- 1Department of Nuclear Medicine, Klinikum Luedenscheid, Luedenscheid, Germany
- 2North Lakes Clinical, Ilkley, UK
- 3Medical Department I, Endocrinology and Diabetology, Bergmannsheil University Hospitals, Ruhr University of Bochum, Bochum, Germany
- 4Ruhr Center for Rare Diseases (CeSER), Ruhr University of Bochum and Witten/Herdecke University, Bochum, Germany
The long-held concept of a proportional negative feedback control between the thyroid and pituitary glands requires reconsideration in the light of more recent studies. Homeostatic equilibria depend on dynamic inter-relationships between thyroid hormones and pituitary thyrotropin (TSH). They display a high degree of individuality, thyroid-state-related hierarchy, and adaptive conditionality. Molecular mechanisms involve multiple feedback loops on several levels of organization, different time scales, and varying conditions of their optimum operation, including a proposed feedforward motif. This supports the concept of a dampened response and multistep regulation, making the interactions between TSH, FT4, and FT3 situational and mathematically more complex. As a homeostatically integrated parameter, TSH becomes neither normatively fixed nor a precise marker of euthyroidism. This is exemplified by the therapeutic situation with l-thyroxine (l-T4) where TSH levels defined for optimum health may not apply equivalently during treatment. In particular, an FT3–FT4 dissociation, discernible FT3–TSH disjoint, and conversion inefficiency have been recognized in l-T4-treated athyreotic patients. In addition to regulating T4 production, TSH appears to play an essential role in maintaining T3 homeostasis by directly controlling deiodinase activity. While still allowing for tissue-specific variation, this questions the currently assumed independence of the local T3 supply. Rather it integrates peripheral and central elements into an overarching control system. On l-T4 treatment, altered equilibria have been shown to give rise to lower circulating FT3 concentrations in the presence of normal serum TSH. While data on T3 in tissues are largely lacking in humans, rodent models suggest that the disequilibria may reflect widespread T3 deficiencies at the tissue level in various organs. As a consequence, the use of TSH, valuable though it is in many situations, should be scaled back to a supporting role that is more representative of its conditional interplay with peripheral thyroid hormones. This reopens the debate on the measurement of free thyroid hormones and encourages the identification of suitable biomarkers. Homeostatic principles conjoin all thyroid parameters into an adaptive context, demanding a more flexible interpretation in the accurate diagnosis and treatment of thyroid dysfunction.
Dual Role of Hormones in Thyroid Homeostasis
The dynamic ability to maintain flexible homeostatic equilibria in response to environmental challenges is a hallmark of a healthy state of the organism. Thyroid hormones assume a dual role in homeostatic regulation, acting as controlling as well as controlled elements. They target a broad spectrum of metabolic effects but concomitantly are strongly regulated themselves. A basic understanding of thyroid control involving pituitary thyrotropin (TSH) has been readily exploited for the diagnosis of thyroid disorders (1–4). As a result, measurement of TSH, though an indirect indicator of thyroid homeostasis, has become central to contemporary thyroid function testing (4, 5). Our knowledge of the mechanisms involved in the regulation of thyroid hormones has greatly evolved in recent years. The underlying system is far more complex than previously thought (Figure 1). This requires a revision of long-held simplistic concepts and promotes a multifactorial concept of the feedback control between the thyroid and the pituitary gland (6–9). In this article, we review the role of thyroid homeostasis in the light of recent developments and discuss the resulting new perspectives for diagnosis and treatment of thyroid dysfunction.
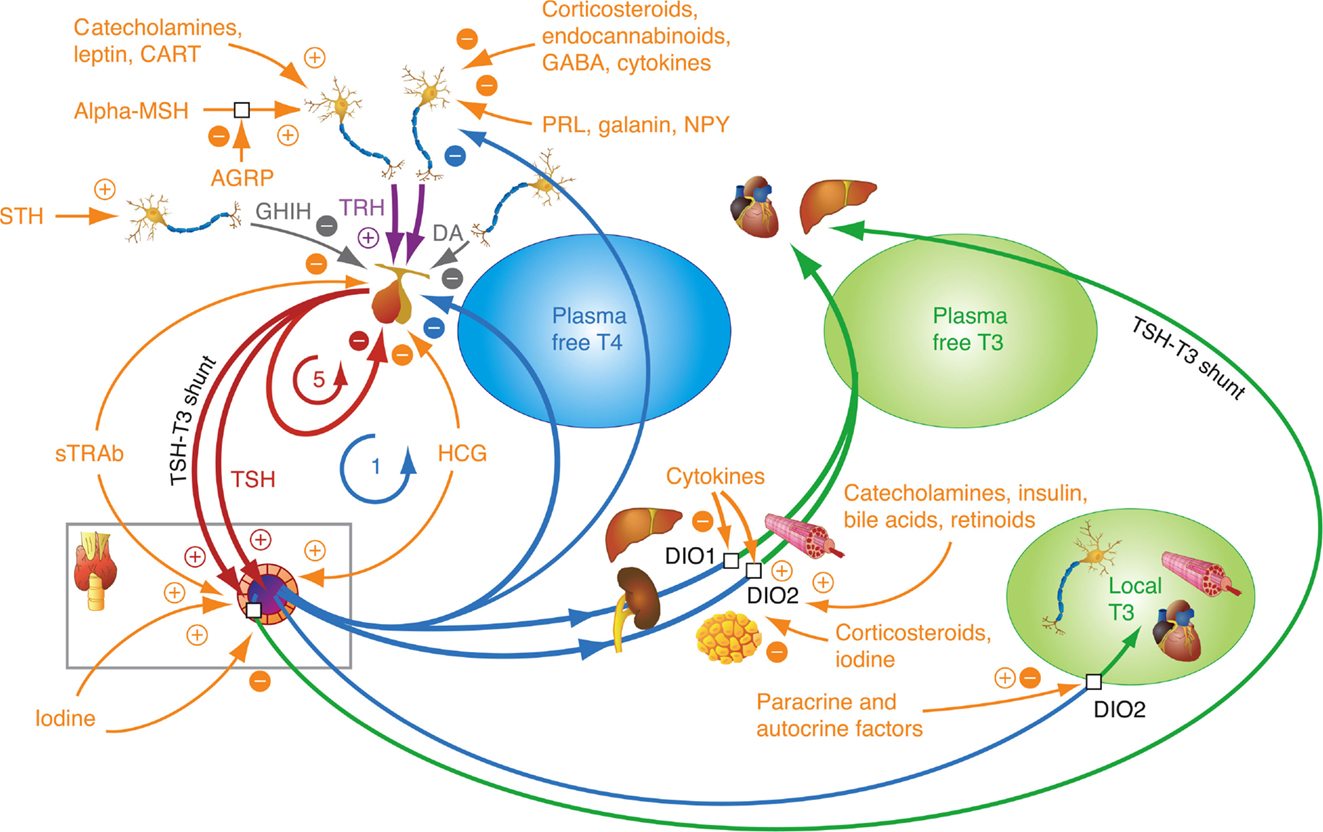
Figure 1. Homeostatic integration of central, thyroidal, and peripheral influences. The integrated control involves several major control loops, a negative feedback control of thyroid hormones on pituitary TSH and hypothalamic TRH, positive stimulatory control of TRH on TSH, ultrashort feedback of TSH on its own secretion, and feedforward control of deiodinases by TSH. Other thyrotropic agonists than TSH, such as TSH receptor antibodies (TSH-R Ab) and human chorionic gonadotropin (hCG), play an important role in diseases, such as Graves’ disease and pregnancy-related hyperthyroidism. A plethora of additional influences may fine-tune the responses at each level of organization. 1 refers to the classical Astwood–Hoskins loop, and 5 indicates ultrashort feedback loop of TSH on its own secretion, described in the text. Additional feedback loops (not shown here) control the binding of thyroid hormones to plasma proteins (8, 10).
Homeostatic Aspects of Thyroid Function Control
From first principles, it is clinically important to understand clearly what distinguishes a controlling parameter from any other. A change in TSH concentration could be either merely adaptive to restore true euthyroidism or a failed attempt to maintain the euthyroid state. Corrective moves of the control parameter may therefore merely imply a change in the mechanism targeted. This depends on whether the correction sought for is successfully achieved or not. Any meaningful interpretation must respect those particularities in TSH, which do not apply to most other laboratory parameters.
The concept of a control loop feeding back information about the state of thyroid production to the pituitary gland was postulated as early as 1940 (11) and established experimentally before 1950 (12, 13). Models initially assumed an inverse linear correlation between TSH and T4 (14–17), but following more detailed analysis this was later changed to a log-linear relationship, which has remained the standard model ever since (18–22). As circulating thyroid hormones are bound to a large extent to transport proteins (TBG, transthyretin, and albumin) TSH has mostly been related to the unbound biologically active hormone, free T4 (FT4). Table 1 summarizes various thyroid–pituitary feedback models that have been proposed in the literature over the last decades (6–10, 14–19, 21–39). The feedforward path linking TSH levels to T4 output has been modeled as a simple linear relation in the majority of these models.
From the perspective of a sufficiently sensitive defensive response, however, linear or log-linear proportional relations between TSH and FT4 would not intuitively appear to be the most adequate solution. As in many technical systems, a dampened response could be more suited to maintain the controlled parameter at a given stable level with a minimal fluctuation. This consideration requires an examination of the system operating beyond the standard log-linear model.
A Reassessment of Thyroid–Pituitary Feedback Control
It would be ideal to follow individuals’ responses during progression from the hypothyroid to the hyperthyroid state to study the changing pituitary response over the entire functional spectrum. Analyses that do not cover the full spectrum from the hypothyroid to the hyperthyroid extremes are problematic to interpret, because wide variations in the slopes of the logTSH–FT4 relationships have been reported (19, 21, 22, 42). Particularly, different weightings of the extreme, statistically most influential dysfunctional examples in the various patient panels impact heavily on the linear regression. Studies restricted to a narrower euthyroid panel have yielded TSH estimates when extrapolating the regression line to the hypothyroid state are much lower than those clinically observed in the hypothyroid patient (21, 22). Large cross-sectional studies have examined the TSH–FT4 relationship over the entire functional range but did not confirm a proportional and log-linear TSH–FT4 relationship, rather suggesting that the TSH response to changes in FT4 is curvilinear and damped in the middle part (6, 7, 9, 33) (Figure 2). Technically, using either a modulatory logistic function, a segmented approach, non-competitive inhibition, or polynomial approximation offers similar ways of examining the same underlying principles of a non-proportional adaptive response dependent on the actual thyroid hormone status (6–8, 33). The non-linearity of the logTSH–FT4 relationship has been independently confirmed by several groups and was replicated in a prospective study involving 1912 subjects (7–9, 33, 34). Thus, the TSH–FT4 relationship is not invariant but is impacted on by the thyroid status itself, which acts as a major determinant of the gradient relating TSH and FT4 (8). Accordingly, the thyroid state may be more vigorously defended, the greater is the deviation from a putative optimum state (6) (Figure 2). This behavior provides a far more flexible response than a simple log-linear template. It may conceivably arise from the integrated action of the multiple feedback loops operating at various levels of organization, as shown in Figure 1. The consequences of the non-proportional relationship for the clinical interpretation are discussed below.
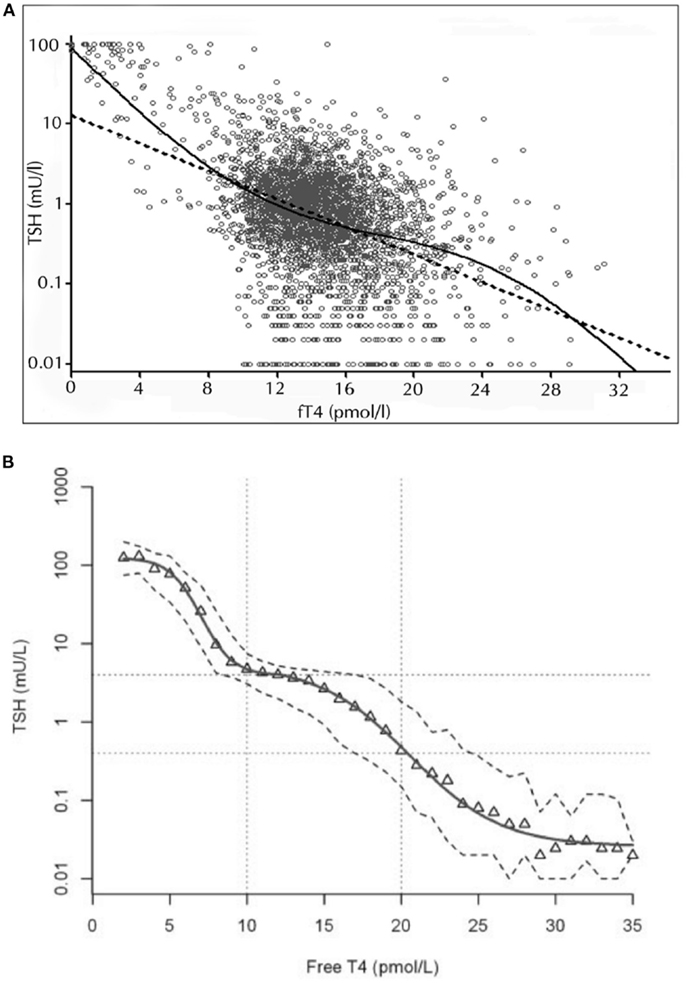
Figure 2. Non-linear relationship between logTSH and free T4. The two studies by Hoermann et al. (6) (A) and Hadlow et al. (7) (B) show that the TSH–FT4 relationship may not follow a proportional log-linear model (dashed straight line), displaying a damped response in the euthyroid range and steeper gradients at the hypothyroid or hyperthyroid spectrum. The superiority of the non-linear modulatory logistic function shown over the standard log-linear model was statistically established by a strict curve-fitting template based on Akaike’s information criterion (6). A multistep regulation of the FT4–TSH feedback control is discussed in the text. Adapted and reproduced with permission from Hoermann et al. (6) and Hadlow et al. (7).
It should be noted that these studies relied on immunometric FT4 assays, since tandem mass spectrometry (LC–MS/MS) is currently not practicable with large patient panels. However, none of the studies gave indication of a TSH–thyroid hormone mismatch. The inverse linear relation between thyroid hormones and logTSH was similarly broken with an immunoassay and LC–MS/MS in a clinically diverse sample contradicting an earlier report (22, 34). A subanalysis was conducted in a cohort of otherwise “healthy” out-patients without relevant comorbidity to ascertain FT3 or FT4 measurements and their relationships were not compromised by problematic conditions, such as pituitary dysfunction and the non-thyroid illness syndrome (6). Reliability of immunological methods and harmonization among various commercially available assays has been questioned (43, 44). In this respect, we have extensively evaluated the analytical performance of the immunoassays used in our inter-relational studies (45). In particular, we verified the reference range in the local population, demonstrated robustness of the relationships despite biological variation, and quantified other influences on the defining relationships, such as age and body mass index (9, 45). A clinically important and specific role of FT3 measurement was further supported by our pilot study in a large unselected predominantly euthyroid sample (46). FT3, in the range from 1 to 10 pmol/l, but not FT4 or TSH, showed a significant u-shaped relationship with the Hospital Anxiety and Depression Score (HADS) as well as the anxiety and depression subscales in a generalized linear-quadratic model (not a widely used, but unsuitable linear model) (46). Together these findings support suitability of FT3 for correlative studies when measured with the same instrument at a single institution but do not negate issues of insufficient validation and standardization with the methods discussed below.
Molecular Mechanisms Involved in the Feedback Control
While this article focuses on homeostatic regulation and an in-depth review of the growing body of molecular details is beyond its scope, it should be briefly shown that key mechanisms are reconcilable with a non-proportional model. Both T3 and T4 following its conversion into T3 bind to specific intracellular TR receptors exerting a repressive action on various genes, including TSHβ and, to a lesser degree, α-subunit (47–52). Among the isoforms of TR expressed in various tissues, TRβ2 is active in the central nervous system, hypothalamus, and pituitary gland, with a reported sensitivity enhanced up to 10-fold to thyroid hormones, compared with TRβ1 (53, 54). Such a differential response should enable the central tissues to anticipate T3/T4 oversupply before it can affect less sensitive peripheral tissues. Similarly, deiodinases in central and peripheral tissues are also regulated differently, enhancing T3 conversion and providing a differentiated mechanism for oversensitively responding to changes in FT4 in the feedback loop (55–63). Specifically, type 2 deiodinase ubiquitination has recently been shown to be instrumental in hypothalamic negative feedback regulation and is expressed in a non-uniform way among various tissues (64). Contrary to earlier assumptions, T3 and T4 do not diffuse freely across the plasma membrane but are actively transported by specialized transport proteins, such as MCT8, MCT10, and OATP1C1 (65). Intracellular trafficking involves intracellular binding substrates (IBSs) of thyroid hormones (e.g., CRYM) (66). These carriers appear to be necessary components of the feedback control, as simulated for IBS and demonstrated for MCT8 deficiency (27, 65). Additionally, in rodents their hypothalamic expression has been shown to be subject to regulation by T3 (67). Transmembrane transport control adds another layer of complexity to the system but is currently not well understood.
While negative thyroid hormone feedback mitigates thyroid hormone overproduction and hyperthyroidism, TRH is a potent defensive mechanism against undersupply, stimulating both pituitary TSH secretion and modulating its bioactivity (68–72). TSH stimulation of thyroid hormone production, in turn, is essential, because the TSH-independent basal capacity of the thyroid gland is limited and unable to maintain a euthyroid state. Tissue-specific glycosylation differentially regulates the hormone allowing for targeted signaling (73). Pars tuberalis-derived TSH has been shown to differ in its glycosylation pattern from the pars distalis-derived hormone, lacks the ability to stimulate the thyroid TSH receptor, and regulates deiodinase type 2 activity related to seasonality and behavior independent of thyroid hormone production (73). Long feedback control of TRH release by thyroid hormones involves both hypophysiotropic TRH neurons and tancytes, responding to humoral and neuronal inputs that can adjust the set point. The latter mechanism may integrate energy metabolism and thyroid function (74–76). This may play an important role in the pathogenesis of non-thyroidal illness (NTI) syndrome or thyroid allostasis in critical illness, tumors, uremia, and starvation (TACITUS) (74, 77–80).
Additionally, an ultrashort feedback loop involving the suppression of TSH by its own concentration has been proposed, mainly by one group (81–83). Our own studies including mathematical modeling of thyroid hormone homeostasis confirmed that this particular loop appears to be a relevant factor in influencing the TSH–FT4 relationship (8, 10).
The primary regulatory role of pituitary thyroid hormone feedback versus TRH stimulation has been studied using transgenic mice, but its relevance is still controversial (69, 70, 84, 85). In the pit-D2 KO mouse, Fonseca et al. (86) demonstrated coordination between the hypothalamic and pituitary T3 pathways that involve type 2 deiodinase. The role of deiodinase in tancytes was increased in the absence of pituitary deiodinase in order to preserve euthyroid serum T3 levels (86). The selective loss of pituitary type deiodinase, while increasing basal TSH in the mouse, diminished TSH response to hypothyroidism (87). However, knock-out animals with various degrees of deficiencies in all types of deiodinase have suffered little as a consequence, being able to maintain sufficient homeostatic regulation (88–90). It appears that multiple adaptive layers exist to protect the basic functionality of the homeostatic feedback control from various challenges. Furthermore, a multitude of physiological and pathophysiological influences modulates the relationship between TSH and thyroid hormones at various sites of action, thereby influencing the location of the set point in health and disease (Table 2) (8, 9, 76, 91–111).
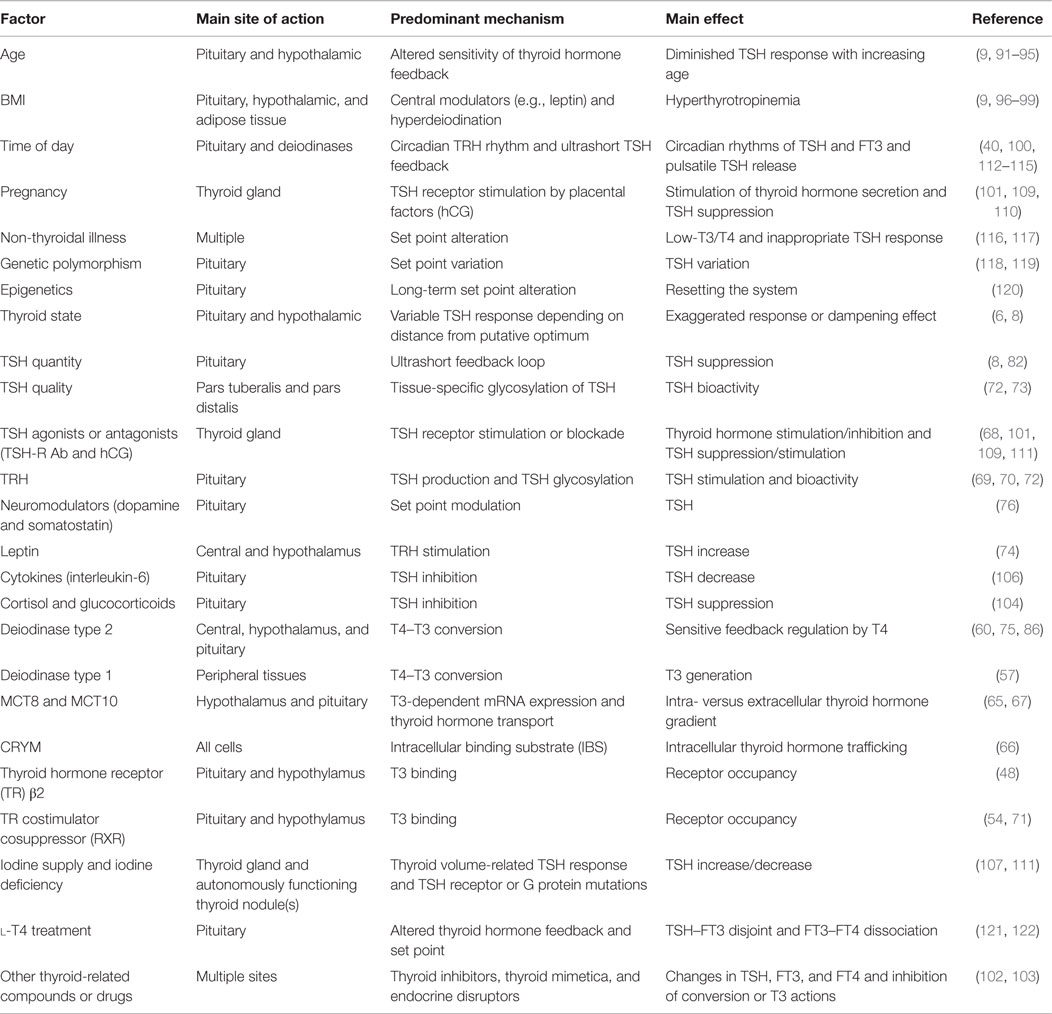
Table 2. Physiological and pathophysiological influences that may modulate the relationship between TSH and thyroid hormones.
Complementing the idea of a multifaceted feedback control, we have recently proposed that a feedforward motif may also be operative, directly linking TSH with deiodinase activity and the control of corporeal conversion from T4 to T3 (123). While this study provides the first documentation for a TSH-deiodinase inter-relation in humans in vivo, the responsiveness of deiodinase type 1 and type 2 to TSH, presumably through a TSH receptor- and cAMP-dependent mechanism, has been well recognized (55, 124–130).
Like other glycoprotein hormones, TSH is secreted in a pulsatile manner. Faster oscillations with a mean pulse amplitude of 0.6 mIU/l and a rate of 5–20/24 h are superimposed on a circadian rhythm with maximum TSH levels shortly after midnight (Figure 3) (112, 113, 131). It is still debated whether fast TSH pulses emerge by pulsatile TRH input, which has been contradicted by Samuels et al. (114), through stochastic signals or via autocrine function of thyrotrophs, i.e. controlled oscillations emanating from ultrashort feedback (10, 40, 115). TSH pulsatility may be beneficial by preventing homologous desensitization of the thyrotropin receptor (132–134). This could partly explain why sialylated TSH has both prolonged half-life and reduced bioactivity (135, 136).
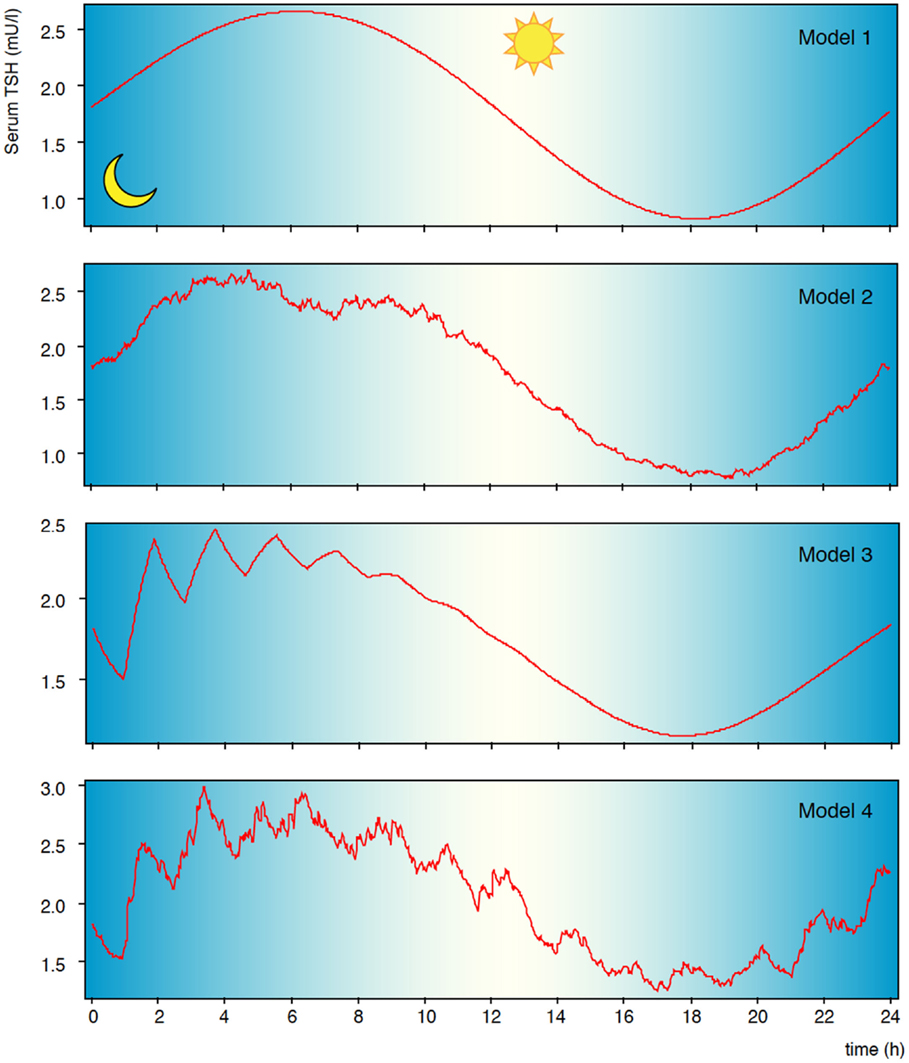
Figure 3. Pulsatility of TSH secretion. Secretion of thyrotropin is subject to circadian and ultradian variation. Shown are results of computer simulations with circadian input only (model 1), additional stochastic afferences (model 2), additional ultrashort feedback of TSH secretion (model 3), and combined stochastic input and ultrashort feedback (model 4). Statistical properties and fractal geometry of model 4 is identical to that of natural time series, while the simpler models differ (10).
A direct TSH-deiodinase link may, at least partly, explain the T3 circadian rhythm accompanying that of TSH, while FT4 shows no such related circadian or seasonal rhythm (137–139). This response may be modulated by regulating TSH receptor density, as shown in rats with severe thyroid dysfunction (140).
Importantly, these mechanisms align the task of defending plasma FT3 with the central control system (123). Supply of T3 to peripheral tissues is therefore no longer to be seen exclusively as a locally and autonomously regulated process, rather as a part of an overarching, integrated, and central-peripheral control system that governs thyroid hormone signaling in both homeostatic and allostatic regulatory modes (123). This is particularly relevant for the treatment situation with levothyroxine, as discussed below (121).
Figure 1 presents a synopsis of central, thyroidal, and peripheral influences and their homeostatic integration. Taken together, the molecular mechanisms defining multiple feedback loops on several levels of organization, different time scales, and varying conditions of their optimum operation may explain the disproportional non-logarithmic behavior of the TSH–FT4 relationship (Figure 2) (6–9, 33, 34). They support a multistep regulation and functionally hierarchical model that has been proposed by our group (8). While we have focused on describing the essential principles, additional physiological contributors, such as ethnicity, gender, age, body mass, iodine intake, selenium supply, T4 treatment, genetic deiodinase polymorphisms, and many others, may all elaborate the complexity of the system. Thus, further fine-tuning of the adaptive responses occurs at both the central and peripheral levels (Table 2) (9, 74, 76, 91, 92, 95, 97–99, 118, 119, 141–144).
Emerging Role of Non-Classical Thyroid Hormones
Some less recognized non-classical thyroid hormones, such as reverse triiodothyronine (rT3), 3,5-diiodothyronine (T2), iodothyroacetates, and thyronamines, have recently been revisited and found to play an active physiological role (Figure 4) (145, 146). rT3 (3,3′5′-T3) is a T3 isomer that is deiodinated in the 3′ position. It is upregulated in fetal life and NTI and interferes by blocking characteristics on thyroid signaling (147). 3,5-T2 exerts agonistic effects at nuclear thyroid hormone receptors, although its concentrations parallel those of rT3 in critical illness (148–150). Elevated 3,5-T2 concentrations in the non-thyroid illness syndrome could, at least in part, explain why patients displaying the low-T3 syndrome may not benefit from substitution therapy with l-thyroxine (l-T4) or l-triiodoythyronine (l-T3) (151).
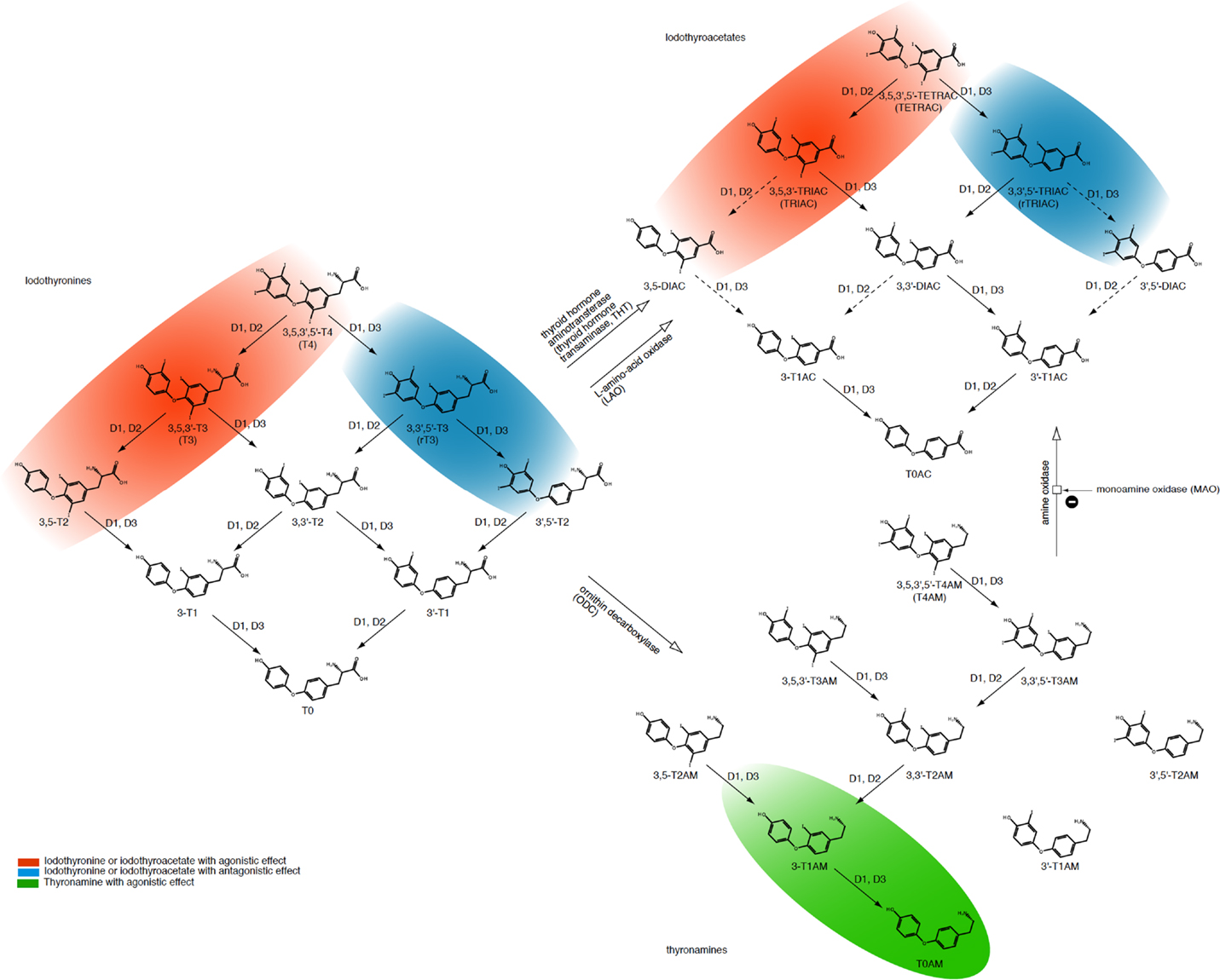
Figure 4. Overview of classical (iodothyronines) and non-classical thyroid hormones (iodothyroacetates and thyronamines) with associated interconversion processes. Adapted from Engler and Burger (152), Piehl et al. (153), Soffer et al. (154), and Hoefig et al. (155).
Iodothyroacetates are smaller, deaminated variants of thyroid hormones and have similar effects to those of iodothyronines (152, 156). However, their plasma half lives and affinity to receptors and transporters differ from the latter (157, 158). Due to its smaller molecule size triiodothyroacetate (TRIAC) is used for the treatment of resistance to thyroid hormone (RTH), but this effect does not seem to be beneficial for all mutant variants (157, 158).
Although thyronamines originate from follicular thyroid tissue and are structurally similar to iodothyronines, their biological effects are different. In many respects, their effects are antagonistic effects to those of the classical thyroid hormones (153, 159, 160). Classical and non-classical thyroid hormones can be interconverted by enzymes in certain body compartments (Figure 4) (153–155). While the effect of non-classical thyroid hormones on the overall behavior of thyroid homeostasis is still to be elucidated in more detail, some molecules including 3,5-T2, TRIAC, and TETRAC have thyromimetic effects at TR-β receptors, thereby exerting TSH-suppressive actions (156, 157, 161–163). This suggests a role of non-classical thyroid hormones as important modulators of the overall control system in supporting feedback loops controlling release and conversion of thyrotropin and the classical thyroid hormones. The resulting complexity of the homeostatic system is reflected in the non-proportional relationship between FT4 and TSH concentrations (Figure 2).
Consequences for Thyroid Function Testing
Accordingly, the novel insights into thyroid–pituitary hypothalamic regulation of thyroid hormones described above have important consequences for thyroid function testing. The initial discovery that pituitary TSH responds inversely to the underlying thyroid hormone concentration has greatly influenced current clinically applied thyroid testing (4). Its exaggerated response allows much greater sensitivity to subtle changes in the thyroid hormone status. The first TSH-based thyroid test strategies emerged in the 1980s (164). Whilst the vast majority of studies concentrated on TSH testing, there were few attempts at physiologically based modeling (Table 1) (10, 26, 101, 165, 166). The consensus of TSH as a more sensitive diagnostic test than FT4 measurement has been summarized repeatedly in laboratory-focused procedures on TSH measurement and clinical guidelines on its practical use (2, 4, 167). Technically, routinely employed TSH assays are now in their third generation with each advance significantly enhancing functional assay sensitivity and the ability to clearly separate suppressed TSH levels observed in overt hyperthyroidism from levels at the lower reference limits seen in euthyroid subjects (168). Clinicians have embraced the availability of such a sensitive and cost-effective instrument. TSH is also employed in numerous prognostic studies which define it as a statistical marker of future outcomes (169–175).
This has important consequences as to how TSH has become viewed by the thyroid community as a simple and efficient diagnostic parameter. The ease of measurement was translated into simplicity of interpretation, ignoring the fact that TSH is both an indirect measure reflective of thyroid hormone homeostasis and a controlling element. Thereby, this concept obscured the intricate relationship of the TSH response with the underlying change in the hormonal milieu. By separating TSH from its physiological roots and primary role as a controlling element (Figure 1), not only did it become a statistical parameter in its own right, but also it thereby gained the role of the dominant thyroid function test. Consequently, definitions of hypothyroidism or hyperthyroidism were adjusted, introducing new laboratory-based and TSH-derived disease entities of subclinical hypothyroidism and hyperthyroidism, which are defined by an abnormal TSH level while FT3 and FT4 still dwell within their reference ranges (176–178). This was a major conceptual shift, as a disease had now become exclusively defined by measuring a single laboratory value, and, as a result, thyroid disease prevalence was thereby linked to the performance of a single test (179).
Homeostasis and the Reference Range of TSH
While acknowledging strategic advantages of TSH measurement, such as ease of use, suitability for first-line screening, detection of subtle functional abnormalities, and association with various health outcomes including mortality, there are considerable risks of distorting its integrated physiological importance. The misconception is highlighted by the ongoing controversy surrounding the reference limits of TSH, particularly its upper limit defining subclinical hypothyroidism (167, 180, 181). Proposed amendments to the range, taking into account additional factors such as hidden autoimmunity, ethnicity, gender, and age, offer minor corrections but still fall short of a satisfactory solution. The issues may be more fundamental in nature (182). Even logarithmic transformation of TSH does not totally succeed in restoring a normal distribution. Some authors have attributed this failure to the presence of hidden pathologies, such as autoimmune disorders, others disagreeing with that conclusion (183, 184). We have adopted an alternative statistical approach to the conventional method of establishing the reference interval (45). This involves extrapolation from a normally distributed, robust middle part of the range to the respective boundaries and is suitable for verifying proposed reference ranges by third parties, such as laboratories and manufacturers, using their own retrospective sample of the target population.
However, this does not overcome the problems of diagnostic interpretation using TSH. Unlike many other laboratory parameters, TSH values are personalized measures exhibiting a high degree of individuality. The ratio of the interindividual to the intraindividual variation may serve as an estimate of “individuality,” being much higher for TSH than most laboratory parameters, for example, 2 in an earlier report and 2.9 in a recent study (45, 185). Accordingly, the same TSH value could be “normal” for one individual but pathological for another. This also holds for patients with subclinical dysfunction, in whom the relationship between FT4 and TSH shows elements both of normality and abnormality (101). Apart from the statistical requirement that a TSH value in the subclinical range must change by 30% to be confidently classified as change rather than variation or fluctuation, the true nature of TSH referencing is bivariate in relation to an appropriate individual TSH level when combined with a certain FT4 level (101, 186–189). Pulsatility of TSH release adds to the intraindividual variation in TSH levels, which is higher than that of circulating FT4 concentrations (45, 190). Circadian and ultradian rhythms of TSH levels reduce diagnostic accuracy unless reference intervals are adapted or blood sampling is restricted to morning time (100).
Furthermore, the observed rather flat TSH–FT4 relationship within the euthyroid range of the population makes a particular TSH measurement more ambiguous in its prediction of the underlying thyroid state than it does when related by a steeper gradient (Figure 2). This questions reliance solely on TSH measurements whenever precise estimates of thyroid function are warranted, but to consider all three thyroid parameters TSH, FT4, and FT3 and their inter-relationships. However, only a few published studies have followed this approach, establishing as a proof of concept truly multivariate reference ranges for thyroid parameters, instead of a combination of two statistically independent univariate reference intervals (191–194). A model that respects the relationship between TSH and thyroid hormones raises the concept of an individually and conditionally determined set point. This is the intersecting point on the overlaid characteristic curves for thyroidal T4 production and pituitary TSH secretion (35, 41, 101). It is important to appreciate that the homeostatic relationship of TSH and FT4 defines the reference range of TSH in a “kite-shaped” graphical configuration, as opposed to the rectangular area obtained by plotting the two univariate parameters (101). A mathematical algorithm has recently been proposed to reconstruct the set point in an individual independently of a population-based reference range (35, 195). The clinical potential of this novel approach awaits further trials. It might prove useful in assessing the appropriateness of a TSH value in a given patient, thus legitimizing a personalized TSH target for thyroid hormone replacement therapy.
While relatively stable in thyroid health, the set point is, however, not fixed but acts as an important physiological integrator and modulator for the homeostatic and allostatic regulation of thyroid hormones (Figure 1) (9, 116). This demands a careful diagnostic interpretation taking into account additional information about the clinical condition and various historical influences that may temporarily or permanently impact on the location of the set point at various hierarchical levels (Table 2). In extremis, the notion of a non-fixed TSH set point is typified in the NTI syndrome and other constellations of thyroid allostasis where TSH measurement fails as a diagnostic test for that reason (117). The persistence of a significant homeostatic deviation for a prolonged period of time, may, in turn, irrevocably alter the position of the set point, which then assumes a “normality” that is now vigorously defended anew (120). This potential plasticity of thyroid homeostasis is part of a broader concept of epigenetic influences where the bidirectional interchange between heredity and the environment plays a defining role.
In conclusion, the conventional reference system and reliability of TSH measurement as a clinically adequate measure of euthyroidism is compromised by its indirect influence dependent on its fundamental relationship with the underlying thyroid hormones. This, however, is neither proportional (log-linear), as previously thought, nor is it unconditional, but rather complex, hierarchical, and highly individual. Consequently, subclinically hypothyroid patients therefore comprise a heterogeneous population of truly dysfunctional and truly euthyroid subjects. Hence, current definitions of subclinical hypothyroidism or hyperthyroidism cannot serve as a satisfactory and consistent aid to an accurate disease classification in itself. Emerging integrated and personalized diagnostic concepts need to be evaluated and appropriate new markers of tissue euthyroidism must be developed.
Homeostatic Considerations in T4-Treated Patients
The rationale for using TSH as an important treatment target is that the patient’s own pituitary gland is generally assumed to be a good determinant for establishing the dose adequacy of l-T4 treatment, even though differences between the T3 utilization of various tissues may exist (4, 5). Thereby, the TSH value derived from optimum health is deemed an appropriate level to aim at for treatment in most patients, excluding systemic NTI and pituitary disorders where the TSH response is compromised. However, when put to the test, we and others found this assumption to be invalid (9). On the contrary, the interlocking inter-relationships between FT3, FT4, and TSH were not invariably fixed but conditionally and homeostatically determined. In l-T4-treated patients, we showed a significant upward or downward shift and change in the gradients of the FT4 or FT3 regression line with logTSH, compared to untreated controls (9). The phenomenon reveals a disjoint in the relationship between TSH and FT3 (122). While earlier studies already hinted that TSH normalization may not suffice to guarantee a normal serum T3, the more detailed inter-relationships have only recently been analyzed (9, 122, 196–199). From a homeostatic point of view, evidence suggests that the stability of serum T3 is maintained over a wide variation in the endogenous thyroid hormone production in healthy subjects but is lost in the l-T4-treated athyreotic patient (Figure 5) (121, 123). This observation raises questions regarding T3 adequacy in treated hypothyroidism. The lower FT3 levels frequently documented in athyreotic l-T4-treated patients, as compared to untreated controls, have received scant attention, being widely dismissed as easily compensated at the tissue level in humans. However, feedback regulation seems clearly compromised in athyreotic patients on l-T4 treatment resulting in T3 instability and homeostatic equilibria that differ significantly from those in healthy subjects (123) (Figure 5). In this respect, the presence of the thyroid gland itself and the size of the remnant thyroid tissue after thyroid surgery have recently been shown to play an important role in stabilizing serum FT3, presumably through TSH stimulated intrathyroidal T3 conversion (123, 200). This may explain why athyreotic patients are particularly vulnerable, with approximately 15% living in a chronically low-T3 state below reference, even if they are able to normalize TSH (122, 198). Three remarkable phenomena have been observed in l-T4-treated patients, (1) a dissociation between FT3 and FT4, (2) a disjoint between TSH and FT3, and (3) an l-T4-related conversion inefficiency (121, 123). Hence, l-T4 dose escalation may not invariably remedy T3 deficiency but could actually hinder its attainment (121, 201). In addition to substrate inhibition and an inhibitory action of reverse T3 on the enzyme activity of deiodinase type 2, experimental studies in the rat elaborate on molecular details involving ubiquitination that may explain a lack of effect of increasing T4 dose in this condition (64). In the rodent, FT3 concentrations in the circulation remained low after escalation of the l-T4 dose (64). More importantly, irrespective of local variations by tissue or type of deiodinase involved, tissue hypothyroidism persisted in all organs examined including brain, liver, and skeletal muscle despite a normal TSH (64). The recent findings are in agreement with earlier studies in the rat (64, 202, 203). T3 supply is locally controlled by several mechanisms, such as active thyroid hormone uptake, tissue-specific expression and activity of two distinct types of deiodinases converting T3 from T4, and thyroid hormone inactivation by deiodination or degradation by sulfatation, deamination, or glucoronidation (57–66). The regulation varies by tissue as the brain predominantly expresses type 2 deiodinase, whereas type 1 deiodinase is abundant in other tissues of the body, and by thyroid state, as T3 excess upregulates type 1 deiodinase but downregulates type 2 deiodinase, which is upregulated in hypothyroidism (57–64). Type 3 deiodinase produces T3 in an inactive form, reverse T3. Although T3 utilization may be locally adjusted to meet the specific demands of each organ, tissue supply is not autonomously independent, but subject to the overarching central control, as discussed above. While corresponding data on tissue T3 in humans are widely lacking and the physiological proportions of T3 derived by conversion versus thyroidal secretion may differ in humans and rodents, the animal models indicate widespread tissue hypothyroidism of target organs in the presence of low serum T3 and normal TSH. This suggests that the disequilibria recognized between circulating FT3 concentrations and both FT4 and TSH in patients on l-T4 may remain intracellularly uncompensated and truly reflective of tissue deficiencies. However, the long-term consequences of the altered ratios are presently unknown. Interestingly, a strong TSH–FT3 relationship was a marker of familial longevity in a recent study confirming the prognostically important role of the equilibria measured in the circulation (204).
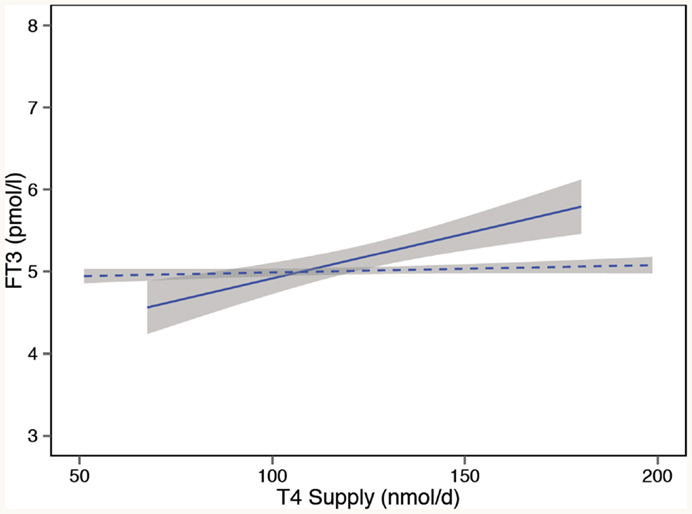
Figure 5. Loss of T3 stability in l-T4-treated athyreotic patients with thyroid carcinomas. In controls (dashed line), serum T3 remains stable over a wide variation in the endogenous thyroid hormone production. In contrast, in l-T4-treated patients (solid line), compensatory T3 regulation is broken, and serum T3 unstably varies with the exogenous T4 supply. Adapted and reproduced with permission from Hoermann et al. (123).
The novel implications of homeostatic regulation require further careful study and clinical follow-up. In humans, quality of life may be reduced in a substantial portion of hypothyroid patients taking levothyroxine, even though normal TSH levels suggest restoration of euthyroidism (205). Importantly, the interpretation of TSH values is not uniform among different pathophysiological conditions. A given TSH value in an athyreotic patient on l-T4 has a diagnostic implication entirely different from the same value in an untreated euthyroid subject. While concentrating on treatment-related aspects of thyroid homeostasis, we have not specifically addressed the treatment of hypothyroidism, which has been covered by several recent specialized articles (205–211). Though optimum treatment targets and modalities invite a fuller debate and further research, it is, however, increasingly clear that l-T4 treatment in its current form, which lacks approximately 10% naturally secreted T3 component, is at base an unphysiological treatment modality where the resulting homeostatic responses operate differently from normality. The diagnostic situation cannot therefore be judged by the same TSH-based criteria defining optimum health (9, 122).
Hence, we suggest that the use of TSH, valuable though it is in many situations, should be scaled back to a supporting role that is more appropriate to its conditional interplay with peripheral thyroid hormones. We emphasize that measurement and consideration of FT3 and conversion efficiency is equally important, particularly in known situations where TSH and FT3 dissociate. This reopens the debate on measurement of free thyroid hormones and encourages the identification of suitable biomarkers. While TSH assays are traceable to a single WHO standard, FT4 and especially FT3 methods are in urgent need of equivalent standardization and harmonization if they are to play a clinically acceptable role in an integrated concept (212). It remains general good clinical practice to only interpret laboratory tests in conjunction with a clinical assessment of the patient history and symptoms and to obtain appropriate confirmation or follow-up before commencing treatment. While TSH may be suitable for screening of asymptomatic conditions, the integrated interpretation of TSH, FT4, and FT3 and their conditional equilibria should benefit decision making, particular on dose adequacy of replacement therapy. Possible adverse effects of the homeostatic disequilibria that arise under the current standard treatment of l-T4 replacement also warrant careful study, and new treatment strategies should aim at maintaining more physiological equilibria.
Summary and Future Outlook
The concept of thyroid homeostasis offers new perspectives to optimize the interpretation of thyroid function tests and minimize the diagnostic misuse of an isolated and inappropriate statistical interpretation of TSH. The latter approach has wrongly assumed a level of diagnostic certainty that is inherently lacking in this indirect, conditional, and highly individual measure of thyroid function. We have described a new integrative concept, in which TSH becomes a context-sensitive conditional variable but is neither a precise marker of euthyroidism nor optimal for the fine-tuning of thyroid control. TSH levels defined for optimum health may not apply in many l-T4-treated patients. Because of a discernible disjoint between FT3 and TSH concentrations in athyreotic patients, this can result in an inability of T4 monotherapy to adequately address their therapeutic needs. Unlike in the healthy subject with adequate correction, FT3 levels now become unstably dependent on exogenous T4 supply. Furthermore, the T4-related conversion inefficiency may outweigh the benefits of escalating the l-T4 dose in some patients. Homeostatic principles question the isolated interpretation and disease-defining diagnostic value of TSH measurements, hence promoting both a more personalized approach and consideration of diagnosis in a more conditional adaptive context.
These perspectives raise a variety of issues that warrant further exploration and require carefully designed clinical studies before advancing to broader clinical application. The questions relate to multivariate reference limits, personalized set point reconstructions, and the additional value of FT3 for defining thyroid status and assessing dose adequacy in thyroid hormone replacement. There also may be clinical consequences and long-term risks of an unphysiological FT3–FT4 ratio, FT3–TSH disjoint, and impaired deiodinase activity on l-T4 replacement, supporting a possible role of combined treatment with T3 and T4 in selected patients with poor conversion efficiency.
Search Strategy
References for this review were identified through the authors’ personal files and searches of PubMed for articles published from January, 1971, to March, 2015, by the use of broader terms, such as thyroid homeostasis, feedback regulation, feedback control, reference interval, TSH, triiodothyronine, deiodinase, set point, and synonyms or combinations of the terms. Historically, relevant articles published between 1918 and 1971 were obtained through searches in the authors’ personal files, Google Scholar, and other Online Archives’ Collections. Articles published in English, French, and German were considered. Articles resulting from these searches and relevant references cited in those articles were reviewed. A more narrow search for homeostasis was done using the following terms: “pituitary AND thyroid AND (feedback OR homeostasis)” and “thyroid AND (simulation OR modeling).” Combined search strategy delivered more than 2000 articles to identify homeostatic models and mechanisms related to thyroid–pituitary feedback. To narrow down the listed references from the vast literature found, individual articles deemed highly original and most relevant were included, and broader concepts were covered by comprehensive review articles whenever possible.
Author Contributions
The authors jointly collaborated in conception of this review article, literature research, interpreting and condensing the results, and drafting of the manuscript. RH, JM, and RL provided Figures 2 and 5, and JD and RH created Figures 1 and 4 and Table 1.
Conflict of Interest Statement
Johannes W. Dietrich received funding and personal fees by Sanofi-Henning, Hexal AG, and Pfizer and is co-owner of the intellectual property rights for the patent “System and Method for Deriving Parameters for Homeostatic Feedback Control of an Individual” (Singapore Institute for Clinical Sciences, Biomedical Sciences Institutes, Application Number 201208940-5, WIPO number WO/2014/088516). All other authors declare that there is no conflict of interest that could be perceived as prejudicing the impartiality of the research reported.
Funding
Fees for publishing this review article were defrayed by an open access fonds of Ruhr University of Bochum based on a grant of the programme Scientific Library Services and Information Systems (LIS) of the German Research Foundation (Deutsche Forschungsgemeinschaft, DFG).
References
1. Larsen PR. Thyroid-pituitary interaction: feedback regulation of thyrotropin secretion by thyroid hormones. N Engl J Med (1982) 306:23–32. doi:10.1056/NEJM198201073060107
2. Baloch ZW, Carayon P, Conte-Devolx B, Demers LM, Feldt-Rasmussen U, Henry J-F, et al. Laboratory medicine practice guidelines. Laboratory support for the diagnosis and monitoring of thyroid disease. Thyroid (2003) 13:3–126. doi:10.1089/105072503321086962
3. Sam S, Frohman LA. Normal physiology of hypothalamic pituitary regulation. Endocrinol Metab Clin North Am (2008) 37:1–22. doi:10.1016/j.ecl.2007.10.007
4. Garber JR, Cobin RH, Gharib H, Hennessey JV, Klein I, Mechanick JI, et al. Woeber for the American Association of Clinical Endocrinologists and American Thyroid Association Taskforce on hypothyroidism in adults KA. Clinical practice guidelines for hypothyroidism in adults: cosponsored by the American Association of Clinical Endocrinologists and the American Thyroid Association. Thyroid (2012) 22:1200–35. doi:10.1089/thy.2012.0205
5. Jonklaas J, Bianco AC, Bauer AJ, Burman KD, Cappola AR, Celi FS, et al. Guidelines for the treatment of hypothyroidism: prepared by the American Thyroid Association task force on thyroid hormone replacement. Thyroid (2014) 24:1670–751. doi:10.1089/thy.2014.0028
6. Hoermann R, Eckl WA, Hoermann C, Larisch R. Complex relationship between free thyroxine and TSH in the regulation of thyroid function. Eur J Endocrinol (2010) 162:1123–9. doi:10.1530/EJE-10-0106
7. Hadlow NC, Rothacker KM, Wardrop R, Brown SJ, Lim EM, Walsh JP. The relationship between TSH and free T4 in a large population is complex and nonlinear and differs by age and sex. J Clin Endocrinol Metab (2013) 98:2936–43. doi:10.1210/jc.2012-4223
8. Midgley JEM, Hoermann R, Larisch R, Dietrich JW. Physiological states and functional relation between thyrotropin and free thyroxine in thyroid health and disease: in vivo and in silico data suggest a hierarchical model. J Clin Pathol (2013) 66:335–42. doi:10.1136/jclinpath-2012-201213
9. Hoermann R, Midgley JEM, Giacobino A, Eckl WA, Wahl HG, Dietrich JW, et al. Homeostatic equilibria between free thyroid hormones and pituitary thyrotropin are modulated by various influences including age, body mass index and treatment. Clin Endocrinol (Oxf) (2014) 81:907–15. doi:10.1111/cen.12527
10. Dietrich JW, Tesche A, Pickardt CR, Mitzdorf U. Thyrotropic feedback control: evidence for an additional ultrashort feedback loop from fractal analysis. Cybern Syst (2004) 35:315–31. doi:10.1080/01969720490443354
12. Stanley MM, Astwood EB. The response of the thyroid gland in normal human subjects to the administration of thyrotropin, as shown by studies with I131. Endocrinology (1949) 44:49–60. doi:10.1210/endo-44-1-49
13. Hoskins RG. The thyroid-pituitary apparatus as a servo (feed-back) mechanism. J Clin Endocrinol Metab (1949) 9:1429–31. doi:10.1210/jcem-9-12-1429
14. Danziger L, Elmergreen GL. The thyroid-pituitary homeostatic mechanism. Bull Math Biophys (1956) 18:1–13. doi:10.1007/BF02477840
15. Norwich KH, Reiter R. Homeostatic control of thyroxin concentration expressed by a set of linear differential equations. Bull Math Biophys (1965) 27:133–44. doi:10.1007/BF02498768
16. Distefano JJ III. A model of the regulation of circulating thyroxin unbound and bound to plasma proteins and its response to pregnancy, drugs, long-acting thyroid stimulator, and temperature stress. Math Biosci (1969) 4:137–52. doi:10.1016/0025-5564(69)90010-8
17. Wilkin TJ, Storey BE, Isles TE, Crooks J, Beck JS. High TSH concentrations in “euthyroidism”: explanation based on control-loop theory. Br Med J (1977) 1:993–6. doi:10.1136/bmj.1.6067.993
18. Leow MKS. A mathematical model of pituitary – thyroid interaction to provide an insight into the nature of the thyrotropin – thyroid hormone relationship. J Theor Biol (2007) 248:275–87. doi:10.1016/j.jtbi.2007.05.016
19. Spencer CA, LoPresti JS, Patel A, Guttler RB, Eigen A, Shen D, et al. Applications of a new chemiluminometric thyrotropin assay to subnormal measurement. J Clin Endocrinol Metab (1990) 70:453–60. doi:10.1210/jcem-70-2-453
20. Nicoloff JT, Spencer CA. Clinical review 12: the use and misuse of the sensitive thyrotropin assays. J Clin Endocrinol Metab (1990) 71:553–8. doi:10.1210/jcem-71-3-553
21. Benhadi N, Fliers E, Visser TJ, Reitsma JB, Wiersinga WM. Pilot study on the assessment of the setpoint of the hypothalamus-pituitary-thyroid axis in healthy volunteers. Eur J Endocrinol (2010) 162:323–9. doi:10.1530/EJE-09-0655
22. van Deventer HE, Mendu DR, Remaley AT, Soldin SJ. Inverse log-linear relationship between thyroid-stimulating hormone and free thyroxine measured by direct analog immunoassay and tandem mass spectrometry. Clin Chem (2011) 57:122–7. doi:10.1373/clinchem.2010.154088
23. Roston S. Mathematical representation of some endocrinological systems. Bull Math Biophys (1959) 21:271–82. doi:10.1007/BF02477915
24. Saratchandran P, Carson ER, Reeve J. An improved mathematical model of human thyroid hormone regulation. Clin Endocrinol (Oxf) (1976) 5:473–83. doi:10.1111/j.1365-2265.1976.tb01976.x
25. Cohen JL. Thyroid-stimulation hormone and its disorders. In: Becker KL, editor. Principles and Practice of Endocrinology and Metabolism. Philadelphia, PA: J B Lippincott Company (1990). p. 144–52.
26. Li G, Liu B, Liu Y. A dynamical model of the pulsatile secretion of the hypothalamo-pituitary-thyroid axis. Biosystems (1995) 35:83–92. doi:10.1016/0303-2647(94)01484-O
27. Dietrich JW. Spektrum medizinischer forschung. In: Schardt F, editor. Der Hypophysen-Schilddrüsen-Regelkreis. Vol 2, Berlin: Logos Verlag (2002).
28. Dietrich JW, Mitzdorf U, Weitkunat R, Pickardt CR. The pituitary-thyroid feedback control: stability and oscillations in a new nonlinear model. J Endocrinol Invest (1997) 20:100.
29. Degon M, Chait Y, Hollot C, Chipkin S, Zoeller T. A quantitative model of the human thyroid: development and observations. Am Control Conf (2005)2:961–6. doi:10.1109/ACC.2005.1470084
30. McLanahan ED, Andersen ME, Fisher JW. A biologically based dose-response model for dietary iodide and the hypothalamic-pituitary-thyroid axis in the adult rat: evaluation of iodide deficiency. Toxicol Sci (2008) 102:241–53. doi:10.1093/toxsci/kfm312
31. Eisenberg M, Samuels M, DiStefano JJ. Extensions, validation, and clinical applications of a feedback control system simulator of the hypothalamo-pituitary-thyroid axis. Thyroid (2008) 18:1071–85. doi:10.1089/thy.2007.0388
32. Eisenberg MC, Santini F, Marsili A, Pinchera A, DiStefano JJ. TSH regulation dynamics in central and extreme primary hypothyroidism. Thyroid (2010) 20:1215–28. doi:10.1089/thy.2009.0349
33. Clark PMS, Holder RL, Haque SM, Hobbs FDR, Roberts LM, Franklyn JA. The relationship between serum TSH and free T4 in older people. J Clin Pathol (2012) 65:463–5. doi:10.1136/jclinpath-2011-200433
34. Jonklaas J, Sathasivam A, Wang H, Gu J, Burman KD, Soldin SJ. Total and free thyroxine and triiodothyronine: measurement discrepancies, particularly in inpatients. Clin Biochem (2014) 47:1272–8. doi:10.1016/j.clinbiochem.2014.06.007
35. Goede SL, Leow MKS, Smit JWA, Dietrich JW. A novel minimal mathematical model of the hypothalamus-pituitary-thyroid axis validated for individualized clinical applications. Math Biosci (2014) 249:1–7. doi:10.1016/j.mbs.2014.01.001
36. DiStefano JJ III, Stear EB. Neuroendocrine control of thyroid secretion in living systems: a feedback control system model. Bull Math Biophys (1968) 30:3–26. doi:10.1007/BF02476936
37. DiStefano JJ, Chang RF. Computer simulation of thyroid hormone binding, distribution, and disposal dynamics in man. Am J Physiol (1971) 221:1529–44.
38. Hatakeyama T, Yagi H. Computer simulation for hormones related to primary thyropathy. Biol Cybern (1985) 52:259–66. doi:10.1007/BF00336982
39. Sorribas A, González A. The power-law formalism as a tool for modeling hormonal systems. J Theor Med (1999) 2:19–38. doi:10.1080/17486709909490786
40. Dietrich JW, Tesche A, Pickardt CR, Mitzdorf U. Fractal properties of the thyrotropic feedback control implications of a nonlinear model compared with empirical data. Proc Cybern Syst (2002) 2:329–34. doi:10.13140/2.1.1351.6803
41. Goede SL, Leow MKS, Smit JWA, Klein HH, Dietrich JW. Hypothalamus-pituitary-thyroid feedback control: implications of mathematical modeling and consequences for thyrotropin (TSH) and free thyroxine (FT4) reference ranges. Bull Math Biol (2014) 76:1270–87. doi:10.1007/s11538-014-9955-5
42. Reichlin S, Utiger RD. Regulation of the pituitary-thyroid axis in man: relationship of TSH concentration to concentration of free and total thyroxine in plasma. J Clin Endocrinol Metab (1967) 27:251–5. doi:10.1210/jcem-27-2-251
43. Korte W, Engler H, Riesen WF, Brinkmann T. Performance evaluation of the Access FT3 and FT4 assays, comparison with Immulite and AxSym, and the relationship to TSH values. Clin Lab (2012) 58:645–57. doi:10.7754/Clin.Lab.2011.110705
44. Thienpont LM, Van Uytfanghe K, Poppe K, Velkeniers B. Determination of free thyroid hormones. Best Pract Res Clin Endocrinol Metab (2013) 27:689–700. doi:10.1016/j.beem.2013.05.012
45. Larisch R, Giacobino A, Eckl WA, Wahl HG, Midgley JEM, Hoermann R. Reference range for thyrotropin. Post hoc assessment. Nuklearmedizin (2015) 54:112–7. doi:10.3413/Nukmed-0671-14-06
46. Larisch R, Schulte S, Hildenbrand G, Hoermann R. The role of thyroid hormones in anxiety and depression (Abstract). Nuklearmedizin (2015) 53:V162.
47. Lazar MA. Thyroid hormone action: a binding contract. J Clin Invest (2003) 112:497–9. doi:10.1172/JCI19479
48. Cheng S-Y, Leonard JL, Davis PJ. Molecular aspects of thyroid hormone actions. Endocr Rev (2010) 31:139–70. doi:10.1210/er.2009-0007
49. Chiamolera MI, Sidhaye AR, Matsumoto S, He Q, Hashimoto K, Ortiga-Carvalho TM, et al. Fundamentally distinct roles of thyroid hormone receptor isoforms in a thyrotroph cell line are due to differential DNA binding. Mol Endocrinol (2012) 26:926–39. doi:10.1210/me.2011-1290
50. Dumitrescu AM, Refetoff S. The syndromes of reduced sensitivity to thyroid hormone. Biochim Biophys Acta (2013) 1830:3987–4003. doi:10.1016/j.bbagen.2012.08.005
51. Astapova I, Hollenberg AN. The in vivo role of nuclear receptor corepressors in thyroid hormone action. Biochim Biophys Acta (2013) 1830:3876–81. doi:10.1016/j.bbagen.2012.07.001
52. Ortiga-Carvalho TM, Sidhaye AR, Wondisford FE. Thyroid hormone receptors and resistance to thyroid hormone disorders. Nat Rev Endocrinol (2014) 10:582–91. doi:10.1038/nrendo.2014.143
53. Wan W, Farboud B, Privalsky ML. Pituitary resistance to thyroid hormone syndrome is associated with T3 receptor mutants that selectively impair beta2 isoform function. Mol Endocrinol (2005) 19:1529–42. doi:10.1210/me.2005-0014
54. Lee S, Young BM, Wan W, Chan IH, Privalsky ML. A mechanism for pituitary-resistance to thyroid hormone (PRTH) syndrome: a loss in cooperative coactivator contacts by thyroid hormone receptor (TR)beta2. Mol Endocrinol (2011) 25:1111–25. doi:10.1210/me.2010-0448
55. Beech SG, Walker SW, Arthur JR, Lee D, Beckett GJ. Differential control of type-I iodothyronine deiodinase expression by the activation of the cyclic AMP and phosphoinositol signalling pathways in cultured human thyrocytes. J Mol Endocrinol (1995) 14:171–7. doi:10.1677/jme.0.0140171
56. Escobar-Morreale HF, Obregón MJ, Hernandez A, Escobar del Rey F, Morereale de Escobar G. Regulation of iodothyronine deiodinase activity as studied in thyroidectomized rats infused with thyroxine or triiodothyronine. Endocrinology (1997) 138:2559–68. doi:10.1210/endo.138.6.5212
57. Bianco AC, Salvatore D, Gereben B, Berry MJ, Larsen PR. Biochemistry, cellular and molecular biology, and physiological roles of the iodothyronine selenodeiodinases. Endocr Rev (2002) 23:38–89. doi:10.1210/edrv.23.1.0455
58. Wagner MS, Morimoto R, Dora JM, Benneman A, Pavan R, Maia AL. Hypothyroidism induces type 2 iodothyronine deiodinase expression in mouse heart and testis. J Mol Endocrinol (2003) 31:541–50. doi:10.1677/jme.0.0310541
59. Bianco AC, Kim BW. Deiodinases: implications of the local control of thyroid hormone action. J Clin Invest (2006) 116:2571–9. doi:10.1172/JCI29812
60. Gereben B, Zavacki AM, Ribich S, Kim BW, Huang SA, Simonides WS, et al. Cellular and molecular basis of deiodinase-regulated thyroid hormone signaling. Endocr Rev (2008) 29:898–938. doi:10.1210/er.2008-0019
61. Salvatore D. Deiodinases: keeping the thyroid hormone supply in balance. J Endocrinol (2011) 209:259–60. doi:10.1530/JOE-11-0058
62. Darras VM, Van Herck SLJ. Iodothyronine deiodinase structure and function: from ascidians to humans. J Endocrinol (2012) 215:189–206. doi:10.1530/JOE-12-0204
63. Abdalla SM, Bianco AC. Defending plasma T3 is a biological priority. Clin Endocrinol (Oxf) (2014) 81:633–41. doi:10.1111/cen.12538
64. Werneck de Castro JP, Fonseca TL, Ueta CB, McAninch EA, Abdalla SM, Wittmann G, et al. Differences in hypothalamic type 2 deiodinase ubiquitination explain localized sensitivity to thyroxine. J Clin Invest (2015) 125:769–81. doi:10.1172/JCI77588
65. Visser WE, Friesema ECH, Visser TJ. Minireview: thyroid hormone transporters: the knowns and the unknowns. Mol Endocrinol (2011) 25:1–14. doi:10.1210/me.2010-0095
66. Takeshige K, Sekido T, Kitahara J-I, Ohkubo Y, Hiwatashi D, Ishii H, et al. Cytosolic T3-binding protein modulates dynamic alteration of T3-mediated gene expression in cells. Endocr J (2014) 61(6):561–70. doi:10.1507/endocrj.EJ13-0418
67. Herwig A, Campbell G, Mayer C-D, Boelen A, Anderson RA, Ross AW, et al. A thyroid hormone challenge in hypothyroid rats identifies T3 regulated genes in the hypothalamus and in models with altered energy balance and glucose homeostasis. Thyroid (2014) 24:1575–93. doi:10.1089/thy.2014.0169
68. Szkudlinski MW, Fremont V, Ronin C, Weintraub BD. Thyroid-stimulating hormone and thyroid-stimulating hormone receptor structure-function relationships. Physiol Rev (2002) 82:473–502. doi:10.1152/physrev.00031.2001
69. Yamada M, Satoh T, Mori M. Mice lacking the thyrotropin-releasing hormone gene: what do they tell us? Thyroid (2003) 13:1111–21. doi:10.1089/10507250360731505
70. Nikrodhanond AA, Ortiga-Carvalho TM, Shibusawa N, Hashimoto K, Liao X-H, Refetoff S, et al. Dominant role of thyrotropin-releasing hormone in the hypothalamic-pituitary-thyroid axis. J Biol Chem (2006) 281:5000–7. doi:10.1074/jbc.M511530200
71. Costa-e-Sousa RH, Astapova I, Ye F, Wondisford FE, Hollenberg AN. The thyroid axis is regulated by NCoR1 via its actions in the pituitary. Endocrinology (2012) 153:5049–57. doi:10.1210/en.2012-1504
72. Estrada JM, Soldin D, Buckey TM, Burman KD, Soldin OP. Thyrotropin isoforms: implications for thyrotropin analysis and clinical practice. Thyroid (2014) 24:411–23. doi:10.1089/thy.2013.0119
73. Ikegami K, Liao X-H, Hoshino Y, Ono H, Ota W, Ito Y, et al. Tissue-specific posttranslational modification allows functional targeting of thyrotropin. Cell Rep (2014) 9:801–10. doi:10.1016/j.celrep.2014.10.006
74. Lechan RM, Fekete C. The TRH neuron: a hypothalamic integrator of energy metabolism. Prog Brain Res (2006) 153:209–35. doi:10.1016/S0079-6123(06)53012-2
75. Fekete C, Lechan RM. Negative feedback regulation of hypophysiotropic thyrotropin-releasing hormone (TRH) synthesizing neurons: role of neuronal afferents and type 2 deiodinase. Front Neuroendocrinol (2007) 28:97–114. doi:10.1016/j.yfrne.2007.04.002
76. Fekete C, Lechan RM. Central regulation of hypothalamic-pituitary-thyroid axis under physiological and pathophysiological conditions. Endocr Rev (2014) 35:159–94. doi:10.1210/er.2013-1087
77. Fekete C, Mihály E, Luo LG, Kelly J, Clausen JT, Mao Q, et al. Association of cocaine- and amphetamine-regulated transcript-immunoreactive elements with thyrotropin-releasing hormone-synthesizing neurons in the hypothalamic paraventricular nucleus and its role in the regulation of the hypothalamic-pituitary-thyroid axis during fasting. J Neurosci (2000) 20:9224–34.
78. Fekete C, Gereben B, Doleschall M, Harney JW, Dora JM, Bianco AC, et al. Lipopolysaccharide induces type 2 iodothyronine deiodinase in the mediobasal hypothalamus: implications for the nonthyroidal illness syndrome. Endocrinology (2004) 145:1649–55. doi:10.1210/en.2003-1439
79. Joseph-Bravo P. Hypophysiotropic thyrotropin-releasing hormone neurons as transducers of energy homeostasis. Endocrinology (2004) 145:4813–5. doi:10.1210/en.2004-0979
80. Mebis L, Debaveye Y, Ellger B, Derde S, Ververs E-J, Langouche L, et al. Changes in the central component of the hypothalamus-pituitary-thyroid axis in a rabbit model of prolonged critical illness. Crit Care (2009) 13:R147. doi:10.1186/cc8043
81. Prummel MF, Brokken LJS, Meduri G, Misrahi M, Bakker O, Wiersinga WM. Expression of the thyroid-stimulating hormone receptor in the folliculo-stellate cells of the human anterior pituitary. J Clin Endocrinol Metab (2000) 85:4347–53. doi:10.1210/jcem.85.11.6991
82. Prummel MF, Brokken LJS, Wiersinga WM. Ultra short-loop feedback control of thyrotropin secretion. Thyroid (2004) 14:825–9. doi:10.1089/thy.2004.14.825
83. Brokken LJS, Bakker O, Wiersinga WM, Prummel MF. Functional thyrotropin receptor expression in the pituitary folliculo-stellate cell line TtT/GF. Exp Clin Endocrinol Diabetes (2005) 113:13–20. doi:10.1055/s-2004-830516
84. Chiamolera MI, Wondisford FE. Minireview: thyrotropin-releasing hormone and the thyroid hormone feedback mechanism. Endocrinology (2009) 150:1091–6. doi:10.1210/en.2008-1795
85. Marsili A, Sanchez E, Singru P, Harney JW, Zavacki AM, Lechan RM, et al. Thyroxine-induced expression of pyroglutamyl peptidase II and inhibition of TSH release precedes suppression of TRH mRNA and requires type 2 deiodinase. J Endocrinol (2011) 211:73–8. doi:10.1530/JOE-11-0248
86. Fonseca TL, Correa-Medina M, Campos MPO, Wittmann G, Werneck de Castro JP, Arrojo e Drigo R, et al. Coordination of hypothalamic and pituitary T3 production regulates TSH expression. J Clin Invest (2013) 123:1492–500. doi:10.1172/JCI61231
87. Luongo C, Martin C, Vella K, Marsili A, Ambrosio R, Dentice M, et al. The selective loss of the type 2 iodothyronine deiodinase in mouse thyrotrophs increases basal TSH but blunts the thyrotropin response to hypothyroidism. Endocrinology (2015) 156:745–54. doi:10.1210/en.2014-1698
88. Schneider MJ, Fiering SN, Pallud SE, Parlow AF, St Germain DL, Galton VA. Targeted disruption of the type 2 selenodeiodinase gene (DIO2) results in a phenotype of pituitary resistance to T4. Mol Endocrinol (2001) 15:2137–48. doi:10.1210/mend.15.12.0740
89. Wagner MS, Wajner SM, Dora JM, Maia AL. Regulation of Dio2 gene expression by thyroid hormones in normal and type 1 deiodinase-deficient C3H mice. J Endocrinol (2007) 193:435–44. doi:10.1677/JOE-07-0099
90. Galton VA, de Waard E, Parlow AF, St Germain DL, Hernández A. Life without the iodothyronine deiodinases. Endocrinology (2014) 155:4081–7. doi:10.1210/en.2014-1184
91. Carle A, Laurberg P, Pedersen IB, Perrild H, Ovesen L, Rasmussen LB, et al. Age modifies the pituitary TSH response to thyroid failure. Thyroid (2007) 17:139–44. doi:10.1089/thy.2006.0191
92. Vadiveloo T, Donnan PT, Murphy MJ, Leese GP. Age- and gender-specific TSH reference intervals in people with no obvious thyroid disease in Tayside, Scotland: the Thyroid Epidemiology, Audit, and Research Study (TEARS). J Clin Endocrinol Metab (2013) 98:1147–53. doi:10.1210/jc.2012-3191
93. Over R, Mannan S, Nsouli-Maktabi H, Burman KD, Jonklaas J. Age and the thyrotropin response to hypothyroxinemia. J Clin Endocrinol Metab (2010) 95:3675–83. doi:10.1210/jc.2010-0281
94. Surks MI, Boucai L. Age- and race-based serum thyrotropin reference limits. J Clin Endocrinol Metab (2010) 95:496–502. doi:10.1210/jc.2009-1845
95. Bremner AP, Feddema P, Leedman PJ, Brown SJ, Beilby JP, Lim EM, et al. Age-related changes in thyroid function: a longitudinal study of a community-based cohort. J Clin Endocrinol Metab (2012) 97:1554–62. doi:10.1210/jc.2011-3020
96. Asvold BO, Bjøro T, Vatten LJ. Association of serum TSH with high body mass differs between smokers and never-smokers. J Clin Endocrinol Metab (2009) 94:5023–7. doi:10.1210/jc.2009-1180
97. Agnihothri RV, Courville AB, Linderman JD, Smith S, Brychta R, Remaley A, et al. Moderate weight loss is sufficient to affect thyroid hormone homeostasis and inhibit its peripheral conversion. Thyroid (2014) 24:19–26. doi:10.1089/thy.2013.0055
98. Knudsen N, Laurberg P, Rasmussen LB, Bülow I, Perrild H, Ovesen L, et al. Small differences in thyroid function may be important for body mass index and the occurrence of obesity in the population. J Clin Endocrinol Metab (2005) 90:4019–24. doi:10.1210/jc.2004-2225
99. Roef GL, Rietzschel ER, Van Daele CM, Taes YE, De Buyzere ML, Gillebert TC, et al. Triiodothyronine and free thyroxine levels are differentially associated with metabolic profile and adiposity-related cardiovascular risk markers in euthyroid middle-aged subjects. Thyroid (2014) 24:223–31. doi:10.1089/thy.2013.0314
100. Ehrenkranz J, Bach PR, Snow GL, Schneider A, Lee JL, Ilstrup S, et al. Circadian and circannual rhythms in thyroid hormones: determining the TSH and free T4 reference intervals based upon time of day, age, and sex. Thyroid (2015) 25:954–61. doi:10.1089/thy.2014.0589
101. Dietrich JW, Landgrafe G, Fotiadou EH. TSH and thyrotropic agonists: key actors in thyroid homeostasis. J Thyroid Res (2012) 2012:1–29. doi:10.1155/2012/351864
102. Gore AC, Chappell VA, Fenton SE, Flaws JA, Nadal A, Prins GS, et al. Executive summary to EDC-2: the endocrine society’s second scientific statement on endocrine-disrupting chemicals. Endocr Rev (2015) 28:er20151093. doi:10.1210/er.2015-1093
103. Jugan M-L, Levi Y, Blondeau J-P. Endocrine disruptors and thyroid hormone physiology. Biochem Pharmacol (2010) 79:939–47. doi:10.1016/j.bcp.2009.11.006
104. Pariante CM. Risk factors for development of depression and psychosis. Ann N Y Acad Sci (2009) 1179:144–52. doi:10.1111/j.1749-6632.2009.04978.x
105. Hage MP, Azar ST. The link between thyroid function and depression. J Thyroid Res (2012) 2012:590648–590648. doi:10.1155/2012/590648
106. Stouthard JM, van der Poll T, Endert E, Bakker PJ, Veenhof CH, Sauerwein HP, et al. Effects of acute and chronic interleukin-6 administration on thyroid hormone metabolism in humans. J Clin Endocrinol Metab (1994) 79:1342–6. doi:10.1210/jcem.79.5.7962327
107. Bjergved L, Jørgensen T, Perrild H, Carle A, Cerqueira C, Krejbjerg A, et al. Predictors of change in serum TSH after iodine fortification: an 11-year follow-up to the DanThyr study. J Clin Endocrinol Metab (2012) 97:4022–9. doi:10.1210/jc.2012-2508
108. Hoermann R, Schumm-Draeger P-MM, Rehbach K, Mann K. Asialoagalacto-human chorionic gonadotropin, a carbohydrate-modified variant of human chorionic gonadotropin, antagonizes the stimulatory actions of bovine thyroid-stimulating hormone on thyroid function and HLA-DR expression in human thyroid in vitro and in vivo. J Clin Invest (1991) 88:1947–54. doi:10.1172/JCI115519
109. Hoermann R, Broecker-Preuss M, Grossmann M, Mann K, Derwahl K-M. Interaction of human chorionic gonadotropin (hCG) and asialo-hCG with recombinant human thyrotropin receptor. J Clin Endocrinol Metab (1994) 78:933–8. doi:10.1210/jcem.78.4.8157724
110. Kato K, Mostafa MH, Mann K, Schindler AE, Hoermann R. The human chorionic gonadotropin molecule from patients with trophoblastic diseases has a high thyrotropic activity but is less active in the ovary. Gynecol Endocrinol (2004) 18:269–77. doi:10.1080/09513590410001667247
111. Vassart G, Dumont JE. The thyrotropin receptor and the regulation of thyrocyte function and growth. Endocr Rev (1992) 13:596–611. doi:10.1210/edrv-13-3-596
112. Brabant G, Prank K, Ranft U, Schuermeyer T, Wagner TO, Hauser H, et al. Physiological regulation of circadian and pulsatile thyrotropin secretion in normal man and woman. J Clin Endocrinol Metab (1990) 70:403–9. doi:10.1210/jcem-70-2-403
113. Samuels MH, Veldhuis JD, Henry P, Ridgway EC. Pathophysiology of pulsatile and copulsatile release of thyroid-stimulating hormone, luteinizing hormone, follicle-stimulating hormone, and alpha-subunit. J Clin Endocrinol Metab (1990) 71:425–32. doi:10.1210/jcem-71-2-425
114. Samuels MH, Henry P, Luther M, Ridgway EC. Pulsatile TSH secretion during 48-hour continuous TRH infusions. Thyroid (1993) 3:201–6. doi:10.1089/thy.1993.3.201
115. Krsmanovic LZ, Hu L, Leung P-K, Feng H, Catt KJ. The hypothalamic GnRH pulse generator: multiple regulatory mechanisms. Trends Endocrinol Metab (2009) 20:402–8. doi:10.1016/j.tem.2009.05.002
116. Fliers E, Kalsbeek A, Boelen A. Beyond the fixed setpoint of the hypothalamus-pituitary-thyroid axis. Eur J Endocrinol (2014) 171:R197–208. doi:10.1530/EJE-14-0285
117. Van den Berghe G. Non-thyroidal illness in the ICU: a syndrome with different faces. Thyroid (2014) 24:1456–65. doi:10.1089/thy.2014.0201
118. Dayan CM, Panicker V. Novel insights into thyroid hormones from the study of common genetic variation. Nat Rev Endocrinol (2009) 5:211–8. doi:10.1038/nrendo.2009.19
119. Hoftijzer HC, Heemstra KA, Visser TJ, le Cessie S, Peeters RP, Corssmit EPM, et al. The type 2 deiodinase ORFa-Gly3Asp polymorphism (rs12885300) influences the set point of the hypothalamus-pituitary-thyroid axis in patients treated for differentiated thyroid carcinoma. J Clin Endocrinol Metab (2011) 96:E1527–33. doi:10.1210/jc.2011-0235
120. Verburg FA, Smit JWA, Grelle I, Visser TJ, Peeters RP, Reiners C. Changes within the thyroid axis after long-term TSH-suppressive levothyroxine therapy. Clin Endocrinol (Oxf) (2012) 76:577–81. doi:10.1111/j.1365-2265.2011.04262.x
121. Midgley JEM, Larisch R, Dietrich JW, Hoermann R. Variation in the biochemical response to L-thyroxine therapy and relationship with peripheral thyroid hormone conversion. Endocr Connect (2015) 4:196–205. doi:10.1530/EC-15-0056
122. Hoermann R, Midgley JEM, Larisch R, Dietrich JW. Is pituitary TSH an adequate measure of thyroid hormone-controlled homoeostasis during thyroxine treatment? Eur J Endocrinol (2013) 168:271–80. doi:10.1530/EJE-12-0819
123. Hoermann R, Midgley JEM, Larisch R, Dietrich JW. Integration of peripheral and glandular regulation of triiodothyronine production by thyrotropin in untreated and thyroxine-treated subjects. Horm Metab Res (2015) 47:674–80. doi:10.1055/s-0034-1398616
124. Wu SY. Thyrotropin-mediated induction of thyroidal iodothyronine monodeiodinases in the dog. Endocrinology (1983) 112:417–24. doi:10.1210/endo-112-2-417
125. Ishii H, Inada M, Tanaka K, Mashio Y, Naito K, Nishikawa M, et al. Induction of outer and inner ring monodeiodinases in human thyroid gland by thyrotropin. J Clin Endocrinol Metab (1983) 57:500–5. doi:10.1210/jcem-57-3-500
126. Köhrle J. Thyrotropin (TSH) action on thyroid hormone deiodination and secretion: one aspect of thyrotropin regulation of thyroid cell biology. Horm Metab Res Suppl (1990) 23:18–28.
127. Murakami M, Kamiya Y, Morimura T, Araki O, Imamura M, Ogiwara T, et al. Thyrotropin receptors in brown adipose tissue: thyrotropin stimulates type II iodothyronine deiodinase and uncoupling protein-1 in brown adipocytes. Endocrinology (2001) 142:1195–201. doi:10.1210/endo.142.3.8012
128. Morimura T, Tsunekawa K, Kasahara T, Seki K, Ogiwara T, Mori M, et al. Expression of type 2 iodothyronine deiodinase in human osteoblast is stimulated by thyrotropin. Endocrinology (2005) 146:2077–84. doi:10.1210/en.2004-1432
129. Koenig RJ. Regulation of type 1 iodothyronine deiodinase in health and disease. Thyroid (2005) 15:835–40. doi:10.1089/thy.2005.15.835
130. Maia AL, Goemann IM, Meyer ELS, Wajner SM. Type 1 iodothyronine deiodinase in human physiology and disease: deiodinases: the balance of thyroid hormone. J Endocrinol (2011) 209:283–97. doi:10.1530/JOE-10-0481
131. Greenspan SL, Klibanski A, Schoenfeld D, Ridgway EC. Pulsatile secretion of thyrotropin in man. J Clin Endocrinol Metab (1986) 63:661–8. doi:10.1210/jcem-63-3-661
132. Lalli E, Sassone-Corsi P. Thyroid-stimulating hormone (TSH)-directed induction of the CREM gene in the thyroid gland participates in the long-term desensitization of the TSH receptor. Proc Natl Acad Sci U S A (1995) 92:9633–7. doi:10.1073/pnas.92.21.9633
133. Nagayama Y, Tanaka K, Hara T, Namba H, Yamashita S, Taniyama K, et al. Involvement of G protein-coupled receptor kinase 5 in homologous desensitization of the thyrotropin receptor. J Biol Chem (1996) 271:10143–8. doi:10.1074/jbc.271.17.10143
134. Saji M, Ikuyama S, Akamizu T, Kohn LD. Increases in cytosolic Ca++ down regulate thyrotropin receptor gene expression by a mechanism different from the cAMP signal. Biochem Biophys Res Commun (1991) 176:94–101. doi:10.1016/0006-291X(91)90894-D
135. Donadio S, Pascual A, Thijssen JHH, Ronin C. Feasibility study of new calibrators for thyroid-stimulating hormone (TSH) immunoprocedures based on remodeling of recombinant TSH to mimic glycoforms circulating in patients with thyroid disorders. Clin Chem (2006) 52:286–97. doi:10.1373/clinchem.2005.058172
136. Persani L, Borgato S, Romoli R, Asteria C, Pizzocaro A, Beck-Peccoz P. Changes in the degree of sialylation of carbohydrate chains modify the biological properties of circulating thyrotropin isoforms in various physiological and pathological states. J Clin Endocrinol Metab (1998) 83:2486–92. doi:10.1210/jc.83.7.2486
137. Maes M, Mommen K, Hendrickx D, Peeters D, D’Hondt P, Ranjan R, et al. Components of biological variation, including seasonality, in blood concentrations of TSH, TT3, FT4, PRL, cortisol and testosterone in healthy volunteers. Clin Endocrinol (Oxf) (1997) 46:587–98. doi:10.1046/j.1365-2265.1997.1881002.x
138. Russell W, Harrison RF, Smith N, Darzy K, Shalet S, Weetman AP, et al. Free triiodothyronine has a distinct circadian rhythm that is delayed but parallels thyrotropin levels. J Clin Endocrinol Metab (2008) 93:2300–6. doi:10.1210/jc.2007-2674
139. Roelfsema F, Pereira AM, Adriaanse R, Endert E, Fliers E, Romijn JA, et al. Thyrotropin secretion in mild and severe primary hypothyroidism is distinguished by amplified burst mass and basal secretion with increased spikiness and approximate entropy. J Clin Endocrinol Metab (2010) 95:928–34. doi:10.1210/jc.2009-1959
140. Denereaz N, Lemarchand-Beraud T. Severe but not mild alterations of thyroid function modulate the density of thyroid-stimulating hormone receptors in the rat thyroid gland. Endocrinology (1995) 136:1694–700. doi:10.1210/endo.136.4.7895680
141. Mariotti S, Barbesino G, Caturegli P, Bartalena L, Sansoni P, Fagnoni F, et al. Complex alteration of thyroid function in healthy centenarians. J Clin Endocrinol Metab (1993) 77:1130–4. doi:10.1210/jcem.77.5.8077303
142. Mariotti S, Franceschi C, Cossarizza A. The aging thyroid. Endocr Rev (1995) 16:686–715. doi:10.1210/edrv-16-6-686
143. Bowers J, Terrien J, Clerget-Froidevaux MS, Gothié JD, Rozing MP, Westendorp RGJ, et al. Thyroid hormone signaling and homeostasis during aging. Endocr Rev (2013) 34:556–89. doi:10.1210/er.2012-1056
144. Johner SA, Thamm M, Stehle P, Nöthlings U, Kriener E, Völzke H, et al. Interrelations between thyrotropin levels and iodine status in thyroid-healthy children. Thyroid (2014) 24:1071–9. doi:10.1089/thy.2013.0480
145. Führer D, Brix K, Biebermann H. [Thyroid hormone action beyond classical concepts. The priority programme “Thyroid Trans Act” (SPP 1629) of the German Research Foundation]. DMW (2014) 139:492–6. doi:10.1055/s-0034-1369822
146. Zucchi R, Chiellini G, Scanlan TS, Grandy DK. Trace amine-associated receptors and their ligands. Br J Pharmacol (2006) 149:967–78. doi:10.1038/sj.bjp.0706948
147. Peeters RP, Wouters PJ, van Toor H, Kaptein E, Visser TJ, Van den Berghe G. Serum 3,3’,5’-triiodothyronine (rT3) and 3,5,3’-triiodothyronine/rT3 are prognostic markers in critically ill patients and are associated with postmortem tissue deiodinase activities. J Clin Endocrinol Metab (2005) 90:4559–65. doi:10.1210/jc.2005-0535
148. Mendoza A, Navarrete-Ramírez P, Hernández-Puga G, Villalobos P, Holzer G, Renaud JP, et al. 3,5-T2 is an alternative ligand for the thyroid hormone receptor β1. Endocrinology (2013) 154:2948–58. doi:10.1210/en.2013-1030
149. Goglia F. The effects of 3,5-diiodothyronine on energy balance. Front Physiol (2014) 5:528. doi:10.3389/fphys.2014.00528
150. Pinna G, Meinhold H, Hiedra L, Thoma R, Hoell T, Gräf KJ, et al. Elevated 3,5-diiodothyronine concentrations in the sera of patients with nonthyroidal illnesses and brain tumors. J Clin Endocrinol Metab (1997) 82:1535–42. doi:10.1210/jcem.82.5.3939
151. Dietrich JW, Müller P, Schiedat F, Schlömicher M, Strauch J, Chatzitomaris A, et al. Nonthyroidal illness syndrome in cardiac illness involves elevated concentrations of 3,5-diiodothyronine and correlates with atrial remodeling. Eur Thyroid J (2015) 4:129–37. doi:10.1159/000381543
152. Engler D, Burger AG. The deiodination of the iodothyronines and of their derivatives in man. Endocr Rev (1984) 5:151–84. doi:10.1210/edrv-5-2-151
153. Piehl S, Hoefig CS, Scanlan TS, Köhrle J. Thyronamines – past, present, and future. Endocr Rev (2010) 32:1–17. doi:10.1210/er.2009-0040
154. Soffer RL, Hechtman P, Savage M. L-Triiodothyronine aminotransferase. J Biol Chem (1973) 248:1224–30.
155. Hoefig CS, Wuensch T, Rijntjes E, Lehmphul I, Daniel H, Schweizer U, et al. Biosynthesis of 3-iodothyronamine from T4 in murine intestinal tissue. Endocrinology (2015) 156:4356–64. doi:10.1210/en.2014-1499
156. Messier N, Laflamme L, Hamann G, Langlois MF. In vitro effect of TRIAC on resistance to thyroid hormone receptor mutants: potential basis for therapy. Mol Cell Endocrinol (2001) 174:59–69. doi:10.1016/S0303-7207(00)00446-9
157. Lameloise N, Siegrist-Kaiser C, O’Connell M, Burger A. Differences between the effects of thyroxine and tetraiodothyroacetic acid on TSH suppression and cardiac hypertrophy. Eur J Endocrinol (2001) 144:145–54. doi:10.1530/eje.0.1440145
158. Kunitake JM, Hartman N, Henson LC, Lieberman J, Williams DE, Wong M, et al. 3,5,3’-triiodothyroacetic acid therapy for thyroid hormone resistance. J Clin Endocrinol Metab (1989) 69:461–6. doi:10.1210/jcem-69-2-461
159. Galli E, Marchini M, Saba A, Berti S, Tonacchera M, Vitti P, et al. Detection of 3-iodothyronamine in human patients: a preliminary study. J Clin Endocrinol Metab (2011) 97:E69–74. doi:10.1210/jc.2011-1115
160. Dinter J, Mühlhaus J, Jacobi SF, Wienchol CL, Cöster M, Meister J, et al. 3-iodothyronamine differentially modulates α-2A-adrenergic receptor-mediated signaling. J Mol Endocrinol (2015) 54:205–16. doi:10.1530/JME-15-0003
161. Liang H, Juge-Aubry CE, O’Connell M, Burger AG. Organ-specific effects of 3,5,3’-triiodothyroacetic acid in rats. Eur J Endocrinol (1997) 137:537–44. doi:10.1530/eje.0.1370537
162. Padron AS, Neto RAL, Pantaleão TU, de Souza dos Santos MC, Araujo RL, de Andrade BM, et al. Administration of 3,5-diiodothyronine (3,5-T2) causes central hypothyroidism and stimulates thyroid-sensitive tissues. J Endocrinol (2014) 221:415–27. doi:10.1530/JOE-13-0502
163. Jonas W, Lietzow J, Wohlgemuth F, Hoefig CS, Wiedmer P, Schweizer U, et al. 3,5-Diiodo-L-thyronine (3,5-T2) exerts thyromimetic effects on hypothalamus-pituitary-thyroid axis, body composition, and energy metabolism in male diet-induced obese mice. Endocrinology (2015) 156:389–99. doi:10.1210/en.2014-1604
164. Caldwell G, Kellett HA, Gow SM, Beckett GJ, Sweeting VM, Seth J, et al. A new strategy for thyroid function testing. Lancet (1985) 1:1117–9. doi:10.1016/S0140-6736(85)92429-8
165. Dietrich JW, Fischer M, Jauch J, Pantke E, Gaertner R, Pickardt CR. SPINA-THYR: a novel systems theoretic approach to determine the secretion capacity of the thyroid gland. Europ J Int Med (1999) 10(Suppl 1):S34.
166. Midgley JEM, Hoermann R. Measurement of total rather than free thyroxine in pregnancy: the diagnostic implications. Thyroid (2013) 23:259–61. doi:10.1089/thy.2012.0469
167. Laurberg P, Andersen S, Carlé A, Karmisholt J, Knudsen N, Pedersen IB. The TSH upper reference limit: where are we at? Nat Rev Endocrinol (2011) 7:232–9. doi:10.1038/nrendo.2011.13
168. Spencer CA, Schwarzbein D, Guttler RB, LoPresti JS, Nicoloff JT. Thyrotropin (TSH)-releasing hormone stimulation test responses employing third and fourth generation TSH assays. J Clin Endocrinol Metab (1993) 76:494–8. doi:10.1210/jcem.76.2.8432796
169. Walsh JP, Bremner AP, Bulsara MK, O’Leary P, Leedman PJ, Feddema P, et al. Subclinical thyroid dysfunction as a risk factor for cardiovascular disease. Arch Intern Med (2005) 165:2467–72. doi:10.1001/archinte.165.21.2467
170. Haymart MR, Repplinger DJ, Leverson GE, Elson DF, Sippel RS, Jaume JC, et al. Higher serum thyroid stimulating hormone level in thyroid nodule patients is associated with greater risks of differentiated thyroid cancer and advanced tumor stage. J Clin Endocrinol Metab (2008) 93:809–14. doi:10.1210/jc.2007-2215
171. Ochs N, Auer R, Bauer DC, Nanchen D, Gussekloo J, Cornuz J, et al. Meta-analysis: subclinical thyroid dysfunction and the risk for coronary heart disease and mortality. Ann Intern Med (2008) 148:832–45. doi:10.7326/0003-4819-148-11-200806030-00225
172. Waring AC, Harrison S, Samuels MH, Ensrud KE, LeBLanc ES, Hoffman AR, et al. Thyroid function and mortality in older men: a prospective study. J Clin Endocrinol Metab (2012) 97:862–70. doi:10.1210/jc.2011-2684
173. Yeap BB, Alfonso H, Hankey GJ, Flicker L, Golledge J, Norman PE, et al. Higher free thyroxine levels are associated with all-cause mortality in euthyroid older men: the Health In Men Study. Eur J Endocrinol (2013) 169:401–8. doi:10.1530/EJE-13-0306
174. Taylor PN, Razvi S, Pearce SH, Dayan CM. Clinical review: a review of the clinical consequences of variation in thyroid function within the reference range. J Clin Endocrinol Metab (2013) 98:3562–71. doi:10.1210/jc.2013-1315
175. Tognini S, Pasqualetti G, Calsolaro V, Polini A, Caraccio N, Monzani F. Cardiovascular risk and quality of life in elderly people with mild thyroid hormone deficiency. Front Endocrinol (2014) 5:153. doi:10.3389/fendo.2014.00153
176. Gharib H, Tuttle RM, Baskin HJ, Fish LH, Singer PA, McDermott MT. Subclinical thyroid dysfunction: a joint statement on management from the American Association of Clinical Endocrinologists, the American Thyroid Association, and the Endocrine Society. J Clin Endocrinol Metab (2005) 90:581–5; discussion 586–7. doi:10.1210/jc.2004-1231
177. Biondi B, Cooper DS. The clinical significance of subclinical thyroid dysfunction. Endocr Rev (2008) 29:76–131. doi:10.1210/er.2006-0043
178. Pearce EN, Hennessey JV, McDermott MT. New American Thyroid Association and American Association of Clinical Endocrinologists guidelines for thyrotoxicosis and other forms of hyperthyroidism: significant progress for the clinician and a guide to future research. Thyroid (2011) 21:573–6. doi:10.1089/thy.2011.0104
179. Völzke H, Lüdemann J, Robinson DM, Spieker KW, Schwahn C, Kramer A, et al. The prevalence of undiagnosed thyroid disorders in a previously iodine-deficient area. Thyroid (2003) 13:803–10. doi:10.1089/105072503768499680
180. Wartofsky L, Dickey RA. The evidence for a narrower thyrotropin reference range is compelling. J Clin Endocrinol Metab (2005) 90:5483–8. doi:10.1210/jc.2005-0455
181. Brabant G, Beck-Peccoz P, Jarzab B, Laurberg P, Orgiazzi J, Szabolcs I, et al. Is there a need to redefine the upper normal limit of TSH? Eur J Endocrinol (2006) 154:633–7. doi:10.1530/eje.1.02136
182. Hoermann R, Midgley JEM. TSH measurement and its implications for personalised clinical decision-making. J Thyroid Res (2012) 2012:1–9. doi:10.1089/thy.2008.0155
183. Spencer CA, Hollowell JG, Kazarosyan M, Braverman LE. National Health and Nutrition Examination Survey III thyroid-stimulating hormone (TSH)-thyroperoxidase antibody relationships demonstrate that TSH upper reference limits may be skewed by occult thyroid dysfunction. J Clin Endocrinol Metab (2007) 92:4236–40. doi:10.1210/jc.2007-0287
184. Surks MI, Hollowell JG. Age-specific distribution of serum thyrotropin and antithyroid antibodies in the US population: implications for the prevalence of subclinical hypothyroidism. J Clin Endocrinol Metab (2007) 92:4575–82. doi:10.1210/jc.2007-1499
185. Andersen S, Bruun NH, Pedersen KM, Laurberg P. Biologic variation is important for interpretation of thyroid function tests. Thyroid (2003) 13:1069–78. doi:10.1089/105072503770867237
186. Karmisholt J, Andersen S, Laurberg P. Interval between tests and thyroxine estimation method influence outcome of monitoring of subclinical hypothyroidism. J Clin Endocrinol Metab (2008) 93:1634–40. doi:10.1210/jc.2008-0101
187. Karmisholt J, Andersen S, Laurberg P. Variation in thyroid function tests in patients with stable untreated subclinical hypothyroidism. Thyroid (2008) 18:303–8. doi:10.1089/thy.2007.0241
188. Karmisholt J, Andersen S, Laurberg P. Analytical goals for thyroid function tests when monitoring patients with untreated subclinical hypothyroidism. Scand J Clin Lab Invest (2010) 70:264–8. doi:10.3109/00365511003782778
189. Karmisholt J, Andersen S, Laurberg P. Variation in thyroid function in subclinical hypothyroidism: importance of clinical follow-up and therapy. Eur J Endocrinol (2011) 164:317–23. doi:10.1530/EJE-10-1021
190. Andersen S, Pedersen KM, Bruun NH, Laurberg P. Narrow individual variations in serum T(4) and T(3) in normal subjects: a clue to the understanding of subclinical thyroid disease. J Clin Endocrinol Metab (2002) 87:1068–72. doi:10.1210/jcem.87.3.8165
191. Kagedal B, Sandstroem A, Tibbling G. Determination of a trivariate reference region for free thyroxine index, free triiodothyronineindex, and thyrotropinfrom results obtained in a health survey of middle-aged women. Clin Chem (1978) 24:11744–50.
192. Meier C, Maisey MN, Lowry A, Müller J, Smith MA. Interindividual differences in the pituitary-thyroid axis influence the interpretation of thyroid function tests. Clin Endocrinol (Oxf) (1993) 39:101–7. doi:10.1111/j.1365-2265.1993.tb01758.x
193. Jostel A, Ryder WDJ, Shalet SM. The use of thyroid function tests in the diagnosis of hypopituitarism: definition and evaluation of the TSH Index. Clin Endocrinol (Oxf) (2009) 71:529–34. doi:10.1111/j.1365-2265.2009.03534.x
194. Ross HA, Heijer den M, Hermus ARMM, Sweep FCGJ. Composite reference interval for thyroid-stimulating hormone and free thyroxine, comparison with common cutoff values, and reconsideration of subclinical thyroid disease. Clin Chem (2009) 55:2019–25. doi:10.1373/clinchem.2009.124560
195. Leow MKS, Goede SL. The homeostatic set point of the hypothalamus-pituitary-thyroid axis – maximum curvature theory for personalized euthyroid targets. Theor Biol Med Model (2014) 11:1–35. doi:10.1186/1742-4682-11-35
196. Fish LH, Schwartz HL, Cavanaugh J, Steffes MW, Bantle JP, Oppenheimer JH. Replacement dose, metabolism, and bioavailability of levothyroxine in the treatment of hypothyroidism. N Engl J Med (1987) 316:764–70. doi:10.1056/NEJM198703263161302
197. Woeber KA. Levothyroxine therapy and serum free thyroxine and free triiodothyronine concentrations. J Endocrinol Invest (2002) 25:106–9. doi:10.1007/BF03343972
198. Gullo D, Latina A, Frasca F, Le Moli R, Pellegriti G, Vigneri R. Levothyroxine monotherapy cannot guarantee euthyroidism in all athyreotic patients. PLoS One (2011) 6:e22552. doi:10.1371/journal.pone.0022552
199. Ito M, Miyauchi A, Morita S, Kudo T, Nishihara E, Kihara M, et al. TSH-suppressive doses of levothyroxine are required to achieve preoperative native serum triiodothyronine levels in patients who have undergone total thyroidectomy. Eur J Endocrinol (2012) 167:373–8. doi:10.1530/EJE-11-1029
200. Ito M, Miyauchi A, Kang S, Hisakado M, Yoshioka W, Ide A, et al. Effect of the presence of remnant thyroid tissue on the serum thyroid hormone balance in thyroidectomized patients. Eur J Endocrinol (2015) 173:1–8. doi:10.1530/EJE-15-0138
201. Solter D, Solter M. Benefit of combined triiodothyronine (LT(3)) and thyroxine (LT(4)) treatment in athyreotic patients unresponsive to LT(4) alone. Exp Clin Endocrinol Diabetes (2012) 120:121–3. doi:10.1055/s-0031-1297253
202. Escobar-Morreale HF, Obregón MJ, Escobar del Rey F, Morereale de Escobar G. Replacement therapy for hypothyroidism with thyroxine alone does not ensure euthyroidism in all tissues, as studied in thyroidectomized rats. J Clin Invest (1995) 96:2828–38. doi:10.1172/JCI118353
203. Escobar-Morreale HF, del Rey FE, Obregón MJ, de Escobar GM. Only the combined treatment with thyroxine and triiodothyronine ensures euthyroidism in all tissues of the thyroidectomized rat. Endocrinology (1996) 137:2490–502. doi:10.1210/endo.137.6.8641203
204. Jansen S, Roelfsema F, van der Spoel E, Akintola A, Postmus I, Ballieux B, et al. Familial longevity is associated with higher tsh secretion and strong TSH-fT3 relationship. J Clin Endocrinol Metab (2015) 100(10):3806–13. doi:10.1210/jc.2015-2624
205. Wiersinga WM. Paradigm shifts in thyroid hormone replacement therapies for hypothyroidism. Nat Rev Endocrinol (2014) 10:164–74. doi:10.1038/nrendo.2013.258
206. Grozinsky-Glasberg S, Fraser A, Nahshoni E, Weizman A, Leibovici L. Thyroxine-triiodothyronine combination therapy versus thyroxine monotherapy for clinical hypothyroidism: meta-analysis of randomized controlled trials. J Clin Endocrinol Metab (2006) 91:2592–9. doi:10.1210/jc.2006-0448
207. Fraser CG, Hyltoft Peterson P, Larsen ML. Setting analytical goals for random analytical error in specific clinical monitoring situations. Clin Chem (1990) 36:1625–8.
208. Biondi B, Wartofsky L. Combination treatment with T4 and T3: toward personalized replacement therapy in hypothyroidism? J Clin Endocrinol Metab (2012) 97:2256–71. doi:10.1210/jc.2011-3399
209. Jonklaas J, Burman KD, Bianco AC. American Thyroid Association Spring 2013 Program Committee. Treatment of hypothyroidism: possibilities on the horizon. Thyroid (2013) 23:ix–xi. doi:10.1089/thy.2013.0171
210. Biondi B, Wartofsky L. Treatment with thyroid hormone. Endocr Rev (2014) 35:433–512. doi:10.1210/er.2013-1083
211. Burch HB, Burman KD, Cooper DS, Hennessey JV. A 2013 survey of clinical practice patterns in the management of primary hypothyroidism. J Clin Endocrinol Metab (2014) 99:2077–85. doi:10.1210/jc.2014-1046
Keywords: homeostasis, feedback regulation, TSH, thyroid hormones, deiodinase, set point
Citation: Hoermann R, Midgley JEM, Larisch R and Dietrich JW (2015) Homeostatic Control of the Thyroid–Pituitary Axis: Perspectives for Diagnosis and Treatment. Front. Endocrinol. 6:177. doi: 10.3389/fendo.2015.00177
Received: 15 September 2015; Accepted: 04 November 2015;
Published: 20 November 2015
Edited by:
Jacqueline Jonklaas, Georgetown University, USAReviewed by:
Tania M. Ortiga-Carvalho, Universidade Federal do Rio de Janeiro, BrazilJoanna Klubo-Gwiezdzinska, National Institutes of Health, USA
Copyright: © 2015 Hoermann, Midgley, Larisch and Dietrich. This is an open-access article distributed under the terms of the Creative Commons Attribution License (CC BY). The use, distribution or reproduction in other forums is permitted, provided the original author(s) or licensor are credited and that the original publication in this journal is cited, in accordance with accepted academic practice. No use, distribution or reproduction is permitted which does not comply with these terms.
*Correspondence: Johannes W. Dietrich, am9oYW5uZXMuZGlldHJpY2gmI3gwMDA0MDtydWhyLXVuaS1ib2NodW0uZGU=