- 1Division of Endocrinology, New York Obesity Nutrition Research Center, Department of Medicine, Columbia University College of Physicians and Surgeons, New York, NY, United States
- 2Translational Research on Diabetes, UMR 1190, Inserm, Université Lille, Lille, France
- 3Endocrine and Metabolic Surgery, CHU Lille, Lille, France
Roux-en-Y gastric bypass results in large and sustained weight loss and resolution of type 2 diabetes in 60% of cases at 1–2 years. In addition to calorie restriction and weight loss, various gastro-intestinal mediated mechanisms, independent of weight loss, also contribute to glucose control. The anatomical re-arrangement of the small intestine after gastric bypass results in accelerated nutrient transit, enhances the release of post-prandial gut hormones incretins and of insulin, alters the metabolism and the entero-hepatic cycle of bile acids, modifies intestinal glucose uptake and metabolism, and alters the composition and function of the microbiome. The amelioration of beta cell function after gastric bypass in individuals with type 2 diabetes requires enteric stimulation. However, beta cell function in response to intravenous glucose stimulus remains severely impaired, even in individuals in full clinical diabetes remission. The permanent impairment of the beta cell may explain diabetes relapse years after surgery.
The prevalence of severe obesity, defines as body mass index (BMI) above 40 kg/m2, is increasing. It is affecting women more than men, and African American women (16.9%) more than Caucasian (9.3%), or Hispanic (8.9%) women (1). The number of bariatric surgeries performed yearly in the US has increased only minimally in the last few years and was estimated at 216,000 in 2016. Hence, only a small percentage of people meeting criteria for bariatric surgery, the most efficient and durable form of weight loss, actually benefit from it. Roux-en-Y gastric bypass (RYGB) was the dominant type of surgery performed in the US up to 2011. Vertical sleeve gastrectomy (VSG) is now the most performed surgery and represented 58% of all bariatric procedures in 2016 (2, 3). However, RYGB is the surgical model that has been studied the most to investigate gut mechanisms, independent of weight loss, that may contribute to post-operative glucose control. In addition, there are more long-term data on clinical remission of type 2 diabetes (T2D) after RYGB. Hence, this review will be more RYGB-centric.
The remarkable effect of bariatric surgery on T2D has generated considerable attention from the surgical, as well as the research community, in the last 12 years. Non-randomized observational studies have shown that bariatric surgery results not only in diabetes remission, but also decreases micro- and macro-vascular complications, cardiovascular disease risk and events, non-alcoholic steato-hepatitis (NASH) (4) and cancers (5–12). Cohort studies have shown increased longevity after bariatric surgery (10, 13). The effect of bariatric surgery on T2D remission is of particular interest. Both observational studies (14) and randomized controlled trials (RCTs) (15) show rates of remission varying from 15 to 100%, depending, in part, of the definition of diabetes remission (16, 17). Determinants of diabetes remission have been reviewed in meta-analysis (18) and the IDF-ADA Translational symposium (19). Pre-intervention β-cell function, use of insulin, known duration of T2D, HbA1C, age, surgery type, weight loss amount, genomics biomarkers, and the duration of follow-up after surgery remission, are all predictors of remission (20–25). The duration of follow up is certainly one of the key variables. In the Swedish Obesity Study (SOS), the rate of T2D remission decreases from 72%, at 2 years, to 36% at 10 years (5). Adams et al. show a decrease in the rate of remission from 75% at 2 years to 51% at 21 years in 84 patients with little attrition (90% follow up) (12). Arterburn et al. using electronic medical records, studied a large cohort of 4, 434 individuals with uncontrolled diabetes prior to surgery; of the 68.2% patients who initially remit their diabetes at 5 years, one third experience diabetes relapse 5–8 years after RYGB surgery (11). Overall, clinical parameters pre-intervention, surgery type, and post-surgery weight loss amount predict about 70% of remission rate. Predictive scores such as DiaRem (26) and ABCD (27) have been developed.
Pooling data from observational studies (14) and RTCs (15, 28–30), the rate of T2D remission is about 60% 2 years after RYGB. The mechanism by which RYGB results in this remarkable high rate of diabetes remission is not fully elucidated. The key question is whether diabetes remission is entirely weight loss dependent or not. If it is weight loss driven, then research should focus on the mechanisms, likely centrally mediated, by which patients eat less, lose about 30% of their total body weight and are able to keep the weight off, all goals unmatched with diet and exercise alone (31), or with pharmacotherapy (32). If some weight loss independent effects are at play in diabetes remission, they are likely gut-mediated. However, although RYGB results in many alterations of gut-mediated endocrine mechanisms, some of which play a role in post-prandial glucose control, their role in diabetes remission has not been fully demonstrated. The understanding of these mechanisms is crucial as it may help identify novel targets for the treatment of T2DM.
Calorie restriction with large (25–30%) and sustained (33–35) weight loss, are clearly important factors in the remission of diabetes after RYGB. They remove the chronic insult on the β-cell resulting from nutrient excess, i.e., glucose and lipid toxicity (36, 37), decrease inflammation (38–41), decrease fat mass and ectopic fat depots (42–44), and improve insulin sensitivity (29), all important modulators of metabolism. However, the benefit of the surgery on glucose control is apparent very rapidly, within days after RYGB surgery, prior to large amount of weight loss (45). In addition, the clinical observations that surgeries that alters the gastro intestinal track, such as RYGB, VSG, or biliopancreatic diversion (BPD), result in greater and more rapid diabetes remission than purely restrictive surgeries such as adjusted gastric banding (AGB), have prompted investigations of gastro-intestinal mediated mechanisms of glucose improvement. The regulation of blood glucose is complex and necessitates cross talk between the central nervous system, the endocrine pancreas, the liver, and the intestine (46). The intestine is the first line of contact with the environment, i.e., nutrient calorie load and composition, and plays a central role in post-prandial glucose control. The small intestine signals other organs via nutrient sensing, glucose transport, satiety and incretin hormones, bile acids metabolism, and the microbiome. Many of these intestinal pathways, reviewed below, contribute to glucose control, independent of weight loss, after RYGB (47).
The gut endocrine system regulates satiety and insulin secretion, both key factors in body weight and glucose control (48). Bariatric surgery alters the gut endocrine system in a favorable way to decrease appetite and improved glucose metabolism (49). After RYGB, ingested food empties rapidly from the small gastric pouch into the alimentary limb, and mix with the biliary and pancreatic exocrine secretion in the common limb (Figure 1). The rapid emptying of the reduced gastric pouch results in accelerated nutrient transit (50, 51) and alters the post-prandial gastro-intestinal hormonal chain of event. It enhances the release of satiety hormone such as cholecystokinin (CCK) (52, 53), peptide yy (PYY) (54), glucagon like peptide 1 (GLP-1) (55–57) and oxyntomodulin (58). A few clinical studies, using octreotide, demonstrated the role of gut peptides in increased satiety and decreased food reward after RYGB surgery (59, 60) (Figure 2). The release of the incretins GLP-1 (51, 61, 62), and of glucose dependent insulin peptide (GIP), in some (63–65) but not all (66) studies, is also enhanced by the accelerated transit; this improves the incretin effect on insulin secretion (55–57, 63, 67, 68) and lowers post-prandial glycemia (20). This exaggerated post-prandial release of GLP-1 occurs rapidly after the surgery (69), is independent of weight loss (51, 70) and can be entirely abolished by administration of the meal and/or glucose in the gastric remnant via a gastrostomy (67, 68). Although mean glucose levels improved after RYGB, the pattern of glucose levels during meals shows greater variability with earlier and higher glucose peaks, and lower post-prandial glycemia, at times in the hypoglycemic range, even if often asymptomatic. A small percentage of individuals experience debilitating neuroglycopenia after RYGB (71, 72), in relation to altered counter regulatory hormone response (73), increased insulin sensitivity (29) and decreased insulin clearance (74). The infusion of the GLP-1 receptor blocker exendin 9–39 prevents the large post-prandial insulin secretion and corrects the post-prandial neuroglycopenia; this illustrates the effect of endogenous GLP-1 on post-prandial glycemic control (75). The effect of exendin 9–39 on post-prandial glucose in individuals with normoglycemia, however, is more modest. Although exendin 9–39 can blunt post-prandial insulin secretion (76, 77), it results only in modest worsening of the glycemia (77–79).
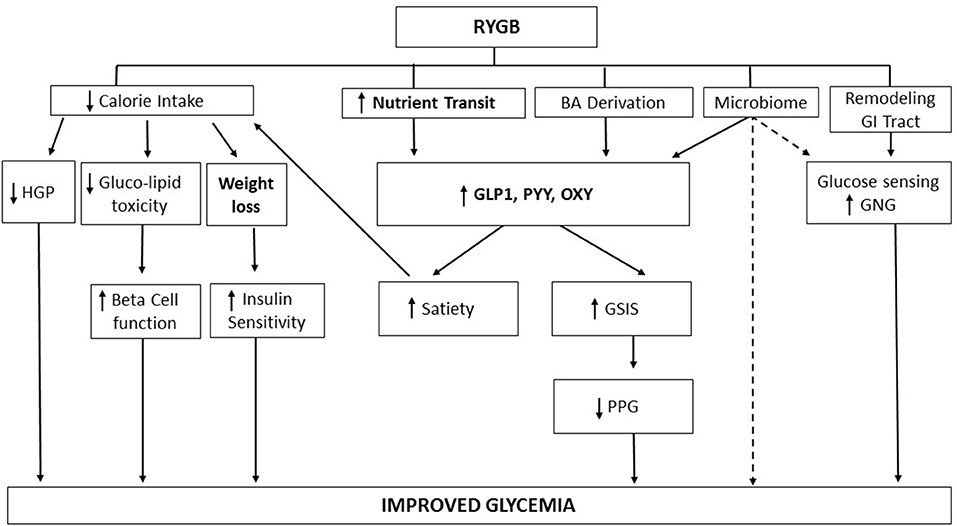
Figure 2. Mechanistic model of improved glycemia after RYGB. RYGB improves glucose metabolism via weight loss, and via weight-independent mechanisms, including stimulation of gut peptides, alteration of bile acids enterohepatic cycle, remodeling of the gastrointestinal track, and alteration of the microbiome. Solid lines: evidence based mechanisms; dashed lines: possible mechanisms. RYGB, Roux-en-Y gastric bypass surgery; HGP, hepatic glucose production; BA derivation, bile acids derivation; GI, gastro intestinal; GNG, gluconeogenesis; GLP-1, glucagon-like-peptide 1; PYY, peptide YY; OXY, oxyntomodulin; GSIS, glucose-stimulated insulin secretion; PPG, post-prandial glucose; ↑: increase; ↓: decrease.
The role of enhanced endogenous GLP-1 on the control of insulin secretion in response to oral glucose after RYGB is well demonstrated; its long-term implication on diabetes remission however remains elusive (79). Beta-cell function, assessed in response to intravenous glucose stimulus, improves only minimally and remains impaired in individuals in clinical diabetes remission and sustained weight loss, up to 3 years after RYGB (76). The reversal of post-prandial hyperinsulinemic hypoglycemia by the administration of food directly via gastrostomy in the remnant stomach, rather than per os, highlights the absence of permanent amelioration of the pancreatic endocrine function years after RYGB (80, 81). Therefore the increased meal-related insulin secretion after RYGB depends on enteric stimulation rather than on improved beta cells responsiveness to glucose (82) or to incretin stimuli (83). The persistent defect of beta cell function, overcome during meals, may explain, in part, the potential for diabetes relapse years after RYGB, in older patients who eat a less restrictive diet and regain some weight.
In addition to the intestinal endocrine function, other aspects of the gastro intestinal track play an important role in glucose control (84). The remodeling and reprogramming of the gastrointestinal track modifies intestinal glucose metabolism and glucose absorption and contributes to whole body metabolism after RYGB (85, 86). Interestingly this may not be the case after VSG (87) (Table 1). Troy et al. show increased intestinal gluconeogenesis after RYGB in mice, and effect abolished in GLUT-2 knockout mice (88). Others have shown an increased expression of genes involved in intestinal glucose transport and gluconeogenesis, in a rat bypass model (89–91), in association with decreased insulin resistance (92). Saeidi et al. demonstrated in rats that the active remodeling of the gastrointestinal tract increased intestinal cholesterol and glucose utilization, and contributed significantly to the improvement of whole body glucose metabolism after RYGB (93). Intestinal glucose transport is one of the key determinants of post-prandial glucose. The sodium-glucose transporter 1 (SGLT1) is responsible for the sodium-dependent, active uptake of glucose across the apical membrane of the small intestine (94). The expression of SGLT-1 increases after duodenal jenunal bypass (DJB) in rats (90) and after RYGB in humans (95). Baud et al. demonstrated, in a well characterized RYGB model in mini pigs (96), that the intestinal uptake of ingested glucose is blunted in the bile-deprived alimentary limb (Figure 1). Glucose absorption can be restored by the addition of either bile or sodium to the glucose meal, and is blocked with phlorizin. These studies provide direct evidence of a novel mechanism, via the reduction of active glucose-sodium transport, of decreased post-prandial glycemia after RYGB (96). More research is needed to understand the role of altered glucose transport, intestinal neoglucogenesis, and re-programming of the intestine on short and long-term glucose control after RYGB in humans, and its contribution to diabetes remission.
The change of bile acids metabolism after RYGB has been studied as potential mechanism of improved glucose control after bariatric surgery (97). Bile acids are synthesized by the liver, stored in the gallbladder and released in the duodenum in response to ingestion of nutrient. In non-operated individuals, after food intake, the chime, bile acids, and pancreatic exocrine secretions mix to enhance intestinal lipid digestion and absorption. After RYGB, in the absence of gastric fundus and pylorus, the ingested food empties rapidly from the gastric pouch; it then mix with bile acids and pancreatic secretions only in the common limb (Figure 1), precluding any duodenal absorption of nutrient (96). In addition to their role on lipid absorption, bile acids act as signaling molecules to regulate metabolism and inflammation (98). Bile acids are ligands of the nuclear receptor farnesoid X receptor (FXR) and the Takeda-G-protein- membrane receptor-5 TGR5 (99, 100), both receptors present in several organs that regulate metabolism. The role of the intestinal bile acids receptors as key regulators of glucose homeostasis was reviewed recently (101). The circulating bile acids concentrations (total molar sum) increase after RYGB in the fasted (102–106) and postprandial (77, 107–113) states in humans, as well as in rats and mini-pigs (114). The composition of the bile acids pool is also altered, and could contribute to the improvement of metabolism (106, 113). The rise of circulating bile acids after the surgery is delayed, occurs only a few months after the surgery and seems to be sustained overtime (106, 111, 113, 115). The underlying mechanisms of the elevated concentrations of circulating bile acids are unknown. Contrary to RYGB, calorie restriction and weight loss, either with (109) or without (112) AGB, decrease circulating bile acids concentrations (Table 1). Therefore, the rise in circulating bile acids after RYGB is not weight loss dependent. Experimental bile diversion, similarly to ileal transposition (116), are associated with increased circulating bile acids, increased postprandial GLP-1, weight loss and improved glucose tolerance (117–119). Possible explanations for the increased systemic pool of bile acids after RYGB are: increased hepatic synthesis and/or intestinal reabsorption, decreased fecal excretion and/or hepatic uptake or change in the microbiota. The increase in the peripheral but not in the portal circulation indicate that increase in bile acids systemic concentration after RYGB can be explained, in part, by a decrease of hepatic recapture, as shown after RYGB in mini pig (120). Whether the elevated systemic concentration of bile acids after RYGB in humans (113) is accompanied by increased concentration of luminal bile acids is unknown. One study in rats, show no change in luminal bile acids metabolism after RYGB and VSG (98, 121). Intestinal FXR is an important modulator of whole body metabolism. Pharmacological intestinal-specific activation of FXR reduces insulin resistance and stimulates adipose tissue browning, reduces lipids, inflammation, and atherosclerosis, while intestinal FXR inhibition favors non-alcoholic hepatic steatosis (NASH) (122). The effect of VSG on the improvement of glucose tolerance is reduced in FXR knock out (KO) mice (123). Bile acids, via activation of TGR5 signaling on the L cells, stimulate GLP-1 and participate in the control of glucose homeostasis (124–126). TGR5 seems to be required for the anti-hyperglycemic effect of VSG, as shown by two independent reports of VSG in TGR-5 KO mice (127, 128).
In all, results from clinical and animal studies suggest an important role of altered bile acids pool, composition, re-routing and signaling that may contribute to the metabolic effects of RYGB or VSG (Table 1). The elegant experiments of bile acids derivation and FXR and TGR5 KO propose a role for luminal bile acids in the improvement of metabolism after bariatric surgery. The clinical translation of these data, however, is still elusive. The temporal dissociation between the immediate rise of GLP-1 and the delayed increase in circulating bile acids, makes it less likely that the two processes are linked, at least in the early months after RYGB. Important information on intraluminal bile acids concentration after RYGB (or VSG) in humans is lacking. The composition, and therefore the function of the bile acids differs amongst species and add to the difficulty of translational research in this field. Finally, the large variability of the circulating concentrations of bile acids in humans studies (115) point out to other mechanisms, perhaps diet and/or microbiome dependent, that may modulate their composition and function.
Specific composition of the gut microbiome associates with pathological conditions such as cardiovascular disease, and with certain phenotypes like obesity and insulin resistance (129, 130). The link between gut microbiota composition and metabolic status is established through transplantation studies in humans and animals (131). However, the mechanism by which the gut microbiome maintains health or contributes to diseases is unknown. The change in microbiota composition, diversity and function is proposed as mechanism of some of the metabolic alterations after bariatric surgery (132–138). Transplantation of gut microbiota from RYGB mice (139) or patients (140) to germ-free mice reduces weight, fat mass, and induces metabolic improvements. Together, these studies indicate a possible link between gut flora modifications and metabolic changes after RYGB. Proposed mechanisms involve changes in glucose transport and sensing, GLP-1, short-chain fatty acids, lipogenesis, food intake, energy expenditure, adipose tissue metabolism, bile acids metabolism (141–146). One study however showed no link between alteration of the microbiome signature after RYGB and VSG and luminal metabolism of bile acids (121). The translational applicability of germ free mice experiments to humans is questionable. Human bariatric microbiota studies are often short term, lack controlled condition (diet, antibiotics and other drugs, metabolic status), favor description of composition rather than function of microbiome, and are based on feces, rather than luminal flora analysis. Future research will help identify whether the pre-surgery microbiome signature can be used to predict the metabolic response to the surgical intervention, and /or whether the change of microbiome composition and function can identify novel pathways of improved metabolism after various types of surgeries.
An important variable often overlooked in cross sectionals studies, is the change over time of many of the mechanisms described above. The accelerated nutrient transit time and stimulated GLP-1 release both occur immediately after RYGB and are sustained over time. However, the variance of the GLP-1 response increases between 1 month and 3 years post-surgery (147). We (113) and others (111) have demonstrated a temporal change of the pool of circulating bile acids after RYGB. Gut adaptation (hypertrophy, density of endocrine cells, glucose sensing, GNG) and the microbiome, are likely to undergo temporal transformation, in part, diet dependent. These data show the complexity of the gut physiology and adaptability, the difficulty of clinical studies, and the importance of longitudinal long-term studies for a better understanding of the contribution of the gut on post-prandial glycemia as well as diabetes remission.
In summary, RYGB results in T2DM remission as a result of large and sustained weight loss. RYGB also triggers weight independent gastro-intestinal mechanisms, including the stimulation of the incretins, the modulation of intestinal glucose transport and metabolism, the alteration of the entero-hepatic bile acids cycle, and change in the microbiome. These gut-related systems are inter-related as bile diversion impairs upper intestinal glucose uptake, nutrient malabsorption and bile acids can stimulate GLP-1, and the microbiome modulates many of these gastrointestinal targets. The mechanisms described above are likely to act in concert to contribute, with weight loss and calorie restriction, to glucose control after bariatric surgery (Figure 2). However, more clinical research needs to be done to understand the molecular mechanisms by which these different systems interact to improve glucose metabolism and to result in diabetes remission. The lack of normalization of beta cell function in response to IV glucose stimulus may be an important determinant of the future risk of diabetes relapse after RYGB surgery.
Author Contributions
All authors listed have made a substantial, direct and intellectual contribution to the work, and approved it for publication.
Conflict of Interest Statement
The authors declare that the research was conducted in the absence of any commercial or financial relationships that could be construed as a potential conflict of interest.
Acknowledgments
We thank Kapila Patel for her help with the formatting of references and Figures. BL and some of the work that she refers to in this review, were supported, in part, by grant by the National Institutes of Health (NIH) R01DK067561, P30DK26687-30, P30DK063608, and in part by the National Center for Advancing Translational Sciences, NIH, through Grant Number UL1TR001873. The content is solely the responsibility of the authors and does not necessarily represent the official views of the NIH. FP was supported by the Fédération Francophone de Recherche sur le Diabète (FFRD).
References
1. Flegal KM, Kruszon-Moran D, Carroll MD, Fryar CD, Ogden CL. Trends in obesity among adults in the united states, 2005 to 2014. JAMA (2016) 315:2284–91. doi: 10.1001/jama.2016.6458
2. Estimate of Bariatric Surgery Numbers 2011-2016. American Society for Metabolic and Bariatric Surgery (2016).
3. Abraham A, Ikramuddin S, Jahansouz C, Arafat F, Hevelone N, Leslie D. Trends in bariatric surgery: procedure selection, revisional surgeries, and readmissions. Obes Surg. (2016) 26:1371–7. doi: 10.1007/s11695-015-1974-2
4. Lassailly G, Caiazzo R, Buob D, Pigeyre M, Verkindt H, Labreuche J, et al. Bariatric surgery reduces features of nonalcoholic steatohepatitis in morbidly obese patients. Gastroenterology (2015) 149:379–88. doi: 10.1053/j.gastro.2015.04.014
5. Sjöström L, Lindroos AK, Peltonen M, Torgerson J, Bouchard C, Carlsson B, et al. Lifestyle, diabetes, and cardiovascular risk factors 10 years after bariatric surgery. N Eng J Med. (2004) 351:2683–93. doi: 10.1056/NEJMoa035622
6. Adams TD, Davidson LE, Litwin SE, Kolotkin RL, LaMonte MJ, Pendleton RC, et al. Health benefits of gastric bypass surgery after 6 years. JAMA (2012) 308:1122–31. doi: 10.1001/2012.jama.11164
7. Sjöström L, Narbro K, Sjöström CD, Karason K, Larsson B, Wedel H, et al. Effects of bariatric surgery on mortality in swedish obese subjects. N Eng J Med. (2007) 357:741–52. doi: 10.1056/NEJMoa066254
8. Sjöström L, Gummesson A, Sjöström CD, Narbro K, Peltonen M, Wedel H, et al. Effects of bariatric surgery on cancer incidence in obese patients in Sweden (Swedish Obese Subjects Study): a prospective, controlled intervention trial. Lancet Oncol. (2009) 10:653–62. doi: 10.1016/S1470-2045(09)70159-7
9. Sjöström L, Peltonen M, Jacobson P, Sjöström CD, Karason K, Wedel H, et al. Bariatric surgery and long-term cardiovascular events. JAMA (2012) 307:56–65. doi: 10.1001/jama.2011.1914
10. Adams TD, Gress RE, Smith SC, Halverson RC, Simper SC, Rosamond WD, et al. Long-term mortality after gastric bypass surgery. N Eng J Med. (2007) 357:753–61. doi: 10.1056/NEJMoa066603
11. Arterburn DE, Bogart A, Sherwood NE, Sidney S, Coleman KJ, Haneuse S, et al. A multisite study of long-term remission and relapse of type 2 diabetes mellitus following gastric bypass. Obes Surg. (2013) 23:93–102. doi: 10.1007/s11695-012-0802-1
12. Adams TD, Davidson LE, Litwin SE, Kim J, Kolotkin RL, Nanjee MN, et al. Weight and metabolic outcomes 12 years after gastric bypass. N Eng J Med. (2018) 378:93–6. doi: 10.1056/NEJMoa1700459
13. Sjostrom L, Peltonen M, Jacobson P, Ahlin S, Andersson-Assarsson J, Anveden A, et al. Association of bariatric surgery with long-term remission of type 2 diabetes and with microvascular and macrovascular complications. JAMA (2014) 311:2297–304. doi: 10.1001/jama.2014.5988
14. Singh AK, Singh R, Kota SK. Bariatric surgery and diabetes remission: Who would have thought it? Indian J Endocrinol Metab. (2015) 19:563–76. doi: 10.4103/2230-8210.163113
15. Schauer PR, Mingrone G, Ikramuddin S, Wolfe B. Clinical outcomes of metabolic surgery: efficacy of glycemic control, weight loss, and remission of diabetes. Diabetes Care (2016) 39:902–11. doi: 10.2337/dc16-0382
16. Pournaras DJ, Aasheim ET, Søvik TT, Andrews R, Mahon D, Welbourn R, et al. Effect of the definition of type II diabetes remission in the evaluation of bariatric surgery for metabolic disorders. Br J Surg. (2011) 99:100–3. doi: 10.1002/bjs.7704
17. Mas-Lorenzo A, Benaiges D, Flores-Le-Roux JA, Pedro-Botet J, Ramon JM, Parri A, et al. Impact of different criteria on type 2 diabetes remission rate after bariatric surgery. Obes Surg. (2014) 24:1881–7. doi: 10.1007/s11695-014-1282-2
18. Wang GF, Yan YX, Xu N, Yin D, Hui Y, Zhang J-P, et al. Predictive factors of type 2 diabetes mellitus remission following bariatric surgery: a meta-analysis. Obes Surg. (2015) 25:199–208. doi: 10.1007/s11695-014-1391-y
19. Panunzi S, Carlsson L, De Gaetano A, Peltonen M, Rice T, Sjöström L, et al. Determinants of diabetes remission and glycemic control after bariatric surgery. Diabetes Care (2016) 39:166–74. doi: 10.2337/dc15-0575
20. Shah A, Laferrère B. Diabetes after bariatric surgery. Can J Diabetes (2017) 41:401–6. doi: 10.1016/j.jcjd.2016.12.009
21. Vu L, Switzer NJ, De Gara C, Karmali S. Surgical interventions for obesity and metabolic disease. Best Pract Res Clin Endocrinol Metab. (2013) 27:239–46. doi: 10.1016/j.beem.2012.12.001
22. Pedersen HK, Gudmundsdottir V, Pedersen MK, Brorsson C, Brunak S, Gupta R. Ranking factors involved in diabetes remission after bariatric surgery using machine-learning integrating clinical and genomic biomarkers. NPJ Genom Med. (2016) 1:16035. doi: 10.1038/npjgenmed.2016.35
23. Nannipieri M, Baldi S, Mari A, Colligiani D, Guarino D, Camastra S, et al. Roux-en-Y gastric bypass and sleeve gastrectomy: mechanisms of diabetes remission and role of gut hormones. J Clin Endocrinol Metab. (2013) 98:4391–9. doi: 10.1210/jc.2013-2538
24. Blanco J, Jiménez A, Casamitjana R, Flores L, Lacy A, Conget I, et al. Relevance of beta-cell function for improved glycemic control after gastric bypass surgery. Surg Obes Relat Dis. (2014) 10:9–13. doi: 10.1016/j.soard.2013.07.020
25. Mathus-Vliegen EM. Long-term weight loss after bariatric surgery in patients visited at home outside the study environment. Obes Surg. (2006) 16:1508–19. doi: 10.1381/096089206778870003
26. Still CD, Wood GC, Benotti P, Petrick AT, Gabrielsen J, Strodel WE, et al. A probability score for preoperative prediction of type 2 diabetes remission following RYGB surgery. Lancet Diabetes Endocrinol. (2014) 2:38–45. doi: 10.1016/S2213-8587(13)70070-6
27. Lee WJ, Hur KY, Lakadawala M, Kasama K, Wong SK, Chen SC, et al. Predicting success of metabolic surgery: age, body mass index, C-peptide, and duration score. Surg Obes Relat Dis. (2013) 9:379–84. doi: 10.1016/j.soard.2012.07.015
28. Schauer PR, Bhatt DL, Kirwan JP, Wolski K, Aminian A, Brethauer SA, et al. Bariatric surgery versus intensive medical therapy for diabetes — 5-year outcomes. N Eng J Med. (2017) 376:641–51. doi: 10.1056/NEJMoa1600869
29. Mingrone G, Cummings DE. Changes of insulin sensitivity and secretion after bariatric/metabolic surgery. Surg Obes Relat Dis. (2016) 12:1199–205. doi: 10.1016/j.soard.2016.05.013
30. Mingrone G, Panunzi S, De Gaetano A, Guidone C, Iaconelli A, Nanni G, et al. Bariatric–metabolic surgery versus conventional medical treatment in obese patients with type 2 diabetes: 5 year follow-up of an open-label, single-centre, randomised controlled trial. Lancet (2015) 386:964–73. doi: 10.1016/S0140-6736(15)00075-6
31. Fothergill E, Guo J, Howard L, Kerns Jennifer C, Knuth Nicolas D, Brychta R, et al. Persistent metabolic adaptation 6 years after “The Biggest Loser” competition. Obesity (2016) 24:1612–9. doi: 10.1002/oby.21538
32. Kushner RF. Weight loss strategies for treatment of obesity: lifestyle management and pharmacotherapy. Prog Cardiovasc Dis. (2018). doi: 10.1016/j.pcad.2018.06.001. [Epub ahead of print].
33. Sjöström L. Review of the key results from the Swedish Obese Subjects (SOS) trial – a prospective controlled intervention study of bariatric surgery. J Intern Med. (2012) 273:219–34. doi: 10.1111/joim.12012
34. Courcoulas A, Christian N, Belle S, Berk P, Flum D, Garcia L. Longitudinal Assessment of Bariatric Surgery (LABS) consortium. Weight change and health outcomes at 3 years after bariatric surgery among individuals with severe obesity. JAMA (2013) 310:2416–25. doi: 10.1001/jama.2013.280928
35. Purnell JQ, Selzer F, Wahed AS, Pender J, Pories W, Pomp A, et al. Type 2 diabetes remission rates after laparoscopic gastric bypass and gastric banding: results of the longitudinal assessment of bariatric surgery study. Diabetes Care (2016) 39:1101–7. doi: 10.2337/dc15-2138
36. Kim JW, Yoon KH. Glucolipotoxicity in pancreatic β-Cells. Diabetes Metab J. (2011) 35:444–50. doi: 10.4093/dmj.2011.35.5.444
37. Poitout V, Robertson RP. Minireview: secondary β-cell failure in type 2 diabetes—a convergence of glucotoxicity and lipotoxicity. Endocrinology (2002) 143:339–42. doi: 10.1210/endo.143.2.8623
38. Shoelson SE, Herrero L, Naaz A. Obesity, inflammation, and insulin resistance. Gastroenterology (2007) 132:2169–80. doi: 10.1053/j.gastro.2007.03.059
39. Tilg H, Moschen AR. Inflammatory mechanisms in the regulation of insulin resistance. Mol Med. (2008) 14:222–31. doi: 10.2119/2007-00119
40. Kratz M, Hagman Derek K, Kuzma Jessica N, Foster-Schubert Karen E, Chan Chun P, Stewart S, et al. Improvements in glycemic control after gastric bypass occur despite persistent adipose tissue inflammation. Obesity (2016) 24:1438–45. doi: 10.1002/oby.21524
41. Hagman DK, Larson I, Kuzma JN, Cromer G, Makar K, Rubinow KB, et al. The short-term and long-term effects of bariatric/metabolic surgery on subcutaneous adipose tissue inflammation in humans. Metabolism (2017) 70:12–22. doi: 10.1016/j.metabol.2017.01.030
42. Luo RB, Suzuki T, Hooker JC, Covarrubias Y, Schlein A, Liu S, et al. How bariatric surgery affects liver volume and fat density in NAFLD patients. Surg Endosc. (2018) 32:1675–82. doi: 10.1007/s00464-017-5846-9
43. Immonen H, Hannukainen JC, Kudomi N, Pihlajamäki J, Saunavaara V, Laine J, et al. Increased liver fatty acid uptake is partly reversed and liver fat content normalized after bariatric surgery. Diabetes Care (2018) 41:368–71. doi: 10.2337/dc17-0738
44. Toro-Ramos T, Goodpaster Bret H, Janumala I, Lin S, Strain Gladys W, Thornton John C, et al. Continued loss in visceral and intermuscular adipose tissue in weight-stable women following bariatric surgery. Obesity (2014) 23:62–9. doi: 10.1002/oby.20932
45. Pories WJ, Swanson MS, MacDonald KG, Long SB, Morris PG, Brown BM, et al. Who would have thought it? An operation proves to be the most effective therapy for adult-onset diabetes mellitus. Ann Surg. (1995) 222:339–52
46. Schwartz MW, Seeley RJ, Tschöp MH, Woods SC, Morton GJ, Myers MG, et al. Cooperation between brain and islet in glucose homeostasis and diabetes. Nature (2013) 503:59–66. doi: 10.1038/nature12709
47. Jain A, Le Roux CW, Puri P, Tavakkoli A, Gletsu-Miller N, Laferrère B, et al. Proceedings of the 2017 ASPEN Research Workshop-Gastric Bypass: role of the Gut. JPEN J Parenter Enteral Nutr. (2018) 42:279–95. doi: 10.1002/jpen.1121
48. Drucker DJ. The role of gut hormones in glucose homeostasis. J Clin Invest. (2007) 117:24–32. doi: 10.1172/JCI30076
49. Quercia I, Dutia R, Kotler DP, Belsley S, Laferrère B. Gastrointestinal changes after bariatric surgery. Diabetes Metab. (2014) 40:87–94. doi: 10.1016/j.diabet.2013.11.003
50. Wang G, Agenor K, Pizot J, Kotler DP, Harel Y, Van Der Schueren BJ, et al. Accelerated gastric emptying but no carbohydrate malabsorption 1 year after gastric bypass surgery (GBP). Obes Surg. (2012) 22:1263–7. doi: 10.1007/s11695-012-0656-6
51. Stano S, Alam F, Wu L, Dutia R, Ng SN, Sala M, et al. Effect of meal size and texture on gastric pouch emptying and glucagon-like peptide 1 after gastric bypass surgery. Surg Obes Relat Dis. (2017) 13:1975–83. doi: 10.1016/j.soard.2017.09.004
52. Steinert RE, Feinle-Bisset C, Asarian L, Horowitz M, Beglinger C, Geary N. Ghrelin, CCK, GLP-1, and PYY(3–36): secretory controls and physiological roles in eating and glycemia in health, obesity, and after RYGB. Physiol Rev. (2016) 97:411–63. doi: 10.1152/physrev.00031.2014
53. Ockander L, Hedenbro JL, Rehfeld JF, Sjölund K. Jejunoileal bypass changes the duodenal cholecystokinin and somatostatin cell density. Obes Surg. (2003) 13:584–90. doi: 10.1381/096089203322190781
54. Olivan B, Teixeira J, Bose M, Bawa B, Chang T, Summe H, et al. Effect of weight loss by diet or gastric bypass surgery on peptide YY3-36 levels. Ann Surg. (2009) 249:948–53. doi: 10.1097/SLA.0b013e3181a6cdb0
55. Vidal J, Nicolau J, Romero F, Casamitjana R, Momblan D, Conget I, et al. Long-term effects of Roux-en-Y gastric bypass surgery on plasma glucagon-like peptide-1 and islet function in morbidly obese subjects. J Clin Endocrinol Metab. (2009) 94:884–91. doi: 10.1210/jc.2008-1620
56. le Roux CW, Welbourn R, Werling M, Osborne A, Kokkinos A, Laurenius A, et al. Gut hormones as mediators of appetite and weight loss after Roux-en-Y gastric bypass. Ann Surg. (2007) 246:780–5. doi: 10.1097/SLA.0b013e3180caa3e3
57. Morinigo R, Moize V, Musri M, Lacy AM, Navarro S, Marin JL, et al. Glucagon-like peptide-1, peptide YY, hunger, and satiety after gastric bypass surgery in morbidly obese subjects. J Clin Endocrinol Metab. (2006) 91:1735–40. doi: 10.1210/jc.2005-0904
58. Laferrère B, Swerdlow N, Bawa B, Arias S, Bose M, Olivan B, et al. Rise of oxyntomodulin in response to oral glucose after gastric bypass surgery in patients with type 2 diabetes. J Clin Endocrinol Metab. (2010) 95:4072–6. doi: 10.1210/jc.2009-2767
59. de Hollanda A, Casals G, Delgado S, Jiménez A, Viaplana J, Lacy AM, et al. Gastrointestinal hormones and weight loss maintenance following Roux-en-Y gastric bypass. J Clin Endocrinol Metab. (2015) 100:4677–84. doi: 10.1210/jc.2015-3065
60. Goldstone AP, Miras AD, Scholtz S, Jackson S, Neff KJ, Pénicaud L, et al. Link between increased satiety gut hormones and reduced food reward after gastric bypass surgery for obesity. J Clin Endocrinol Metab. (2016) 101:599–609. doi: 10.1210/jc.2015-2665
61. Laferrère B, Teixeira J, McGinty J, Tran H, Egger JR, Colarusso A, et al. Effect of weight loss by gastric bypass surgery versus hypocaloric diet on glucose and incretin levels in patients with type 2 diabetes. J Clin Endocrinol Metab. (2008) 93:2479–85. doi: 10.1210/jc.2007-2851
62. Morinigo R, Lacy AM, Casamitjana R, Delgado S, Gomis R, Vidal J. GLP-1 and changes in glucose tolerance following gastric bypass surgery in morbidly obese subjects. Obes Surg. (2006) 16:1594–601. doi: 10.1381/096089206779319338
63. Laferrère B, Heshka S, Wang K, Khan Y, McGinty J, Teixeira J, et al. Incretin levels and effect are markedly enhanced 1 month after Roux-en-Y gastric bypass surgery in obese patients with type 2 diabetes. Diabetes Care (2007) 30:1709–16. doi: 10.2337/dc06-1549
64. Purnell JQ, Johnson GS, Wahed AS, Dalla Man C, Piccinini F, Cobelli C, et al. Prospective evaluation of insulin and incretin dynamics in obese adults with and without diabetes for 2 years after Roux-en-Y gastric bypass. Diabetologia (2018) 61:1142–54. doi: 10.1007/s00125-018-4553-y
65. Lee CJ, Brown TT, Cheskin LJ, Choi P, Moran TH, Peterson L, et al. Effects of meal composition on postprandial incretin, glucose and insulin responses after surgical and medical weight loss. Obes Sci Pract. (2015) 1:104–9. doi: 10.1002/osp4.17
66. Xiong SW, Cao J, Liu XM, Deng XM, Liu Z, Zhang FT. Effect of modified Roux-en-Y gastric bypass surgery on GLP-1, GIP in patients with type 2 diabetes mellitus. Gastroenterol Res Pract. (2015) 2015:625196. doi: 10.1155/2015/625196
67. Lindqvist A, Spégel P, Ekelund M, Mulder H, Groop L, Hedenbro J, et al. Effects of ingestion routes on hormonal and metabolic profiles in gastric-bypassed humans. J Clin Endocrinol Metab. (2013) 98:E856–61. doi: 10.1210/jc.2012-3996
68. Pournaras DJ, Aasheim ET, Bueter M, Ahmed AR, Welbourn R, Olbers T, et al. Effect of bypassing the proximal gut on gut hormones involved with glycemic control and weight loss. Surg Obes Relat Dis. (2012) 8:371–4. doi: 10.1016/j.soard.2012.01.021
69. Falken Y, Hellstrom PM, Holst JJ, Naslund E. Changes in glucose homeostasis after Roux-en-Y gastric bypass surgery for obesity at day three, two months, and one year after surgery: role of gut peptides. J Clin Endocrinol Metab. (2011) 96:2227–35. doi: 10.1210/jc.2010-2876
70. Bose M, Teixeira J, Olivan B, Bawa B, Arias S, Machineni S, et al. Weight loss and incretin responsiveness improve glucose control independently after gastric bypass surgery. J Diabetes (2010) 2:47–55. doi: 10.1111/j.1753-0407.2009.00064.x
71. Raverdy V, Baud G, Pigeyre M, Verkindt H, Torres F, Preda C, et al. Incidence and predictive factors of postprandial hyperinsulinemic hypoglycemia after Roux-en-Y gastric bypass: a five year longitudinal study. Ann Surg. (2016) 264:878–85. doi: 10.1097/sla.0000000000001915
72. Patti ME, Goldfine AB. Hypoglycemia after gastric bypass: the dark side of GLP-1. Gastroenterology (2014) 146:605–8. doi: 10.1053/j.gastro.2014.01.038
73. Abrahamsson N, Börjesson JL, Sundbom M, Wiklund U, Karlsson FA, Eriksson JW. Gastric bypass reduces symptoms and hormonal responses in hypoglycemia. Diabetes (2016) 65:2667–75. doi: 10.2337/db16-0341
74. Salehi M, Gastaldelli A, D'Alessio DA. Altered islet function and insulin clearance cause hyperinsulinemia in gastric bypass patients with symptoms of postprandial hypoglycemia. J Clin Endocrinol Metab. (2014) 99:2008–17. doi: 10.1210/jc.2013-2686
75. Salehi M, Gastaldelli A, D'Alessio DA. Blockade of glucagon-like peptide 1 receptor corrects postprandial hypoglycemia after gastric bypass. Gastroenterology (2014) 146:669–80 e2. doi: 10.1053/j.gastro.2013.11.044
76. Dutia R, Brakoniecki K, Bunker P, Paultre F, Homel P, Carpentier AC, et al. Limited recovery of beta-cell function after gastric bypass despite clinical diabetes remission. Diabetes (2014) 63:1214–23. doi: 10.2337/db13-1176
77. Jorgensen NB, Dirksen C, Bojsen-Moller KN, Jacobsen SH, Worm D, Hansen DL, et al. Exaggerated glucagon-like peptide 1 response is important for improved beta-cell function and glucose tolerance after Roux-en-Y gastric bypass in patients with type 2 diabetes. Diabetes (2013) 62:3044–52. doi: 10.2337/db13-0022
78. Shah M, Law JH, Micheletto F, Sathananthan M, Dalla Man C, Cobelli C, et al. Contribution of endogenous glucagon-like peptide 1 to glucose metabolism after Roux-en-Y gastric bypass. Diabetes (2014) 63:483–93. doi: 10.2337/db13-0954
79. Vidal J, de Hollanda A, Jiménez A. GLP-1 is not the key mediator of the health benefits of metabolic surgery. Surg Obes Relat Dis. (2016) 12:1225–9. doi: 10.1016/j.soard.2016.02.029
80. McLaughlin T, Peck M, Holst J, Deacon C. Reversible hyperinsulinemic hypoglycemia after gastric bypass: a consequence of altered nutrient delivery. J Clin Endocrinol Metab. (2010) 95:1851–5. doi: 10.1210/jc.2009-1628
81. Davis DB, Khoraki J, Ziemelis M, Sirinvaravong S, Han JY, Campos GM. Roux en Y gastric bypass hypoglycemia resolves with gastric feeding or reversal: Confirming a non-pancreatic etiology. Mol Metab. (2018) 9:15–27. doi: 10.1016/j.molmet.2017.12.011
82. Salehi M, Gastaldelli A, D'Alessio, David A. Beta-cell sensitivity to glucose is impaired after gastric bypass surgery. Diabetes Obes Metab. (2017) 20:872–8. doi: 10.1111/dom.13165
83. Dirksen C, Bojsen-Moller KN, Jorgensen NB, Jacobsen SH, Kristiansen VB, Naver LS, et al. Exaggerated release and preserved insulinotropic action of glucagon-like peptide-1 underlie insulin hypersecretion in glucose-tolerant individuals after Roux-en-Y gastric bypass. Diabetologia (2013) 56:2679–87. doi: 10.1007/s00125-013-3055-1
84. Fujita Y, Kojima H, Hidaka H, Fujimiya M, Kashiwagi A, Kikkawa R. Increased intestinal glucose absorption and postprandial hyperglycaemia at the early step of glucose intolerance in Otsuka Long-Evans Tokushima Fatty Rats. Diabetologia (1998) 41:1459–66. doi: 10.1007/s001250051092
85. le Roux CW, Borg C, Wallis K, Vincent RP, Bueter M, Goodlad R, et al. Gut hypertrophy after gastric bypass is associated with increased glucagon-like peptide 2 and intestinal crypt cell proliferation. Ann Surg. (2010) 252:50–6. doi: 10.1097/SLA.0b013e3181d3d21f
86. Hansen CF, Bueter M, Theis N, Lutz T, Paulsen S, Dalbøge LS, et al. Hypertrophy dependent doubling of L-cells in Roux-en-Y gastric bypass operated rats. PLOS ONE (2013) 8:e65696. doi: 10.1371/journal.pone.0065696
87. Mumphrey MB, Hao Z, Townsend RL, Patterson LM, Berthoud H-R. Sleeve gastrectomy does not cause hypertrophy and reprogramming of intestinal glucose metabolism in rats. Obes Surg. (2015) 25:1468–73. doi: 10.1007/s11695-014-1547-9
88. Troy S, Soty M, Ribeiro L, Laval L, Migrenne S, Fioramonti X, et al. Intestinal gluconeogenesis is a key factor for early metabolic changes after gastric bypass but not after gastric lap-band in mice. Cell Metab. (2008) 8:201–11. doi: 10.1016/j.cmet.2008.08.008
89. Yan Y, Zhou Z, Kong F, Feng S, Li X, Sha Y, et al. Roux-en-Y gastric bypass surgery suppresses hepatic gluconeogenesis and increases intestinal gluconeogenesis in a T2DM rat model. Obes Surg. (2016) 26:2683–90. doi: 10.1007/s11695-016-2157-5
90. Kim M, Son YG, Kang YN, Ha TK, Ha E. Changes in glucose transporters, gluconeogenesis, and circadian clock after duodenal–jejunal bypass surgery. Obes Surg. (2015) 25:635–41. doi: 10.1007/s11695-014-1434-4
91. Sun D, Wang K, Yan Z, Zhang G, Liu S, Liu F, et al. Duodenal–jejunal bypass surgery up-regulates the expression of the hepatic insulin signaling proteins and the key regulatory enzymes of intestinal gluconeogenesis in diabetic goto–kakizaki rats. Obes Surg. (2013) 23:1734–42. doi: 10.1007/s11695-013-0985-0
92. Gutierrez-Repiso C, Garcia-Serrano S, Moreno-Ruiz FJ, Alcain-Martinez G, Rodriguez-Pacheco F, Garcia-Fuentes E. Jejunal gluconeogenesis associated with insulin resistance level and its evolution after Roux-en-Y gastric bypass. Surg Obes Relat Dis. (2017) 13:623–30. doi: 10.1016/j.soard.2016.11.021
93. Saeidi N, Meoli L, Nestoridi E, Gupta NK, Kvas S, Kucharczyk J, et al. Reprogramming of intestinal glucose metabolism and glycemic control in rats after gastric bypass. Science (2013) 341:406–10. doi: 10.1126/science.1235103
94. Wright EM, Hirayama BA, Loo DF. Active sugar transport in health and disease. J Intern Med (2007) 261:32–43. doi: 10.1111/j.1365-2796.2006.01746.x
95. Nguyen NQ, Debreceni TL, Bambrick JE, Chia B, Deane AM, Wittert G, et al. Upregulation of intestinal glucose transporters after Roux-en-Y gastric bypass to prevent carbohydrate malabsorption. Obesity (2014) 22:2164–71. doi: 10.1002/oby.20829
96. Baud G, Daoudi M, Hubert T, Raverdy V, Pigeyre M, Hervieux E, et al. Bile Diversion in Roux-en-Y gastric bypass modulates sodium-dependent glucose intestinal uptake. Cell Metab. (2016) 23:547–53. doi: 10.1016/j.cmet.2016.01.018
97. Albaugh VL, Banan B, Ajouz H, Abumrad NN, Flynn CR. Bile acids and bariatric surgery. Mol Aspects Med. (2017) 56:75–89. doi: 10.1016/j.mam.2017.04.001
98. Chávez-Talavera O, Tailleux A, Lefebvre P, Staels B. Bile acid control of metabolism and inflammation in obesity, type 2 diabetes, dyslipidemia, and nonalcoholic fatty liver disease. Gastroenterology (2017) 152:1679–94.e3. doi: 10.1053/j.gastro.2017.01.055
99. Pols TWH, Noriega LG, Nomura M, Auwerx J, Schoonjans K. The bile acid membrane receptor TGR5 as an emerging target in metabolism and inflammation. J Hepatol. (2011) 54:1263–72. doi: 10.1016/j.jhep.2010.12.004
100. Lefebvre P, Cariou B, Lien F, Kuipers F, Staels B. Role of bile acids and bile acid receptors in metabolic regulation. Physiol Rev. (2009) 89:147–91. doi: 10.1152/physrev.00010.2008
101. Trabelsi MS, Lestavel S, Staels B, Collet X. Intestinal bile acid receptors are key regulators of glucose homeostasis. Proc Nutr Soc. (2017) 76:192–202. doi: 10.1017/S0029665116002834
102. Nakatani H, Kasama K, Oshiro T, Watanabe M, Hirose H, Itoh H. Serum bile acid along with plasma incretins and serum high-molecular weight adiponectin levels are increased after bariatric surgery. Metabolism (2009) 58:1400–7. doi: 10.1016/j.metabol.2009.05.006
103. Pournaras DJ, Glicksman C, Vincent RP, Kuganolipava S, Alaghband-Zadeh J, Mahon D, et al. The role of bile after Roux-en-Y gastric bypass in promoting weight loss and improving glycaemic control. Endocrinology (2012) 153:3613–9. doi: 10.1210/en.2011-2145
104. Simonen M, Dali-Youcef N, Kaminska D, Venesmaa S, Kakela P, Paakkonen M, et al. Conjugated bile acids associate with altered rates of glucose and lipid oxidation after Roux-en-Y gastric bypass. Obes Surg. (2012) 22:1473–80. doi: 10.1007/s11695-012-0673-5
105. Gerhard GS, Styer AM, Wood GC, Roesch SL, Petrick AT, Gabrielsen J, et al. A role for fibroblast growth factor 19 and bile acids in diabetes remission after Roux-en-Y gastric bypass. Diabetes Care (2013) 36:1859–64. doi: 10.2337/dc12-2255
106. Ferrannini E, Camastra S, Astiarraga B, Nannipieri M, Castro-Perez J, Xie D, et al. Increased bile acid synthesis and deconjugation after biliopancreatic diversion. Diabetes (2015) 64:3377–85. doi: 10.2337/db15-0214
107. Patti ME, Houten SM, Bianco AC, Bernier R, Larsen PR, Holst JJ, et al. Serum bile acids are higher in humans with prior gastric bypass: potential contribution to improved glucose and lipid metabolism. Obesity (2009) 17:1671–7. doi: 10.1038/oby.2009.102
108. Werling M, Vincent RP, Cross GF, Marschall HU, Fandriks L, Lonroth H, et al. Enhanced fasting and post-prandial plasma bile acid responses after Roux-en-Y gastric bypass surgery. Scand J Gastroenterol. (2013) 48:1257–64. doi: 10.3109/00365521.2013.833647
109. Kohli R, Bradley D, Setchell KD, Eagon JC, Abumrad N, Klein S. Weight loss induced by Roux-en-Y gastric bypass but not laparoscopic adjustable gastric banding increases circulating bile acids. J Clin Endocrinol Metab. (2013) 98:E708–12. doi: 10.1210/jc.2012-3736
110. Ahmad NN, Pfalzer A, Kaplan LM. Roux-en-Y gastric bypass normalizes the blunted postprandial bile acid excursion associated with obesity. Int J Obes. (2013) 37:1553–9. doi: 10.1038/ijo.2013.38
111. Steinert RE, Peterli R, Keller S, Meyer-Gerspach AC, Drewe J, Peters T, et al. Bile acids and gut peptide secretion after bariatric surgery: a 1-year prospective randomized pilot trial. Obesity (2013) 21:E660–8. doi: 10.1002/oby.20522
112. Sachdev S, Wang Q, Billington C, Connett J, Ahmed L, Inabnet W, et al. FGF 19 and bile acids increase following Roux-en-Y gastric bypass but not after medical management in patients with type 2 diabetes. Obes Surg. (2016) 26:957–65. doi: 10.1007/s11695-015-1834-0
113. Dutia R, Embrey M, O'Brien S, Haeusler RA, Agenor KK, Homel P, et al. Temporal changes in bile acid levels and 12alpha-hydroxylation after Roux-en-Y gastric bypass surgery in type 2 diabetes. Int J Obes. (2016) 40:554. doi: 10.1038/ijo.2015.250
114. Spinelli V, Lalloyer F, Baud G, Osto E, Kouach M, Daoudi M, et al. Influence of Roux-en-Y gastric bypass on plasma bile acid profiles: a comparative study between rats, pigs and humans. Int J Obes (2016) 40:1260–7. doi: 10.1038/ijo.2016.46
115. Jørgensen NB, Dirksen C, Bojsen-Møller KN, Kristiansen VB, Wulff BS, Rainteau D, et al. Improvements in glucose metabolism early after gastric bypass surgery are not explained by increases in total bile acids and fibroblast growth factor 19 concentrations. J Clin Endocrinol Metab. (2015) 100:E396–406. doi: 10.1210/jc.2014-1658
116. Tsuchiya T, Kalogeris TJ, Tso P. Ileal transposition into the upper jejunum affects lipid and bile salt absorption in rats. Am J Physiol Gastrointest Liver Physiol. (1996) 271:G681–91. doi: 10.1152/ajpgi.1996.271.4.G681
117. Han H, Wang L, Du H, Jiang J, Hu C, Zhang G, et al. Expedited biliopancreatic juice flow to the distal gut benefits the diabetes control after duodenal-jejunal bypass. Obes Surg. (2015) 25:1802–9. doi: 10.1007/s11695-015-1633-7
118. Weng SG, Zhang B, Wang X, Chen H. Effects of duodenal-jejunal exclusion and new bilio-pancreatic diversion on blood glucose in rats with type 2 diabetes mellitus. Obes Surg. (2017) 27:2067–72. doi: 10.1007/s11695-017-2599-4
119. Kohli R, Setchell KD, Kirby M, Myronovych A, Ryan KK, Ibrahim SH, et al. A surgical model in male obese rats uncovers protective effects of bile acids post-bariatric surgery. Endocrinology (2013) 154:2341–51. doi: 10.1210/en.2012-2069
120. Chávez-Talavera O, Baud G, Spinelli V, Daoudi M, Kouach M, Goossens JF, et al. Roux-en-Y gastric bypass increases systemic but not portal bile acid concentrations by decreasing hepatic bile acid uptake in minipigs. Int J Obes. (2017) 41:664–8. doi: 10.1038/ijo.2017.7
121. Duboc H, Nguyen CC, Cavin J-B, Ribeiro-Parenti L, Jarry A-C, Rainteau D, et al. Roux-en-Y gastric-bypass and sleeve gastrectomy induces specific shifts of the gut microbiota without altering the metabolism of bile acids in the intestinal lumen. Int J Obes. (2018). doi: 10.1038/s41366-018-0015-3. [Epub ahead of print].
122. Mazuy C, Helleboid A, Staels B, Lefebvre P. Nuclear bile acid signaling through the farnesoid X receptor. Cell Mol Life Sci. (2015) 72:1631–50. doi: 10.1007/s00018-014-1805-y
123. Ryan KK, Tremaroli V, Clemmensen C, Kovatcheva-Datchary P, Myronovych A, Karns R, et al. FXR is a molecular target for the effects of vertical sleeve gastrectomy. Nature (2014) 509:183–8. doi: 10.1038/nature13135
124. Kuhre RE, Wewer Albrechtsen NJ, Larsen O, Jepsen SL, Balk-Møller E, Andersen DB, et al. Bile acids are important direct and indirect regulators of the secretion of appetite- and metabolism-regulating hormones from the gut and pancreas. Mol Metab. (2018) 11:84–95. doi: 10.1016/j.molmet.2018.03.007
125. Brighton CA, Rievaj J, Kuhre RE, Glass LL, Schoonjans K, Holst JJ, et al. Bile Acids Trigger GLP-1 release predominantly by accessing basolaterally located G protein–coupled bile acid receptors. Endocrinology (2015) 156:3961–70. doi: 10.1210/en.2015-1321
126. Katsuma S, Hirasawa A, Tsujimoto G. Bile acids promote glucagon-like peptide-1 secretion through TGR5 in a murine enteroendocrine cell line STC-1. Biochem Biophys Res Commun. (2005) 329:386–90. doi: 10.1016/j.bbrc.2005.01.139
127. Ding L, Sousa Kyle M, Jin L, Dong B, Kim BW, Ramirez R, et al. Vertical sleeve gastrectomy activates GPBAR-1/TGR5 to sustain weight loss, improve fatty liver, and remit insulin resistance in mice. Hepatology (2016) 64:760–73. doi: 10.1002/hep.28689
128. McGavigan AK, Garibay D, Henseler ZM, Chen J, Bettaieb A, Haj FG, et al. TGR5 contributes to glucoregulatory improvements after vertical sleeve gastrectomy in mice. Gut (2017) 66:226–34. doi: 10.1136/gutjnl-2015-309871
129. Clemente Jose C, Ursell Luke K, Parfrey Laura W, Knight R. The impact of the gut microbiota on human health: an integrative view. Cell (2012) 148:1258–70. doi: 10.1016/j.cell.2012.01.035
130. Cox LM, Blaser MJ. Pathways in microbe-induced obesity. Cell Metab. (2013) 17:883–94. doi: 10.1016/j.cmet.2013.05.004
131. Udayappan SD, Hartstra AV, Dallinga-Thie GM, Nieuwdorp M. Intestinal microbiota and faecal transplantation as treatment modality for insulin resistance and type 2 diabetes mellitus. Clin Exp Immunol. (2014) 177:24–9. doi: 10.1111/cei.12293
132. Zhang H, DiBaise JK, Zuccolo A, Kudrna D, Braidotti M, Yu Y, et al. Human gut microbiota in obesity and after gastric bypass. Proc Natl Acad Sci U S A. (2009) 106:2365–70. doi: 10.1073/pnas.0812600106
133. Furet JP, Kong LC, Tap J, Poitou C, Basdevant A, Bouillot JL, et al. Differential adaptation of human gut microbiota to bariatric surgery–induced weight loss: links with metabolic and low-grade inflammation markers. Diabetes (2010) 59:3049–57. doi: 10.2337/db10-0253
134. Kong LC, Tap J, Aron-Wisnewsky J, Pelloux V, Basdevant A, Bouillot JL, et al. Gut microbiota after gastric bypass in human obesity: increased richness and associations of bacterial genera with adipose tissue genes. Am J Clin Nutr. (2013) 98:16–24. doi: 10.3945/ajcn.113.058743
135. Graessler J, Qin Y, Zhong H, Zhang J, Licinio J, Wong ML, et al. Metagenomic sequencing of the human gut microbiome before and after bariatric surgery in obese patients with type 2 diabetes: correlation with inflammatory and metabolic parameters. Pharmacogenomics J. (2012) 13:514–22. doi: 10.1038/tpj.2012.43
136. Murphy R, Tsai P, Jüllig M, Liu A, Plank L, Booth M. Differential changes in gut microbiota after gastric bypass and sleeve gastrectomy bariatric surgery vary according to diabetes remission. Obes Surg. (2017) 27:917–25. doi: 10.1007/s11695-016-2399-2
137. Palleja A, Kashani A, Allin KH, Nielsen T, Zhang C, Li Y, et al. Roux-en-Y gastric bypass surgery of morbidly obese patients induces swift and persistent changes of the individual gut microbiota. Genome Med. (2016) 8:67. doi: 10.1186/s13073-016-0312-1
138. Anhê FF, Varin TV, Schertzer JD, Marette A. The gut microbiota as a mediator of metabolic benefits after bariatric surgery. Can J Diabetes (2017) 41:439–47. doi: 10.1016/j.jcjd.2017.02.002
139. Liou AP, Paziuk M, Luevano JM, Machineni S, Turnbaugh PJ, Kaplan LM. Conserved shifts in the gut microbiota due to gastric bypass reduce host weight and adiposity. Sci Transl Med. (2013) 5:178ra41. doi: 10.1126/scitranslmed.3005687
140. Tremaroli V, Karlsson F, Werling M, Ståhlman M, Kovatcheva-Datchary P, Olbers T, et al. Roux-en-Y gastric bypass and vertical banded gastroplasty induce long-term changes on the human gut microbiome contributing to fat mass regulation. Cell Metab. (2015) 22:228–38. doi: 10.1016/j.cmet.2015.07.009
141. Begley M, Gahan CG, Hill C. The interaction between bacteria and bile. FEMS Microbiol Rev. (2005) 29:625–51. doi: 10.1016/j.femsre.2004.09.003
142. Ridlon JM, Kang DJ, Hylemon PB. Bile salt biotransformations by human intestinal bacteria. J Lipid Res. (2006) 47:241–59. doi: 10.1194/jlr.R500013-JLR200
143. Sayin SI, Wahlstrom A, Felin J, Jantti S, Marschall HU, Bamberg K, et al. Gut microbiota regulates bile acid metabolism by reducing the levels of tauro-beta-muricholic acid, a naturally occurring FXR antagonist. Cell Metab. (2013) 17:225–35. doi: 10.1016/j.cmet.2013.01.003
144. Swann JR, Want EJ, Geier FM, Spagou K, Wilson ID, Sidaway JE, et al. Systemic gut microbial modulation of bile acid metabolism in host tissue compartments. Proc Natl Acad Sci. (2011) 108(Suppl. 1):4523–30. doi: 10.1073/pnas.1006734107
145. Duca FA, Lam TK. Gut microbiota, nutrient sensing and energy balance. Diabetes Obes Metab. (2014) 16:68–76. doi: 10.1111/dom.12340
146. Bauer PV, Duca FA, Waise TM, Rasmussen BA, Abraham MA, Dranse HJ, et al. Metformin alters upper small intestinal microbiota that impact a glucose-SGLT1-sensing glucoregulatory pathway. Cell Metab. (2018) 27:101–17.e5. doi: 10.1016/j.cmet.2017.09.019
Keywords: gastric bypass surgery, beta cell function, glucagon-like peptide 1 (GLP-1), bile acids, microbiome, sodium glucose transporter 1 (SGLt1), type 2 diabetes
Citation: Laferrère B and Pattou F (2018) Weight-Independent Mechanisms of Glucose Control After Roux-en-Y Gastric Bypass. Front. Endocrinol. 9:530. doi: 10.3389/fendo.2018.00530
Received: 21 June 2018; Accepted: 22 August 2018;
Published: 10 September 2018.
Edited by:
Derek LeRoith, Icahn School of Medicine at Mount Sinai, United StatesReviewed by:
Yukihiro Fujita, Asahikawa Medical University, JapanJennifer Kirby, University of Virginia, United States
Copyright © 2018 Laferrère and Pattou. This is an open-access article distributed under the terms of the Creative Commons Attribution License (CC BY). The use, distribution or reproduction in other forums is permitted, provided the original author(s) and the copyright owner(s) are credited and that the original publication in this journal is cited, in accordance with accepted academic practice. No use, distribution or reproduction is permitted which does not comply with these terms.
*Correspondence: Blandine Laferrère, bbl14@columbia.edu