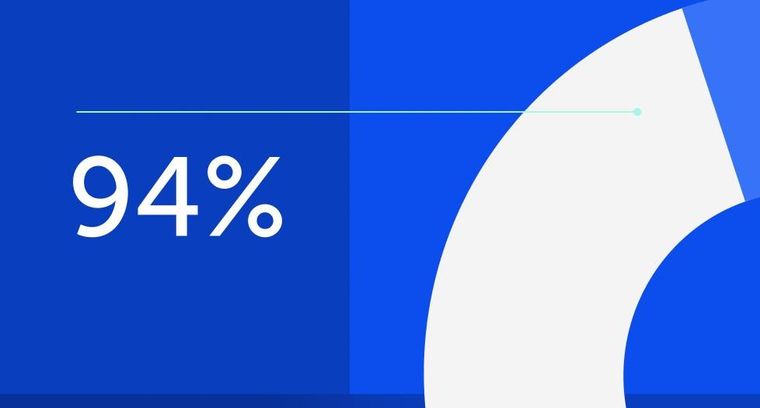
94% of researchers rate our articles as excellent or good
Learn more about the work of our research integrity team to safeguard the quality of each article we publish.
Find out more
REVIEW article
Front. Endocrinol., 18 February 2019
Sec. Molecular and Structural Endocrinology
Volume 10 - 2019 | https://doi.org/10.3389/fendo.2019.00053
This article is part of the Research TopicReceptor Heterodimerization: an Underappreciated Confounding Factor in Health and Disease?View all 4 articles
The discovery of receptor-receptor interactions (RRI) has expanded our understanding of the role that G protein-coupled receptors (GPCRs) play in intercellular communication. The finding that GPCRs can operate as receptor complexes, and not only as monomers, suggests that several different incoming signals could already be integrated at the plasma membrane level via direct allosteric interactions between the protomers that form the complex. Most research in this field has focused on neuronal populations and has led to the identification of a large number of RRI. However, RRI have been seen to occur not only in neurons but also in astrocytes and, outside the central nervous system, in cells of the cardiovascular and endocrine systems and in cancer cells. Furthermore, RRI involving the formation of macromolecular complexes are not limited to GPCRs, being also observed in other families of receptors. Thus, RRI appear as a widespread phenomenon and oligomerization as a common mechanism for receptor function and regulation. The discovery of these macromolecular assemblies may well have a major impact on pharmacology. Indeed, the formation of receptor complexes significantly broadens the spectrum of mechanisms available to receptors for recognition and signaling, which may be implemented through modulation of the binding sites of the adjacent protomers and of their signal transduction features. In this context, the possible appearance of novel allosteric sites in the receptor complex structure may be of particular relevance. Thus, the existence of RRI offers the possibility of new therapeutic approaches, and novel pharmacological strategies for disease treatment have already been proposed. Several challenges, however, remain. These include the accurate characterization of the role that the receptor complexes identified so far play in pathological conditions and the development of ligands specific to given receptor complexes, in order to efficiently exploit the pharmacological properties of these complexes.
The concept of “receptor” was independently proposed by Ehrlich and Langley (1) at the beginning of the 20th century to explain the selective effects of drugs and suggested that the action of a drug involved the formation of specific complexes with molecular agents in the target cells, thereby eliciting a cell response. In the decades that followed, this hypothesis was demonstrated, receptor molecules were biochemically identified, and their structures discovered, thus enabling the key role that they play in physiology to be fully understood. More than 4% of the human genome encodes cell receptors (2); these are organized into different families [see (3)] including matrix receptors (e.g., integrins), ligand-gated (LGIC, 76 members in the human genome) and voltage-gated (VGIC, 143 members) ion channels, intracellular receptors, such as nuclear hormone receptors (NHRs, 48 members), enzyme-linked receptors, such as receptor tyrosine kinases (RTKs, 58 members), and G protein-coupled receptors (GPCRs). GPCRs constitute the largest family; in mammals, they contribute to almost all physiological processes and are currently very common targets for drugs (2, 4). In humans, the GPCR family is made up of about 800 receptors; these are classified in five major groups, namely classes A (the largest group), B, C, frizzled, and adhesion (5), mainly on the basis of their structural and functional similarities (6). GPCRs have a highly conserved overall structure [see (7, 8)], exhibiting seven α-helixes that span the plasma membrane (transmembrane domains, TM) and are connected to one another by extra- and intracellular loops (ECL and ICL). The stability of the TM region is provided by interhelical bonds and hydrophobic interactions between highly conserved residues. The extracellular domain (encompassing the N-terminus of the protein) displays high structural variability among the different classes of GPCRs, being very large in class C GPCRs (9). In several GPCRs (e.g., class C GPCRs) it is the domain that hosts the ligand-binding site, while in others (e.g., most of class A GPCRs) the ligand-binding pocket is positioned in the extracellular half of the TM bundle (10). When ligand binding occurs, it induces a conformational change of the TM core, allowing the activation of downstream signaling pathways. In vitro and in vivo experiments have demonstrated that GPCRs can recognize and decode signals (of chemical or physical nature) as monomers. On this issue, studies of particular interest have shown that monomers of three class A GPCRs (namely rhodopsin, β2-adrenergic, and μ-opioid receptors) trapped inside nanodiscs are able to signal (11–13). In addition, intrinsic plasticity has been found to characterize signaling from GPCR monomers, in that they can assume multiple active conformations because of their binding with ligands, thereby initiating different patterns of signal transduction [see (14)], such as G protein and/or arrestin pathways (15).
However, evidence of negative cooperativity between β-adrenergic receptors has also emerged (16) and in the 1980 s in vitro and in vivo experiments by Agnati et al. (17, 18) and Fuxe et al. (19) provided indirect biochemical and functional evidence that structural receptor-receptor interactions (RRI) could be established between GPCR monomers [see (20) for further historical details]. These findings led to the hypothesis that supramolecular complexes of receptors consisting of different types of GPCRs could form at the cell membrane and could modulate synaptic weight (21), probably affecting learning and memory processes (22). It was also suggested that receptor–receptor interactions could allow the integration of synaptic (wiring transmission) and extrasynaptic (volume transmission) signals (23), one of the mechanisms underlying the appearance of polymorphic networks [see (24)]. The term RRI was subsequently proposed in order to emphasize the concept of an interaction between receptor proteins that required direct physical contact between the receptors and which led to the formation of dimers or high-order oligomers at the cell membrane. The first observations indicating the dimerization of GPCRs were made by Fraser and Venter (25) and by Paglin and Jamieson (26), and a breakthrough in the field of RRI came with the discovery of the GABAB receptor heterodimer (27). In the years that followed, the existence of receptor complexes formed by GPCRs was supported by more direct evidence provided by several groups, and the amount of available data increased significantly with the development (and widespread diffusion) of biophysical techniques aimed at detecting the spatial proximity of protein molecules [see (8, 28) for reviews].
It is now well recognized that class C GPCRs constitutively form homomers or heteromers (29) and some evidence has also suggested that class B GPCRs could also be involved in oligomerization processes [see (30, 31)]. With regard to class A GPCRs, their involvement in receptor complex formation in living tissues is debated [see (32)]. Indeed, some authors contend that no single experimental approach can, as yet, conclusively demonstrate these complexes in vivo (33). The possibility of class A GPCR complexes in native systems, however, is strongly supported by the available evidence as a whole. Indeed, several different approaches have provided consistent results pointing to the existence of class A GPCR complexes (34). Moreover, it should be noted that the above-mentioned class A GPCRs able to signal as monomers have also been seen to form receptor complexes (35–37). Thus, the existence of functional assemblies of class A GPCRs cannot be excluded [a discussion of this topic was recently provided by Franco et al. (38)]. In this respect, interesting studies have shown that a monomer-dimer equilibrium characterizes class A GPCRs in the cell membrane, where the half-lives of dimers (as determined from the rate of association and dissociation) indicate that they are often transient (39). This may help explain opposing views on the role of class A GPCR oligomerization (40).
The number of RRI involving GPCRs that have been identified so far is quite high and continuously increasing [see (7, 8) for recent reviews]. Most of these are stored in the GPCR Oligomerization Knowledge Base [http://www.gpcr-okb.org (41)], and, for what concerns the heteromers, in the GPCR-HetNet [http://www.iiia.csic.es/~ismel/GPCR-Nets/index.html (42)], which together comprise more than 500 entries. The research that has yielded most of these findings has focused on neurons and synapses [see (43)]. RRI between GPCRs, however, have also been seen to occur in other cell types and in districts other than the central nervous system (CNS). Furthermore, direct RRI involving the formation of receptor complexes is a feature observed in the other families of receptor molecules [see (44)]. Thus, RRI appear as a widespread phenomenon, and oligomerization as a common mechanism for receptor function and regulation.
Allosteric interactions [see (45)] are the basic molecular mechanism underlying the formation of these receptor assemblies. As recently outlined by Changeux and Christopoulos (44), the monomers forming these assemblies display a cooperative behavior, which is enabled by the action of orthosteric and allosteric ligands. Thus, the cell-decoding apparatus becomes endowed with elaborate dynamics in terms of recognition and signaling. To emphasize the “integrated output” of this input unit, the term “receptor mosaic” (RM) was also proposed, in order to indicate a multiple assembly of receptors (46). This term, indeed, stressed the concept that the emergent properties of the assembly depend not only on the type of allosteric interactions (entropic and/or enthalpic) within the integrative complex (47, 48), but also on the location and the order of activation of the participating receptors (49). On this basis, the suggestion was made (50–52) that RRI could pave the way to new strategies aimed at new targets for drug treatment. In recent years this idea has become the subject of intense research to identify receptor complexes that could constitute promising targets for the treatment of pathological conditions, and novel pharmacological strategies have already been proposed [see (7, 28, 53) for recent reviews].
Here, we will briefly review the available data on the occurrence of direct RRI between receptor proteins, the fundamentals of receptor complex formation and the impact that receptor oligomerization may have from a pharmacological standpoint.
In recent decades, GPCRs have become the main focus of studies aimed at characterizing RRI, with specific regard to the CNS. Indeed, the formation of receptor complexes is considered to be of key importance in neurophysiology (7), especially in the emerging field of “connectomics” [see (43) for a review], since integration of the input signals, already at the level of the plasma membrane, can significantly contribute to setting and tuning synaptic strength and, more generally, the efficiency of intercellular communication. Furthermore, receptor complexes may be of great importance in neuropsychopharmacology [see (7, 28, 53–55) for extensive recent reviews], and have become appealing potential targets for the development of novel therapeutic strategies in serious diseases of the CNS, such as depression and schizophrenia [see (50, 56)], Parkinson's disease [see (57)], addiction (52), neuropathic pain (58), and eating disorders (59).
GPCR homomers and heteromers, however, can be found in cell types other than the central neurons, and receptor oligomerization is not limited to GPCRs.
In the CNS, astroglia constitutes the main glial population, and increasing evidence suggests that, at the level of excitatory synapses, neurons and astrocytes interact bidirectionally, a finding that has led to the proposal of the concept of the “tripartite synapse” (60). To monitor the extracellular environment [see (57, 61)] astrocytes express specific receptors and channels, the activation of which elicits Ca2+ responses in the cells (62); these responses can, in turn, induce the release of gliotransmitters (glutamate, D-serine, ATP), thereby actively modulating synaptic transmission (63). Specifically, there is evidence that adult striatal astrocytes express both adenosine A2A receptors (64) and D2 receptors for dopamine (65). Interestingly, in vivo studies have indicated that astrocytic A2A receptor dysfunction disrupts glutamate homeostasis (66), while D2 receptors modulate immune responses in neuroinflammation-associated disorders and increase the resistance of neurons to toxic damage (67).
A considerable number of investigations conducted on these GPCRs in cell models have demonstrated that, when D2 and A2A receptors are expressed on the same cell, they can interact and heterodimerize (68–70). Moreover, functional and physical evidence has shown that, in striatal neurons, native A2A and D2 receptors can form heterodimers (71) with antagonistic A2A-D2 interactions within the receptor complex (72). Thus, it can be hypothesized that A2A and D2 receptors could give rise to receptor complexes in astrocytes as well. The first demonstration of RRI between native A2A and D2 receptors in astrocytes was recently provided by Cervetto and collaborators (73). In their study, A2A and D2 receptors co-localized in the same striatal astrocytes, where they functionally interacted in the control of glutamate release. The results also suggested that this interaction involved the formation of A2A-D2 heterodimers, since administration of the synthetic peptide VLRRRRKRVN, which is able to interfere with the D2 receptor domain involved in electrostatic interactions critical to receptor heteromerization (74, 75), eliminated the A2A-mediated inhibition of the response to D2 receptor activation.
Further evidence of RRI between GPCRs in astroglial cells has emerged from studies on adenosine A1 and P2Y1 purinergic receptors (76, 77). These studies revealed a high level of co-localization and reciprocal functional interaction of the two receptors in human hippocampal astrocytes. Furthermore, co-immunoprecipitation data indicated the existence of A1-P2Y1 heteromeric complexes in the cells.
While GPCR complexes in the CNS have been the subject of considerable research, their identification and the characterization of their functional features in peripheral tissues have so far received less attention. There is, however, significant evidence that GPCR oligomerization could play a major role in the physiology and pathology of other districts of the organism. Available examples are summarized in Table 1.
In this respect, studies on the angiotensin II type 1 receptor (AT1) are of particular interest [see (90)]. AT1 has a central role in vascular homeostasis, since it supports the structural and functional integrity of the arterial wall; however, it is also implicated in the pathogenesis of hypertension (91, 92). AT1 has been reported to heterodimerize with various other GPCRs [see (90)], suggesting that a cross-regulation arises between angiotensin II and other signaling pathways. Heteromerization has been predicted to involve the fourth to seventh TM domains of the receptor (93), and the DRY ligand-binding motif of AT1 seems to be critical to the functional activation of signaling from oligomerized AT1 (94). Of relevance, in this context, was the indication of the existence of heterodimers between AT1 and β-adrenergic receptors in cardiomyocytes and related cell lines (78), where a single antagonist (AT1 or β-adrenergic receptor antagonist) proved able to induce a inhibition of both receptors. It has also been shown that the contribution of AT1 to specific forms of hypertension is modulated by the formation of receptor complexes with the B2 bradykinin receptor (79) in renal mesangial cells, and with purinergic P2Y6 receptors in mouse smooth-muscle cells (80), while physical interactions with the apelin receptor have been proposed to regulate the effect of angiotensin II in mouse models of atherosclerosis (95). A sure sign of major cardiovascular diseases that contribute to cardiac dysfunction is the hypersecretion of noradrenalin (NA). In this regard, the receptor complex between AT1 and the α2C adrenergic receptor in sympathetic neurons was found to be involved in NA secretion, since the dual occupancy of the protomers by agonists produced a heterodimer conformation different from that induced when a single protomer was activated; this triggered atypical Gs-cAMP-PKA signaling, promoting NA hypersecretion (81). Taken together, these findings suggest that receptor complexes involving AT1 may be promising targets for novel treatments of cardiovascular diseases (96) especially in hypertension and preeclampsia (97, 98).
Apart from its role in blood pressure regulation, AT1 contributes to the development of fibrosis in a number of organs (90). For instance, it is well-expressed in activated hepatic stellate cells, which are primary agents of the fibrogenic response in the liver (99). It has been shown that the AT1-mediated increase in profibrogenic markers in hepatic stellate cells of rats chronically treated with ethanol is completely blocked by an antagonist of the cannabinoid receptor CB1. These data have prompted the analysis of interactions between these two receptors, and the heteromerization of CB1 and AT1 receptors in this cell type has been demonstrated by means of co-localization, co-immunoprecipitation and BRET assays (82). Analysis of the signaling properties of the heteromer has shown that AT1 receptor agonists induce a rapid, dose-dependent increase in ERK1/2 phosphorylation, which is potentiated by CB1 receptor agonists and blocked by CB1 antagonists, suggesting that the CB1-AT1 heteromer may be a possible novel therapeutic target in the treatment of liver fibrosis.
Key players in the regulation of the cardiovascular system [see (100)] are endothelin and serotonin receptors. These are both expressed in many cardiovascular tissues, and in vitro results (mainly of a functional type or obtained on cell lines) have suggested that they could be part of receptor complexes (101, 102). In native cells and tissues, however, their involvement in heteromerization processes remains to be assessed.
Very recently, it has also been hypothesized (87) that receptor complexes exist in the carotid body (CB), a small peripheral chemoreceptor that plays a basic role in conditions such as hypercapnia, hypoxia, hypoglycemia and acidosis, in which it triggers an adequate cardiovascular and respiratory response. This hypothesis is based on the large repertoire of GPCRs expressed (most of which are able to form receptor complexes in other tissues) and on functional data providing indirect evidence of the existence of GPCR complexes in the CB. Specifically, an antagonistic RRI between dopamine D2 and adenosine A2B receptors in CB type I cells has been suggested. Indeed, it has been shown that D2 agonists reduce catecholamine release and inhibit cAMP production in these cells, and that these effects are prevented by adenosine A2B receptor agonists. Conversely, A2B receptor antagonists counteract the increased catecholamine release induced by D2 antagonists (103, 104).
GPCRs are also of central importance in the endocrine system [see (100, 105)], and increasing evidence points to GPCR oligomerization as a significant aspect of endocrine regulation [see (106) for a recent detailed review]. For instance, a growing number of reports have suggested that GPCR heterodimerization may play significant roles in reproduction, including the secretion of hormones and the growth and maturation of follicles and oocytes [see (107) for a review specifically addressing this topic]. Indeed, several GPCRs are involved in the regulation of reproductive functions at the level of the reproductive organs and the hypothalamic-pituitary axes. Luteinizing hormone (LH), which is secreted by the adenohypophysis, stimulates testosterone production in Leydig cells of the male, and in females triggers ovulation by acting on the LH receptor (LHR), a class A GPCR. Biophysical and pharmacological assays have shown that LHR homomers displaying negative cooperativity between the receptor partners can be formed in vitro (83) and more recently a trans-complementation assay has been used to investigate the presence of LHR homomers and their functional relevance in vivo (108). To regulate pubertal maturation and reproductive processes, LH acts together with follicle-stimulating hormone (FSH); FSH is also produced by the anterior pituitary and binds the class A GPCR FSH receptor (FSHR). On the basis of crystallographic data, it has been hypothesized that FSHR has a dimeric structure and that, upon binding, it gives rise to a tetrameric complex composed of an FSH dimer that bridges the dimeric FSHR (109). Subsequent studies have pointed to a central role of the TM region of FSHR in stabilizing constitutive dimers (110). More recently, BRET assay (85) and fluorescence correlation spectroscopy (84) have also revealed heteromers between LHR and FSHR, in which heteromerization leads to an enhanced ligand dissociation rate and a negative regulation of cAMP production (84). LHR-FSHR receptor complexes are of potential physiological significance in females, since during the peri-ovulatory period co-expression of these receptors primarily occurs in granulosa cells [see (105)]. GPCR heteromers also impact on glucose metabolism, as indicated by FRET-based studies demonstrating heteromerization of growth hormone secretagogue receptor (GHSR) and somatostatin 5a receptor (SST5a) in β islet cells of the pancreas (86). In these studies, heteromerization changed the preferred G protein-coupling of GHSR from Gαq11 to Gαi/0, mediating the inhibition of the glucose-stimulated insulin secretion induced by ghrelin and somatostatin.
With regard to pathological tissues, the possibility of a GPCR heteromer-based strategy in oncology has been proposed by Moreno and collaborators (89). This is based on the finding that the cannabinoid CB2 receptor and the GPCR55 (GPR55) are overexpressed in cancer cells and human tumors and that they form heterodimers displaying antagonistic CB2-GPR55 interactions in cancer cells. Moreover, it has been shown that GHSR and neurotensin receptor 1 (NTS1) can establish direct structural interactions in vitro, and neuromedin-U has been indicated as a ligand for this heteromer (88). These findings are of interest to oncology. Indeed, in non-small cell lung cancer, it has been suggested that GHSR-NTS1 heteromers are involved in an autocrine growth-promoting pathway (88). Although preliminary, these data suggest that these heteroreceptor complexes may constitute novel targets in future cancer studies.
Advances in crystallographic techniques have revealed the structural architecture of many receptors. Although receptor proteins operating as monomers have been observed [see (111)] oligomeric organization appears to be quite a common feature in the different receptor families, as illustrated in Figure 1 [see (44) for a detailed review]. This probably constitutes an efficient mechanism for modulating the functionality of receptor proteins, including those able to signal as monomers, like GPCRs.
Figure 1. Multimeric molecular structures of receptors from different families, as determined by crystallographic studies. The protomers forming each complex are shown in different colors. (A) Top view (from the extracellular side) of a pentameric LGCI, namely a cationic ligand-gated ion channel [PDB code: 5HCJ; (112)]. The arrow indicates the interface between subunits, where the orthosteric binding site is located, halfway between the membrane and the top of the extracellular domain. (B) Bottom view of a tetrameric VGIC, the human transient receptor potential ion channel M4 [PDB code: 6BQV; (113)]. The arrow indicates the interface between neighboring monomers. The cytoplasmic domain involves four homology regions (MHR1 to MHR4) and MHR1 of one subunit interacts with MHR3 of the adjacent subunit to form the interface. (C) Dimeric HNR, the human estrogen receptor 1 [PDB code: 1X7E; (114)]. In each monomer, the arrow indicates helix 10/11, where the dimer interface is formed; (D) Dimeric extracellular domain of a human RTK, the EGFR [PDB code: 5WB7; (115)]. Arrows indicate the dimerization arms mediating dimer formation. (E) GPCR homodimer of β1-adrenergic receptors [PDB code: 4GPO; (116)]. N and C terminals are indicated. The dimerization interface has been shown to involve TM4 and TM5 (117). As illustrated, oligomerization plays an important role in the function of all receptor families, including GPCRs. Although GPCRs mostly signal as monomers, there might also be stable GPCR dimers/oligomers or transient quaternary structures that are constantly formed and dissociated at the cell membrane.
The LGIC family (see Figure 1A), for instance, mainly consists of constitutively pentameric ion channels (118), including nicotinic, serotonin and GABAA receptors. Tetrameric and trimeric receptors are also part of this family (119). These include ionotropic glutamate receptors and purinergic P2X receptors, respectively. Although some homomeric LGICs exist, the majority of receptors in this family are hetero-oligomers made up of various subunits. The structures that have so far been characterized reveal strikingly similar 3D arrangements, showing features of symmetry with the ion channel lying along the central axis of symmetry (118) and ligand-binding sites mostly at subunit interfaces.
VGIC receptors also have an oligomeric structure [see (120)]. They are characterized by a α subunit (~260 kDa) that forms a large channel and one or two β subunits of 30–40 kDa. In addition to the well-known examples of VGIC, such as those for potassium, calcium, and sodium, the transient receptor potential (TRP) channels also belong to this family (121). These, however, are symmetrical homotetramers (Figure 1B) with a 3D structure resembling that of LGICs (122).
Regarding NHRs, these are ligand-regulated transcription factors with a disordered N-terminal domain, a central DNA-binding domain, and a C-terminal domain containing the pocket for the ligand. It is well-acknowledged that only one subset of NHRs is made up of monomeric receptors [see (123)], the majority of NHRs operating as homo- or hetero-dimers (Figure 1C).
Finally, RTKs (which function as receptors for growth factors and related hormones) all possess an extra-cellular domain of variable length that recognizes the ligand (Figure 1D), a single TM region and an intracellular domain linked to the tyrosine kinase domain, this latter performing the catalytic process which initiates signal transduction (124). With some exceptions, such as the insulin receptor (125), in the absence of a ligand most RTKs are monomeric; however, in almost all instances [some exceptions have been reported very recently, see (126)], dimerization is needed for their activation (127). Four mechanisms of dimerization have been hypothesized [see (44)]. These are: cross-linking of two receptor proteins by a bivalent ligand (e.g., nerve growth factor binding to its TrkA receptor); bivalent ligand binding combined with interaction between specific interfaces on the receptors to form the dimer (as when stem cell factor binds to the KIT receptor); the need for multiple contacts involving the agonist, the receptor and accessory proteins (e.g., FGF and its receptor); and “unmasking” of buried dimerization interfaces following the conformational rearrangement induced by ligand binding (e.g., EGF and its receptor). Due to this variety of possible mechanisms underlying RTK dimerization, it has been suggested that both symmetric and asymmetric arrangements of the extracellular domains may occur (128). Moreover, some data suggest that some RTKs (e.g., the PDGFβ receptor) could form high-order aggregates (129) and also directly interact with other RTKs (130), such as the EGF receptor (EGFR).
Thus, as recently pointed out by Changeux and Christopoulos (44), oligomerization plays an important role in the function of all receptor families, with the ion channel receptors (where multimerization is necessary) being located at one end of the spectrum and GPCRs (Figure 1E) at the other. Indeed, GPCRs may signal not only as monomers, but also as stable dimers/oligomers, or give rise to transient quaternary structures, which are constantly formed and dissociated at the cell membrane [see (8)].
In this context, RRI involving receptors from different families are also of interest. It is well-known that receptors can functionally interact, without coming into contact with each other, through mechanisms of transactivation or by sharing signaling pathways (131, 132). Recently, however, the formation (by direct RRI) of receptor complexes involving an RTK receptor, the fibroblast growth factor receptor 1, and GPCRs such as the serotonin 5-HT1A receptor (133) or the muscarinic M1 receptor (134) has been associated with increased neurite densities in hippocampal cell cultures after agonist coactivation. In striatal glutamate synapses, a direct structural interaction between dopamine D2 and NMDA receptors that leads to inhibition of NMDA receptor signaling has been identified (135). Furthermore, recent data have prompted speculation that a possible direct interaction takes place between hyperpolarization-activated nucleotide-gated (HCN) cation channels and D1 dopamine receptors in the prefrontal cortex. Indeed, HCN and D1 receptors are co-localized in layer III of the dorsolateral prefrontal cortex and blocking the HCN channels has been seen to prevent the inhibition of neuronal firing induced by D1 signaling. Correspondingly, the blockade of HCN channels in the prefrontal cortex of rats has proved able to prevent working memory impairments induced by D1 stimulation or pharmacological stress (136).
A clear discussion of allostery in receptors has recently been provided by Changeux and Christopoulos (44), and, for what concerns GPCR homomers and heteromers, extensive reviews have been provided by Kenakin and Miller (137) and by Smith and Milligan (138). Here, some basic concepts will be briefly summarized.
Allostery [see (139)] is a mode of communication between distant sites in proteins, in which the energy associated with dynamic or conformational changes at one site can be transported along specific pathways within the structure of the protein to other sites, which change their dynamic or conformational properties accordingly (140). In this respect, receptor molecules are undoubtedly “allosteric machines” (141), since their activation mechanism involves the recognition of an extracellular signal at the ligand-binding domain, and the changes induced are transmitted to the biologically active site of the protein, which, as in transmembrane receptors, may be located tens of Å away. Since changes in protein conformation underlie allosteric processes, the possibility for a protein to be allosterically modulated depends on its ability to acquire new conformations. Therefore, a protein with a rigid structure is less predisposed to be allosterically modulated than one that possesses segments that do not fold into a stable secondary structure, i.e., segments endowed with a high degree of intrinsic disorder (142, 143). Intrinsically disordered regions have been identified in all classes of membrane receptors. Mechanisms of structural change from order to disorder (or vice versa), for instance, have been hypothesized to underlie the activation of receptors of the RTK family (144) and intrinsic disorder of the N-terminal domain appears to play a significant role in the functionality of NHRs [see (145)]. GPCRs exhibit disordered segments extracellularly (in the N-terminus) and large disordered sequences in the cytosolic region, mainly in the intracellular loops—particularly ICL3—and in the C-terminal domain (142, 146).
Malleability and structural plasticity, however, are of importance not only because they enable conformational fluctuations and intra-receptor interactions to take place, but also because they allow the formation and dynamics of receptor complexes. Indeed, when two protomers establish direct RRI, thereby giving rise to a quaternary structure, the energy associated with a perturbation at one site of one protomer can propagate over the interface between receptors into the nearby protomers, thus changing their conformation and functional features and leading to a cooperative behavior of the complex (147).
Identifying the residues that specifically interact to form the interaction interface is therefore of significant interest in current research on receptor oligomerization (148) as these residues influence the models of potential allosteric interactions between receptor partners.
Pentameric LGCIs derive from the assembly of subunits containing an N-terminal extracellular domain (ECD), four transmembrane segments (named M1 to M4) and a cytoplasmic domain between M3 and M4 of highly variable sequence and length (118). To ensure the correct assembly of the channel, a very specific inter-subunit interface is formed in the extracellular domain through mixtures of salt bridges, van der Waals contacts and hydrogen bonds (149). In the GABAA receptor, for instance, inter-subunit contacts between the central portion of the ECD involve β4, β5, β5′, and β6 strands and flanking loops (149). The same concept can be applied to trimeric (150) and tetrameric (151) LGCIs. AMPA-type glutamate receptors are an example (151). Subunits first form dimers, which subsequently assemble into tetramers. Dimerization is driven by specific interfaces in the most superficial layer of the extra-cellular region (the N-terminal domain), while tetramerization is mediated by contact points in all layers of that region. By contrast, specific interfaces in the cytoplasmic region of the receptor complex are implicated in the assembly of VGCIs (152, 153). Studies of the TRPV6 channel, for instance, have identified a domain encompassing an ankyrin repeat in the intracellular region of the monomers; this domain is key to mediating the correct assembly of the subunits in order to obtain a functional channel (153).
The superfamily of nuclear receptors is composed of ligand-dependent transcription factors. These regulate a diversity of cellular processes, including development, differentiation, growth, metabolism, and reproduction. Nuclear receptors are proteins composed of a C-terminal ligand-binding domain (LBD), a conserved DNA-binding domain (DBD), and a variable amino-terminal region (154). They operate as homo- or hetero-dimers, binding to hormone response elements of target genes. A specific dimerization interface (also named D box) resides within the DBD and corresponds to a zinc-binding module (155).
As mentioned earlier, RTKs are single-pass trans-membrane proteins with an extracellular N-terminal domain containing motifs involved in ligand binding. The TM domain is followed by a juxta-membrane region and an intracellular catalytic domain. RTKs operate as dimers, and helix-helix interactions in the TM domain are key to providing the stability of full-length dimers and maintaining a signaling-competent dimeric conformation (156, 157). Specifically, as observed in the FGF3 receptor (158) and the ErbB2 EGFR (156), GxxxG motifs, also called SmallxxxSmall motifs, are part of the dimer interface. These motifs are characterized by the presence of small amino acids (Ala, Gly, Ser, and Thr) in i, i+4 positions and drive interactions between hydrophobic helices in membranes (157).
In comparison with the other receptor families, GPCRs are endowed with some distinctive features in terms of interfaces for dimerization. Our knowledge of interaction interfaces has been extended both through the application of bioinformatics methods [see (8, 159)], in order to predict amino acid sequences potentially involved, and by experimental investigation. Indeed, recent improvements in experimental procedures have provided researchers with a range of methods and tools for identifying and characterizing interaction interfaces in GPCRs. Significant advances in GPCR crystallization techniques, for instance, have led to an increase in the number of experimentally assessed structures in recent years (160). Further experimental tools that are currently available include: atomic force microscopy (147); new super-resolution imaging approaches, such as photoactivated localization microscopy (PALM) (161); far-UV CD spectroscopy, and SDS-PAGE using synthetic peptides corresponding to different transmembrane domains (162). By using mass spectrometry combined with collision-induced dissociation experiments, Woods et al. (74, 75) investigated intracellular domains (e.g., ICL3 and C-terminus) and demonstrated strong electrostatic interactions in GPCR heteroreceptor complexes. Experimental results concerning dimerization interfaces are reported in Table 2 for a number of GPCRs.
The first noteworthy feature to emerge from both computational and experimental studies concerns the ability of GPCR structures to interact via multiple interfaces. The A2A-D2 heteromer is probably an example of this. In the study by Woods and coworkers (74) dimer formation was found to occur at the intracellular level through electrostatic interactions between the ICL3 of D2 and the C-tail from A2A. Very recently, however, the interaction between TM4 and TM5 helices was also shown to support the heteromerization of these receptors (164). In quite a large number of GPCR complexes, TM4, TM5, TM6, and ICL3 were found to be the main interfaces. Regarding the possible involvement of extracellular loops in RRI, this has been demonstrated for some class A GPCRs (116), while in some class C GPCRs, interactions between extracellular domains through disulfide bridges (29) have been demonstrated.
A further interesting finding to emerge from computational and experimental studies on GPCRs oligomerization is the presence at the interface of motifs that appear to be of particular importance in the allosteric interaction. As demonstrated by Woods et al. (75, 170, 171), electrostatic interactions between intracellular domains may occur between a positively charged arginine (Arg)-rich motif of one receptor and a negatively charged serine-phosphate-containing motif of another receptor. Once established, this interaction possesses a covalent-like stability, which probably constitutes a significant mechanism for the assembly of the receptor complex. As in RTKs, Small-xxx-Small motifs have been reported to promote TM1 self-association in some GPCRs (172), and, by means of a bioinformatics approach, Tarakanov and Fuxe (173) identified a set of triplet homologies, mainly located at the receptor-receptor interface, that may be responsible for RRI. Most of these are motifs containing leucine. Another set of triplets contains charged amino acids. It has been suggested that the electrostatic interaction between triplets may guide and clasp the interactions between protein partners (51, 174).
The evidence that a given GPCR can exploit multiple interaction interfaces implies at least two significant consequences with regard to the architecture of the resulting receptor complexes:
a. The first concerns the number of subunits forming the complex (i.e., its stoichiometry), since the possibility exists that a given GPCR could be involved in oligomeric assemblies of different orders (48). Among the first to provide evidence of the role played by interaction interfaces between protomers in arranging the quaternary structure of receptor complexes were Navarro and coworkers (165). Their study focused on dopamine D2, adenosine A2A, and cannabinoid CB1 receptors. Each of these possesses two intracellular domains that are able to specifically interact with intracellular domains of the other two protomers via electrostatic interactions, leading not only to the formation of dimers (A2A-D2, A2A-CB1, CB1-D2) but also to the assembly of an A2A-D2-CB1 heterotrimer. Indeed, trimeric receptor complexes have been identified (175); examples include the muscarinic M2 homotrimer (176), the A2A-D2-mGlu5 (177) heteroreceptor complex, the dynamic Gal1-5HT1A-GPR39 heterotrimer (178), and the putative Gal1-Gal2-5HT1A heterotrimer (179). With regard to tetrameric arrangements, the possible occurrence of a heterotetrameric structure for the complexes formed by adenosine A1 and A2A receptors has recently been proposed (163). In this complex, homodimerization is supported by a TM4-TM5 interface, and a TM5-TM6 interface mediates heterodimerization. Evidence that tetrameric assemblies of β2-adrenergic receptors (β2AR) occur spontaneously following reconstitution into phospholipid vesicles (36) was provided by Kobilka et al. who suggested that oligomerization was an intrinsic property of β2AR. Evidence of higher-order GPCR oligomers has also been reported. Combined BRET/FRET and complementation studies, for instance, have revealed that, in the plasma membrane of living mammalian cells, the association of dopamine D2 receptors by means of symmetrical interfaces at TM4 and TM1 can generate an assembly composed of at least four protomers (167). Moreover, studies based on the analysis of PALM data have led to the hypothesis that, depending on the specific membrane microenvironment, direct RRI among GPCRs could allow the formation of high-order oligomers, such as tetramers, octamers, and complexes of larger size (180).
b. Secondly, the notion that GPCRs can exploit multiple interaction interfaces opens up the possibility that a given set of interacting GPCRs could associate according to different geometrical arrangements (181); these associations would depend on a variety of conditions that include not only the physical features of the protomers involved (hydrophobicity, surface charge, etc.) but also the characteristics of the microenvironment surrounding the interacting monomers. The functional behavior of a receptor complex may be significantly influenced by its topological arrangement. In this regard, Agnati et al. carried out a theoretical analysis based on thermodynamic considerations and which focused on the role that the spatial arrangement of GPCR monomers may play within a receptor complex (182). They showed that, for each given set of binding and interaction constants, the theoretical saturation curves of trimeric or tetrameric receptor complexes were dependent on the geometry of the assembly formed. Interesting experimental evidence of this concept was recently provided by Jonas et al. (183), who adopted a super-resolution imaging approach. Their study focused on two mutant luteinizing hormone receptors that can function only via intermolecular cooperation in which the oligomeric forms are favored over the dimeric ones. Their PD-PALM images of trimers and tetramers showed that monomers associated through helix interfaces according to a variety of distinct spatial arrangements that were also different from one another in terms of signal sensitivity and strength.
The importance of supramolecular assemblies of receptors can be appreciated when we consider the possible emergence of integrative functions from the collective dynamics of a receptor complex (147). Indeed, a configuration change in a given protomer due to allosteric RRI will modulate the probability of configuration change in the adjacent receptors in the complex, and propagation of this effect throughout the cluster will lead to an integrated regulation of multiple effectors (184). These concepts have been well-described by mathematical models of cooperative dynamics in receptor assemblies [see (8, 159) for reviews], based on discrete dynamics (49) or on thermodynamics-based approaches (185). These models have allowed receptor complexes to be described as possessing “emergent properties”, i.e., biochemical and functional features that could not be fully anticipated on the basis of the characteristics of the single receptor partners. According to a metaphor proposed by Kenakin (186), since receptor complexes are not just “on-off” switches but exhibit quite a high ability to elaborate incoming information, they would operate as a sort of molecular “microprocessor”.
Thus, when RRI take place at the membrane, the actual signaling outcomes of receptor complexes depend on several factors, including the composition of the complex and its topological organization, the traffic of the receptor complex, the effects of ligands on the formation of the assembly and on its stability, and, quite often, crosstalk with alternative signaling pathways (48, 187). Together, these factors may strongly influence the chain of events linking ligand recognition to signal transduction from the single protomers. Figure 2 schematically summarizes some of the potential signaling consequences of the allosteric modulations occurring when a receptor complex forms.
Figure 2. As a result of allosteric RRI, receptor complexes appear to be endowed with pharmacological features that cannot be fully derived from the characteristics of the single participating protomers (see text).
These can be briefly summarized as follows [see (187) and, with regard to GPCRs, (7, 8, 28, 53) for reviews]:
a. In a variety of receptor complexes, modulation of the binding sites has been reported as a consequence of allosteric RRI. One of the first examples was the A2A-D2 heterodimer, where the binding of the adenosine A2A agonist CGS21680 reduced the affinity of the dopamine D2 agonist-binding site (188). In this GPCR heterodimer, the interaction between D2 and A2A is reciprocal, since the A2A-induced increase in cAMP accumulation via Gi/o at the level of the adenylate cyclase is inhibited by D2 receptor activation (189). A similar reciprocal modulation occurs in the CCR2b-CCR5 chemokine receptor dimer. When this heteroreceptor complex forms, the CCR5, which is normally insensitive to monocyte chemoattractant protein-1 (MCP-1), becomes able to bind MCP-1. Likewise, the CCR2b receptor, which is normally unresponsive to the CCR5 chemokine ligand macrophage inflammatory protein-1β (CCL4), binds CCL4 when in complex with CCR5 (190). Modulation of the binding sites consequent to subunit assembly may also occur in RTKs, as suggested by studies (191) on the insulin receptor (IR). The human IR is a glycoprotein that exists as two isoforms, which have a dimeric structure consisting of two α subunits and two β subunits linked by disulfide bonds. It is transcribed from a single gene encoding both α and β subunits. The two IR isoforms differ by 12 amino acids, which are absent (IR-A) or present (IR-B) at the C-terminal part of the α subunit. IR-A and IR-B exhibit at most a 2-fold difference in insulin affinity, but the two hormones, insulin-like growth factor 1 and insulin-like growth factor 2, have been found to have up to 5-fold higher affinity for IR-A than for IR-B.
b. Changes in the decoding of signals reaching protomers constitute a second mechanism induced by allosteric RRI. This aspect seems to be of particular importance in GPCRs. Indeed, many functional/pharmacological and structural-based studies have shown that a GPCR does not act as a simple switch that turns a given signaling pathway “on” or “off”; rather, it can assume multiple conformations once it is bound by a given ligand or through interactions with other signaling partners. This means that GPCRs are multidimensional transducers that can engage, and differentially regulate, diverse signaling pathways, such as distinct G protein classes or β-arrestins. The discovery of molecules able to activate distinct pathways after interacting with the same receptor led to the concept of functional selectivity and biased agonism, which was used to describe these GPCR-based signaling processes [this topic was recently extensively reviewed by Costa-Neto et al. (192), Pupo et al. (193), Goupil et al. (14)]. Thus, when a receptor complex forms, the pattern of possible configurations that each GPCR protomer can assume is influenced not only by the ligands, but also by RRI with the other partners in the complex, potentially leading to functional selectivity of signaling downstream (14, 137). Changes in the decoding of signals associated to GPCR complex formation have been reported. The heterodimer formed by dopamine D1 and histamine H3 receptors provides a first example (194). In the experimental conditions used in this study, when the receptor complex forms, the D1 receptor changes its coupling from the Gs to the Gi protein, to which H3 receptors are already coupled. As a consequence, in the presence of the H3 receptor, D1 receptors can no longer activate adenylyl cyclase, but, being coupled to Gi, they transduce the signal toward the MAPK pathway. The recruitment of G proteins other than those expected for the monomers has been observed after D1-D2 dimerization (195) and a switch between G protein and β-arrestin signaling (196) has been documented after κ-μ and κ-δ opioid receptor heteromerization (197). Processes of this type can also be hypothesized in some RTKs. IR and the closely related insulin-like growth factor receptor 1 (IGF1) are present in the membrane as preformed dimeric complexes, and both bind insulin and members of the insulin-like peptide family. Signaling through IR and IGF1, however, has different physiological outcomes [see (187)], with IGF1 signaling being essentially mitogenic (through the Ras/MAPK pathway) and IR signaling mainly producing metabolic effects (through the PDK/Akt pathway). The EGFR provides a further example. Crystallography and other approaches (115) have shown that different ligands stabilize different dimeric conformations of the EGFR extracellular region, leading to different signaling dynamics.
c. A relevant aspect of receptor complex formation is the possibility that novel specific allosteric sites suitable for the binding of some modulators could appear in the quaternary structure resulting from the assemblage of the protomers. Thus, ligands specific to the receptor complex as such may also exist [see (96)]. Since the early discovery of benzodiazepines as allosteric activators of the GABAA receptor, it has been shown that, in addition to the orthosteric site, most constitutively dimerized/oligomerized cellular receptors possess spatially distinct sites that modulate their allosteric transitions. Pharmacologically, allosteric ligands can be classified as “positive allosteric modulators” (PAM), when they enhance the effect of the orthosteric ligand, “negative allosteric modulators”, when they reduce the effect of the orthosteric ligand, and “neutral allosteric ligands”, if their binding to the allosteric site does not modulate the effect of the orthosteric ligand. Sometimes a PAM may activate the receptor even in the absence of an agonist, and is therefore referred to as an “allosteric agonist”. Combinations of these properties are also possible [see (44) for a discussion of the topic]. The same concepts apply to GPCR monomers, where allosteric binding sites may be present in various domains of the protein (198). Allosteric binding sites of class A GPCRs are, in most cases, located in the same region as the orthosteric site (i.e., within the seven-transmembrane domain), while the two types of sites are usually well-separated in class C GPCRs [see (199)]. The formation of a GPCR receptor complex, however, can result in significant structural and functional changes in the allosteric binding sites on single monomers [see (200)] and in the appearance of new allosteric sites. In this respect, a first example of the possible existence of allosteric modulators specific to a GPCR receptor complex was provided by studies on the effect of homocysteine (142, 201, 202) on the A2A-D2 heterodimer (Figure 3). In Chinese hamster ovary cells stably cotransfected with dopamine D2 and adenosine A2A receptors (201) homocysteine was found to selectively reduce the internalization of the receptor complexes induced by D2 receptor stimulation, and in astrocytes (202) homocysteine reduced D2-mediated inhibition of glutamate release without altering the A2A-D2 interaction, since the A2A-mediated antagonism of the D2 effect was maintained. Mass spectrometric analysis (201) provided mechanistic support for these findings. This revealed that, by exploiting an Arg-thiol electrostatic interaction, homocysteine formed non-covalent complexes with the two Arg-rich epitopes of the ICL3 in the D2 receptor, one of which was also involved in the dimerization interface. FRET experiments, however, showed that homocysteine was unable to disrupt or prevent the heteromerization of A2A and D2 receptors, suggesting that it probably behaves as a modulator of the allosteric process of energy transmission between the two partners.
Figure 3. Docking [obtained by means of the Rosetta software, (203)] of the crystallographically-assessed structures of adenosine A2A [PDB code: 4EIY; (204)] and dopamine D2 [PDB code: 6CM4; (205)] arranged to form the A2A-D2 heterodimer through a TM4-TM5 interface as recently described by (164). The docking of homocysteine (HCy) to the receptor complex is also shown. This occurs in an Arg-rich region [the epitope 115VLRRRRKRVN] of the D2 receptor ICL3 and is consistent with an electrostatic interaction between the negatively charged thiol group in HCy and the positively charged guanidinium group of arginine (201).
A final aspect that deserves to be mentioned [see (8, 187) for a more detailed discussion] is the cell environment in which receptors and receptor complexes are located. Indeed, their signaling outcome is also influenced by the network of molecular interactions they can establish with other biochemical components. For what concerns membrane receptors, the term “horizontal molecular network” (48) has also been proposed to illustrate this concept. By 2003, 50, or more GPCR interacting proteins (GIP) had already been discovered and, in a review article, Bockaert et al. (206) drew attention to the C terminal tail of the GPCRs as an important site for the establishment of functional protein networks. The available findings indicate that receptor complexes are often involved in multiple receptor-protein interactions that may influence their assemblage and stoichiometry [see (8)]. Many GPCR interacting proteins act as scaffolding or adapter proteins, modulating the physical receptor-receptor interactions in receptor complexes (207). An association of particular interest occurs between GPCRs and a set of three homologous transmembrane proteins, which have been named RAMP (receptor activity-modifying membrane protein) (208). When RAMPs associate with the calcitonin-like receptor (CLR), complexes with very different functional profiles are generated: the RAMP1-CLR complex behaves phenotypically as a calcitonin gene-related peptide receptor, whereas the assembly of RAMP2 or RAMP3 with CLR provides specificity for adrenomedullin (209). RAMPs have also been shown to associate with other B family GPCRs, including glucagon receptors and parathyroid hormone [see (137)]. With regard to nuclear receptors, within the cytoplasm they are often found to be complexed with other proteins, which act as co-activators or co-repressors, while within the nucleus, nuclear receptors are part of larger transcriptional regulatory complexes (210).
Thus, in view of the multiplicity of support proteins with which receptors operate within the cell, it is realistic to surmise that these support proteins could have a significant impact on the properties of the receptors.
For what concerns membrane receptors, the lipid environment is also important, since this has been shown to influence receptor function [see (8)]. For instance, several aging-related health disorders have been found to be associated to membrane composition changes that can alter GPCR signaling (211). Furthermore, membrane features may regulate receptor assembly in membrane nanodomains through hydrophobic interactions (212).
Intercellular communication is a key process in the physiology of living beings, and the fundamental mode of communication in biological systems involves interaction between specific receptors expressed by the target cells and chemicals or energy forms released by a source. Thus, it is not surprising that the majority of the drugs currently used to treat pathological conditions are basically agonists or antagonists of some classes of receptors. Until relatively recently, drug design was based on the concept that ligands compete for interaction with a common “rigid” site [see (213)]. The discovery of flexible allosteric proteins and of allosteric modulatory sites in all receptor families [see (44)] paved the way to the design of new drugs that interacted with topographically distinct active sites on the receptor protein, and which often provided greater selectivity in receptor targeting. Subsequently, GPCRs (the largest family of receptors) were found to be even more versatile allosteric machines than previously believed, being able to alter their configuration to accommodate ligands and engage distinct signaling effector subsets [see (192)]. Moreover, GPCRs were seen to operate not only as monomers, but also as quaternary structures (17, 19) in which the configuration of the single receptors and of the entire complex is shaped by networks of electrostatic interactions (hydrogen bonds, van der Waals forces), thereby enabling incoming signals to be integrated already at the plasma membrane level. Once established, these integrative mechanisms can change the function of the GPCRs involved, leading to a sophisticated dynamic of the receptor assembly in terms of modulation of recognition and signaling [see (28)]. However, further research is needed in order to gain a deeper understanding of the signaling features of GPCR complexes, in terms of their possible configurations and downstream signaling pathways, a goal which would undoubtedly be of substantial interest.
Although RRI have so far been mainly studied and characterized in central neurons, they appear to be a widespread phenomenon, contributing to the metabolic regulation of several cell types and tissues other than the CNS. Moreover, oligomerization is not limited to GPCRs, as demonstrated in the other receptor families, in which the active form of most of the receptors is the result of the proper dimeric/oligomeric association of protein subunits. Both of these issues warrant further research.
In addition [see (187)], increasing evidence has shown that responses to specific ligands are critically influenced by the environment in which receptors and receptor complexes are located, and, in particular, by other proteins and biochemical constituents that establish structural or functional interactions with them. Within this context, signaling cannot be viewed exclusively as the output of a single receptor-agonist pair; rather, it often results from the modification of the targeted receptor or receptor complex by scaffolding proteins and other signaling partners.
Taken together, these findings have at least two important consequences for the study of new pharmacological tools, in particular for what concerns GPCRs, which constitute the target of about 50% of currently available drugs (28). On the one hand, RRI may be potential sources of undesired side effects of new drugs that are assumed to be specific agonists or antagonists of a given receptor, since the fine-tuned integrated response obtained through allosteric RRI could lead to unexpected outcomes. Indeed, as pointed out by Kleinau et al. (106), future studies should strive to characterize the receptor complexes typically expressed in pathological human tissues and to carefully distinguish the functional effects induced by monomers from those induced by receptor complexes. On the other hand, however, RRI may provide new opportunities to optimize pharmacological treatments in terms of receptor targets and tissue selectivity or to develop completely new pharmacological interventions that specifically target receptor complexes. In this regard, very promising results have emerged from studies on high-affinity antibodies (214), ligands for allosteric sites unique to oligomeric assemblies (215), and bivalent ligands selective for dimeric receptor complexes (105, 216).
DG and LA projected the paper and DG wrote the text. DG, MM, CT, and GM performed bibliographic search and collected relevant sources. All the authors discussed and revised the text before submission.
The authors declare that the research was conducted in the absence of any commercial or financial relationships that could be construed as a potential conflict of interest.
1. Limbird LE. The receptor concept: a continuing evolution. Mol Interv. (2004) 4:326–36. doi: 10.1124/mi.4.6.6
2. Lefkowitz RJ. Seven transmembrane receptors: a brief personal retrospective. Biochim Biophys Acta (2007) 1768:748–55. doi: 10.1016/j.bbamem.2006.11.001
3. Humphrey PPA, Barnard EA. International union of pharmacology. XIX The IUPHAR receptor code: a proposal for an alphanumeric classification system. Pharmacol Rev. (1998) 50:271–7.
4. Wise A, Gearing K, Rees S. Target validation of G-protein coupled receptors. Drug Disc Today (2002) 7:235–46. doi: 10.1016/S1359-6446(01)02131-6
5. Foord SM, Jupe S, Holbrook J. Bioinformatics and type II G-protein-coupled receptors, Biochem. Soc Trans. (2002) 30:473–9. doi: 10.1042/bst0300473
6. Attwood TK, Findlay JBC. Fingerprinting G-protein-coupled receptors. Protein Eng. (1994) 7:195–203. doi: 10.1093/protein/7.2.195
7. Farran B. An update on the physiological and therapeutic relevance of GPCR oligomers. Pharmacol Res. (2017) 117:303–27. doi: 10.1016/j.phrs.2017.01.008
8. Guidolin D, Marcoli M, Tortorella C, Maura G, Agnati LF. G protein-coupled receptor-receptor interactions give integrative dynamics to intercellular communication. Rev. Neurosci. (2018) 29:703–26. doi: 10.1515/revneuro-2017-0087
9. Kunishima N, Shimada Y, Tsuji Y, Sato T, Yamamoto M, Kumasaka T, et al. Structural basis of glutamate recognition by a dimeric metabotropic glutamate receptor. Nature (2000) 407:971–7. doi: 10.1038/35039564
10. Lee S-M, Booe JM, Pioszak AA. Structural insights into ligand recognition and selectivity for class A, B, and C GPCRs. Eur. J. Pharmacol. (2015) 763:196–205. doi: 10.1016/j.ejphar.2015.05.013
11. Bayburt TH, Leitz AJ, Xie G, Oprian DD, Sligar SG. Transducin activation by nanoscale lipid bilayers containing one and two rhodopsins. J Biol Chem. (2007) 282:14875–81. doi: 10.1074/jbc.M701433200
12. Whorton MR, Bokoch MP, Rasmussen SG, Huang B, Zare RN, Kobilka B, et al. A monomeric G protein-coupled receptor isolated in a high-density lipoprotein particle efficiently activates its G protein. Proc Natl Acad Sci USA. (2007) 104:7682–7. doi: 10.1073/pnas.0611448104
13. Kuszak AJ, Pitchiaya S, Anand JP, Mosberg HI, Walter NG, Sunahara RK. Purification and functional reconstitution of monomeric mu-opioid receptors: allosteric modulation of agonist binding by Gi2. J Biol Chem. (2009) 284:26732–41. doi: 10.1074/jbc.M109.026922
14. Goupil E, Laporte SA, Hébert TE. Functional selectivity in GPCR signaling: understanding the full spectrum of receptor conformations. Mini-Rev Med Chem. (2012) 12:817–30.
15. Zidar DA, Violin JD, Whalen EJ, Lefkowitz RJ. Selective engagement of G protein coupled receptor kinases (GRKs) encodes distinct functions of biased ligands. Proc Natl Acad Sci USA (2009) 106:9649–54. doi: 10.1073/pnas.0904361106
16. Limbird LE, Meyts PD, Lefkowitz RJ. Beta-adrenergic receptors: evidence for negative cooperativity. Biochem Biophys Res Commun. (1975) 64:1160–8. doi: 10.1016/0006-291X(75)90815-3
17. Agnati LF, Fuxe K, Zini I, Lenzi P, Hökfelt T. Aspects on receptor regulation and isoreceptor identification. Med Biol. (1980) 58:182–7.
18. Agnati LF, Fuxe K, Giardino L, Calzà L, Zoli M, Battistini N, et al. Evidence for cholecystokinin-dopamine receptor interactions in the central nervous system of the adult and old rat. Studies on their functional meaning. Ann. NY Acad. Sci. (1985) 448, 315–333.
19. Fuxe K, Agnati LF, Benfenati F, Celani M, Zini I, Zoli M, et al. Evidence for the existence of receptor-receptor interactions in the central nervous system. Studies on the regulation of monoamine receptors by neuropeptides. J Neural Transm. (1983) 18:165–79.
20. Fuxe K, Canals M, Torvinen M, Marcellino D, Terasmaa A, Genedani S, et al. Intramembrane receptor-receptor interactions: a novel principle in molecular medicine. J Neural Transm. (2007) 114:49–75. doi: 10.1007/s00702-006-0589-0
21. Fuxe K, Agnati LF. Receptor–receptor interactions in the central nervous system. A new integrative mechanism in synapses Med Res Rev. (1985) 5:441–82. doi: 10.1002/med.2610050404
22. Zoli M, Guidolin D, Fuxe K, Agnati LF. The receptor mosaic hypothesis of the engram: possible relevance of boolean network modeling. Int J Neural Syst. (1996) 7:363–8. doi: 10.1142/S0129065796000324
23. Agnati L, Zoli M, Merlo-Pich E, Benfenati F, Fuxe K. Aspects of neural plasticity in the central nervous system. VII Theoretical aspects of brain communication and computation Neurochem Int. (1990) 16:479–500. doi: 10.1016/0197-0186(90)90008-H
24. Agnati LF, Fuxe K. Volume transmission as a key feature of information handling in the central nervous system possible new interpretative value of the Turing's B-type machine. Prog Brain Res. (2000) 125:3–19. doi: 10.1016/S0079-6123(00)25003-6
25. Fraser CM, Venter JC. The size of the mammalian lung beta 2-adrenergic receptor as determined by target size analysis and immunoaffinity chromatography. Biochem Biophys Res Commun. (1982) 109:21–9. doi: 10.1016/0006-291X(82)91560-1
26. Paglin S, Jamieson JD. Covalent crosslinking of angiotensin II to its binding sites in rat adrenal membranes. Proc Natl Acad Sci USA (1982) 79:3739–43. doi: 10.1073/pnas.79.12.3739
27. Marshall FH, Jones KA, Kaupmann K, Bettler B. GABAB receptors – the first 7TM heterodimers. Trends Pharmacol Sci. (1999) 20:396–9. doi: 10.1016/S0165-6147(99)01383-8
28. Guidolin D, Agnati LF, Marcoli M, Borroto-Escuela D, Fuxe K. G-protein-coupled receptor type A heteromers as an emerging therapeutic target. Exp Opin Ther Targets (2015) 19:265–83. doi: 10.1517/14728222.2014.981155
29. Kniazeff J, Prezeau L, Rondard P, Pin JP, Goudet C. Dimers and beyond: the functional puzzles of class C GPCRs. Pharmacol Ther. (2011) 130:9–25. doi: 10.1016/j.pharmthera.2011.01.006
30. Roed SN, Orgaard A, Jorgensen R, De Meyts P. Receptor oligomerization in family B1 of G-protein-coupled receptors: focus on BRET investigations and the link between GPCR oligomerization and binding cooperativity. Front Endocrinol. (2012) 3:62. doi: 10.3389/fendo.2012.00062
31. Ng HK, Chow BK. Oligomerization of family B GPCRs: exploration in inter-family oligomer formation. Front Endocrinol. (2015) 6:10. doi: 10.3389/fendo.2015.00010
32. Milligan G. G protein-coupled receptor hetero-dimerization: contribution to pharmacology and function. Br J Pharmacol. (2009) 158:5–14. doi: 10.1111/j.1476-5381.2009.00169.x
33. Lambert NA, Javitch JA. Crosstalk opposing view: weighing the evidence for class A GPCR dimers, the jury is still out. J Physiol. (2014) 592:2443–5. doi: 10.1113/jphysiol.2014.272997
34. Bouvier M, Hebert TE. CrossTalk proposal: weighing the evidence for class A GPCR dimers, the evidence favours dimers. J Physiol. (2014) 592:2439–41. doi: 10.1113/jphysiol.2014.272252
35. Fotiadis D, Liang Y, Filipek S, Saperstein DA, Engel A, Palczewski K. Atomic force microscopy: rhodopsin dimers in native disc membranes. Nature (2003) 421:127–8. doi: 10.1038/421127a
36. Fung JJ, Deupi X, Pardo L, Yao XJ, Velez-Ruiz GA, Devree BT, et al. Ligand-regulated oligomerization of β2-adrenoceptors in a model lipid bilayer. EMBO J. (2009) 28:3315–28. doi: 10.1038/emboj.2009.267
37. Manglik A, Kruse AC, Kobilka TS, Thian FS, Mathiesen JM, Sunahara RK, et al. Crystal structure of the μ-opioid receptor bound to a morphinan antagonist. Nature (2012) 485:321–6. doi: 10.1038/nature10954
38. Franco R, Martinez-Pinilla E, Lanciego JL, Navarro G. Basic pharmacological and structural evidence for class A G-protein-coupled receptor heteromerization. Front Pharmacol. (2016) 7:76. doi: 10.3389/fphar.2016.00076
39. Gurevich VV, Gurevich EV. How and why do GPCRs dimerize? Trends Pharmacol Sci. (2008) 29:234–40. doi: 10.1016/j.tips.2008.02.004
40. Herrick-Davis K, Grinde E, Cowan A, Mazurkiewicz JE. Fluorescence correlation spectroscopy analysis of serotonin, adrenergic, muscarinic, and dopamine receptor dimerization: the oligomer number puzzle. Mol Pharmacol. (2013) 84:630–42. doi: 10.1124/mol.113.087072
41. Khelashvili G, Dorff K, Shan J, Camacho-Artacho M, Skrabanek L, Vroling B, et al. GPCR-OKB: the G protein-coupled receptor oligomer knowledge base. Bioinformatics (2010) 26:1804–5. doi: 10.1093/bioinformatics/btq264
42. Borroto-Escuela DO, Brito I, Romero-Fernandez W, Di Palma M, Oflijan J, Skieterska K, et al. The G protein- coupled receptor heterodimer network (GPCR-HetNet) and its hub components. Int J Mol Sci. (2014) 15:8570–859. doi: 10.3390/ijms15058570
43. Guidolin D, Marcoli M, Maura G, Agnati LF. New dimensions of connectomics and network plasticity in the central nervous system. Rev Neurosci. (2017) 28:113–32. doi: 10.1515/revneuro-2016-0051
44. Changeux JP, Christopoulos A. Allosteric modulation as a unifying mechanism for receptor function and regulation. Diabetes Obes Metab. (2017) 19:4–21. doi: 10.1111/dom.12959
45. Changeux JP. The origins of allostery: from personal memories to material for the future. J Mol Biol. (2013) 425:1396–406. doi: 10.1016/j.jmb.2013.02.033
46. Agnati LF, Fuxe K, Zoli M, Rondanini C, Ogren SO. New vistas on synaptic plasticity: the receptor mosaic hypothesis of the engram. Med Biol. (1982) 60:183–90.
47. Fuxe K, Marcellino D, Guidolin D, Woods AS, Agnati LF. Brain receptor mosaics and their intramembrane receptor-receptor interactions: molecular integration in transmission and novel target for drug development. J Acupunct Meridian Stud. (2009) 2:1–25. doi: 10.1016/S2005-2901(09)60011-X
48. Agnati LF, Guidolin D, Vilardaga JP, Ciruela F, Fuxe K. On the expanding terminology in the GPCR field: the meaning of receptor mosaics and receptor heteromers. J Receptor Signal Transduct Res. (2010) 30:287–303. doi: 10.3109/10799891003786226
49. Agnati LF, Guidolin D, Leo G, Fuxe K. A Boolean network modelling of receptor mosaics relevance of topology and cooperativity. J Neural Transm. (2007) 114:77–92. doi: 10.1007/s00702-006-0567-6
50. Fuxe K, Borroto-Escuela DO, Tarakanov A, Romero Fernandez W, Manger P, Rivera A, et al. Understanding the balance and integration of volume and synaptic transmission. Relevan Psychiatry Neurol Psychiatry Brain Res. (2013) 19:141–58. doi: 10.1016/j.npbr.2013.10.002
51. Fuxe K, Borroto-Escuela DO, Romero-Fernandez W, Palkovits M, Tarakanov AO, Ciruela F, et al. Moonlighting proteins and protein-protein interactions as neurotherapeutic targets in the G protein-coupled receptor field. Neuropsychopharmacol Rev. (2014) 39:131–55. doi: 10.1038/npp.2013.242
52. Gomes I, Fujita W, Chandrakala MV, Devi LA. Disease-specific heteromerization of G-protein-coupled receptors that target drugs of abuse. Prog Mol Biol Transl Sci. (2013) 117:207–65. doi: 10.1016/B978-0-12-386931-9.00009-X
53. Borroto-Escuela DO, Carlsson J, Ambrogini P, Narváez M, Wydra K, Tarakanov AO, et al. Understanding the role of GPCR heteroreceptor complexes in modulating the brain networks in health and disease. Front Cell Neurosci. (2017) 11:37. doi: 10.3389/fncel.2017.00037.eCollection2017
54. Ferré S, Casadò V, Devi LA, Filizola M, Jockers R, Lohse MJ, et al. G protein-coupled receptor oligomerization revisited: functional and pharmacological perspectives. Pharmacol Rev. (2014) 66, 413–434. doi: 10.1124/pr.113.008052
55. Fuxe K, Borroto-Escuela DO. Heteroreceptor complexes and their allosteric receptor-receptor interactions as a novel biological principle for integration of communication in the CNS: targets for drug development. Neuropsychopharmacology (2016) 41:380–2. doi: 10.1038/npp.2015.244
56. Sahlholm K, Gómez-Soler M, Valle-León M, Lopez-Cano M, Taura JJ, Ciruela F, et al. Antipsychotic-like efficacy of dopamine D2 receptor-biased ligands is dependent on adenosine A2A receptor expression. Mol Neurobiol. (2018) 55:4952–8. doi: 10.1007/s12035-017-0696-y
57. Fuxe K, Guidolin D, Agnati LF, Borroto-Escuela DO. Dopamine heteroreceptor complexes as therapeutic targets in Parkinson's disease. Exp Opin Ther Targets (2015) 19:377–98. doi: 10.1517/14728222.2014.981529
58. Bushlin I, Gupta A, Stockton SD Jr, Miller LK, Devi LA. Dimerization with cannabinoid receptors allosterically modulates δ opioid receptor activity during neuropathic pain. PLoS ONE (2012) 7:e49789. doi: 10.1371/journal.pone.0049789
59. Kern A, Albarran-Zeckler R, Walsh HE, Smith RG. Apo-ghrelin receptor forms heteromers with DRD2 in hypothalamic neurons and is essential for anorexigenic effects of DRD2 agonism. Neuron (2012) 73:317–32. doi: 10.1016/j.neuron.2011.10.038
60. Araque A, Parpura V, Sanzgiri RP, Haydon PG. Tripartite synapses: glia, the unacknowledged partner. Trends Neurosci. (1999) 22:208–15. doi: 10.1016/S0166-2236(98)01349-6
61. Nedergaard M, Verkhratsky A. Artifact versus reality-how astrocytes contribute to synaptic events. Glia (2012) 60:1013–23. doi: 10.1002/glia.22288
62. Hirase H, Qian L, Barth P, Buzsàki G. Calcium dynamics of cortical astrocytic networks in vivo. PLoS Biol. (2004) 2:E96. doi: 10.1371/journal.pbio.0020096
63. Volterra A, Meldolesi J. Astrocytes, from brain glue to communication elements: the revolution continues. Nat Rev Neurosci. (2005) 6:626–40. doi: 10.1038/nrn1722
64. Matos M, Augusto E, Agostinho P, Cunha RA, Chen JF. Antagonistic interaction between adenosine A2A receptors and Na+/K + -ATPase-a2 controlling glutamate uptake in astrocytes. J Neurosci. (2013) 33:18492–502. doi: 10.1523/JNEUROSCI.1828-13.2013
65. Miyazaki I, Asanuma M, Diaz-Corrales FJ, Miyoshi K, Ogawa N. Direct evidence for expression of dopamine receptors in astrocytes from basal ganglia. Brain Res. (2004) 1029:120–3. doi: 10.1016/j.brainres.2004.09.014
66. Matos M, Shen H-Y, Augusto E, Wang Y, Wei CJ, Wang YT, et al. Deletion of adenosine A2A receptors from astrocytes disrupts glutamate homeostasis leading to psychomotor and cognitive impairment: relevance to schizophrenia. Biol Psychiatry (2015) 78:763–74. doi: 10.1016/j.biopsych.2015.02.026
67. Shao W, Zhang SZ, Tang M, Zhang XH, Zhou Z, Yin YQ, et al. Suppression of neuroinflammation by astrocytic dopamine D2 receptors via alphaB-crystallin. Nature (2013) 494:90–4. doi: 10.1038/nature11748
68. Canals M, Marcellino D, Fanelli F, Ciruela F, de Benedetti P, Goldberg SR, et al. Adenosine A2A-dopamine D2 receptor-receptor heteromerization: qualitative and quantitative assessment by fluorescence and bioluminescence energy transfer. J Biol Chem. (2003) 278:46741–9. doi: 10.1074/jbc.M306451200
69. Kamiya T, Saitoh O, Yoshioka K, Nakata H. Oligomerization of adenosine A2A and dopamine D2 receptors in living cells. Biochem Biophys Res Commun. (2003) 306:544–9. doi: 10.1016/S0006-291X(03)00991-4
70. Fuxe K, Ferré S, Canals M, Torvinen M, Terasmaa A, Marcellino D, et al. Adenosine A2A and dopamine D2 heteromeric receptor complexes and their function. J. Mol. Neurosci. (2005) 26:209–20. doi: 10.1385/JMN:26:2-3:209
71. Azdad K, Gall D, Woods AS, Ledent C, Ferré S, Schiffmann SN. Dopamine D2 and adenosine A2A receptors regulate NMDA- mediated excitation in accumbens neurons through A2A-D2 receptor heteromerization. Neuropsychopharmacology (2009) 34, 972–986. doi: 10.1038/npp.2008.144
72. Diaz-Cabiale Z, Hurd Y, Guidolin D, Finnman UB, Zoli M, Agnati LF, et al. Adenosine A2A agonist CGS 21680 decreases the affinity of dopamine D2 receptors for dopamine in human striatum. NeuroReport (2001) 12:1831–4. doi: 10.1097/00001756-200107030-00014
73. Cervetto C, Venturini A, Passalacqua M, Guidolin D, Genedani S, Fuxe K, et al. A2A-D2 receptor-receptor interaction modulates gliotransmitter release from striatal astrocyte processes. J Neurochem. (2017) 140:268–79. doi: 10.1111/jnc.13885
74. Ciruela F, Burgueno J, Casado V, Canals M, Marcellino D, Goldberg SR, et al. Combining mass spectrometry and pull-down techniques for the study of receptor heteromerization. direct epitope-epitope electrostatic interactions between adenosine A2A and dopamine D2 receptors. Anal. Chem. (2004) 76:5354–5363. doi: 10.1021/ac049295f
75. Woods AS, Ciruela F, Fuxe K, Agnati LF, Lluis C, Franco R, et al. Role of electrostatic interaction in receptor-receptor heteromerization. J Mol Neurosci. (2005) 26:125–32. doi: 10.1385/JMN:26:2-3:125
76. Tonazzini I, Trincavelli ML, Storm-Mathisen J, Martini C, Bergersen LH. Co-localization and functional cross-talk between A1 and P2Y1 purine receptors in rat hippocampus. Eur J Neurosci. (2007) 26:890–902. doi: 10.1111/j.1460-9568.2007.05697.x
77. Tonazzini I, Trincavelli ML, Montali M, Martini C. Regulation of A1 adenosine receptor functioning induced by P2Y1 purinergic receptor activation in human astroglial cells. J Neurosci Res. (2008) 86:2857–66. doi: 10.1002/jnr.21727
78. Barki-Harrington L, Luttrell LM, Rockman HA. Dual inhibition of beta-adrenergic and angiotensin II receptors by a single antagonist: a functional role for receptor-receptor interaction in vivo. Circulation (2003) 108:1611–8. doi: 10.1161/01.CIR.0000092166.30360.78
79. AbdAlla S, Abdel-Baset A, Lother H, el Massiery A, Quitterer U. Mesangial AT1/B2 receptor heterodimers contribute to angiotensin II hyperresponsiveness in experimental hypertension. J. Mol. Neurosci. (2005) 26:185–92. doi: 10.1385/JMN:26:2-3:185
80. Nishimura A, Sunggip C, Tozaki-Saitoh H, Shimauchi T, Numaga-Tomita T, Hirano K, et al. Purinergic P2Y6 receptors heterodimerize with angiotensin AT1 receptors to promote angiotensin II-induced hypertension. Sci Signal. (2016) 9:ra7. doi: 10.1126/scisignal.aac9187
81. Bellot M, Galandrin S, Boularan C, Matthies HJ, Despas F, Denis C, et al. Dual agonist occupancy of AT1-R-a2C-AR heterodimers results in atypical Gs-PKA signaling. Nat. Chem. Biol. (2015) 11:271–9. doi: 10.1038/nchembio.1766
82. Rozenfeld R, Gupta A, Gagnidze K, Lim MP, Gomes I, Lee-Ramos D, et al. AT1R-CBR heteromerization reveals a new mechanism for the pathogenic properties of angiotensin II. EMBO J. (2011) 30:2350–63. doi: 10.1038/emboj.2011.139
83. Urizar E, Montanelli L, Loy T, Bonomi M, Swillens S, Gales C, et al. Glycoprotein hormone receptors: link between receptor homodimerization and negative cooperativity. EMBO J. (2005) 24:1954–64. doi: 10.1038/sj.emboj.7600686
84. Feng X, Zhang M, Guan R, Segaloff DL. Heterodimerization between the lutropin and follitropin receptors is associated with an attenuation of hormone-dependent signaling. Endocrinology (2013) 154:3925–30. doi: 10.1210/en.2013-1407
85. Mazurkiewicz JE, Herrick-Davis K, Barroso M, Ulloa-Aguirre A, Lindau-Shepard B, Thomas RM, et al. Single-molecule analyses of fully functional fluorescent protein-tagged follitropin receptor reveal homodimerization and specific heterodimerization with lutropin receptor. Biol Reprod. (2015) 92:100. doi: 10.1095/biolreprod.114.125781
86. Park S, Jiang H, Zhang H, Smith RG. Modification of ghrelin receptor signaling by somatostatin receptor-5 regulates insulin release. Proc Natl Acad Sci USA. (2012) 109:19003–8. doi: 10.1073/pnas.1209590109
87. Porzionato A, Stocco E, Guidolin D, Agnati LF, Macchi V, De Caro R. Receptor-receptor interactions of G protein-coupled receptors in the carotid body: a working hypothesis. Front Physiol. (2018) 9:697. doi: 10.3389/fphys.2018.00697
88. Takahashi K, Furukawa C, Takano A, Ishikawa N, Kato T, Hayama S, et al. The neuromedin U-growth hormone secretagogue receptor 1b/neurotensin receptor 1 oncogenic signaling path- way as a therapeutic target for lung cancer. Cancer Res. (2006) 66:9408–19. doi: 10.1158/0008-5472.CAN-06-1349
89. Moreno E, Andradas C, Medrano M, Caffarel MM, Pérez-Gómez E, Blasco-Benito S, et al. Targeting CB2-GPR55 receptor heteromers modulates cancer cell signaling. J Biol Chem. (2014) 289:21960–72. doi: 10.1074/jbc.M114.561761
90. Tòth AD, Turu G, Hunyady L, Balla A. Novel mechanisms of GPCR functions: AT1 angiotensin receptor acts as a signaling hub and focal point of receptor cross-talk. Best Pract Res Clin Endocrinol Metab. (2018) 32:69–82. doi: 10.1016/j.beem.2018.02.003
91. Daugherty A, Cassis L. Angiotensin II-mediated development of vascular diseases. Trends Cardiovasc Med. (2004) 14:117–20. doi: 10.1016/j.tcm.2004.01.002
92. Hunyady L, Catt KJ. Pleiotropic AT1 receptor signaling pathways mediating physiological and pathogenic actions of angiotensin II. Mol Endocrinol. (2006) 20:953–70. doi: 10.1210/me.2004-0536
93. Young BM, Nguyen E, Chedrawe MA, Rainev JK, Dupré DJ. Differential contribution of transmembrane domains IV, V, VI, and VII to human angiotensin II Type 1 receptor homomer formation. J. Biol. Chem. (2017) 292, 3341–3350. doi: 10.1074/jbc.M116.750380
94. Szalai B, Barkai L, Turu G, Szidonya L, Várnai P, Hunyady L. Allosteric interactions within the AT1 angiotensin receptor homodimer: role of the conserved DRY motif. Biochem. Pharmacol. (2012) 84, 477–485. doi: 10.1016/j.bcp.2012.04.014
95. Chun HJ, Ali ZA, Kojima Y, Kundu RK, Sheikh AY, Agrawal R, et al. Apelin signaling antagonizes Ang II effects in mouse models of atherosclerosis. J Clin Invest. (2008) 118:3343–54. doi: 10.1172/JCI34871
96. Fuxe K, Marcellino D, Borroto-Escuela DO, Frankowska M, Ferraro L, Guidolin D, et al. The changing world of G protein-coupled receptors: from monomers to dimers and receptor mosaics with allosteric receptor-receptor interactions. J Rec Signal Transduct. (2010) 30:272–83. doi: 10.3109/10799893.2010.506191
97. Quitterer U, Lother H, AbdAlla S. AT1 receptor heterodimers and angiotensin II responsiveness in preeclampsia. Semin Nephrol. (2004) 24:115–9. doi: 10.1016/j.semnephrol.2003.11.007
98. Ariza AC, Bobadilla NA, Nalhali A. Endothelin 1 and angiotensin II in preeclampsia. Rev Invest Clin. (2007) 59:48–56.
99. Friedman SL. Hepatic stellate cells: protean, multifunctional, and enigmatic cells of the liver. Physiol Rev. (2008) 88:125–72. doi: 10.1152/physrev.00013.2007
100. Kamal M, Jockers R. Biological significance of GPCR heteromerization in the neuro-endocrine system. Front Endocrinol. (2011) 2:2. doi: 10.3389/fendo.2011.00002
101. Evans NJ, Walker JW. Endothelin receptor dimers evaluated by FRET, ligand binding, and calcium mobilization. Biophys J. (2008) 95:483–92. doi: 10.1529/biophysj.107.119206
102. Derangeon M, Bozon V, Defamie N, Peineau N, Bourmeyster N, Sarrouilhe D, et al. 5-HT4 and 5-HT2 receptors antagonistically influence gap junctional coupling between rat auricular myocytes. J Mol Cell Cardiol. (2010) 48:220–9. doi: 10.1016/j.yjmcc.2009.07.005
103. Conde SV, Gonzalez C, Batuca JR, Monteiro EC, Obeso A. An antagonistic interaction between A2B adenosine and D2 dopamine receptors modulates the function of rat carotid body chemoreceptor cells. J Neurochem. (2008) 107:1369–81. doi: 10.1111/j.1471-4159.2008.05704.x
104. Conde SV, Obeso A, Monteiro EC, Gonzalez C. The A(2B)-D(2) receptor interaction that controls carotid body catecholamines release locates between the last two steps of hypoxic transduction cascade. Adv Exp Med Biol. (2009) 648:161–8. doi: 10.1007/978-90-481-2259-2_18
105. Jonas KC, Hanyaloglu AC. Impact of G protein-coupled receptor heteromers in endocrine systems. Mol Cell Endocrinol. (2017) 449:21–7. doi: 10.1016/j.mce.2017.01.030
106. Kleinau G, Müller A, Biebermann H. Oligomerization of GPCRs involved in endocrine regulation. J Mol Endocrinol. (2016) 57:859–80. doi: 10.1530/JME-16-0049
107. Satake H, Matsubara S, Aoyama M, Kawada T, Sakai T. GPCR heterodimerization in the reproductive system: functional regulation and implications for biodiversity. Front Endocrinol. (2013) 4:100. doi: 10.3389/fendo.2013.00100
108. Rivero-Muller A, Chou YY, Ji I, Lajic S, Hanyaloglu AC, Jonas K, et al. Rescue of defective G protein-coupled receptor function in vivo by intermolecular cooperation. Proc Natl Acad Sci USA (2010) 107:2319–24. doi: 10.1073/pnas.0906695106
109. Fan QR, Hendrickson WA. Structure of human follicle- stimulating hormone in complex with its receptor. Nature (2005) 433:269–77. doi: 10.1038/nature03206
110. Guan R, Wu X, Feng X, Zhang M, Hebert TE, Segaloff DL. Structural determinants underlying constitutive dimerization of unoccupied human follitropin receptors. Cell Signal (2010) 22:247–56. doi: 10.1016/j.cellsig.2009.09.023
111. Cui Q, Karplus M. Allostery and cooperativity revisited. Protein Sci. (2008) 17:1295–307. doi: 10.1110/ps.03259908
112. Laurent B, Murail S, Shahsavar A, Sauguet L, Delarue M, Baaden M. Sites of anesthetic inhibitory action on a cationic ligand-gated ion channel. Structure (2016) 24:595–605. doi: 10.1016/j.str.2016.02.014
113. Autzen HE, Myasnikov AG, Campbell MG, Asarnow D, Julius D, Cheng Y. Structure of the human TRPM4 ion channel in a lipid nanodisc. Science (2018) 359:228–32. doi: 10.1126/science.aar4510
114. Manas ES, Unwalla RJ, Xu ZB, Malamas MS, Miller CP, Harris HA, et al. Structure-based design of estrogen receptor-beta selective ligands. J Am Chem Soc. (2004) 126:15106–19. doi: 10.1021/ja047633o
115. Freed DM, Bessman NJ, Kiyatkin A, Salazar-Cavazos E, Byrne PO, Moore JO, et al. EGFR ligands differentially stabilize receptor dimers to specify signaling kinetics. Cell (2017) 171:683–95. doi: 10.1016/j.cell.2017.09.017
116. Huang J, Chen S, Zhang J, Huang X-Y. Crystal structure of oligomeric β1-adrenergic G protein-coupled receptors in ligand-free basal state. Nat Struct Mol Biol. (2013) 20:419–25. doi: 10.1038/nsmb.2504
117. Cordomì A, Navarro G, Aymerich MS, Franco R. Structures for G-protein-coupled receptor tetramers in complex with G-proteins. Trends Biochem. Sci. (2015) 40:548–51. doi: 10.1016/j.tibs.2015.07.007
118. Corringer P-J, Baaden M, Bocquet N, Delarue M, Dufresne V, Nury H, et al. Atomic structure and dynamics of pentameric ligand-gated ion channels: new insight from bacterial homologues. J Physiol. (2010) 588:565–72. doi: 10.1113/jphysiol.2009.183160
119. Baconguis I, Hattori M, Gouaux E. Unanticipated parallels in architecture and mechanism between ATP-gated P2X receptors and acid sensing ion channels. Curr Opin Struct Biol. (2013) 23:277–84. doi: 10.1016/j.sbi.2013.04.005
120. Catterall WA, Goldin AL, Waxman SG. International Union of Pharmacology. XLVII Nomenclature and structure-function relationships of voltage-gated sodium channels. Pharmacol Rev. (2005) 57:397–409. doi: 10.1124/pr.57.4.4
121. Islam MS. (ed.). (2011). Transient Receptor Potential Channels. Dordrecht: Springer. doi: 10.1007/978-94-007-0265-3
122. Cao E, Liao M, Cheng Y, Julius D. TRPV1 structures in distinct conformations reveal activation mechanisms. Nature (2013) 504:113–8. doi: 10.1038/nature12823
123. Burris TP, Solt LA, Wang Y, Crumbley C, Banerjee S, Griffett K, et al. Nuclear receptors and their selective pharmacologic modulators. Pharmacol Rev. (2013) 65:710–78. doi: 10.1124/pr.112.006833
124. Lemmon MA, Schlessinger J. Cell signaling by receptor tyrosine kinases. Cell (2010) 141:1117–34. doi: 10.1016/j.cell.2010.06.011
125. Lee J, Pilch PF. The insulin receptor: structure, function and signaling. Am J Physiol. (1994) 266:C319–34. doi: 10.1152/ajpcell.1994.266.2.C319
126. Zahavi EE, Steinberg N, Altman T, Chein M, Joshi Y, Gradus-Pery T, et al. The receptor tyrosine kinase TrkB signals without dimerization at the plasma membrane. Sci Signal. (2018) 11:eaao4006. doi: 10.1126/scisignal.aao4006
127. Ullrich A, Schlessinger J. Signal transduction by receptors with tyrosine kinase activity. Cell (1990) 61:203–12. doi: 10.1016/0092-8674(90)90801-K
128. Endres NF, Barros T, Cantor AJ, Kuriyan J. Emerging concepts in the regulation of the EGF receptor and other receptor tyrosine kinases. Trends Biochem Sci. (2014) 39:437–46. doi: 10.1016/j.tibs.2014.08.001
129. Oates J, King G, Dixon AM. Strong oligomerization behavior of PDGF beta receptor transmembrane domain and its regulation by the juxtamembrane regions. Biochim Biophys Acta (2010) 1798:605–15. doi: 10.1016/j.bbamem.2009.12.016
130. Saito Y, Haendeler J, Hojo Y, Yamamoto K, Berk BC. Receptor heterodimerization: essential mechanism for platelet-derived growth factor-induced epidermal growth factor receptor transactivation. Mol Cell Biol. (2001) 21:6387–94. doi: 10.1128/MCB.21.19.6387-6394.2001
131. Guidolin D, Albertin G, Spinazzi R, Sorato E, Mascarin A, Cavallo D, et al. Adrenomedullin stimulates angiogenic response in cultured human vascular endothelial cells: involvement of the vascular endothelial growth factor receptor 2. Peptides (2008) 29:2013–23. doi: 10.1016/j.peptides.2008.07.009
132. Köse M. GPCRs and EGFR – cross-talk of membrane receptors in cancer. Bioorg Med Chem Lett. (2017) 27:3611–20. doi: 10.1016/j.bmcl.2017.07.002
133. Borroto-Escuela DO, Romero-Fernandez W, Mudò G, Pérez-Alea M, Ciruela F, Tarakanov AO, et al. Fibroblast growth factor receptor 1-5-hydroxytryptamine 1A heteroreceptor complexes and their enhancement of hippocampal plasticity. Biol. Psychiatry (2012) 71:84–91. doi: 10.1016/j.biopsych.2011.09.012
134. Di Liberto V, Borroto-Escuela DO, Frinchi M, Verdi V, Fuxe K, Belluardo N, et al. Existence of muscarinic acetylcholine receptor (mAChR) and fibroblast growth factor receptor (FGFR) heteroreceptor complexes and their enhancement of neurite outgrowth in neural hippocampal cultures. Biochim Biophys Acta (2017) 1861:235–45. doi: 10.1016/j.bbagen.2016.10.026
135. Liu T, Whitten ST, Hilser VJ. Ensemble-based signatures of energy propagation in proteins: a new view of an old phenomenon. Proteins (2006) 62:728–38. doi: 10.1002/prot.20749
136. Gamo NJ, Lur G, Higley MJ, Wang M, Paspalas CD, Vijairhagavan S, et al. Stress impairs prefrontal cortical function via D1 dopamine receptor interactions with HCN channels. Biol Psychiatry (2015) 78:860–70. doi: 10.1016/j.biopsych.2015.01.009
137. Kenakin T, Miller LJ. Seven transmembrane receptors as shape shifting proteins: the impact of allosteric modulation and functional selectivity on new drug discovery. Pharmacol Rev. (2010) 62:265–304. doi: 10.1124/pr.108.000992
138. Smith NJ, Milligan G. Allostery at G protein-coupled receptor homo- and heteromers: uncharted pharmacological landscapes. Pharmacol Rev. (2010) 62:701–25. doi: 10.1124/pr.110.002667
139. Liu J, Nussinov R. Allostery: an overview of its history, concepts, methods and applications. PLoS Comput Biol. (2016) 12:e1004966. doi: 10.1371/journal.pcbi.1004966
140. Liu J, Nussinov R. Energetic redistribution in allostery to execute protein function. Proc Natl Acad Sci USA (2017) 114:7480–2. doi: 10.1073/pnas.1709071114
141. Christopoulos A, Kenakin T. G protein-coupled receptor allosterism and complexing. Pharmacol Rev. (2002) 54:323–74. doi: 10.1124/pr.54.2.323
142. Agnati LF, Leo G, Genedani S, Andreoli N, Marcellino D, Woods A, et al. Structural plasticity in G-protein coupled receptors as demonstrated by the allosteric actions of homocysteine and computer-assisted analysis of disordered domains. Brain Res Rev. (2008) 58:459–74. doi: 10.1016/j.brainresrev.2007.10.003
143. Wright PE, Dyson HJ. Intrinsically disordered proteins in cellular signalling and regulation. Nat Rev Mol Cell Biol. (2015) 16:18–29. doi: 10.1038/nrm3920
144. Shan Y, Eastwood MP, Zhang X, Kim ET, Arkhipov A, Dror RO, et al. Oncogenic mutations counteract intrinsic disorder in the EGFR kinase and promote receptor dimerization. Cell (2012) 149:860–70. doi: 10.1016/j.cell.2012.02.063
145. Simons SS Jr, Edwards DP, Kumar R. Minireview: dynamic structures of nuclear hormone receptors: new promises and challenges. Mol Endocrinol. (2014) 28:173–82. doi: 10.1210/me.2013-1334
146. Tovo-Rodrigues L, Roux A, Hutz MH, Rohde LA, Woods AS. Functional characterization of G-protein-coupled receptors: a bioinformatics approach. Neuroscience (2014) 277:764–79. doi: 10.1016/j.neuroscience.2014.06.049
147. Agnati LF, Guidolin D, Leo G, Carone C, Genedani S, Fuxe K. Receptor-receptor interactions: a novel concept in brain integration. Prog. Neurobiol. (2010) 90:157–75. doi: 10.1016/j.pneurobio.2009.10.004
148. Skrabanek L, Murcia M, Bouvier M, Devi L, George SR, Lohse MJ, et al. Requirements and ontology for a G protein-coupled receptor oligomerization knowledge base. BMC Bioinform. (2007) 8:177. doi: 10.1186/1471-2105-8-177
149. Miller PS, Aricescu AR. Crystal structure of a human GABAA receptor. Nature (2014) 512:270–5. doi: 10.1038/nature13293
150. Hausmann R, Kless A, Schmalzing G. Key sites for P2X receptor function and multimerization: overview of mutagenesis studies on a structural basis. Curr Med Chem. (2015) 22:799–818. doi: 10.2174/0929867322666141128163215
151. Greger IH, Watson JF, Cull-Candy SG. Structural and functional architecture of AMPA-type glutamate receptors and their auxiliary proteins. Neuron (2017) 94:713–30. doi: 10.1016/j.neuron.2017.04.009
152. Xu J, Yu W, Jan YN, Jan LY, Li M. Assembly of voltage-gated potassium channels. Conserved hydrophilic motifs determine subfamily-specific interactions between the alpha-subunits J Biol Chem. (1995) 270:24761–8. doi: 10.1074/jbc.270.42.24761
153. Erler I, Hirnet D, Wissenbach U, Flockerzi V, Niemeyer BA. Ca2+-selective transient receptor potential V channel architecture and function require a specific ankyrin repeat. J Biol Chem. (2004) 279:34456–68. doi: 10.1074/jbc.M404778200
154. Beato M, Klug J. Steroid hormone receptors: an update. Hum Reprod Update (2000) 6:225–36. doi: 10.1093/humupd/6.3.225
155. Roemer SC, Donham DC, Sherman L, Pon VH, Edwards DP, Churchill MEA. Structure of the progesterone receptor-deoxyribonucleic acid complex: novel interactions required for binding to half-site response elements. Mol. Endocrinol. (2006) 20:3042–52. doi: 10.1210/me.2005-0511
156. Li E, Hristova K. Receptor tyrosine kinase transmembrane domains. Cell Adh Migr. (2010) 4:249–54. doi: 10.4161/cam.4.2.10725
157. Sarabipour S, Hristova K. Mechanism of FGF receptor dimerization and activation. Nat Comm. (2016) 7:10262. doi: 10.1038/ncomms10262
158. Bocharov EV, Lesovoy DM, Goncharuk SA, Goncharuk MV, Hristova K, Arseniev AS, et al. Structure of FGFR3 transmembrane domain dimer: implications for signaling and human pathologies. Structure (2013) 21:2087–93. doi: 10.1016/j.str.2013.08.026
159. Guidolin D, Ciruela F, Genedani S, Guescini M, Tortorella C, Albertin G, et al. Bioinformatics and mathematical modelling in the study of receptor-receptor interactions and receptor oligomerization. Focus on adenosine receptors Biochim Biophys Acta (2011) 1808:1267–83. doi: 10.1016/j.bbamem.2010.09.022
160. Grisshammer R. New approaches towards the understanding of integral membrane proteins: a structural perspective on G protein-coupled receptors. Protein Sci. (2017) 26:1493–504. doi: 10.1002/pro.3200
161. Jonas KC, Huhtaniemi I, Hanyaloglu AC. Single-molecule resolution of G protein-coupled receptor (GPCR) complexes. Methods Cell Biol. (2016) 132:55–72. doi: 10.1016/bs.mcb.2015.11.005
162. Thevenin D, Lazarova T. Stable interactions between the transmembrane domains of the adenosine A2A receptor. Protein Sci. (2008) 17:1188–99. doi: 10.1110/ps.034843.108
163. Navarro G, Cordomi A, Zelman-Femiak M, Brugarolas M, Moreno E, Aguinaga D, et al. Quaternary structure of a G-protein-coupled receptor heterotetramer in complex with Gi and Gs. BMC Biol. (2016) 14:26. doi: 10.1186/s12915-016-0247-4
164. Borroto-Escuela DO, Rodriguez D, Romero-Fernandez W, Kapla J, Jalteh M, Ranganathan A, et al. Mapping the interface of a GPCR dimer: a structural model of the A2A adenosine and D2 dopamine receptor heteromer. Front Pharmacol. (2018) 9:829. doi: 10.3389/fphar.2018.00829
165. Navarro G, Ferré S, Cordomi A, Moreno E, Mallol J, Casado V, et al. Interactions between intracellular domains as key determinants of the quaternary structure and function of receptor heteromers. J. Biol. Chem. (2010) 285:27346–59. doi: 10.1074/jbc.M110.115634
166. Hernanz-Falcon P, Rodriguez-Frade JM, Serrano A, Juan D, del Sol A, Soriano SF, et al. Identification of amino acid residues crucial for chemokine receptor dimerization. Nat Immunol (2004) 5:216–23. doi: 10.1038/ni1027
167. Guo W, Urizar E, Kralikova M, Mobarec JC, Shi L, Filizola M, et al. Dopamine D2 receptors form higher order oligomers at physiological expression levels. EMBO J. (2008) 27:2293–304. doi: 10.1038/emboj.2008.153
168. Johnston JM, Aburi M, Provasi D, Bortolato A, Urizar E, Lambert NA, et al. Making structural sense of dimerization interfaces of delta opioid receptor homodimers. Biochemistry (2011) 50:1682–90. doi: 10.1021/bi101474v
169. Borroto-Escuela DO, Correia PA, Perez Alea M, Narvaez M, Garriga P, Fuxe K, et al. Impaired M(3) muscarinic acetylcholine receptor signal transduction through blockade of binding of multiple proteins to its third intracellular loop. Cell Physiol Biochem. (2010) 25:397–408. doi: 10.1159/000303044
170. Woods AS, Ferré S. Amazing stability of the arginine-phosphate electrostatic interaction. Proteome Res. (2005) 4:1397–402. doi: 10.1021/pr050077s
171. Woods AS, Jackson SN. How adenylate cyclase choreographs the pas de deux of the receptors heteromerization dance. Neuroscience (2013) 238:335–44. doi: 10.1016/j.neuroscience.2013.02.006
172. Lock A, Forfar R, Weston C, Bowsher L, Upton GJG, Reynolds CA, et al. One motif to bind them: a small-XXX-small motif affects transmembrane domain 1 oligomerization, function, localization and cross-talk between two yeast GPCR. Biochim Biophys Acta (2014) 1838:3036–51. doi: 10.1016/j.bbamem.2014.08.019
173. Tarakanov AO, Fuxe KG. Triplet puzzle: homologies of receptor heteromers. J Mol Neurosci. (2010) 41:294–303. doi: 10.1007/s12031-009-9313-5
174. Borroto-Escuela DO, Tarakanov AO, Guidolin D, Ciruela F, Agnati LF, Fuxe K. Moonlighting characteristics of G protein-coupled receptors: focus on receptor heteromers and relevance for neurodegeneration. IUBMB Life (2011) 63:463–72. doi: 10.1002/iub.473
175. Gandia J, Galino J, Amaral OB, Soriano A, Lluìs C, Franco R, et al. Detection of higher-order G protein- coupled receptor oligomers by a combined BRET-BiFC technique. FEBS Lett. (2008) 582:2979–84. doi: 10.1016/j.febslet.2008.07.045
176. Park PS, Wells JW. Oligomeric potential of the M2 muscarinic cholinergic receptor. J Neurochem. (2004) 90:537–48. doi: 10.1111/j.1471-4159.2004.02536.x
177. Cabello N, Gandìa J, Bertarelli DC, Watanabe M, Lluis C, Franco R, et al. Metabotropic glutamate type 5, dopamine D2 and adenosine A2a receptors form higher-order oligomers in living cells. J Neurochem. (2009) 109:1497–507. doi: 10.1111/j.1471-4159.2009.06078
178. Tena-Campos M, Ramon E, Borroto-Escuela DO, Fuxe K, Garriga P. The zinc binding receptor GPR39 interacts with 5-HT1A and GalR1 to form dynamic heteroreceptor complexes with signaling diversity. Biochim Biophys Acta (2015) 1852:2585–92. doi: 10.1016/j.bbadis.2015.09.003
179. Millón C, Flores-Burgess A, Narváez M, Borroto-Escuela DO, Santín L, Gago B, et al. Galanin (1–15) enhances the antidepressant effects of the 5-HT1A receptor agonist 8-OH-DPAT. Involvement of the raphe- hippocampal 5-HT neuron system. Brain Struct. Funct. (2016) 221:4491–504. doi: 10.1007/s00429-015-1180-y
180. Scarselli M, Annibale P, McCormick PJ, Kolachalam S, Aringhieri S, Radenovic A, et al. Revealing G-protein-coupled receptor oligomerization at the single-molecule level through a nanoscopic lens: methods, dynamics and biological function. FEBS J. (2016) 283:1197–217. doi: 10.1111/febs.13577
181. Agnati LF, Fuxe K, Woods AS, Genedani S, Guidolin D. Theoretical considerations on the topological organization of receptor mosaics. Curr Prot Pept Sci. (2009) 10:559–69. doi: 10.2174/138920309789630606
182. Agnati LF, Guidolin D, Albertin G, Trivello E, Ciruela F, Genedani S, et al. An integrated view on the role of receptor mosaics at perisynaptic level: focus on adenosine A2A, dopamine D2, cannabinoid CB1, and metabotropic glutamate mGlu5 receptors. J Rec Signal Transduct. (2010) 30:355–69. doi: 10.3109/10799893.2010.487492
183. Jonas KC, Fanelli F, Huhtaniemi IT, Hanyaloglu AC. Single molecule analysis of functionally asymmetric G protein-coupled receptor (GPCR) oligomers reveals diverse spatial and structural assemblies. J Biol Chem. (2015) 290:3875–92. doi: 10.1074/jbc.M114.622498
184. Fuxe K, Borroto-Escuela DO, Marcellino D, Romero-Fernandez W, Frankowska M, Guidolin D, et al. GPCR heteromers and their allosteric receptor-receptor interactions. Curr Med Chem. (2012) 19:356–63. doi: 10.2174/092986712803414259
185. Jackson MB. Molecular and Cellular Biophysics. Cambridge, UK: Cambridge University Press (2006). doi: 10.1017/CBO9780511754869
186. Kenakin T. Cellular assays as portals to seven-transmembrane receptor-based drug discovery. Nat Rev Drug Discov. (2009) 8:617–26. doi: 10.1038/nrd2838
187. Romero GG. The role of the cell background in biased signaling. In: Arey BJ. editor. Biased Signaling in Physiology, Pharmacology and Therapeutics. San Diego, CA: Elsevier (2014). doi: 10.1016/B978-0-12-411460-9.00002-1
188. Fuxe K, Ferré S, Zoli M, Agnati LF. Integrated events in central dopamine transmission as analyzed at multiple levels. Evidence for intramembrane adenosine A2A/dopamine D2 and adenosine A1/dopamine D1 receptor interactions in the basal ganglia. Brain Res. Rev. (1998) 26, 258–273.
189. Kull B, Ferré S, Arslan G, Svenningsson P, Fuxe K, Owman C, et al. Reciprocal interactions between adenosine A2A and dopamine D2 receptors in Chinese hamster ovary cells co-transfected with the two receptors. Biochem. Pharmacol. (1999) 58:1035–45.
190. El-Asmar L, Springael JY, Ballet S, Andrieu EU, Vassart G, Parmentier M. Evidence for negative binding cooperativity within CCR5-CCR2b heterodimers. Mol Pharmacol. (2005) 67:460–9. doi: 10.1124/mol.104.003624
191. Andersen M, Nørgaard-Pedersen D, Brandt J, Pettersson I, Slaabi R. IGF1 and IGF2 specificities to the two insulin receptor isoforms are determined by insulin receptor amino acid 718. PLoS ONE (2017) 12:e0178885. doi: 10.1371/journal.pone.0178885
192. Costa-Neto CM, Parreiras-e-Silva LT, Bouvier M. A pluridimensional view of biased agonism. Mol. Pharmacol. (2017) 90:587–96. doi: 10.1124/mol.116.105940
193. Pupo AS, Duarte DA, Lima V, Teixeira LB, Parreira-e-Silva LT, Costa-Neto CM. Recent updates on GPCR biased agonism. Pharmacol. Res. (2016) 112:49–57. doi: 10.1016/j.phrs.2016.01.031
194. Ferrada C, Moreno E, Casadò V, Bongers G, Cortés A, Mallol J, et al. Marked changes in signal transduction upon heteromerization of dopamine D1 and histamine H3 receptors. Br. J. Pharmacol. (2009) 157:64–75. doi: 10.1111/j.1476-5381.2009.00152.x
195. Rashid AJ, So CH, Kong MM, Furtak T, El-Ghundi M, Cheng R, et al. D1-D2 dopamine receptor heterooligomers with unique pharmacology are coupled to rapid activation of Gq/11 in the striatum. Proc Natl Acad Sci USA (2007) 104:654–59. doi: 10.1073/pnas.0604049104
196. Rozenfeld R, Bushlin I, Gomes I, Tzavaras N, Gupta A, Neves S, et al. Receptor heteromerization expands the repertoire of cannabinoid signaling in rodent neurons. PLoS ONE (2012) 7:e29239. doi: 10.1371/journal.pone.0029239
197. Le Naour M, Lunzer MM, Powers MD, Kalyuzhny AE, Benneyworth MA, Thomas MJ, et al. Putative kappa opioid heteromers as targets for developing analgesics free of adverse effects. J Med Chem. (2014) 57:6383–92. doi: 10.1021/jm500159d
198. Bartuzi D, Kaczor A, Matosiuk D. Signaling within allosteric machines: signal transmission pathways inside G protein-coupled receptors. Molecules (2017) 22:1188. doi: 10.3390/molecules22071188
199. Wu H, Wang C, Gregory KJ, Won Han G, Cho HP, Xia Y, et al. Structure of a class C GPCR metabotropic glutamate receptor 1 bound to an allosteric modulator. Science (2014) 344:58–64. doi: 10.1126/science.1249489
200. Shivnaraine RV, Kelly B, Sankar KS, Redka D, Han YR, Huang F, et al. Allosteric modulation in monomers and oligomers of a G protein-coupled receptor. eLife (2016) 2016:e11685. doi: 10.7554/eLife.11685
201. Agnati LF, Ferré S, Genedani S, Leo G, Guidolin D, Filaferro M, et al. Allosteric modulation of dopamine D2 receptors by homocysteine. J. Proteome Res. (2006) 5:3077–83. doi: 10.1021/pr0601382
202. Cervetto C, Venturini A, Guidolin D, Maura G, Passalacqua M, Tacchetti C, et al. Homocysteine and A2A-D2 receptor-receptor interaction at striatal astrocyte processes. J Mol Neurosci. (2018) 65:456–66. doi: 10.1007/s12031-018-1120-4
203. Lyskov S, Chou FC, Conchúir SÓ, Der BS, Drew K, Kuroda D, et al. Serverification of molecular modeling applications: the rosetta online server that includes everyone (ROSIE). PLoS ONE (2013) 8:e63906. doi: 10.1371/journal.pone.006390
204. Liu W, Chun E, Thompson AA, Chubukov P, Xu F, Katritch V, et al. Structural basis for allosteric regulation of GPCRs by sodium ions. Science (2012) 6091:232–6. doi: 10.1126/science.1219218
205. Wang S, Che T, Levit A, Stoichet BK, Wacker D, Roth BL. Structure of the D2 dopamine receptor bound to the atypical antipsychotic drug risperidone. Nature (2018) 555:269–73. doi: 10.1038/nature25758
206. Bockaert J, Pin JP. Molecular tinkering of G protein-coupled receptors: an evolutionary success. EMBO J. (1999) 18:1723–9. doi: 10.1093/emboj/18.7.1723
207. Franco R, Ciruela F, Casado V, Cortes A, Canela EI, Mallol J, et al. Partners for adenosine A1 receptors. J Mol Neurosci. (2005) 26:221–32. doi: 10.1385/JMN:26:2-3:221
208. Foord SM, Marshall FH. RAMPs: accessory proteins for seven transmembrane domain receptors. Trends Pharmacol Sci. (1999) 20:184–7. doi: 10.1016/S0165-6147(99)01347-4
209. Poyner DR, Sexton PM, Marshall J, Smith DM, Quiron R, Born W, et al. International Union of Pharmacology. XXXII. 2002. the mammalian calcitonin gene-related peptides, adrenomedullin, amylin and calcitonin receptors. Pharmacol. Rev. (2002) 54:233–46. doi: 10.1124/pr.54.2.233
210. Araud T, Wonnacott S, Bertrand D. Associated proteins: the universal toolbox controlling ligand gated ion channel function. Biochem Pharmacol. (2010) 80:160–9. doi: 10.1016/j.bcp.2010.03.017
211. Alemany R, Perona JS, Sánchez-Dominguez JM, Montero E, Cañizares J, Bressani R, et al. G protein-coupled receptor systems and their lipid environment in health disorders during aging. Biochim Biophys Acta (2007) 1768:964–75. doi: 10.1016/j.bbamem.2006.09.024
212. Killian J. Hydrophobic mismatch between proteins and lipids in membranes. BBA RevBiomembr. (1998) 1376:401–16. doi: 10.1016/S0304-4157(98)00017-3
213. Black J. Drugs from emasculated hormones: the principle of syntopic antagonism. Science (1989) 245:486–93. doi: 10.1126/science.2569237
214. Gomes I, Gupta A, Bushlin I, Devi LA. Antibodies to probe endogenous G protein-coupled receptor heteromer expression, regulation, and function. Front Pharmacol. (2014) 3:268. doi: 10.3389/fphar.2014.00268
215. Gomes I, Fujita W, Gupta A, Saldanha SA, Negri A, Pinello CE, et al. Identification of a μ-δ opioid receptor heteromer-biased agonist with antinociceptive activity. Proc Natl Acad Sci USA (2013) 110:12072–7. doi: 10.1073/pnas.1222044110
Keywords: allosteric modulation, oligomerization, receptors, GPCR, signaling, pharmacology
Citation: Guidolin D, Marcoli M, Tortorella C, Maura G and Agnati LF (2019) Receptor-Receptor Interactions as a Widespread Phenomenon: Novel Targets for Drug Development? Front. Endocrinol. 10:53. doi: 10.3389/fendo.2019.00053
Received: 24 October 2018; Accepted: 21 January 2019;
Published: 18 February 2019.
Edited by:
Anne-Marie Baird, St. James's Hospital, IrelandReviewed by:
Gunnar Kleinau, Charité Medical University of Berlin, GermanyCopyright © 2019 Guidolin, Marcoli, Tortorella, Maura and Agnati. This is an open-access article distributed under the terms of the Creative Commons Attribution License (CC BY). The use, distribution or reproduction in other forums is permitted, provided the original author(s) and the copyright owner(s) are credited and that the original publication in this journal is cited, in accordance with accepted academic practice. No use, distribution or reproduction is permitted which does not comply with these terms.
*Correspondence: Diego Guidolin, ZGllZ28uZ3VpZG9saW5AdW5pcGQuaXQ=
Disclaimer: All claims expressed in this article are solely those of the authors and do not necessarily represent those of their affiliated organizations, or those of the publisher, the editors and the reviewers. Any product that may be evaluated in this article or claim that may be made by its manufacturer is not guaranteed or endorsed by the publisher.
Research integrity at Frontiers
Learn more about the work of our research integrity team to safeguard the quality of each article we publish.