- Nutrigenomics and Fish Growth Endocrinology, Institute of Aquaculture Torre de la Sal (IATS-CSIC), Castellón, Spain
The Gh/Prl/Sl family has evolved differentially through evolution, resulting in varying relationships between the somatotropic axis and growth rates within and across fish species. This is due to a wide range of endogenous and exogenous factors that make this association variable throughout season and life cycle, and the present minireview aims to better define the nutritional and environmental regulation of the endocrine growth cascade over precisely defined groups of fishes, focusing on Mediterranean farmed fishes. As a result, circulating Gh and Igf-i are revitalized as reliable growth markers, with a close association with growth rates of gilthead sea bream juveniles with deficiency signs in both macro- or micro-nutrients. This, together with other regulated responses, promotes the use of Gh and Igf-i as key performance indicators of growth, aerobic scope, and nutritional condition in gilthead sea bream. Moreover, the sirtuin-energy sensors might modulate the growth-promoting action of somatotropic axis. In this scenario, transcripts of igf-i and gh receptors mirror changes in plasma Gh and Igf-i levels, with the ghr-i/ghr-ii expression ratio mostly unaltered over season. However, this ratio is nutritionally regulated, and enriched plant-based diets or diets with specific nutrient deficiencies downregulate hepatic ghr-i, decreasing the ghr-i/ghr-ii ratio. The same trend, due to a ghr-ii increase, is found in skeletal muscle, whereas impaired growth during overwintering is related to increase in the ghr-i/ghr-ii and igf-ii/igf-i ratios in liver and skeletal muscle, respectively. Overall, expression of insulin receptors and igf receptors is less regulated, though the expression quotient is especially high in the liver and muscle of sea bream. Nutritional and environmental regulation of the full Igf binding protein 1–6 repertoire remains to be understood. However, tissue-specific expression profiling highlights an enhanced and nutritionally regulated expression of the igfbp-1/-2/-4 clade in liver, whereas the igfbp-3/-5/-6 clade is overexpressed and regulated in skeletal muscle. The somatotropic axis is, therefore, highly informative of a wide-range of growth-disturbing and stressful stimuli, and multivariate analysis supports its use as a reliable toolset for the assessment of growth potentiality and nutrient deficiencies and requirements, especially in combination with selected panels of other nutritionally regulated metabolic biomarkers.
Expansion of Gh/Prl/Sl Family
The fish growth hormone (Gh) and prolactin (Prl) family was initially expanded with the discovery of somatolactin (Sl) in olive flounder (1) and Atlantic cod (2). Thereafter, Sl has been identified, purified or recombinantly produced from a multitude of teleost species, including chum salmon (3), sole (4, 5), gilthead sea bream (6, 7), goldfish (8), eel (9), rainbow trout (10), and European sea bass (11, 12). Sl has also been identified in primitive fishes, such as the white sturgeon and the West African lungfish (13), which suggests that the ancestors of Gh and Sl were present before the divergence of lobe-finned fishes (Sarcopteygii) and ray finned fishes (Actinopterygii). Thereafter, the sl gene was duplicated in the basal teleost tetraploidization (3R), giving rise to slα and slβ, which were first identified in zebrafish (14) and Atlantic salmon (15). However, the slβ gene was lost from the lineage, leading to spiny-rayed fishes (Acanthomorpha), so it is not found in the most diverse and species-rich group of modern teleosts (16). Conversely, Prl2, the last member of the fish Gh/Prl/Sl family, has been identified in almost all non-mammalian vertebrate species ranging from cartilaginous fishes to tetrapods (17, 18). Despite this, both the sl and prl2 genes were lost twice independently in tetrapods: once at the base of the amphibian lineage and once early in mammalian evolution (16, 19).
At a closer look, proteins of the Gh/Prl/Sl family share a common genomic organization with five to six exons in almost all fish species examined, including cyprinids, salmonids, flat fishes and Perciformes (20–22). Another characteristic feature is the cysteine residues involved in disulfide bridges, one linking distant parts of the polypeptide chain and another forming a loop close to the C-terminus that are strictly retained through vertebrate evolution. However, an additional N-terminal disulphide loop appeared in the Sl and Prl/Prl2 branches, prior to the divergence of bony fishes from the lineage leading to tetrapods, and thereafter the specific sl and prl2 deletion events occurred between lungfish and amphibian lineages (23). In this context, the precise timing of gene losses and early duplication events is difficult to establish, though analyses of phylogeny and conserved synteny suggest that the gh and prl/prl2 genes arose likely from a local duplication before 1R tetraploidization, and a second local gene duplication within the same time window gave rise to sl (16, 24) (see Figure 1).
Synteny approaches also reveal that the Gh receptors (Ghr) and Prl receptors (Prlr) are located on the same chromosome, probably as the result of an early local gene duplication (25). By contrast, the Sl receptors (Slr), identified as belonging to the Ghr-i clade, might have arisen much later in the 3R tetraploidization. This would be an example of subfunctionalization, where the named Ghr-i and Ghr-ii might have evolved for differential ligand binding preferences for Sl or Gh, respectively. However, in contrast to the ligand binding study in masu salmon (26), subsequent studies in black sea bream (27), and zebrafish (28) did not reveal differences between Ghr-i and Ghr-ii in terms of ligand binding affinities. Moreover, Ghr-i preferentially binds Gh rather than Sl in Japanese eel (29) and trout (30) binding assays. It appears, therefore, that the observed fish species differences in Ghr binding are more likely to be due to the different natures of Gh/Sl preparations or other factors that are hitherto unknown. Moreover, Bergan-Roller and Sheridan (31) pointed out that one Ghr would be more responsive for transmitting lipolytic or stress signals of Gh/Sl, while the other Ghr subtype would be more active in transmitting growth-promoting signals. In this context, it is of relevance to define clear patterns of Ghr expression in combination with changes in other growth- and metabolic-related factors and, more importantly, how the somatotropic axis is affected as a whole by the advent of new rearing systems and diet formulations for the intensification of fish farming in the 2030 horizon. In this regard, the aim of this minireview is to update the environmentally-mediated changes in fish somatotropic axis activity, linking them with a more accurate phenotyping of fish nutritional and metabolic status through the development and productive cycles. Attention is focused on marine fishes with special emphasis on gilthead sea bream (Sparus aurata) as the most important farmed fish of the Mediterranean aquaculture.
Fish Gh/Sl Sub-Functionalization
Life-history decisions are not fixed and often depend on critical size and sufficient energy at a specific stage (“opportunity window”) several months prior to biological transformations. For instance, the decisive factor in salmonids to become smolts or sexually mature is linked to growth and fat depositions at mid-summer and spring (32, 33). Thus, plasma Gh levels in most fishes, including gilthead sea bream, peak at late spring and early summer, whereas the peaks of Prl and Sl are delayed to mid-summer and autumn, respectively (20, 34). Therefore, Gh, Prl and Sl are differentially regulated on a seasonal basis, but also in response to energy availability (Figure 2). Certainly, circulating Sl increases transitorily with fasting in gilthead sea bream, but, opposite to Gh, a persistent increase in plasma Sl is found with the increase in adiposity in overfed or old animals (35, 36). This supports a close association between Sl and lipostatic signals in fishes and gilthead sea bream in particular. In agreement with this, murine leptin is able to stimulate the in vitro secretion of pituitary Sl as part of the nutritional mechanisms driving the onset of puberty in European sea bass (37), though the seasonal pattern of Sl is more erratic in European sea bass than in gilthead sea bream, and it is difficult to infer a characteristic Sl pattern when PIT-tagged animals are considered individually (38). In any case, studies in gilthead sea bream highlight that recombinant Sl triggers a transient inhibition of feed intake, a satiety effect that is related to decreases in the respiratory quotient (CO2 output per O2 uptake) (12, 36). This pattern of gas exchange suggests the activation of lipid catabolism, which is consistent with the Sl inhibition of the hepatic activity of acetyl-coenzyme-A carboxylase (12). In salmonids, a role of Sl in energy mobilization during reproduction, acute stress or exhaustive exercise (39–42) has also been proposed. Therefore, Sl may help to expedite growth-reproductive processes following replenishment of fat stores and/or mediate the adaptation to fasting until the lipolytic action of Gh and/or other endocrine factors is fully accomplished. Contrary to this, other authors point out that the skin-color regulation is the only definite role of Sl so far demonstrated in fishes and medaka in particular (43). This still remains under debate, but the consensus is that Sl does not enhance in vivo or in vitro the production of insulin-like growth factors (Igfs) (12, 44, 45).
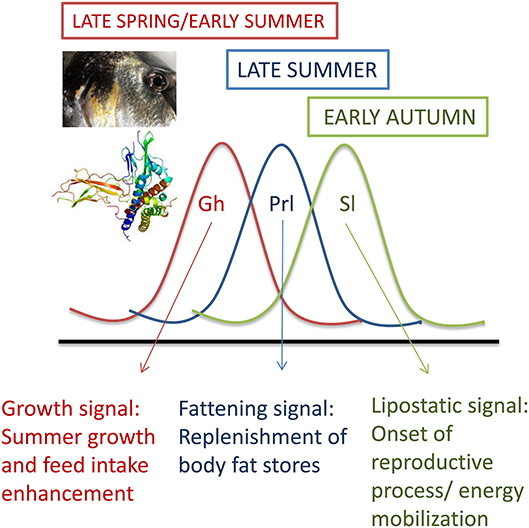
Figure 2. Seasonal patterns of plasma levels of Gh, Prl, and Sl in juveniles of gilthead sea bream. Adapted from Pérez-Sánchez et al. (20).
Gh-Dependent Growth
Pituitary cells producing GH are the main source of this hormone in the body, and its circulating concentration is barely detectable following hypophysectomy or impaired somatotroph differentiation in humans and birds (46). Hence, pituitary GH is largely responsible for the endocrine actions of GH during perinatal and postnatal growth, and according to the classical somatomedin hypothesis GH is released into the systemic circulation and then transported to target tissues to act via specific receptors to regulate growth and metabolism, directly or indirectly through the induction of hepatic or locally produced IGFs (47). However, this concept changed in the last quarter of twentieth century with the evidence of a widespread distribution of GH in most extrapituitary tissues, including neuronal, reproductive, immune, and gastrointestinal tissues, which may be independent on the pituitary-specific transcription factor-1 (PIT-1) (48). Indeed, pituitary GH expression does not occur in early embryonic or fetal development, whereas extrapituitary GH is expressed much earlier, prior to its ontogenic appearance in pituitary somatotrophs. In other words, as highlighted in previous reviews (49–52), embryonic or early fetal growth is independent of pituitary GH in higher vertebrates, and the persistence of extrapituitary GH through the life cycle is indicative of paracrine or autocrine roles for locally-produced GH. Indeed, the functional relevance of this extrapituitary Gh in postnatal growth would vary among vertebrates. For instance, the decreased expression of extrapituitary GH is not related to dwarf phenotypes in humans (51), whereas interactions between systemic and locally produced GH may contribute to explain the inconsistent relationship of circulating GH and growth in chickens (49).
As in birds and humans, extrapituitary Gh has been detected in immunorelevant and reproductive tissues of gilthead sea bream, trout and Japanese eel (53–55). The gh gene is transcribed and translated early during fish larval development before or after pituitary differentiation (29, 56, 57). Moreover, the main components of the somatotropic axis (Gh, Ghrs, Igfs) are produced as soon as transcription starts in fish embryos (58), and binding studies revealed a high concentration of actively transcribed Ghrs on the head of gilthead sea bream larvae a few days after hatching, which is consistent with their allometric growth. All this evidence together suggests a direct action of Gh rather than systemic effects via hepatic Igfs during early life stages (59), but later in life this complex trade-off would have evolved in a different manner within each group of vertebrates.
Circulating GH and IGF-i
Growth and IGF-I Association
IGFs are the primary mediators of the growth-promoting effects of GH, operating in an autocrine, paracrine and endocrine manner (60). They affect many biological processes, including protein synthesis and turnover, cell proliferation and differentiation, and cell apoptosis and tissue maintenance, which make IGFs good candidates as growth indexes. Unlike GH and insulin, IGFs are not stored or released in pulses, and clearance rates are retarded by the action of a suite of IGF-binding proteins (IGFBPs), a feature that allows relatively constant levels of IGFs in the blood. However, as reviewed by Beckman (61), the correlation between Igf-i and growth ranges from tight to non-discernible in fishes, probably reflecting a changing and sometimes confounding scenario. Thus, ration size, circulating Igf-i and growth rates are often positively correlated (62–64), though the Igf system appears to have a notable inertia after extended fasting or feed-restriction periods.
Overall, fast-growing fish strains also share an enhanced somatotropic axis activity (65, 66). However, the relationship between Igf-i and growth is largely affected by a wide-range of endogenous and exogenous factors, including gender, developmental and maturity state, photoperiod, temperature and salinity as well as stress and disease condition, which makes the Igf-i and growth relationship variable over season and productive cycles (61). Accordingly, Igf-i is a reliable growth index over precisely defined groups of fishes, but conservative approaches are needed for comparisons of Igf-i and growth rates across experiments within and between different fish species. For instance, the true effects of different photoperiods on growth and plasma Igf-i level are difficult to discern in trout and salmon (67–69). Likewise, some seasonal delay exists between growth rates and plasma Igf-i level over the productive cycle of gilthead sea bream (34), though circulating Igf-i continues to be perceived as one of the most reliable markers of growth performance in a wide range of fishes, including the hybrid striped bass (70), juvenile lingcod (71), or Nile tilapia (72). Furthermore, a concordant Igf-i and growth relationship has been reported combining data from juveniles of three Mediterranean farmed fishes (European sea bass, gilthead sea bream, common dentex) reared in the same indoor experimental facilities (35). A relatively high degree of concordance was also found for the decreased growth rates and circulating level of Igf-i in 1-, 2-, and 3-year old gilthead sea bream (73). Likewise, the recovery of plasma Igf-i during refeeding highly reflects the increase in weight gain during the phase of compensatory growth (74). More recently, the circulating level of Igf-i was concordant with the growth-promoting effects of moderate exercise in juveniles of gilthead sea bream (75, 76). Also in gilthead sea bream, a linear increase in growth rates and circulating Igf-i was reported in fingerlings in response to a single dose of recombinant bovine GH (77). However, these growth/endocrine patterns highly differ from those found in salmonids and, for the same or even higher growth rates, the circulating concentrations of Gh and Igf-i are often 3–10 times lower in trout juveniles than in Mediterranean farmed fishes (78).
Fish-species differences in the regulation of the Gh/Igf system also apply to the osmoregulatory action of Gh, which may act synergistically with cortisol and Igf-i to enhance the hypo-osmoregulatory ability, mainly in salmonid species. Thus, anadromous migrations from rivers to hyperosmotic environments stimulates the somatotropic axis for rapid growth and triggers different osmoregulatory actions related to the development of preparatory mechanisms for seawater entry (79). In this regard, Gh action can be mediated by Ghrs to stimulate Igf-i production not only in liver, but also in osmoregulatory organs (gills, kidney, and intestine), which in turn orchestrates ion and water movements to preserve or achieve a new steady state of plasma osmolality, as reported in Sakamoto et al. (80). However, confounding results have been reported in non-salmonid species. In several tilapia species (Oreochromis mossambicus and O. niloticus), no significant differences have been reported in pituitary gh expression or in Gh plasma levels in juvenile or larval stages in freshwater-, brackishwater- or seawater-acclimated fishes (81–83), whereas other studies suggest a role of Gh during osmotic acclimation as a result of modifications in plasma Gh level after hyperosmotic transfer (84–86). In eels, no changes in plasma Gh have been reported with transfer from freshwater to seawater (87, 88). In meager and gilthead sea bream, a clear increase in pituitary gh transcripts is found in hyperosmotic environments (89, 90). This response is maintained during acclimation to isosmotic salinity in silver sea bream (91, 92), black sea bream (93) or gilthead sea bream (94), which in turn would trigger the improvement of growth rate (95) through changes in the pentose phosphate pathway or synthesis of stress proteins (91, 93, 96). The ultimate mechanisms remain to be established, but importantly, microarray gene expression profiling of liver, gills and hypothalamus after hypo- or hyperosmotic challenges identified more than 750 differentially expressed genes in gilthead sea bream, with three major clusters of overlapping canonical pathways corresponding to energy metabolism, oxidative stress and cell and tissue architecture (97).
Sirtuin Energy-Sensing and GH/IGF-I
Differences in key performance indicators necessarily reflect different uses of nutrients and energy, as voluntary feed intake and growth are limited by the capacity to preserve the redox balance (98–100). In that sense, animals with enhanced feed intake and growth rates are able to grow efficiently in a cellular milieu with enhanced risk of oxidative stress, and the differential regulation of sirtuins (SIRTs) contributes to the readjustment and preservation of metabolic homeostasis. These enzyme deacetylases use NAD+ as cofactor and couple the acetylation status of histone and non-histone substrates with the energy status of the cell via NAD+/NADH ratio. SIRTs are virtually ubiquitous through all kingdoms of life, and the number of family members increases with the organismal complexity: prokaryotes have one to two family members, fission yeast three, worms four, flies five and higher vertebrates (including fishes) seven (101, 102). This, together with different cellular locations (nuclear, SIRT1 and SIRT6-7; cytoplasmic, SIRT2; mitochondrial, SIRT3-5) offers the possibility of complementary but also non-redundant and tissue-specific energy-sensing mechanisms strongly influenced by nutrient availability, energy demand and tissue-specific metabolic capabilities (103–105).
The ultimate mechanisms by which changes in SIRT expression and activity modulate the action of metabolic hormones and the GH/IGF system remains poorly studied. However, studies in mice have found that in vivo knockdown of hepatic Sirt1 restores the fasting-induced decrease in serum IGF-I and enhances the GH-dependent increase in IGF-I (106). Knockdown of Sirt1 in mice enhances the acetylation and GH-induced tyrosine phosphorylation of STAT5, indicating that SIRT1 negatively regulates GH-dependent IGF-I production via deacetylation of transcription factor STAT5. Additionally, SIRT1 acts at the brain level as a link between somatotropic signaling and calorie restriction (107), and brain-specific Sirt1 knockout mice have dwarfism and reduced plasma GH and IGF-I (108), displaying similar phenotypes to those of long-lived mutant mice (109). By contrast, SIRT1 activation with resveratrol suppresses GH synthesis in pituitary rat cells by reducing PIT-1 availability to the Gh promoter via the transcriptional suppression of Creb (110). Unlike this apparent controversy, the net effect of SIRT1 activation continues to be the suppression of the GH/IGF tonus, which would serve to drive a decreased energy demand for growth purposes in a cellular milieu with a reduced availability of metabolic fuels.
Relatively less is known about the regulation of sirts in fish, but recent studies in gilthead sea bream highlight that short-term fasting does not alter significantly the sirt1 gene expression in liver, whereas the expression pattern for other sirt isotypes (sirt2-6) is an overall suppression linked to reduced energy demand for hepatic lipogenesis (102, 111). In contrast, gilthead sea bream strains that regularly perform better than other genetically different strains have a reduced hepatic sirt1 expression, in combination with a more active feeding behavior and Gh/Igf-i system, resulting in enhanced growth and higher circulating Igf-i (112). The expression of sirt genes is also highly regulated by energy demand in the white skeletal muscle of gilthead sea bream, and sirt2 mRNA is clearly upregulated in fast growing fish, whereas the mitochondrial sirt5 emerges as a more responsive element during forced fasting (102) or natural fasting when comparisons are made between fishes of different size during the cold season (unpublished results). In both cases, this occurs in combination with the enhancement of the lipolytic machinery and reduced energy wastage, evidenced by the downregulation of muscle mitochondrial uncoupling proteins and changes in the expression of many markers of lipid metabolism and oxidative phosphorylation, as was also highlighted by the muscle microarray gene expression profiling of fishes fed at maintenance ratio (113).
For comparative purposes, it is relevant that physiological studies in humans have revealed a regulatory role of SIRT2 in muscle stem cell proliferation and differentiation (114–116). Moreover, single-nucleotide polymorphisms of Sirt2 have been associated with different body size traits in Qinchuan cattle (117). Adiposity is also a main factor affecting SIRT expression, and SIRT6 activity is depressed in the adipose tissue of obese human patients (118). In this way, it is likely that the concurrent upregulation of sirt5 and sirt6 in the adipose tissue of our fast-growing fish strains orchestrate a lean phenotype, resulting in a reduced adipose tissue mass with an increased mobilization of fatty acids toward skeletal muscle and liver. All of the above evidences a complex metabolic crosstalk between Sirt-energy sensors and the Gh/Igf system, with perhaps the double aim of (i) avoiding or minimizing the loss of muscle protein mass during stages of negative energy balance and (ii) precisely tuning the growth energy-demanding processes of organisms to the exogenous supply and availability of metabolic fuels (see Figure 3). However, we are far from fully understand this complex picture, and current studies aiming to highlight whether tissue-specific differences in fish Sirt profile result from nutritional, genetic or epigenetic sources of variation as key players of genome stability during environmental stimuli and stress response are underway, as reviewed by Bosch-Presegué and Vaquero (119).
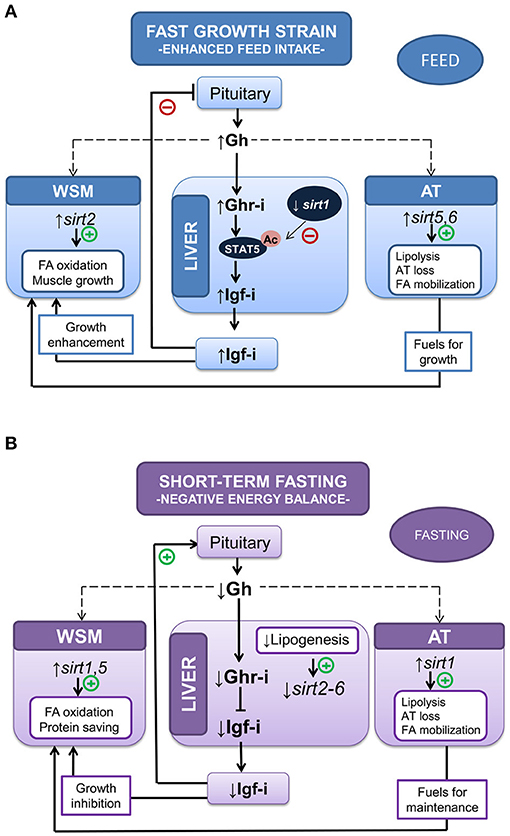
Figure 3. Metabolic crosstalk between Sirtuin-energy sensors and the Gh/Igf system in liver, white skeletal muscle (WSM) and adipose tissue (AT) in two different experimental models: (A) accelerated growth in fishes with enhanced feed and (B) negative energy balance (10-day-fasted fishes). Adapted from Simó-Mirabet et al. (#B102">102, 112).
Gh/Igf Responsiveness in Hypoxic and Crowded Fishes
Animals overexpressing Gh combat oxidative stress less efficiently than normal and dwarf mice (120, 121). In the same way, gh-transgenic fishes have a lower capacity to manage a hypoxic environment efficiently (122, 123), though paradoxically early studies in mammals indicate that circulating Gh can be increased by either the increase in O2 requirements or the reduction of O2 availability (124). A possible explanation is that regulation of circulating Gh level in hypoxic animals mirrors the changing energy needs rather than the availability of metabolic fuels. In this way, circulating Gh increased markedly in salmonids submitted to maximum swimming (125, 126). The same response has been found in gilthead sea bream, and plasma Gh levels are of predictive value of the aerobic scope in swimming test chambers, as circulating Gh before exercise is a surrogate marker of critical speed (swimming speed that could theoretically be maintained indefinitely without exhaustion) (127). The opposite is also true, and circulating Gh is lowered after acute or chronic confinement exposure in a wide range of fishes, including gilthead sea bream (128–130), trout (131), Atlantic salmon (132), and Nile tilapia (133). However, as first pointed out by Pickering et al. (131), hypoxia, crowding and water quality induce confounding effects that include the activation of the hypothalamic-pituitary-interrenal axis (HPI-axis) and the overall downregulation of hepatic igf and ghr genes, concomitant with growth inhibition and lowered plasma Igf-i in both crowded and hypoxic fishes (130, 134).
Fine adjustments of metabolism machinery also take place at the mitochondrial transcriptional level, and experimental evidence reveals a reduced production of energy and reactive oxygen species (ROS) when fishes are crowded (135) or exposed to multiple stressors that mimic daily aquaculture operations (136). In that sense, the capacity of fish to efficiently manage the allostatic load (defined as the maintenance of internal homeostasis through changes of a number of stress mediators) by readjustments of O2-carrying capacities and metabolic suppression are of high value to finally reach the internal equilibrium in a hypoxic-challenging scenario, which would prime a reduced production/accumulation of toxic byproducts from anaerobic metabolism (137–139). In hypoxic gilthead sea bream, this metabolic suppression is exemplified by the overall depression of catalytic, assembly, and regulatory enzyme subunits of complex I, II, III, and V of the mitochondrial respiratory chain, which is concurrent with the upregulation of catalytic and regulatory elements of complex IV (last electron donor to oxygen acceptor) (134). This dualism offers the possibility of a reduced but more efficient mitochondrial respiration during exposure or recovery from severe hypoxia, as has been shown, at least in part, in fishes fed with seaweed extracts as dietary surplus to protect against oxidative stress (140). Most of these metabolic readjustments, linked with endocrine disturbances (130, 134), have been reported after exposure to severe hypoxia (18–19% oxygen saturation, 20–22°C) for 4 h under steady-state conditions, but most analyzed parameters require lasting periods to be responsive when the water O2 concentration is fixed close to limiting oxygen saturation (LOS), defined as the threshold level where regulatory mechanisms are no longer sufficient to maintain the O2 consumption rate with changes of the level of O2 saturation (141, 142). Thus, juveniles of gilthead sea bream reared at 40% O2 saturation and 20–22°C exhibit a reduced, but active feeding behavior, that is adjusted to meet dietary O2 demands according to the oxystatic theory (143). This allows fishes to grow efficiently at slower rates, which is consistent with a total or partial recovery of control plasma levels of cortisol and Igf-i regardless of a persistent hypersomatotropic state that would reflect an enhanced demand for metabolic fuels (Figure 4). These endocrine signatures clearly indicate the different dynamics of the HPI and the Gh/Igf system in response to severe and moderate hypoxia, which merits consideration for metabolic phenotyping of farmed fishes in a scenario of increasing temperatures and global change.
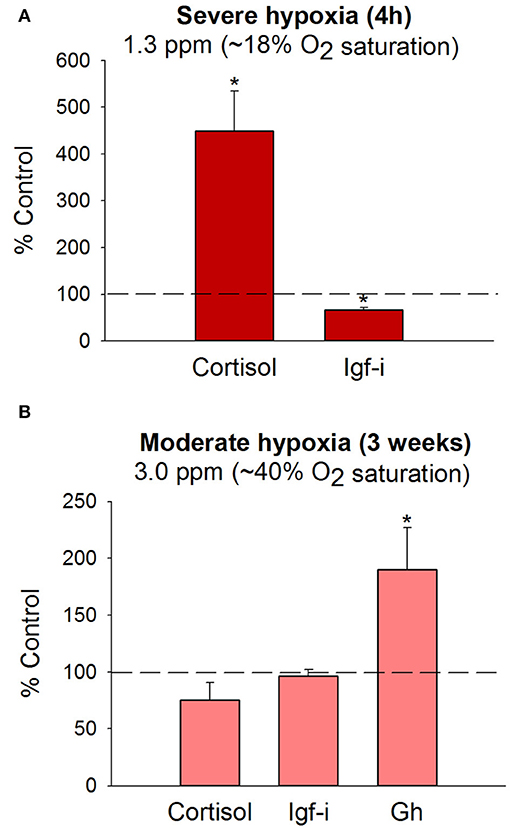
Figure 4. Plasma hormone levels in juveniles of gilthead sea bream following exposure to severe (A) or moderate hypoxia (B) at 20–22°C. Bars indicate changes in plasma cortisol, Gh and Igf-i levels in comparison to normoxic fishes (>85% O2 saturation). Data are presented as the mean ± SEM (n = 10–20). Asterisks indicate statistically significant differences (P < 0.05, t-test). Adapted from Martos-Sitcha et al. (134) and Magnoni et al. (140).
Nutritional Background and Gh/Igf Status
The current stagnation of fish meal (FM) and fish oil (FO) production from wild fisheries limits further growth of aquaculture (144). In the meantime, the most immediate alternatives are plant products, which have been used for years in salmonids and marine fish feeds to reduce the reliance of European aquaculture on marine fishery resources. Thus, most carnivorous farmed fish, including European sea bass (145) and gilthead sea bream (146, 147), can be successfully reared with plant-based diets containing < 10% marine feedstuffs. Moreover, complete replacement of FO by vegetable oils is feasible when the theoretical requirements for phospholipids and n-3 long-chain polyunsaturated fatty acids (LC-PUFA) are met by the lipids contained in FM (148–150), but the concomitant FM/FO replacement continues to be challenging despite the important research efforts within the framework of PEPPA (2001–2004), AQUAMAX (2006–2010), and ARRAINA (2012–2016) EU projects for meeting the nutrient requirements of European farmed fish in terms of growth, health, and welfare criteria. In that respect, important progress has been made in salmonid and non-salmonid fishes on the diagnosis of nutrient deficiencies or the re-evaluation of nutritional requirements of vitamins, minerals, and other key nutrients by means of surrogate markers resulting from blood biochemistry and hematology profiling, histopathological and organo-somatic index scoring or measures among others of enzyme activities or vertebral mineral concentrations (151–155).
Often, plant-based diets have an impact on the gastro-intestinal transcriptome, mucus intestinal proteome or intestinal microbiome, but the use of feed additives (e.g., butyrate) helps to preserve the wild phenotype of fish fed FM/FO-based diets, improving the disease outcomes of gilthead sea bream challenged with bacteria or intestinal parasites (156–158). Historically, the somatotropic axis has also been used as an endocrine marker of the effectiveness of alternative feed formulations to support maximum growth. A first study combining measures of circulating Gh, Igf-I, and Igfbps with those of Gh-binding and gh and igf transcripts was conducted by Gómez-Requeni et al. (78) to investigate the physiological consequences of the partial or total replacement of FM by plant ingredients in trout juveniles. Likewise, measurements of circulating Gh and Igf-i in combination with those of transcripts of ghrs, igfs, igfbps, and other growth-related markers, including molecular chaperones, myogenic factors, energy sensors, and markers of protein turnover, lipid metabolism, oxidative phosphorylation (OXPHOS) and mitochondria respiration uncoupling, are highly informative for a wide-ranging assessment of growth performance; first with the replacement of FM by plant proteins (159) and second with the combined and maximized replacement of FM and FO by plant proteins and vegetable oils (146, 160–162). This results in differences in growth rates with a high degree of concordance of growth and growth-promoting factors between trials (9–11 weeks) conducted during the summer growing period with juvenile fishes of the same strain (Atlantic fish strain) and class of size (14–16 g initial body weight).
The above observations further support a consistent growth output for a given Igf-i concentration that makes feasible meta-analysis of data from highly controlled experiments. In that sense, the evolutionary past of living animals is marked by periods of undernutrition that are characterized by protein-calorie deficiencies or vitamin and mineral deficiencies and even starvation, which makes survival dependent on the body's ability to mobilize energy stores (163). Since GH plays a key role in mobilizing energy (lipolytic role non-dependent of IGFs) its elevated circulating levels in the setting of low IGF-I confers a metabolic advantage during undernutrition periods. Therefore, a state of GH resistance is highly conserved through the evolution of fishes and higher vertebrates as part of the adaptive response to inappropriate nutritional conditions. This reflects a reduced responsiveness of target tissues to the anabolic GH action, which may be due to receptor or postreceptor defects in the transmission of GH signaling. Thus, diets deficient in tryptophan resulted in reduced growth and plasma Igf-i in juveniles of the hybrid striped bass (164). Hevrøy et al. (165) also found that lysine-enriched diets resulted in a significant increase in muscle protein deposition and hepatic igf-i mRNA in Atlantic salmon. Likewise, methionine availability modulates the expression of genes involved in the Gh/Igf response and protein turnover, further affecting growth performance in trout (166). Previous studies in trout indicate that plasma Gh level is not affected by either methionine or taurine supplementation, and even more, methionine excess has been associated with decreased plasma Igf-i in fishes fed FM-free diets (167). This apparent discordance indicates the complexity of endocrine growth regulation within and between fish species. However, it is noteworthy that recent studies in gilthead sea bream highlight a close linear relationship between growth and circulating levels of Gh and Igf-i in fishes fed semipurified diets formulated for deficiencies in methionine, n-3 LC-PUFA, phospholipids, phosphorus, vitamins, or minerals (Figure 5). This association between growth and Gh/Igf-i is also concordant with that found in juveniles fed practical diets with varying degrees of FM and FO replacement. Therefore, the somatotropic axis highly reflects the drawback effects on growth performance due to a reduced nutritive value of the main dietary protein or lipid sources, but also in response to specific nutrient deficiencies mimicking those induced by enriched plant-based diets.
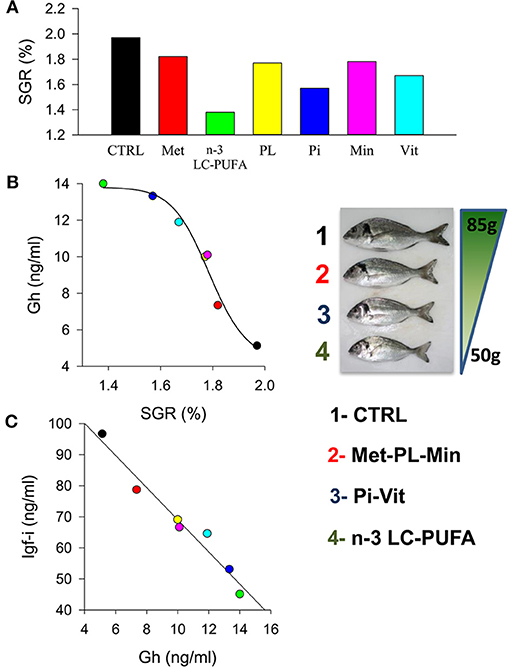
Figure 5. (A) Effect of nutrient deficiencies on the specific growth rates (SGR) in juveniles of gilthead sea bream fed to visual satiety for 13 weeks from May to July. (B) Curvilinear correlation between SGR and plasma Gh levels. Insert image shows fish size differences at the end of trial. (C) Correlation between plasma Gh and Igf-i levels. Colors indicate each experimental group: CTRL, control (black); Met, methionine (red); n-3 LC-PUFA, n-3 long-chain polyunsaturated fatty acids (green); PL, phospholipids (yellow); Pi, phosphorus (blue); Min, minerals (pink); Vit, vitamins (cyan). Adapted from Ballester-Lozano et al. (152).
More recently, it was found that the growth-promoting action of medium-chain fatty acids in juveniles of gilthead sea bream fed FO-based diets resulted in a slight increase in the Igf-i/Gh ratio (168). In this way, the combined use of circulating Igf-i and the Igf-i/Gh quotient have been revitalized as reliable key indicators of growth performance in fishes, highly reflecting the magnitude of a wide range of nutritional and growth derangements. Certainly, circulating Gh and Igf-i are either the up- or downstream factors of the Gh/Igf system, but they are informative of the intensity of growth impairment rather than the nature or origin of disturbing processes. Hence, as indicated below, measures of transcripts of the main actors of the somatotropic axis alone or in combination with other markers of protein mass accretion and cellular stress are required for a refined diagnosis of a given nutrient deficiency or even for the re-evaluation of species-specific nutrient requirements.
Regulation at the Transcriptional Level
Effects of Nutrition and Season on ghrs
Fish ghrs were first cloned and sequenced in goldfish (169), turbot (170), and sparid fishes (171, 172). Nucleotide sequences encoding for fish ghrs were also available in coho and masu salmon at the end of the last century, and several authors suggested a divergent evolution of salmonid and non-salmonid ghrs. However, as first pointed out by Saera-Vila et al. (173), duplicated fish ghrs are actively transcribed in trout, European sea bass or gilthead sea bream and they are more similar to each other than to Ghrs of tetrapods. Searches for ghrs in fish genome databases also confirmed their occurrence in almost all the analyzed fishes, but unfortunately the initial controversy over ghr phylogeny has made the nomenclature of fish ghrs somewhat confusing. To solve this issue, the convention for fish ghr nomenclature is the clade ghr type I (also written type 1 or slr in masu salmon) for the initially described ghr of non-salmonid fishes, while the clade ghr type II (also written type 2) corresponds to the initially described ghr isotype of salmonids [see Reindl and Sheridan (60)], where the chromosomal location of duplicated sequences of ghrs of type I and II are consistent with the generation of paralogous blocks in the salmonid tetraploidization (174).
Most of what we know about GH signaling comes from mammalian species and cell lines, but intracellular signaling pathways are generally well conserved, and many commercially-available antibodies developed to target mammalian signaling molecules also detect orthologs in piscine species (175). This opens new research opportunities, and several attempts have been made to support the possibility that a given Ghr subtype might be responsible for transmitting the lipolytic action of Gh, while the other Ghr subtype would be more active in transmitting the growth-promoting action of Gh (31). To our knowledge, more work is needed to establish these explicit links, though it is well-recognized that Gh differentially activates disparate signaling pathways when stimulating growth through Jak-sat, Pi3k-akt, and Mapk (176), and lipolysis through Plc-pkc, MapK, and Hsl (177–179). Meanwhile, the differential and tissue-specific regulation of ghr subtypes by nutritional and environmental factors becomes especially clear in farmed gilthead sea bream, in which hepatic transcripts of ghr-i (in a low extend ghr-ii) mirror changes in growth rates, plasma Igf-i level, and hepatic igf-i transcription through development and production cycles, indicating a prominent role of Ghr-i rather than Ghr-ii in the systemic growth-promoting action of Gh (73, 146, 160). Moreover, the hepatic ghr-i/ghr-ii gene expression ratio remains mostly unaltered during seasonal changes of growth rates. However, this gene expression ratio is upregulated in white skeletal muscle from 2 to 3 in summer to more than 4 in winter. This gene expression ratio is also highly regulated at the nutritional level, and fishes fed unbalanced plant-based diets or semipurified diets formulated for specific nutrient deficiencies downregulate hepatic ghr-i, decreasing the ghr-i/ghr-ii ratio from 1.8 to 2 to < 1 (146, 152, 160). Likewise, in white skeletal muscle, the ghr-i/ghr-ii ratio decreases from 2.5 to 3 to close to 1 in fishes with signs of nutrient deficiencies, but this molecular feature seems to be the result of the counterregulatory upregulated expression of ghr-ii. Conversely, impaired growth during overwintering is not able to alter significantly the hepatic ghr-i/ghr-ii mRNA ratio regardless of the overall depressed gene expression during the cold season (see Figure 6).
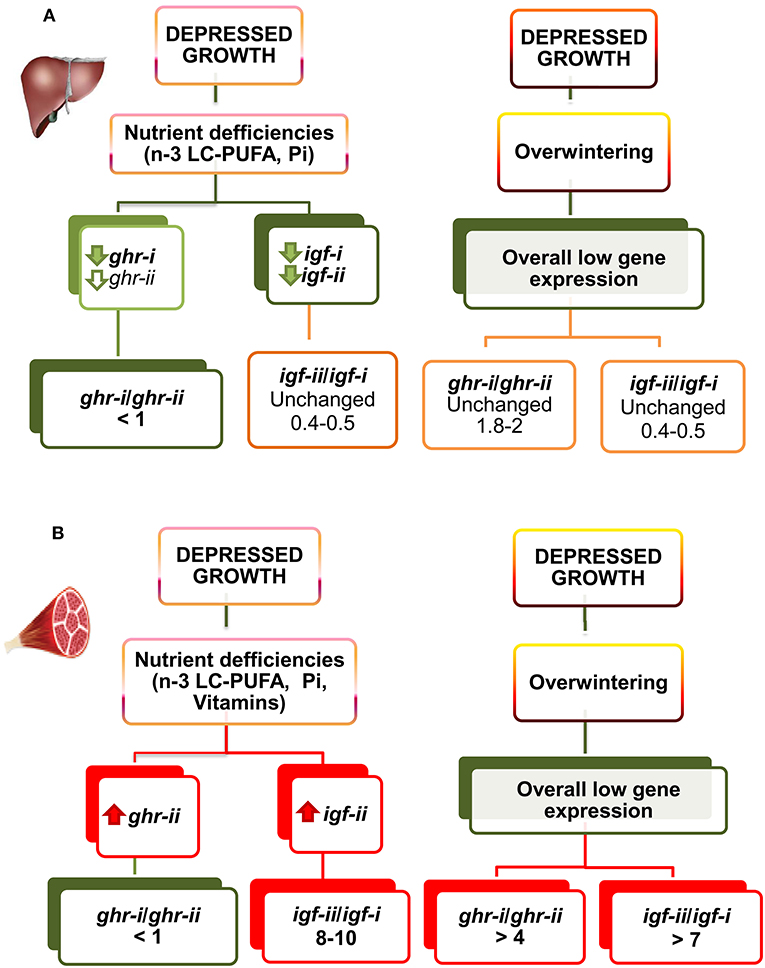
Figure 6. Regulated gene expression of igfs and ghrs by diet composition and season in liver (A) and skeletal muscle (B) of gilthead sea bream juveniles. The direction of change is represented by color (red, increase; green, decrease; orange, unchanged). For full details of gene expression profiling see Tables S1–S6. Adapted from Benedito-Palos et al. (146, 160) and Ballester-Lozano et al. (152).
Insulin/Igf System: Evolutionary Prospect
Insulin, IGF-I and IGF-II are structurally similar and they are derived from a common ancestral molecule through a series of gene duplications and mutations (180). Fishes are the first group in the vertebrate tree in which there is evidence of distinct insulin and Igf molecules and receptors (181). However, certain cross-interaction between ligands and receptors of insulin and Igfs occurs. This is especially evident for Igf-ii, which exerts its mitogenic action through insulin and Igf-i receptors (182, 183). Additionally, important differences regarding receptors specificity and abundance through the evolution have been reported. Indeed, fish Igf-i receptors show a higher degree of specificity than insulin receptors (184, 185), and binding studies in cardiac and skeletal muscle highly support that the number of insulin receptors is lower than the number of Igf-i receptors in fishes, amphibians and reptiles (186–188), whereas the opposite is found in birds and mammals (189, 190). Nevertheless, at the expression level, the relative abundance of insulin receptors in juveniles of gilthead sea bream fed practical diets from early life stages is higher than initially expected. This is especially evident in liver, where the expression quotient ratio for insulin receptors and Igf receptors remains almost equal along seasons. The same trend, but less evident, is found in white skeletal muscle, where the expression level of insulin receptors is almost equal to that of the igf-i receptor in both summer and winter, and higher than that of the igf-ii receptor in summer (see Figure 7). Since gilthead sea bream is an euryhaline, eurythermal, and protandrous hermaphrodite fish, it is tempting to speculate that the increase in the insulin receptor/Igf receptor expression ratio with organismal complexity from ectotherms to endotherms also applies to a fish with a well-recognized growth and metabolic plasticity, and improved resilience to aquaculture stressors (112, 135, 136, 191).
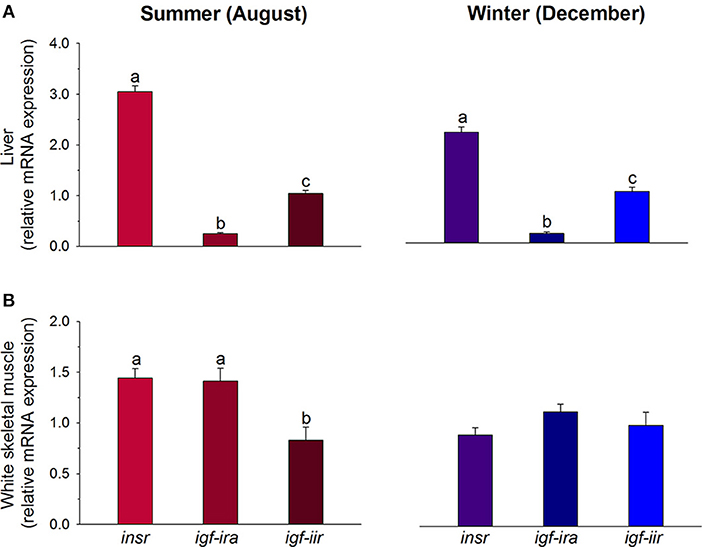
Figure 7. Regulated gene expression of receptors of insulin (insr), igf-i (igf-ira), and igf-ii (igf-iir) in liver (A) and skeletal muscle (B) of gilthead sea bream juveniles during summer (red colors) and winter (blue colors). For each tissue and season, data values (mean ± SEM, n = 6–7) are normalized to the expression level of igf-iir (arbitrary value of 1). Different superscript letters indicate significant differences (P < 0.05; ANOVA followed by Student-Newman–Keuls test). Data derived from samples from Benedito-Palos et al. (146).
igf-ii/igf-i Expression Ratio
A growing body of evidence from more than 25 years indicates that IGFs play key roles in the growth and development of mammals and chickens. As a result of multiple transcription initiation sites and alternative splicing, the Igf-i gene gives rise to different transcripts in higher vertebrates (192, 193), encoding for several Igf-i precursor polypeptides. The biological significance of these splice variants still remains under debate, although a differential expression profile has been reported in response to varying conditions and pathologies (194, 195) and potentially different bioactivity of the Igf-i isoforms is suggested. Multiple forms of Igf-i have been also detected in fishes, including gilthead sea bream (196–199). This functional plasticity is not found in IGF-II, which is considered a primary growth factor for embryonic and fetal growth (200, 201), while IGF-I is required for achievement of maximal postnatal growth (202). Indeed, postnatally elevated levels of Igf-ii transcripts fail to rescue the dwarfism of Igf-i-deficient mice (203), and Igf-ii transcripts decrease quickly during the postnatal development of mice and rats (204). However, substantial IGF-II amounts are found later in life in humans and in a wide-range of fishes, including common carp (205), trout (206), Nile tilapia (207), channel catfish (208), and gilthead sea bream (209). Overexpression of igf-ii is especially evident in extrahepatic tissues: in gilthead sea bream, the igf-ii/igf-i expression ratio ranges from 0.5 in liver and 3–9 in skeletal muscle, adipose tissue, gills and brain to 75–150 in heart, intestine and gonads. In these studies, igf-i measures considered the expression of the totality of transcripts given the retention of the core mature peptide in all Igf-i precursors (159).
Regarding in depth igf-ii expression, compensatory increases in skeletal muscle igf-ii mRNA also occurs in juveniles of gilthead sea bream fed FO-free diets to counteract, at least in part, the suppressed growth and expression of hepatic igfs (160). However, more recent data using fishes fed semipurified diets formulated for nutrient deficiencies indicate that this type of muscle response is perhaps more informative of deficiencies in vitamins rather than n-3 LC-PUFA. The igf-ii/igf-i expression ratio is also sensitive to seasonal changes in growth rates, and the apparent winter suppression of muscle igf expression is more evident for the lowest-expressed igf gene. The igf-ii/igf-i ratio in juvenile fishes varies from 10 in summer to 3–5 in winter. By contrast, the hepatic igf-ii/igf-i ratio remains almost unaltered and near 0.5 over season in both well and malnourished fishes. This is because the two hepatic igf transcripts are similarly regulated when facing different environmental and nutritional stimuli (see Figure 6). The regulation of igf expression quotient is, therefore, different from that reported for ghrs, as the expression of ghr-i is more regulated in liver than in skeletal muscle, whereas ghr-ii and igf-ii appear more regulated at the muscle local level, as part of some kind of compensatory growth response. This finding helps to clarify the different functions of ghr and igf duplicated genes, helping to refine the molecular signatures of a given growth and nutritional condition through production cycles.
Fish igfbp Repertoire
The ancestral Igfbp gene was duplicated in tandem during an early stage of vertebrate evolution to produce a pair of Igfbps that gave rise in subsequent genome duplication events the two Igfbp clades of modern vertebrates (Igfbp-1/-2/-4; Igfbp-3/-5/-6) (210–213). Additionally, the third and fourth round of whole-genome duplications created the corresponding paralog pairs. The resulting number of Igfbp subtypes is thus variable between fish lineages, but always higher than in mammals and other vertebrates. For example, zebrafish have retained nine actively transcribed genes that include igfbp-3a and paralog pairs of igfbp-1, -2, -5, and -6 (214–217). Atlantic salmon possesses 22 unique igfbp genes with 11 paralog pairs of igfbp-1a, -1b, -2a, -2b, -3a, -3b, -5a, -5b, -6a, and -6b. Common carp retains 17 igfbp genes including igfbp-2a and paralog pairs of igfbp-1a, -1b, -2b, -3a, -5a, -5b, -6a, and -6b (218). Likewise, searches in the gilthead sea bream genome database (http://nutrigroup-iats.org/seabreamdb/) have identified 11 igfbp genes, covering the full igfbp-1 to -6 repertoire with paralog pairs of igfbp-1, -2, -3, -5, and -6. These findings evidence a different and perhaps divergent evolution of the igfbp repertoire in fish species, though high quality reference genomes, with all genes properly annotated and assembled into corresponding chromosomes, are required to confirm this idea. At this stage, to avoid nomenclature confusion, new and previous igfbp sequences of gilthead sea bream are annotated, and uploaded to GenBank, according to the proposed nomenclature of salmonids. The identity of the annotated igfbp sequences has been corroborated by phylogenetic analyses, which evidenced the differential expansion of igfbp genes within salmonids, carp, northern pike and gilthead sea bream (Figure 8).
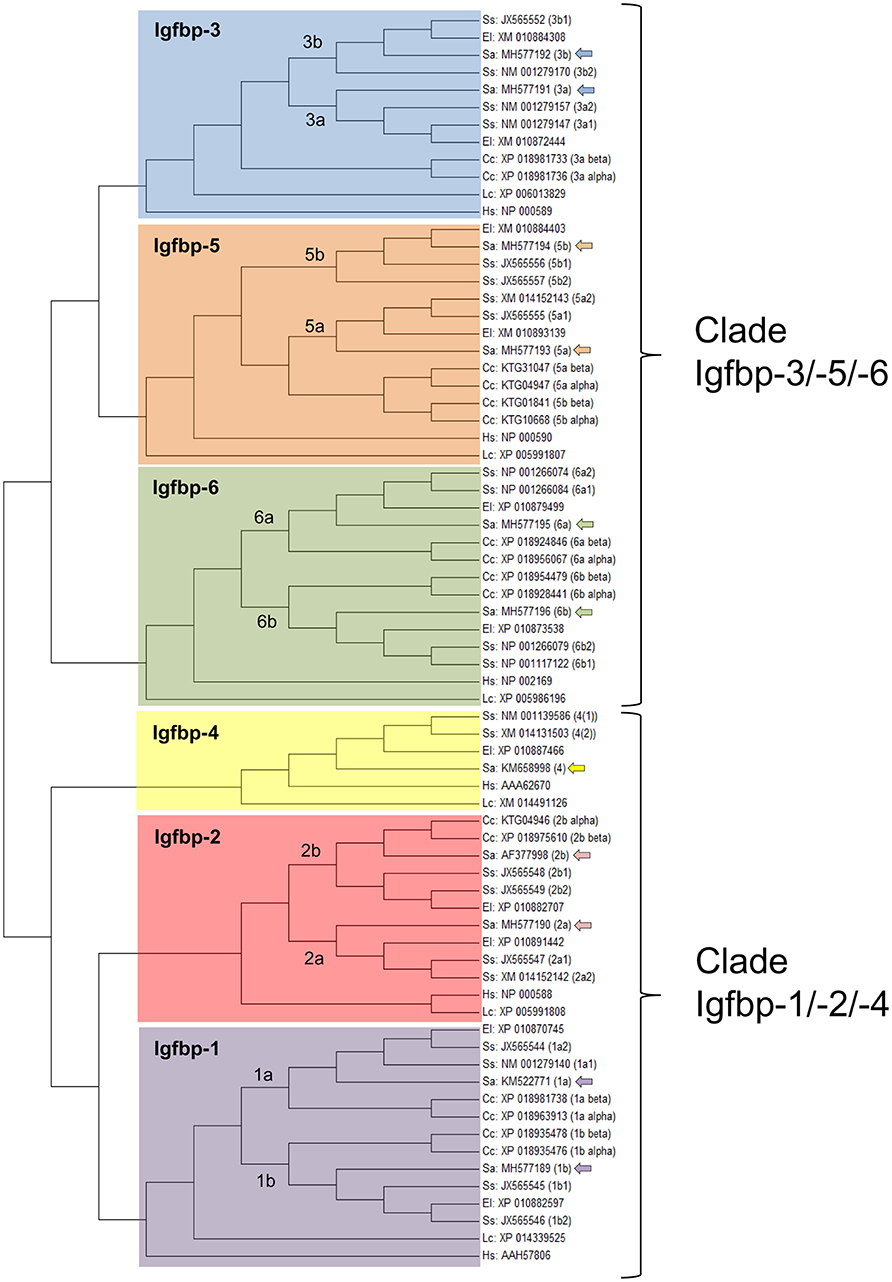
Figure 8. Phylogenetic analysis of the Igfbp family, evidencing the two Igfbp modern vertebrate clades and the expansion of the family in teleosts. The unrooted tree was constructed by means of Mega 6.0 using the maximum likelihood method, with the amino acid alignment of 72 Igfbp sequences from Human, Homo sapiens (Hs); Comoran coelacanth, Latimeria chalumnae (Lc); northern pike, Esox lucius (El); carp, Cyprinus carpio (Cc); Atlantic salmon, Salmo salar (Ss); and gilthead sea bream, Sparus aurata (Sa). GenBank accession numbers are given for all sequences. Duplication events in teleosts are shown. The 11 gilthead sea bream sequences, including igfbp-4 and paralog pairs of igfbp-1, -2, -3, -5, and -6 are marked with arrows.
Regarding the expression profile, the clearest pattern is the different tissue-specific profile of igfbps in liver and skeletal muscle. Of note, transcripts of the igfbp-1/-2/-4 clade are highly represented in the liver tissue of gilthead sea bream juveniles: igfbp-2b and igfbp-4 comprise more than 70 and 20% of igfbp mRNAs, respectively. In contrast, the igfbp-3/-5/-6 clade is overrepresented in skeletal muscle, and igfbp-3 and igfbp-4 transcripts make up 90% of mRNAs coming from igfbp genes. This clear divergence of expression patterns for the two igfbp clades is also a characteristic feature of Atlantic salmon in liver and muscle tissues (213). By contrast, this dichotomy is not retained by the adipose tissue where a relatively high expression level is found for both igfbp-4 (igfbp-1/-2/-4 clade) and igfbp-5b (igfbp-3/-5/-6 clade) genes in gilthead sea bream (Figure 9). In blood, the presence of different Igfbps within a molecular range of 20–50 kDa has been reported by Igf-binding assays in various fish species but, unlike in mammals, Igfbp-2 and not Igfbp-3 is emerging as the major blood Igf-i carrier in fish species (219), which is consistent with the elevated expression pattern of igfbp-2 genes in the liver tissue of this group of vertebrates.
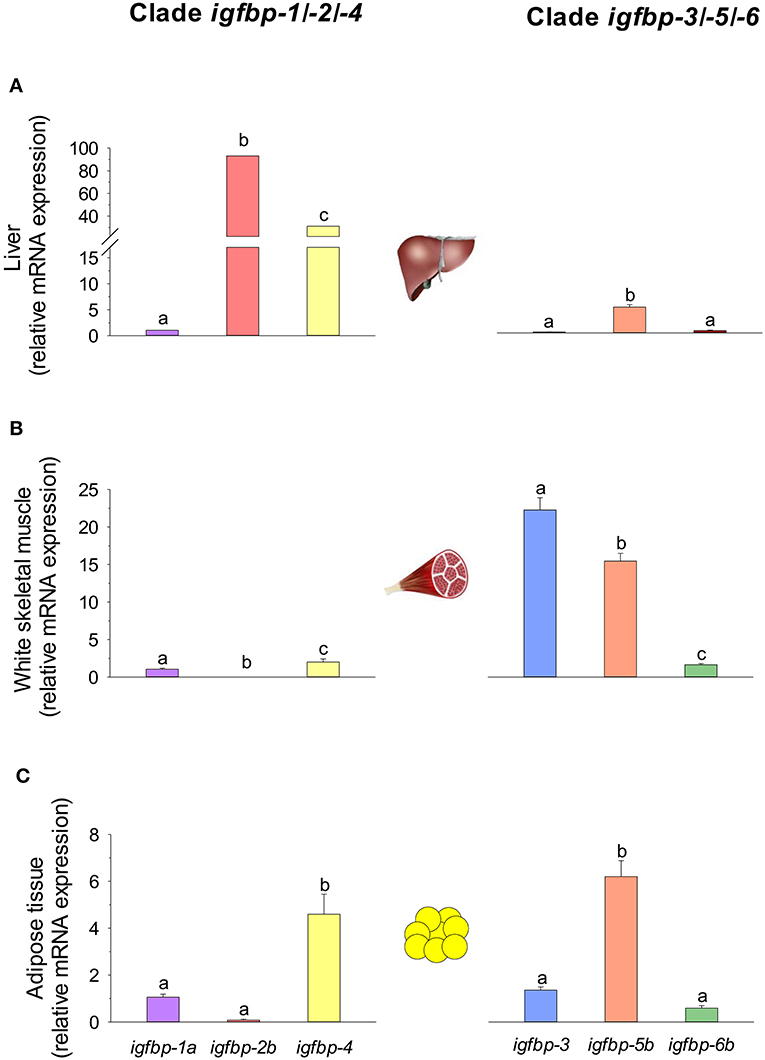
Figure 9. igfbp gene expression profile in (A) liver, (B) white skeletal muscle, and (C) adipose tissue of juveniles of gilthead sea bream. RT-qPCR of tissue total RNA was conducted as previously reported (134). Analyzed genes of clade igfbp-1/-2/-4 were igfbp-1a, igfbp-2b, and igfbp-4. For clade igfbp-3/-5/-6, primers for igfbp-3 detected both igfbp-3a and -3b paralogs, and expression levels of igfbp-5b and igfbp-6b were analyzed. For each tissue, data values (mean ± SEM, n = 8–9) are normalized to the expression level of igfbp-1a (arbitrary value of 1). In each tissue and clade, different superscript letters indicate significant differences (P < 0.05; ANOVA followed by Student-Newman–Keuls test).
More controversial are the main roles of each IGFBP as a growth-promoting or inhibiting factor through their IGF- or non-IGF-mediated effects. Recently, this has been extensively reviewed (210, 218), though it remains difficult to draw a general conclusion since most of the physiological roles, when conserved, differ across species and physiological contexts. Moreover, the lack of substantial phenotypes when Igfbp-3, -4, and -5 were knocked out together in mice suggests a high degree of functional redundancy and/or genetic compensatory mechanisms (220), which reflects versatile modes of regulation in response to specific stressful or aberrant conditions. In any case, a large body of evidence in higher vertebrates and fasted, refed, or gh-transgenic fishes supports a main role of Igfbp-1 as a negative regulator of teleost growth. For instance, knockdown of igfbp-1 alleviates the hypoxia-induced growth retardation and development delay in zebrafish, whereas overexpression of igfbp-1 causes growth and developmental retardation under normoxia (221). Along the same lines, we found that hepatic igfbp-1a expression remains mostly repressed in grow-out gilthead sea bream during both summer and winter periods (Figures 10A,B). Mixed results exist on the regulation of Igfbp-2 genes. Mouse embryos overexpressing Igfbp-2 show a reduced growth rate, likely through reduced IGF availability (222). By contrast, work in Atlantic salmon highlights an increase in circulating Igfbp-2 in response to Gh (218), while early studies in zebrafish reported that Gh inhibits igfbp-2 expression (223). As in salmonids, data on igfbp-2b expression in gilthead sea bream support a growth-promoting action of Igfbp-2, which is substantiated by its seasonal expression pattern and its depressed expression in fishes with signs of essential fatty acid deficiencies (Figure 10C). On a seasonal basis, the expression pattern of igfbp-4, recognized as a growth-promoting factor in salmonids (218), is similar to that of igfbp-2b at a lower expression level, but importantly the regulation of igfbp-4 appears especially sensitive to nutrient deficiencies in essential fatty acids and phosphorus (Figures 10A–C).
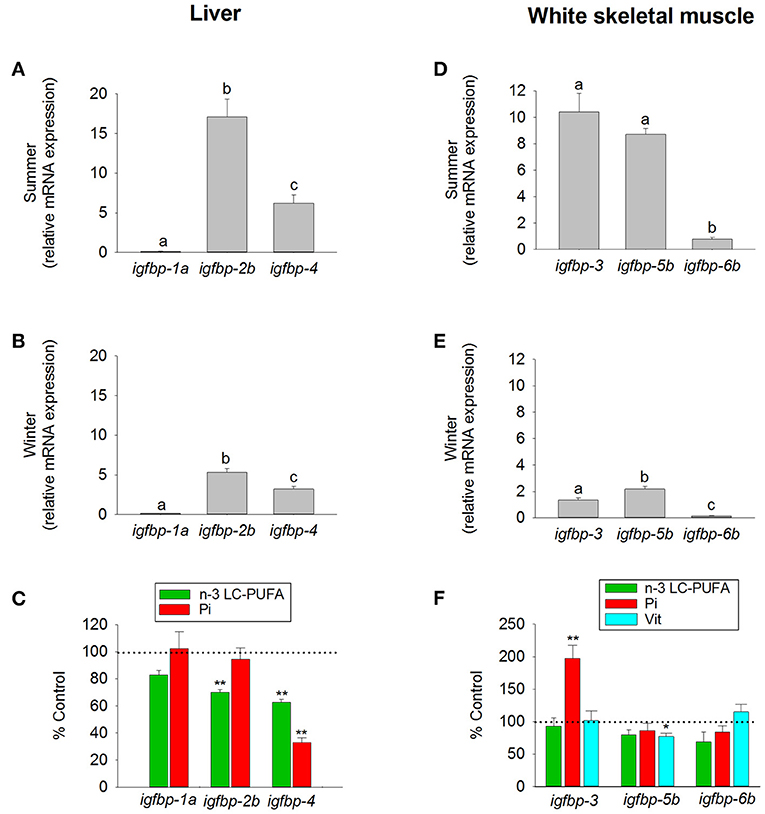
Figure 10. Tissue-specific regulation of igfbp by season and diet in juveniles of gilthead sea bream. Liver expression of igfbp-1a, igfbp-2b, and igfbp-4 (clade igfbp-1/-2/-4) was analyzed in fishes during (A) summer, (B) winter, and (C) after a dietary challenge with a diet deficient in n-3 LC-PUFA (green bars) or phosphorous (Pi, red bars). White skeletal muscle expression of igfbp-3 (primers detected both igfbp-3a and -3b paralogs), igfbp-5b, and igfbp-6b (clade igfbp-3/-5/-6) was analyzed in fishes during (D) summer, (E) winter, and (F) after a dietary challenge with a diet deficient in n-3 LC-PUFA (green bars), phosphorus (Pi, red bars) or vitamins (Vit, cyan bars). RT-qPCR of tissue total RNA was conducted as previously reported (134). Data are represented as the mean ± SEM (n = 6–7). For each tissue and season (A,B,D,E), different superscript letters indicate significant differences (P < 0.05; ANOVA followed by Student-Newman–Keuls test). Asterisks in (C,F) indicate significant differences compared with paralog expression in fishes from group fed a control diet (t-test, *P < 0.05; **P < 0.001). Data derived from samples from Benedito-Palos et al. (146) and Ballester-Lozano et al. (152).
Igfbp-3 seems to have less of a common role among fish lineages. Indeed, studies in zebrafish, flounder and yellowtail reported a significant increase in the expression level of igfbp-3 in liver and/or muscle in response to fasting (224–226), which may act to restrict Igf signaling. By contrast, studies in trout and Atlantic salmon have reported no changes in the expression of igfbp-3 in response to fasting (63, 218), whereas the muscle expression of igfbp-3a1 is enhanced by gh-transgenesis in coho salmon (227), which is consistent with a growth-promoting action in salmonids. To make matters more complicated, in gilthead sea bream, the expression of muscle igfbp-3 is elevated not only during the summer growth enhancement but also during growth impairments due to phosphorus deficiencies, which supports both growth-promoting and -inhibiting roles depending on the physiological context. In contrast, the main role of Igfbp-5b is to promote growth in response to changes in gene expression with the season and nutritional status (Figures 10D–F). Overall, the available evidence for Igfbp-5 supports a growth-promoting function in salmonids, but again functional divergence has been reported across species and physiological contexts [see García de la Serrana and Macqueen (218)]. Conversely, Igfbp-6 is emerging as a growth-inhibitory factor, though this Igfbp is particularly understudied, and it is difficult to draw overarching conclusions. However, taking into account its relatively low expression in the liver and skeletal muscle of well-nourished fishes, the proposed role for Igbp-6b in gilthead sea bream is closer to a growth-inhibiting rather than a growth-promoting action.
Concluding Remarks and Prospects
The GH/IGF system plays a key role in the endocrine cascade of growth, and overall changes at the protein and mRNA levels closely reflect differences in growth performance through development and production cycles. However, this relationship varies within and across fish species and physiological contexts from tight to non-discernible correlations due to the actions of a wide range of endogenous and exogenous factors. Despite this, changes in circulating GH and IGF-I levels continue to be one of the most robust markers of growth performance through vertebrate evolution. This is especially relevant in fish species since gene expansion by local or whole-genome duplications offers the possibility of a complex gene subfunctionalization and/or acquisition of novel functions. This is evidenced at the ligand level for Gh and Sl and at the receptor level for the ghr-i and ghr-ii genes, which are differentially regulated in liver and skeletal muscle, helping to distinguish stressful and growth disturbances due to overwintering or malnutrition as a result of changes in feed intake, protein and lipid feedstuffs or any other specific nutrient. Close links between oxygen availability, energy status and the somatotropic axis are also now emerging via Sirts, which are potential markers of informing of energy status and can modulate the anabolic action of Gh by inhibiting Ghr signaling. The differential regulation of igf-i and igf-ii genes in liver and skeletal muscle also offers the possibility of a more refined analysis of growth potentiality and nutritional condition. Igfbps are emerging as highly regulated components of the Gh/Igf system, though the puzzle is far from complete because their tissue-specific regulation has not been established for all paralogs across fish species and different physiological conditions. Thus, further research is needed to combine the search for a robust, highly specific set of biomarkers for a given growth derangement, which may have an impact later in life by means of different epigenetic mechanisms, involving changes in DNA methylation and histone acetylation, among others (228).
The combination of conventional and different -omic approaches (functional genomics, proteomics, metabolomics, metagenomics) have been gaining acceptance as an option to assess the nutritionally and environmentally mediated effects on the growth performance, metabolic homeostasis, stress responsiveness and health condition of farmed fishes. However, at the present stage, a reliable diagnostic should combine measures of conventional biomarkers (e.g., data on blood biochemistry and histopathological scoring) with molecular signatures of the different components of the Gh/Igf system in addition to other related markers of growth and cell differentiation and proliferation, protein breakdown, protein folding and assembly, inflammatory/anti-inflammatory response, energy sensing, OXPHOS, mitochondrial respiration uncoupling, and lipolytic/lipogenic activity. In our hands, a key point for the simultaneous gene expression profiling of all the genes included in our growth PCR-array is the operation of the analytical platform by handling robots, resulting in a minimal variation between technical replicates. By means of multivariate analysis, this offers the possibility to identify at a high level of confidence the most responsive tissues and biomarkers in animals facing a given stressful rearing condition. This is exemplified herein by comparing the molecular signatures of liver and skeletal muscle of fishes with signs of nutrient deficiencies in n-3 LC-PUFA, phosphorus, or vitamins (Figures 11, 12). These three types of nutrient deficiencies were chosen because they are considered the most constraining factors of FM/FO replacement by alternative plant ingredients in marine farmed fishes. Using this approach, discriminant analysis (PLS-DA) is able to explain more than 93% of the variance (R) and to predict more than 72% of the total variance (Q). Thus, from this meta-analysis, it is conclusive that the liver tissue is especially responsive to deficiencies in essential fatty acids or phosphorus, whereas skeletal muscle is emerging as the main target tissue for the diagnosis of vitamin deficiencies. This is supported by variable importance (VIP) analysis, which highlights the different contribution of the 73–84 genes analyzed in our growth-arrays. This is just one example of what can be done with this type of approach, helping to establish the normal range of variance of highly informative biomarkers as a function of developmental stage and nutritional background. This procedure is based on the ARRAINA-derived biomarkers (229), and current research is taking advantage of this knowledge within the PerformFISH and GAIN H2020 EU Projects to validate, at the pilot scale and farm levels, new rearing systems and diet formulations for European aquaculture intensification. How all this is affected by nutrition and genome interactions is, however, a major challenge in efficiently managing aquaculture breeding programs and producing more robust farmed fishes, fed sustainable diets in a changing environment. In this regard, integrative studies on fish endocrinology can help to establish the best phenotype and the normal range of reference for different growth-promoting factors in animals with different nutritional and environmental backgrounds, allowing us to re-evaluate the nutritional status and nutrient requirements. How this can also include other criteria, such as functional microbiota, requires more research, but the endocrine system can help explain the now-emerging distal effects of intestinal microbiota on productive traits other than those more directly related to intestinal health. For instance, a growing body of evidence is pointing out that microbiota modulates host circulating Igf-i levels (230), a feature that seems to be conserved in fishes (231), and probably mediated by microbiota production of short-chain fatty acids. However, studies in fishes relating the composition of the core microbiota to a wide range of endogenous and exogenous factors are still in their infancy.
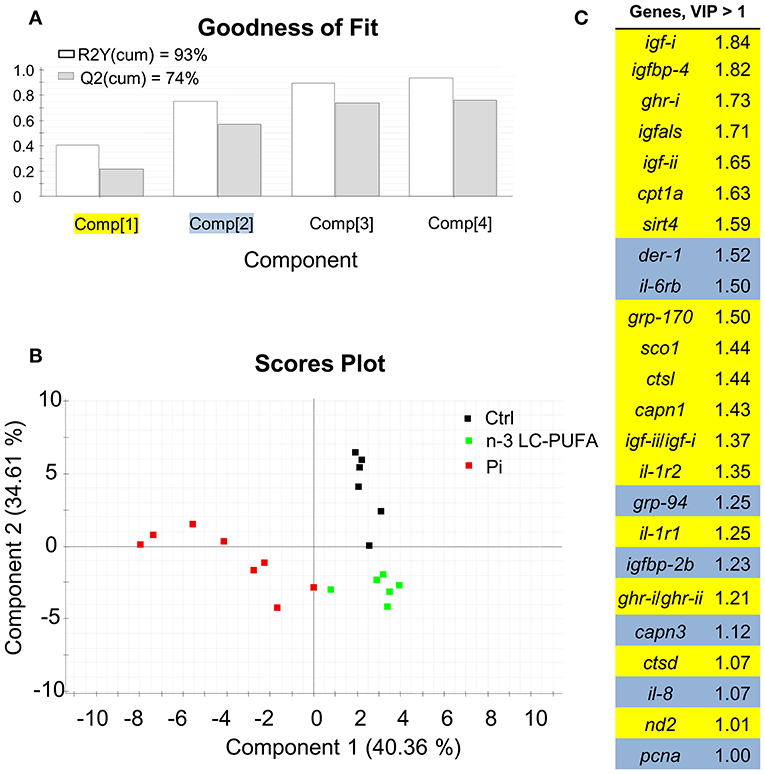
Figure 11. Discriminant analysis (PLS-DA) of liver molecular signatures of fishes fed nutrient deficient diets. Relative expression data of the 73 genes included in the array can be found in Table S1. (A) Cumulative coefficients of goodness of fit (R2, white bars) and prediction (Q2, gray bars) by each component; the two first components explained 74.97% of total variance. (B) PLS-DA score plot of acquired data from dietary challenged individuals along the two main components. Individuals fed the phosphorus deficient diet (Pi, red squares) are separated along the first component, and component 2 separates individuals fed the control (Ctrl, black squares) and LC-PUFA-deficient diets (green squares). (C) Ordered list of markers by variable importance (VIP) in the projection of PLS-DA model for group differentiation. Markers with VIP values >1 after the first or second component are highlighted in yellow and blue, respectively. Data derived from samples from Ballester-Lozano et al. (152).
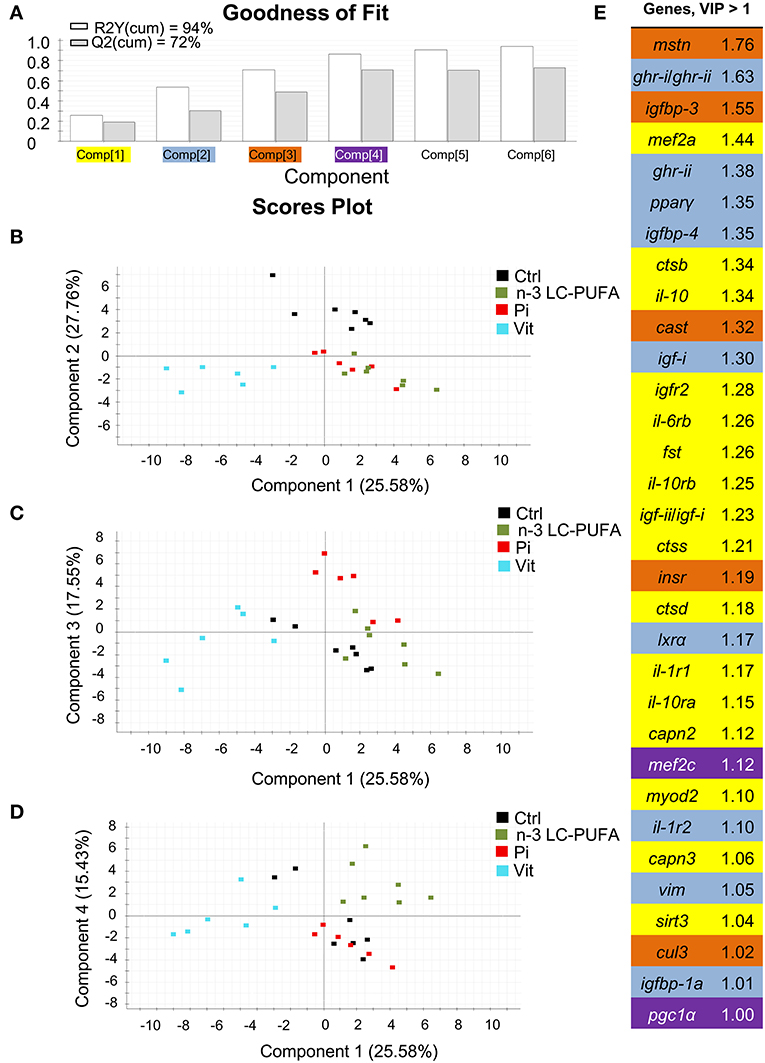
Figure 12. Discriminant analysis (PLS-DA) of skeletal muscle molecular signatures of fishes fed nutrient deficient diets. Relative expression data of 84 genes can be found in Table S2. (A) Cumulative coefficients of goodness of fit (R2, white bars) and prediction (Q2, gray bars) by each component; 86.32% of total variance is explained by four components. (B) PLS-DA score plot of acquired data from dietary-challenged individuals along components 1 and 2. Individuals fed the vitamin-deficient diet (Vit, cyan squares) are separated along the first component. (C) PLS-DA score plot of acquired data from dietary-challenged individuals along components 1 and 3. Component 3 separates individuals fed the phosphorus-deficient diet (Pi, red squares). (D) PLS-DA score plot of acquired data from dietary-challenged individuals along components 1 and 4. Component 4 separates individuals fed the LC-PUFA-deficient diet (green squares). (E) Ordered list of markers by variable importance (VIP) in the projection of PLS-DA model for group differentiation. Markers with VIP values >1 after the first, second, third and fourth components are highlighted in yellow, blue, orange and purple, respectively. Data derived from samples from Ballester-Lozano et al. (152).
Author Contributions
JP-S: writing, reviewing, and conception; PS-M, FN-C, JM-S, EP, AB-N, and LB-P: writing; JC-G: writing and reviewing.
Funding
This work was funded by ARRAINA (Advanced Research Initiatives for Nutrition & Aquaculture; KBBE-2011-288925) EU Project. Additional funding was obtained from Spanish MI2-Fish Project (MINECO, AGL2013-48560) and PerformFISH (Integrating Innovative Approaches for Competitive and Sustainable Performance across the Mediterranean Aquaculture Value Chain; H2020-SFS-2016-2017; 727610) EU Project. This publication reflects the views only of the authors, and the European Commission cannot be held responsible for any use which may be made of the information contained therein.
Conflict of Interest Statement
The authors declare that the research was conducted in the absence of any commercial or financial relationships that could be construed as a potential conflict of interest.
Supplementary Material
The Supplementary Material for this article can be found online at: https://www.frontiersin.org/articles/10.3389/fendo.2018.00687/full#supplementary-material
References
1. Ono M, Takayama Y, Rand-Weaver M, Sakata S, Yasunaga T, Noso T, et al. cDNA cloning of somatolactin, a pituitary protein related to growth hormone and prolactin. Proc Natl Acad Sci USA. (1990) 87:4330–4.
2. Rand-Weaver M, Noso T, Muramoto K, Kawauchi H. Isolation and characterization of somatolactin, a new protein related to growth hormone and prolactin from Atlantic cod (Gadus morhua) pituitary glands. Biochemistry (1991) 30:1509–15. doi: 10.1021/bi00220a010
3. Takayama Y, Ono M, Rand-Weaver M, Kawauchi H. Greater conservation of somatolactin, a presumed pituitary hormone of the growth hormone/prolactin family, than of growth hormone in teleost fish. Gen Comp Endocrinol. (1991) 83:366–74. doi: 10.1016/0016-6480(91)90141-R
4. Pendón C, Martínez-Barberá JP, Valdivia MM. Cloning of a somatolactin-encoding cDNA from sole (Solea senegalensis). Gene (1994) 147:227–30. doi: 10.1016/0378-1119(94)90071-X
5. Calduch-Giner JA, Pendón C, Valdivia MM, Pérez-Sánchez J. Recombinant somatolactin as a stable and bioactive protein in a cell culture bioassay: development and validation of a sensitive and reproducible radioimmunoassay. J Endocrinol. (1998) 156:441–7. doi: 10.1677/joe.0.1560441
6. Cavari B, Noso T, Kawauchi H. Isolation and characterization of somatolactin from pituitary glands of gilthead sea bream Sparus aurata. Aquaculture (1995) 137:171–8. doi: 10.1016/0044-8486(95)01113-7
7. Astola A, Pendón C, Ortíz M, Valdivia MM. Cloning and expression of somatolactin, a pituitary hormone related to growth hormone and prolactin from gilthead seabream, Sparus aurata. Gen Comp Endocrinol. (1996) 104:330–6. doi: 10.1006/gcen.1996.0178
8. Cheng KW, Chan YH, Chen YD, Yu KL, Chan KM. Sequence of a cDNA clone encoding a novel somatolactin in goldfish, Carassius auratus. Biochem Biophys Res Commun. (1997) 232:282–7. doi: 10.1006/bbrc.1997.6271
9. May D, Todd CM, Rand-Weaver M. cDNA cloning of eel (Anguilla anguilla) somatolactin. Gene (1997) 188:63–7. doi: 10.1016/S0378-1119(96)00777-9
10. Yang BY, Arab M, Chen TT. Cloning and characterization of rainbow trout (Oncorhynchus mykiss) somatolactin cDNA and its expression in pituitary and nonpituitary tissues. Gen Comp Endocrinol. (1997) 106:271–80. doi: 10.1006/gcen.1996.6880
11. Company R, Calduch-Giner JA, Mingarro M, Pérez-Sánchez J. cDNA cloning and sequence of European sea bass (Dicentrarchus labrax) somatolactin. Comp Biochem Physiol. (2000) 127:183–92. doi: 10.1016/S0305-0491(00)00250-9
12. Vega-Rubín de Celis S, Gómez P, Calduch-Giner JA, Médale F, Pérez-Sánchez J. Expression and characterization of European sea bass (Dicentrarchus labrax) somatolactin: assessment of in vivo metabolic effects. Mar Biotechnol. (2003) 5:92–101. doi: 10.1007/s10126-002-0053-6
13. Amemiya Y, Sogabe Y, Nozaki M, Takahashi A, Kawauchi H. Somatolactin in the white sturgeon and African lungfish and its evolutionary significance. Gen Comp Endocrinol. (1999) 114:181–90. doi: 10.1006/gcen.1998.7250
14. Zhu Y, Stiller JW, Shaner MP, Baldini A, Scemama JL, Capehart AA. Cloning of somatolactin alpha and beta cDNAs in zebrafish and phylogenetic analysis of two distinct somatolactin subtypes in fish. J Endocrinol. (2004) 182:509–18. doi: 10.1677/joe.0.1820509
15. Benedet S, Björnsson BT, Taranger GL, Andersson E. Cloning of somatolactin alpha, beta forms and the somatolactin receptor in Atlantic salmon: seasonal expression profile in pituitary and ovary of maturing female broodstock. Reprod Biol Endocrinol. (2008) 6:42. doi: 10.1186/1477-7827-6-42
16. Ocampo Daza D, Larhammar D. Evolution of the growth hormone, prolactin, prolactin 2 and somatolactin family. Gen Comp Endocrinol. (2018) 264:94–112. doi: 10.1016/j.ygcen.2018.01.007
17. Huang X, Hui MN, Liu Y, Yuen DS, Zhang Y, Chan WY, et al. Discovery of a novel prolactin in non-mammalian vertebrates: evolutionary perspectives and its involvement in teleost retina development. PLoS ONE (2009) 4:e6163. doi: 10.1371/journal.pone.0006163
18. Wang Y, Li J, Kwok AHY, Ge W, Leung FC. A novel prolactin-like protein (PRL-L) gene in chickens and zebrafish: cloning and characterization of its tissue expression. Gen Comp Endocrinol. (2010) 166:200–10. doi: 10.1016/j.ygcen.2009.10.007
19. Wallis M, Wallis OC. Growth hormone and prolactin in new world monkeys. In: Barrera-Saldaña HA, editor. Brain Development, Social and Hormonal Mechanisms and Zoonotic Diseases. New York, NY: Animal Science, Issues and Professions Nova Publishers (2014). p. 165–84.
20. Pérez-Sánchez J, Calduch-Giner JA, Mingarro M, de Celis SVR, Gómez-Requeni P, Saera-Vila A, et al. Overview of fish growth hormone family. New insights in genomic organization and heterogeneity of growth hormone receptors. Fish Physiol Biochem. (2002) 27:243–58. doi: 10.1023/B:FISH.0000032729.72746.c8
21. Power DM. Developmental ontogeny of prolactin and its receptor in fish. Gen Comp Endocrinol. (2005) 142:25–33. doi: 10.1016/j.ygcen.2004.10.003
22. Whittington CM, Wilson AB. The role of prolactin in fish reproduction. Gen Comp Endocrinol. (2013) 191:123–36. doi: 10.1016/j.ygcen.2013.05.027
23. Forsyth IA, Wallis M. Growth hormone and prolactin—molecular and functional evolution. J Mammary Gland Biol Neoplasia (2002) 7:291–312. doi: 10.1023/A:1022804817104
24. Ocampo Daza D, Sundström G, Larsson TA, Larhammar D. Evolution of the growth hormone-prolactin-somatolactin system in relation to vertebrate tetraploidizations. Ann NY Acad Sci. (2009) 1163:491–3. doi: 10.1111/j.1749-6632.2008.03671.x
25. Ocampo Daza D, Larhammar D. Evolution of the receptors for growth hormone, prolactin, erythropoietin and thrombopoietin in relation to the vertebrate tetraploidizations. Gen Comp Endocrinol. (2018) 257:143–60. doi: 10.1016/j.ygcen.2017.06.021
26. Fukada H, Ozaki Y, Pierce AL, Adachi S, Yamauchi K, Hara A, et al. Identification of the salmon somatolactin receptor, a new member of the cytokine receptor family. Endocrinology (2005) 146:2354–61. doi: 10.1210/en.2004-1578
27. Jiao B, Huang X, Chan CB, Zhang L, Wang D, Cheng CH. The co-existence of two growth hormone receptors in teleost fish and their differential signal transduction, tissue distribution and hormonal regulation of expression in seabream. J Mol Endocrinol. (2006) 36:23–40. doi: 10.1677/jme.1.01945
28. Chen M, Huang X, Yuen DS, Cheng CH. A study on the functional interaction between the GH/PRL family of polypeptides with their receptors in zebrafish: evidence against GHR1 being the receptor for somatolactin. Mol Cell Endocrinol. (2011) 337:114–21. doi: 10.1016/j.mce.2011.02.006
29. Ozaki Y, Fukada H, Tanaka H, Kagawa H, Ohta H, Adachi S, et al. Expression of growth hormone family and growth hormone receptor during early development in the Japanese eel (Anguilla japonica). Comp Biochem Physiol B Biochem Mol Biol. (2006) 145:27–34. doi: 10.1016/j.cbpb.2006.05.009
30. Reindl KM, Kittilson JD, Sheridan MA. Differential ligand binding and agonist-induced regulation characteristics of the two rainbow trout GH receptors, Ghr1 and Ghr2, in transfected cells. J Endocrinol. (2009) 202:463–71. doi: 10.1677/JOE-09-0057
31. Bergan-Roller HE, Sheridan MA. The growth hormone signaling system: insights into coordinating the anabolic and catabolic actions of growth hormone. Gen Comp Endocrinol. (2018) 258:119–33. doi: 10.1016/j.ygcen.2017.07.028
32. Shearer KD, Swanson P. The effect of whole body lipid on early sexual maturation of 1+ age male chinook salmon (Oncorhynchus tshawytscha). Aquaculture (2000) 190:343–67. doi: 10.1016/S0044-8486(00)00406-3
33. Silverstein JT, Shearer KD, Dickhoff WW, Plisetskaya EM. Effects of growth and fatness on sexual development of chinook salmon (Oncorhynchus tshawytscha) parr. Can J Fish Aquat Sci. (1998) 55:2376–82. doi: 10.1139/f98-111
34. Mingarro M, de Celis SVR, Astola A, Pendón C, Valdivia MM, Pérez-Sánchez J. Endocrine mediators of seasonal growth in gilthead sea bream (Sparus aurata): the growth hormone and somatolactin paradigm. Gen Comp Endocrinol. (2002) 128:102–11. doi: 10.1016/S0016-6480(02)00042-4
35. Company R, Mingarro M, Astola A, Pendón C, Valdivia M, Pérez-Sánchez J. Nutrient and endocrine regulation of growth and adiposity. Growth hormone and somatolactin relationship. Comp Biochem Physiol. (2001) 130:435–45. doi: 10.1016/S1532-0456(01)00269-1
36. Vega-Rubín S, Rojas P, Gómez-Requeni P, Albalat A, Gutiérrez J, Médale F, et al. Nutritional assessment of somatolactin function in gilthead sea bream (Sparus aurata): concurrent changes in somatotropic axis and pancreatic hormones. Comp Biochem Physiol A Mol Integr Physiol. (2004) 138:533–42. doi: 10.1016/j.cbpb.2004.06.007
37. Peyon P, de Celis SVR, Gómez-Requeni P, Zanuy S, Pérez-Sánchez J, Carrillo M. In vitro effect of leptin on somatolactin release in the European sea bass (Dicentrarchus labrax): dependence on the reproductive status and interaction with NPY and GnRH. Gen Comp Endocrinol. (2003) 132:284–92. doi: 10.1016/S0016-6480(03)00097-2
38. Vega-Rubín de Celis S, Gómez-Requeni P, Pérez-Sánchez J. Production and characterization of recombinantly derived peptides and antibodies for accurate determinations of somatolactin, growth hormone and insulin-like growth factor-I in European sea bass (Dicentrarchus labrax). Gen Comp Endocrinol. (2004) 139:266–77. doi: 10.1016/j.ygcen.2004.09.017
39. Rand-Weaver M, Swanson P, Kawauchi H, Dickhoff WW. Somatolactin, a novel pituitary protein: purification and plasma levels during reproductive maturation of coho salmon. J Endocrinol. (1992) 133:393–403. doi: 10.1677/joe.0.1330393
40. Rand-Weaver M, Pottinger TG, Sumpter JP. Plasma somatolactin concentrations in salmonid fish are elevated by stress. J Endocrinol. (1993) 138:509–15. doi: 10.1677/joe.0.1380509
41. Kakizawa S, Kaneko T, Hasegawa S, Hirano T. Effects of feeding, fasting, background adaptation, acute stress, and exhaustive exercise on the plasma somatolactin concentrations in rainbow trout. Gen Comp Endocrinol. (1995) 98:137–46. doi: 10.1006/gcen.1995.1054
42. Kakizawa S, Kaneko T, Ogasawara T, Hirano T. Changes in plasma somatolactin levels during spawning migration of chum salmon (Oncorhynchus keta). Fish Physiol Biochem. (1995) 14:93–101. doi: 10.1007/BF00002453
43. Sasano Y, Yoshimura A, Fukamachi S. Reassessment of the function of somatolactin alpha in lipid metabolism using medaka mutant and transgenic strains. BMC Genet. (2012) 13:64. doi: 10.1186/1471-2156-13-64
44. Duan C, Duguay SJ, Plisetskaya EM. Insulin-like growth factor I (IGF-I) mRNA expression in coho salmon, Oncorhynchus kisutch: tissue distribution and effects of growth hormone/prolactin family proteins. Fish Physiol Biochem. (1993) 11:371–9. doi: 10.1007/BF00004587
45. Duan C, Duguay SJ, Swanson P, Dickhoff WW, Plisetskaya EM. Tissue-specific expression of insulin-like growth factor I mRNAs in salmonids: developmental, hormonal, and nutritional regulation. In: Davey KG, Tobe SS, Peter DE, editors. Perspectives in Comparative Endocrinology. Toronto, ON: National Research Council of Canada (1994). p. 365–72.
46. Harvey S, Azumaya Y, Hull KL. Pituitary and extrapituitary growth hormone: pit-1 dependence? Can J Physiol Pharmacol. (2000) 78:1013–28. doi: 10.1139/y00-095
47. Wood AW, Duan C, Bern HA. Insulin-like growth factor signaling in fish. Int Rev Cytol. (2005) 243:215–85. doi: 10.1016/S0074-7696(05)43004-1
48. Harvey S. Extrapituitary growth hormone. Endocrine (2010) 38:335–59. doi: 10.1007/s12020-010-9403-8
49. Harvey S. Growth hormone and growth? Gen Comp Endocrinol. (2013) 190:3–9. doi: 10.1016/j.ygcen.2007.01.045
50. Harvey S, Baudet ML. Extrapituitary growth hormone and growth? Gen Comp Endocrinol. (2014) 205:55–61. doi: 10.1016/j.ygcen.2014.03.041
51. Pérez-Ibave DC, Rodríguez-Sánchez IP, de Lourdes Garza-Rodríguez M, Barrera-Saldaña HA. Extrapituitary growth hormone synthesis in humans. Growth Horm IGF Res. (2014) 24:47–53. doi: 10.1016/j.ghir.2014.01.005
52. Harvey S, Martínez-Moreno CG, Luna M, Arámburo C. Autocrine/paracrine roles of extrapituitary growth hormone and prolactin in health and disease: an overview. Gen Comp Endocrinol. (2015) 220:103–11. doi: 10.1016/j.ygcen.2014.11.004
53. Calduch-Giner JA, Pérez-Sánchez J. Expression of growth hormone (GH) gene in the head kidney of sea bream (Sparus aurata). J Exp Zool. (1999) 283:326–30. doi: 10.1002/(SICI)1097-010X(19990215)283:3<326::AID-JEZ10>3.0.CO;2-3
54. Gomez JM, Mourot B, Fostier A, Le Gac F. Growth hormone receptors in ovary and liver during gametogenesis in female rainbow trout (Oncorhynchus mykiss). J Reprod Fertil. (1999) 115:275–85. doi: 10.1530/jrf.0.1150275
55. Miura C, Shimizu Y, Uehara M, Ozaki Y, Young G, Miura T. Growth hormone is produced by the testis of Japanese eel and stimulates proliferation of spermatogonia. Reproduction (2011) 142:869–77. doi: 10.1530/REP-11-0203
56. Ayson FG, Kaneko T, Hasegawa S, Hirano T. Differential expression of two prolactin and growth hormone genes during early development of tilapia (Oreochromis mossambicus) in fresh water and seawater: implications for possible involvement in osmoregulation during early life stages. Gen Comp Endocrinol. (1994) 95:143–52. doi: 10.1006/gcen.1994.1111
57. Yang BY, Greene M, Chen TT. Early embryonic expression of the growth hormone family protein genes in the developing rainbow trout, Oncorhynchus mykiss. Mol Reprod Dev. (1999) 53:127–34. doi: 10.1002/(SICI)1098-2795(199906)53:2<127::AID-MRD1>3.0.CO;2-H
58. Besseau L, Fuentès M, Sauzet S, Beauchaud M, Chatain B, Covès D, et al. Somatotropic axis genes are expressed before pituitary onset during zebrafish and sea bass development. Gen Comp Endocrinol. (2013) 194:133–41. doi: 10.1016/j.ygcen.2013.08.018
59. Martí-Palanca H, Pérez-Sánchez J. Developmental regulation of growth hormone binding in the gilthead sea bream, Sparus aurata. Growth Regul. (1994) 4:14–9.
60. Reindl KM, Sheridan MA. Peripheral regulation of the growth hormone-insulin-like growth factor system in fish and other vertebrates. Comp Biochem Physiol A Mol Integr Physiol. (2012) 163:231–45. doi: 10.1016/j.cbpa.2012.08.003
61. Beckman BR. Perspectives on concordant and discordant relations between insulin-like growth factor 1 (IGF1) and growth in fishes. Gen Comp Endocrinol. (2011) 170:233–52. doi: 10.1016/j.ygcen.2010.08.009
62. Pérez-Sánchez J, Martí-Palanca H, Kaushik SJ. Ration size and protein intake affect circulating growth hormone concentration, hepatic growth hormone binding and plasma insulin-like growth factor-I immunoreactivity in a marine teleost, the gilthead sea bream (Sparus aurata). J Nutr. (1995) 125:546–52. doi: 10.1093/jn/125.3.546
63. Gabillard JC, Kamangar BB, Montserrat N. Coordinated regulation of the GH/IGF system genes during refeeding in rainbow trout (Oncorhynchus mykiss). J Endocrinol. (2006) 191:15–24. doi: 10.1677/joe.1.06869
64. Shimizu M, Cooper KA, Dickhoff WW, Beckman BR. Postprandial changes in plasma growth hormone, insulin, insulin-like growth factor (IGF)-I, and IGF-binding proteins in coho salmon fasted for varying periods. Am J Physiol Regul Integr Comp Physiol. (2009) 297:R352–61. doi: 10.1152/ajpregu.90939.2008
65. Li MH, Peterson BC, Janes CL, Robinson EH. Comparison of diets containing various fish meal levels on growth performance, body composition, and insulin-like growth factor-I of juvenile channel catfish Ictalurus punctatus of different strains. Aquaculture (2006) 253:628–35. doi: 10.1016/j.aquaculture.2005.09.024
66. Lankford SE, Weber GM. Associations between plasma growth hormone, insulin-like growth factor-I, and cortisol with stress responsiveness and growth performance in a selective breeding program for rainbow trout. N Am J Aquac. (2006) 68:151–9. doi: 10.1577/A05-014.1
67. Pierce AL, Beckman BR, Shearer KD, Larsen DA, Dickhoff WW. Effects of ration on somatotropic hormones and growth in Coho salmon. Comp Biochem Physiol B Biochem Mol Biol. (2001) 128:255–64. doi: 10.1016/S1096-4959(00)00324-9
68. Beckman BR, Larsen DA, Moriyama S, Lee-Pawlak B, Dickhoff WW. Insulin-like growth factor-I and environmental modulation of growth during smoltification of spring Chinook salmon (Oncorhynchus tshawytscha). Gen Comp Endocrinol. (1998) 109:325–35. doi: 10.1006/gcen.1997.7036
69. Taylor JF, Migaud H, Porter MJR, Bromage NR. Photoperiod influences growth rate and plasma insulin-like growth factor-I levels in juvenile rainbow trout, Oncorhynchus mykiss. Gen Comp Endocrinol. (2005) 142:169–85. doi: 10.1016/j.ygcen.2005.02.006
70. Picha ME, Turano MJ, Tipsmark CK, Borski RJ. Regulation of endocrine and paracrine sources of Igfs and Gh receptor during compensatory growth in hybrid striped bass (Morone chrysops × Morone saxatilis). J Endocrinol. (2008) 199:81–94. doi: 10.1677/JOE-07-0649
71. Andrews KS, Beckman BR, Beaudreau AH, Larsen DA, Williams GD, Levin PS. Suitability of insulin-like growth factor 1 (IGF1) as a measure of relative growth rates in lingcod. Mar Coast Fish. (2011) 3:250–60. doi: 10.1080/19425120.2011.588921
72. Vera EM, Brown CL, Luckenbach JA, Picha ME, Bolivar RB, Borski RJ. Insulin-like growth factor-I cDNA cloning, gene expression and potential use as a growth rate indicator in Nile tilapia, Oreochromis niloticus. Aquaculture (2006) 251:585–95. doi: 10.1016/j.aquaculture.2005.06.039
73. Saera-Vila A, Calduch-Giner JA, Pérez-Sánchez J. Co-expression of IGFs and GH receptors (GHRs) in gilthead sea bream (Sparus aurata L.): sequence analysis of the GHR-flanking region. J Endocrinol. (2007) 194:361–72. doi: 10.1677/JOE-06-0229
74. Montserrat N, Gómez-Requeni P, Bellini G, Capilla E, Pérez-Sánchez J, Navarro I, et al. Distinct role of insulin and IGF-I and its receptors in white skeletal muscle during the compensatory growth of gilthead sea bream (Sparus aurata). Aquaculture (2007) 267:188–98. doi: 10.1016/j.aquaculture.2007.04.024
75. Blasco J, Moya A, Millán-Cubillo A, Vélez EJ, Capilla E, Pérez-Sánchez J, et al. Growth-promoting effects of sustained swimming in fingerlings of gilthead sea bream (Sparus aurata L.). J Comp Physiol B (2015) 185:859–68. doi: 10.1007/s00360-015-0933-5
76. Vélez EJ, Azizi S, Millán-Cubillo A, Fernández-Borràs J, Blasco J, Chan SJ, et al. Effects of sustained exercise on GH-IGFs axis in gilthead sea bream (Sparus aurata). Am J Physiol Regul Integr Comp Physiol. (2016) 310:R313–22. doi: 10.1152/ajpregu.00230.2015
77. Vélez EJ, Perelló M, Azizi S, Moya A, Lutfi E, Pérez-Sánchez J, et al. Recombinant bovine growth hormone (rBGH) enhances somatic growth by regulating the GH-IGF axis in fingerlings of gilthead sea bream (Sparus aurata). Gen Comp Endocrinol. (2018) 257:192–202. doi: 10.1016/j.ygcen.2017.06.019
78. Gómez-Requeni P, Calduch-Giner J, de Celis SVR, Médale F, Kaushik SJ, Pérez-Sánchez J. Regulation of the somatotropic axis by dietary factors in rainbow trout (Oncorhynchus mykiss). Br J Nutr. (2005) 94:353–61. doi: 10.1111/j.1365-2109.2009.02173.x
79. Mancera JM, McCormick SD. Osmoregulatory actions of the GH/IGF axis in non-salmonid teleosts. Comp Biochem Physiol B Biochem Mol Biol. (1998) 121:43–8. doi: 10.1016/S0305-0491(98)10112-8
80. Sakamoto T, McCormick SD, Hirano T. Osmoregulatory actions of growth hormone and its mode of action in salmonids: a review. Fish Physiol Biochem. (1993) 11:155–64. doi: 10.1007/BF00004562
81. Ayson FG, Kaneko T, Tagawa M, Hasegawa S, Grau EG, Nishioka RS, et al. Effects of acclimation to hypertonic environment on plasma and pituitary levels of two prolactins and growth hormone in two species of tilapia, Oreochromis mossambicus and Oreochromis niloticus. Gen Comp Endocrinol. (1993) 89:138–48. doi: 10.1006/gcen.1993.1017
82. Nishioka RS, de Jesus EGT, Hyodo S. Localization of mRNAs for a pair of prolactins and growth hormone in the tilapia pituitary using in situ hybridization with oligonucleotide probes. Gen Comp Endocrinol. (1993) 89:72–81. doi: 10.1006/gcen.1993.1010
83. Auperin B, Leguen I, Rentier-Delrue F, Smal J, Prunet P. Absence of a tiGH effect on adaptability to brackish water in tilapia (Oreochromis niloticus). Gen Comp Endocrinol. (1995) 97:145–59. doi: 10.1006/gcen.1995.1014
84. Yada T, Hirano T, Grau EG. Changes in plasma levels of the two prolactins and growth hormone during adaptation to different salinities in the euryhaline tilapia, Oreochromis mossambicus. Gen Comp Endocrinol. (1994) 93:214–23. doi: 10.1006/gcen.1994.1025
85. Vijayan M, Morgan J, Sakamoto T, Grau E, Iwama G. Food-deprivation affects seawater acclimation in tilapia: hormonal and metabolic changes. J Exp Biol. (1996) 199:2467–75.
86. Morgan JD, Sakamoto T, Grau EG, Iwama GK. Physiological and respiratory responses of the Mozambique tilapia (Oreochromis mossambicus) to salinity acclimation. Comp Biochem Physiol A Mol Integr Physiol. (1997) 117:391–8. doi: 10.1016/S0300-9629(96)00261-7
87. Suzuki R, Kishida M, Hirano T. Growth hormone secretion during longterm incubation of the pituitary of the Japanese eel, Anguilla japonica. Fish Physiol Biochem. (1990) 8:159–65. doi: 10.1007/BF00004443
88. Suzuki R, Kaneko T, Hirano T. Effects of osmotic pressure on prolactin and growth hormone secretion from organ-cultured eel pituitary. J Comp Physiol B (1991) 161:147–53.
89. Laiz-Carrión R, Fuentes J, Redruello B, Guzmán JM, del Río MPM, Power D, et al. Expression of pituitary prolactin, growth hormone and somatolactin is modified in response to different stressors (salinity, crowding and food-deprivation) in gilthead sea bream Sparus auratus. Gen Comp Endocrinol. (2009) 162:293–300. doi: 10.1016/j.ygcen.2009.03.026
90. Mohammed-Geba K, González AA, Suárez RA, Galal-Khallaf A, Martos-Sitcha JA, Ibrahim HM, et al. Molecular performance of Prl and Gh/Igf1 axis in the Mediterranean meager, Argyrosomus regius, acclimated to different rearing salinities. Fish Physiol Biochem. (2017) 43:203–16. doi: 10.1007/s10695-016-0280-9
91. Deane EE, Woo NY. Differential gene expression associated with euryhalinity in sea bream (Sparus sarba). Am J Physiol Regul Integr Comp Physiol. (2004) 287:R1054–63. doi: 10.1152/ajpregu.00347.2004
92. Deane EE, Woo NY. Molecular cloning of growth hormone from silver sea bream: effects of abiotic and biotic stress on transcriptional and translational expression. Biochem Biophys Res Commun. (2006) 342:1077–82. doi: 10.1016/j.bbrc.2006.02.069
93. Deane EE, Woo NY. Upregulation of the somatotropic axis is correlated with increased G6PDH expression in black sea bream adapted to isoosmotic salinity. Ann NY Acad Sci. (2005) 1040:293–6. doi: 10.1196/annals.1327.045
94. Mancera JM, Fernandez-Llebrez P, Perez-Figares JM. Effect of decreased environmental salinity on growth hormone cells in the gilthead sea bream (Sparus aurata). J Fish Biol. (1995) 46:494–500. doi: 10.1111/j.1095-8649.1995.tb05990.x
95. Boeuf G, Payan P. How should salinity influence fish growth? Comp Biochem Physiol C Toxicol Pharmacol. (2001) 130:411–23. doi: 10.1016/S1532-0456(01)00268-X
96. Deane EE, Woo NY. Expression studies on glucose-6-phosphate dehydrogenase in sea bream: effects of growth hormone, somatostatin, salinity and temperature. J Exp Zool A Comp Exp Biol. (2005) 303:676–88. doi: 10.1002/jez.a.201
97. Martos-Sitcha JA, Mancera JM, Calduch-Giner JA, Yúfera M, Martínez-Rodríguez G, Pérez-Sánchez J. Unraveling the tissue-specific gene signatures of gilthead sea bream (Sparus aurata L.) after hyper-and hypo-osmotic challenges. PLoS ONE (2016) 11:e0148113. doi: 10.1371/journal.pone.0148113
98. Saravanan S, Schrama JW, Figueiredo-Silva AC, Kaushik SJ, Verreth JA, Geurden I. Constraints on energy intake in fish: the link between diet composition, energy metabolism, and energy intake in rainbow trout. PLoS ONE (2012) 7:e34743. doi: 10.1371/journal.pone.0034743
99. Rise ML, Hall JR, Nash GW, Xue X, Booman M, Katan T, et al. Transcriptome profiling reveals that feeding wild zooplankton to larval Atlantic cod (Gadus morhua) influences suites of genes involved in oxidation-reduction, mitosis, and selenium homeostasis. BMC Genomics (2015) 16:1016. doi: 10.1186/s12864-015-2120-1
100. Danzmann RG, Kocmarek AL, Norman JD, Rexroad CE, Palti Y. Transcriptome profiling in fast versus slow-growing rainbow trout across seasonal gradients. BMC Genomics (2016) 17:60. doi: 10.1186/s12864-016-2363-5
101. Blander G, Guarente L. The Sir2 family of protein deacetylases. Annu Rev Biochem. (2004) 73:417–35. doi: 10.1146/annurev.biochem.73.011303.073651
102. Simó-Mirabet P, Bermejo-Nogales A, Calduch-Giner J, Pérez-Sánchez J. Tissue-specific gene expression and fasting regulation of sirtuin family in gilthead sea bream (Sparus aurata). J Comp Physiol B Biochem Syst Environ Physiol. (2017) 187:153–63. doi: 10.1007/s00360-016-1014-0
103. Kelly G. A review of the sirtuin system, its clinical implications, and the potential role of dietary activators like resveratrol: part 1. Altern Med Rev. (2010) 15:245–63.
104. Chang HC, Guarente L. SIRT1 and other sirtuins in metabolism. Trends Endocrinol Metab. (2014) 25:138–45. doi: 10.1016/j.tem.2013.12.001
105. Zullo A, Simone E, Grimaldi M, Gagliardi M, Zullo L, Matarazzo MR, et al. Effect of nutrient deprivation on the expression and the epigenetic signature of sirtuin genes. Nutr Metab Cardiovasc Dis. (2018) 28:418–24. doi: 10.1016/j.numecd.2018.02.004
106. Yamamoto M, Iguchi G, Fukuoka H, Suda K, Bando H, Takahashi M, et al. SIRT1 regulates adaptive response of the growth hormone-insulin-like growth factor-I axis under fasting conditions in liver. Proc Natl Acad Sci USA. (2013) 110:14948–53. doi: 10.1073/pnas.1220606110
107. Satoh A, Brace CS, Ben-Josef G, West T, Wozniak DF, Holtzman DM, et al. SIRT1 promotes the central adaptive response to diet restriction through activation of the dorsomedial and lateral nuclei of the hypothalamus. J Neurosci. (2010) 30:10220–32. doi: 10.1523/JNEUROSCI.1385-10.2010
108. Cohen DE, Supinski AM, Bonkowski MS, Donmez G, Guarente LP. Neuronal SIRT1 regulates endocrine and behavioral responses to calorie restriction. Genes Dev. (2009) 23:2812–7. doi: 10.1101/gad.1839209
109. Chen YF, Wu CY, Kao CH, Tsai TF. Longevity and lifespan control in mammals: lessons from the mouse. Ageing Res Rev. (2010) 9:S28–35. doi: 10.1016/j.arr.2010.07.003
110. Monteserin-García J, Al-Massadi O, Seoane LM, Alvarez CV, Shan B, Stalla J, et al. Sirt1 inhibits the transcription factor CREB to regulate pituitary growth hormone synthesis. FASEB J. (2013) 27:1561–71. doi: 10.1096/fj.12-220129
111. Bermejo-Nogales A, Calduch-Giner JA, Pérez-Sánchez J. Unraveling the molecular signatures of oxidative phosphorylation to cope with the nutritionally changing metabolic capabilities of liver and muscle tissues in farmed fish. PLoS ONE (2015) 10:e0122889. doi: 10.1371/journal.pone.0122889
112. Simó-Mirabet P, Perera E, Calduch-Giner JA, Afonso JM, Pérez-Sánchez J. Co-expression analysis of sirtuins and related metabolic biomarkers in juveniles of gilthead sea bream (Sparus aurata) with differences in growth performance. Front Physiol. (2018) 9:608. doi: 10.3389/fphys.2018.00608
113. Calduch-Giner JA, Echasseriau Y, Crespo D, Baron D, Planas JV, Prunet P, et al. Transcriptional assessment by microarray analysis and large-scale meta-analysis of the metabolic capacity of cardiac and skeletal muscle tissues to cope with reduced nutrient availability in gilthead sea bream (Sparus aurata L.). Mar Biotechnol. (2014) 16:423–35. doi: 10.1007/s10126-014-9562-3
114. Dryden SC, Nahhas FA, Nowak JE, Goustin AS, Tainsky MA. Role for human SIRT2 NAD-dependent deacetylase activity in control of mitotic exit in the cell cycle. Mol Cell Biol. (2003) 23:3173–85. doi: 10.1128/MCB.23.9.3173-3185.2003
115. Wu G, Song C, Lu H, Jia L, Yang G, Shi X, et al. Sirt2 induces C2C12 myoblasts proliferation by activation of the ERK1/2 pathway. Acta Biochim Biophys Sin. (2014) 46:342–5. doi: 10.1093/abbs/gmt151
116. Stanton DA, Alway SE, Mohamed JS. The role of sirtuin 2 in the regulation of myogenesis. FASEB J. (2017) 31:877–913. doi: 10.1096/fasebj.31.1_supplement.877.13
117. Gui L, Hao R, Zhang Y, Zhao X, Zan L. Haplotype distribution in the class I sirtuin genes and their associations with ultrasound carcass traits in Qinchuan cattle (Bos taurus). Molec Cell Probes (2015) 29:102–7. doi: 10.1016/j.mcp.2015.03.007
118. Kuang J, Zhang Y, Liu Q, Shen J, Pu S, Cheng S, et al. Fat-specific Sirt6 ablation sensitizes mice to high-fat diet-induced obesity and insulin resistance by inhibiting lipolysis. Diabetes (2017) 66:1159–71. doi: 10.2337/db16-1225
119. Bosch-Presegue L, Vaquero A. Sirtuins in stress response: guardians of the genome. Oncogene (2014) 33:3764. doi: 10.1038/onc.2013.344
120. Brown-Borg HM, Bode AM, Bartke A. Antioxidative mechanisms and plasma growth hormone levels. Endocrine (1999) 11:41–8. doi: 10.1385/ENDO:11:1:41
121. Brown-Borg HM, Rakoczy SG. Catalase expression in delayed and premature aging mouse models. Exp Gerontol. (2000) 35:199–212. doi: 10.1016/S0531-5565(00)00079-6
122. McKenzie DJ, Martinez R, Morales A, Acosta J, Morales R, Taylor EW, et al. Effects of growth hormone transgenesis on metabolic rate, exercise performance and hypoxia tolerance in tilapia hybrids. J Fish Biol. (2003) 63:398–409. doi: 10.1046/j.1095-8649.2003.00162.x
123. Almeida DV, Bianchini A, Marins LF. Growth hormone overexpression generates an unfavorable phenotype in juvenile transgenic zebrafish under hypoxic conditions. Gen Comp Endocrinol. (2013) 194:102–9. doi: 10.1016/j.ygcen.2013.08.017
124. VanHelder WP, Casey K, Radomski MW. Regulation of growth hormone during exercise by oxygen demand and availability. Eur J Appl Physiol Occup Physiol. (1987) 56:628–32. doi: 10.1007/BF00424801
125. Barrett BA, McKeown BA. Sustained exercise augments long-term starvation increases in plasma growth hormone in the steelhead trout, Salmo gairdneri. Can J Zool. (1988) 66:853–5. doi: 10.1139/z88-126
126. Nielsen ME, Boesgaard L, Sweeting RM, McKeown BA, Rosenkilde P. Plasma levels of lactate, potassium, glucose, cortisol, growth hormone and triiodo-L-thyronine in rainbow trout (Oncorhynchus mykiss) during exercise at various levels for 24 h. Can J Zool. (1994) 72:1643–7. doi: 10.1139/z94-219
127. Martos-Sitcha JA, Simó-Mirabet P, Piazzon MC, de las Heras V, Calduch-Giner JA, Puyalto M, et al. Dietary sodium heptanoate helps to improve feed efficiency, growth hormone status and swimming performance in gilthead sea bream (Sparus aurata). Aquac Nutr. (2018) 2018:1–14. doi: 10.1111/anu.12799
128. Rotllant J, Balm PHM, Ruane NM, Pérez-Sánchez J, Wendelaar-Bonga SE, Tort L. Pituitary proopiomelanocortin-derived peptides and hypothalamus-pituitary-interrenal axis activity in gilthead sea bream (Sparus aurata) during prolonged crowding stress: differential regulation of adrenocorticotropin hormone and α-melanocyte-stimulating hormone release by corticotropin-releasing hormone and thyrotropin-releasing hormone. Gen Comp Endocrinol. (2000) 119:152–63. doi: 10.1006/gcen.2000.7508
129. Rotllant J, Balm PHM, Pérez-Sánchez J, Wendelaar-Bonga SE, Tort L. Pituitary and interrenal function in gilthead sea bream (Sparus aurata L., Teleostei) after handling and confinement stress. Gen Comp Endocrinol. (2001) 121:333–42. doi: 10.1006/gcen.2001.7604
130. Saera-Vila A, Calduch-Giner JA, Prunet P, Pérez-Sánchez J. Dynamics of liver GH/IGF axis and selected stress markers in juvenile gilthead sea bream (Sparus aurata) exposed to acute confinement: differential stress response of growth hormone receptors. Comp Biochem Physiol A Mol Integr Physiol. (2009) 154:197–203. doi: 10.1016/j.cbpa.2009.06.004
131. Pickering AD, Pottinger TG, Sumpter JP, Carragher JF, Le Bail PY. Effects of acute and chronic stress on the levels of circulating growth hormone in the rainbow trout, Oncorhynchus mykiss. Gen Comp Endocrinol. (1991) 83:86–93. doi: 10.1016/0016-6480(91)90108-I
132. Wilkinson RJ, Porter M, Woolcott H, Longland R, Carragher JF. Effects of aquaculture related stressors and nutritional restriction on circulating growth factors (GH, IGF-I and IGF-II) in Atlantic salmon and rainbow trout. Comp Biochem Physiol A Mol Integr Physiol. (2006) 145:214–24. doi: 10.1016/j.cbpa.2006.06.010
133. Auperin B, Baroiller JF, Ricordel MJ, Fostier A, Prunet P. Effect of confinement stress on circulating levels of growth hormone and two prolactins in freshwater-adapted tilapia (Oreochromis niloticus). Gen Comp Endocrinol. (1997) 108:35–44. doi: 10.1006/gcen.1997.6938
134. Martos-Sitcha JA, Bermejo-Nogales A, Calduch-Giner JA, Pérez-Sánchez J. Gene expression profiling of whole blood cells supports a more efficient mitochondrial respiration in hypoxia-challenged gilthead sea bream (Sparus aurata). Front Zool. (2017) 14:34. doi: 10.1186/s12983-017-0220-2
135. Calduch-Giner JA, Davey G, Saera-Vila A, Houeix B, Talbot A, Prunet P, et al. Use of microarray technology to assess the time course of liver stress response after confinement exposure in gilthead sea bream (Sparus aurata L.). BMC Genomics (2010) 11:193. doi: 10.1186/1471-2164-11-193
136. Bermejo-Nogales A, Nederlof M, Benedito-Palos L, Ballester-Lozano GF, Folkedal O, Olsen RE, et al. Metabolic and transcriptional responses of gilthead sea bream (Sparus aurata L.) to environmental stress: new insights in fish mitochondrial phenotyping. Gen Comp Endocrinol. (2014) 205:305–15. doi: 10.1016/j.ygcen.2014.04.016
137. Donohoe PH, Boutilier RG. The protective effects of metabolic rate depression in hypoxic cold submerged frogs. Respir Physiol. (1998) 111:325–36. doi: 10.1016/S0034-5687(97)00125-4
138. Gracey AY, Troll JV, Somero GN. Hypoxia-induced gene expression profiling in the euryoxic fish Gillichthys mirabilis. Proc Natl Acad Sci USA. (2001) 98:1993–8. doi: 10.1073/pnas.98.4.1993
139. Gamboa JL, Andrade FH. Muscle endurance and mitochondrial function after chronic normobaric hypoxia: contrast of respiratory and limb muscles. Pflügers Arch Eur J Physiol. (2012) 463:327–38. doi: 10.1007/s00424-011-1057-8
140. Magnoni LJ, Martos-Sitcha JA, Queiroz A, Calduch-Giner JA, Gonçalves JFM, Rocha CM, et al. Dietary supplementation of heat-treated Gracilaria and Ulva seaweeds enhanced acute hypoxia tolerance in gilthead Seabream (Sparus aurata). Biol Open (2017) 6:897–908. doi: 10.1242/bio.024299
141. Remen M, Nederlof MA, Folkedal O, Thorsheim G, Sitjà-Bobadilla A, Pérez-Sánchez J, et al. Effect of temperature on the metabolism, behaviour and oxygen requirements of Sparus aurata. Aquacult Environ Interact. (2015) 7:115–23. doi: 10.3354/aei00141
142. Remen M, Sievers M, Torgersen T, Oppedal F. The oxygen threshold for maximal feed intake of Atlantic salmon post-smolts is highly temperature-dependent. Aquaculture (2016) 464:582–92. doi: 10.1016/j.aquaculture.2016.07.037
143. Saravanan S, Geurden I, Figueiredo-Silva AC, Kaushik SJ, Haidar MN, Verreth JA, et al. Control of voluntary feed intake in fish: a role for dietary oxygen demand in Nile tilapia (Oreochromis niloticus) fed diets with different macronutrient profiles. Br J Nutr. (2012) 108:1519–29. doi: 10.1017/S0007114511006842
144. Tacon AG, Metian M. Feed matters: satisfying the feed demand of aquaculture. Rev Fish Sci Aquac. (2015) 23:1–10. doi: 10.1080/23308249.2014.987209
145. Kousoulaki K, Sæther BS, Albrektsen S, Noble C. Review on European sea bass (Dicentrarchus labrax, Linnaeus, 1758) nutrition and feed management: a practical guide for optimizing feed formulation and farming protocols. Aquacult Nutr. (2015) 21:129–51. doi: 10.1111/anu.12233
146. Benedito-Palos L, Ballester-Lozano GF, Simó P, Karalazos V, Ortiz Á, Calduch-Giner J, et al. Lasting effects of butyrate and low FM/FO diets on growth performance, blood haematology/biochemistry and molecular growth-related markers in gilthead sea bream (Sparus aurata). Aquaculture (2016) 454:8–18. doi: 10.1016/j.aquaculture.2015.12.008
147. Simó-Mirabet P, Felip A, Estensoro I, Martos-Sitcha JA, de las Heras V, Calduch-Giner J, et al. Impact of low fish meal and fish oil diets on the performance, sex steroid profile and male-female sex reversal of gilthead sea bream (Sparus aurata) over a three-year production cycle. Aquaculture (2018) 490:64–74. doi: 10.1016/j.aquaculture.2018.02.025
148. Regost C, Arzel J, Robin J, Rosenlund G, Kaushik SJ. Total replacement of fish oil by soybean or linseed oil with a return to fish oil in turbot (Psetta maxima): 1. Growth performance, flesh fatty acid profile, and lipid metabolism. Aquaculture (2003) 217:465–82. doi: 10.1016/S0044-8486(02)00259-4
149. Piedecausa MA, Mazón MJ, García BG, Hernández MD. Effects of total replacement of fish oil by vegetable oils in the diets of sharpsnout seabream (Diplodus puntazzo). Aquaculture (2007) 263:211–9. doi: 10.1016/j.aquaculture.2006.09.039
150. Bouraoui L, Sánchez-Gurmaches J, Cruz-García L, Gutiérrez J, Benedito-Palos L, Pérez-Sánchez J, et al. Effect of dietary fish meal and fish oil replacement on lipogenic and lipoprotein lipase activities and plasma insulin in gilthead sea bream (Sparus aurata). Aquacult Nutr. (2011) 17:54–63. doi: 10.1111/j.1365-2095.2009.00706.x
151. Hansen AC, Waagbø R, Hemre GI. New B vitamin recommendations in fish when fed plant-based diets. Aquacult Nutr. (2015) 21:507–27. doi: 10.1111/anu.12342
152. Ballester-Lozano GF, Benedito-Palos L, Estensoro I, Sitjà-Bobadilla A, Kaushik S, Pérez-Sánchez J. Comprehensive biometric, biochemical and histopathological assessment of nutrient deficiencies in gilthead sea bream fed semi-purified diets. Br J Nutr. (2015) 114:713–26. doi: 10.1017/S0007114515002354
153. Hamre K, Sissener NH, Lock EJ, Olsvik PA, Espe M, Torstensen BE, et al. Antioxidant nutrition in Atlantic salmon (Salmo salar) parr and post-smolt, fed diets with high inclusion of plant ingredients and graded levels of micronutrients and selected amino acids. Peer J. (2016) 4:e2688. doi: 10.7717/peerj.2688
154. Hemre GI, Lock EJ, Olsvik PA, Hamre K, Espe M, Torstensen BE, et al. Atlantic salmon (Salmo salar) require increased dietary levels of B-vitamins when fed diets with high inclusion of plant based ingredients. Peer J. (2016) 4:e2493. doi: 10.7717/peerj.2493
155. Prabhu AJP, Schrama JW, Kaushik SJ. Mineral requirements of fish: a systematic review. Rev Aquacult. (2016) 8:172–219. doi: 10.1111/raq.12090
156. Estensoro I, Ballester-Lozano G, Benedito-Palos L, Grammes F, Martos-Sitcha JA, Mydland LT, et al. Dietary butyrate helps to restore the intestinal status of a marine teleost (Sparus aurata) fed extreme diets low in fish meal and fish oil. PLoS ONE (2016) 11:e0166564. doi: 10.1371/journal.pone.0166564
157. Piazzon MC, Galindo-Villegas J, Pereiro P, Estensoro I, Calduch-Giner JA, Gómez-Casado E, et al. Differential modulation of IgT and IgM upon parasitic, bacterial, viral, and dietary challenges in a Perciform fish. Front Immunol. (2016) 7:637. doi: 10.3389/fimmu.2016.00637
158. Piazzon MC, Calduch-Giner JA, Fouz B, Estensoro I, Simó-Mirabet P, Puyalto M, et al. Under control: how a dietary additive can restore the gut microbiome and proteomic profile, and improve disease resilience in a marine teleostean fish fed vegetable diets. Microbiome (2017) 5:164. doi: 10.1186/s40168-017-0390-3
159. Gómez-Requeni P, Mingarro M, Calduch-Giner JA, Médale F, Martin SAM, Houlihan DF, et al. Protein growth performance, amino acid utilisation and somatotropic axis responsiveness to fish meal replacement by plant protein sources in gilthead sea bream (Sparus aurata). Aquaculture (2004) 232:493–510. doi: 10.1016/S0044-8486(03)00532-5
160. Benedito-Palos L, Saera-Vila A, Calduch-Giner JA, Kaushik S, Pérez-Sánchez J. Combined replacement of fish meal and oil in practical diets for fast growing juveniles of gilthead sea bream (Sparus aurata L.): networking of systemic and local components of GH/IGF axis. Aquaculture (2007) 267:199–212. doi: 10.1016/j.aquaculture.2007.01.011
161. Benedito-Palos L, Navarro JC, Kaushik S, Pérez-Sánchez J. Tissue-specific robustness of fatty acid signatures in cultured gilthead sea bream (Sparus aurata L.) fed practical diets with a combined high replacement of fish meal and fish oil. J Anim Sci. (2010) 88:1759–70. doi: 10.2527/jas.2009-2564
162. Benedito-Palos L, Ballester-Lozano G, Pérez-Sánchez J. Wide-gene expression analysis of lipid-relevant genes in nutritionally challenged gilthead sea bream (Sparus aurata). Gene (2014) 547:34–42. doi: 10.1016/j.gene.2014.05.073
163. Fazeli PK, Klibanski A. Anorexia nervosa and bone metabolism. Bone (2014) 66:39–45. doi: 10.1016/j.bone.2014.05.014
164. Gaylord TG, Rawles SD, Davis KB. Dietary tryptophan requirement of hybrid striped bass (Morone chrysops × M. saxatilis). Aquacult Nutr. (2005) 11:367–74. doi: 10.1111/j.1365-2095.2005.00360.x
165. Hevrøy EM, El-Mowafi A, Taylor RG, Olsvik PA, Norberg B, Espe M. Lysine intake affects gene expression of anabolic hormones in Atlantic salmon, Salmo salar. Gen Comp Endocrinol. (2007) 152:39–46. doi: 10.1016/j.ygcen.2007.02.015
166. Rolland M, Dalsgaard J, Holm J, Gómez-Requeni P, Skov PV. Dietary methionine level affects growth performance and hepatic gene expression of GH-IGF system and protein turnover regulators in rainbow trout (Oncorhynchus mykiss) fed plant protein-based diets. Comp Biochem Physiol B Biochem Mol Biol. (2015) 181:33–41. doi: 10.1016/j.cbpb.2014.11.009
167. Gaylord TG, Barrows FT, Teague AM, Johansen KA, Overturf KE, Shepherd B. Supplementation of taurine and methionine to all-plant protein diets for rainbow trout (Oncorhynchus mykiss). Aquaculture (2007) 269:514–24. doi: 10.1016/j.aquaculture.2007.04.011
168. Simó-Mirabet P, Piazzon MC, Calduch-Giner JA, Ortiz Á, Puyalto M, Sitjà-Bobadilla A, et al. Sodium salt medium-chain fatty acids and Bacillus-based probiotic strategies to improve growth and intestinal health of gilthead sea bream (Sparus aurata). Peer J. (2017) 5:e4001. doi: 10.7717/peerj.4001
169. Lee LT, Nong G, Chan YH, Dicky LY, Cheng CH. Molecular cloning of a teleost growth hormone receptor and its functional interaction with human growth hormone. Gene (2001) 270:121–9. doi: 10.1016/S0378-1119(01)00488-7
170. Calduch-Giner JA, Duval H, Chesnel F, Boeuf G, Pérez-Sánchez J, Boujard D. Fish growth hormone receptor: molecular characterization of two membrane-anchored forms. Endocrinology (2001) 142:3269–73. doi: 10.1210/endo.142.7.8407
171. Tse DLY, Tse MCL, Chan CB, Deng L, Zhang WM, Lin HR, et al. Seabream growth hormone receptor: molecular cloning and functional studies of the full-length cDNA, and tissue expression of two alternatively spliced forms. Biochim Biophys Acta (2003) 1625:64–76. doi: 10.1016/S0167-4781(02)00591-2
172. Calduch-Giner JA, Mingarro M, de Celis SVR, Boujard D, Pérez-Sánchez J. Molecular cloning and characterization of gilthead sea bream (Sparus aurata) growth hormone receptor (GHR). Assessment of alternative splicing. Comp Biochem Physiol B Biochem Mol Biol. (2003) 136:1–13. doi: 10.1016/S1096-4959(03)00150-7
173. Saera-Vila A, Calduch-Giner JA, Pérez-Sánchez J. Duplication of growth hormone receptor (GHR) in fish genome: gene organization and transcriptional regulation of GHR type I and II in gilthead sea bream (Sparus aurata). Gen Comp Endocrinol. (2005) 142:193–203. doi: 10.1016/j.ygcen.2004.11.005
174. Lien S, Koop BF, Sandve SR, Miller JR, Kent MP, Nome T, et al. The Atlantic salmon genome provides insights into rediploidization. Nature (2016) 533:200. doi: 10.1038/nature17164
175. Schaefer MH, Yang JS, Serrano L, Kiel C. Protein conservation and variation suggest mechanisms of cell type-specific modulation of signaling pathways. PLoS Comput Biol. (2014) 10:e1003659. doi: 10.1371/journal.pcbi.1003659
176. Reindl KM, Kittilson JD, Bergan HE, Sheridan MA. Growth hormone-stimulated insulin-like growth factor-1 expression in rainbow trout (Oncorhynchus mykiss) hepatocytes is mediated by ERK, PI3K-AKT, and JAK-STAT. Am J Physiol Regul Integr Comp Physiol. (2011) 301:R236–43. doi: 10.1152/ajpregu.00414.2010
177. Bergan HE, Kittilson JD, Sheridan MA. Nutrition-regulated lipolysis in rainbow trout (Oncorhynchus mykiss) is associated with alterations in the ERK, PI3K-Akt, JAK-STAT, and PKC signaling pathways. Gen Comp Endocrinol. (2012) 176:367–76. doi: 10.1016/j.ygcen.2011.12.013
178. Bergan H, Kittilson JD, Sheridan M. PKC and ERK mediate growth hormone-stimulated lipolysis. J Mol Endocrinol. (2013) 51:213–24. doi: 10.1530/JME-13-0039
179. Bergan HE, Kittilson JD, Sheridan MA. Nutritional state modulates growth hormone-stimulated lipolysis. Gen Comp Endocrinol. (2015) 217:1–9. doi: 10.1016/j.ygcen.2015.04.017
180. Kelley KM, Prakash D, Roth JT, Haigwood JT, Arope SA, Flores RM, et al. Evolution of endocrine growth regulation: the insulin like growth factors (IGFs), their regulatory binding proteins (IGFBPs), and IGF receptors in fishes and other ectothermic vertebrates. In: Nagabhushanam R, editor. Recent Advances in Marine Biotechnology. Volume 4: Aquaculture. Part B: Fishes. Enfield, CT: Science Publishers, Inc. (2000). p. 189–228.
181. Planas JV, Méndez E, Baños N, Capilla E, Castillo J, Navarro I, et al. Fish insulin, IGF-I and IGF-II receptors: a phylogenetic approach. Integr Comp Biol. (2000) 40:223–33. doi: 10.1093/icb/40.2.223
182. Adashi EY, Resnick CE, Hernandez ER, Hurwitz A, Rosenfeld RG. Ovarian granulosa cell-derived insulin-like growth factor (IGF) binding proteins: release of low molecular weight, high-affinity IGF-selective species. Mol Cell Endocrinol. (1990) 74:175–84. doi: 10.1016/0303-7207(90)90222-T
183. Morrione A, Valentinis B, Xu SQ, Yumet G, Louvi A, Efstratiadis A, et al. Insulin-like growth factor II stimulates cell proliferation through the insulin receptor. Proc Natl Acad Sci USA. (1997) 94:3777–82. doi: 10.1073/pnas.94.8.3777
184. Gutiérrez J, Parrizas M, Carneiro N, Maestro JL, Maestro MA, Planas J. Insulin and IGF-I receptors and tyrosine kinase activity in carp ovaries: changes with reproductive cycle. Fish Physiol Biochem. (1993) 11:247–54. doi: 10.1007/BF00004572
185. Gutiérrez J, Parrizas M, Maestro MA, Navarro I, Plisetskaya EM. Insulin and IGF-I binding and tyrosine kinase activity in fish heart. J Endocrinol. (1995) 146:35–44. doi: 10.1677/joe.0.1460035
186. Hainaut P, Kowalski A, Giorgetti S, Baron V, Van Obberghen E. Insulin and insulin-like-growth-factor-I (IGF-I) receptors in Xenopus laevis oocytes. Comparison with insulin receptors from liver and muscle. Biochem J. (1991) 273:673–8. doi: 10.1042/bj2730673
187. Janicot M, Flores-Riveros JR, Lane MD. The insulin-like growth factor 1 (IGF-1) receptor is responsible for mediating the effects of insulin, IGF-1, and IGF-2 in Xenopus laevis oocytes. J Biol Chem. (1991) 266:9382–91.
188. Parrizas M, Maestro MA, Banos N, Navarro I, Planas J, Gutiérrez J. Insulin/IGF-I binding ratio in skeletal and cardiac muscles of vertebrates: a phylogenetic approach. Am J Physiol. (1995) 269:R1370–7. doi: 10.1152/ajpregu.1995.269.6.R1370
189. Armstrong DG, Hogg CO. The expression of a putative insulin-like growth factor-I receptor gene in the liver of the developing chick. J Mol Endocrinol. (1992) 8:193–201. doi: 10.1677/jme.0.0080193
190. Dardevet D, Sornet C, Attaix D, Baracos VE, Grizard J. Insulin-like growth factor-1 and insulin resistance in skeletal muscles of adult and old rats. Endocrinology (1994) 134:1475–84. doi: 10.1210/endo.134.3.8119189
191. Feidantsis K, Pörtner HO, Antonopoulou E, Michaelidis B. Synergistic effects of acute warming and low pH on cellular stress responses of the gilthead seabream Sparus aurata. J Comp Physiol. (2015) 185:185–205. doi: 10.1007/s00360-014-0875-3
192. Gilmour RS. The implications of insulin-like growth factor mRNA heterogeneity. J Endocrinol. (1994) 140:1–3. doi: 10.1677/joe.0.1400001
193. Wallis M. New insulin-like growth factor (IGF)-precursor sequences from mammalian genomes: the molecular evolution of IGFs and associated peptides in primates. Growth Horm IGF Res. (2009) 19:12–23. doi: 10.1016/j.ghir.2008.05.001
194. McKay BR, O'Reilly CE, Phillips SM, Tarnopolsky MA, Parise G. Co-expression of IGF-1 family members with myogenic regulatory factors following acute damaging muscle-lengthening contractions in humans. J Physiol. (2008) 586:5549–60. doi: 10.1113/jphysiol.2008.160176
195. Aperghis M, Velloso CP, Hameed M, Brothwood T, Bradley L, Bouloux PMG, et al. Serum IGF-I levels and IGF-I gene splicing in muscle of healthy young males receiving rhGH. Growth Horm IGF Res. (2009) 19:61–7. doi: 10.1016/j.ghir.2008.07.002
196. Shamblott MJ, Chen TT. Age-related and tissue-specific levels of five forms of insulin-like growth factor mRNA in a teleost. Mol Marine Biol Biotechnol. (1993) 2:351–61.
197. Duguay SJ, Swanson P, Dickhoff WW. Differential expression and hormonal regulation of alternatively spliced IGF-I mRNA transcripts in salmon. J Mol Endocrinol. (1994) 12:25–37. doi: 10.1677/jme.0.0120025
198. Tanaka M, Taniguchi T, Yamamoto I, Sakaguchi K, Yoshizato H, Ohkubo T, et al. Gene and cDNA structures of flounder insulin-like growth factor-I (IGF-I): multiple mRNA species encode a single short mature IGF-I. DNA Cell Biol. (1998) 17:859–68. doi: 10.1089/dna.1998.17.859
199. Tiago DM, Laize V, Cancela ML. Alternatively spliced transcripts of Sparus aurata insulin-like growth factor 1 are differentially expressed in adult tissues and during early development. Gen Comp Endocrinol. (2008) 157:107–15. doi: 10.1016/j.ygcen.2008.04.006
200. Pan Z, Zhang J, Zhang J, Zhou B, Chen J, Jiang Z, et al. Expression profiles of the insulin-like growth factor system components in liver tissue during embryonic and postnatal growth of erhualian and yorkshire reciprocal cross F1 pigs. Asian-Austr J Anim Sci. (2012) 25:903–12. doi: 10.5713/ajas.2011.11385
201. Shimizu M. Insulin-like growth factor-II. In: Takei Y, Ando H, Tsutsui K, editors. Handbook of Hormones Comparative Endocrinology for Basic and Clinical Research. Oxford: Academic Press (2016). p. 164–6.
202. Gerrard DE, Okamura CS, Ranalletta MA, Grant AL. Developmental expression and location of IGF-I and IGF-II mRNA and protein in skeletal muscle. J Anim Sci. (1998) 76:1004–11. doi: 10.2527/1998.7641004x
203. Moerth C, Schneider MR, Renner-Mueller I, Blutke A, Elmlinger MW, Erben RG, et al. Postnatally elevated levels of insulin-like growth factor (IGF)-II fail to rescue the dwarfism of IGF-I-deficient mice except kidney weight. Endocrinology (2007) 148:441–51. doi: 10.1210/en.2006-0385
204. Daughaday WH, Rotwein P. Insulin-like growth factors I and II. Peptide, messenger ribonucleic acid and gene structures, serum, and tissue concentrations. Endocr Rev. (1989) 10:68–91. doi: 10.1210/edrv-10-1-68
205. Vong QP, Chan KM, Cheng CH. Quantification of common carp (Cyprinus carpio) IGF-I and IGF-II mRNA by real-time PCR: differential regulation of expression by GH. J. Endocrinol. (2003) 178:513–21. doi: 10.1677/joe.0.1780513
206. Chauvigné F, Gabillard JC, Weil C, Rescan PY. Effect of refeeding on IGFI, IGFII, IGF receptors, FGF2, FGF6, and myostatin mRNA expression in rainbow trout myotomal muscle. Gen Comp Endocrinol. (2003) 132:209–15. doi: 10.1016/S0016-6480(03)00081-9
207. Caelers A, Berishvili G, Meli ML, Eppler E, Reinecke M. Establishment of a real-time RT-PCR for the determination of absolute amounts of IGF-I and IGF-II gene expression in liver and extrahepatic sites of the tilapia. Gen Comp Endocrinol. (2004) 137:196–204. doi: 10.1016/j.ygcen.2004.03.006
208. Peterson BC, Waldbieser GC, Bilodeau L. IGF-I and IGF-II mRNA expression in slow and fast growing families of USDA103 channel catfish (Ictalurus punctatus). Comp Biochem Physiol A Mol Integr Physiol. (2004) 139:317–23. doi: 10.1016/j.cbpb.2004.09.015
209. Duguay SJ, Lai-Zhang J, Steiner DF, Funkenstein B, Chan SJ. Developmental and tissue-regulated expression of IGF-I and IGF-II mRNAs in Sparus aurata. J Mol Endocrinol. (1996) 16:123–32. doi: 10.1677/jme.0.0160123
210. Allard JB, Duan C. IGF-binding proteins: why do they exist and why are there so many? Front Endocrinol. (2018) 9:117. doi: 10.3389/fendo.2018.00117
211. Ocampo Daza D, Sundström G, Bergqvist CA, Duan C, Larhammar D. Evolution of the insulin-like growth factor binding protein (IGFBP) family. Endocrinology (2011) 152:2278–89. doi: 10.1210/en.2011-0047
212. Sundström G, Larsson TA, Larhammar D. Phylogenetic and chromosomal analyses of multiple gene families syntenic with vertebrate Hox clusters. BMC Evol Biol. (2008) 8:254. doi: 10.1186/1471-2148-8-254
213. MacQueen DJ, Garcia de la Serrana D, Johnston IA. Evolution of ancient functions in the vertebrate insulin-like growth factor system uncovered by study of duplicated salmonid fish genomes. Mol Biol Evol. (2013) 30:1060–76. doi: 10.1093/molbev/mst017
214. Kamei H, Lu L, Jiao S, Li Y, Gyrup C, Laursen LS, et al. Duplication and diversification of the hypoxia-inducible IGFBP-1 gene in zebrafish. PLoS ONE (2008) 3:e3091. doi: 10.1371/journal.pone.0003091
215. Zhou J, Li W, Kamei H, Duan C. Duplication of the IGFBP-2 gene in teleost fish: protein structure and functionality conservation and gene expression divergence. PLoS ONE (2008) 3:e3926. doi: 10.1371/journal.pone.0003926
216. Dai W, Kamei H, Zhao Y, Ding J, Du Z, Duan C. Duplicated zebrafish insulin-like growth factor binding protein-5 genes with split functional domains: evidence for evolutionarily conserved IGF binding, nuclear localization, and transactivation activity. FASEB J. (2010) 24:2020–9. doi: 10.1096/fj.09-149435
217. Wang X, Lu L, Li Y, Li M, Chen C, Feng Q, et al. Molecular and functional characterization of two distinct IGF binding protein-6 genes in zebrafish. Am J Physiol Regul Integr Comp Physiol. (2009) 296:R1348–57. doi: 10.1152/ajpregu.90969.2008
218. Garcia de la Serrana D, Macqueen DJ. Insulin-like growth factor-binding proteins of teleost fishes. Front Endocrinol. (2018) 9:80. doi: 10.3389/fendo.2018.00080
219. Shimizu M, Dickhoff WW. Circulating insulin-like growth factor binding proteins in fish: their identities and physiological regulation. Gen Comp Endocrinol. (2017) 252:150–61. doi: 10.1016/j.ygcen.2017.08.002
220. Ning Y, Schuller AG, Bradshaw S, Rotwein P, Ludwig T, Frystyk J, et al. Diminished growth and enhanced glucose metabolism in triple knockout mice containing mutations of insulin-like growth factor binding protein-3,−4, and−5. Mol Endocrinol. (2006) 20:2173–86. doi: 10.1210/me.2005-0196
221. Kajimura S, Aida K, Duan C. Insulin-like growth factor-binding protein-1 (IGFBP-1) mediates hypoxia-induced embryonic growth and developmental retardation. Proc Natl Acad Sci USA. (2005) 102:1240–5. doi: 10.1073/pnas.0407443102
222. Hoeflich A, Wu M, Mohan S, Föll J, Wanke R, Froehlich T, et al. Overexpression of insulin-like growth factor-binding protein-2 in transgenic mice reduces postnatal body weight gain. Endocrinology (1999) 140:5488–96. doi: 10.1210/endo.140.12.7169
223. Duan C, Ding J, Li Q, Tsai W, Pozios K. Insulin-like growth factor binding protein 2 is a growth inhibitory protein conserved in zebrafish. Proc Natl Acad Sci USA. (1999) 96:15274–9. doi: 10.1073/pnas.96.26.15274
224. Chen JY, Chen JC, Huang WT, Liu CW, Hui CF, Chen TT. Molecular cloning and tissue-specific, developmental-stage-specific, and hormonal regulation of IGFBP3 gene in zebrafish. Mar Biotechnol. (2004) 6:1–7. doi: 10.1007/s10126-002-0115-9
225. Pedroso FL, Fukada H, Masumoto T. Molecular characterization, tissue distribution patterns and nutritional regulation of IGFBP-1,-2,-3 and−5 in yellowtail, Seriola quinqueradiata. Gen Comp Endocrinol. (2009) 161:344–53. doi: 10.1016/j.ygcen.2009.01.010
226. Safian D, Fuentes EN, Valdés JA, Molina A. Dynamic transcriptional regulation of autocrine/paracrine igfbp1, 2, 3, 4, 5, and 6 in the skeletal muscle of the fine flounder during different nutritional statuses. J Endocrinol. (2012) 214:95–108. doi: 10.1530/joe-12-0057
227. Alzaid A, Kim JH, Devlin RH, Martin SA, MacQueen DJ. Growth hormone transgenesis in coho salmon disrupts muscle immune function impacting cross-talk with growth systems. J Exp Biol. (2018) 221(Pt 13):jeb173146. doi: 10.1242/jeb.173146
228. Granada L, Lemos MFL, Cabral HN, Bossier P, Novais SC. Epigenetics in aquaculture—The last frontier. Rev Aquac. (2017) 10:994–1013. doi: 10.1111/raq.12219
229. Pérez-Sánchez J, Kaushik S, Corraze G, Izquierdo M, Torrecillas S, Sitjà-Bobadilla A, et al. Fish Nutrition Research: Recent Advances Perspectives. Madrid: CSIC (2018). Available online at: http://nutrigroup-iats.org/files/Training_course_on_fish_nutrition_research.pdf
230. Yan J, Charles JF. Gut microbiota and IGF-I. Calcified Tissue Int. (2018) 102:406–14. doi: 10.1007/s00223-018-0395-3
Keywords: growth hormone, insulin-like growth factors, insulin-like growth factor binding proteins, growth hormone receptors, insulin and IGF receptors, sirtuins, oxygen availability, energy status
Citation: Pérez-Sánchez J, Simó-Mirabet P, Naya-Català F, Martos-Sitcha JA, Perera E, Bermejo-Nogales A, Benedito-Palos L and Calduch-Giner JA (2018) Somatotropic Axis Regulation Unravels the Differential Effects of Nutritional and Environmental Factors in Growth Performance of Marine Farmed Fishes. Front. Endocrinol. 9:687. doi: 10.3389/fendo.2018.00687
Received: 08 August 2018; Accepted: 02 November 2018;
Published: 27 November 2018.
Edited by:
Oliana Carnevali, Università Politecnica delle Marche, ItalyReviewed by:
Peggy Biga, University of Alabama at Birmingham, United StatesLluis Tort, Autonomous University of Barcelona, Spain
Copyright © 2018 Pérez-Sánchez, Simó-Mirabet, Naya-Català, Martos-Sitcha, Perera, Bermejo-Nogales, Benedito-Palos and Calduch-Giner. This is an open-access article distributed under the terms of the Creative Commons Attribution License (CC BY). The use, distribution or reproduction in other forums is permitted, provided the original author(s) and the copyright owner(s) are credited and that the original publication in this journal is cited, in accordance with accepted academic practice. No use, distribution or reproduction is permitted which does not comply with these terms.
*Correspondence: Jaume Pérez-Sánchez, amFpbWUucGVyZXouc2FuY2hlekBjc2ljLmVz
†Present Address: Juan Antonio Martos-Sitcha, Department of Biology, Faculty of Marine and Environmental Sciences, Campus de Excelencia Internacional del Mar (CEI-MAR), University of Cádiz, Cádiz, Spain
Azucena Bermejo-Nogales, Endocrine Disruption and Toxicity of Contaminants, Department of Environment, INIA, Madrid, Spain