- 1Vagal Afferent Research Group, Centre for Nutrition and Gastrointestinal Disease, Adelaide Medical School, University of Adelaide, Adelaide, SA, Australia
- 2Nutrition and Metabolism, South Australian Health and Medical Research Institute, Adelaide, SA, Australia
The ion channel TRPV1 is involved in a wide range of processes including nociception, thermosensation and, more recently discovered, energy homeostasis. Tightly controlling energy homeostasis is important to maintain a healthy body weight, or to aid in weight loss by expending more energy than energy intake. TRPV1 may be involved in energy homeostasis, both in the control of food intake and energy expenditure. In the periphery, it is possible that TRPV1 can impact on appetite through control of appetite hormone levels or via modulation of gastrointestinal vagal afferent signaling. Further, TRPV1 may increase energy expenditure via heat production. Dietary supplementation with TRPV1 agonists, such as capsaicin, has yielded conflicting results with some studies indicating a reduction in food intake and increase in energy expenditure, and other studies indicating the converse. Nonetheless, it is increasingly apparent that TRPV1 may be dysregulated in obesity and contributing to the development of this disease. The mechanisms behind this dysregulation are currently unknown but interactions with other systems, such as the endocannabinoid systems, could be altered and therefore play a role in this dysregulation. Further, TRPV1 channels appear to be involved in pancreatic insulin secretion. Therefore, given its plausible involvement in regulation of energy and glucose homeostasis and its dysregulation in obesity, TRPV1 may be a target for weight loss therapy and diabetes. However, further research is required too fully elucidate TRPV1s role in these processes. The review provides an overview of current knowledge in this field and potential areas for development.
Introduction
Obesity has become the fifth leading cause of death, and the second leading cause of preventable death worldwide, closely following tobacco smoking (1, 2). There are multiple hormonal, neurotransmitter, and receptor systems involved in the regulation of energy balance. Pharmacological attempts to favorably modulate these systems to encourage weight loss have been somewhat effective, although not without adverse side effects. This has led to the search for more suitable targets. One such group of receptors/ion channels gaining attention for their possible role in energy homeostasis are the Transient Receptor Potential (TRP) channels.
TRP channels are a superfamily of about 28 non-selective cation channels divided into 7 subfamilies including TRP vanilloid (TRPV), and TRP ankyrin (TRPA) (3). They were first identified in 1969 from an irregular electroretinogram in a mutant strain of the Drosophila fly (4). The electroretinogram presented a short increase in retinal potential which gave rise to the name “transient receptor potential” (5). Since their discovery, TRP channels have been identified as osmo- and mechano-sensitive (6). For example, TRPA1 is associated with pain sensations and inflammation (7), and TRPV1 is associated with pain and temperature regulation (8).
Endotherms use energy to create heat to maintain body temperature and in colder climates it has been shown that humans expend more energy for thermoregulation compared to warmer climates (9). Given the high energy costs of generating heat to maintain an optimal cellular environment thermoregulation can also play an important role in energy homeostasis. TRPV1 channels are involved in thermoregulation, making them a possible target for the modulation of energy expenditure. Further, it is becoming apparent that TRPV1 may be involved in the regulation of appetite via the modulation of appetite hormones and/or by acting on gastrointestinal vagal afferents. This is a process that may involve interaction with the endocannabinoid system considering that endocannabinoids such as anandamide (AEA), produced in the gastrointestinal tract are also endogenous TRPV1 agonists. In addition, there are suggestions that TRPV1 may be involved in the regulation of insulin secretion in the pancreas. Studies in obese individuals have suggested that TRPV1 may be dysfunctional or dysregulated due to loss of effect on energy homeostasis. For this reason TRPV1 may be a potential target for pharmacological manipulation to aid in weight loss with recent studies suggesting selective blockade or activation of specific functions of TRPV1. However, due to its complexity this may prove difficult. This review explores TRPV1 structure and modulation and will focus on its involvement in energy homeostasis, diabetes, and possible pharmacological manipulation.
TRPV1 Channels
TPRV1, the first channel in the vanilloid family, is highly permeable to calcium and was discovered in 1997 by cloning dorsal root ganglia expressed genes in human embryonic kidney cells (10). It is expressed in a wide range of central and peripheral tissues. Centrally, TRPV1 is highly expressed in the brain stem, mid-brain, hypothalamus and limbic system (11). Peripherally it is expressed in many tissues including the vagal and spinal sensory nerves (12), stomach (13), and adipose tissue (14).
TRPV1 Structure
The TRPV1 channel consists of four identical subunits located in the plasma membrane with each subunit (Figure 1) consisting of an N-terminus, a transmembrane region, and a C-terminus (15, 16). The N-terminus contains an ankyrin repeating domain consisting of 6 ankyrin subunits (16) which in its tertiary structure forms six α-helices connected by finger loops (15). Sites on the N-terminus are capable of phosphorylation by protein kinases with the S116 phosphorylation site being one of functionality (17). A linker section connects the N-terminus to the transmembrane region via the pre-helical segment (pre-S1), and connects TPRV1 subunits together (15–18).
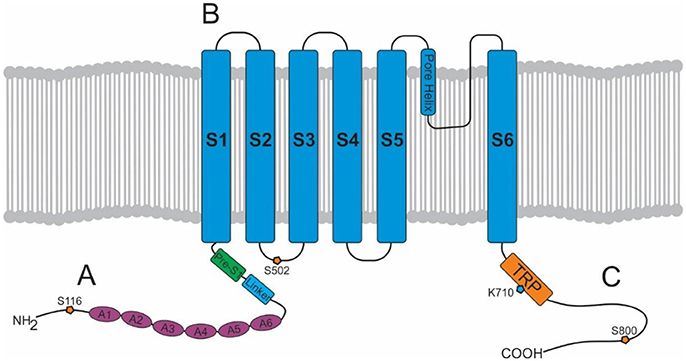
Figure 1. Structure of a TRPV1 subunit. (A) N-terminus containing 6 ankyrin subunits (A1–A6) and a linking region consisting of a linker and a Pre-S1 helix segment. (B) Transmembrane region with 6 helical segments (S1–S6). (C) C-terminus containing a TRP domain and binding sites for PKA, PKC, PIP2, and calmodulin.
The transmembrane region of each TRPV1 subunit comprises 6 helical segments (S1–S6), where S1–S4 contribute to the voltage-sensing domain, and S5–S6 contribute to the pore-forming domain (16). S1-S4 are connected to S5-S6 by a linker segment, and act as a foundation which allows the linker segment to move, contributing to pore opening and TRPV1 activation. The transmembrane region also contains binding sites for several ligands. For example, vanilloids (e.g., capsaicin) are capable of binding to S3 and S4, and protons (H+) are capable of binding to S5 and the S5–S6 linker (pore helix) (15).
Lastly, the C-terminus consists of a TRP domain (TRP-D) which interacts with pre-S1 suggesting a structural role (16). Following the TRP domain are several protein kinase A (PKA) and protein kinase C (PKC) phosphorylation sites, and sites for binding calmodulin and phosphatidylinositol-4,5-bisphosphate (PIP2) (15, 16).
TRPV1 Channel Activation or Modulation
TRPV1 is activated by a wide variety of different stimuli including heat, protons (pH < 5.9) (8, 19), capsaicin the irritant compound in hot chilies (10), allicin and diallyl sulfides from garlic (20, 21), peperine from black pepper (22), and gingerol from ginger (23). Spider and jellyfish venom-derived toxins are also TRPV1 agonists (24, 25).
Endogenous agonists are referred to as endovanilloids. To qualify as an endovanilloid the compound should be produced and released in sufficient amount to evoke a TRPV1-mediated response by direct binding and subsequent activation of the channel. Further, to permit regulation of the channel the signal should have a short half-life. Therefore, the mechanisms for synthesis and breakdown of the endovanilloid should be in close proximity to TRPV1. As the binding sites for endogenous ligands of TRPV1 are intracellular (26, 27) then the ligand could also be produced within the cell or there should be a mechanism to bring it into the cell. Three different classes of lipid are known to activate TRPV1 i.e., N-acyl-ethanolamines [NAEs, e.g., AEA (28)], some lipoxygenase products of arachidonic acid and N-acyl-dopamines (e.g., N-arachidonoyldopamine, N-oleoyldopamine) (29). Further, adipose tissue B lymphocytes (B1 cells) that regulate local inflammatory responses produce leukotrienes including leukotriene B4 which is also a TRPV1 agonist (30).
Intracellularly, calmodulin, a calcium-binding messenger, mediates the negative feedback loop formed by calcium (31). Calcium binds and activates calmodulin allowing it to bind to the N-terminus or C-terminus of TRPV1 inhibiting TRPV1 activity (15). Other secondary messengers such as PKA, PKC, and PIP2 are also capable of modulating TRPV1 activity. PKA can enhance or activate TRPV1 through phosphorylation of sites (S116 and T370) on the N-terminal (32) and may play a role in the capsaicin induced Ca2+ dependent desensitization of TRPV1 activation, a phenomenon which has been extensively reviewed elsewhere (33). PKC directly activates TRPV1 through phosphorylation of the S2–S3 linker region (S502) and C-terminal sites (S800), and also potentiates the effect of other ligands such as protons (34, 35). PIP2 is a negative regulator, inhibiting TRPV1 activity when bound to the C-terminal sites (TRP domain: K710) (36).
Interactions Between TRPV1 and the Endocannabinoid System
The Endocannabinoid System
The endocannabinoid system consists of endocannabinoids, their receptors and the enzymes involved in endocannabinoid synthesis and degradation. This system is involved in many physiological processes including memory, mood, and relevant to this review promotion of food intake (37). Endocannabinoids are endogenous lipid messengers (e.g., AEA and 2-arachydonoyl-glycerol) which activate their receptors, cannabinoid receptor-1 (CB1) and cannabinoid receptor-2 (CB2) (38, 39).
These endogenous lipid messengers are synthesized on demand and degraded by cellular uptake and enzymatic hydrolysis [see review (40) and Figure 2]. Briefly, the first step in the synthesis of AEA and NAEs is the transacylation of membrane phosphatidyethanolamine-containing phospholipids to N-acylphosphatidyl-ethanolamines (NAPEs) (41, 42). There are a number of ways that NAPEs are metabolized to their corresponding NAE including catalyzed hydrolysis by the NAPE-hydrolysing enzyme phospholipase D (NAPE-PLD) (43). In contrast, diacylglycerol lipase (DAGL) is responsible for the formation of 2-AG (44). There is still some controversy on whether there is an endocannabinoid membrane transporter [See reviews (45, 46)]. Nonetheless, endocannabinoids can be cleared from the extracellular space. Further, there are intracellular proteins that can shuttle these lipids to specific intracellular locations (e.g., TRPV1 for AEA) (47, 48), the best characterized of these are the fatty acid-binding proteins (FABP e.g., FABP5 and 7) (49). The enzymes responsible for the breakdown of AEA and NAEs are fatty acid amide hydrolase (FAAH) and N-acylethanolamine-hydrolysing acid-amidase (NAAA). NAAA is predominantly located in the lungs where it is localized to the lysosomes of macrophages (50, 51). FAAH is more ubiquitous and FAAH-1 is located on the endoplasmic reticulum whereas FAAH-2 (not found in rodents) is located in the lipid rafts (52, 53). Monoacylglycerol lipase (MAGL) is the enzyme responsible for the majority of 2-AG hydrolysis in most tissues (54–56).
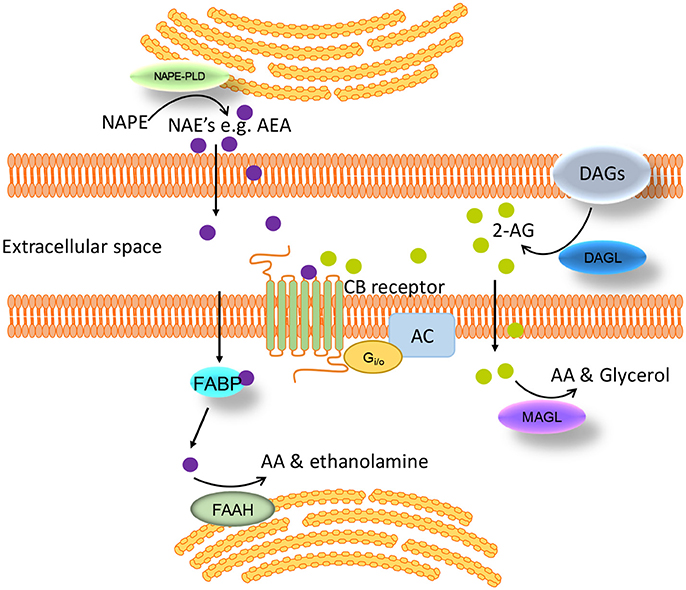
Figure 2. Schematic of the synthesis, degradation and action of endocannabinoids at cannabinoid receptors. Endogenous lipid messengers, such as AEA and 2-AG, act on cannabinoid receptors. AEA and 2-AG are synthesized on demand and degraded by cellular uptake and enzymatic hydrolysis by FAAH and MAGL respectively. FABP carries AEA from the cell membrane to the endoplasmic reticulum where it is finally converted to AA by FAAH. AA, arachidonic acid; AC, adenylate cyclase; AEA, anandamide; 2-AG, 2-arachidonoylglycerol; CB, cannabinoid; DAGs, diacylglycerols; DAGL, diacylglycerol lipase; FAAH, fatty acid amide hydrolase; FABP, fatty acid-binding protein; MAGL, monoacylglycerol lipase; NAE, N-acylethanolamines; NAPE, N-acylphosphatidylethanolamine; NAPE-PLD, NAPE-specific phospholipase D.
The receptors for endocannabinoids, CB1 and CB2 are members of the G-protein coupled receptor family, being predominantly coupled to the Gi/oα proteins that inhibit adenylyl cyclase thereby reducing cellular cAMP levels (57, 58). However, coupling to other effector proteins has also been reported, including activation of Gq and Gs proteins, inhibition of voltage-gated calcium channels, activation of inwardly rectifying potassium channels, β-arrestin recruitment and activation of mitogen-activated protein kinase (MAPK) signaling pathways (59). As a result of CB receptor signaling through multiple effector proteins the probability of biased signaling (ligand-dependent selectivity for specific signal transduction pathways) increases. Biased signaling is thought to occur when different ligands bind to the receptor causing different conformational changes to the receptor enabling the receptor to preferentially signal one pathway over the other (60, 61). This is attractive, in terms of development of pharmacotherapies for various diseases, as it suggests the possibility of being able to design a drug that will activate/inhibit a specific intracellular pathway.
The endocannabinoid system drives food intake via CB1 (62). Administration of CB1 agonists induces feeding in rodents (63) and humans (64), while blocking CB1 reduces food intake (65). Further, overactivity of the endocannabinoid system perpetuates the problems associated with obesity (62) and drugs targeting CB1 have been used therapeutically to manage obesity but withdrawn due to CNS side effects (66). New evidence indicates the endocannabinoid system can control food intake by a peripheral mechanism of action (66). Peripherally-restricted CB1 antagonists, with no direct central effects, reduce food intake and body weight in rodents (67, 68).
The Endocannabinoid System and TRPV1
Endocannabinoids, such as AEA, are also endogenous ligands for TRPV1 (69). Capsaicin, an agonist of TRPV1, has an anti-obesity effect in rodents (14) and reduces food intake in humans (70). Therefore, the effects of endocannabinoids on food intake will depend on the site of action (Figure 3). This is complicated further as effects may be mediated via cross talk between TRPV1 and CB1. It has been demonstrated that CB1 can enhance or inhibit TRPV1 channel activity depending on whether it activates the phospholipase C (PLC)-PKC or inhibits the adenylate cyclase (AC)-PKA pathways respectively (71) (Figure 3). This interaction appears to be dose-dependent. Moderate to high concentrations of AEA (1–10 μM) have been shown to activate TRPV1 in a PKC dependent manner (34, 35). Conversely, low doses of AEA (3–30 nM) inhibit TRPV1 activity (72, 73), presumably through CB1 mediated inhibition of AC (74). Therefore, enzymatic synthesis and breakdown of endocannabinoids are potentially important determinants of TRPV1 activity in tissue, such as neuronal tissue, that co-express TRPV1 and CB1 (71). A clearer understanding of the role endocannabinoids play in food intake regulation in health and obesity is required to determine the physiological relevance of these different interactions. In mice, the levels of AEA in the small intestinal mucosa and plasma were elevated in high fat diet-induced obese mice compared to controls (68) but still within the low dose range (3–30 nM) shown to inhibit TRPV1 (72, 73). Consistent with these observations food intake was reduced by treatment with a peripherally restricted CB1 antagonist (68). Similar observations were made in humans with plasma anandamide levels elevated in obese compared to overweight or lean individuals, again to levels consistent with TRPV1 inhibition. Therefore, the physiological significance of TRPV1 activation observed at moderate to high concentrations of AEA remain to be determined.
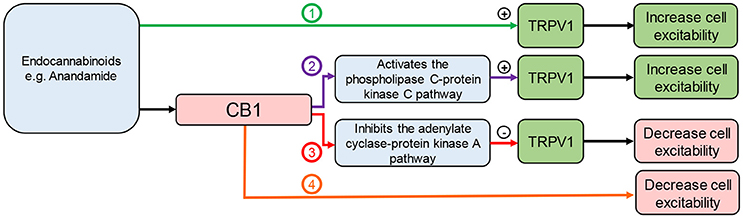
Figure 3. Interaction between endocannabinoids, TRPV1, and CB1. Endocannabinoids can: (1) directly activate TRPV1 leading to cannabinoid receptor 1 (CB1)-independent effects; (2) activate CB1 leading to activation of the phospholipase C pathway enhancing TRPV1 activity; (3) activate CB1 leading to inhibition of the adenylate cyclase pathway inhibiting TRPV1 activity; (4) activate CB1 leading to TRPV1-independent effects.
Involvement of TRPV1 in Energy Homeostasis
Reports on TRPV1 mediated regulation of energy intake and expenditure are conflicting. Nonetheless, epidemiological data indicate that consumption of food containing capsaicin is associated with a lower prevalence of obesity (75, 76). Further, in a clinical trial capsinoid supplementation for 12 weeks decreased body weight in overweight individuals compared to the placebo control group (77). In a separate trial, capsinoid supplementation for only 4 weeks resulted in a trend toward a decrease in body weight (78). Under laboratory conditions, dietary capsaicin supplementation had no effect on body weight (14) in mice fed a standard laboratory diet. However, in high-fat diet-induced obese mice, dietary supplementation of capsaicin significantly reduced weight gain (14, 79, 80). Further, reduced weight gain was also observed in high fat diet mice after topical application of capsaicin (81). This appears to be consistent across species as a study in rabbits, fed a standard laboratory diet supplemented with cholesterol and corn oil, demonstrated that dietary capsaicin reduced weight gain (82). In contrast, it has been shown that a 6 week dietary capsaicin treatment had no effect on body weight in high fat diet mice (83). Weight gain in TRPV1-knockout (KO) mice has been reported to be reduced (84), increased (85) or unchanged (86, 87) compared to wild type mice. This variability may reflect the study design. For example, TRPV1 channels can be activated by the endogenous ligand AEA (69). The production of AEA is dependent on dietary fat and therefore even slight changes in diet will impact on research outcomes. A summary of the effect of TRPV1 on energy homeostasis in humans can be found in Table 1. The following sections integrate the data on energy intake and expenditure from human and animal studies in an attempt to draw some clear conclusions and directions for further study.
Role of TRPV1 in Energy Intake
The effects of capsaicin supplementation on satiety and food intake are illustrated in Table 1. In human studies, dietary supplementation of a TRPV1 agonist such as capsaicin, or the less pungent sweet form capsiate, caused a short-term trend or significant decrease in energy intake along with an increase in satiety (88, 89, 91, 92, 97). These effects could at least in part be due to the effect of TRPV1 on appetite hormones and/or gastrointestinal vagal afferents. This will be discussed in detail below.
Conversely, other data from human (90, 92) and animal studies (82, 105–108) suggest that dietary supplementation of capsaicin has no effects on energy intake. This could be due to capsaicin mediated TRPV1 desensitization where food intake is initially reduced, due to capsaicin activation of the TRPV1 channel, but shortly returns to normal, due to a desensitization of the channel following the initial transient activation (14). In a Chinese adult cohort study, it has been shown that energy intake depends on the amount of chili consumed with individuals with chili consumption below 20 g per day and above 50 mg per day having reduced and increased energy intake respectively, compared to non-consumers (76). Therefore, it is possible that at low levels of consumption capsaicin activates TRPV1 leading to a reduction in food intake and at high levels it could be desensitizing TRPV1 leading to an increase in food intake. However, this is highly speculative and requires further investigation.
Dietary supplementation of capsaicin can also influence nutrient preference. It has been demonstrated that capsaicin ingestion reduced the desire for and subsequent intake of fatty foods (91, 97, 98), whilst also increasing the desire for and intake of carbohydrates (92, 97). Conversely, in other studies, capsaicin ingestion reduced the desire for and consumption of carbohydrates, and increased the desire for salt rich foods (91, 102). The sensory mechanisms responsible for the changes in food preferences remain to be determined.
TRPV1 and Appetite Hormones
There is evidence to indicate that TPRV1 interacts with appetite regulating hormones, most notably, ghrelin, leptin, and glucagon-like peptide-1 (GLP-1). Ghrelin is an orexigenic peptide mainly expressed in the stomach as an endogenous ligand for the growth hormone secretagogue receptor (GHSR) (109). It is involved in many processes including appetite regulation, secretion of gastric acid, gastrointestinal motility, and regulation of energy storage (110). It has been reported that TRPV1 activation reduced plasma ghrelin levels (93), which may account for the reduced food intake observed after capsaicin supplementation (88, 89, 91, 92, 97). However, this requires more intensive investigation. Within the stomach ghrelin maybe involved in the interaction between the endocannabinoid system and TRPV1. CB1 receptors co-expressed with ghrelin in specialized cells within the stomach wall (111). Ghrelin reduces gastric vagal afferent mechanosensitivity, in a manner dependent on nutritional status, via action at GHSR expressed on vagal afferents (112–115). Therefore, although the eating stimulatory effects of ghrelin are not thought to be mediated by vagal afferents (113), ghrelin acting on vagal afferents may impact on the amount of food consumed after the initiation of a meal. Inhibition of CB1 decreases gastric ghrelin secretion with subsequent, vagal afferent mediated, reductions in food intake (111). Therefore, part of the effect of endocannabinoids on vagal afferent activity maybe mediated indirectly via the activation of CB1 on ghrelin-producing cells. It is conceivable that the inhibitory effects of ghrelin are mediated via TRPV1 considering that, in the CNS, ghrelin effects on supraoptic magnocellular neurons are mediated via TRPV1 (116). Similar, interactions with the endocannabinoid system are observed centrally in areas associated with appetite regulation, including the hypothalamic arcuate and paraventricular nuclei (117). A comprehensive investigation of the interactions between the endocannabinoid system, ghrelin and TRPV1 is required to fully understand their role in appetite regulation.
Leptin is a satiety hormone produced and secreted in proportion to the amount of white adipose tissue (WAT). Data suggests that it is also secreted by gastric cells (118). There is evidence to suggest that TRPV1 and leptin may interact, since TRPV1 -/- mice exhibit increased basal leptin levels, even when normalized to WAT mass (85). Exogenous administration of leptin normally results in decreased food intake; however, this was not observed in TPRV1 -/- mice (85). Furthermore, there is evidence for direct interactions between leptin and TRPV1 in certain brain stem regions. For example, TRPV1 activation increased the frequency of miniature excitatory synaptic currents in leptin receptor containing neurons of gastric-related dorsal motor nucleus of the vagus (DMV) (119). These data suggest that TRPV1 may mediate the effects of leptin; however, further research is needed to substantiate these claims and to determine if leptin effects in the periphery are also mediated through TRPV1.
GLP-1 is a peptide hormone secreted by intestinal L-cells, pancreatic α-cells, and neurons in the brainstem and hypothalamus (120). Evidence suggests it is involved in appetite regulation, gastric emptying, gastrointestinal motility (121), insulin secretion, and glucagon inhibition (122). Capsaicin supplementation enhanced the increase in plasma GLP-1 levels observed after a meal (93) suggesting TRPV1 channel activation may play a role in GLP-1 secretion. This requires further investigation but has the potential to be a peripheral target for the treatment of obesity and/or diabetes.
TRPV1 and Gastrointestinal Vagal Afferents
Gastrointestinal vagal afferents are an important link between the gut and brain. They relay information on the arrival, amount and nutrient composition of a meal to the hindbrain where it is processed and gastrointestinal reflexes are coordinated with behavioral responses and sensations such as satiety and fullness (123–125). The role of gastrointestinal vagal afferents in the control of food intake has been extensively reviewed previously (126). Briefly, as food is ingested the vagal afferents innervating the stomach respond to mechanical stimulation as undigested food enters, fills and distends the stomach wall. There are two fundamental classes of mechanosensitive vagal afferent ending in the stomach according to location and response to mechanical stimulation (127, 128): mucosal receptors respond to fine tactile stimulation and tension receptors respond to distension and contraction of the stomach wall. Gastric mechanosensitive vagal afferents can be modulated by gut hormones and adipokines in a nutritional status dependent manner (114, 129, 130). As gastric emptying occurs, nutrients enter the small intestine and interact with nutrient receptors on the surface of specialized cells within the intestinal mucosa. This initiates an intracellular cascade that culminates in the release of gut hormones (126). These hormones can act in a paracrine fashion on vagal afferent endings innervating the small intestine and/or act as true hormones by coordinating activities within the gut or by entering the circulation and acting in the brain.
It has been demonstrated that TRPV1 is expressed in rat duodenal (131), mouse jejunal (132) and mouse gastric vagal afferents (13, 87). Activation of TRPV1, by oleoylethanolamide (OEA), caused depolarisation of nodose neurons and decreased short-term food intake (133). Further, OEA increased gastric vagal afferent tension receptor mechanosensitivity in lean but not high fat diet-induced obese mice (87). In standard laboratory diet fed TRPV1−/− mice, the response of gastric vagal afferent tension (but not mucosal) receptors to mechanical stimulation was reduced compared to TRPV1+/+ mice (13, 87). This was associated with an increase in food intake in the standard laboratory diet fed TRPV1−/− mice (87). However, the increase in food intake could also be due to the involvement of TRPV1 in gut hormone release (93, 97) or its interaction with leptin in central regions, such as the DMV (119), as described in detail above. Nonetheless, this data suggests that TRPV1 is involved in gastric vagal afferent signaling.
In high fat diet-induced obese mice the response of gastric tension receptors to distension was dampened (87) an effect also observed in jejunal vagal afferents (134). Gastric tension receptor mechanosensitivity in high fat diet-fed TRPV1−/− mice was not significantly different compared to standard laboratory diet fed TRPV1−/− mice (87). This suggests that disrupted TRPV1 signaling plays a role in the dampened vagal afferent signaling observed in high fat diet-induced obesity, however, this requires further investigation. Interestingly, CB1 receptors are also expressed in vagal afferent neurons (135, 136) and therefore it is conceivable that there is an interaction between TRPV1 and CB1 in gastric vagal afferent signaling, however, this has yet to be confirmed.
Role of TRPV1 in Energy Expenditure
There is increasing evidence that capsaicin ingestion may have desirable metabolic outcomes such as increased metabolic rate and fat oxidation. It was reported that dietary capsaicin supplementation lowered the respiratory quotient indicating decreased carbohydrate oxidation and increased fat oxidation (90, 91, 96, 100). In contrast, there is data demonstrating that dietary capsaicin increased the respiratory quotient (104, 103). The differences in study design, which may account for the different outcomes, include method of ingestion (capsule vs. meal), active ingredient (capsinoid vs. capsaicin) and the population studied (habitual chili consumers, non-habitual, normal weight, overweight, fitness level). For example, the study by Lim et al. specifically used “runners” for their investigation (103). There is some evidence that capsaicin can elevate energy expenditure by action on the sympathetic nervous system (SNS) or adipose tissue; this is discussed below.
TRPV1 and the Sympathetic Nervous System
The SNS is involved in many processes and is probably best known for its involvement in the “flight or fight” response. Dietary supplementation of capsaicin increases postprandial SNS activity (70, 101, 102). Capsaicin excites TRPV1 containing afferent nerves, carrying a signal to the spinal cord (137). Efferent nerves are then excited by the central nervous system leading to elevated catecholamine (e.g., epinephrine, norepinephrine, and dopamine) release from the adrenal medulla (137–139). Catecholamines can bind β-adrenergic receptors increasing expenditure and thermogenic activity (104, 140). This suggests that TRPV1 may directly stimulate heat production. Further, these effects of TRPV1 on SNS activity are lost in obese subjects suggesting TRPV1 dysfunction in obesity (101).
TRPV1 and Adipose Tissue
Adipose tissue plays a key role in energy homeostasis (141). WAT generally stores excess energy as lipids, and oxidizes these stores when required, whereas, brown adipose tissue (BAT) is specialized for energy dissipation (142, 143). TRPV1 has been shown to be expressed in 3T3-L1 and HB2 adipocyte cell lines, brown adipocytes, 144–BAT and WAT (147). Data indicate that TRPV1 may prevent the development of mature adipocytes from pre-adipocytes, and decrease their lipid content by increasing lipolysis (14). This may partially explain the decreased lipid accumulation during dietary supplementation of capsaicin. Further, it has been demonstrated that capsaicin induces browning in differentiating 3T3-L1 preadipocytes (145). Therefore, TRPV1 could be involved in the browning of WAT and the thermogenic activity of brown adipose tissue (BAT). The levels of TRPV1 mRNA in BAT and WAT are reduced in HFD-induced obesity and leptin receptor deficient mice (147) suggesting possible involvement in the development of obesity.
Browning is a process whereby WAT becomes thermogenic in nature, similar to BAT. The calcium influx from TRPV1 activation may mediate this process by activating the peroxisome proliferator-activated receptor gamma (PPARγ) and positive regulatory domain containing 16 (PRDM16) pathways (107). Calcium binds and activates calmodulin-dependent protein kinase II (CaMKII) leading to the subsequent activation of adenosine monophosphate activated protein kinase (AMPK) and sirtuin-1 (SIRT-1). SIRT-1 deacetylates PRDM and PPARγ causing browning events such as thermogenesis (Figure 4) (107).
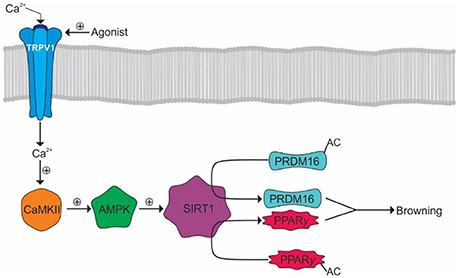
Figure 4. Browning of WAT by TRPV1 activation. Activation of TRPV1 results in Ca2+ influx and the subsequent activation of CaMKII. CaMKII facilitates the subsequent activation of AMPK and SIRT1 allowing the deacetylation of PRDM16 and PPARγ allowing their interaction and promotion of WAT browning.
TRPV1 activation may also promote BAT thermogenesis either through modulation of the SNS or via direct activation of BAT. However, research in this area is limited. The PPARγ and PRDM16 pathway, previously mentioned in WAT, has been shown to be activated by TRPV1, via SIRT1, in BAT (107, 108). Further, SIRT1 also activates peroxisome proliferator-activated receptor gamma coactivator 1-α (PGC-1α). PGC-1α transcriptionally activates PPARα subsequently leading to the production of uncoupling protein-1 (UCP-1) (108). UCP-1 is a mitochondrial protein that uncouples the respiratory chain triggering a more efficient substrate oxidation and thus heat generation (148). Lastly, TRPV1 activates bone morphogenic protein 8b in BAT, which also contributes to thermogenesis (Figure 5) (108).
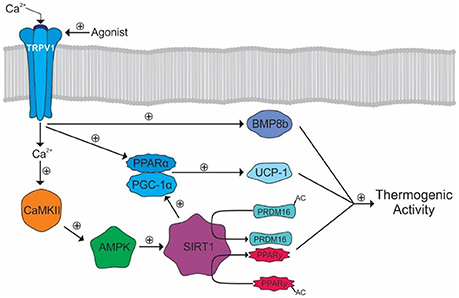
Figure 5. TRPV1 mediated activation of thermogenic activity in BAT. The calcium influx activates CaMKII leading to the eventual activation of SIRT1 and deacetylation of PRDM16 and PPARγ. SIRT1 also activates PGC-1α leading to the activation of PPARα. PGC-1α and PPARα transcriptionally activate UCP-1. TRPV1 also activates BMP8b which, along with UCP-1, PRDM, and PPARγ, cause increased thermogenic activity.
Regulation of BAT by TRPV1 can also be via an indirect mechanism through modulation of the SNS. Activation of TRPV1 in the gastrointestinal tract, by capsaicin or its analogs, has been shown to enhance thermogenesis and activate UCP-1 in BAT in mice (149, 150) via a mechanism mediated via extrinsic nerves innervating the gastrointestinal tract (149). This is consistent with reports that TRPV1 ligands (capsaicin and acid) increase gastrointestinal afferent activity (151, 152) via TRPV1 (152). Further, it has been demonstrated that ingestion of capsinoids increases energy expenditure through activation of BAT in humans (153). Gastrointestinal vagal afferents have central endings in the nucleus tractus solitaries (NTS). The NTS has projections to BAT (154) where it regulates the sympathetic tone to BAT (155) and has been directly implicated in the control of thermogenesis (156, 157). Lipid activation of duodenal vagal afferents has been shown to increase BAT temperature via a cholecystokinin dependent mechanism (158). In contrast, it has been reported that vagal afferent activation decreases BAT sympathetic nerve activity and BAT thermogenesis in rats (159). Further, glucagon-like peptide-1 activation of gastrointestinal vagal afferents leads to a reduction in energy expenditure and BAT thermogenesis in mice (160). It is possible that different subtypes of gastrointestinal vagal afferent have different roles in the control of BAT thermogenesis, however, this requires further investigation along with the role of TRPV1 in this gut-brain-BAT pathway.
Capsaicin can also evoke a heat-loss response which could conceivably result in compensatory thermogenesis to maintain thermal homeostasis. Capsaicin evoked complex heat-loss responses have been shown in various mammals including the rat, mouse, guinea-pig, rabbit, dog, goat, and humans (161). In humans, cutaneous vasodilation and sweating in response to hot chili consumption is well recognized (161). In the rat it has been demonstrated that capsaicin elicited cutaneous vasodilation resulting in a reduction in core body temperature (162). Simultaneously, capsaicin also enhanced heat production (162). In these experiments, it appeared that capsaicin independently activated pathways for heat production and heat loss and therefore the observed thermogenesis may not be a simple compensatory mechanism in response to heat loss, however, this requires further investigation.
Involvement of TRPV1 in Diabetes
Type 1 Diabetes
Insulin is a hormone, secreted by β-cells of the pancreatic islets, which regulates blood glucose levels. Type 1 diabetes is an autoimmune disease involving T cell-targeted destruction of pancreatic β-cells. TRPV1 is expressed in sensory nerves innervating the pancreas. Chemical denervation of these TRPV1 containing pancreatic afferents, using high doses of capsaicin (approximately 150 mg/kg body weight), significantly reduced blood glucose levels and increased plasma insulin (163), suggesting that TRPV1 containing pancreatic afferents negatively regulate insulin secretion. Further, chemical destruction of TRPV1 expressing neurons in neonatal mice (164) was able to protect the mice from autoimmune diabetes (165). Chemical denervation of TRPV1 containing pancreatic afferents significantly reduced the levels of pre-type 1 diabetes immune markers such as CD4+ and CD25+ T-regulatory cells in pancreatic lymph tissue and reduced the infiltration of CD8-CD69 positive effector T-cells (165, 166); immune cells implicated in the destruction of pancreatic islets in type 1 diabetes. However, as the afferents are destroyed and the treatment is not selective for TRPV1, it is plausible that the observed effects have nothing to do with TRPV1. Pancreatic islets also include resident dendritic cells which are generally believed to express TRPV1 (167–169) although there is some controversy (170). Activation of TRPV1 channels on dentritic cells could activate cell function including antigen presentation to CD4+ T cells. Further, TRPV1 channels have been shown to be expressed on rat pancreatic β-cells where they control the release of insulin leading to reduced blood glucose levels (171) and capsaicin has been shown to reduce blood glucose by increasing insulin levels in a streptozotocin-induced diabetic rat model (172). Taken together, these data suggest that TRPV1 may influence insulin secretion and type 1 diabetes acting via a number of different cell types within the pancreas.
Type 2 Diabetes
Insulin resistance, closely linked to obesity (173), occurs when cells are less responsive to insulin. As a consequence there is reduced blood glucose uptake leading to increased blood glucose levels. Pancreatic β-cells normally respond to this by increasing output of insulin to meet the needs of the tissues. Development of type 2 diabetes stems from a failure of the β-cells to adequately compensate for insulin resistance (174). It has been demonstrated that postprandial insulin levels were lower after the consumption of a standardized meal seasoned with cayenne pepper (175). As the plasma glucose levels were not significantly different from the control group the authors suggested that glucose clearance occurred similarly with lower levels of insulin, implying increased insulin sensitivity after the consumption of the hot meal. Further, consumption of chili has been shown to decrease postprandial insulin levels in obese subjects (176). In support of these studies, TRPV1−/− mice have been shown to be more insulin resistant than wild type mice (85).
Type 2 diabetes is believed to be associated with inflammation (177, 178). It is believed that inflammation in the pancreas leads to an increase in the activity of TRPV1 which contributes to increasing levels of calcitonin gene-related peptide (CGRP) (179). CGRP is known to promote insulin resistance and obesity by decreasing insulin release from β-cells (180).
TRPV1 as a Pharmacological Target for Obesity and Diabetes
Behavioral interventions (e.g., diet and exercise) alone are seldom sufficient for the intervention of obesity and diabetes. Combining behavioral and pharmacological approaches is becoming increasingly more attractive. However, pharmacological interventions can have hard to access targets and/or adverse side effects. TRPV1 is present in the periphery making it an easily accessible target compared to drugs that target the central nervous system. However, TRPV1 interacts with other systems and shares pathways commonly used by other signaling molecules. Therefore, without a clear understanding of the interactions of TRPV1 with other systems, the targeting TRPV1 for the treatment of obesity and diabetes is unlikely to be successful, as evident from the numerous contradictory studies looking at the effect of capsaicin analogs on food intake and weight gain. Data suggest that manipulation of TRPV1 may be possible in such a way to reduce or eliminate any unwanted side effects. For example, three different TRPV1 ligands known to antagonize TRPV1 had different effects on thermoregulation (e.g., hyperthermia, hypothermia, or no effect) (181). In fact, TRPV1 can be manipulated in such a way, by action at different domains, to eliminate some functions of the TRPV1 channels without affecting others. For example, some antagonists block activation by capsaicin and high temperatures but not activation by low pH (182), and other antagonists block activation by capsaicin but not the activation by high temperature (183). However, this raises further questions on whether, for example, the observed effects are cell type specific. Again, this highlights the lack of fundamental knowledge on the role of TRPV1 in energy homeostasis and therefore the current challenges of targeting TRPV1 for the treatment of obesity.
Conclusion
TRPV1 appears to be involved in energy homeostasis at a number of levels. In the periphery, TRPV1 activation or inhibition can have an impact of appetite and food intake through the control of appetite hormone levels or via the modulation of gastrointestinal vagal afferents, important for determining meal size and meal duration. In addition, TRPV1 plays a role in energy expenditure via heat production, either via direct thermogenesis or as a compensatory mechanism in response to TRPV1 induced heat-loss. Dietary supplementation with TRPV1 analogs, such as capsaicin, has yielded conflicting results with some studies demonstrating a decrease in food intake and increase in energy expenditure and others indicating the converse. This is probably reflective of the involvement of TRPV1 in a multitude of processes regulating food intake and energy expenditure. The story is complicated further by the interaction TRPV1 has with other systems involved in energy homeostasis, such as the endocannabinoid system. In addition, TRPV1 appears to be dysregulated in obesity, possibly due to alterations in the interaction with other systems. Therefore, although it is clear that TRPV1 plays a role in energy homeostasis without improved knowledge of the fundamental physiological mechanisms involved and the interactions with other systems it is impossible to target this system for the treatment of obesity, the maintenance of weight loss and the metabolic diseases associated with obesity.
Author Contributions
SC wrote the review under the supervision of GW and AP. HL edited the article and along with GW and AP provided assistance with the structure of the review.
Funding
AP and GW were awarded a Diabetes Australia Research Trust grant.
Conflict of Interest Statement
The authors declare that the research was conducted in the absence of any commercial or financial relationships that could be construed as a potential conflict of interest.
Abbreviations
AC, adenylyl cyclase; AEA, anandamide; AMPK, adenosine monophosphate kinase; BAT, brown adipose tissue; BGL, blood glucose level; BMP, bone morphogenic protein; CAMK, calmodulin dependent kinase; CB, cannabinoid; DMV, dorsal motor nucleus of the vagus; GI, gastrointestinal; GLP, glucagon like peptide; PGC, PPAR gamma coactivator; PI3K, phosphatidylinositol 4-5 bisphosphate 3-kinase; PIP2, phosphatidylinositol-4, 5-bisphosphate; PKA, protein kinase A; PKC, protein kinase C; PLC, phospholipase C; PPAR, peroxisome proliferator activated receptor; PRDM, positive regulatory domain; SIRT, sirtuin; SNS, somatic nervous system; TRPV, transient receptor potential vanilloid; UCP, uncoupling protein; WAT, white adipose tissue.
References
1. Gupta N, Goel K, Shah P, Misra A. Childhood obesity in developing countries: epidemiology, determinants, and prevention. Endocrine Rev. (2012) 33:48–70. doi: 10.1210/er.2010-0028
2. Smith KB, Smith MS. Obesity statistics. Prim Care (2016) 43:121–35. doi: 10.1016/j.pop.2015.10.001
3. Venkatachalam K, Montell C. TRP channels. Annu Rev Biochem. (2007) 76:387–417. doi: 10.1146/annurev.biochem.75.103004.142819
4. Cosens DJ, Manning A. Abnormal electroretinogram from a Drosophila mutant. Nature (1969) 224:285–7. doi: 10.1038/224285a0
5. Montell C, Rubin GM. Molecular characterization of the Drosophila trp locus: a putative integral membrane protein required for phototransduction. Neuron (1989) 2:1313–23. doi: 10.1016/0896-6273(89)90069-X
6. Tracey W. D Jr, Wilson RI, Laurent G, Benzer S, Painless, a Drosophila gene essential for nociception. Cell (2003) 113:261–73. doi: 10.1016/S0092-8674(03)00272-1
7. Asgar J, Zhang Y, Saloman JL, Wang S, Chung MK, Ro JY. The role of TRPA1 in muscle pain and mechanical hypersensitivity under inflammatory conditions in rats. Neuroscience (2015) 310:206–15. doi: 10.1016/j.neuroscience.2015.09.042
8. Cao E, Cordero-Morales JF, Liu B, Qin F, Julius D. TRPV1 channels are intrinsically heat sensitive and negatively regulated by phosphoinositide lipids. Neuron (2013) 77:667–79. doi: 10.1016/j.neuron.2012.12.016
9. Ocobock C. Human energy expenditure, allocation, and interactions in natural temperate, hot, and cold environments. Am J Phys Anthropol. (2016) 161:667–5. doi: 10.1002/ajpa.23071
10. Caterina MJ, Schumacher MA, Tominaga M, Rosen TA, Levine JD, Julius D. The capsaicin receptor: a heat-activated ion channel in the pain pathway. Nature (1997) 389:816–24. doi: 10.1038/39807
11. Edwards JG. TRPV1 in the central nervous system: synaptic plasticity, function, and pharmacological implications. Prog Drug Res. (2014) 68:77–104. doi: 10.1007/978-3-0348-0828-6_3
12. Eid SR, Cortright DN. (2009). Transient receptor potential channels on sensory nerves. Handb Exp Pharmacol. 194:261–81. doi: 10.1007/978-3-540-79090-7_8
13. Bielefeldt K, Davis BM. Differential effects of ASIC3 and TRPV1 deletion on gastroesophageal sensation in mice. Am J Physiol Gastrointest Liver Physiol. (2008) 294:G130–8. doi: 10.1152/ajpgi.00388.2007
14. Zhang LL, Yan Liu D, Ma LQ, Luo ZD, Cao TB, Zhong J, et al. Activation of transient receptor potential vanilloid type-1 channel prevents adipogenesis and obesity. Circ Res. (2007) 100:1063–70. doi: 10.1161/01.RES.0000262653.84850.8b
15. Cao E, Liao M, Cheng Y, Julius D. TRPV1 structures in distinct conformations reveal activation mechanisms. Nature (2013) 504:113–8. doi: 10.1038/nature12823
16. Liao M, Cao E, Julius D, Cheng Y. Structure of the TRPV1 ion channel determined by electron cryo-microscopy. Nature (2013) 504:107–12. doi: 10.1038/nature12822
17. Bhave G, Zhu W, Wang H, Brasier DJ, Oxford GS, Gereau RWT. cAMP-dependent protein kinase regulates desensitization of the capsaicin receptor (VR1) by direct phosphorylation. Neuron (2002) 35:721–31. doi: 10.1016/S0896-6273(02)00802-4
18. Lishko PV, Procko E, Jin X, Phelps CB, Gaudet R. The ankyrin repeats of TRPV1 bind multiple ligands and modulate channel sensitivity. Neuron (2007) 54:905–18. doi: 10.1016/j.neuron.2007.05.027
19. Dhaka A, Uzzell V, Dubin AE, Mathur J, Petrus M, Bandell M, et al. TRPV1 is activated by both acidic and basic pH. J Neurosci. (2009) 29:153–8. doi: 10.1523/JNEUROSCI.4901-08.2009
20. Salazar H, Llorente I, Jara-Oseguera A, Garcia-Villegas R, Munari M, Gordon SE, et al. A single N-terminal cysteine in TRPV1 determines activation by pungent compounds from onion and garlic. Nat Neurosci. (2008) 11:255–61. doi: 10.1038/nn2056
21. Koizumi K, Iwasaki Y, Narukawa M, Iitsuka Y, Fukao T, Seki T, et al. Diallyl sulfides in garlic activate both TRPA1 and TRPV1. Biochem Biophys Res Commun. (2009) 382:545–8. doi: 10.1016/j.bbrc.2009.03.066
22. Okumura Y, Narukawa M, Iwasaki Y, Ishikawa A, Matsuda H, Yoshikawa M, et al. Activation of TRPV1 and TRPA1 by black pepper components. Biosci Biotechnol Biochem. (2010) 74:1068–72. doi: 10.1271/bbb.90964
23. Iwasaki Y, Morita A, Iwasawa T, Kobata K, Sekiwa Y, Morimitsu Y, et al. A nonpungent component of steamed ginger–[10]-shogaol–increases adrenaline secretion via the activation of TRPV1. Nutr Neurosci. (2006) 9:169–78. doi: 10.1080/110284150600955164
24. Siemens J, Zhou S, Piskorowski R, Nikai T, Lumpkin EA, Basbaum AI, et al. Spider toxins activate the capsaicin receptor to produce inflammatory pain. Nature (2006) 444:208–12. doi: 10.1038/nature05285
25. Cromer BA, Mcintyre P. Painful toxins acting at TRPV1. Toxicon (2008) 51:163–73. doi: 10.1016/j.toxicon.2007.10.012
26. De Petrocellis L, Bisogno T, Maccarrone M, Davis JB, Finazzi-Agro A, Di Marzo V. The activity of anandamide at vanilloid VR1 receptors requires facilitated transport across the cell membrane and is limited by intracellular metabolism. J Biol Chem. (2001a) 276:12856–63. doi: 10.1074/jbc.M008555200
27. Jordt SE, Julius D. Molecular basis for species-specific sensitivity to “hot” chili peppers. Cell (2002) 108:421–30. doi: 10.1016/S0092-8674(02)00637-2
28. Smart D, Gunthorpe MJ, Jerman JC, Nasir S, Gray J, Muir AI, et al. The endogenous lipid anandamide is a full agonist at the human vanilloid receptor (hVR1). Br J Pharmacol. (2000) 129:227–30. doi: 10.1038/sj.bjp.0703050
29. Van Der Stelt M, Di Marzo V. Endovanilloids. Putative endogenous ligands of transient receptor potential vanilloid 1 channels. Eur J Biochem. (2004) 271:1827–34. doi: 10.1111/j.1432-1033.2004.04081.x
30. Vigna SR, Shahid RA, Nathan JD, Mcvey DC, Liddle RA. Leukotriene B4 mediates inflammation via TRPV1 in duct obstruction-induced pancreatitis in rats. Pancreas (2001) 40:708–14. doi: 10.1097/MPA.0b013e318214c8df
31. Rosenbaum T, Gordon-Shaag A, Munari M, Gordon SE. Ca2+/calmodulin modulates TRPV1 activation by capsaicin. J General Physiol. (2004) 123:53–62. doi: 10.1085/jgp.200308906
32. De Petrocellis L, Harrison S, Bisogno T, Tognetto M, Brandi I, Smith GD, et al. The vanilloid receptor (VR1)-mediated effects of anandamide are potently enhanced by the cAMP-dependent protein kinase. J Neurochem. (2001b) 77:1660–63. doi: 10.1046/j.1471-4159.2001.00406.x
33. Vyklicky L, Novakova-Tousova K, Benedikt J, Samad A, Touska F, Vlachova V. Calcium-dependent desensitization of vanilloid receptor TRPV1: a mechanism possibly involved in analgesia induced by topical application of capsaicin. Physiol Res. (2008) 57(Suppl. 3):S59–68.
34. Premkumar LS, Ahern GP. Induction of vanilloid receptor channel activity by protein kinase C. Nature (2000) 408:985–90. doi: 10.1038/35050121
35. Vellani V, Mapplebeck S, Moriondo A, Davis JB, Mcnaughton PA. Protein kinase C activation potentiates gating of the vanilloid receptor VR1 by capsaicin, protons, heat and anandamide. J Physiol. (2001) 534:813–25. doi: 10.1111/j.1469-7793.2001.00813.x
36. Chuang HH, Prescott ED, Kong H, Shields S, Jordt SE, Basbaum AI, et al. Bradykinin and nerve growth factor release the capsaicin receptor from PtdIns(4,5)P2-mediated inhibition. Nature (2001) 411:957–62. doi: 10.1038/35082088
37. Lu Y, Anderson HD. Cannabinoid signaling in health and disease. Can J Physiol Pharmacol. (2017) 95:311–27. doi: 10.1139/cjpp-2016-0346
38. Harrold JA, Williams G. The cannabinoid system: a role in both the homeostatic and hedonic control of eating? Br J Nutr. (2003) 90:729–34. doi: 10.1079/BJN2003942
39. Cristino L, Becker T, Di Marzo V. Endocannabinoids and energy homeostasis: an update. Biofactors (2014) 40:389–97. doi: 10.1002/biof.1168
40. Fowler CJ, Doherty P, Alexander SPH. Endocannabinoid turnover. Adv Pharmacol. (2017) 80:31–66. doi: 10.1016/bs.apha.2017.03.006
41. Natarajan V, Reddy PV, Schmid PC, Schmid HH. N-Acylation of ethanolamine phospholipids in canine myocardium. Biochim Biophys Acta (1982) 712:342–55. doi: 10.1016/0005-2760(82)90352-6
42. Natarajan V, Schmid PC, Reddy PV, Zuzarte-Augustin ML, Schmid HH. Biosynthesis of N-acylethanolamine phospholipids by dog brain preparations. J Neurochem. (1983) 41:1303–12. doi: 10.1111/j.1471-4159.1983.tb00825.x
43. Schmid PC, Reddy PV, Natarajan V, Schmid HH. Metabolism of N-acylethanolamine phospholipids by a mammalian phosphodiesterase of the phospholipase D type. J Biol Chem. (1983) 258:9302–6.
44. Bisogno T, Howell F, Williams G, Minassi A, Cascio MG, Ligresti A, et al. Cloning of the first sn1-DAG lipases points to the spatial and temporal regulation of endocannabinoid signaling in the brain. J Cell Biol. (2003) 163:463–8. doi: 10.1083/jcb.200305129
45. Fowler CJ. Transport of endocannabinoids across the plasma membrane and within the cell. FEBS J. (2013) 280:1895–904. doi: 10.1111/febs.12212
46. Nicolussi S, Gertsch J. Endocannabinoid transport revisited. Vitam Horm. (2015) 98:441–85. doi: 10.1016/bs.vh.2014.12.011
47. Kaczocha M, Glaser ST, Deutsch DG. Identification of intracellular carriers for the endocannabinoid anandamide. Proc Natl Acad Sci USA. (2009) 106:6375–80. doi: 10.1073/pnas.0901515106
48. Oddi S, Fezza F, Pasquariello N, D'agostino A, Catanzaro G, De Simone C, et al. Molecular identification of albumin and Hsp70 as cytosolic anandamide-binding proteins. Chem Biol. (2009) 16:624–32. doi: 10.1016/j.chembiol.2009.05.004
49. Sanson B, Wang T, Sun J, Wang L, Kaczocha M, Ojima I, et al. Crystallographic study of FABP5 as an intracellular endocannabinoid transporter. Acta Crystallogr D Biol Crystallogr. (2014) 70:290–8. doi: 10.1107/S1399004713026795
50. Ueda N, Yamanaka K, Yamamoto S. Purification and characterization of an acid amidase selective for N-palmitoylethanolamine, a putative endogenous anti-inflammatory substance. J Biol Chem. (2001) 276:35552–7. doi: 10.1074/jbc.M106261200
51. Tsuboi K, Zhao LY, Okamoto Y, Araki N, Ueno M, Sakamoto H, et al. Predominant expression of lysosomal N-acylethanolamine-hydrolyzing acid amidase in macrophages revealed by immunochemical studies. Biochim Biophys Acta (2007) 1771:623–32. doi: 10.1016/j.bbalip.2007.03.005
52. Wei BQ, Mikkelsen TS, Mckinney MK, Lander ES, Cravatt BF. A second fatty acid amide hydrolase with variable distribution among placental mammals. J Biol Chem. (2006) 281:36569–78. doi: 10.1074/jbc.M606646200
53. Kaczocha M, Glaser ST, Chae J, Brown DA, Deutsch DG. Lipid droplets are novel sites of N-acylethanolamine inactivation by fatty acid amide hydrolase-2. J Biol Chem. (2010) 285:2796–806. doi: 10.1074/jbc.M109.058461
54. Blankman JL, Simon GM, Cravatt BF. A comprehensive profile of brain enzymes that hydrolyze the endocannabinoid 2-arachidonoylglycerol. Chem Biol. (2007) 14:1347–56. doi: 10.1016/j.chembiol.2007.11.006
55. Pan B, Wang W, Zhong P, Blankman JL, Cravatt BF, Liu QS. Alterations of endocannabinoid signaling, synaptic plasticity, learning, and memory in monoacylglycerol lipase knock-out mice. J Neurosci. (2011) 31:13420–30. doi: 10.1523/JNEUROSCI.2075-11.2011
56. Zhong P, Pan B, Gao XP, Blankman JL, Cravatt BF, Liu QS. Genetic deletion of monoacylglycerol lipase alters endocannabinoid-mediated retrograde synaptic depression in the cerebellum. J Physiol. (2011) 589:4847–55. doi: 10.1113/jphysiol.2011.215509
57. Howlett AC. Reverse pharmacology applied to the cannabinoid receptor. Trends Pharmacol Sci. (1990) 11:395–7. doi: 10.1016/0165-6147(90)90142-U
58. Howlett AC. Pharmacology of cannabinoid receptors. Annu Rev Pharmacol Toxicol. (1995) 35:607–34. doi: 10.1146/annurev.pa.35.040195.003135
59. Ibsen MS, Connor M, Glass M. Cannabinoid CB1 and CB2 receptor signaling and bias. Cannabis Cannabinoid Res. (2017) 2:48–60. doi: 10.1089/can.2016.0037
60. Khajehali E, Malone DT, Glass M, Sexton PM, Christopoulos A, Leach K. Biased agonism and biased allosteric modulation at the CB1 cannabinoid receptor. Mol Pharmacol. (2015) 88:368–79. doi: 10.1124/mol.115.099192
61. Laprairie RB, Bagher AM, Kelly ME, Denovan-Wright EM. Biased type 1 cannabinoid receptor signaling influences neuronal viability in a cell culture model of Huntington disease. Mol Pharmacol. (2016) 89:364–75. doi: 10.1124/mol.115.101980
62. Sharkey KA, Pittman QJ. Central and peripheral signaling mechanisms involved in endocannabinoid regulation of feeding: a perspective on the munchies. Sci STKE (2005) 2005:pe15. doi: 10.1126/stke.2772005pe15
63. Jarbe TU, Dipatrizio NV. Delta9-THC induced hyperphagia and tolerance assessment: interactions between the CB1 receptor agonist delta9-THC and the CB1 receptor antagonist SR-141716 (rimonabant) in rats. Behav Pharmacol. (2005) 16:373–80. doi: 10.1097/00008877-200509000-00009
64. Foltin RW, Brady JV, Fischman MW. Behavioral analysis of marijuana effects on food intake in humans. Pharmacol Biochem Behav. (1986) 25:577–82. doi: 10.1016/0091-3057(86)90144-9
65. Scheen AJ, Finer N, Hollander P, Jensen MD, Van Gaal LF, Group RI-DS. Efficacy and tolerability of rimonabant in overweight or obese patients with type 2 diabetes: a randomised controlled study. Lancet (2006) 368:1660–72. doi: 10.1016/S0140-6736(06)69571-8
66. Shrestha N, Cuffe JSM, Hutchinson DS, Headrick JP, Perkins AV, Mcainch AJ, et al. Peripheral modulation of the endocannabinoid system in metabolic disease. Drug Discov Today (2018) 23:592–604. doi: 10.1016/j.drudis.2018.01.029
67. Cluny NL, Vemuri VK, Chambers AP, Limebeer CL, Bedard H, Wood JT, et al. A novel peripherally restricted cannabinoid receptor antagonist, AM6545, reduces food intake and body weight, but does not cause malaise, in rodents. Br J Pharmacol. (2010) 161:629–42. doi: 10.1111/j.1476-5381.2010.00908.x
68. Argueta DA, Dipatrizio NV. Peripheral endocannabinoid signaling controls hyperphagia in western diet-induced obesity. Physiol Behav. (2017) 171:32–9. doi: 10.1016/j.physbeh.2016.12.044
69. Ross RA. Anandamide and vanilloid TRPV1 receptors. Br J Pharmacol. (2003) 140:790–801. doi: 10.1038/sj.bjp.0705467
70. Yoshioka M, Doucet E, Drapeau V, Dionne I, Tremblay A. Combined effects of red pepper and caffeine consumption on 24 h energy balance in subjects given free access to foods. Br J Nutr. (2001) 85:203–11. doi: 10.1079/BJN2000224
71. Hermann H, De Petrocellis L, Bisogno T, Schiano Moriello A, Lutz B, Di Marzo V. Dual effect of cannabinoid CB1 receptor stimulation on a vanilloid VR1 receptor-mediated response. Cell Mol Life Sci. (2003) 60:607–16. doi: 10.1007/s000180300052
72. Mahmud A, Santha P, Paule CC, Nagy I. Cannabinoid 1 receptor activation inhibits transient receptor potential vanilloid type 1 receptor-mediated cationic influx into rat cultured primary sensory neurons. Neuroscience (2009) 162:1202–11. doi: 10.1016/j.neuroscience.2009.05.024
73. Santha P, Jenes A, Somogyi C, Nagy I. The endogenous cannabinoid anandamide inhibits transient receptor potential vanilloid type 1 receptor-mediated currents in rat cultured primary sensory neurons. Acta Physiol Hung. (2010) 97:149–58. doi: 10.1556/APhysiol.97.2010.2.1
74. Matsuda LA, Lolait SJ, Brownstein MJ, Young AC, Bonner TI. Structure of a cannabinoid receptor and functional expression of the cloned cDNA. Nature (1990) 346:561–4. doi: 10.1038/346561a0
75. Wahlqvist ML, Wattanapenpaiboon N. Hot foods–unexpected help with energy balance? Lancet (2001) 358:348–9. doi: 10.1016/S0140-6736(01)05586-6
76. Shi Z, Riley M, Taylor AW, Page A. Chilli consumption and the incidence of overweight and obesity in a Chinese adult population. Int J Obes. (2017) 41:1074–9. doi: 10.1038/ijo.2017.88
77. Snitker S, Fujishima Y, Shen H, Ott S, Pi-Sunyer X, Furuhata Y, et al. Effects of novel capsinoid treatment on fatness and energy metabolism in humans: possible pharmacogenetic implications. Am J Clin Nutr. (2009) 89:45–50. doi: 10.3945/ajcn.2008.26561
78. Inoue N, Matsunaga Y, Satoh H, Takahashi M. Enhanced energy expenditure and fat oxidation in humans with high BMI scores by the ingestion of novel and non-pungent capsaicin analogues (capsinoids). Biosci Biotechnol Biochem. (2007) 71:380–9. doi: 10.1271/bbb.60341
79. Baboota RK, Murtaza N, Jagtap S, Singh DP, Karmase A, Kaur J, et al. Capsaicin-induced transcriptional changes in hypothalamus and alterations in gut microbial count in high fat diet fed mice. J Nutr Biochem. (2014a) 25:893–902. doi: 10.1016/j.jnutbio.2014.04.004
80. Shen W, Shen M, Zhao X, Zhu H, Yang Y, Lu S, et al. Anti-obesity effect of capsaicin in mice fed with high-fat diet is associated with an increase in population of the gut bacterium Akkermansia muciniphila. Front Microbiol. (2017) 8:272. doi: 10.3389/fmicb.2017.00272
81. Lee GR, Shin MK, Yoon DJ, Kim AR, Yu R, Park NH, et al. Topical application of capsaicin reduces visceral adipose fat by affecting adipokine levels in high-fat diet-induced obese mice. Obesity (2013) 21:115–22. doi: 10.1002/oby.20246
82. Yu Q, Wang Y, Yu Y, Li Y, Zhao S, Chen Y, et al. Expression of TRPV1 in rabbits and consuming hot pepper affects its body weight. Mol Biol Rep. (2012) 39:7583–9. doi: 10.1007/s11033-012-1592-1
83. Song JX, Ren H, Gao YF, Lee CY, Li SF, Zhang F, et al. Dietary capsaicin improves glucose homeostasis and alters the gut microbiota in obese diabetic ob/ob mice. Front Physiol. (2017) 8:602. doi: 10.3389/fphys.2017.00602
84. Motter AL, Ahern GP. TRPV1-null mice are protected from diet-induced obesity. FEBS Lett. (2008) 582:2257–62. doi: 10.1016/j.febslet.2008.05.021
85. Lee E, Jung DY, Kim JH, Patel PR, Hu X, Lee Y, et al. Transient receptor potential vanilloid type-1 channel regulates diet-induced obesity, insulin resistance, and leptin resistance. FASEB J. (2015) 29:3182–92. doi: 10.1096/fj.14-268300
86. Marshall NJ, Liang L, Bodkin J, Dessapt-Baradez C, Nandi M, Collot-Teixeira S, et al. A role for TRPV1 in influencing the onset of cardiovascular disease in obesity. Hypertension (2013) 61:246–52. doi: 10.1161/HYPERTENSIONAHA.112.201434
87. Kentish SJ, Frisby CL, Kritas S, Li H, Hatzinikolas G, O'donnell TA, et al. TRPV1 channels and gastric vagal afferent signalling in lean and high fat diet induced obese mice. PLoS ONE (2015) 10:e0135892. doi: 10.1371/journal.pone.0135892
88. Janssens PL, Hursel R, Westerterp-Plantenga MS. Capsaicin increases sensation of fullness in energy balance, and decreases desire to eat after dinner in negative energy balance. Appetite (2014) 77:44–9. doi: 10.1016/j.appet.2014.02.018
89. Smeets AJ, Janssens PL, Westerterp-Plantenga MS. Addition of capsaicin and exchange of carbohydrate with protein counteract energy intake restriction effects on fullness and energy expenditure. J Nutr. (2013) 143:442–7. doi: 10.3945/jn.112.170613
90. Janssens PL, Hursel R, Martens EA, Westerterp-Plantenga MS. Acute effects of capsaicin on energy expenditure and fat oxidation in negative energy balance. PLoS ONE (2013) 8:e67786. doi: 10.1371/journal.pone.0067786
91. Ludy MJ, Mattes RD. The effects of hedonically acceptable red pepper doses on thermogenesis and appetite. Physiol Behav. (2011) 102:251–8. doi: 10.1016/j.physbeh.2010.11.018
92. Reinbach HC, Martinussen T, Moller P. Effects of hot spices on energy intake, appetite and sensory specific desires in humans. Food Quality Preference (2010) 21:655–61. doi: 10.1016/j.foodqual.2010.04.003
93. Smeets A, Westerterp-Plantenga M. The acute effects of a lunch containing capsaicin on energy and substrate utilization, hormones, and satiety. Eur J Nutr. (2009) 48:229–34. doi: 10.1007/s00394-009-0006-1
94. Chaiyasit K, Khovidhunkit W, Wittayalertpanya S. Pharmacokinetic and the effect of capsaicin in Capsicum frutescens on decreasing plasma glucose level. J Med Assoc Thai (2009) 92:108–113.
95. Reinbach HC, Smeets A, Martinussen T, Moller P, Westerterp-Plantenga MS. Effects of capsaicin, green tea and CH-19 sweet pepper on appetite and energy intake in humans in negative and positive energy balance. Clin Nutr. (2009) 28:260–5. doi: 10.1016/j.clnu.2009.01.010
96. Shin KO, Moritani T. Alterations of autonomic nervous activity and energy metabolism by capsaicin ingestion during aerobic exercise in healthy men. J Nutr Sci Vitaminol. (2007) 53:124–32. doi: 10.3177/jnsv.53.124
97. Westerterp-Plantenga MS, Smeets A, Lejeune MP. Sensory and gastrointestinal satiety effects of capsaicin on food intake. Int J Obes. (2005) 29:682–8. doi: 10.1038/sj.ijo.0802862
98. Yoshioka M, Imanaga M, Ueyama H, Yamane M, Kubo Y, Boivin A, et al. Maximum tolerable dose of red pepper decreases fat intake independently of spicy sensation in the mouth. Br J Nutr. (2004) 91:991–5. doi: 10.1079/BJN20041148
99. Chaiyata P, Puttadechakum S, Komindr S. Effect of chili pepper (Capsicum frutescens) ingestion on plasma glucose response and metabolic rate in Thai women. J Med Assoc Thai (2003) 86:854–60.
100. Lejeune MP, Kovacs EM, Westerterp-Plantenga MS. Effect of capsaicin on substrate oxidation and weight maintenance after modest body-weight loss in human subjects. Br J Nutr. (2003) 90:651–9. doi: 10.1079/BJN2003938
101. Matsumoto T, Miyawaki C, Ue H, Yuasa T, Miyatsuji A, Moritani T. Effects of capsaicin-containing yellow curry sauce on sympathetic nervous system activity and diet-induced thermogenesis in lean and obese young women. J Nutr Sci Vitaminol. (2000) 46:309–15. doi: 10.3177/jnsv.46.309
102. Yoshioka M, St-Pierre S, Drapeau V, Dionne I, Doucet E, Suzuki M, et al. Effects of red pepper on appetite and energy intake. Br J Nutr. (1999) 82:115–23.
103. Lim K, Yoshioka M, Kikuzato S, Kiyonaga A, Tanaka H, Shindo M, et al. Dietary red pepper ingestion increases carbohydrate oxidation at rest and during exercise in runners. Med Sci Sports Exerc. (1997) 29:355–61. doi: 10.1097/00005768-199703000-00010
104. Yoshioka M, Lim K, Kikuzato S, Kiyonaga A, Tanaka H, Shindo M, et al. Effects of red-pepper diet on the energy metabolism in men. J Nutr Sci Vitaminol. (1995) 41:647–56. doi: 10.3177/jnsv.41.647
105. Ohnuki K, Haramizu S, Oki K, Watanabe T, Yazawa S, Fushiki T. Administration of capsiate, a non-pungent capsaicin analog, promotes energy metabolism and suppresses body fat accumulation in mice. Biosci Biotechnol Biochem. (2001) 65:2735–40. doi: 10.1271/bbb.65.2735
106. Kang JH, Goto T, Han IS, Kawada T, Kim YM, Yu R. Dietary capsaicin reduces obesity-induced insulin resistance and hepatic steatosis in obese mice fed a high-fat diet. Obesity (2010) 18:780–7. doi: 10.1038/oby.2009.301
107. Baskaran P, Krishnan V, Ren J, Thyagarajan B. Capsaicin induces browning of white adipose tissue and counters obesity by activating TRPV1 channel-dependent mechanisms. Br J Pharmacol. (2016) 173:2369–89. doi: 10.1111/bph.13514
108. Baskaran P, Krishnan V, Fettel K, Gao P, Zhu Z, Ren J, et al. TRPV1 activation counters diet-induced obesity through sirtuin-1 activation and PRDM-16 deacetylation in brown adipose tissue. Int J Obes. (2017) 41:739–49. doi: 10.1038/ijo.2017.16
109. Kojima M, Hosoda H, Date Y, Nakazato M, Matsuo H, Kangawa K. Ghrelin is a growth-hormonereleasing acylated peptide from stomach. Nature (1999) 402:656–60. doi: 10.1038/45230
110. Sato T, Nakamura Y, Shiimura Y, Ohgusu H, Kangawa K, Kojima M. Structure, regulation and function of ghrelin. J Biochem. (2012) 151:119–28. doi: 10.1093/jb/mvr134
111. Senin LL, Al-Massadi O, Folgueira C, Castelao C, Pardo M, Barja-Fernandez S, et al. The gastric CB1 receptor modulates ghrelin production through the mTOR pathway to regulate food intake. PLoS ONE (2013) 8:e80339. doi: 10.1371/journal.pone.0080339
112. Date Y, Murakami N, Toshinai K, Matsukura S, Niijima A, Matsuo H, et al. The role of the gastric afferent vagal nerve in ghrelin-induced feeding and growth hormone secretion in rats. Gastroenterology (2002) 123:1120–8. doi: 10.1053/gast.2002.35954
113. Arnold M, Mura A, Langhans W, Geary N. Gut vagal afferents are not necessary for the eating-stimulatory effect of intraperitoneally injected ghrelin in the rat. J Neurosci. (2006) 26:11052–60. doi: 10.1523/JNEUROSCI.2606-06.2006
114. Page AJ, Slattery JA, Milte C, Laker R, O'donnell T, Dorian C, et al. Ghrelin selectively reduces mechanosensitivity of upper gastrointestinal vagal afferents. Am J Physiol Gastrointest Liver Physiol. (2007) 292:G1376–84. doi: 10.1152/ajpgi.00536.2006
115. Kentish S, Li H, Philp LK, O'donnell TA, Isaacs NJ, Young RL, et al. Diet-induced adaptation of vagal afferent function. J. Physiol. (2012) 590:209–21. doi: 10.1113/jphysiol.2011.222158
116. Yokoyama T, Saito T, Ohbuchi T, Suzuki H, Otsubo H, Okamoto T, et al. Ghrelin potentiates miniature excitatory postsynaptic currents in supraoptic magnocellular neurones. J Neuroendocrinol. (2009) 21:910–20. doi: 10.1111/j.1365-2826.2009.01911.x
117. Kola B, Farkas I, Christ-Crain M, Wittmann G, Lolli F, Amin F, et al. The orexigenic effect of ghrelin is mediated through central activation of the endogenous cannabinoid system. PLoS ONE (2008) 3:e1797. doi: 10.1371/journal.pone.0001797
118. Sobhani I, Bado A, Vissuzaine C, Buyse M, Kermorgant S, Laigneau J, et al. Leptin secretion and leptin receptor in the human stomach. Gut (2000) 47:178–83. doi: 10.1136/gut.47.2.178
119. Zsombok A, Jiang Y, Gao H, Anwar IJ, Rezai-Zadeh K, Enix CL, et al. Regulation of leptin receptor-expressing neurons in the brainstem by TRPV1. Physiol Rep. (2014) 2:e12160. doi: 10.14814/phy2.12160
120. Baggio LL, Drucker DJ. Biology of incretins: GLP-1 and GIP. Gastroenterology (2007) 132:2131–57. doi: 10.1053/j.gastro.2007.03.054
121. Torekov SS, Madsbad S, Holst JJ. Obesity–an indication for GLP-1 treatment? Obesity pathophysiology and GLP-1 treatment potential. Obesity Rev. (2011) 12:593–601. doi: 10.1111/j.1467-789X.2011.00860.x
122. Ryan D, Acosta A. GLP-1 receptor agonists: nonglycemic clinical effects in weight loss and beyond. Obesity (2015) 23:1119–29. doi: 10.1002/oby.21107
123. Broberger C. Brain regulation of food intake and appetite: molecules and networks. J Intern Med. (2005) 258:301–27. doi: 10.1111/j.1365-2796.2005.01553.x
124. Berthoud HR. The vagus nerve, food intake and obesity. Regul Pept (2008) 149:15–25. doi: 10.1016/j.regpep.2007.08.024
125. Hameed S, Dhillo WS, Bloom SR. Gut hormones and appetite control. Oral Dis. (2009) 15:18–26. doi: 10.1111/j.1601-0825.2008.01492.x
126. Page AJ, Kentish S. Plasticity of gastrointestingal vagal afferent satiety signals. Neurogastroenterol Motil. (2016) 29:e12973. doi: 10.1111/nmo.12973
127. Page AJ, Blackshaw LA. An in vitro study of the properties of vagal afferent fibres innervating the ferret oesophagus and stomach. J Physiol. (1998b) 512:907–16. doi: 10.1111/j.1469-7793.1998.907bd.x
128. Page AJ, Martin CM, Blackshaw LA. Vagal mechanoreceptors and chemoreceptors in mouse stomach and esophagus. J Neurophysiol. (2002) 87:2095–103. doi: 10.1152/jn.00785.2001
129. Kentish SJ, O'donnell TA, Isaacs NJ, Young RL, Li H, Harrington AM, et al. Gastric vagal afferent modulation by leptin is influenced by food intake status. J Physiol. (2013) 591:1921–34. doi: 10.1113/jphysiol.2012.247577
130. Kentish SJ, Li H, Frisby CL, Page AJ. Nesfatin-1 modulates murine gastric vagal afferent mechanosensitivity in a nutritional state dependent manner. Peptides (2017) 89:35–41. doi: 10.1016/j.peptides.2017.01.005
131. Zhoa H, Sprunger LK, Simasko SM. Expression of transient receptor potential channels and two-pore potassium channels in subtypes of vagal afferent neurons in rat. Am J Physiol Gastrointest Liver Physiol. (2009) 298:G212–21. doi: 10.1152/ajpgi.00396.2009
132. Tan LL, Bornstein JC, Anderson CR. Neurochemical and morphological phenotypes of vagal afferent neurons innervating the adult mouse jejunum. Neurogastroenterology Motil. (2009) 21:994–1001. doi: 10.1111/j.1365-2982.2009.01322.x
133. Wang X, Miyares RL, Ahern GP. Oleoylethanolamide excites vagal sensory neurones, induces visceral pain and reduces short-term food intake in mice via capsaicin receptor TRPV1. J Physiol. (2005) 564:541–7. doi: 10.1113/jphysiol.2004.081844
134. Daly DM, Park SJ, Valinsky WC, Beyak MJ. Impaired intestinal afferent nerve satiety signalling and vagal afferent excitability in diet induced obesity in the mouse. J Physiol. (2011) 589:2857–70. doi: 10.1113/jphysiol.2010.204594
135. Partosoedarso ER, Abrahams TP, Scullion RT, Moerschbaecher JM, Hornby PJ. Cannabinoid1 receptor in the dorsal vagal complex modulates lower oesophageal sphincter relaxation in ferrets. J Physiol. (2003) 550:149–58. doi: 10.1113/jphysiol.2003.042242
136. Burdyga G, Lal S, Varro A, Dimaline R, Thompson DG, Dockray GJ. Expression of cannabinoid CB1 receptors by vagal afferent neurons is inhibited by cholecystokinin. J Neurosci. (2004) 24:2708–15. doi: 10.1523/JNEUROSCI.5404-03.2004
137. Longhurst JC, Kaufman MP, Ordway GA, Musch TI. Effects of bradykinin and capsaicin on endings of afferent fibers from abdominal visceral organs. Am J Physiol. (1984) 247:R552–9. doi: 10.1152/ajpregu.1984.247.3.R552
138. Watanabe T, Kawada T, Yamamoto M, Iwai K. Capsaicin, a pungent principle of hot red pepper, evokes catecholamine secretion from the adrenal medulla of anesthetized rats. Biochem Biophys Res Commun. (1987) 142:259–64. doi: 10.1016/0006-291X(87)90479-7
139. Watanabe T, Kawada T, Kurosawa M, Sato A, Iwai K. Adrenal sympathetic efferent nerve and catecholamine secretion excitation caused by capsaicin in rats. Am J Physiol. (1988) 255:E23–27. doi: 10.1152/ajpendo.1988.255.1.E23
140. Kawada T, Watanabe T, Takaishi T, Tanaka T, Iwai K. Capsaicin-induced beta-adrenergic action on energy metabolism in rats: influence of capsaicin on oxygen consumption, the respiratory quotient, and substrate utilization. Proc Soc Exp Biol Med. (1986) 183:250–6. doi: 10.3181/00379727-183-42414
141. Spiegelman BM, Flier JS. Adipogenesis and obesity: rounding out the big picture. Cell (1996) 87:377–89. doi: 10.1016/S0092-8674(00)81359-8
142. Nedergaard J, Ricquier D, Kozak LP. Uncoupling proteins: current status and therapeutic prospects. EMBO Rep. (2005) 6:917–21. doi: 10.1038/sj.embor.7400532
143. Feldmann HM, Golozoubova V, Cannon B, Nedergaard J. UCP1 ablation induces obesity and abolishes diet-induced thermogenesis in mice exempt from thermal stress by living at thermoneutrality. Cell Metab. (2009) 9:203–9. doi: 10.1016/j.cmet.2008.12.014
144. Bishnoi M, Kondepudi KK, Gupta A, Karmase A, Boparai RK. Expression of multiple transient receptor potential channel genes in murine 3T3-L1 cell lines and adipose tissue. Pharmacol Rep. (2013) 65:751–5. doi: 10.1016/S1734-1140(13)71055-7
145. Baboota RK, Singh DP, Sarma SM, Kaur J, Sandhir R, Boparai RK, et al. Capsaicin induces “brite” phenotype in differentiating 3T3-L1 preadipocytes. PLoS ONE (2014b) 9:e103093. doi: 10.1371/journal.pone.0103093
146. Kida R, Yoshida H, Murakami M, Shirai M, Hashimoto O, Kawada T, et al. Direct action of capsaicin in brown adipogenesis and activation of brown adipocytes. Cell Biochem Funct. (2016) 34:34–41. doi: 10.1002/cbf.3162
147. Sun W, Li C, Zhang Y, Jiang C, Zhai M, Zhou Q, et al. Gene expression changes of thermo-sensitive transient receptor potential channels in obese mice. Cell Biol Int. (2017) 41:908–13. doi: 10.1002/cbin.10783
148. Brondani LDA, Assmann TS, Coutinho G, Duarte K, Gross JL, Canani LH, et al. The role of the uncoupling protein 1 (UCP1) on the development of obesity and type 2 diabetes mellitus. Arq Bras Endocrinol Metab. (2012) 56:215–25. doi: 10.1590/S0004-27302012000400001
149. Kawabata F, Inoue N, Masamoto Y, Matsumura S, Kimura W, Kadowaki M, et al. Non-pungent capsaicin analogs (capsinoids) increase metabolic rate and enhance thermogenesis via gastrointestinal TRPV1 in mice. Biosci Biotechnol Biochem. (2009) 73:2690–7. doi: 10.1271/bbb.90555
150. Okamatsu-Ogura Y, Tsubota A, Ohyama K, Nogusa Y, Saito M, Kimura K. Capsinoids suppress diet-induced obesity through uncoupling protein 1-dependent mechanism in mice. J Funct Foods (2015) 19:1–9. doi: 10.1016/j.jff.2015.09.005
151. Page AJ, Blackshaw LA. An in vitro study of the properties of vagal afferent fibres innervating the ferret oesophagus and stomach. J Physiol. (1998a). 512 (Pt 3):907–16.
152. Rong W, Hillsley K, Davis JB, Hicks G, Winchester WJ, Grundy D. Jejunal afferent nerve sensitivity in wild-type and TRPV1 knockout mice. J Physiol. (2004) 560:867–81. doi: 10.1113/jphysiol.2004.071746
153. Yoneshiro T, Aita S, Kawai Y, Iwanaga T, Saito M. Nonpungent capsaicin analogs (capsinoids) increase energy expenditure through the activation of brown adipose tissue in humans. Am J Clin Nutr. (2012) 95:845–50. doi: 10.3945/ajcn.111.018606
154. Bamshad M, Song CK, Bartness TJ. CNS origins of the sympathetic nervous system outflow to brown adipose tissue. Am J Physiol. (1999) 276:R1569–78. doi: 10.1152/ajpregu.1999.276.6.R1569
155. Madden CJ, Morrison SF. Hypoxic activation of arterial chemoreceptors inhibits sympathetic outflow to brown adipose tissue in rats. J Physiol. (2005) 566:559–73. doi: 10.1113/jphysiol.2005.086322
156. Skibicka KP, Grill HJ. Hypothalamic and hindbrain melanocortin receptors contribute to the feeding, thermogenic, and cardiovascular action of melanocortins. Endocrinology (2009) 150:5351–61. doi: 10.1210/en.2009-0804
157. Cao WH, Madden CJ, Morrison SF. Inhibition of brown adipose tissue thermogenesis by neurons in the ventrolateral medulla and in the nucleus tractus solitarius. Am J Physiol Regul Integr Comp Physiol. (2010) 299:R277–90. doi: 10.1152/ajpregu.00039.2010
158. Blouet C, Schwartz GJ. Duodenal lipid sensing activates vagal afferents to regulate non-shivering brown fat thermogenesis in rats. PLoS ONE (2012) 7:e51898. doi: 10.1371/journal.pone.0051898
159. Madden CJ, Santos Da Conceicao EP, Morrison SF. Vagal afferent activation decreases brown adipose tissue (BAT) sympathetic nerve activity and BAT thermogenesis. Temperature (2017) 4:89–96. doi: 10.1080/23328940.2016.1257407
160. Krieger JP, Santos Da Conceicao EP, Sanchez-Watts G, Arnold M, Pettersen KG, Mohammed M, et al. Glucagon-like peptide-1 regulates brown adipose tissue thermogenesis via the gut-brain axis in rats. Am J Physiol Regul Integr Comp Physiol. (2018). doi: 10.1152/ajpregu.00068.2018 [Epub ahead of print].
161. Szolcsanyi J. Capsaicin-type pungent agents producing pyrexia. In: Milton AS, editor. Handbook of Experimental Pharmacology. Berlin: Springer Verlag. (1982). p. 437–78.
162. Kobayashi A, Osaka T, Namba Y, Inoue S, Lee TH, Kimura S. Capsaicin activates heat loss and heat production simultaneously and independently in rats. Am J Physiol. (1998) 275:R92–8. doi: 10.1152/ajpregu.1998.275.1.R92
163. Gram DX, Ahren B, Nagy I, Olsen UB, Brand CL, Sundler F, et al. Capsaicin-sensitive sensory fibers in the islets of Langerhans contribute to defective insulin secretion in Zucker diabetic rat, an animal model for some aspects of human type 2 diabetes. Eur J Neurosci. (2007) 25:213–23. doi: 10.1111/j.1460-9568.2006.05261.x
164. Jancso G, Kiraly E, Jancso-Gabor A. Pharmacologically induced selective degeneration of chemosensitive primary sensory neurones. Nature (1977) 270:741–3. doi: 10.1038/270741a0
165. Razavi R, Chan Y, Afifiyan FN, Liu XJ, Wan X, Yantha J, et al. TRPV1+ sensory neurons control beta cell stress and islet inflammation in autoimmune diabetes. Cell (2006) 127:1123–35. doi: 10.1016/j.cell.2006.10.038
166. Diaz-Garcia CM, Morales-Lazaro SL, Sanchez-Soto C, Velasco M, Rosenbaum T, Hiriart M. Role for the TRPV1 channel in insulin secretion from pancreatic beta cells. J Membr Biol. (2014) 247:479–91. doi: 10.1007/s00232-014-9658-8
167. Basu S, Srivastava P. Immunological role of neuronal receptor vanilloid receptor 1 expressed on dendritic cells. Proc Natl Acad Sci USA. (2005) 102:5120–5. doi: 10.1073/pnas.0407780102
168. Toth BI, Benko S, Szollosi AG, Kovacs L, Rajnavolgyi E, Biro T. Transient receptor potential vanilloid-1 signaling inhibits differentiation and activation of human dendritic cells. FEBS Lett. (2009) 583:1619–24. doi: 10.1016/j.febslet.2009.04.031
169. Szollosi AG, Olah A, Toth IB, Papp F, Czifra G, Panyi G, et al. Transient receptor potential vanilloid-2 mediates the effects of transient heat shock on endocytosis of human monocyte-derived dendritic cells. FEBS Lett. (2013) 587:1440–5. doi: 10.1016/j.febslet.2013.03.027
170. O'connell PJ, Pingle SC, Ahern GP. Dendritic cells do not transduce inflammatory stimuli via the capsaicin receptor TRPV1. FEBS Lett. (2005) 579:5135–9. doi: 10.1016/j.febslet.2005.08.023
171. Akiba Y, Kato S, Katsube K, Nakamura M, Takeuchi K, Ishii H, et al. Transient receptor potential vanilloid subfamily 1 expressed in pancreatic islet beta cells modulates insulin secretion in rats. Biochem Biophys Res Commun. (2004) 321:219–25. doi: 10.1016/j.bbrc.2004.06.149
172. Zhang S, Ma X, Zhang L, Sun H, Liu X. Capsaicin reduces blood glucose by increasing insulin levels and glycogen content better than capsiate in streptozotocin-induced diabetic rats. J Agric Food Chem. (2017) 65:2323–30. doi: 10.1021/acs.jafc.7b00132
173. Kahn SE, Hull RL, Utzschneider KM. Mechanisms linking obesity to insulin resistance and type 2 diabetes. Nature (2006) 444:840–6. doi: 10.1038/nature05482
174. Fonseca VA. Defining and characterizing the progression of type 2 diabetes. Diabetes Care (2009) 32(Suppl. 2):S151–6. doi: 10.2337/dc09-S301
175. Ahuja KD, Robertson IK, Geraghty DP, Ball MJ. Effects of chili consumption on postprandial glucose, insulin, and energy metabolism. Am J Clin Nutr. (2006) 84:63–9. doi: 10.1093/ajcn/84.1.63
176. Kroff J, Hume DJ, Pienaar P, Tucker R, Lambert EV, Rae DE. The metabolic effects of a commercially available chicken peri-peri (African bird's eye chilli) meal in overweight individuals. Br J Nutr. (2017) 117:635–44. doi: 10.1017/S0007114515003104
177. Festa A, D'agostino R Jr, Howard G, Mykkanen L, Tracy RP, Haffner SM. Chronic subclinical inflammation as part of the insulin resistance syndrome: the Insulin Resistance Atherosclerosis Study (IRAS). Circulation (2000) 102:42–7. doi: 10.1161/01.CIR.102.1.42
178. Wu T, Dorn JP, Donahue RP, Sempos CT, Trevisan M. Associations of serum C-reactive protein with fasting insulin, glucose, and glycosylated hemoglobin: the third national health and nutrition examination survey, 1988-1994. Am J Epidemiol. (2002) 155:65–71. doi: 10.1093/aje/155.1.65
179. Gram DX, Hansen AJ, Wilken M, Elm T, Svendsen O, Carr RD, et al. Plasma calcitonin gene-related peptide is increased prior to obesity, and sensory nerve desensitization by capsaicin improves oral glucose tolerance in obese Zucker rats. Eur J Endocrinol. (2005) 153:963–9. doi: 10.1530/eje.1.02046
180. Pettersson M, Ahren B, Bottcher G, Sundler F. Calcitonin gene-related peptide: occurrence in pancreatic islets in the mouse and the rat and inhibition of insulin secretion in the mouse. Endocrinology (1986) 119:865–9. doi: 10.1210/endo-119-2-865
181. Gomtsyan A, Mcdonald HA, Schmidt RG, Daanen JF, Voight EA, Segreti JA, et al. TRPV1 ligands with hyperthermic, hypothermic and no temperature effects in rats. Temperature (2015) 2:297–301. doi: 10.1080/23328940.2015.1046013
182. Gavva NR, Tamir R, Klionsky L, Norman MH, Louis JC, Wild KD, et al. Proton activation does not alter antagonist interaction with the capsaicin-binding pocket of TRPV1. Mol Pharmacol. (2005) 68:1524–33. doi: 10.1124/mol.105.015727
183. Lehto SG, Tamir R, Deng H, Klionsky L, Kuang R, Le A, et al. Antihyperalgesic effects of (R,E)-N-(2-hydroxy-2,3-dihydro-1H-inden-4-yl)-3-(2-(piperidin-1-yl)-4-(trifluorom ethyl)phenyl)-acrylamide (AMG8562), a novel transient receptor potential vanilloid type 1 modulator that does not cause hyperthermia in rats. J Pharmacol Exp Ther. (2008) 326:218–29. doi: 10.1124/jpet.107.132233
Keywords: TRPV1, appetite regulation, metabolism, obesity, endovanilloid, endocannabinoid
Citation: Christie S, Wittert GA, Li H and Page AJ (2018) Involvement of TRPV1 Channels in Energy Homeostasis. Front. Endocrinol. 9:420. doi: 10.3389/fendo.2018.00420
Received: 30 March 2018; Accepted: 04 July 2018;
Published: 31 July 2018.
Edited by:
Andrew J. McAinch, Victoria University, AustraliaReviewed by:
Denis P. Blondin, Université de Sherbrooke, CanadaThomas Alexander Lutz, Universität Zürich, Switzerland
Copyright © 2018 Christie, Wittert, Li and Page. This is an open-access article distributed under the terms of the Creative Commons Attribution License (CC BY). The use, distribution or reproduction in other forums is permitted, provided the original author(s) and the copyright owner(s) are credited and that the original publication in this journal is cited, in accordance with accepted academic practice. No use, distribution or reproduction is permitted which does not comply with these terms.
*Correspondence: Amanda J. Page, QW1hbmRhLnBhZ2VAYWRlbGFpZGUuZWR1LmF1