- 1Department of Psychology, Psychosomatic Research Group, Universidade Ibirapuera, São Paulo, Brazil
- 2Department of Pharmacy, Psychosomatic Research Group, Universidade Ibirapuera, São Paulo, Brazil
- 3Department of Psychobiology, Escola Paulista de Medicina, Universidade Federal de São Paulo, São Paulo, Brazil
Sleep homeostasis depends on the length and quality (occurrence of stressful events, for instance) of the preceding waking time. Forced wakefulness (sleep deprivation or sleep restriction) is one of the main tools used for the understanding of mechanisms that play a role in homeostatic processes involved in sleep regulation and their interrelations. Interestingly, forced wakefulness for periods longer than 24 h activates stress response systems, whereas stressful events impact on sleep pattern. Hypothalamic peptides (corticotropin-releasing hormone, prolactin, and the CLIP/ACTH18–39) play an important role in the expression of stress-induced sleep effects, essentially by modulating rapid eye movement sleep, which has been claimed to affect the organism resilience to the deleterious effects of stress. Some of the mechanisms involved in the generation and regulation of sleep and the main peptides/hypothalamic hormones involved in these responses will be discussed in this review.
Introduction
The purpose of the present review is not to present a detailed description of the neural basis of sleep generation and maintenance. For that, we refer to a number of previous review papers that cover this subject (1–5).
Sleep is a fundamental behavior for the individual’s survival and is redundantly regulated by the interaction of several neurotransmitter and neuropeptide systems acting on several brain structures, mainly located in the hypothalamus and brain stem [for review, see Ref. (6)]. In humans and rodents, sleep is classified into two main stages: non-rapid eye movement sleep (NREMS) and rapid eye movement sleep (REMS), which electroencephalographic features, in rats, are represented in Figure 1. In humans, NREMS encompasses three stages, N1, N2, and N3, characterized by synchronized, high amplitude, and low frequency cortical waves, whereas in rats, NREMS or slow wave sleep can be distinguished into two substages, differing in the amplitude of these slow waves (low- and high-amplitude waves). REMS or paradoxical sleep (used for rats, since they show very little eye movements) is characterized by desynchronized, high frequency, low-amplitude cortical waves, very similar to wakefulness, and hippocampal theta waves. In addition, muscle atonia is a main tonic feature of this sleep stage (7, 8).
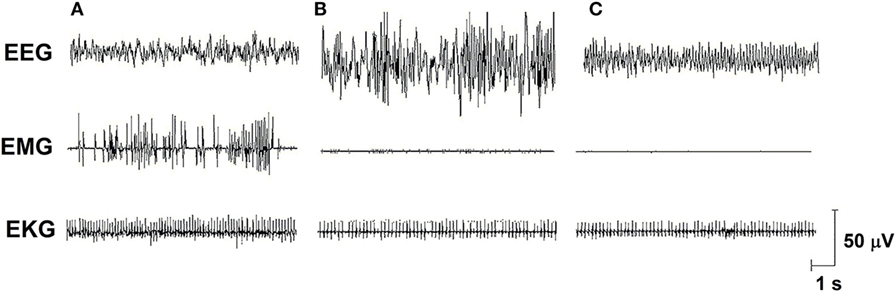
Figure 1. Electro-oscillographic signs of wakefulness and sleep in the Wistar rat. (A) Active wake (with low voltage and fast EEG frequency, concomitant a high EMG activity and EKG is fast), (B) non-rapid eye movement sleep (NREMS—high amplitude and slow wave EEG, activity in EMG is low and EKG is low), (C) rapid eye movement sleep (REMS—theta 6–8 Hz activity is present in this medial EEG, EMG is almost quiet, and EKG shows an intermediate activity). EEG, electroencephalogram (obtained from a medial frontoparietal bipolar deviation); EMG, electromyogram (from the trapezius muscle); EKG, electrocardiogram (from intercostal electrodes). Signs were calibrated with 50-µV pulse. Data from our group.
Sleep Regulation and Homeostasis
Circadian and Homeostatic Mechanisms
Sleep is regulated by a combination of homeostatic and circadian mechanisms. The homeostatic process refers to sleep needs or pressure, and the circadian one, entrainment to the light/dark cycle. Besides the homeostatic factor, the circadian aspect is important and is related to the animal’s expression of daily preference for sleep/rest. Also, the duration of the sleep episodes appears to be greater in animals that are at the top of the food chain, since preys need to monitor the environment constantly to ensure their integrity, thus sleeping very short bouts (9, 10). The interaction between homeostatic (called “S process”) and circadian factors (called “C process”) in sleep regulation led some authors to propose a model in which the two processes would act together. Sleep begins wherever there is a conjunction of larger homeostatic pressure (need for sleep) and greater circadian predisposition (proximity to the phase of the cycle that sleep normally occurs), whereas it ends when this interaction decreases (11, 12).
Sleep homeostasis depends, among many factors, on the length and quality of the preceding waking period. Therefore, longer periods of waking lead to greater compensatory sleep, also known as rebound sleep. Interestingly, brief periods of sleep deprivation (SD) (3–6 h) result only in increased NREMS without affecting REMS (13). A total of 12–24 h of total sleep deprivation increases both NREMS and REMS (14–16), whereas total SD for 96 h induces a very pronounced increase in REMS (17). In 1960, few years after the discovery of REMS, William Dement (18) reported, for the first time in humans, that selective REMS deprivation induces a compensatory increase of this specific phase, e.g., REMS rebound. These effects have been replicated in rats, using the platform method that produces a complete suppression of REMS and some loss of NREMS (19, 20).
Effects of Stress
It is interesting to note that REMS deprivation induces the activation of the hypothalamic–pituitary–adrenal (HPA) axis, with increased production (21, 22) and release (23) of corticotropin-releasing hormone (CRH), and increased ACTH (24, 25) and corticosterone plasma levels (22, 26–28). This constellation of neuroendocrine changes indicates the stressful nature of this manipulation, thereby leading some authors to question whether sleep rebound would be an outcome of stress exposure. It soon became clear that stressful events can alter sleep pattern in a stimulus- and length-related fashion, inasmuch as immobilization stress increases REMS (29), social conflict (30) and exposure to cold (31) induce NREMS, whereas unpredictable footshock stress decreases REM sleep (32, 33). One to two hours of immobilization stress in the beginning of the dark phase, increase REMS, but longer periods of stress blunt the expression of sleep rebound (34), similar to what is seen with repeated immobilization (29). These opposite effects between acute and chronic exposure to the stress seem to be due to an adaptation phenomenon, which is also observed with footshock stress (32, 35). Either increased or reduced stress-induced REMS appear to be related to corticosterone secretion, in an inverted U shape fashion (36, 37).
Hormonal Regulation of Stress-Induced Sleep Rebound
HPA Axis
Abnormal functioning of the HPA axis substantially changes the sleep pattern, as observed in Addison’s disease patients who exhibit adrenal insufficiency and display more NREMS and less REMS (34); this sleep abnormality can be corrected by corticoid replacement therapy (38). Conversely, patients with Cushing’s syndrome, who exhibit exaggerated cortisol secretion, display less NREMS and more awakenings during the night, which can also be corrected by interventions that decrease production of glucocorticoids (GCs) (39, 40). These findings strongly suggest that optimal GC concentrations are essential for normal sleep patterns in humans (41, 42). In rats, a similar phenomenon is also observed, with high levels of corticosterone being especially detrimental to NREMS (43).
The 41-aminoacid peptide CRH, its mRNA, and receptors are increased in stress situations, such as electric footshock (44), immobilization (45), restriction of food (46), and sleep deprivation (21, 23). CRH stimulates the release of other proopiomelonocortin (POMC)-derived peptides, including β-endorphin and alpha-melanocyte-stimulating hormone (α-MSH) (47–49). It differentially stimulates the activity of the prohormone convertases (PC1 and PC2, mainly), which cleave POMC in its bioactive peptides (50, 51).
CRH reduces REM and NREMS and increases awakenings when injected intravenously (52) or after i.c.v. administration (53), whereas CRH type 1 receptor (CRH-R1) antagonists promote sleep (54, 55). CRH effects on sleep homeostatic regulation are also evident, for its administration immediately after sleep deprivation increases REMS rebound (56). Conversely, α-helical-CRH9−41, a CRH-R1 antagonist inhibits REMS rebound induced by immobilization (57). Recently, we have demonstrated that both i.c.v. CRH or α-helical-CRH9−41 administration during REMS deprivation impairs sleep homeostasis, thereby decreasing the length of REMS episodes in the theta band energy (6.0–9.0 Hz), and decreases the time of REM and NREMS in the recovery period (58).
Corticotropin-Like Intermediate Peptide (CLIP or ACTH18–39)
CLIP is a POMC derivative, well known for inducing long REMS episodes (59, 60). ACTH cleavage to CLIP and α-MSH is mediated by prohormone convertase 2 (PC2) and is stimulated by serotonin, via 5-HT2C receptors (61–63). This process occurs in the melanocytes of the pituitary pars intermedia and in two other distinct locations in the brain: the arcuate nucleus—Arc (and peri-arcuate) in the basomedial hypothalamus and in a cell group of the nucleus of the solitary tract (NST) (64, 65). There are also CLIP-containing fibers, originating in the Arc and projecting to the lateral, paraventricular, basal and preoptic hypothalamic regions, dorsal and medial raphe nucleus (DRN and MRN, respectively), and septal area (66–70) (Figure 2).
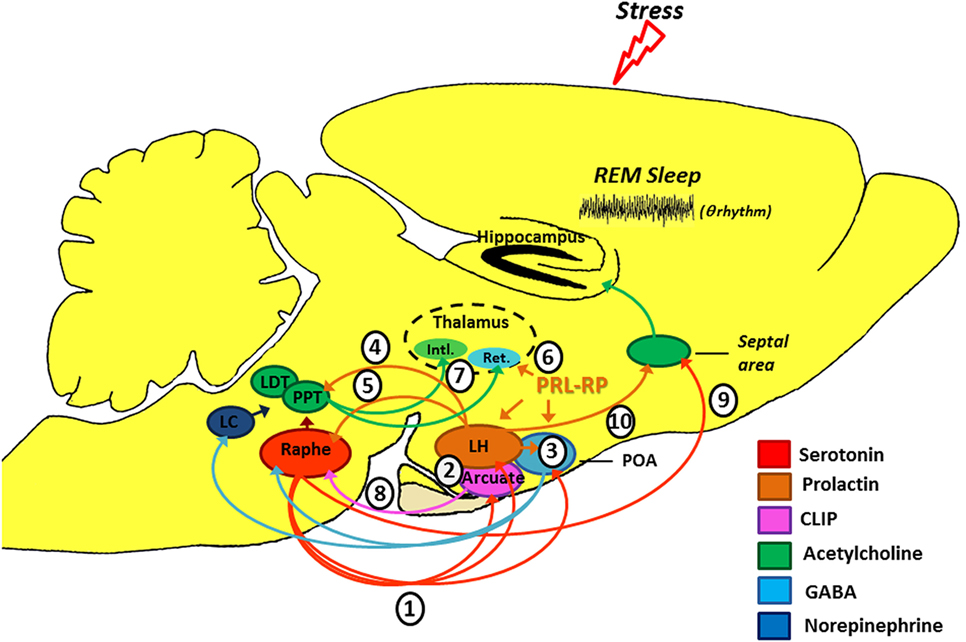
Figure 2. Schematic model of the possible modulation of stress-induced rapid eye movement sleep rebound by PRL, CLIP, and PRL-RP. LC, locus coeruleus; LDT, laterodorsal tegmental nucleus; PPT, pedunculopontine tegmental nucleus; LH, lateral hypothalamic area; POA, preoptic area; Arcuate, arcuate nucleus of hypothalamus; Intl., thalamic intralaminar nuclei; Ret., reticular thalamic nucleus. For more details, please refer to the main text.
Initial studies on the effects of stress-induced REMS rebound show that 1 h of immobilization stress increases REMS 3 h after the end of the stressor, a time point that corresponds to the increased content of phosphorylated CLIP in the Arc (71, 72). Conversely, micro-infusion of CLIP into the DRN increases REMS, similar to that observed after acute immobilization stress (73–75). It has been hypothesized that stress induces 5-HT release from the raphe nuclei, which projections to the Arc lead to CLIP processing from POMC. When the stress finishes, 5-HT is reuptaken, whereas CLIP synthesis and release from the Arc still go on. Interestingly, immunoreactive CLIP fibers are found in the DRN, which are postulated to trigger the dendritic release of serotonin (76) by a process of extracellular diffusion (67). The dendritic release of 5-HT within the DRN inhibits further 5-HT synthesis and release, via 5HT1A autoreceptors (77, 78), a process commonly observed during REMS (79, 80). Confirmation of this mechanism was obtained by electrical stimulation of the DRN leading to release of 5-HT and indol compounds in the Arc, followed, 3 h later, by increased REMS (81) (Figure 2). It is worth mentioning that REMS induced by CLIP, more precisely the amino-terminal fragment ACTH20–24 (Val-Lys-Tyr-Pro), is characterized by extremely long episodes, usually above 7 min. This effect on REMS is not obtained with ACTH (ACTH1–39) or other active fragments, such as ACTH18–24 (60).
Prolactin and Prolactin-Releasing Peptide
Prolactin (PRL) is a polypeptide hormone consisting of 199 amino acid residues (in rats and mice, by 197 amino acid residues), which is synthesized and secreted by the anterior pituitary lactotrophs, under tonic dopaminergic inhibitory control (82, 83); the main function of pituitary PRL is to stimulate lactation in mammals (84). In humans, PRL is secreted mostly in the second half of the night (85), with a very clear circadian rhythm (86).
This hormone exhibits anxiolytic effects (87), inhibits the development of stress-induced gastric ulcers (88), and mitigates hormonal and neuronal responses to various stressors (89, 90). PRL also has an important neurotrophic effect and is involved with neurogenesis in various brain structures and cell cultures (91–96), preventing stress-induced decrease in neurogenesis in an animal model of chronic stress (96). PRL is closely associated with physiological stress response (97–99), and immunoreactive neurons are found in the lateral hypothalamus, Arc, and surrounding areas, thereby innervating other hypothalamic nuclei, amygdala, nucleus accumbens, olfactory cortex, septum, the reticular formation region, parabrachial region, locus coeruleus, periaqueductal gray, and DRN (100), and presenting practically the same distribution of POMC and its derivatives, as evidenced by double labeling studies (101). Interestingly, such structures are particularly involved in the regulation of sleep and stress response (Figure 2).
Numerous evidence indicates that PRL may be another mediator of stress-induced REMS rebound phenomenon. On the one hand, movement restriction (102) and ether vapor exposure (103) increase PRL plasma levels and time in REMS in rodents. On the other hand, PRL infusion in the dorsolateral hypothalamus or in the lateral ventricle increases REMS during the light phase (104, 105). Recently, we also showed that footshock stress applied concomitantly to REMS deprivation produces substantial REMS rebound, by virtue of very long REM episodes. Plasma PRL and hypothalamic serotonin (5-HT) were the likely mediators of the major increase in REMS episode length (37). Serotonin stimulates PRL release (106, 107) and PRL also increases serotonin synthesis (108–111) (Figure 2). In addition, both PRL micro-infusion into the DRN (112) and systemic administration of PRL increases REMS (113), whereas anti-PRL antibody suppresses REMS in rats (114), and this phase of sleep is naturally reduced in mice genetically deficient for PRL (115).
Prolactin-releasing peptide (PRL-RP) acts on a seven-transmembrane G protein-coupled receptor promoting PRL release in vitro and in vivo (116, 117). Central PRL-RP administration increases REMS in rats in parallel to PRL circulating levels (118, 119). Intriguingly, high PRL-RP concentrations also increase NREMS and have no effect on REMS (118, 119). In a somewhat contradictory way, PRL-RP has the ability to reduce the oscillatory activity in sections of the reticular thalamic tissue (120), which plays a fundamental role in the generation of NREMS (121).
Concluding Remarks
Several experimental stressors (1) (122, 123) and SD protocols (124, 125) promote serotonin release from the dorsal raphe (see Figure 2). Ascending serotonergic projections stimulate CLIP and PRL production and release by the Arc and lateral hypothalamic area (LH), respectively (2), which depend on gene transcription and protein synthesis (hence, the rebound happens only a few hours after exposure to the stressful stimulus). Serotonin- and PRL-dependent self-stimulating neural circuits (and probably PrRP of the anterior preoptic area) (3), producing GABAergic inactivation of the DRN and LC on pontine nuclei (pedunculopontine tegmental/laterodorsal tegmental) (126). Furthermore, PRL can also activate the pontine cholinergic neurons directly (4) (127). Prolactinergic projections to DRN (5) (100, 101) induce serotonin release (112) that, at first, can feedback on the system leading to additional production and release of CLIP and PRL. However, 5-HT excess can stimulate self-receptors (5-HT1A) in the DRN, thereby inhibiting its activity (128, 129). All these phenomena contribute positively to REM sleep expression. Additionally, PrRP reduces the oscillatory activity of the reticular thalamic neurons (Ret.) (6) (120), an important structure for the generation of synchronized sleep (121), which also has positive effect on REM sleep. Without the inhibitory influence of the DRN and LC (130, 131), pontine cholinergic nuclei (7) stimulate the thalamic intralaminar nuclei (Intl.), which induces cortical activation during desynchronized sleep and inhibits GABAergic neurons of thalamic reticular nucleus (132–134). We should emphasize that CLIP has inhibitory action (8) on serotonin release by the DRN (71), thereby contributing also to halt the suppressive activity that it has on pontine nuclei. It is noteworthy that there is a serotonergic ascending pathway, from the DRN to the septal area (9) that is responsible for the inhibitory activity in the cholinergic basal forebrain area, essential for hippocampal theta rhythm during REM sleep (135). This region also has a high density of PRL receptors (136) and can also be a potential site of prolactin action. Thus, PRL (10) also contributes to REM sleep expression.
REMS in mammals is involved with various functions, such as brain maturation of neonates (10), maintenance of minimal brain activity during sleep [which would allow a quick wake up and avoid a possible coma during sleep (137)], memory consolidation (138, 139), and maintenance of brain monoaminergic neurotransmitter systems (140–143), among others. Recently, however, studies have brought the attention to the importance of REMS in emotion regulation and the main adaptive function of REMS rebound after stress. This is well exemplified by studies showing that patients with posttraumatic stress disorder (PTSD) display longer latency to REMS, and short REM episodes and sleep fragmentation (144, 145). In a recent work, Mellman and colleagues assessed the sleep of individuals after a traumatic event and found a negative correlation between the duration of REMS episodes and development of PTSD. Furthermore, PTSD patients display reduced EEG high frequency activity during REMS, indicating low cognitive activation during this sleep phase (146), thereby pointing to the need for long and consolidated REMS events to elaborate and integrate traumatic memories in conscious level (147). Therefore, we do not hesitate to propose, for reasons of evolutionary logic, that in rodents, increased REMS observed after some kinds of stress has a similar role (148).
Author Contributions
RM and DS contributed equally to this work.
Conflict of Interest Statement
The authors declare that the research was conducted in the absence of any commercial or financial relationships that could be construed as a potential conflict of interest.
Funding
This work was supported by grants from FAPESP (CEPID program #98/14303-3) and Conselho Nacional de Desenvolvimento Cientifico e Tecnológico (CNPq #486769/2013-5). RM was the recipient of research fellowship from Fundação de Amparo à Pesquisa do Estado de São Paulo (FAPESP #2010/09087-3), and DS is the recipient of a research fellowship from CNPq (#302294/2012-0). Payment of this publication was possible by FAPESP grant # 2015/26364-4.
References
1. McGinty D, Gong H, Suntsova N, Alam MN, Methippara M, Guzman-Marin R, et al. Sleep-promoting functions of the hypothalamic median preoptic nucleus: inhibition of arousal systems. Arch Ital Biol (2004) 142(4):501–9.
2. Monti JM. The role of dorsal raphe nucleus serotonergic and non-serotonergic neurons, and of their receptors, in regulating waking and rapid eye movement (REM) sleep. Sleep Med Rev (2010) 14(5):319–27. doi:10.1016/j.smrv.2009.10.003
3. Sinton CM, McCarley RW. Neuroanatomical and neurophysiological aspects of sleep: basic science and clinical relevance. Semin Clin Neuropsychiatry (2000) 5(1):6–19.
4. Jones BE. The neural basis of consciousness across the sleep-waking cycle. Adv Neurol (1998) 77:75–94.
5. Fuller PM, Saper CB, Lu J. The pontine REM switch: past and present. J Physiol (2007) 584(Pt 3):735–41. doi:10.1113/jphysiol.2007.140160
6. Saper CB, Chou TC, Scammell TE. The sleep switch: hypothalamic control of sleep and wakefulness. Trends Neurosci (2001) 24(12):726–31. doi:10.1016/S0166-2236(00)02002-6
7. Iber CAmerican Academy of Sleep Medicine. The AASM Manual for the Scoring of Sleep and Associated Events: Rules, Terminology and Technical Specifications. Westchester, IL: American Academy of Sleep Medicine (2007).
8. Timo-Iaria C, Negrao N, Schmidek WR, Hoshino K, Lobato de Menezes CE, Leme da Rocha T. Phases and states of sleep in the rat. Physiol Behav (1970) 5(9):1057–62. doi:10.1016/0031-9384(70)90162-9
9. Capellini I, Barton RA, McNamara P, Preston BT, Nunn CL. Phylogenetic analysis of the ecology and evolution of mammalian sleep. Evolution (2008) 62(7):1764–76. doi:10.1111/j.1558-5646.2008.00392.x
10. Zepelin H, Siegel JM, Tobler I. Mammalian sleep. 4 ed. In: Kryger MH, Roth T, Dement WC, editors. Principles and Practice of Sleep Medicine. Philadelphia: Elsevier Saunders (2005). p. 91–100.
11. Borbely AA. Refining sleep homeostasis in the two-process model. J Sleep Res (2009) 18(1):1–2. doi:10.1111/j.1365-2869.2009.00750.x
12. Achermann P. The two-process model of sleep regulation revisited. Aviat Space Environ Med (2004) 75(3 Suppl):A37–43.
13. Tobler I, Borbely AA. The effect of 3-h and 6-h sleep deprivation on sleep and EEG spectra of the rat. Behav Brain Res (1990) 36(1–2):73–8. doi:10.1016/0166-4328(90)90161-7
14. Tobler I, Borbely AA. Sleep EEG in the rat as a function of prior waking. Electroencephalogr Clin Neurophysiol (1986) 64(1):74–6. doi:10.1016/0013-4694(86)90044-1
15. Franken P, Dijk DJ, Tobler I, Borbely AA. Sleep deprivation in rats: effects on EEG power spectra, vigilance states, and cortical temperature. Am J Physiol (1991) 261(1 Pt 2):R198–208.
16. Schwierin B, Borbely AA, Tobler I. Prolonged effects of 24-h total sleep deprivation on sleep and sleep EEG in the rat. Neurosci Lett (1999) 261(1–2):61–4. doi:10.1016/S0304-3940(98)01006-4
17. Rechtschaffen A, Bergmann BM, Gilliland MA, Bauer K. Effects of method, duration, and sleep stage on rebounds from sleep deprivation in the rat. Sleep (1999) 22(1):11–31.
18. Dement W. The effect of dream deprivation. Science (1960) 131:1705–7. doi:10.1126/science.131.3415.1705
19. Grahnstedt S, Ursin R. Platform sleep deprivation affects deep slow wave sleep in addition to REM sleep. Behav Brain Res (1985) 18(3):233–9. doi:10.1016/0166-4328(85)90031-2
20. Machado RB, Hipolide DC, Benedito-Silva AA, Tufik S. Sleep deprivation induced by the modified multiple platform technique: quantification of sleep loss and recovery. Brain Res (2004) 1004(1–2):45–51. doi:10.1016/j.brainres.2004.01.019
21. Koban M, Le WW, Hoffman GE. Changes in hypothalamic corticotropin-releasing hormone, neuropeptide Y, and proopiomelanocortin gene expression during chronic rapid eye movement sleep deprivation of rats. Endocrinology (2006) 147(1):421–31. doi:10.1210/en.2005-0695
22. Galvao MD, Sinigaglia-Coimbra R, Kawakami SE, Tufik S, Suchecki D. Paradoxical sleep deprivation activates hypothalamic nuclei that regulate food intake and stress response. Psychoneuroendocrinology (2009) 34(8):1176–83. doi:10.1016/j.psyneuen.2009.03.003
23. Fadda P, Fratta W. Stress-induced sleep deprivation modifies corticotropin releasing factor (CRF) levels and CRF binding in rat brain and pituitary. Pharmacol Res (1997) 35(5):443–6. doi:10.1006/phrs.1997.0155
24. Suchecki D, Tufik S. Social stability attenuates the stress in the modified multiple platform method for paradoxical sleep deprivation in the rat. Physiol Behav (2000) 68(3):309–16. doi:10.1016/S0031-9384(99)00181-X
25. Suchecki D, Lobo LL, Hipolide DC, Tufik S. Increased ACTH and corticosterone secretion induced by different methods of paradoxical sleep deprivation. J Sleep Res (1998) 7(4):276–81. doi:10.1046/j.1365-2869.1998.00122.x
26. Porkka-Heiskanen T, Smith SE, Taira T, Urban JH, Levine JE, Turek FW, et al. Noradrenergic activity in rat brain during rapid eye movement sleep deprivation and rebound sleep. Am J Physiol (1995) 268(6 Pt 2):R1456–63.
27. Palma BD, Suchecki D, Catallani B, Tufik S. Effect of sleep deprivation on the corticosterone secretion in an experimental model of autoimmune disease. Neuroimmunomodulation (2007) 14(2):72–7. doi:10.1159/000107421
28. Perry JC, D’Almeida V, Antunes IB, Tufik S. Distinct behavioral and neurochemical alterations induced by intermittent hypoxia or paradoxical sleep deprivation in rats. Prog Neuropsychopharmacol Biol Psychiatry (2008) 32(1):87–94. doi:10.1016/j.pnpbp.2007.07.017
29. Rampin C, Cespuglio R, Chastrette N, Jouvet M. Immobilisation stress induces a paradoxical sleep rebound in rat. Neurosci Lett (1991) 126(2):113–8. doi:10.1016/0304-3940(91)90532-X
30. Meerlo P, Pragt BJ, Daan S. Social stress induces high intensity sleep in rats. Neurosci Lett (1997) 225(1):41–4. doi:10.1016/S0304-3940(97)00180-8
31. Palma BD, Suchecki D, Tufik S. Differential effects of acute cold and footshock on the sleep of rats. Brain Res (2000) 861(1):97–104. doi:10.1016/S0006-8993(00)02024-2
32. Adrien J, Dugovic C, Martin P. Sleep-wakefulness patterns in the helpless rat. Physiol Behav (1991) 49(2):257–62. doi:10.1016/0031-9384(91)90041-L
33. Sanford LD, Yang L, Wellman LL, Liu X, Tang X. Differential effects of controllable and uncontrollable footshock stress on sleep in mice. Sleep (2010) 33(5):621–30.
34. Gillin JC, Jacobs LS, Snyder F, Henkin RI. Effects of decreased adrenal corticosteroids: changes in sleep in normal subjects and patients with adrenal cortical insufficiency. Electroencephalogr Clin Neurophysiol (1974) 36(3):283–9. doi:10.1016/0013-4694(74)90170-9
35. Kant GJ, Pastel RH, Bauman RA, Meininger GR, Maughan KR, Robinson TN III, et al. Effects of chronic stress on sleep in rats. Physiol Behav (1995) 57(2):359–65. doi:10.1016/0031-9384(94)00241-V
36. Marinesco S, Bonnet C, Cespuglio R. Influence of stress duration on the sleep rebound induced by immobilization in the rat: a possible role for corticosterone. Neuroscience (1999) 92(3):921–33. doi:10.1016/S0306-4522(99)00045-7
37. Machado RB, Tufik S, Suchecki D. Chronic stress during paradoxical sleep deprivation increases paradoxical sleep rebound: association with prolactin plasma levels and brain serotonin content. Psychoneuroendocrinology (2008) 33(9):1211–24. doi:10.1016/j.psyneuen.2008.06.007
38. Garcia-Borreguero D, Wehr TA, Larrosa O, Granizo JJ, Hardwick D, Chrousos GP, et al. Glucocorticoid replacement is permissive for rapid eye movement sleep and sleep consolidation in patients with adrenal insufficiency. J Clin Endocrinol Metab (2000) 85(11):4201–6. doi:10.1210/jc.85.11.4201
39. Krieger DT, Glick SM. Sleep EEG stages and plasma growth hormone concentration in states of endogenous and exogenous hypercortisolemia or ACTH elevation. J Clin Endocrinol Metab (1974) 39(6):986–1000. doi:10.1210/jcem-39-6-986
40. Shipley JE, Schteingart DE, Tandon R, Starkman MN. Sleep architecture and sleep apnea in patients with Cushing’s disease. Sleep (1992) 15(6):514–8.
41. Anjos KF, Boery RNSO, Pereira R. Quality of life of relative caregivers of elderly dependents at home. Texto & Contexto – Enfermagem (2014) 23:600–8. doi:10.1590/0104-07072014002230013
42. Franza F, Del Buono G, Pellegrino F. Psychiatric caregiver stress: clinical implications of compassion fatigue. Psychiatr Danub (2015) 27(Suppl 1):S321–7.
43. Vazquez-Palacios G, Retana-Marquez S, Bonilla-Jaime H, Velazquez-Moctezuma J. Further definition of the effect of corticosterone on the sleep-wake pattern in the male rat. Pharmacol Biochem Behav (2001) 70(2–3):305–10. doi:10.1016/S0091-3057(01)00620-7
44. Dunn AJ, Berridge CW. Physiological and behavioral responses to corticotropin-releasing factor administration: is CRF a mediator of anxiety or stress responses? Brain Res Brain Res Rev (1990) 15(2):71–100. doi:10.1016/0165-0173(90)90012-D
45. Bonaz B, Rivest S. Effect of a chronic stress on CRF neuronal activity and expression of its type 1 receptor in the rat brain. Am J Physiol (1998) 275(5 Pt 2):R1438–49.
46. Suemaru S, Hashimoto K, Hattori T, Inoue H, Kageyama J, Ota Z. Starvation-induced changes in rat brain corticotropin-releasing factor (CRF) and pituitary-adrenocortical response. Life Sci (1986) 39(13):1161–6. doi:10.1016/0024-3205(86)90347-4
47. Henryk S, Josko J, Jedrzejowska-Szypulka H, Jarzab B, Dohler KD. Corticotropin releasing hormone (CRH) increases beta-endorphin (beta-end like) concentration in cerebrospinal fluid of rats with vasospasm following subarachnoid hemorrhage. J Physiol Pharmacol (1999) 50(3):419–28.
48. Conte-Devolx B, Rey M, Boudouresque F, Giraud P, Castanas E, Millet Y, et al. Effect of 41-CRF antiserum on the secretion of ACTH, B-endorphin and alpha-MSH in the rat. Peptides (1983) 4(3):301–4.
49. Meunier H, Lefevre G, Dumont D, Labrie F. CRF stimulates alpha-MSH secretion and cyclic AMP accumulation in rat pars intermedia cells. Life Sci (1982) 31(19):2129–35. doi:10.1016/0024-3205(82)90105-9
50. Bloomquist BT, Eipper BA, Mains RE. Prohormone-converting enzymes: regulation and evaluation of function using antisense RNA. Mol Endocrinol (1991) 5(12):2014–24. doi:10.1210/mend-5-12-2014
51. Dong W, Seidel B, Marcinkiewicz M, Chretien M, Seidah NG, Day R. Cellular localization of the prohormone convertases in the hypothalamic paraventricular and supraoptic nuclei: selective regulation of PC1 in corticotrophin-releasing hormone parvocellular neurons mediated by glucocorticoids. J Neurosci (1997) 17(2):563–75.
52. Tsuchiyama Y, Uchimura N, Sakamoto T, Maeda H, Kotorii T. Effects of hCRH on sleep and body temperature rhythms. Psychiatry Clin Neurosci (1995) 49(5–6):299–304. doi:10.1111/j.1440-1819.1995.tb01906.x
53. Ehlers CL, Reed TK, Henriksen SJ. Effects of corticotropin-releasing factor and growth hormone-releasing factor on sleep and activity in rats. Neuroendocrinology (1986) 42(6):467–74. doi:10.1159/000124489
54. Chang FC, Opp MR. Blockade of corticotropin-releasing hormone receptors reduces spontaneous waking in the rat. Am J Physiol (1998) 275(3 Pt 2):R793–802.
55. Chang FC, Opp MR. Pituitary CRH receptor blockade reduces waking in the rat. Physiol Behav (1999) 67(5):691–6. doi:10.1016/S0031-9384(99)00139-0
56. Marrosu F, Gessa GL, Giagheddu M, Fratta W. Corticotropin-releasing factor (CRF) increases paradoxical sleep (PS) rebound in PS-deprived rats. Brain Res (1990) 515(1–2):315–8. doi:10.1016/0006-8993(90)90614-H
57. Gonzalez MM, Valatx JL. Effect of intracerebroventricular administration of alpha-helical CRH (9-41) on the sleep/waking cycle in rats under normal conditions or after subjection to an acute stressful stimulus. J Sleep Res (1997) 6(3):164–70. doi:10.1046/j.1365-2869.1997.00042.x
58. Machado RB, Tufik S, Suchecki D. Modulation of sleep homeostasis by corticotropin releasing hormone in REM sleep-deprived rats. Int J Endocrinol (2010) 2010:326151. doi:10.1155/2010/326151
59. Wetzel W, Balschun D, Janke S, Vogel D, Wagner T. Effects of CLIP (corticotropin-like intermediate lobe peptide) and CLIP fragments on paradoxical sleep in rats. Peptides (1994) 15(2):237–41. doi:10.1016/0196-9781(94)90008-6
60. Wetzel W, Wagner T, Vogel D, Demuth HU, Balschun D. Effects of the CLIP fragment ACTH 20-24 on the duration of REM sleep episodes. Neuropeptides (1997) 31(1):41–5. doi:10.1016/S0143-4179(97)90018-4
61. Takahashi A, Mizusawa K. Posttranslational modifications of proopiomelanocortin in vertebrates and their biological significance. Front Endocrinol (2013) 4:143.
62. Xu Y, Jones JE, Kohno D, Williams KW, Lee CE, Choi MJ, et al. 5-HT2CRs expressed by pro-opiomelanocortin neurons regulate energy homeostasis. Neuron (2008) 60(4):582–9. doi:10.1016/j.neuron.2008.09.033
63. Tanaka S. Comparative aspects of intracellular proteolytic processing of peptide hormone precursors: studies of proopiomelanocortin processing. Zoolog Sci (2003) 20(10):1183–98. doi:10.2108/zsj.20.1183
64. Emson PC, Corder R, Ratter SJ, Tomlin S, Lowry PJ, Ress LH, et al. Regional distribution of pro-opiomelanocortin-derived peptides in the human brain. Neuroendocrinology (1984) 38(1):45–50. doi:10.1159/000123864
65. Leger L, Lema F, Chastrette N, Charnay Y, Cespuglio R, Mazie JC, et al. A monoclonal antibody directed against CLIP (ACTH 18-39). Anatomical distribution of immunoreactivity in the rat brain and hypophysis with quantification of the hypothalamic cell group. J Chem Neuroanat (1990) 3(4):297–308.
66. Zaphiropoulos A, Charnay Y, Vallet P, Constantinidis J, Bouras C. Immunohistochemical distribution of corticotropin-like intermediate lobe peptide (CLIP) immunoreactivity in the human brain. Brain Res Bull (1991) 26(1):99–111. doi:10.1016/0361-9230(91)90194-O
67. Zheng Z, Leger L, Cespuglio R, Jouvet M. Distribution of the pro-opiomelanocortin-immunoreactive axons in relation to the serotoninergic neurons in the dorsal raphe nucleus of the rat. Neurosci Lett (1991) 130(1):17–21. doi:10.1016/0304-3940(91)90217-H
68. Covenas R, de Leon M, Narvaez JA, Aguirre JA, Tramu G, Gonzalez-Baron S. ACTH/CLIP immunoreactivity in the cat brain stem. Peptides (1997) 18(7):965–70. doi:10.1016/S0196-9781(97)00048-X
69. Leger L, Bonnet C, Cespuglio R, Jouvet M. Immunocytochemical study of the CLIP/ACTH-immunoreactive nerve fibres in the dorsal raphe nucleus of the rat. Neurosci Lett (1994) 174(2):137–40. doi:10.1016/0304-3940(94)90005-1
70. Leger L, Zheng Z, Bonnet C, Cespuglio R. Ultrastructural relationships of the pro-opiomelanocortin axons with the serotoninergic neurons in the dorsal raphe nucleus of the rat. Neurosci Lett (1997) 222(3):155–8. doi:10.1016/S0304-3940(97)13363-8
71. Bonnet C, Leger L, Baubet V, Debilly G, Cespuglio R. Influence of a 1 h immobilization stress on sleep states and corticotropin-like intermediate lobe peptide (CLIP or ACTH18-39, Ph-ACTH18-39) brain contents in the rat. Brain Res (1997) 751(1):54–63. doi:10.1016/S0006-8993(96)01390-X
72. Bonnet C, Marinesco S, Debilly G, Kovalzon V, Cespuglio R. Influence of a 1-h immobilization stress on sleep and CLIP (ACTH(18-39)) brain contents in adrenalectomized rats. Brain Res (2000) 853(2):323–9. doi:10.1016/S0006-8993(99)02313-6
73. Chastrette N, Cespuglio R, Jouvet M. Proopiomelanocortin (POMC)-derived peptides and sleep in the rat. Part 1 – hypnogenic properties of ACTH derivatives. Neuropeptides (1990) 15(2):61–74. doi:10.1016/0143-4179(90)90043-X
74. el Kafi B, Cespuglio R, Leger L, Marinesco S, Jouvet M. Is the nucleus raphe dorsalis a target for the peptides possessing hypnogenic properties? Brain Res (1994) 637(1–2):211–21. doi:10.1016/0006-8993(94)91235-1
75. el Kafi B, Leger L, Seguin S, Jouvet M, Cespuglio R. Sleep permissive components within the dorsal raphe nucleus in the rat. Brain Res (1995) 686(2):150–9. doi:10.1016/0006-8993(95)00390-C
76. Cespuglio R, Marinesco S, Baubet V, Bonnet C, el Kafi B. Evidence for a sleep-promoting influence of stress. Adv Neuroimmunol (1995) 5(2):145–54. doi:10.1016/0960-5428(95)00005-M
77. Pan ZZ, Wessendorf MW, Williams JT. Modulation by serotonin of the neurons in rat nucleus raphe magnus in vitro. Neuroscience (1993) 54(2):421–9. doi:10.1016/0306-4522(93)90263-F
78. McDevitt RA, Neumaier JF. Regulation of dorsal raphe nucleus function by serotonin autoreceptors: a behavioral perspective. J Chem Neuroanat (2011) 41(4):234–46. doi:10.1016/j.jchemneu.2011.05.001
79. McGinty DJ, Harper RM. Dorsal raphe neurons: depression of firing during sleep in cats. Brain Res (1976) 101(3):569–75. doi:10.1016/0006-8993(76)90480-7
80. Monti JM, Monti D. Role of dorsal raphe nucleus serotonin 5-HT1A receptor in the regulation of REM sleep. Life Sci (2000) 66(21):1999–2012. doi:10.1016/S0024-3205(99)00649-9
81. Houdouin F, Cespuglio R, Jouvet M. Effects induced by the electrical stimulation of the nucleus raphe dorsalis upon hypothalamic release of 5-hydroxyindole compounds and sleep parameters in the rat. Brain Res (1991) 565(1):48–56. doi:10.1016/0006-8993(91)91735-J
82. MacLeod RM, Fontham EH, Lehmeyer JE. Prolactin and growth hormone production as influenced by catecholamines and agents that affect brain catecholamines. Neuroendocrinology (1970) 6(5):283–94. doi:10.1159/000121933
83. Birge CA, Jacobs LS, Hammer CT, Daughaday WH. Catecholamine inhibition of prolactin secretion by isolated rat adenohypophyses. Endocrinology (1970) 86(1):120–30. doi:10.1210/endo-86-1-120
84. Riddle O, Bates RW, Dykshorn SW. The preparation, identification and assay of prolactin: a hormone of anterior pituitary. Am J Physiol (1933) 105:15.
85. Sassin JF, Frantz AG, Weitzman ED, Kapen S. Human prolactin: 24-hour pattern with increased release during sleep. Science (1972) 177(55):1205–7. doi:10.1126/science.177.4055.1205
86. Van Cauter E. Diurnal and ultradian rhythms in human endocrine function: a minireview. Horm Res (1990) 34(2):45–53. doi:10.1159/000181794
87. Torner L, Toschi N, Pohlinger A, Landgraf R, Neumann ID. Anxiolytic and anti-stress effects of brain prolactin: improved efficacy of antisense targeting of the prolactin receptor by molecular modeling. J Neurosci (2001) 21(9):3207–14.
88. Fujikawa T, Soya H, Tamashiro KL, Sakai RR, McEwen BS, Nakai N, et al. Prolactin prevents acute stress-induced hypocalcemia and ulcerogenesis by acting in the brain of rat. Endocrinology (2004) 145(4):2006–13. doi:10.1210/en.2003-1446
89. Donner N, Bredewold R, Maloumby R, Neumann ID. Chronic intracerebral prolactin attenuates neuronal stress circuitries in virgin rats. Eur J Neurosci (2007) 25(6):1804–14. doi:10.1111/j.1460-9568.2007.05416.x
90. Torner L, Toschi N, Nava G, Clapp C, Neumann ID. Increased hypothalamic expression of prolactin in lactation: involvement in behavioural and neuroendocrine stress responses. Eur J Neurosci (2002) 15(8):1381–9. doi:10.1046/j.1460-9568.2002.01965.x
91. DeVito WJ, Okulicz WC, Stone S, Avakian C. Prolactin-stimulated mitogenesis of cultured astrocytes. Endocrinology (1992) 130(5):2549–56. doi:10.1210/en.130.5.2549
92. Gregg C, Shikar V, Larsen P, Mak G, Chojnacki A, Yong VW, et al. White matter plasticity and enhanced remyelination in the maternal CNS. J Neurosci (2007) 27(8):1812–23. doi:10.1523/JNEUROSCI.4441-06.2007
93. Mangoura D, Pelletiere C, Leung S, Sakellaridis N, Wang DX. Prolactin concurrently activates src-PLD and JAK/Stat signaling pathways to induce proliferation while promoting differentiation in embryonic astrocytes. Int J Dev Neurosci (2000) 18(7):693–704. doi:10.1016/S0736-5748(00)00031-9
94. Mohammad YN, Perone M, Wang L, Ingleton PM, Castro MG, Lovejoy DA. Expression of prolactin receptors and regulation of cell proliferation by prolactin, corticotropin-releasing factor, and corticosterone in a neuroblastoma cell line. Biochem Cell Biol (2002) 80(4):475–82. doi:10.1139/o02-036
95. Shingo T, Gregg C, Enwere E, Fujikawa H, Hassam R, Geary C, et al. Pregnancy-stimulated neurogenesis in the adult female forebrain mediated by prolactin. Science (2003) 299(5603):117–20. doi:10.1126/science.1076647
96. Torner L, Karg S, Blume A, Kandasamy M, Kuhn HG, Winkler J, et al. Prolactin prevents chronic stress-induced decrease of adult hippocampal neurogenesis and promotes neuronal fate. J Neurosci (2009) 29(6):1826–33. doi:10.1523/JNEUROSCI.3178-08.2009
97. Dijkstra I, Tilders FJ, Aguilera G, Kiss A, Rabadan-Diehl C, Barden N, et al. Reduced activity of hypothalamic corticotropin-releasing hormone neurons in transgenic mice with impaired glucocorticoid receptor function. J Neurosci (1998) 18(10):3909–18.
98. Faron-Gorecka A, Kusmider M, Kolasa M, Zurawek D, Gruca P, Papp M, et al. Prolactin and its receptors in the chronic mild stress rat model of depression. Brain Res (2014) 1555:48–59. doi:10.1016/j.brainres.2014.01.031
99. Lennartsson AK, Jonsdottir IH. Prolactin in response to acute psychosocial stress in healthy men and women. Psychoneuroendocrinology (2011) 36(10):1530–9. doi:10.1016/j.psyneuen.2011.04.007
100. Paut-Pagano L, Roky R, Valatx JL, Kitahama K, Jouvet M. Anatomical distribution of prolactin-like immunoreactivity in the rat brain. Neuroendocrinology (1993) 58(6):682–95. doi:10.1159/000126609
101. Harlan RE, Shivers BD, Fox SR, Kaplove KA, Schachter BS, Pfaff DW. Distribution and partial characterization of immunoreactive prolactin in the rat brain. Neuroendocrinology (1989) 49(1):7–22. doi:10.1159/000125085
102. Meerlo P, Easton A, Bergmann BM, Turek FW. Restraint increases prolactin and REM sleep in C57BL/6J mice but not in BALB/cJ mice. Am J Physiol Regul Integr Comp Physiol (2001) 281(3):R846–54.
103. Bodosi B, Obal F Jr, Gardi J, Komlodi J, Fang J, Krueger JM. An ether stressor increases REM sleep in rats: possible role of prolactin. Am J Physiol Regul Integr Comp Physiol (2000) 279(5):R1590–8.
104. Roky R, Valatx JL, Jouvet M. Effect of prolactin on the sleep-wake cycle in the rat. Neurosci Lett (1993) 156(1–2):117–20. doi:10.1016/0304-3940(93)90453-R
105. Roky R, Valatx JL, Paut-Pagano L, Jouvet M. Hypothalamic injection of prolactin or its antibody alters the rat sleep-wake cycle. Physiol Behav (1994) 55(6):1015–9. doi:10.1016/0031-9384(94)90382-4
106. Freeman ME, Kanyicska B, Lerant A, Nagy G. Prolactin: structure, function, and regulation of secretion. Physiol Rev (2000) 80(4):1523–631.
107. Balsa JA, Sanchez-Franco F, Pazos F, Lara JI, Lorenzo MJ, Maldonado G, et al. Direct action of serotonin on prolactin, growth hormone, corticotropin and luteinizing hormone release in cocultures of anterior and posterior pituitary lobes: autocrine and/or paracrine action of vasoactive intestinal peptide. Neuroendocrinology (1998) 68(5):326–33. doi:10.1159/000054381
108. Bole-Feysot C, Goffin V, Edery M, Binart N, Kelly PA. Prolactin (PRL) and its receptor: actions, signal transduction pathways and phenotypes observed in PRL receptor knockout mice. Endocr Rev (1998) 19(3):225–68. doi:10.1210/edrv.19.3.0334
109. Brown RS, Herbison AE, Grattan DR. Differential changes in responses of hypothalamic and brainstem neuronal populations to prolactin during lactation in the mouse. Biol Reprod (2011) 84(4):826–36. doi:10.1095/biolreprod.110.089185
110. Sakowski SA, Geddes TJ, Thomas DM, Levi E, Hatfield JS, Kuhn DM. Differential tissue distribution of tryptophan hydroxylase isoforms 1 and 2 as revealed with monospecific antibodies. Brain Res (2006) 1085(1):11–8. doi:10.1016/j.brainres.2006.02.047
111. Schraenen A, Lemaire K, de Faudeur G, Hendrickx N, Granvik M, Van Lommel L, et al. Placental lactogens induce serotonin biosynthesis in a subset of mouse beta cells during pregnancy. Diabetologia (2010) 53(12):2589–99. doi:10.1007/s00125-010-1913-7
112. Machado RB, Rocha MR, Suchecki D. Brain prolactin is involved in stress-induced REM sleep rebound. Horm Behav (in press).
113. Roky R, Obal F Jr, Valatx JL, Bredow S, Fang J, Pagano LP, et al. Prolactin and rapid eye movement sleep regulation. Sleep (1995) 18(7):536–42.
114. Obal F Jr, Kacsoh B, Alfoldi P, Payne L, Markovic O, Grosvenor C, et al. Antiserum to prolactin decreases rapid eye movement sleep (REM sleep) in the male rat. Physiol Behav (1992) 52(6):1063–8. doi:10.1016/0031-9384(92)90460-J
115. Obal F Jr, Garcia-Garcia F, Kacsoh B, Taishi P, Bohnet S, Horseman ND, et al. Rapid eye movement sleep is reduced in prolactin-deficient mice. J Neurosci (2005) 25(44):10282–9. doi:10.1523/JNEUROSCI.2572-05.2005
116. Hinuma S, Habata Y, Fujii R, Kawamata Y, Hosoya M, Fukusumi S, et al. A prolactin-releasing peptide in the brain. Nature (1998) 393(6682):272–6. doi:10.1038/30515
117. Matsumoto H, Noguchi J, Horikoshi Y, Kawamata Y, Kitada C, Hinuma S, et al. Stimulation of prolactin release by prolactin-releasing peptide in rats. Biochem Biophys Res Commun (1999) 259(2):321–4. doi:10.1006/bbrc.1999.0789
118. Zhang SQ, Inoue S, Kimura M. Sleep-promoting activity of prolactin-releasing peptide (PrRP) in the rat. Neuroreport (2001) 12(15):3173–6. doi:10.1097/00001756-200110290-00006
119. Zhang SQ, Kimura M, Inoue S. Effects of prolactin-releasing peptide (PrRP) on sleep regulation in rats. Psychiatry Clin Neurosci (2000) 54(3):262–4. doi:10.1046/j.1440-1819.2000.00670.x
120. Lin SH, Arai AC, Espana RA, Berridge CW, Leslie FM, Huguenard JR, et al. Prolactin-releasing peptide (PrRP) promotes awakening and suppresses absence seizures. Neuroscience (2002) 114(1):229–38. doi:10.1016/S0306-4522(02)00248-8
121. Llinas RR, Steriade M. Bursting of thalamic neurons and states of vigilance. J Neurophysiol (2006) 95(6):3297–308. doi:10.1152/jn.00166.2006
122. Briones-Aranda A, Rocha L, Picazo O. Influence of forced swimming stress on 5-HT1A receptors and serotonin levels in mouse brain. Prog Neuropsychopharmacol Biol Psychiatry (2005) 29(2):275–81. doi:10.1016/j.pnpbp.2004.11.011
123. Adell A, Casanovas JM, Artigas F. Comparative study in the rat of the actions of different types of stress on the release of 5-HT in raphe nuclei and forebrain areas. Neuropharmacology (1997) 36(4–5):735–41. doi:10.1016/S0028-3908(97)00048-8
124. Senthilvelan M, Ravindran R, Samson J, Devi RS. Serotonin turnover in discrete regions of young rat brain after 24 h REM sleep deprivation. Neurochem Res (2006) 31(1):81–4. doi:10.1007/s11064-005-9139-7
125. Zant JC, Leenaars CH, Kostin A, Van Someren EJ, Porkka-Heiskanen T. Increases in extracellular serotonin and dopamine metabolite levels in the basal forebrain during sleep deprivation. Brain Res (2011) 1399:40–8. doi:10.1016/j.brainres.2011.05.008
127. Takahashi K, Koyama Y, Kayama Y, Yamamoto M. The effects of prolactin on the mesopontine tegmental neurons. Psychiatry Clin Neurosci (2000) 54(3):257–8. doi:10.1046/j.1440-1819.2000.00668.x
128. Hajos M, Hajos-Korcsok E, Sharp T. Role of the medial prefrontal cortex in 5-HT1A receptor-induced inhibition of 5-HT neuronal activity in the rat. Br J Pharmacol (1999) 126(8):1741–50. doi:10.1038/sj.bjp.0702510
129. Sprouse JS, Aghajanian GK. Electrophysiological responses of serotoninergic dorsal raphe neurons to 5-HT1A and 5-HT1B agonists. Synapse (1987) 1(1):3–9. doi:10.1002/syn.890010103
130. Leonard CS, Llinas R. Serotonergic and cholinergic inhibition of mesopontine cholinergic neurons controlling REM sleep: an in vitro electrophysiological study. Neuroscience (1994) 59(2):309–30. doi:10.1016/0306-4522(94)90599-1
131. Aston-Jones G, Bloom FE. Activity of norepinephrine-containing locus coeruleus neurons in behaving rats anticipates fluctuations in the sleep-waking cycle. J Neurosci (1981) 1(8):876–86.
132. McCarley RW, Hobson JA. Neuronal excitability modulation over the sleep cycle: a structural and mathematical model. Science (1975) 189(4196):58–60. doi:10.1126/science.1135627
133. Sakai K, el Mansari M, Jouvet M. Inhibition by carbachol microinjections of presumptive cholinergic PGO-on neurons in freely moving cats. Brain Res (1990) 527(2):213–23.
134. Jones BE. The organization of central cholinergic systems and their functional importance in sleep-waking states. Prog Brain Res (1993) 98:61–71. doi:10.1016/S0079-6123(08)62381-X
135. Vertes RP, Kocsis B. Brainstem-diencephalo-septohippocampal systems controlling the theta rhythm of the hippocampus. Neuroscience (1997) 81(4):893–926.
136. Roky R, Paut-Pagano L, Goffin V, Kitahama K, Valatx JL, Kelly PA, et al. Distribution of prolactin receptors in the rat forebrain. Immunohistochemical study. Neuroendocrinology (1996) 63(5):422–9. doi:10.1159/000127067
137. Vertes RP. A life-sustaining function for REM sleep: a theory. Neurosci Biobehav Rev (1986) 10(4):371–6. doi:10.1016/0149-7634(86)90002-3
138. Stickgold R, Hobson JA, Fosse R, Fosse M. Sleep, learning, and dreams: off-line memory reprocessing. Science (2001) 294(5544):1052–7. doi:10.1126/science.1063530
139. Smith C. Sleep states, memory processes and synaptic plasticity. Behav Brain Res (1996) 78(1):49–56. doi:10.1016/0166-4328(95)00218-9
140. Siegel JM, Rogawski MA. A function for REM sleep: regulation of noradrenergic receptor sensitivity. Brain Res (1988) 472(3):213–33. doi:10.1016/0165-0173(88)90007-0
141. Hipolide DC, Tufik S, Raymond R, Nobrega JN. Heterogeneous effects of rapid eye movement sleep deprivation on binding to alpha- and beta-adrenergic receptor subtypes in rat brain. Neuroscience (1998) 86(3):977–87. doi:10.1016/S0306-4522(98)00067-0
142. Hipolide DC, Moreira KM, Barlow KB, Wilson AA, Nobrega JN, Tufik S. Distinct effects of sleep deprivation on binding to norepinephrine and serotonin transporters in rat brain. Prog Neuropsychopharmacol Biol Psychiatry (2005) 29(2):297–303. doi:10.1016/j.pnpbp.2004.11.015
143. Pedrazzoli M, Benedito MA. Rapid eye movement sleep deprivation-induced down-regulation of beta-adrenergic receptors in the rat brainstem and hippocampus. Pharmacol Biochem Behav (2004) 79(1):31–6. doi:10.1016/j.pbb.2004.06.001
144. Breslau N, Roth T, Burduvali E, Kapke A, Schultz L, Roehrs T. Sleep in lifetime posttraumatic stress disorder: a community-based polysomnographic study. Arch Gen Psychiatry (2004) 61(5):508–16. doi:10.1001/archpsyc.61.5.508
145. Habukawa M, Uchimura N, Maeda M, Kotorii N, Maeda H. Sleep findings in young adult patients with posttraumatic stress disorder. Biol Psychiatry (2007) 62(10):1179–82. doi:10.1016/j.biopsych.2007.01.007
146. Mellman TA, Pigeon WR, Nowell PD, Nolan B. Relationships between REM sleep findings and PTSD symptoms during the early aftermath of trauma. J Trauma Stress (2007) 20(5):893–901. doi:10.1002/jts.20246
147. Stickgold R. Of sleep, memories and trauma. Nat Neurosci (2007) 10(5):540–2. doi:10.1038/nn0507-540
Keywords: sleep, stress, prolactin, CLIP, serotonin, CRH, homeostasis, REM sleep
Citation: Machado RB and Suchecki D (2016) Neuroendocrine and Peptidergic Regulation of Stress-Induced REM Sleep Rebound. Front. Endocrinol. 7:163. doi: 10.3389/fendo.2016.00163
Received: 23 June 2016; Accepted: 09 December 2016;
Published: 23 December 2016
Edited by:
Jacques Epelbaum, French Institute of Health and Medical Research, FranceReviewed by:
Erin Hanlon, Pritzker School of Medicine, USAJeremy Terrien, Museum National d’Histoires Naturelles, France
Copyright: © 2016 Machado and Suchecki. This is an open-access article distributed under the terms of the Creative Commons Attribution License (CC BY). The use, distribution or reproduction in other forums is permitted, provided the original author(s) or licensor are credited and that the original publication in this journal is cited, in accordance with accepted academic practice. No use, distribution or reproduction is permitted which does not comply with these terms.
*Correspondence: Ricardo Borges Machado, bWFjaGFkby5yaWNhcmRvYm9yZ2VzQGdtYWlsLmNvbQ==