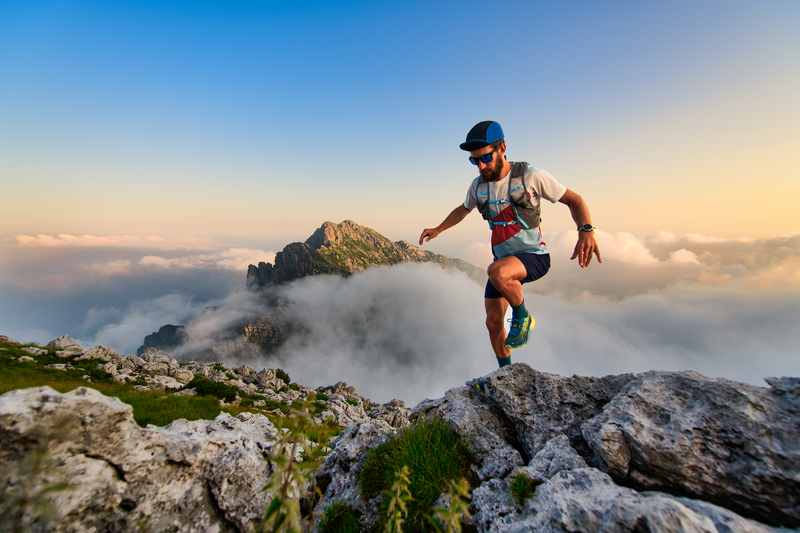
95% of researchers rate our articles as excellent or good
Learn more about the work of our research integrity team to safeguard the quality of each article we publish.
Find out more
OPINION article
Front. Endocrinol. , 24 April 2015
Sec. Systems Endocrinology
Volume 6 - 2015 | https://doi.org/10.3389/fendo.2015.00055
This article is part of the Research Topic Linking hypoxia to obesity View all 6 articles
The number of studies in the field of adipose tissue biology has increased exponentially over the last decade. This shift in research focus is primarily driven by the tremendous increase in the prevalence of obesity and related chronic diseases, including cardiovascular disease and type 2 diabetes mellitus. Adipose tissue is a fascinating and complex organ, with marked effects on whole-body physiology. Intriguingly, expansion of adipose tissue does not necessarily translate into increased metabolic and cardiovascular disease risk. A proportion of obese individuals seems to be relatively protected against worsening of metabolic health (1), suggesting that adipose tissue dysfunction, rather than the amount of fat mass, may be a key factor in the pathophysiology of obesity-related metabolic and cardiovascular diseases (2–4). It is widely accepted that impairments in adipose tissue lipid metabolism, a decreased adipose tissue blood flow (ATBF) and an increased production of pro-inflammatory cytokines by hypertrophic adipocytes and infiltrating adaptive and innate immune cells are characteristics of dysfunctional adipose tissue in obesity (2, 5). These impairments not only induce insulin resistance locally in the adipose tissue but also have detrimental effects at the whole-body level, thereby affecting metabolic health. The reason for this is that adipose tissue dysfunction in obesity is accompanied by lipid spillover in the circulation and subsequent lipid accumulation in non-adipose tissues (ectopic fat storage), and may contribute to systemic low-grade inflammation, thereby accelerating the development and progression of obesity-related insulin resistance and chronic metabolic diseases (Figure 1) (2).
Figure 1. Adipose tissue dysfunction in obesity is related to impaired metabolic health. A long-term positive energy balance, leading to body weight gain, will increase adipocyte size. Adipocyte hypertrophy in obesity is accompanied by disturbances in lipid metabolism and alterations in adipokine secretion, which a shift toward a pro-inflammatory phenotype. The secretion of pro-inflammatory factors, which also impair adipocyte differentiation, is further boosted by the infiltration of several adaptive and innate immune cells into the adipose tissue in obesity. Together, the impairments in lipid metabolism and the secretory function of adipose tissue not only induce insulin resistance locally within the tissue (via autocrine/paracrine effects) but also have detrimental effects at the whole-body level. The reason for this is that adipose tissue dysfunction in obesity is accompanied by lipid spillover in the circulation and subsequent lipid accumulation in non-adipose tissues (ectopic fat storage), and may contribute to systemic low-grade inflammation, thereby accelerating the development and progression of obesity-related insulin resistance and chronic diseases.
Since adipose tissue dysfunction has been recognized as a key process in the pathophysiology of obesity-related disorders (2, 5), the number of studies aimed at identifying the trigger of adipose tissue dysfunction in obesity has increased substantially. A prevailing concept is that an insufficient amount of oxygen within adipose tissue, commonly referred to as “adipose tissue hypoxia,” may underlie adipose tissue dysfunction in obesity (6, 7).
It has been postulated that adipose tissue angiogenesis is insufficient to maintain normoxia in the entire fat depot during the progressive development of obesity (8). In other words, a reduced supply of oxygen to the tissue has been proposed to instigate adipose tissue dysfunction. Indeed, a lower expression of angiogenic genes (e.g., VEGF) and lower capillary density have been found in abdominal subcutaneous adipose tissue of obese as compared to lean individuals (9, 10). The net result of structural and functional properties of the adipose tissue vasculature determines tissue blood flow. A consistent observation made by our lab and others is that fasting and postprandial ATBF is decreased in obese insulin resistant versus lean insulin sensitive subjects (9, 11–13), indicating that oxygen delivery to adipose tissue is indeed impaired in obesity. We have recently demonstrated, for the first time, that both pharmacological (local administration of vasoactive agents into adipose tissue) and physiological (oral glucose drink) manipulation of ATBF induce concomitant alterations in adipose tissue oxygen partial pressure (AT PO2) in humans (9), indicating that adipose tissue oxygen supply indeed affects AT PO2. A second argument that has been put forward to develop the concept of adipose tissue hypoxia in obesity is that the diameter of hypertrophic adipocytes in obesity exceeds the normal diffusion distance of oxygen across tissues (100–200 μm) (14). However, in human adipose tissue, there seems to be only a very small proportion of adipocytes with a diameter >100 μm (9, 15, 16). Therefore, the significance of reduced oxygen diffusion from the capillaries to hypertrophic fat cells in obese humans can be questioned.
Several rodent studies have been performed to investigate whether adipose tissue hypoxia is present in obesity. These experiments have shown that massive and rapid weight gain in ob/ob, KKAy, and dietary-induced obese mice was accompanied by increased expression of hypoxia-responsive genes, more hypoxic areas (assessed using pimonidazole hydrochloride) and lower PO2 in white adipose tissue (17–19). Thus, multiple lines of evidence suggest that AT PO2 is lower in rodent models of obesity as compared to lean control animals. Importantly, impaired angiogenesis might be a more important determinant of AT PO2 in animal models of obesity than in human obesity, since the rate and extent of fat mass gain is much higher in mouse models of obesity (20). Intriguingly, there is only marginal evidence to substantiate the view that a relative oxygen deficit is present in adipose tissue in human obesity. So far, three studies have addressed whether abdominal subcutaneous adipose tissue of obese humans is “hypoxic” (9, 10, 21), of which two have directly measured AT PO2 (9, 10). First, Pasarica and colleagues (10) have found that AT PO2 (measured using polarographic Clark electrodes) was significantly lower in overweight/obese versus lean subjects. In this study however, groups were not matched for age, gender, ethnicity, and the presence of type 2 diabetes, which may have influenced the results. In contrast, we have recently demonstrated, using continuous optochemical PO2 monitoring, that obese insulin resistant subjects had significantly higher AT PO2 compared to lean insulin sensitive individuals, matched for age, gender, and ethnicity, despite significantly lower ATBF in the obese (9). Finally, Hodson and co-workers (21) have recently assessed a metabolic signature of abdominal subcutaneous adipose tissue by arterio-venous difference methodology. If large areas of adipose tissue would be “hypoxic” in obesity, this is likely reflected by a switch to anaerobic metabolism, i.e., an increased secretion of lactate and pyruvate from adipose tissue into the venous blood draining adipose tissue. However, the authors did not find any evidence for a metabolic signature typical for adipose tissue hypoxia in obese humans. Currently, we are investigating for the first time whether diet-induced weight loss will alter AT PO2 in overweight and obese individuals. This study will provide further insight into AT PO2 in human obesity.
What could explain a higher AT PO2, if indeed present, in human obesity? Since AT PO2 is the result of the balance between oxygen supply (ATBF) and the metabolic rate of the tissue (20), it may be that adipose tissue oxygen consumption is substantially lower in obesity. Indeed, animal studies have shown that mitochondrial morphology is abnormal, that mitochondrial biogenesis and mass are reduced, and that oxygen consumption is lower in both white and brown adipose tissue of obese Zucker rats (22), ob/ob mice (23), db/db, and high-fat diet-fed mice (24). In line, microarray-based gene expression profiling has demonstrated that increases in fat mass were paralleled by progressive downregulation of metabolic pathways, including mitochondrial energy metabolism, and upregulation of inflammatory pathways in both visceral and abdominal subcutaneous adipose tissue in humans (25). In accordance, data from our lab and others seem to indicate that in vivo adipose tissue oxygen consumption is lower in obese than lean subjects (9, 21). More recently, it has been demonstrated that human adipocyte mitochondrial content and mitochondrial oxygen consumption by white adipocytes is lower in obese compared to lean subjects (26). Interestingly, the latter study found that both small and large white adipocytes of obese individuals have a lower metabolic rate compared to adipocytes derived from lean donors (26), suggesting that impaired mitochondrial oxygen consumption might be a primary factor that contributes to adipocyte hypertrophy. Taken together, there is substantial evidence that the metabolic rate of adipose tissue is lower in obese humans, which may underlie the higher AT PO2 that we have previously found in obese humans (9). Clearly, it is the balance between oxygen supply and consumption that determines AT PO2 (20).
Many in vitro studies have been performed to investigate whether oxygen tension is involved in the regulation of metabolic and inflammatory processes in adipose tissue, as extensively reviewed elsewhere (6, 20). Earlier studies examined the effects of acute, short-term (1–24 h) exposure to extremely low PO2 (usually 1% O2) as compared to a ~20-fold higher O2 concentration (ambient air, 21% O2) on the expression and/or secretion of key adipokines involved in inflammation and metabolism. Most of these studies have demonstrated that extremely low PO2 induces a pro-inflammatory response in 3T3-L1 adipocytes (18, 27–31), human adipocytes (32), stromal–vascular cells (33, 34), and macrophages (18, 35), although conflicting results have also been reported (36, 37). On the other hand, 95% O2 also increased pro-inflammatory gene expression and reactive oxygen species (ROS) content, and reduced glucose uptake in 3T3-L1 adipocytes (38). These in vitro studies should be interpreted with some caution, because these cells were acutely exposed to extremely low (or high) PO2. In addition, two independent laboratories, including ours, have recently provided evidence that human abdominal subcutaneous AT PO2 ranges between ~3 and 11% O2 (~23–84 mmHg) (9, 10). Therefore, cell culture experiments investigating the effects of chronic exposure to more physiological PO2 are urgently warranted.
Studies that have examined the relationship between in vivo AT PO2 and the inflammatory phenotype of adipose tissue in humans have yielded conflicting results, showing both positive (9) and inverse (10) correlations. Interestingly, human primary adipocytes have recently been exposed to physiological PO2 levels (5 versus 10 versus 21% O2) during differentiation (14 days) (39). Of note, 5 and 10% O2 reflect the mean AT PO2 values that we have previously found in lean insulin sensitive and obese insulin resistant individuals, respectively (9). The authors were able to demonstrate that exposure to 10% O2 increased adipocyte triacylglycerol (TAG) content and boosted the secretion rates of IL-6 and DPP-4 (39). Interestingly, DPP-4 is involved in cross talk between adipose tissue and skeletal muscle, and has been shown to inhibit skeletal muscle insulin signaling (40).
Based on the human data from our laboratory, showing increased AT PO2 in obese insulin resistant subjects, a positive correlation between AT PO2 and adipose tissue gene expression of several pro-inflammatory markers, and an inverse association between AT PO2 and peripheral insulin sensitivity (9), we questioned whether chronic hypoxia exposure would have beneficial effects on the adipose tissue inflammatory and metabolic phenotype. Therefore, we exposed 52-week-old C57Bl/6J mice to chronic hypoxia (8% O2) or normoxia (21% O2) for 21 days, after which adipose tissue and plasma were collected. Chronic hypoxia exposure improved adipose tissue function in these mice, evidenced by decreased adipocyte size, increased adipose tissue gene expression of mitochondrial function markers, and decreased adipose tissue macrophage infiltration and gene expression of inflammatory markers (41), which may contribute to improved insulin sensitivity. More recently, the same concept has been applied to humans. Interestingly, exposure to moderate hypoxia (15% O2) for 10 subsequent nights significantly increased whole-body insulin sensitivity in obese men (42). Although not commented upon by the authors, moderate hypoxia exposure also tended to reduce AT PO2 (42). Therefore, these findings may imply that the decreased AT PO2 after moderate hypoxia exposure for 10 consecutive nights has contributed or even driven the improved peripheral insulin sensitivity, as recently postulated (43). Importantly, obstructive sleep apnea syndrome (OSAS), which is characterized by cycles of severe intermittent hypoxia resulting from periodic collapse of the upper airway during sleep, is an independent risk factor for insulin resistance (44, 45), and treatment with continuous positive airway pressure (CPAP) reverses several metabolic abnormalities in OSAS patients (46). Therefore, it might be that the severity, pattern, and duration of hypoxia exposure may determine the effects on metabolic and cardiovascular health.
Adipose tissue dysfunction in obesity is a key factor in the pathophysiology of obesity-related chronic metabolic and cardiovascular diseases. Recent studies have indicated that alterations in AT PO2 may drive adipose tissue dysfunction in obesity, although many questions remain. Clearly, more clinical observational and intervention studies in well-phenotyped humans are needed to further investigate AT PO2 in obesity, as well as the functional consequences of altered AT PO2. Important aspects that need to be taken into account in future studies are the severity, duration, and pattern of PO2 exposure, since different experimental conditions may underlie different study outcomes. Moreover, it is of particular interest to examine fat depot-differences (e.g., lower-body versus upper-body) in AT PO2, and to unravel whether metabolic and inflammatory responses to chronic physiological O2 levels are related to specific fat depots, cell types [e.g., (pre)adipocyte, stromal–vascular cells, macrophages], and/or donor characteristics. In addition, it would be important to understand whether AT PO2 relates more strongly to the metabolic phenotype (e.g., insulin resistance) in obesity than to the increased adipose tissue mass itself. Finally, human intervention studies need to be undertaken to determine whether AT PO2 can be modified (e.g., weight loss, oxygen therapy) and will subsequently evoke alterations in the metabolic and inflammatory phenotype. These studies should ideally be of integrative nature, combining in vivo assessment of adipose tissue and whole-body physiology with measurements in tissue biopsies and cell culture experiments. Studies in this exciting field of research may lead to new opportunities for therapeutic intervention in obese individuals with dysfunctional adipose tissue, thereby improving cardiometabolic health.
GG drafted the manuscript. GG and EB revised the manuscript and gave final approval of the manuscript to be published.
The authors declare that the research was conducted in the absence of any commercial or financial relationships that could be construed as a potential conflict of interest.
The authors are grateful to the colleagues who have contributed to the studies performed by our research group and cited in this article, and would like to thank the Dutch Diabetes Research Foundation (Innovative Pilot Research Grant, grant No. 2008.11.010 to GG) and the European Foundation for the Study of Diabetes (EFSD Clinical Research Grants Award 2013 to GG) for financial support.
1. Bluher M. Adipose tissue dysfunction contributes to obesity related metabolic diseases. Best Pract Res Clin Endocrinol Metab (2013) 27:163–77. doi: 10.1016/j.beem.2013.02.005
Pubmed Abstract | Pubmed Full Text | CrossRef Full Text | Google Scholar
2. Goossens GH. The role of adipose tissue dysfunction in the pathogenesis of obesity-related insulin resistance. Physiol Behav (2008) 94:206–18. doi:10.1016/j.physbeh.2007.10.010
Pubmed Abstract | Pubmed Full Text | CrossRef Full Text | Google Scholar
3. Berg AH, Scherer PE. Adipose tissue, inflammation, and cardiovascular disease. Circ Res (2005) 96:939–49. doi:10.1161/01.RES.0000163635.62927.34
Pubmed Abstract | Pubmed Full Text | CrossRef Full Text | Google Scholar
4. Browning JD, Horton JD. Molecular mediators of hepatic steatosis and liver injury. J Clin Invest (2004) 114:147–52. doi:10.1172/JCI22422
Pubmed Abstract | Pubmed Full Text | CrossRef Full Text | Google Scholar
5. Rosen ED, Spiegelman BM. What we talk about when we talk about fat. Cell (2014) 156:20–44. doi:10.1016/j.cell.2013.12.012
Pubmed Abstract | Pubmed Full Text | CrossRef Full Text | Google Scholar
6. Trayhurn P. Hypoxia and adipocyte physiology: implications for adipose tissue dysfunction in obesity. Annu Rev Nutr (2014) 34:207–36. doi:10.1146/annurev-nutr-071812-161156
Pubmed Abstract | Pubmed Full Text | CrossRef Full Text | Google Scholar
7. Ye J. Emerging role of adipose tissue hypoxia in obesity and insulin resistance. Int J Obes (Lond) (2009) 33:54–66. doi:10.1038/ijo.2008.229
Pubmed Abstract | Pubmed Full Text | CrossRef Full Text | Google Scholar
8. Trayhurn P, Wood IS. Adipokines: inflammation and the pleiotropic role of white adipose tissue. Br J Nutr (2004) 92:347–55. doi:10.1079/BJN20041213
Pubmed Abstract | Pubmed Full Text | CrossRef Full Text | Google Scholar
9. Goossens GH, Bizzarri A, Venteclef N, Essers Y, Cleutjens JP, Konings E, et al. Increased adipose tissue oxygen tension in obese compared with lean men is accompanied by insulin resistance, impaired adipose tissue capillarization, and inflammation. Circulation (2011) 124:67–76. doi:10.1161/CIRCULATIONAHA.111.027813
10. Pasarica M, Sereda OR, Redman LM, Albarado DC, Hymel DT, Roan LE, et al. Reduced adipose tissue oxygenation in human obesity: evidence for rarefaction, macrophage chemotaxis, and inflammation without an angiogenic response. Diabetes (2009) 58:718–25. doi:10.2337/db08-1098
Pubmed Abstract | Pubmed Full Text | CrossRef Full Text | Google Scholar
11. Goossens GH, Jocken JW, Blaak EE, Schiffers PM, Saris WH, van Baak MA. Endocrine role of the renin-angiotensin system in human adipose tissue and muscle: effect of beta-adrenergic stimulation. Hypertension (2007) 49:542–7. doi:10.1161/01.HYP.0000256091.55393.92
Pubmed Abstract | Pubmed Full Text | CrossRef Full Text | Google Scholar
12. Goossens GH, McQuaid SE, Dennis AL, van Baak MA, Blaak EE, Frayn KN, et al. Angiotensin II: a major regulator of subcutaneous adipose tissue blood flow in humans. J Physiol (2006) 571:451–60. doi:10.1113/jphysiol.2005.101352
Pubmed Abstract | Pubmed Full Text | CrossRef Full Text | Google Scholar
13. Karpe F, Fielding BA, Ilic V, Macdonald IA, Summers LK, Frayn KN. Impaired postprandial adipose tissue blood flow response is related to aspects of insulin sensitivity. Diabetes (2002) 51:2467–73. doi:10.2337/diabetes.51.8.2467
Pubmed Abstract | Pubmed Full Text | CrossRef Full Text | Google Scholar
14. Brahimi-Horn MC, Pouyssegur J. Oxygen, a source of life and stress. FEBS Lett (2007) 581:3582–91. doi:10.1016/j.febslet.2007.06.018
Pubmed Abstract | Pubmed Full Text | CrossRef Full Text | Google Scholar
15. Goossens GH, Moors CC, van der Zijl NJ, Venteclef N, Alili R, Jocken JW, et al. Valsartan improves adipose tissue function in humans with impaired glucose metabolism: a randomized placebo-controlled double-blind trial. PLoS One (2012) 7:e39930. doi:10.1371/journal.pone.0039930
Pubmed Abstract | Pubmed Full Text | CrossRef Full Text | Google Scholar
16. Skurk T, Alberti-Huber C, Herder C, Hauner H. Relationship between adipocyte size and adipokine expression and secretion. J Clin Endocrinol Metab (2007) 92:1023–33. doi:10.1210/jc.2006-1055
Pubmed Abstract | Pubmed Full Text | CrossRef Full Text | Google Scholar
17. Rausch ME, Weisberg S, Vardhana P, Tortoriello DV. Obesity in C57BL/6J mice is characterized by adipose tissue hypoxia and cytotoxic T-cell infiltration. Int J Obes (2008) 32:451–63. doi:10.1038/sj.ijo.0803744
Pubmed Abstract | Pubmed Full Text | CrossRef Full Text | Google Scholar
18. Ye J, Gao Z, Yin J, He Q. Hypoxia is a potential risk factor for chronic inflammation and adiponectin reduction in adipose tissue of ob/ob and dietary obese mice. Am J Physiol Endocrinol Metab (2007) 293:E1118–28. doi:10.1152/ajpendo.00435.2007
Pubmed Abstract | Pubmed Full Text | CrossRef Full Text | Google Scholar
19. Yin J, Gao Z, He Q, Zhou D, Guo Z, Ye J. Role of hypoxia in obesity-induced disorders of glucose and lipid metabolism in adipose tissue. Am J Physiol Endocrinol Metab (2009) 296:E333–42. doi:10.1152/ajpendo.90760.2008
Pubmed Abstract | Pubmed Full Text | CrossRef Full Text | Google Scholar
20. Goossens GH, Blaak EE. Adipose tissue oxygen tension: implications for chronic metabolic and inflammatory diseases. Curr Opin Clin Nutr Metab Care (2012) 15:539–46. doi:10.1097/MCO.0b013e328358fa87
Pubmed Abstract | Pubmed Full Text | CrossRef Full Text | Google Scholar
21. Hodson L, Humphreys SM, Karpe F, Frayn KN. Metabolic signatures of human adipose tissue hypoxia in obesity. Diabetes (2013) 62:1417–25. doi:10.2337/db12-1032
Pubmed Abstract | Pubmed Full Text | CrossRef Full Text | Google Scholar
22. Levin BE, Finnegan MB, Marquet E, Sullivan AC. Defective brown adipose oxygen consumption in obese Zucker rats. Am J Physiol (1984) 247: E94–100.
23. Wilson-Fritch L, Nicoloro S, Chouinard M, Lazar MA, Chui PC, Leszyk J, et al. Mitochondrial remodeling in adipose tissue associated with obesity and treatment with rosiglitazone. J Clin Invest (2004) 114:1281–9. doi:10.1172/JCI200421752
Pubmed Abstract | Pubmed Full Text | CrossRef Full Text | Google Scholar
24. Rong JX, Qiu Y, Hansen MK, Zhu L, Zhang V, Xie M, et al. Adipose mitochondrial biogenesis is suppressed in db/db and high-fat diet-fed mice and improved by rosiglitazone. Diabetes (2007) 56:1751–60. doi:10.2337/db06-1135
Pubmed Abstract | Pubmed Full Text | CrossRef Full Text | Google Scholar
25. Klimcakova E, Roussel B, Marquez-Quinones A, Kovacova Z, Kovacikova M, Combes M, et al. Worsening of obesity and metabolic status yields similar molecular adaptations in human subcutaneous and visceral adipose tissue: decreased metabolism and increased immune response. J Clin Endocrinol Metab (2011) 96:E73–82. doi:10.1210/jc.2010-1575
Pubmed Abstract | Pubmed Full Text | CrossRef Full Text | Google Scholar
26. Yin X, Lanza IR, Swain JM, Sarr MG, Nair KS, Jensen MD. Adipocyte mitochondrial function is reduced in human obesity independent of fat cell size. J Clin Endocrinol Metab (2014) 99:E209–16. doi:10.1210/jc.2013-3042
Pubmed Abstract | Pubmed Full Text | CrossRef Full Text | Google Scholar
27. Mazzatti D, Lim FL, O’Hara A, Wood IS, Trayhurn P. A microarray analysis of the hypoxia-induced modulation of gene expression in human adipocytes. Arch Physiol Biochem (2012) 118:112–20. doi:10.3109/13813455.2012.654611
Pubmed Abstract | Pubmed Full Text | CrossRef Full Text | Google Scholar
28. Wree A, Mayer A, Westphal S, Beilfuss A, Canbay A, Schick RR, et al. Adipokine expression in brown and white adipocytes in response to hypoxia. J Endocrinol Invest (2012) 35:522–7. doi:10.3275/7964
Pubmed Abstract | Pubmed Full Text | CrossRef Full Text | Google Scholar
29. Yu J, Shi L, Wang H, Bilan PJ, Yao Z, Samaan MC, et al. Conditioned medium from hypoxia-treated adipocytes renders muscle cells insulin resistant. Eur J Cell Biol (2011) 90:1000–15. doi:10.1016/j.ejcb.2011.06.004
Pubmed Abstract | Pubmed Full Text | CrossRef Full Text | Google Scholar
30. Hosogai N, Fukuhara A, Oshima K, Miyata Y, Tanaka S, Segawa K, et al. Adipose tissue hypoxia in obesity and its impact on adipocytokine dysregulation. Diabetes (2007) 56:901–11. doi:10.2337/db06-0911
31. Chen B, Lam KS, Wang Y, Wu D, Lam MC, Shen J, et al. Hypoxia dysregulates the production of adiponectin and plasminogen activator inhibitor-1 independent of reactive oxygen species in adipocytes. Biochem Biophys Res Commun (2006) 341:549–56. doi:10.1016/j.bbrc.2006.01.004
Pubmed Abstract | Pubmed Full Text | CrossRef Full Text | Google Scholar
32. Wang B, Wood IS, Trayhurn P. Dysregulation of the expression and secretion of inflammation-related adipokines by hypoxia in human adipocytes. Pflugers Arch (2007) 455:479–92. doi:10.1007/s00424-007-0301-8
Pubmed Abstract | Pubmed Full Text | CrossRef Full Text | Google Scholar
33. O’Rourke RW, White AE, Metcalf MD, Olivas AS, Mitra P, Larison WG, et al. Hypoxia-induced inflammatory cytokine secretion in human adipose tissue stromovascular cells. Diabetologia (2011) 54:1480–90. doi:10.1007/s00125-011-2103-y
Pubmed Abstract | Pubmed Full Text | CrossRef Full Text | Google Scholar
34. Rehman J, Traktuev D, Li J, Merfeld-Clauss S, Temm-Grove CJ, Bovenkerk JE, et al. Secretion of angiogenic and antiapoptotic factors by human adipose stromal cells. Circulation (2004) 109:1292–8. doi:10.1161/01.CIR.0000121425.42966.F1
Pubmed Abstract | Pubmed Full Text | CrossRef Full Text | Google Scholar
35. Murdoch C, Muthana M, Lewis CE. Hypoxia regulates macrophage functions in inflammation. J Immunol (2005) 175:6257–63. doi:10.4049/jimmunol.175.10.6257
Pubmed Abstract | Pubmed Full Text | CrossRef Full Text | Google Scholar
36. Geiger K, Leiherer A, Muendlein A, Stark N, Geller-Rhomberg S, Saely CH, et al. Identification of hypoxia-induced genes in human SGBS adipocytes by microarray analysis. PLoS One (2011) 6:e26465. doi:10.1371/journal.pone.0026465
Pubmed Abstract | Pubmed Full Text | CrossRef Full Text | Google Scholar
37. Famulla S, Horrighs A, Cramer A, Sell H, Eckel J. Hypoxia reduces the response of human adipocytes towards TNFalpha resulting in reduced NF-kappaB signaling and MCP-1 secretion. Int J Obes (Lond) (2012) 36:986–92. doi:10.1038/ijo.2011.200
Pubmed Abstract | Pubmed Full Text | CrossRef Full Text | Google Scholar
38. Quintero P, Gonzalez-Muniesa P, Garcia-Diaz DF, Martinez JA. Effects of hyperoxia exposure on metabolic markers and gene expression in 3T3-L1 adipocytes. J Physiol Biochem (2012) 68:663–9. doi:10.1007/s13105-012-0169-8
Pubmed Abstract | Pubmed Full Text | CrossRef Full Text | Google Scholar
39. Famulla S, Schlich R, Sell H, Eckel J. Differentiation of human adipocytes at physiological oxygen levels results in increased adiponectin secretion and isoproterenol-stimulated lipolysis. Adipocyte (2012) 1:132–81. doi:10.4161/adip.19962
Pubmed Abstract | Pubmed Full Text | CrossRef Full Text | Google Scholar
40. Lamers D, Famulla S, Wronkowitz N, Hartwig S, Lehr S, Ouwens DM, et al. Dipeptidyl peptidase 4 is a novel adipokine potentially linking obesity to the metabolic syndrome. Diabetes (2011) 60:1917–25. doi:10.2337/db10-1707
Pubmed Abstract | Pubmed Full Text | CrossRef Full Text | Google Scholar
41. van den Borst B, Schols AM, de Theije C, Boots AW, Kohler SE, Goossens GH, et al. Characterization of the inflammatory and metabolic profile of adipose tissue in a mouse model of chronic hypoxia. J Appl Physiol (1985) (2013) 114:1619–28. doi:10.1152/japplphysiol.00460.2012
Pubmed Abstract | Pubmed Full Text | CrossRef Full Text | Google Scholar
42. Lecoultre V, Peterson CM, Covington JD, Ebenezer PJ, Frost EA, Schwarz JM, et al. Ten nights of moderate hypoxia improves insulin sensitivity in obese humans. Diabetes Care (2013) 36:e197–8. doi:10.2337/dc13-1350
43. Goossens GH. Comment on Lecoultre et al. Ten nights of moderate hypoxia improves insulin sensitivity in obese humans. Diabetes care 2013;36:e197-e198. Diabetes Care (2014) 37:e155–6. doi:10.2337/dc14-0097
44. Ip MS, Lam B, Ng MM, Lam WK, Tsang KW, Lam KS. Obstructive sleep apnea is independently associated with insulin resistance. Am J Respir Crit Care Med (2002) 165:670–6. doi:10.1164/ajrccm.165.5.2103001
Pubmed Abstract | Pubmed Full Text | CrossRef Full Text | Google Scholar
45. Punjabi NM, Shahar E, Redline S, Gottlieb DJ, Givelber R, Resnick HE. Sleep-disordered breathing, glucose intolerance, and insulin resistance: the Sleep Heart Health Study. Am J Epidemiol (2004) 160:521–30. doi:10.1093/aje/kwh261
Pubmed Abstract | Pubmed Full Text | CrossRef Full Text | Google Scholar
Keywords: adipose tissue, oxygen tension, obesity, hypoxia, hyperoxia, blood flow, metabolism, inflammation
Citation: Goossens GH and Blaak EE (2015) Adipose tissue dysfunction and impaired metabolic health in human obesity: a matter of oxygen? Front. Endocrinol. 6:55. doi: 10.3389/fendo.2015.00055
Received: 03 March 2015; Accepted: 06 April 2015;
Published: 24 April 2015
Edited by:
Daniela Patrizia Foti, Università degli Studi “Magna Graecia” di Catanzaro, ItalyReviewed by:
Helene Choquet, University of California San Francisco, USACopyright: © 2015 Goossens and Blaak. This is an open-access article distributed under the terms of the Creative Commons Attribution License (CC BY). The use, distribution or reproduction in other forums is permitted, provided the original author(s) or licensor are credited and that the original publication in this journal is cited, in accordance with accepted academic practice. No use, distribution or reproduction is permitted which does not comply with these terms.
*Correspondence: Gijs H. GoossensRy5Hb29zc2Vuc0BtYWFzdHJpY2h0dW5pdmVyc2l0eS5ubA==
Disclaimer: All claims expressed in this article are solely those of the authors and do not necessarily represent those of their affiliated organizations, or those of the publisher, the editors and the reviewers. Any product that may be evaluated in this article or claim that may be made by its manufacturer is not guaranteed or endorsed by the publisher.
Research integrity at Frontiers
Learn more about the work of our research integrity team to safeguard the quality of each article we publish.