important in defining the common precursor cell for myocytes and brown adipocytes and thus have distinct roles in energy‐storing versus energy‐dissipating cells. The miR-455 is related to browning of WAT induction, upregulating UCP-1 expression. Adipose-specific miR-455 transgenic mice exhibit brown fat characteristics in subcutaneous white fat, after cold exposure. miR-455 boosts AMPKα1 (AMP-activated kinase) activation through HIF-1α (hypoxia inducible factor 1 α subunit) inhibitor (62). The AMPKα1 contributes to brown adipogenic effect and induces mitochondrial biogenesis (91). The classic myogenic miR-1 and miR-206 are absent in murine white adipocytes and expressed both in brown pre‐ and mature adipocytes, strengthening the concept that brown adipocytes and myocytes can share a similar progenitor cell lineage that specifies energy‐dissipating cells (92).
A higher miR-34a expression was detected in obese mice (60) and on serum analysis from patients with type 2 diabetes (93). The miR-34 suppresses browning of WAT, directly affecting adiposity, adipocyte differentiation, and metabolism. It targets FGFR1 (Fibroblast Growth Factor Receptor 1) and SIRT 1(sirtuin 1) (60). The suppression of SIRT1 (sirtuin) is related to impaired transcriptional regulation of brown and beige markers through deacetylation of PPARGC1-α (60, 61). The lentiviral-mediated knockdown of miR-34a under a high-fat diet have shown a different pattern of perirenal and gonadal WAT depots distribution, and less ill metabolic effects (94).
Microarray analysis of human WAT has revealed distinct miRNA expression that may be dysregulated in patients with obesity and other comorbidities, such as type 2 diabetes mellitus and heart diseases (95). Dysregulation of miR-20, miR-98, miR-197, and miR-331 were common in patients with obesity and type 2 diabetes mellitus (96). The molecular pattern of miRNAs may be distinct in patients with morbid obesity, as seen in a circulating genome-wide miRNA pattern from obese patients before and after bariatric surgery. The results revealed a differentiated profile of miRNA expression in cases of severe obesity. Among these miRNAs the miR-142-3p, miR-140-5p, miR-15a, miR-520c-3p, and miR-423-5p were identified as potential new biomarkers of severe obesity (97).
The overall miRNA screening of white subcutaneous adipocytes performed in obese patients and in lean patients reported an overexpression of miR-143-3p and miR-652-3p in obese patients when compared to lean patients, also detected a higher chemokine CCL2 production and lipolysis from necrotic adipocytes from obese WAT, demonstrating an inflammatory response triggered by these miRNAs overexpression. The expression of these miRNAs has been reported to increase insulin-stimulated lipogenesis, affecting genes at transcriptional and post-transcriptional levels of insulin signaling (98).
The dysregulation of miRNAs expression in white and BATs can be a valuable target and biomarker in diseases associated with adipose tissue dysfunction, such as metabolic syndrome and cancer (99, 100).
The Function of Adipose Tissue miRNAs in Metabolic Syndrome
Metabolic syndrome is a cluster of cardiometabolic risk factors characterized with obesity, hyperglycemia, dyslipidemia, hypertension, atherogenic, prothrombotic, and proinflammatory states, NAFLD, often associated with a condition of insulin resistance (101, 102). Adipose tissue miRNAs have direct impact on metabolically important organs, modifying cell physiology and acting as key mediators of metabolic syndrome features. miRNAs can regulate multiple pathways including insulin signaling, meta-inflammation, adipogenesis, lipid metabolism, and food consumption (103). The miRNAs are strongly related to immune response dysfunctions in metabolic syndrome, as in macrophages polarization (104), adipogenesis regulators and mediators of the fatty acids metabolism, also contributing to adipocyte hypertrophy in obesity, hepatic steatosis, and lipid homeostasis disorders, insulin signaling pathways, β-pancreatic cells mass, and endocrine function (105).
The first evidence highlighting miRNAs role in metabolic syndrome was described in the studies using Drosophila adipocytes, demonstrating that miR-14 had a suppressive effect on fat metabolism through the MAPK and p38 pathways in this model (99). The screening of several miRNAs demonstrated correlation between obesity and metabolic syndrome, specially linked to insulin resistance and regulation of β-pancreatic cells. The beta-pancreatic cell death can trigger pancreatic dysfunction and promote type 2 diabetes. Studies have reported the overexpression of the miR-200 family in pancreatic islets. The analysis of diabetic mice showed a negative regulation of miR-200 in anti-apoptosis pathways, thus favoring beta-pancreatic cell death (106). The overexpression of miR-7 in beta cells from mice showed an impairment of insulin granule exocytosis (107).
Meta-inflammation is a common feature of metabolic syndrome, and miRNAs can actively regulate this process, by altering macrophages phenotype and modulating the inflammatory molecules secretion within the adipose tissue. Studies reported the ablation of miR-223 in mice undergoing a high-fat diet, resulted in increased M1 macrophages infiltration, as well, higher pro-inflammatory cytokines secretion, such as IL-6, IL-12, TNF-α, and IL-1β (108). This meta-inflammation is also closely related to changes in the proliferative pathways, as seen for miR-21 dysfunction in obesity, affecting angiogenesis and cell growth (103). These miRNAs-mediated alterations detected in metabolic syndrome, such as exacerbated meta-inflammation, enhanced c proliferative and a pro-angiogenic microenvironment in WAT, could partially explain the higher predisposition to several types of cancer observed in people with obesity.
The miRNAs dysfunction in obesity and metabolic syndrome was detected on the FXR/SHP signaling pathway. Fat metabolism and cholesterol homeostasis are one of the axes that can be unbalanced in metabolic syndrome. The miR-33 is related to cholesterol trafficking in vitro and HDL synthesis also regulates cholesterol transport and lipid homeostasis (68, 109). The FXR/SHP can inhibit miR-34a expression (110). The Farnesoid X Receptor (FXR) is a nuclear receptor, controlling the regulation of genes expression related to fatty acids metabolism, cholesterol levels, through inhibition of cytochrome P450 7A1, which is necessary for hepatic bile acids synthesis. The effect of miR-34a inhibition leads to an upregulation of SIRT1 and dysregulation of FXR/SHP pathway in diet- induced obese mice (111). The miR-34a can also reduce the NAD+ (Nicotinamide Adenine Dinucleotide) and SIRT1 activity, targeting NAMPT (Nicotinamide Phosphoribosyltransferase), a key enzyme for NAD+ synthesis. The overexpression of miR-34a increases mRNA degradation of NAMPT, resulting in decreased levels of NAMPT/NAD+. The dysregulation on this pathway alters PGC-1α (Peroxisome Proliferator-Activated Receptor Gamma Coactivator 1-alpha), SREBP-1c (Sterol Regulatory Element-Binding Transcription Factor 1), and NF-κB, enhancing obesity. The antagonism of miR-34a reduces hepatic steatosis, glucose intolerance, and inflammation. The inhibition of miR-34a represents a potential therapeutic target against obesity and metabolic syndrome (112).
The miR-30c is related to hMADS (human multipotent adipose-derived stem cells) and promotes adipogenesis and accumulation of triglycerides. The miR-30c targets PAI-1 (Plasminogen Activator Inhibitor-1) and ALK2 (Activin-like kinase receptor 2), which are downregulated by miR-30c in adipogenesis. The correlation of miR-30c and PAI-1 was observed in WAT from obese mice, suggesting an endocrine regulation role of these molecules during obesity (113).
Concerning the insulin regulation by miRNAs, recent reports suggest post-transcriptional mediated by IGF-1 (insulin-like growth factor 1) and INSR (insulin receptor) activation (114), GLUT4 protein expression (115), pancreatic beta cells proliferation, and differentiation (116, 117). The inhibition of miRNA-320 resulted in increased insulin sensitivity, improved AKT phosphorylation and GLUT4 expression (67, 118). The miRNA-93 expression was inversely correlated with GLUT4 levels in adipose tissue from non-obese insulin-resistant patients and its overexpression in adipocytes downregulated GLUT4 expression (119).
miRNA-375 is one of the most abundant miRNAs in pancreatic beta cells and overexpression of miR-375 can suppress glucose-induced insulin secretion, and conversely, inhibition of endogenous miR-375 function can enhance insulin secretion (110). This process is mediated by myotrophin and PDK1 (Phosphoinositide-dependent kinase-1), a key molecule in PI 3-kinase signaling (120). The silencing of miR-375 in leptin-deficient diabetic mice resulted in hyperglycemia, increased glucagon concentration, and gluconeogenesis. In addition, miRNA-375 knockout mice presented an altered pancreatic β cell mass, resulting in severe diabetes (69). The miR-375 low levels in pancreatic beta cells are associated with pancreatic cancer progression, metastasis, and angiogenesis (121).
Indeed, growing data have indicated a strong association of metabolic syndrome or its components with cancer development and cancer-related mortality (122–127). It is possible for the association between metabolic syndrome and cancer to be mediated by the dysfunctional adipose tissue, exacerbated meta-inflammation, coexistence of obesity status, and insulin resistance as the main factors. Importantly, adipose tissue miRNAs could be the one of the key molecules orchestrating this crosstalk linking metabolic syndrome and cancer.
The Role of Adipose Tissue miRNAs in Cancer
Hanahan e Weinberg described the hallmarks of cancer, providing new insights of carcinogenesis establishment, such as self-sufficiency in growth signals, evading apoptosis, insensitivity to anti-growth signals, sustained angiogenesis, limitless replicative potential, tissue invasion and metastasis, immune response evasion and metabolic reprogramming, genetic instability, mutations, and tumor-promoting inflammation (128, 129). The high plasticity of the adipose tissue and its inflammatory content can directly regulate the tumor microenvironment, defining cancer establishment and progression (12). The crosstalk between adipocytes and other components of the adipose tissue, such as immune cells, can be crucial to determine the cancer cells fate (12). Adipose tissue–derived circulating miRNAs, considered novel adipokines (130), can affect important signaling pathways, directly impacting the carcinogenesis pillars.
Several studies reported a higher risk of cancer in patients with obesity. There are at least 13 types of cancer which this correlation is already described, such as esophagus (131), gastric (132), colon and rectum (133), liver (134), prostate (135), ovary (136), kidney (137), meningioma (138), thyroid (139), and multiple myeloma (140). miRNA expression in adipose tissue from people with obesity can play a crucial role in tumor progression, cancer growth and metastasis. miRNAs mediate the crosstalk of adipocytes and macrophages, modulating the inflammatory and angiogenic microenvironment (141, 142). miRNAs are indeed dysregulated in most, if not all, cancers (143). However, the function of several miRNAs in cancer is very controversial in the literature (144). Many reports are demonstrating unique miRNAs acting both as miRNAs oncogenic (oncomiR) and tumor-suppressive. Specific miRNAs can simultaneously trigger anti-cancer effects by suppressing oncogenic mRNAs, and pro-tumoral effects by suppressing tumor suppressive mRNAs. A balance between these two signaling pathways will determine whether a miRNA is an oncomiR or a tumor-suppressive molecule (144).
The miRNA-377 is considered the master adipogenesis regulator under stress stimulus in WAT (70). Studies reported miRNA-377 targeting SIRT1 and reducing its expression in diet obese mice, causing inflammation and insulin resistance in 3T3-L1 cells (70). SIRT1 exerts a role in tumor initiation, progression, and angiogenic pathways (145), as depicted in murine hepatocellular carcinoma (146), highlighting the potential antitumoral role of this miRNA, through a SIRT pathway. SIRT1 is also correlated with inflammation and insulin resistance, which contributes to the development of a pro-tumoral environment, increasing the risk of ROS (reactive oxygen species) production, thus leading to DNA damage and mutations, genome instability, one of the hallmarks of carcinogenesis (147).
The miRNA-34 family includes the miRNA-34a, miRNA-34b, and miRNA-34c clusters, found in WAT. The tumoral suppressor p53 is a direct transcriptional target of miR-34. The p53 is activated under cellular stresses and promotes cell cycle arrest, senescence, and apoptosis (148). Recent data also reports the role of p53 in immune response, metabolism, and inflammation (149). The miRNA-34 inhibits the proliferation of breast cancer cells through a mechanism of SIRT1 and Bcl-2 downregulation (61), and thus miR-34 seems to have an anti-tumor effect (150).
The cluster of miR-221 and miR-222 exerts a role in vascular remodeling in atherogenic processes, inhibiting the angiogenic recruitment of endothelial cells, thus facilitating the atherogenic calcification (63). These miRNAs are also related to human breast cancer development, controlling the epithelial-to-mesenchymal transition, downstream the oncogenic RAS-RAF-MEK pathway (64). The miR-223 controls macrophage polarization preventing mice from inflammation induced by diet and insulin resistance. The anti-inflammatory mechanism described for miR-223 is the suppression of classical proinflammatory M1 macrophages response in adipose tissue (108).
The miR-125b controls mitochondria integrity and is related to metabolic adaptations of monocytes, driving inflammation, and apoptosis (65). The miR-100 was identified as an important factor maintaining the M2 phenotype of tumor associated adipose tissue macrophages. The miR-100 targets the mTOR pathway and was also observed in some studies a higher expression in tumor-associated macrophages isolated from mouse breast tumor tissue. The therapeutic strategy of combining miR-100 inhibition with cisplatin could suppress mouse lung metastasis (151).
The miR-193b/126/26a are up-regulators of adiponectin secretion in human white adipocytes, modifying the expression of adiponectin regulators genes, such as the nuclear receptor-interacting protein 1 and nuclear transcription factor Yα (152). Adiponectin augments adipogenesis and prevent ectopic lipid accumulation as liver steatosis, exerting a protective effect against insulin resistance and cardiovascular diseases (153). Meta-analysis studies demonstrated that low levels of adiponectin are correlated to a higher risk of postmenopausal breast cancer (154) and increased adiponectin serum levels confered a protective effect on endometrial cancer (155).
Adipogenesis and immunological recruitment can alter the tumor microenvironment. The miR-183 targets low-density lipoprotein receptor-related protein in 3T3-L1 cells, promoting differentiation and adipogenesis by interfering with the Wnt/β catenin pathway (156) and tumor-derived TGF-β promotes miR-183 expression, silencing NK cells, through a mechanism mediated by DAP12 suppression. The DAP12 downregulation facilitates the cancer establishment, avoiding NK cells to recognize and eliminate the tumor cells, maintaining cancer cells survival and spread, in a pathway dependent on miR-183, suggesting a pro-tumoral role for this miRNA (157).
The miR-21 contributes with adipogenic differentiation of human adipose tissue–derived mesenchymal stem cells, through TGF-β signaling (71) and is also associated with cancer. The overexpression of miR-21 was detected in pancreatic, colon, breast, and glioblastoma tumors (158). Studies reported the overexpression of miR-21 in colorectal cancer with advanced metastasis stage. miR-21 is associated with anti-apoptotic effects, promoting cellular proliferation by targeting tumor suppressors, such as PTEN. Moreover, this event was inversely correlated with miR-21 expression, as summarized in Figure 1 (72). Some miRNAs act inhibiting tumor progression, leading to oncogenes mRNA degradation or repression of the oncogenic mRNA translation (159). The miR-15a/miR-16-1 cluster interacts with Bcl-2 mRNA, resulting in leukemic cell apoptosis (160).
The secretion of miR-31 from adipose tissue–derived stem cells is associated with endothelial cell migration and angiogenesis (161). Furthermore, Factor Inhibiting HIF-1α (FIH), an anti-angiogenic gene is one of the targets of miR-31, indicating a possible correlation between hypoxia, adipose tissue dysfunction, and tumoral signaling pathways (161). Besides angiogenic pathways facilitating the tumor cells spread, the mitochondrial biogenesis and oxygen consumption can also be associated with tumor growth (162). miRNAs can induce mitochondrial metabolic alterations and a shift from oxidative to glycolytic metabolism, by targeting components involved in electron transport chain/oxidative phosphorylation (OXPHOS) and in tricarboxylic acid cycle, thus contributing to Warburg effect and cancer progression (163, 164). miR-126 can affect mitochondrial function and mitochondrial metabolism in cancer cells, leading to tumor suppression of cancer cells by inducing tumor cells metabolic reprogramming (165). Obesity can reduce the miR-126 expression (166), and this miRNA is often lost in several types of cancer (167).
In both humans and mice, the miR-125b expression is higher in WAT than in BAT, especially during obesity. It has been shown that miR-125b-5p can play an important role in the repression of brite adipocyte function by modulating oxygen consumption and mitochondrial gene expression (66). The higher expression of miR-125b was shown to be related to prostate cancer risk, in a mechanism associated with androgenic receptor stimulus, also promoting tumor growth by modulating the cell cycle, apoptosis, and cell migration (168). The role of miR-125b in stem-cells hematopoiesis, as well as the activation and expression of this miRNA in human leukemia, shows its function as an oncomiR (168). However, the role of miR-125 in cancer is very controversial, since miR-125b can act not only as an oncomiR in the vast majority of hematologic malignancies, but also as a tumor suppressor in many solid tumors (169, 170).
The miRNA-130 family, including miRNA-130a and miRNA-130b, is deeply related to cancer progression (109, 171). It has been demonstrated the correlation of miR-130a and miR-130b expression in preadipocytes isolated from abdominal subcutaneous human adipose tissue and PPARγ repression, inhibiting adipogenesis (90). Likewise, PPAR γ activation could be protective against gastric cancer (172). Increased levels of miR-130b were found in advanced tumor stages (III–IV) of colorectal cancer patients (109). The pharmacological inhibition of miR-130 family molecules by seed-targeting with an 8-mer tiny locked nucleic acid (LNA) inhibited bladder cancer cell growth, migration, and invasion by repressing stress fiber formation (173). Overall, miRNA-130 is highly expressed in patients with different types of cancer and is correlated with severe tumors and poor cancer prognosis (174).
The miR-193b-365 cluster is defined as a brown fat-enriched cluster, already depicted in lineage determination of brown adipocytes, and brown adipocyte adipogenesis in mice studies (105). The miR-193 has shown an anti-tumoral effect in breast cancer, by decreasing cell proliferation and migration (175). Besides, miR-193a-3p suppressed cancer gastric growth and motility (176). The miR-365 expression was downregulated in glioblastoma (177) and inhibited cell proliferation of glioma and malignant melanoma (177–179).
Studies reported the silencing of miR-145 associated with increased liver inflammation in mice. Likewise, the lentivirus-mediated miR-145 inhibited macrophage infiltration, ameliorated glucose metabolism, and weight loss (180). This target has been characterized as a tumor-suppressor miRNA, impacting cell growth (59), leading to Myc proto-oncogene downregulation (181). The miR-145 suppressed gastric cancer cell proliferation through cell cycle arrest and apoptosis (182).
Another crucial miRNA related to BAT, is the miRNA-155. The miRNA-155 acts in the adipogenic transcription factor CCAAT/enhancer-binding protein β (84) and HIF-1α (73). Studies demonstrated a higher expression of miR-155 in mice intestinal tissue under hypoxia stimulus (73). Inflammation upregulates miRNA-155 as seen in biopsies taken from patients with obesity, and TNFα- treated adipocytes, contributing to impair adipocytes function, as summarized in Figure 1 (183). It was also described the correlation of miR-155 expression in lung cancer and hypoxia, which impacts tumor microenvironment and cancer progression (74). The miR-155 can be involved in the chemotherapy resistance, and this could be due to hypoxia and inflammation signaling pathways (184).
According to the miRNA target, the miRNA-based therapies can follow two opposite directions, acting as tumor suppressor or oncogenic molecules. The therapeutic strategy can be based on miRNA antagonist molecules such as synthetic oligonucleotides (antagomiRs), locked nucleic acids (LNA), a class of nucleic acid analogs, capable of forming base pairs with complementary nucleosides, demonstrating high affinity and resistant nucleases degradation (185), silencing the oncogenic miRNA and finally the miRNA mimetics in the tumor, restoring the protective action of the miRNAs. AntagomiRs are oligonucleotides developed to silence specific miRNA. Studies have reported that intravenous administration of antagomiRs in mice accomplished great results, with high specificity and low toxicity (186).
The MRX34 drug, the liposomal formulation of a synthetic miRNA-34a followed to phase 1 clinical trial. It was administered intravenously in patients under advanced tumor stage and refractory to standard chemotherapy. The drug strategy was based on mimic miRNA therapy, to restore tumor suppressor miRNAs levels, in solid cancers, such as liver and spleen (187). However, due to serious adverse events, including immune reactions, the clinical trials did not proceed to phase 2. Although advances to clinical phase 3 have not been described, new candidates, such as the drug RGLS5579, targeting miR-10b in glioblastoma and inhibitors of miR-155 and miR-21 in the treatment of hematological, breast and lung tumors have been developed (188). In addition, LNA-anti-miR-21 for targeting miR-21 expression to treat colon adenocarcinoma has been investigated (189).
Remarkably, miRNAs are related to all hallmarks of cancer, including uncontrolled cell proliferation and growth capacity, cell death evasion, tumor-promoting inflammation signals, antigrowth signals, improved pro-angiogenic function, metastatic potential, energy metabolism reprogramming, immune response evasion, and genomic instability. Overall, several epigenetic and genetic modifications may occur in cancer cells and lead to the gain of function of oncomiRs and loss of function of tumor suppressor miRNAs. It is important to note that several miRNAs can act both as oncomiR and tumor suppressor molecules. Therapies based on miRNA must also consider that cancer cells present an altered miRNAs expression, and tumor phenotype can be changed by the modulation of the miRNA expression. Therefore, a careful design and approach, is essential to use miRNAs as very prominent therapeutic tool against cancer.
Conclusion
Obesity can often lead to metabolic impairment, triggering inflammation, and contributing to disorders related to adipogenesis and lipid metabolism. Adipose tissue–derived miRNAs exert a crucial role in adipocyte differentiation, adipogenesis, and hormone homeostasis being deeply related to the establishment of metabolic syndrome, obesity and cancer. miRNAs can act as oncogenes and tumor suppressor factors, depending on its expression and mRNA target, demonstrating a defying way to pharmacological strategies, according to the tumor microenvironment and the impaired signaling pathways. The ever growing data showing the involvement of miRNAs in cancer progression and invasion is supporting the arising use of miRNAs for clinical assessment of cancer aggressiveness and tumor biomarkers, as well as turning miRNAs in promising molecules for therapeutic anti-cancer approaches. miRNAs can deeply change important physiologic characteristics of white and BATs, impacting browning of the WAT, modulating the inflammatory response, and therefore influencing directly the obesity and metabolic syndrome status, and thus cancer predisposition. Moreover, adipose tissue is considered as the major source of circulating miRNAs, acting both in paracrine and endocrine signaling, modulating tumor microenvironment, affecting cancer establishment and progression. Therefore, adipose tissue–derived miRNAs may represent a promising therapeutic target against metabolic syndrome, obesity, and cancer.
Author Contributions
All authors contributed to the article and approved the submitted version.
Conflict of Interest
The authors declare that the research was conducted in the absence of any commercial or financial relationships that could be construed as a potential conflict of interest.
References
1. Koliaki C, Liatis S, Kokkinos A. Obesity and cardiovascular disease: revisiting an old relationship. Metabolism (2019) 92:98–107. doi: 10.1016/j.metabol.2018.10.011
2. Jung UJ, Choi MS. Obesity and its metabolic complications: The role of adipokines and the relationship between obesity, inflammation, insulin resistance, dyslipidemia and nonalcoholic fatty liver disease. Int J Mol Sci (2014) 15:6184–223. doi: 10.3390/ijms15046184
3. Hotamisligil GS, Arner P, Caro JF, Atkinson RL, Spiegelman BM. Rapid Publication Obesity and Insulin Resistance. J Clin Invest (1995) 95:2409–15. doi: 10.1172/JCI117936.Rapid
4. De Pergola G, Silvestris F. Obesity as a major risk factor for cancer. J Obes (2013) 2013:291546. doi: 10.1155/2013/291546
5. Howe LR, Subbaramaiah K, Hudis CA, Dannenberg AJ. Molecular Pathways: Adipose Inflammation as a Mediator of Obesity-Associated Cancer. Clin Cancer Res (2013) 19:6074–83. doi: 10.1158/1078-0432.CCR-12-2603
6. Olefsky JM, Glass CK. Macrophages, Inflammation, and Insulin Resistance. Annu Rev Physiol (2010) 72:219–46. doi: 10.1146/annurev-physiol-021909-135846
7. Ginsberg HN, MacCallum PR. The obesity, metabolic syndrome, and type 2 diabetes mellitus pandemic: Part I. Increased cardiovascular disease risk and the importance of atherogenic dyslipidemia in persons with the metabolic syndrome and type 2 diabetes mellitus. J Cardiometab Syndr (2009) 4:113–9. doi: 10.1111/j.1559-4572.2008.00044.x.The
8. Kang YE, Kim JM, Joung KH, Lee JH, You BR, Choi MJ, et al. The roles of adipokines, proinflammatory cytokines, and adipose tissue macrophages in obesity-associated insulin resistance in modest obesity and early metabolic dysfunction. PloS One (2016) 11:1–14. doi: 10.1371/journal.pone.0154003
9. Sharma P. Inflammation and the Metabolic Syndrome. Indian J Biochem (2011) 26:317–8. doi: 10.1155/2010/289645.123
10. Li C, Xu MM, Wang K, Adler AJ, Vella AT, Zhou B. Macrophage polarization and meta-inflammation. Transl Res (2018) 191:29–44. doi: 10.1016/j.trsl.2017.10.004
11. Weisberg SP, McCann D, Desai M, Rosenbaum M, Leibel RL, Ferrante AW. Obesity is associated with macrophage accumulation in adipose tissue. J Clin Invest (2003) 112:1796–808. doi: 10.1172/JCI19246
12. Corrêa LH, Heyn GS, Magalhaes KG. The Impact of the Adipose Organ Plasticity on Inflammation and Cancer Progression. Cells (2019) 8:662. doi: 10.3390/cells8070662
13. Ejarque M, Ceperuelo-Mallafré V, Serena C, Maymo-Masip E, Duran X, Díaz-Ramos A, et al. Adipose tissue mitochondrial dysfunction in human obesity is linked to a specific DNA methylation signature in adipose-derived stem cells. Int J Obes (2019) 43:1256–68. doi: 10.1038/s41366-018-0219-6
14. Peirce V, Carobbio S, Vidal-Puig A. The different shades of fat. Nature (2014) 510:76–83. doi: 10.1038/nature13477
15. Villarroya J, Cereijo R, Gavaldà-Navarro A, Peyrou M, Giralt M, Villarroya F. New insights into the secretory functions of brown adipose tissue. J Endocrinol (2019) 243:R19–27. doi: 10.1530/JOE-19-0295
16. Deshmukh AS, Peijs L, Beaudry JL, Jespersen NZ, Nielsen CH, Ma T, et al. Proteomics-Based Comparative Mapping of the Secretomes of Human Brown and White Adipocytes Reveals EPDR1 as a Novel Batokine. Cell Metab (2019) 30:963–975.e7. doi: 10.1016/j.cmet.2019.10.001
17. Kulyté A, Kwok KHM, de Hoon M, Carninci P, Hayashizaki Y, Arner P, et al. MicroRNA-27a/b-3p and PPARG regulate SCAMP3 through a feed-forward loop during adipogenesis. Sci Rep (2019) 9:13891. doi: 10.1038/s41598-019-50210-3
18. Ikeda K, Maretich P, Kajimura S. The Common and Distinct Features of Brown and Beige Adipocytes. Trends Endocrinol Metab (2018) 29:191–200. doi: 10.1016/j.tem.2018.01.001
19. Giordano A, Smorlesi A, Frontini A, Barbatelli G, Cinti S. MECHANISMS IN ENDOCRINOLOGY: White, brown and pink adipocytes: the extraordinary plasticity of the adipose organ. Eur J Endocrinol (2014) 170:R159–71. doi: 10.1530/EJE-13-0945
20. Cinti S. Pink Adipocytes. Trends Endocrinol Metab (2018) 29:651–66. doi: 10.1016/j.tem.2018.05.007
21. Gual P, Le Marchand-Brustel Y, Tanti JF. Positive and negative regulation of insulin signaling through IRS-1 phosphorylation. Biochimie (2005) 87:99–109. doi: 10.1016/j.biochi.2004.10.019
22. Han MS, Jung DY, Morel C, Lakhani SA, Kim JK, Flavell RA, et al. JNK Expression by Macrophages Promotes Obesity-induced Insulin Resistance and Inflammation. Sci (80- ) (2013) 339:218–22. doi: 10.1038/jid.2014.371
23. Weisberg SP, Mccann D, Desai M, Rosenbaum M, Leibel RL, Ferrante AW. Obesity is associated with macrophage accumulation. J Clin Invest (2003) 12:112. doi: 10.1172/JCI200319246.Introduction
24. Xu X, Grijalva A, Skowronski A, van Eijk M, Serlie MJ, Ferrante AW. Obesity Activates a Program of Lysosomal-Dependent Lipid Metabolism in Adipose Tissue Macrophages Independently of Classic Activation. Cell Metab (2013) 18:816–30. doi: 10.1016/j.cmet.2013.11.001
25. Faria SS, Corrêa LH, Heyn GS, de Sant’Ana LP, Almeida RDN, Magalhães KG. Obesity and breast cancer: The role of crown-like structures in breast adipose tissue in tumor progression, prognosis, and therapy. J Breast Cancer (2020) 23:233–45. doi: 10.4048/jbc.2020.23.e35
26. Gordon S, Martinez FO. Alternative activation of macrophages: Mechanism and functions. Immunity (2010) 32:593–604. doi: 10.1016/j.immuni.2010.05.007
27. Liu Y-C, Zou X-B, Chai Y-F, Yao Y-M. Macrophage Polarization in Inflammatory Diseases. Int J Biol Sci (2014) 10:520–9. doi: 10.7150/ijbs.8879
28. Raggi F, Pelassa S, Pierobon D, Penco F, Gattorno M, Novelli F, et al. Regulation of human Macrophage M1-M2 Polarization Balance by hypoxia and the Triggering receptor expressed on Myeloid cells-1. Front Immunol (2017) 8:1097. doi: 10.3389/fimmu.2017.01097
29. Lumeng CN, Delproposto JB, Westcott DJ, Saltiel AR. Phenotypic switching of adipose tissue macrophages with obesity is generated by spatiotemporal differences in macrophage subtypes. Diabetes (2008) 57:3239–46. doi: 10.2337/db08-0872
30. Zhang Y, Zhang M, Zhong M, Suo Q, Lv K. Expression profiles of miRNAs in polarized macrophages. Int J Mol Med (2013) 31:797–802. doi: 10.3892/ijmm.2013.1260
31. Miranda K, Yang X, Bam M, Murphy EA, Nagarkatti PS, Nagarkatti M. MicroRNA-30 modulates metabolic inflammation by regulating Notch signaling in adipose tissue macrophages. Int J Obes (2018) 42:1140–50. doi: 10.1038/s41366-018-0114-1
32. Virtue A, Johnson C, Lopez-Pastraña J, Shao Y, Fu H, Li X, et al. MicroRNA-155 deficiency leads to decreased atherosclerosis, increased white adipose tissue obesity, and non-alcoholic fatty liver disease a novel mouse model of obesity paradox. J Biol Chem (2017) 292:1267–87. doi: 10.1074/jbc.M116.739839
33. Virtue AT, McCright SJ, Wright JM, Jimenez MT, Mowel WK, Kotzin JJ, et al. The gut microbiota regulates white adipose tissue inflammation and obesity via a family of microRNAs. Sci Transl Med (2019) 11:1–13. doi: 10.1126/scitranslmed.aav1892
34. Williams MD, Mitchell GM. MicroRNAs in insulin resistance and obesity. Exp Diabetes Res (2012) 2012:1–9. doi: 10.1155/2012/484696
35. Jazwa A, Kasper L, Bak M, Sobczak M, Szade K, Jozkowicz A, et al. Differential Inflammatory MicroRNA and Cytokine Expression in Pulmonary Sarcoidosis. Arch Immunol Ther Exp (Warsz) (2015) 63:139–46. doi: 10.1007/s00005-014-0315-9
36. Lu J, Clark AG. Impact of microRNA regulation on variation in human geneexpression. Genome Res (2012) 7:1243–54. doi: 10.1101/gr.132514.111.22
37. Treiber T, Treiber N, Meister G. Regulation of microRNA biogenesis and its crosstalk with other cellular pathways. Nat Rev Mol Cell Biol (2019) 20:5–20. doi: 10.1038/s41580-018-0059-1
38. O’Brien J, Hayder H, Zayed Y, Peng C. Overview of microRNA biogenesis, mechanisms of actions, and circulation. Front Endocrinol (Lausanne) (2018) 9:402. doi: 10.3389/fendo.2018.00402
39. Rorbach G, Unold O, Konopka BM. Distinguishing mirtrons from canonical miRNAs with data exploration and machine learning methods. Sci Rep (2018) 8:1–13. doi: 10.1038/s41598-018-25578-3
40. Eichhorn SW, Guo H, McGeary SE, Rodriguez-Mias RA, Shin C, Baek D, et al. MRNA Destabilization Is the dominant effect of mammalian microRNAs by the time substantial repression ensues. Mol Cell (2014) 56:104–15. doi: 10.1016/j.molcel.2014.08.028
41. McGregor RA, Choi MS. microRNAs in the regulation of adipogenesis and obesity. Curr Mol Med (2011) 11:304–16. doi: 10.2174/156652411795677990
42. Mori MA, Thomou T, Boucher J, Lee KY, Lallukka S, Kim JK, et al. Altered miRNA processing disrupts brown/white adipocyte determination and associates with lipodystrophy. J Clin Invest (2014) 124:3339–51. doi: 10.1172/JCI73468
43. Fiorenza CG, Chou SH, Mantzoros CS. Lipodystrophy : Pathophysiology and advances intreatment. Nature Reviews Endocrinol (2011) 7:137–50. doi: 10.1038/nrendo.2010.199
44. Huang Y, Yan Y, Xv W, Qian G, Li C, Zou H. Li Y. A New Insight into the Roles of MiRNAs in Metabolic Syndrome. BioMed Res Int (2018) 2018:1–15. doi: 10.1155/2018/7372636
45. Zhong H, Ma M, Liang T, Guo L. Role of MicroRNAs in Obesity-Induced Metabolic Disorder and Immune Response. J Immunol Res (2018) 2018:1–8. doi: 10.1155/2018/2835761
46. Ji C, Guo X. The clinical potential of circulating microRNAs in obesity. Nat Rev Endocrinol (2019) 15:731–43. doi: 10.1038/s41574-019-0260-0
47. Castaño C, Kalko S, Novials A, Párrizas M. Obesity-associated exosomal miRNAs modulate glucose and lipid metabolism in mice. Proc Natl Acad Sci (2018) 115:12158–63. doi: 10.1073/pnas.1808855115
48. Sun L, Xie H, Mori MA, Alexander R, Yuan B, Hattangadi SM, et al. MiR-193b-365, a brown fat enriched microRNA cluster, is essential for brown fat differentiation. Nat Cell Biol (2012) 13:958–65. doi: 10.1038/ncb2286.MiR-193b-365
49. Mori M, Nakagami H, Rodriguez-Araujo G, Nimura K, Kaneda Y. Essential Role for miR-196a in Brown Adipogenesis of White Fat Progenitor Cells. PloS Biol (2012) 10:e1001314. doi: 10.1371/journal.pbio.1001314
50. Xie H, Sun L, Lodish HF. Targeting microRNAs in obesity. Expert Opin Ther Targets (2009) 13:1227–38. doi: 10.1517/14728220903190707
51. Trajkovski M, Hausser J, Soutschek J, Bhat B, Akin A, Zavolan M, et al. MicroRNAs 103 and 107 regulate insulin sensitivity. Nature (2011) 474:649–53. doi: 10.1038/nature10112
52. Yin H, Pasut A, Soleimani VD, Bentzinger CF, Thorn S, Seale P, et al. MicroRNA-133 controls brown adipose determination in skeletal muscle satellite cells by targeting Prdm16. Cell Metab (2014) 17:210–24. doi: 10.1016/j.cmet.2013.01.004.MicroRNA-133
53. Liu W, Bi P, Shan T, Yang X, Yin H, Wang Y-X, et al. miR-133a Regulates Adipocyte Browning In Vivo. PloS Genet (2013) 9:e1003626. doi: 10.1371/journal.pgen.1003626
54. Zhang Z, Liu S, Shi R, Zhao G. MiR-27 promotes human gastric cancer cell metastasis by inducing epithelial-to-mesenchymal transition. Cancer Genet (2011) 204:486–91. doi: 10.1016/j.cancergen.2011.07.004
55. Sun L, Trajkovski M. MiR-27 orchestrates the transcriptional regulation of brown adipogenesis. Metabolism (2014) 63:272–82. doi: 10.1016/j.metabol.2013.10.004
56. Karbiener M, Pisani DF, Frontini A, Oberreiter LM, Lang E, Vegiopoulos A, et al. MicroRNA-26 family is required for human adipogenesis and drives characteristics of brown adipocytes. Stem Cells (2014) 32:1578–90. doi: 10.1002/stem.1603
57. Xu G, Ji C, Song G, Zhao C, Shi C, Song L, et al. MiR-26b modulates insulin sensitivity in adipocytes by interrupting the PTEN/PI3K/AKT pathway. Int J Obes (2015) 39:1523–30. doi: 10.1038/ijo.2015.95
58. Jordan SD, Krüger M, Willmes DM, Redemann N, Wunderlich FT, Brönneke HS, et al. Obesity-induced overexpression of miRNA-143 inhibits insulin-stimulated AKT activation and impairs glucose metabolism. Nat Cell Biol (2011) 13:434–48. doi: 10.1038/ncb2211
59. Cho WC, Chow AS, Au JS. MiR-145 inhibits cell proliferation of human lung adenocarcinoma by targeting EGFR and NUDT1. RNA Biol (2011) 8:125–31. doi: 10.4161/rna.8.1.14259
60. Fu T, Seok S, Choi S, Huang Z, Suino-Powell K, Xu HE, et al. MicroRNA 34a Inhibits Beige and Brown Fat Formation in Obesity in Part by Suppressing Adipocyte Fibroblast Growth Factor 21 Signaling and SIRT1 Function. Mol Cell Biol (2014) 34:4130–42. doi: 10.1128/MCB.00596-14
61. Li L, Yuan L, Luo J, Gao J, Guo J, Xie X. MiR-34a inhibits proliferation and migration of breast cancer through down-regulation of Bcl-2 and SIRT1. Clin Exp Med (2013) 13:109–17. doi: 10.1007/s10238-012-0186-5
62. Zhang H, Guan M, Townsend KL, Huang TL, An D, Yan X, et al. MicroRNA-455 regulates brown adipogenesis via a novel HIF1an-AMPK-PGC1 signaling network. EMBO Rep (2015) 16:1378–93. doi: 10.15252/embr.201540837
63. Chistiakov DA, Sobenin IA, Orekhov AN, Bobryshev YV. Human miR-221/222 in physiological and atherosclerotic vascularremodeling. BioMed Res Int (2015) 2015:1–19. doi: 10.1155/2015/354517
64. Shah MY, Calin GA. MicroRNAs miR-221 and miR-222: A new level of regulation inaggressive breast cancer. Genome Med (2011) 3:1–4. doi: 10.1186/gm272
65. Duroux-Richard I, Roubert C, Ammari M, Présumey J, Grün JR, Häupl T, et al. MiR-125b controls monocyte adaptation to inflammation through mitochondrial metabolism and dynamics. Blood (2016) 128:3125–36. doi: 10.1182/blood-2016-02-697003
66. Giroud M, Pisani DF, Karbiener M, Barquissau V, Ghandour RA, Tews D, et al. miR-125b affects mitochondrial biogenesis and impairs brite adipocyte formation and function. Mol Metab (2016) 5:615–25. doi: 10.1016/j.molmet.2016.06.005
67. Ling HY, Ou HS, Feng SD, Zhang XY, Tuo QH, Chen LX, et al. Changes in microrna (mir) profile and effects of mir-320 in insulin-resistant 3t3-l1 adipocytes. Clin Exp Pharmacol Physiol (2009) 36:32–9. doi: 10.1111/j.1440-1681.2009.05207.x
68. Najafi-Shoushtari SH, Kristo F, Li Y, Shioda T, Cohen DE, Gerszten RE, et al. MicroRNA-33 and the SREBP Host Genes Cooperate to Control Cholesterol Homeostasis. Sci (80- ) (2010) 328:233–43. doi: 10.1016/j.devcel.2010.12.007.Peroxiredoxin
69. Poy MN, Hausser J, Trajkovski M, Braun M, Collins S, Rorsman P, et al. miR-375 maintains normal pancreatic alpha- and beta-cell mass. Proc Natl Acad Sci (2009) 106:5813–8. doi: 10.1073/pnas.0810550106
70. Peng J, Wu Y, Deng Z, Zhou Y, Song T, Yang Y, et al. MiR-377 promotes white adipose tissue inflammation and decreases insulin sensitivity in obesity via suppression of sirtuin-1 (SIRT1). Oncotarget (2017) 8:70550–63. doi: 10.18632/oncotarget.19742
71. Jeong Kim Y, Jin Hwang S, Chan Bae Y, Sup Jung J. MiR-21 regulates adipogenic differentiation through the modulation of TGF-β signaling in mesenchymal stem cells derived from human adipose tissue. Stem Cells (2009) 27:3093–102. doi: 10.1002/stem.235
72. Wu Y, Song Y, Xiong Y, Wang X, Xu K, Han B, et al. MicroRNA-21 (Mir-21) Promotes Cell Growth and Invasion by Repressing Tumor Suppressor PTEN in Colorectal Cancer. Cell Physiol Biochem (2017) 43:945–58. doi: 10.1159/000481648
73. Bruning U, Cerone L, Neufeld Z, Fitzpatrick SF, Cheong A, Scholz CC, et al. MicroRNA-155 Promotes Resolution of Hypoxia-Inducible Factor 1 Activity during Prolonged Hypoxia. Mol Cell Biol (2011) 31:4087–96. doi: 10.1128/mcb.01276-10
74. Babar IA, Czochor J, Steinmetz A, Weidhaas JB, Glazer PM, Slack FJ. Inhibition of hypoxia-induced miR-155 radiosensitizes hypoxic lung cancer cells. Cancer Biol Ther (2011) 12:908–14. doi: 10.4161/cbt.12.10.17681
75. Karbiener M, Scheideler M. MicroRNA functions in brite/brown fat - Novel perspectives towards anti-obesity strategies. Comput Struct Biotechnol J (2014) 11:101–5. doi: 10.1016/j.csbj.2014.09.005
76. Lee Y-H, Mottillo EP, Granneman JG. Adipose tissue plasticity from WAT to BAT and in between. Biochim Biophys Acta Mol Basis Dis (2014) 1842:358–69. doi: 10.1016/j.bbadis.2013.05.011
77. Brandão BB, Guerra BA, Mori MA. Shortcuts to a functional adipose tissue: The role of small non-coding RNAs. Redox Biol (2017) 12:82–102. doi: 10.1016/j.redox.2017.01.020
78. Kim H-J, Cho H, Alexander R, Patterson HC, Gu M, Lo KA, et al. MicroRNAs Are Required for the Feature Maintenance and Differentiation of Brown Adipocytes. Diabetes (2014) 63:4045–56. doi: 10.2337/db14-0466
79. Cypess AM, Kahn CR. Brown fat as a therapy for obesity and diabetes. Curr Opin Endocrinol Diabetes Obes (2010) 17:143–9. doi: 10.1097/MED.0b013e328337a81f.Brown
80. Zuriaga MA, Fuster JJ, Gokce N, Walsh K. Humans and Mice Display Opposing Patterns of “Browning” Gene Expression in Visceral and Subcutaneous White Adipose Tissue Depots. Front Cardiovasc Med (2017) 4:27. doi: 10.3389/fcvm.2017.00027
81. Li M, Liu Z, Zhang Z, Liu G, Sun S, Sun C. miR-103 promotes 3T3-L1 cell adipogenesis through AKT/mTOR signal pathway with its target being MEF2D. Biol Chem (2015) 396:235–44. doi: 10.1515/hsz-2014-0241
82. Zhang Z, Wu S, Muhammad S, Ren Q, Sun C. miR-103/107 promote ER stress-mediated apoptosis via targeting the Wnt3a/β-catenin/ATF6 pathway in preadipocytes. J Lipid Res (2018) 59:843–53. doi: 10.1194/jlr.M082602
83. Trajkovski M, Ahmed K, Esau CC, Stoffel M. MyomiR-133 regulates brown fat differentiation through Prdm16. Nat Cell Biol (2012) 14:1330–5. doi: 10.1038/ncb2612
84. Chen Y, Siegel F, Kipschull S, Haas B, Fröhlich H, Meister G, et al. miR-155 regulates differentiation of brown and beige adipocytes via a bistable circuit. Nat Commun (2013) 4:1769. doi: 10.1038/ncomms2742
85. Lin Q, Gao Z, Alarcon RM, Ye J, Yun Z. A role of miR-27 in the regulation of adipogenesis. FEBS J (2009) 276:2348–58. doi: 10.1111/j.1742-4658.2009.06967.x
86. Zhu Y, Zhang X, Ding X, Wang H, Chen X, Zhao H, et al. miR-27 inhibits adipocyte differentiation via suppressing CREB expression. Acta Biochim Biophys Sin (Shanghai) (2014) 46:590–6. doi: 10.1093/abbs/gmu036
87. Chen S-Z, Xu X, Ning L-F, Jiang W-Y, Xing C, Tang Q-Q, et al. miR-27 impairs the adipogenic lineage commitment via targeting lysyl oxidase. Obesity (2015) 23:2445–53. doi: 10.1002/oby.21319
88. Kang T, Lu W, Xu W, Anderson L, Bacanamwo M, Thompson W, et al. MicroRNA-27 (miR-27) Targets Prohibitin and Impairs Adipocyte Differentiation and Mitochondrial Function in Human Adipose-derived Stem Cells. J Biol Chem (2013) 288:34394–402. doi: 10.1074/jbc.M113.514372
89. Li G, Ning C, Ma Y, Jin L, Tang Q, Li X, et al. miR-26b Promotes 3T3-L1 Adipocyte Differentiation Through Targeting PTEN. DNA Cell Biol (2017) 36:672–81. doi: 10.1089/dna.2017.3712
90. Lee EK, Lee MJ, Abdelmohsen K, Kim W, Kim MM, Srikantan S, et al. miR-130 Suppresses Adipogenesis by Inhibiting Peroxisome Proliferator-Activated Receptor Expression. Mol Cell Biol (2011) 31:626–38. doi: 10.1128/MCB.00894-10
91. Wankhade UD, Shen M, Yadav H, Thakali KM. Novel Browning Agents, Mechanisms, and Therapeutic Potentials ofBrown Adipose Tissue. BioMed Res Int (2016) 2016:1–16. doi: 10.1155/2016/2365609
92. Walden TB, Timmons JA, Keller P, Nedergaard J, Cannon B. Distinct expression of muscle-specific microRNAs (myomirs) in brown adipocytes. J Cell Physiol (2009) 218:444–9. doi: 10.1002/jcp.21621
93. Kong L, Zhu J, Han W, Jiang X, Xu M, Zhao Y, et al. Significance of serum microRNAs in pre-diabetes and newly diagnosed type 2 diabetes: A clinical study. Acta Diabetol (2011) 48:61–9. doi: 10.1007/s00592-010-0226-0
94. Shamsi F, Zhang H, Tseng YH. MicroRNA regulation of brown adipogenesis and thermogenic energy expenditure. Front Endocrinol (Lausanne) (2017) 8:205. doi: 10.3389/fendo.2017.00205
95. Arner E, Mejhert N, Kulyté A, Balwierz PJ, Pachkov M, Cormont M, et al. Adipose tissue MicroRNAs as regulators of CCL2 production in human obesity. Diabetes (2012) 61:1986–93. doi: 10.2337/db11-1508
96. Yu J, Kong X, Liu J, Lv Y, Sheng Y, Lv S, et al. Expression profiling of PPARγ-regulated MicroRNAs in human subcutaneous and visceral adipogenesis in both genders. Endocrinology (2014) 155:2155–65. doi: 10.1210/en.2013-2105
97. Ortega FJ, Mercader JM, Catalán V, Moreno-Navarrete JM, Pueyo N, Sabater M, et al. Targeting the circulating microRNA signature of obesity. Clin Chem (2013) 59:781–92. doi: 10.1373/clinchem.2012.195776
98. Dahlman I, Belarbi Y, Laurencikiene J, Pettersson AM, Arner P, Kulyté A. Comprehensive functional screening of miRNAs involved in fat cell insulin sensitivity among women. Am J Physiol Endocrinol Metab (2017) 312:E482–94. doi: 10.1152/ajpendo.00251.2016
99. Landrier J-F, Derghal A, Mounien L. MicroRNAs in Obesity and Related Metabolic Disorders. Cells (2019) 8:859. doi: 10.3390/cells8080859
100. Tan W, Liu B, Qu S, Liang G, Luo W, Gong C. MicroRNAs and cancer: Key paradigms in molecular therapy (Review). Oncol Lett (2018) 15:2735–42. doi: 10.3892/ol.2017.7638
101. Alberti KGM, Zimmet P, Shaw J. The metabolic syndrome—a new worldwide definition. Lancet (2005) 366:1059–62. doi: 10.1016/S0140-6736(05)67402-8
102. Grundy SM, Cleeman JI, Daniels SR, Donato KA, Eckel RH, Franklin BA, et al. Diagnosis and Management of the Metabolic Syndrome. Circulation (2005) 112:2735–52. doi: 10.1161/CIRCULATIONAHA.105.169404
103. Deiuliis JA. MicroRNAs as regulators of metabolic disease: pathophysiologic significance and emerging role as biomarkers and therapeutics. Int J Obes (2016) 40:88–101. doi: 10.1038/ijo.2015.170
104. Huang C, Liu XJ, QunZhou, Xie J, Ma TT, Meng XM, et al. MiR-146a modulates macrophage polarization by inhibiting Notch1 pathway in RAW264.7 macrophages. Int Immunopharmacol (2016) 32:46–54. doi: 10.1016/j.intimp.2016.01.009
105. Alexander R, Lodish H, Sun L. MicroRNAs in adipogenesis and as therapeutic targets forobesity. Expert Opin Ther Targets (2011) 5:623–36. doi: 10.1517/14728222.2011.561317
106. Belgardt BF, Ahmed K, Spranger M, Latreille M, Denzler R, Kondratiuk N, et al. The microRNA-200 family regulates pancreatic beta cell survival in type 2 diabetes. Nat Med (2015) 21:619–27. doi: 10.1038/nm.3862
107. Latreille M, Hausser J, Stützer I, Zhang Q, Hastoy B, Gargani S, et al. MicroRNA-7a regulates pancreatic β cell function. J Clin Invest (2014) 124:2722–35. doi: 10.1172/JCI73066
108. Zhuang G, Meng C, Guo X, Cheruku PS, Shi L, Xu H, et al. A novel regulator of macrophage activation: MiR-223 in obesity-associated adipose tissue inflammation. Circulation (2012) 125:2892–903. doi: 10.1161/CIRCULATIONAHA.111.087817
109. Colangelo T, Fucci A, Votino C, Sabatino L, Pancione M, Laudanna C, et al. MicroRNA-130b Promotes Tumor Development and Is Associated with Poor Prognosis in Colorectal Cancer. Neoplasia (2013) 15:1086–99. doi: 10.1593/neo.13998
110. Lee J, Padhye A, Sharma A, Song G, Miao J, Mo Y-Y, et al. A Pathway Involving Farnesoid X Receptor and Small Heterodimer Partner Positively Regulates Hepatic Sirtuin 1 Levels via MicroRNA-34a Inhibition. J Biol Chem (2010) 285:12604–11. doi: 10.1074/jbc.M109.094524
111. Kemper JK, Sharma A, Lee J, Padhye A, Mo Y-Y, Miao J, et al. A Pathway Involving Farnesoid X Receptor and Small Heterodimer Partner Positively Regulates Hepatic Sirtuin 1 Levels via MicroRNA-34a Inhibition. J Biol Chem (2010) 285:12604–11. doi: 10.1074/jbc.m109.094524
112. Choi S, Fu T, Seok S, Kim D, Yu E, Lee K, et al. Elevated microRNA-34a in obesity reduces NAD+ levels and SIRT1 activity by directly targeting NAMPT. Aging Cell (2013) 12:1062–72. doi: 10.1111/acel.12135
113. Karbiener M, Neuhold C, Opriessnig P, Prokesch A, Bogner-strauss JG, Scheideler M, et al. MicroRNA-30c promotes human adipocyte differentiation and co-represses PAI-1 and ALK2. RNA Biol (2011) 8:850–60. doi: 10.4161/rna.8.5.16153
114. Jung HJ, Suh Y. Regulation of IGF -1 signaling by microRNAs. Front Genet (2015) 5:472. doi: 10.3389/fgene.2014.00472
115. Esteves JV, Enguita FJ, Machado UF. MicroRNAs-Mediated Regulation of Skeletal Muscle GLUT4 Expressionand Translocation in Insulin Resistance. J Diabetes Res (2017) 2017:1–12. doi: 10.1155/2017/7267910
116. Qin J, Li R, Raes J, Arumugam M, Burgdorf KS, Manichanh C, et al. A human gut microbial gene catalogue established by metagenomic sequencing. Nature (2010) 464:59–65. doi: 10.1038/nature08821
117. LaPierre MP, Stoffel M. MicroRNAs as stress regulators in pancreatic beta cells and diabetes. Mol Metab (2017) 6:1010–23. doi: 10.1016/j.molmet.2017.06.020
118. Ling H, Li X, Yao CH, Hu B, Liao D, Feng S, et al. The physiological and pathophysiological roles of adipocyte miRNAs. Biochem Cell Biol (2013) 91:195–202. doi: 10.1139/bcb-2012-0053
119. Chen YH, Heneidi S, Lee JM, Layman LC, Stepp DW, Gamboa GM, et al. Mirna-93 inhibits glut4 and is overexpressed in adipose tissue of polycystic ovary syndrome patients and women with insulin resistance. Diabetes (2013) 62:2278–86. doi: 10.2337/db12-0963
120. El Ouaamari A, Baroukh N, Martens GA, Lebrun P, Pipeleers D, van Obberghen E. miR-375 targets 3’-phosphoinositide-dependent protein kinase-1 and regulated glucose-induced biological responses in pancreatic beta-cells. Diabetes (2008) 57:2708–17. doi: 10.2337/db07-1614
121. Song S, Zhou J, He S, Zhu D, Zhang Z, Zhao H, et al. Expression levels of microRNA-375 in pancreatic cancer. BioMed Rep (2013) 1:393–8. doi: 10.3892/br.2013.88
122. Chen Y, Zhang L, Liu W, Wang K. Case-control study of metabolic syndrome and ovarian cancer in Chinese population. Nutr Metab (Lond) (2017) 14:21. doi: 10.1186/s12986-017-0176-4
123. Esposito K, Chiodini P, Colao A, Lenzi A, Giugliano D. Metabolic Syndrome and Risk of Cancer: A systematic review and meta-analysis. Diabetes Care (2012) 35:2402–11. doi: 10.2337/dc12-0336
124. Montella M, Di Maso M, Crispo A, Grimaldi M, Bosetti C, Turati F, et al. Metabolic syndrome and the risk of urothelial carcinoma of the bladder: a case-control study. BMC Cancer (2015) 15:720. doi: 10.1186/s12885-015-1769-9
125. Esposito K, Chiodini P, Capuano A, Bellastella G, Maiorino MI, Parretta E, et al. Effect of metabolic syndrome and its components on prostate cancer risk: Meta-analysis. J Endocrinol Invest (2013) 36:132–9. doi: 10.1007/BF03346748
126. Li C, Liu P, Liu L, Zhang X, Yang P, Sheng H, et al. Metabolic syndrome in hematologic malignancies survivors: a meta-analysis. Med Oncol (2015) 32:422. doi: 10.1007/s12032-014-0422-9
127. Bhaskaran K, Douglas I, Forbes H, Dos-Santos-Silva I, Leon DA, Smeeth L. Body-mass index and risk of 22 specific cancers: a population-based cohort study of 5·24 million UK adults. Lancet (2014) 384:755–65. doi: 10.1016/S0140-6736(14)60892-8
128. Hanahan D, Weinberg R. The Hallmarks of Cancer. Cell (2000) 100:57–70. doi: 10.1016/S0092-8674(00)81683-9
129. Hanahan D, Weinberg RA. Hallmarks of cancer: The next generation. Cell (2011) 144:646–74. doi: 10.1016/j.cell.2011.02.013
130. Withers SB, Dewhurst T, Hammond C, Topham CH. MiRNAs as novel adipokines: Obesity-related circulating MiRNAsinfluence chemosensitivity in cancer patients. Non Cod RNA (2020) 6:1–29. doi: 10.3390/ncrna6010005
131. Turati F, Tramacere I, La Vecchia C, Negri E. A meta-analysis of body mass index and esophageal and gastric cardia adenocarcinoma. Ann Oncol (2013) 24:609–17. doi: 10.1093/annonc/mds244
132. Yang P, Zhou Y, Chen B, Wan HW, Jia GQ, Bai HL, et al. Overweight, obesity and gastric cancer risk: Results from a meta-analysis of cohort studies. Eur J Cancer (2009) 45:2867–73. doi: 10.1016/j.ejca.2009.04.019
133. Liu PH, Wu K, Ng K, Zauber AG, Nguyen LH, Song M, et al. Association of Obesity with Risk of Early-Onset Colorectal Cancer among Women. JAMA Oncol (2019) 5:37–44. doi: 10.1001/jamaoncol.2018.4280
134. Larsson SC, Wolk A. Overweight, obesity and risk of liver cancer: A meta-analysis of cohort studies. Br J Cancer (2007) 97:1005–8. doi: 10.1038/sj.bjc.6603932
135. Lavalette C, Cénée S, Rebillard X, Lamy P-J, Trétarre B, Menegaux F. Abdominal obesity and prostate cancer risk: epidemiological evidence from the EPICAP study. Rev Epidemiol Sante Publique (2018) 66:S248. doi: 10.1016/j.respe.2018.05.040
136. Feng YH. The association between obesity and gynecological cancer. Gynecol Minim Invasive Ther (2015) 4:102–5. doi: 10.1016/j.gmit.2015.03.003
137. Liu X, Sun Q, Hou H, Zhu K, Wang Q, Liu H, et al. The association between BMI and kidney cancer risk. Med (Balt) (2018) 97:e12860. doi: 10.1097/md.0000000000012860
138. Shao C, Bai LP, Qi ZY, Hui GZ, Wang Z. Overweight, obesity and meningioma risk: Ameta-analysis. PloS One (2014) 9:1–7. doi: 10.1371/journal.pone.0090167
139. Zhao ZG, Guo XG, Ba CX, Wang W, Yang YY, Wang J, et al. Overweight, obesity and thyroid cancer risk: A meta-analysis of cohort studies. J Int Med Res (2012) 40:2041–50. doi: 10.1177/030006051204000601
140. Sergentanis TN, Zagouri F, Tsilimidos G, Tsagianni A, Tseliou M, Dimopoulos MA, et al. Risk Factors for Multiple Myeloma: A Systematic Review of Meta-Analyses. Clin Lymphoma Myeloma Leuk (2015) 15:563–577.e3. doi: 10.1016/j.clml.2015.06.003
141. Hotamisligil GS. Inflammation, metaflammation and immunometabolic disorders. Nature (2017) 542:177–85. doi: 10.1038/nature21363
142. Ogawa R, Tanaka C, Sato M, Nagasaki H, Sugimura K, Okumura K, et al. Adipocyte-derived microvesicles contain RNA that is transported into macrophages and might be secreted into blood circulation. Biochem Biophys Res Commun (2010) 398:723–9. doi: 10.1016/j.bbrc.2010.07.008
143. Peng Y, Croce CM. The role of MicroRNAs in human cancer. Signal Transduct Target Ther (2016) 1:15004. doi: 10.1038/sigtrans.2015.4
144. Svoronos AA, Engelman DM, Slack FJ. OncomiR or Tumor Suppressor? The Duplicity of MicroRNAs in Cancer. Cancer Res (2016) 76:3666–70. doi: 10.1158/0008-5472.CAN-16-0359
145. Liu T, Liu PY, Marshall GM. The critical role of the class III histone deacetylase SIRT1 in cancer. Cancer Res (2009) 69:1702–5. doi: 10.1158/0008-5472.CAN-08-3365
146. Chen J, Zhang B, Wong N, Lo AWI, To KF, Chan AWH, et al. Sirtuin 1 is upregulated in a subset of hepatocellular carcinomas where it is essential for telomere maintenance and tumor cell growth. Cancer Res (2011) 71:4138–49. doi: 10.1158/0008-5472.CAN-10-4274
147. Arcidiacono B, Iiritano S, Nocera A, Possidente K, Nevolo MT, Ventura V, et al. Insulin resistance and cancer risk: An overview of the pathogeneticmechanisms. Exp Diabetes Res (2012) 2012:1–13. doi: 10.1155/2012/789174
148. Chang T-C, Wentzel EA, Kent OA, Ramachandran K, Mullendore M, Lee KH, et al. Transactivation of miR-34a by p53 Broadly Influences Gene Expression and Promotes Apoptosis. Mol Cell (2007) 26:745–52. doi: 10.1016/j.molcel.2007.05.010
149. Muñoz-Fontela C, Mandinova A, Aaronson SA, Lee SW. Emerging roles of p53 and other tumour-suppressor genes in immune regulation. Nat Rev Immunol (2016) 16:741–50. doi: 10.1038/nri.2016.99
150. Wang B, Li D, Kovalchuk I, Apel IJ, Chinnaiyan AM, Wóycicki RK, et al. miR-34a directly targets tRNA i Met precursors and affects cellular proliferation, cell cycle, and apoptosis. Proc Natl Acad Sci (2018) 115:7392–7. doi: 10.1073/pnas.1703029115
151. Wang W, Liu Y, Guo J, He H, Mi X, Chen C, et al. miR-100 maintains phenotype of tumor-associated macrophages bytargeting mTOR to promote tumor metastasis via Stat5a/IL-1ra pathway in mouse breastcancer. Oncogenesis (2018) 7:1–17. doi: 10.1038/s41389-018-0106-y
152. Belarbi Y, Mejhert N, Lorente-Cebrián S, Dahlman I, Arner P, Rydén M, et al. MicroRNA-193b controls adiponectin production in human white adipose tissue. J Clin Endocrinol Metab (2015) 100:E1084–8. doi: 10.1210/jc.2015-1530
153. Ouchi N, Walsh K. Adiponectin as an anti-inflammatory factor. Clin Chim Acta (2009) 380:24–30. doi: 10.1016/j.cca.2007.01.026.Adiponectin
154. Ye J, Jia J, Dong S, Zhang C, Yu S, Li L, et al. Circulating adiponectin levels and the risk of breast cancer: A meta-analysis. Eur J Cancer Prev (2014) 23:158–65. doi: 10.1097/CEJ.0b013e328364f293
155. Zheng Q, Wu H, Cao J. Circulating adiponectin and risk of endometrial cancer. PloS One (2015) 10:1–11. doi: 10.1371/journal.pone.0129824
156. Chen C, Xiang H, Peng Y, Peng J, Jiang S. Mature miR-183, negatively regulated by transcription factor GATA3, promotes 3T3-L1 adipogenesis through inhibition of the canonical Wnt/β-catenin signaling pathway by targeting LRP6. Cell Signal (2014) 26:1155–65. doi: 10.1016/j.cellsig.2014.02.003
157. Donatelli SS, Zhou JM, Gilvary DL, Eksioglu EA, Chen X, Cress WD, et al. TGF-β-inducible microRNA-183 silences tumor-associated natural killer cells. Proc Natl Acad Sci USA (2014) 111:4203–8. doi: 10.1073/pnas.1319269111
158. Feng YH, Tsao CJ. Emerging role of microRNA-21 in cancer (Review). BioMed Rep (2016) 5:395–402. doi: 10.3892/br.2016.747
159. Ji W, Sun B, Su C. Targeting MicroRNAs in Cancer Gene Therapy. Genes (Basel) (2017) 8:1–15. doi: 10.3390/genes8010021
160. Cimmino A, Calin GA, Fabbri M, Iorio MV, Ferracin M, Shimizu M, et al. miR-15 and miR-16 induce apoptosis by targeting BCL2. Proc Natl Acad Sci USA (2005) 102:13944–9. doi: 10.1073/pnas.0506654102
161. Kang T, Jones TM, Naddell C, Bacanamwo M, Calvert JW, Thompson WE, et al. Adipose-Derived Stem Cells Induce Angiogenesis via Microvesicle Transport of miRNA-31. Stem Cells Transl Med (2016) 5:440–50. doi: 10.5966/sctm.2015-0177
162. Zong W, Rabinowitz JD, White E. Mitochondria and Cancer Wei-Xing. Mol Cell (2016) 61:667–76. doi: 10.1016/j.molcel.2016.02.011.Mitochondria
163. Warburg O. On respiratory impairment in cancer cells.Sci (80- ) (1956)124:269–70. doi: 10.1126/science.124.3215.267
164. Zheng J. Energy metabolism of cancer: Glycolysis versus oxidative phosphorylation (Review). Oncol Lett (2012) 4:1151–7. doi: 10.3892/ol.2012.928
165. Tomasetti M, Nocchi L, Staffolani S, Manzella N, Amati M, Goodwin J, et al. MicroRNA-126 Suppresses Mesothelioma Malignancy by Targeting IRS1 and Interfering with the Mitochondrial Function. Antioxid Redox Signal (2014) 21:2109–25. doi: 10.1089/ars.2013.5215
166. Togliatto G, Dentelli P, Gili M, Gallo S, Deregibus C, Biglieri E, et al. Obesity reduces the pro-angiogenic potential of adipose tissue stem cell-derived extracellular vesicles (EVs) by impairing miR-126 content: impact on clinical applications. Int J Obes (2016) 40:102–11. doi: 10.1038/ijo.2015.123
167. Duarte F, Palmeira C, Rolo A. The Role of microRNAs in Mitochondria: Small Players Acting Wide. Genes (Basel) (2014) 5:865–86. doi: 10.3390/genes5040865
168. Bousquet M, Harris MH, Zhou B, Lodish HF. MicroRNA miR-125b causes leukemia. Proc Natl Acad Sci (2010) 107:21558–63. doi: 10.1073/pnas.1016611107
169. Shaham L, Binder V, Gefen N, Borkhardt A, Izraeli S. MiR-125 in normal and malignant hematopoiesis. Leukemia (2012) 26:2011–8. doi: 10.1038/leu.2012.90
170. Sun Y-M, Lin K-Y, Chen Y-Q. Diverse functions of miR-125 family in different cell contexts. J Hematol Oncol (2013) 6:6. doi: 10.1186/1756-8722-6-6
171. Hirono T, Jingushi K, Nagata T, Sato M, Minami K, Aoki M, et al. MicroRNA-130b functions as an oncomiRNA in non-small cell lung cancer by targeting tissue inhibitor of metalloproteinase-2. Sci Rep (2019) 9:6956. doi: 10.1038/s41598-019-43355-8
172. Lu J, Imamura K, Nomura S, Mafune KI, Nakajima A, Kadowaki T, et al. Chemopreventive effect of peroxisome proliferator-activated receptor γ on gastric carcinogenesis in mice. Cancer Res (2005) 65:4769–74. doi: 10.1158/0008-5472.CAN-04-2293
173. Egawa H, Jingushi K, Hirono T, Hirose R, Nakatsuji Y, Ueda Y, et al. Pharmacological regulation of bladder cancer by miR-130 familyseed-targeting LNA. Integr Mol Med (2016) 3:1–7. doi: 10.15761/IMM.1000187
174. Peng Z, Duan F, Yin J, Feng Y, Yang Z, Shang J. Prognostic values of microRNA-130 family expression in patients with cancer: a meta-analysis and database test. J Transl Med (2019) 17:347. doi: 10.1186/s12967-019-2093-y
175. Hulin JA, Tommasi S, Elliot D, Hu DG, Lewis BC, Mangoni AA. MiR-193b regulates breast cancer cell migration and vasculogenic mimicry by targeting dimethylarginine dimethylaminohydrolase. Sci Rep (2017) 7:1–15. doi: 10.1038/s41598-017-14454-1
176. Chou N-H, Lo Y-H, Wang K-C, Kang C-H, Tsai C-Y, Tsai K-W. MiR-193a-5p and -3p Play a Distinct Role in Gastric Cancer: miR-193a-3p Suppresses Gastric Cancer Cell Growth by Targeting ETS1 and CCND1. Anticancer Res (2018) 38:3309–18. doi: 10.21873/anticanres.12596
177. Yuan F, Liu J, Pang H, Tian Y, Yuan K, Li Y, et al. MicroRNA-365 suppressed cell proliferation and migration viatargeting PAX6 in glioblastoma. Am J Transl Res (2019)11:361–9. 1943-8141/AJTR0082995
178. Zhu Y, Zhao H, Rao M, Xu S. MicroRNA-365 inhibits proliferation, migration and invasion of glioma by targeting PIK3R3. Oncol Rep (2017) 37:2185–92. doi: 10.3892/or.2017.5458
179. Bai J, Zhang Z, Li X, Liu H. MicroRNA-365 inhibits growth, invasion and metastasis of malignant melanoma by targeting NRP1 expression. Cancer Biomarkers (2015) 15:599–608. doi: 10.3233/CBM-150500
180. He M, Wu N, Leong MC, Zhang W, Ye Z, Li R, et al. miR-145 improves metabolic inflammatory disease through multiple pathways. J Mol Cell Biol (2020) 12:152–62. doi: 10.1093/jmcb/mjz015
181. Wang F, Xia J, Wang N, Zong H. miR-145 Inhibits Proliferation and Invasion of Esophageal Squamous Cell Carcinoma in Part by Targeting c-Myc. Oncol Res Treat (2013) 36:8–8. doi: 10.1159/000356978
182. Wang J, Sun Z, Yan S, Gao F. Effect of miR−145 on gastric cancer cells. Mol Med Rep (2019) 5:3403–10. doi: 10.3892/mmr.2019.10015
183. Karkeni E, Astier J, Tourniaire F, El Abed M, Romier B, Gouranton E, et al. Obesity-associated inflammation induces microRNA-155 expression in adipocytes and adipose tissue: Outcome on adipocyte function. J Clin Endocrinol Metab (2016) 101:1615–26. doi: 10.1210/jc.2015-3410
184. Bayraktar R, Van Roosbroeck K. miR-155 in cancer drug resistance and as target for miRNA-based therapeutics. Cancer Metastasis Rev (2018) 37:33–44. doi: 10.1007/s10555-017-9724-7
185. Chabot S, Orio J, Castanier R, Bellard E, Nielsen SJ, Golzio M, et al. LNA-based oligonucleotide electrotransfer for miRNA inhibition. Mol Ther (2012) 20:1590–8. doi: 10.1038/mt.2012.95
186. Krützfeldt J, Rajewsky N, Braich R, Rajeev KG, Tuschl T, Manoharan M, et al. Silencing of microRNAs in vivo with “antagomirs.” Nature (2005) 438:685–9. doi: 10.1038/nature04303
187. Beg MS, Brenner AJ, Sachdev J, Borad M, Kang YK, Stoudemire J, et al. Phase I study of MRX34, a liposomal miR-34a mimic, administered twice weekly in patients with advanced solid tumors. Invest New Drugs (2017) 35:180–8. doi: 10.1007/s10637-016-0407-y
188. Hanna J, Hossain GS, Kocerha J. The potential for microRNA therapeutics and clinicalresearch. Front Genet (2019) 10:1–6. doi: 10.3389/fgene.2019.00478
Keywords: miRNA, adipose tissue, obesity, cancer, metabolic syndrome
Citation: Heyn GS, Corrêa LH and Magalhães KG (2020) The Impact of Adipose Tissue–Derived miRNAs in Metabolic Syndrome, Obesity, and Cancer. Front. Endocrinol. 11:563816. doi: 10.3389/fendo.2020.563816
Received: 19 May 2020; Accepted: 18 September 2020;
Published: 06 October 2020.
Edited by:
Hendrik Lehnert, University of Lübeck, GermanyReviewed by:
Juergen Eckel, German Diabetes Center (DDZ), GermanyMarc Thibonnier, AptamiR Therapeutics, Inc., United States
Copyright © 2020 Heyn, Corrêa and Magalhães. This is an open-access article distributed under the terms of the Creative Commons Attribution License (CC BY). The use, distribution or reproduction in other forums is permitted, provided the original author(s) and the copyright owner(s) are credited and that the original publication in this journal is cited, in accordance with accepted academic practice. No use, distribution or reproduction is permitted which does not comply with these terms.
*Correspondence: Kelly Grace Magalhães, a2VsbHltYWdhbGhhZXNAdW5iLmJy