- 1Helsinki University Central Hospital, Children’s Hospital, University of Helsinki, Helsinki, Finland
- 2Hochschule Mannheim – University of Applied Sciences, Mannheim, Germany
- 3St. Louis Children’s Hospital, Washington University School of Medicine, St. Louis, MO, USA
The adrenal cortex is divided into concentric zones. In humans the major cortical zones are the zona glomerulosa, zona fasciculata, and zona reticularis. The adrenal cortex is a dynamic organ in which senescent cells are replaced by newly differentiated ones. This constant renewal facilitates organ remodeling in response to physiological demand for steroids. Cortical zones can reversibly expand, contract, or alter their biochemical profiles to accommodate needs. Pools of stem/progenitor cells in the adrenal capsule, subcapsular region, and juxtamedullary region can differentiate to repopulate or expand zones. Some of these pools appear to be activated only during specific developmental windows or in response to extreme physiological demand. Senescent cells can also be replenished through direct lineage conversion; for example, cells in the zona glomerulosa can transform into cells of the zona fasciculata. Adrenocortical cell differentiation, renewal, and function are regulated by a variety of endocrine/paracrine factors including adrenocorticotropin, angiotensin II, insulin-related growth hormones, luteinizing hormone, activin, and inhibin. Additionally, zonation and regeneration of the adrenal cortex are controlled by developmental signaling pathways, such as the sonic hedgehog, delta-like homolog 1, fibroblast growth factor, and WNT/β-catenin pathways. The mechanisms involved in adrenocortical remodeling are complex and redundant so as to fulfill the offsetting goals of organ homeostasis and stress adaptation.
Introduction
The adrenal cortex is a major source of steroid hormones, which are synthesized from cholesterol through the sequential actions of a series of cytochrome P450 (CYP) enzymes and hydroxysteroid dehydrogenases (HSDs) (Figure 1) (1). Anatomically and functionally distinct zones in the adrenal cortex synthesize specific steroid hormones in response to endocrine and paracrine signals. The regulation of adrenocortical development and homeostasis has been the subject of intensive investigation over the past decade (2–4). This review article summarizes recent advances in our understanding of adrenocortical zonation, renewal, and remodeling. Animal models useful for studies of adrenocortical biology, such as the mouse, rat, and ferret, are highlighted.
Adrenocortical Zonation in Humans and Animal Models
The adrenal cortex of humans is composed of three concentric layers: the zona glomerulosa (zG), zona fasciculata (zF), and zona reticularis (zR) [reviewed in Ref. (2)]. The outermost layer, the zG, functions as part of the renin-angiotensin-aldosterone system (RAAS). In response to angiotensin II (Ang II) or elevated plasma potassium ion (K+) concentrations, zG cells secrete aldosterone, a mineralocorticoid that induces the retention of sodium ion (Na+) and water and the excretion of K+ by the kidney. Cells in the zG express the Ang II receptor (AT1R) and aldosterone synthase (CYP11B2). At the ultrastructural level, zG cells are typified by numerous mitochondria with lamelliform cristae and a few cytoplasmic lipid droplets (Figure 2A). Cells in the zF produce glucocorticoids as part of the hypothalamic-pituitary-adrenal (HPA) axis. zF cells respond to adrenocorticotropic hormone (ACTH) via its receptor (MC2R) and the accessory protein MRAP. Cells in the zF are organized in cord-like structures, or fascicles, that are surrounded by fenestrated capillaries. Cells in this zone contain numerous mitochondria with tubulovesicular cristae, many cytoplasmic lipid droplets, and prominent smooth endoplasmic reticulum (Figure 2B) (5, 6). The innermost layer of the cortex, the zR, secretes the weak androgen dehydroepiandrosterone (DHEA) and its sulfated form DHEA-S (1). Cells of the zR resemble those of the zF but contain fewer lipid droplets and more lysosomes and vacuoles (6). The adrenal gland is covered by a fibrous capsule that serves as both a support structure and a reservoir of stem/progenitor cells for the cortex (see Section “Adrenocortical Stem Cells”) (7).
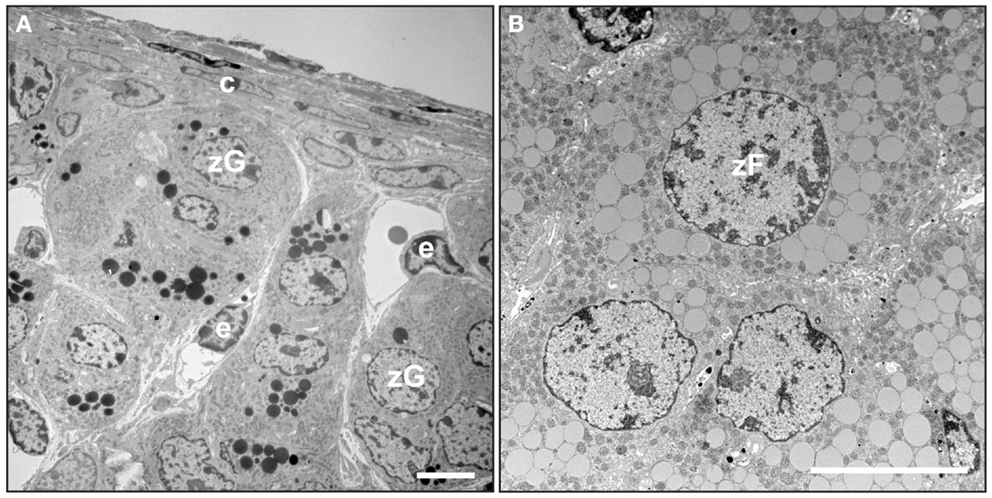
Figure 2. Electron microscopy of mouse adrenal cortex. Adrenal glands from a 4-month-old female mouse were fixed in Karnovsky’s solution, postfixed in 2% OsO4, dehydrated, and then embedded in epon. Thin sections were stained with uranyl acetate plus lead citrate and examined by transmission electron microscopy. (A) Adrenal capsule and zona glomerulosa. (B) Zona fasciculata. Abbreviations: c, capsule; e, endothelial cell; zF, zona fasciculata cell; zG, zona glomerulosa cell. Bars, 4 μm.
Species differ in their adrenocortical zonation patterns (8) (Figure 3). In the mouse and rat, the adrenal cortex contains zG and zF, but there is no recognizable zR. The adrenal cortex of the young mouse contains an additional, ephemeral layer known as the X-zone (9, 10). The function of the X-zone remains controversial, but it may be involved in progesterone catabolism (11). The rat adrenal cortex contains a less prominent layer, the undifferentiated zone (zU), located between the zG and zF (12). The zU has been implicated in adrenocortical homeostasis and remodeling (see Section “Delta-like Homologue 1 Pathway”) (12, 13). Cells in the inner aspect of the zU express MC2R and cholesterol side-chain cleavage enzyme (CYP11A1), which catalyzes the first reaction in steroidogenesis. The inner zU lacks expression of markers of the zG (Cyp11b2) or zF (steroid 11β-hydroxylase; Cyp11b1) (14). Thus, the inner zU may represent a transitional population of cells committed to the steroidogenic phenotype. An analogous layer, the zona intermedia (zI), is present in the adrenal glands of ferrets (15). Recently, the spiny mouse (genus Acomys) has attracted attention as a novel model for the study of adrenocortical development and function. In contrast to the laboratory mouse (genus Mus), the adrenal cortex of the spiny mouse contains the zR and secretes both cortisol and DHEA (16). In this respect the adrenal gland of the spiny mouse mimics that of humans.
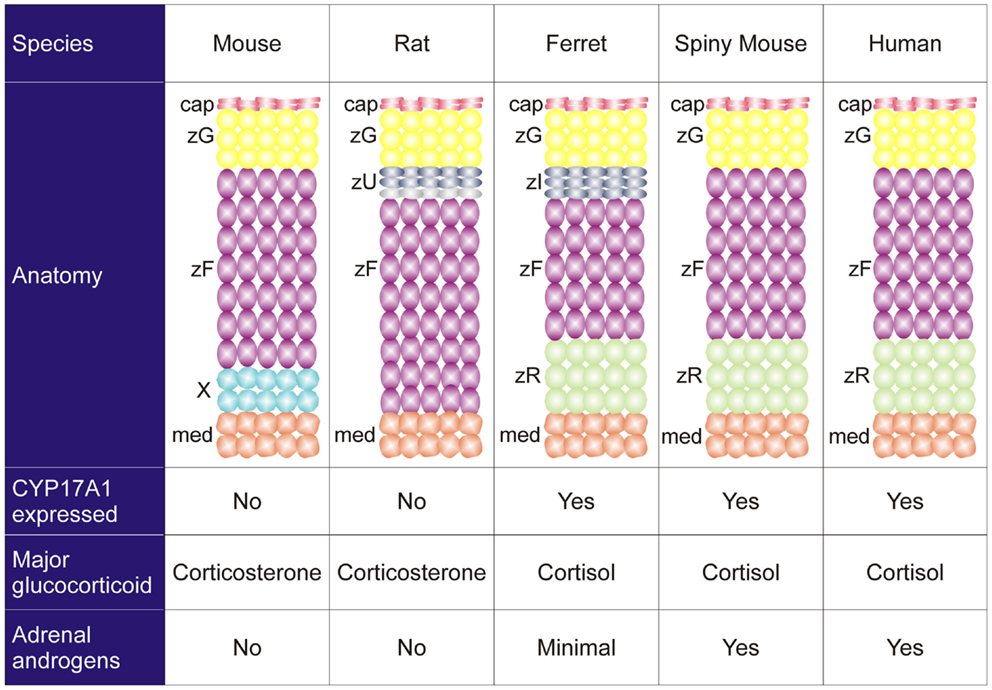
Figure 3. Comparative anatomy and physiology of the adrenal cortex. The undifferentiated zone of the rat adrenal is subdivided into outer (dark gray) and inner (light gray) zones that differ in marker expression and function (see the text). Abbreviations: cap, capsule; med, medulla; X, X-zone; zF, zona fasciculata; zG, zona glomerulosa; zI, zona intermedia; zR, zona reticularis; zU, undifferentiated zone.
Species also vary in the repertoire of steroidogenic enzymes and cofactors expressed in the adrenal cortex, and these differences impact function (Figure 3). Two factors that are differentially expressed among species are 17α-hydroxylase/17,20 lyase (CYP17A1) and cytochrome b5 (CYB5). CYP17A1, a bifunctional enzyme, catalyzes the 17α-hydroxylation reaction required for cortisol synthesis and the 17,20-lyase reaction required for the androgen production (1). The lyase activity is enhanced by allosteric interactions with CYB5 (1). Cells in the zF and zR of humans and ferrets have 17α-hydroxylase activity, so cortisol is the principal glucocorticoid secreted by the adrenal gland of these organisms (8). In humans the adrenal cortex begins to produce DHEA and DHEA-S at adrenarche, contemporaneous with increased expression of CYB5 in the zR (1). The adrenal glands of ferrets produce only limited amounts of androgens due to low CYB5 expression (8, 17). Cells in the adrenal cortex of adult mice and rats lack CYP17A1, so corticosterone is the principal glucocorticoid secreted, and adrenal androgens are not produced (8). The relative strengths and weaknesses of established and emerging animal models are summarized in Table 1.
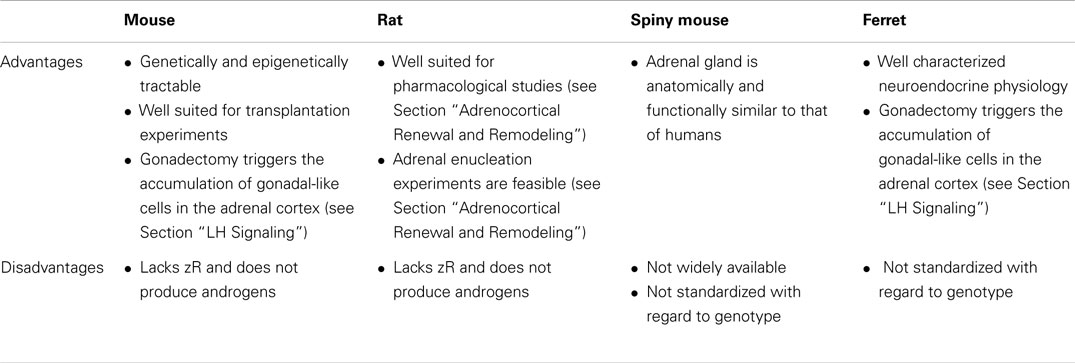
Table 1. Advantages and disadvantages of various animal models for studies of adrenocortical zonation and remodeling.
Adrenocortical Renewal and Remodeling
The adult adrenal cortex is a dynamic tissue. Cells lost through senescence or injury are continually replenished through cell division and differentiation (2, 4). In the adult adrenal gland, most cell proliferation occurs near the periphery of the cortex, as shown by bromodeoxyuridine and [3H]thymidine labeling experiments [reviewed in Ref. (3)]. The remarkable regenerative capacity of the organ is evidenced by rat adrenal enucleation experiments, wherein the gland is incised and squeezed so as to extrude the cortex. Within weeks a new adrenal cortex regenerates from the remaining capsule and adherent subcapsular cells [reviewed in Ref. (18)].
Constant cellular turnover in the adrenal cortex facilitates rapid organ remodeling in response to physiological demand for steroids. Zones can reversibly enlarge, shrink, or alter their biochemical profiles to accommodate physiological needs or in response to experimental manipulations (Table 2). For example, administration of captopril, an inhibitor of the RAAS, leads to contraction of the zG in rats [reviewed in Ref. (2)].
Overview of Adrenocortical Development
Embryogenesis and early postnatal development provide a contextual framework for understanding the mechanisms involved in adrenocortical zonation and homeostasis. Although structurally and functionally distinct, the adrenal cortex, ovary, and testis arise from a common progenitor, the adrenocortical primordium (AGP). The AGP is derived from a specialized region of celomic epithelium known as the urogenital ridge (Figure 4), which also gives rise to the kidney and progenitors of definitive hematopoiesis. Cells in the AGP co-express the transcription factor genes Wilms tumor suppressor-1 (Wt1), GATA-binding protein 4 (Gata4), and steroidogenic factor-1 (Sf1, also called AdBP4 or Nr5a1) [reviewed in Ref. (2, 24, 25)]. As development proceeds, progenitors of the adrenal cortex and the gonad separate and activate different transcriptional programs. Adrenal progenitor cells in the AGP migrate dorsomedially into subjacent mesenchyme, upregulate expression of Sf1, and downregulate expression of Wt1 and Gata4 (25, 26). In contrast, gonadal progenitor cells in the AGP migrate dorsolaterally and maintain expression of Sf1, Wt1, and Gata4. Adrenal precursors combine with neural-crest derived sympathoblasts, the precursors of chromaffin cells in the medulla, to form the adrenal anlagen. Gonadal progenitors combine with primordial germ cells to form the bipotential gonad. Subsequently, the nascent adrenal glands become enveloped by capsule cells, which are derived from both surrounding mesenchyme and fetal adrenal cells that previously expressed Sf1 [reviewed in Ref. (27)].
In rodents, zonal patterns of steroidogenic enzyme expression first become evident during embryonic development [reviewed in Ref. (24)]. In mice, expression of Cyp11a1 is first detectable in the nascent adrenal at embryonic day (E) 11.5–12.5 (26, 28), and there is a concurrent increase in the level of endogenous biotin (29). Expression of the zF marker Cyp11b1 begins at E13.5, whereas expression of the zG markers Ang II receptor type 1 (At1b) and Cyp11b2 appears in the periphery of the cortex just before birth, and Cyp11b2 and Cyp11b1 expression domains are mutually exclusive at this stage (30–32).
By the eighth week of gestation in humans, the fetal adrenal cortex contains two morphologically distinct layers: an inner fetal zone (Fz) and an outer definitive zone (Dz) (33). The Fz is thick and contains large, eosinophilic cells, whereas the Dz is thin and contains small, basophilic cells. Functionally, the Fz resembles the adult zR. The Fz expresses CYP17A1 and CYB5 and produces large amounts of DHEA and DHEA-S, which are converted by the sequential actions of the liver and placenta into estrogens. A third cortical zone, termed the transitional zone (Tz), becomes evident shortly thereafter. The Tz produces cortisol, and an early burst of cortisol production during the ninth week of gestation, coinciding with a transient increase in expression of 3β-hydroxysteroid dehydrogenase type 2 (HSD3B2), is thought to safeguard female sexual development by suppressing the fetal HPA axis and thereby inhibiting adrenal androgen production (34). At birth, the adrenal gland is almost as large as the kidney, but the size of the organ decreases dramatically over first 2 weeks of neonatal life; the Fz involutes via apoptosis, and there is a concomitant reduction in adrenal androgen production (1). The mouse X-zone, a remnant of the fetal adrenal that regresses postnatally (9), is thought to be the analog of the human Fz. Postnatally, the human Dz differentiates into the anatomically and functionally distinct zones of the adult cortex.
Adrenocortical Stem Cells
The adrenal cortex contains stem/progenitor cells that can divide and differentiate to replenish senescing cells and maintain or expand zones (Table 3) [reviewed in Ref. (4)]. In one long-standing model of adrenal zonation, the cell migration model, stem/progenitor cells in the periphery of the adrenal cortex differentiate and migrate centripetally to repopulate the gland before undergoing apoptosis in the juxtamedullary region (35). Aspects of this model have been validated through lineage tracing analyses (24, 30, 36), but recent studies indicate that the regulation of zonation is more complex than originally appreciated [reviewed in Ref. (13)]. It is now clear that distinct pools of stem/progenitor cells exist in the adrenal capsule, subjacent cortex, juxtamedullary region, and other sites (Table 3). Some of these pools appear to be activated only during specific developmental windows or in response to extreme physiological demand. Under certain experimental conditions, adrenocortical zones can be replenished by centrifugal migration (37, 38). For example, stem/progenitor cells in the juxtamedullary region can proliferate, differentiate, and centrifugally repopulate the cortex with fetal-like cells, as is seen in gonadectomy (GDX)-induced secondary X-zone formation and in a genetic model of dysregulated cAMP production (37, 39, 40). The mechanisms that govern centripetal and centrifugal migration are not well understood. Whether centrifugal migration operates under basal conditions is unknown.
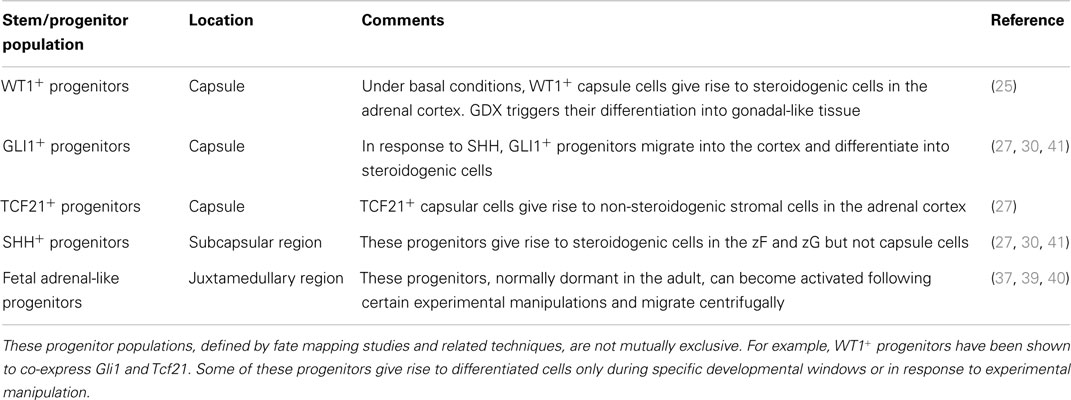
Table 3. Stem/progenitor cell populations that give rise to steroidogenic and non-steroidogenic cells in the adrenal cortex.
Adrenocortical Cell Plasticity
Cell plasticity is another mechanism for replenishing adrenocortical cells lost to senescence or injury. Plasticity refers to the ability of cells to adopt an alternate functional identity in response to cues from the hormonal milieu and cellular microenvironment. One form of plasticity entails trans-differentiation, the direct conversion of one differentiated cell into a differentiated cell of another lineage (42). A second form of plasticity involves de-differentiation, wherein a differentiated cell reverts to a less differentiated cell within the same tissue lineage (42). Interconversion of differentiated cells, either through trans- or de-differentiation, provides an alternative to regeneration via mobilization of stem/progenitor cells. Such functional redundancy ensures organ homeostasis and an optimal adaptation to stress (13).
The plasticity of differentiated adrenocortical cells was elegantly demonstrated in fate mapping studies by Freedman et al. (36), who used Cyp11b2-Cre to permanently mark zG cells and their descendants with green fluorescent protein (GFP). By tracing the fate of GFP+ cells, the investigators showed that adrenocortical zonation is orchestrated in part by direct lineage conversion of zG cells into zF cells (Figure 5). To show that zG-to-zF conversion participates in adrenocortical remodeling, Freedman et al. treated adult mice with glucocorticoids to inhibit the HPA axis (36). Glucocorticoid treatment caused contraction of the zF and loss of GFP+ cells in this zone. Following withdrawal of exogenous glucocorticoids, zG-to-zF conversion resumed and the zF expanded. Remarkably, when conversion of zG to zF cells was abrogated through conditional deletion of the Sf1 gene in CYP11B2+ cells, a functional zF still formed, implying the existence of alternate routes for differentiation of zF cells. These alternative sources for zF cells remain the subject of active investigation. Collectively, these results support a model in which differentiated cells undergo lineage conversion during adrenocortical renewal and remodeling.
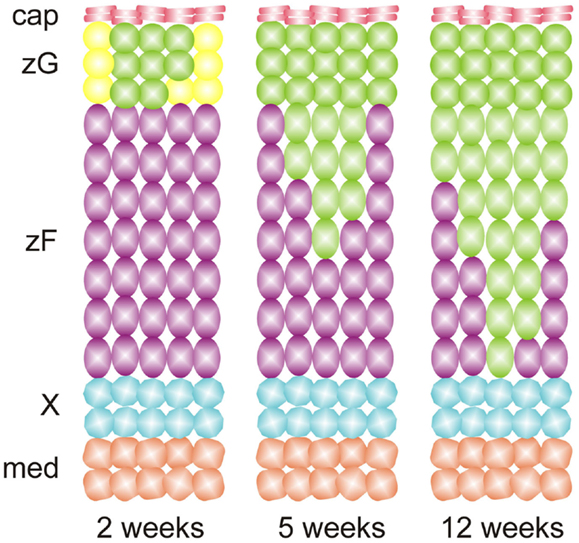
Figure 5. Adrenocortical zonation during postnatal mouse development results from lineage conversion of zG cells into zF cells, as evidenced by fate mapping using Cyp11b2-cre and a GFP reporter. Recombination of the reporter in zG leads to expression of GFP (green cells). The resultant cells migrate inward and differentiate into zF cells. Abbreviations: cap, capsule; med, medulla; X, X-zone; zF, zona fasciculata; zG, zona glomerulosa.
Developmental Signaling Pathways Implicated in Adrenocortical Zonation, Renewal, or Remodeling
Developmental signaling pathways control cell pluripotency, differentiation, and patterning in various tissues. As detailed below, some of these signaling pathways play key roles during the exponential growth phase of adrenal cortex development (12, 24, 43, 44). Additionally, these pathways regulate renewal and remodeling in the adult organism.
Hedgehog Pathway
The hedgehog family of morphogens comprises sonic hedgehog (SHH), Indian hedgehog, and desert hedgehog. Each of these ligands binds to Patched-1 (PTCH1), a transmembrane receptor that is expressed on target cells (45). In the absence of hedgehog binding, PTCH1 inhibits the G protein-coupled receptor Smoothened (SMO) [reviewed in Ref. (2, 46)]. As a result, the zinc finger transcription factors GLI2 and GLI3 are proteolytically digested and lose their activation domains (47). The resultant truncated forms of GLI2 and GLI3 repress transcription. Binding of hedgehog ligands to PTCH1 relieves the inhibition it exerts on SMO, thereby preventing the proteolytic processing of the GLI factors. Full-length GLI2 and GLI3 act as transcriptional activators. The related transcriptional activator, GLI1, is not expressed in the absence of hedgehog ligand, but is upregulated by activation of the pathway. Consequently Gli1 expression serves as a useful marker for active hedgehog signaling (48).
SHH, the only member of the hedgehog family produced in the adrenal cortex, is secreted by subcapsular cells that express Sf1 but not the terminal enzymes required for corticoid synthesis (30, 41, 49). Capsular cells, which do not express Sf1, respond to SHH by expressing Gli1 (Figure 6). Some of these GLI1+ capsule cells migrate centripetally into the cortex, lose responsiveness to SHH, and become steroidogenic, as evidenced by upregulation of Sf1 and differentiation markers characteristic of the zG (Cyp11b2) or zF (Cyp11b1) (Table 2). GLI1+ progenitor cells efficiently contribute to steroidogenic lineages during the exponential phase of cortical growth in embryo, fetus, and newborn mouse (30). In the adult mouse, GLI1+ progenitors contribute to the cortex with low efficiency, but the pathway can be activated in the adult following experimental manipulations such as dexamethasone-induced cortical atrophy. Conditional deletion of Shh in steroidogenic cells of the mouse adrenal results in cortical hypoplasia and capsular thinning, but does not cause major alterations in zonation (30, 41, 49).
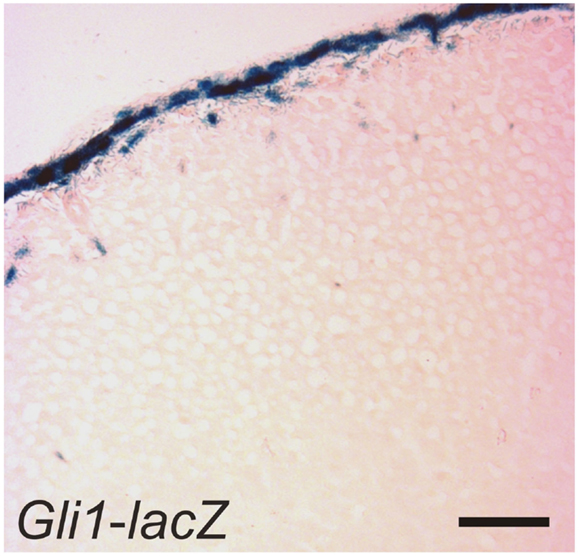
Figure 6. GLI1+ cells in the adrenal capsule. An adrenal gland from a 1-month-old female Gli1-lacZ mouse was whole mount stained with X-gal, cryosectioned, and counterstained with eosin. Bar, 50 μm.
Delta-Like Homolog 1 Pathway
A related signaling protein implicated in adrenocortical homeostasis is Delta-like homolog 1 (DLK1). This factor, also known as preadipocyte factor-1 (PREF-1), is a transmembrane protein related to the Notch family of signaling molecules. DLK1 was originally identified as an important regulator of the undifferentiated state in preadipocytes (50). Cleavage of the extracellular domain of DLK1 by TNF-α converting enzyme produces a biologically active soluble peptide that inhibits the differentiation of preadipocytes into mature adipocytes (50). Subsequent studies showed that DLK1 controls the quiescence of stem/progenitor cells in not only adipose tissue but also other tissue types, including the adrenal cortex (12, 50).
Adrenal enucleation experiments have shown that Dlk1 expression is downregulated and not re-established until zonation of the cortex is complete, suggesting that DLK1 is a negative regulator of adrenocortical differentiation (51). Dlk1 is co-expressed with Shh in the outer zU of the rat (Figure 7) (12). Soluble DLK1, like SHH, modulates Gli1 expression in nearby capsule cells. In addition to being co-expressed, Dlk1 and Shh are coordinately regulated (12). Both genes are downregulated in the adrenals of mice fed a low Na+ diet. Conversely, Dlk1 and Shh are upregulated in the adrenals of mice treated with captopril. These findings suggest that DLK1 and SHH may act together to fine tune the activation of signal receiving cells in the adrenal capsule of the rat. The expression pattern of Dlk1 differs between rats and mice; in mice Dlk1 is expressed in the adrenal capsule rather than the underlying cortex. Nevertheless, indirect evidence suggests that in mice, as in rats, DLK1 may negatively regulate the differentiation of GLI1+ capsular progenitor cells (43).
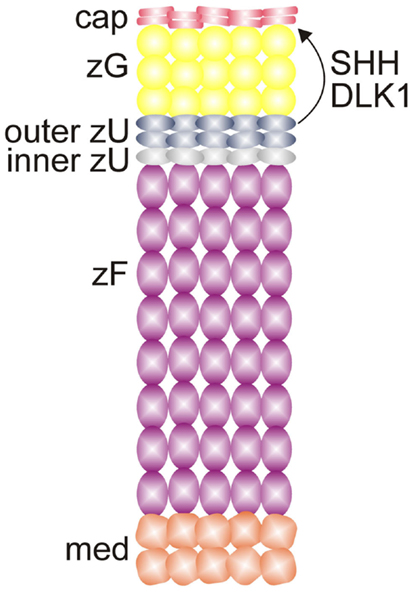
Figure 7. SHH and DLK1 are co-expressed in the outer zU of the rat adrenal cortex and may act in concert to regulate stem/progenitor cells in the adrenal capsule. Abbreviations: cap, capsule; DLK1, delta-like homolog-1; med, medulla; SHH, sonic hedgehog; X, X-zone; zF, zona fasciculata; zG, zona glomerulosa; zU, undifferentiated zone.
Fibroblast Growth Factor Pathway
Mouse genetic studies have implicated the FGF signaling pathway in adrenocortical development and maintenance [reviewed in Ref. (2, 43)]. The FGF family comprises a large group of extracellular ligands that signal through a family of tyrosine kinase receptors, the FGF receptors (FGFRs). In mammals, the FGFR family consists of four genes, FGFR1-4, which undergo alternative splicing to generate an array of receptors that differ in ligand affinities (52). In the presence of heparin, FGFs bind to their cognate receptors, promoting receptor dimerization and autophosphorylation. This in turn stimulates downstream signaling pathways, including the phosphatidylinositol 3-kinase (PI3K), Janus kinase and signal transducer and activator of transcription (JAK–STAT), and mitogen-activated protein kinase (MAPK) pathways. FGF signaling is essential for proper patterning of the embryo, and this pathway participates in stem cell maintenance (53). Factors in the FGF pathway are expressed in both the adrenal capsule and cortex, as summarized in Table 4.
WNT/β-Catenin Signaling
β-catenin exists in two pools: a cytoskeletal pool controls the interaction of cadherin complexes with adherens junctions, while a cytoplasmic pool participates in canonical WNT signaling, acting as a co-activator for transcription factors of the TCF/LEF family [reviewed in Ref. (2)]. Transcriptionally active β-catenin has been demonstrated in the AGP, the adrenal primordium, and adrenal subcapsular cells of the fetus and adult (61) (Figure 8). WNT/β-catenin signaling is thought to maintain the undifferentiated state of adrenocortical stem/progenitor cells (7, 62). Targeted mutagenesis of β-catenin in SF1+ cells causes late onset adrenal hypoplasia, presumed to be the result of stem/progenitor cell pool depletion (61). On the other hand, constitutive activation of β-catenin in steroidogenic cells expressing aldo-keto reductase family 1, member B7 (Akr1b7) causes abnormal accumulation of undifferentiated cells in the capsule and subcapsule and a concomitant increase in Shh mRNA expression (40).
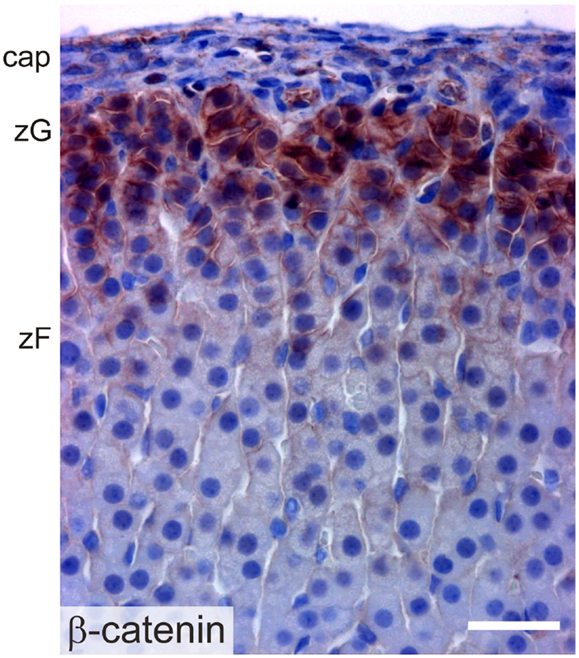
Figure 8. Immunoperoxidase staining of β-catenin in the adrenal cortex of a 2-month-old female mouse. Abbreviations: cap, capsule; zF, zona fasciculata; zG, zona glomerulosa; Bar, 30 μm.
Regulation of the WNT/β-catenin pathway is complex and entails not only a family of WNT ligands but also multiple receptors, co-receptors, decoy receptors, and other modulators (Table 5). This complexity allows fine tuning of the response to morphogen gradients. Stem cell self-renewal mechanisms are frequently co-opted to drive oncogenesis, and WNT signaling is the pathway most frequently mutated in adrenocortical carcinomas (63) (Table 5).
In addition to its proposed role in stem cell maintenance and recruitment, the WNT/β-catenin pathway has been implicated in tissue patterning in the adult organism. For example, proper zonation of the liver requires restriction of WNT/β-catenin signaling to hepatocytes near the central vein (64). In an analogous fashion, restriction of WNT signaling to the periphery of the adrenal cortex is thought to direct zonation in this tissue. Constitutive activation of β-catenin signaling in the mouse zF using Akr1b7-cre triggers the ectopic expression of the zG marker Cyp11b2 and increased production of aldosterone (40, 65). Moreover, studies have shown that β-catenin directly regulates the expression of genes critical for zG function, including At1r and Cyp11b2 (66).
Recent studies have shown that proper differentiation of zF cells requires suppression of WNT/β-catenin signaling (67). In vitro treatment of a zF cell line (ATCL7) with a chemical inducer of canonical WNT signaling (BIO) resulted in down-regulation of genes essential for zF function, including Mc2r, Cyp11a1, and Cyp11b1 (68). Promoter analyses suggested that the molecular basis for this repression may involve the displacement of SF1 from steroidogenic gene promoters by β-catenin (68). These experiments also identified CCDC80 as a novel secreted inhibitor of zF steroidogenesis. Collectively these studies suggest that coordinated regulation of WNT/β-catenin signaling is critical for adrenocortical patterning; WNT/β-catenin signaling must be active for zG determination and must be extinguished for zF determination.
Other Signaling Pathways Implicated in Adrenocortical Growth and Remodeling
Adrenocortical growth and homeostasis are controlled by a diverse array of endocrine/paracrine factors, including ACTH, Ang II, and insulin-related growth factors (IGFs) (15, 24). Hormones traditionally associated with reproductive function, including luteinizing hormone (LH), activin, inhibin, and prolactin, also influence the differentiation and function of adrenocortical cells [reviewed in Ref. (15)].
cAMP Signaling
Many of the hormones that regulate adrenocortical cell proliferation bind to G-protein coupled receptors on the surface of cells [reviewed in Ref. (38)]. Activation of these receptors stimulates adenylate cyclase, resulting in cAMP production. cAMP binds to the regulatory subunits of PKA, allowing the catalytic subunits of protein kinase A (PKA) to phosphorylate downstream effectors, including transcription factors that enhance expression of steroidogenic genes (38).
Inactivating mutations in the protein kinase-A regulatory subunit gene (PRKAR1A) lead to excessive cAMP production. Such mutations cause Carney complex, a syndrome associated with pituitary-independent Cushing syndrome and adrenocortical neoplasia. Conditional deletion of Prkar1a in the adrenal cortex of mice (using Akr1b7-cre) leads to disrupted stem/progenitor cell differentiation, excess cell proliferation, and impaired apoptosis in the adrenal cortex (37). This resistance to apoptosis is mediated in part by crosstalk between the PKA and mammalian target of rapamycin (mTOR) pathways (39). As these mice age, a new zone composed of cells that express Cyp17a1 and secrete cortisol appears in the inner aspect of the cortex. This ectopic X-like zone is thought to arise from normally dormant stem/progenitor cells in the juxtamedullary region (37, 38). These studies and others (38) indicate that normal adrenocortical cell differentiation and proliferation require proper regulation of PKA activity.
IGF Signaling
This pathway has been implicated in growth and differentiation of adrenocortical cells. The IGF family consists of two ligands, IGF1 and IGF2, which bind to the receptor tyrosine kinase IGF1R and promote mitosis/survival via signaling through the MAPK and PI3K pathways (76, 77). IGF1 and IGF2 are expressed at comparable levels in the adult adrenal cortex, whereas IGF2 is highly and preferentially expressed in the fetal adrenal cortex. IGF1R is enriched in the subcapsular region (78). The activity of IGFs is modulated by a family of six IGF-binding proteins (IGFBPs), which can bind and either stimulate or inhibit the activity of IGFs (76).
Mice deficient in both the Igf1r and the insulin receptor (Insr) genes exhibit adrenal agenesis and male-to-female sex reversal (79). The AGP of the double knockout mice contains half the number of SF1+ cells found in wild-type mice. These data indicate that IGF signaling is pivotal for adrenocortical cell specification. Additionally, IGFs have been shown to enhance basal and ACTH-induced steroidogenesis in fetal and adult adrenocortical cells (80).
Transforming Growth Factor β Signaling
The Transforming growth factor β (TGF-β) signaling pathway has been implicated in the maintenance and differentiation of stem/progenitor cells (81). The TGF-β superfamily consists of a diverse array of ligands. Two members of this family, activin and inhibin, are expressed in the fetal and adult adrenal cortex, and have been shown to regulate the growth, function, and survival of adrenocortical cells. Activin signaling is mediated by type I and type II receptors, which are integral membrane receptor serine/threonine kinases. Intracellular SMAD proteins transduce signals from these receptors to the nucleus (81). Activin has been shown to inhibit adrenocortical cell growth, enhance apoptosis of X-zone cells, and modulate steroidogenesis (23, 82, 83). By binding beta-glycan and ActRIA, inhibin blocks activin binding to the type II receptor and subsequent recruitment of the signaling type I receptor (83).
Following GDX, ovarian-like tissue accumulates in the adrenal cortex of Inha-/- mice in an LH dependent manner (23, 84, 85). The loss of Inha results in constitutive TGF-β2 activation in adrenocortical progenitor cells, with subsequent expansion of cells that express Gata4 and other gonadal-like markers. Thus, Inha impacts cell fate decisions (adrenal vs. gonadal) in adrenal cortex.
LH Signaling
This glycoprotein hormone is composed of a common gonadotropin α-subunit and hormone-specific β-subunit. LH is secreted from the pituitary in response to gonadotropin releasing hormone (GnRH). LH binds to G-protein–coupled surface receptor, LHCGR, present on gonadal steroidogenic cells and activates downstream signals, including the cAMP/PKA, MAPK, and PI3K pathways (15). This in turn leads to enhanced expression of steroidogenic enzyme genes, resulting in increased production of sex steroids. Activation of LHCGR also has pleiotropic effects on cell growth and differentiation.
Cells in the adrenal glands express LHCGR and can respond to surges in LH, as evidenced by the phenomenon of GDX-induced adrenocortical neoplasia (71). Following GDX, gonadal-like neoplasms accumulate in the subcapsular region of the adrenal cortex of certain strains of mice. This phenomenon is thought to reflect LH-induced metaplasia of stem/progenitor cells in the adrenal cortex, although the term “neoplasia” is used more often than “metaplasia” to describe the process, because with time these lesions can evolve into frank adenomas or carcinomas. The neoplastic cells express gonadal-like markers (e.g., Lhcgr, Gata4, and Cyp17a1) and secrete sex steroids (86). This phenomenon occurs in other species such as ferrets and goats [reviewed in Ref. (71)]. Moreover, adrenocortical tumors with histologic features resembling luteinized ovarian stroma (“thecal metaplasia”) have been reported, albeit rarely, in postmenopausal women and men with acquired testicular atrophy. Genetic and pharmacologic experiments using mice or ferrets support the premise that LH has a central role in GDX-induced adrenocortical neoplasia [reviewed in Ref. (15, 71)]. The formation of ectopic gonadal-like tissue in the adrenal gland can be viewed as an extreme example of adrenocortical remodeling in response to GDX (13, 25).
Transcription Factors Implicated in Renewal and Remodeling
SF1
SF1 is a master regulator of adrenocortical development and the prototype of steroidogenic transcription factors. SF1 regulates a wide array of genes required for steroidogenic cell function (87, 88). Traditionally, SF1 has been classified as an orphan nuclear receptor, but recent studies have shown that certain phospholipids and sphingolipids bind and regulate this transcription factor [reviewed in Ref. (89)]. For example, the activity of SF1 can be modulated by phosphorylation of the 3-position of the inositol head group of phosphatidylinositol-4,5-bisphosphate PI(4,5)P2 while this phospholipid is bound to SF1 (90). Thus, it is hypothesized that multiple bioactive lipids function as ligands for SF1 and differentially regulate SF1 activity in a context-dependent manner (89).
Sf1-/- mice exhibit degeneration of the AGP due to apoptosis, which results in agenesis of both the adrenal glands and gonads (91). Similarly, targeted mutagenesis of transcription factors that activate Sf1 expression, such as Wt1, Pbx1, and Cited, severely impairs adrenal gland development [reviewed in Ref. (25, 26, 92)]. Sf1± mice have small adrenal glands, reduced corticosterone production in response to stress, and impaired compensatory growth response following unilateral adrenalectomy (91, 93). Individuals with mutations in the DNA-binding domain of SF1 exhibit primary adrenal failure and gonadal dysgenesis. In addition to regulating steroidogenesis, this transcription factor has been implicated in the control of other fundamental cellular processes including glycolysis (87, 88).
Mice harboring multiple copies of Sf1, mimicking the amplification of Sf1 seen in childhood adrenocortical carcinoma (94, 95), develop adrenocortical neoplasms that express gonadal-like markers. This suggests that SF1 can influence cell fate determination. Intriguingly, genetic ablation of the SF1 target gene Vnn1, encoding the gonadal-like marker Vanin-1, has been shown to reduce the severity of neoplastic lesions in the Sf1 transgenic mice (96). Similarly, mice in which the endogenous Sf1 gene of the mouse has been replaced with a mutant lacking a key SUMOylation site exhibit abnormal cell fate specification in steroidogenic tissues, including ectopic expression of gonadal markers (97). The mutant mice also exhibit persistence of the X-zone (97).
Dosage-Sensitive Sex Reversal, Adrenal Hypoplasia Critical Region on Chromosome X (DAX1)
The activity of SF1 is modulated by Dax1 (also called Nr0b1), an X-linked gene that encodes a repressor of steroidogenic gene expression (98). In response to ACTH, SF1-positive subcapsular progenitors downregulate Dax1 and differentiate into adrenocorticoid-producing cells. DAX1 deficiency in humans and mice leads to excessive differentiation of subcapsular progenitors and eventual depletion of the stem/progenitor cell compartment (99, 100). Cytomegaly, a hallmark of adrenal dysfunction associated with Dax1 deficiency (98, 99, 101), is thought to be a compensatory response to a reduced number of cortical cells or to progenitor cell exhaustion (100).
TCF21
TCF21 (also known as POD1) is a basic helix-loop-helix transcription factor functions as a repressor of Sf1 (102). Tcf21 is expressed in the adrenal capsule of adult mice (103), and adrenal glands from Tcf21-/- mice exhibit ectopic expression of Sf1 in the capsule (103). As mentioned previously, some capsule cells are derived from progenitors in the fetal adrenal cortex, and it has been proposed that TCF21 downregulates Sf1 expression in these cells upon recruitment into the capsule (27). Lineage tracing studies have shown that TCF21+ capsular cells give rise to non-steroidogenic stromal cells in the adrenal cortex, but not to steroidogenic cells (27). Collectively these studies suggest that TCF21+ cells in the adrenal capsule participate in adrenocortical homeostasis.
WT1
Fate mapping studies of WT1+ cells have identified long-lived progenitor population in the adrenal capsule characterized by expression of Wt1 and Gata4, markers of the AGP (25, 104). Under basal conditions these AGP-like cells give rise to normal adrenocortical cells (Figure 9). GDX activates these WT1+ progenitors and drives their differentiation into gonadal-like steroidogenic tissue. Hence, WT1+ capsular cells represent a reserve stem/progenitor cell population with AGP-like features that can be mobilized in response to extreme physiological demand (i.e., the hormonal changes associated with GDX).
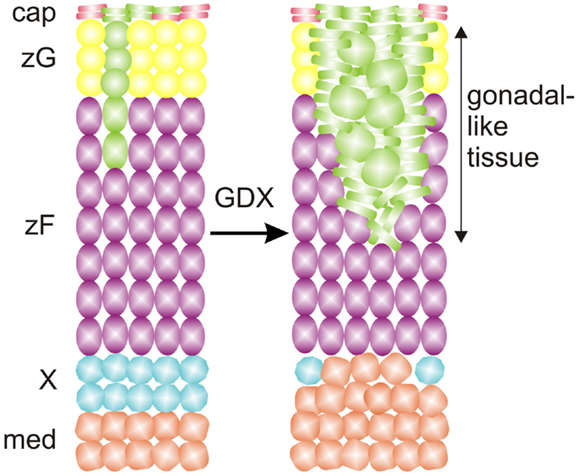
Figure 9. WT1 marks a population of AGP-like progenitors within the adrenal capsule of the mouse. Under basal conditons, AGP-like cells give rise to normal steroidogenic cells in the cortex, as evidenced by lineage tracing analysis with a GFP reporter. Gonadectomy (GDX) triggers the differentiation of AGP-like cells into wedges of gonadal-like steroidogenic tissue. Secretion of sex steroids and other hormones by the ectopic gonadal tissue causes regression of the subjacent X-zone. Abbreviations: cap, capsule; med, medulla; X, X-zone; zF, zona fasciculata; zG, zona glomerulosa.
In the mouse embryo Wt1 repression is necessary for proper expression of Sf1 and differentiation of stem/progenitor cells into adrenocortical cells (25, 104). Ectopic expression of a transcriptionally active isoform of WT1 in SF1+ progenitors causes adrenocortical hypoplasia, increased expression of Gata4, Gli1, and Tcf21, and contraction of the X-zone. WT1 directly regulates the expression of Gli1 in adrenal tissue suggesting that ectopic expression of Wt1 prevents differentiation into SF1+ adrenocortical steroidogenic cells by maintaining cells in a GLI1+ progenitor state.
GATA Binding Protein-6 (GATA6)
This transcription factor is expressed in the adrenal cortex of the fetal mouse (105). Postnatally, adrenal expression of Gata6 is limited to capsular and subcapsular cells (106). Targeted deletion of Gata6 in SF1+ cells results in a pleiotropic adrenal phenotype that includes a thin adrenal cortex, cytomegaly, blunted corticoid production, ectopic chromaffin cells, and aberrant expression of gonadal-like markers (106). Thus, GATA6 is thought to limit the differentiation of adrenal stem/progenitor cells into gonadal-like cells.
Gata6 mutant mice also exhibit abnormal adrenocortical zonation: virgin females lack an X-zone, and castrate males lack a secondary X-zone (Figures 10A,B) (106). Gata6 is not expressed in the X-zone of postnatal wild-type mice, arguing that the effect of Gata6 ablation on X-zone development is either a non-cell autonomous phenomenon or that it occurs in fetal adrenal cells that co-express Gata6 and Sf1-cre (106). Recently, Sergei Tevosian’s laboratory reported that Gata4/Gata6 double knockout mice generated with Sf1-cre exhibit severe adrenal hypoplasia; female double knockout mice die from adrenocortical insufficiency, whereas their male counterparts survive due to heterotopic glucocorticoid production by cells in the testes (107).
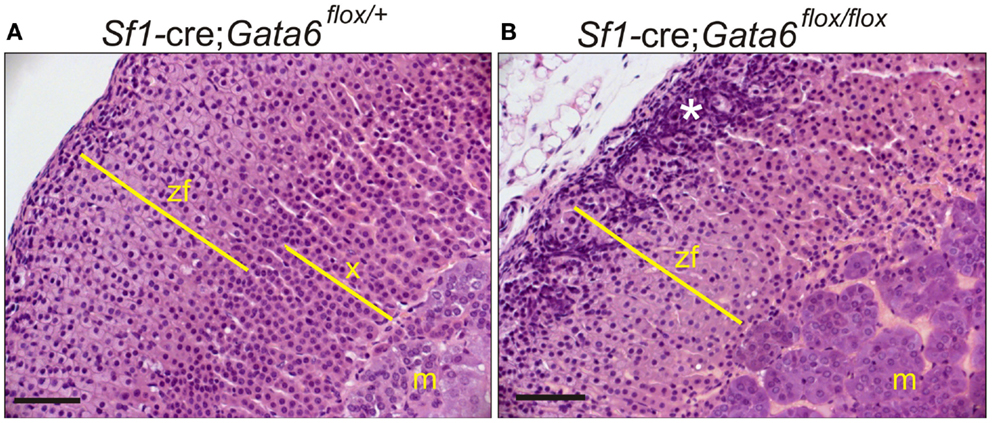
Figure 10. GATA6 is required for formation of a secondary X-zone. (A,B) 3-week-old Sf1-cre; Gata6flox/+ or Sf1-cre; Gata6flox/flox mice were orchiectomized. Adrenal tissue was harvested 1 month later, and paraffin sections were stained with H&E. Note the absence of a secondary X-zone in the mutant mice. The asterisk highlights gonadal-like cells in the subcapsular region. Bar, 50 μm.
Circumstantial evidence from other organ systems suggests that GATA6 may modulate developmental signaling pathways in the adrenal cortex. In epithelial cells of the lung and intestine, GATA6 interacts with the WNT/β-catenin and TGF-β signaling pathways to regulate the balance between stem/progenitor cell expansion and differentiation (108–113). Hindlimb buds express Gata6 in an anterior-posterior gradient, and conditional deletion of Gata6 in limb bud mesenchyme of mice leads to ectopic expression of Shh and its target gene Gli1. The mutant mice develop hindlimb preaxial polydactyly. Conversely, enforced expression of Gata6 in the limb bud represses expression of Shh and results in hypomorphic limbs. In an analogous fashion, GATA6 may repress transcription of Shh and Gli1 in the adrenal cortex. Consistent with this notion, Gli1 has been shown to be upregulated in the adrenal glands of gonadectomized Gata6flox/flox;Sf1-cre mice (106).
Summary and Perspectives
The continual remodeling of the zones of the adrenal cortex requires the precise control of cell growth and differentiation. The process involves distinct pools of stem/progenitor cells in the capsule, subcapsule, and elsewhere. Direct lineage conversion of mature steroidogenic cells is also integral to adrenocortical zonation and remodeling. The pathways involved are complex and redundant so as to fulfill the offsetting goals of organ homeostasis and stress adaptation. Disruption of these pathways can lead to neoplasia.
Although much has been learned about the regulation of adrenocortical homeostasis and regeneration, there are still many unanswered questions. It has proven difficult to isolate and characterize adrenocortical stem cell populations, and we do not know how these populations vary with age. Nor do we understand the relative contributions of the hedgehog, DLK1, FGF, and WNT/β-catenin signaling pathways to adrenocortical differentiation, or how these pathways interface with classic endocrine signaling systems, such as the RAAS and the HPA axis. The positional cues that mediate differentiation during centripetal (or centrifugal) migration also remain enigmatic. In other epithelial organs (e.g., liver, intestine, and lung) the development of in vitro systems, such as organoid cultures and induced pluripotent stem cell models, has helped to elucidate the regulation of differentiation (114). To date, there has been little progress in the development of in vitro models to study adrenocortical differentiation. Hopefully, such techniques will emerge in the coming years and help drive the field forward.
Conflict of Interest Statement
The authors declare that the research was conducted in the absence of any commercial or financial relationships that could be construed as a potential conflict of interest.
Acknowledgments
This work was supported by the following funding agencies: American Heart Association (13GRNT16850031) to DW, DOD (PC141008) to DW, NIH (DK52574) supporting the histology core laboratory at Washington University, Sigrid Jusélius Foundation to MH, and the Academy of Finland to MH.
References
1. Miller WL, Auchus RJ. The molecular biology, biochemistry, and physiology of human steroidogenesis and its disorders. Endocr Rev (2011) 32(1):81–151. doi: 10.1210/er.2010-0013
Pubmed Abstract | Pubmed Full Text | CrossRef Full Text | Google Scholar
2. Yates R, Katugampola H, Cavlan D, Cogger K, Meimaridou E, Hughes C, et al. Adrenocortical development, maintenance, and disease. Curr Top Dev Biol (2013) 106:239–312. doi:10.1016/B978-0-12-416021-7.00007-9
Pubmed Abstract | Pubmed Full Text | CrossRef Full Text | Google Scholar
3. Mitani F. Functional zonation of the rat adrenal cortex: the development and maintenance. Proc Jpn Acad Ser B Phys Biol Sci (2014) 90(5):163–83. doi:10.2183/pjab.90.163
Pubmed Abstract | Pubmed Full Text | CrossRef Full Text | Google Scholar
4. Walczak EM, Hammer GD. Regulation of the adrenocortical stem cell niche: implications for disease. Nat Rev Endocrinol (2015) 11(1):14–28. doi:10.1038/nrendo.2014.166
Pubmed Abstract | Pubmed Full Text | CrossRef Full Text | Google Scholar
5. Zelander T. The ultrastructure of the adrenal cortex of the mouse. Z Zellforsch Mikrosk Anat (1957) 46(6):710–6. doi:10.1007/BF00339373
7. Simon DP, Hammer GD. Adrenocortical stem and progenitor cells: implications for adrenocortical carcinoma. Mol Cell Endocrinol (2012) 351(1):2–11. doi:10.1016/j.mce.2011.12.006
Pubmed Abstract | Pubmed Full Text | CrossRef Full Text | Google Scholar
8. Beuschlein F, Galac S, Wilson DB. Animal models of adrenocortical tumorigenesis. Mol Cell Endocrinol (2012) 351(1):78–86. doi:10.1016/j.mce.2011.09.045
9. Morohashi K, Zubair M. The fetal and adult adrenal cortex. Mol Cell Endocrinol (2011) 336(1–2):193–7. doi:10.1016/j.mce.2010.11.026
Pubmed Abstract | Pubmed Full Text | CrossRef Full Text | Google Scholar
10. Hirokawa N, Ishikawa H. Electron microscopic observations on postnatal development of the X zone in mouse adrenal cortex. Z Anat Entwicklungsgesch (1974) 144(1):85–100. doi:10.1007/BF00518635
11. Hershkovitz L, Beuschlein F, Klammer S, Krup M, Weinstein Y. Adrenal 20alpha-hydroxysteroid dehydrogenase in the mouse catabolizes progesterone and 11-deoxycorticosterone and is restricted to the X-zone. Endocrinology (2007) 148(3):976–88. doi:10.1210/en.2006-1100
Pubmed Abstract | Pubmed Full Text | CrossRef Full Text | Google Scholar
12. Guasti L, Cavlan D, Cogger K, Banu Z, Shakur A, Latif S, et al. Dlk1 upregulates Gli1 expression in male rat adrenal capsule cells through the activation of beta1 integrin and ERK1-2. Endocrinology (2013) 154(12):4675–84. doi:10.1210/en.2013-1211
Pubmed Abstract | Pubmed Full Text | CrossRef Full Text | Google Scholar
13. Pihlajoki M, Heikinheimo M, Wilson DB. Never underestimate the complexity of remodeling. Endocrinology (2013) 154(12):4446–9. doi:10.1210/en.2013-1982
14. Gorrigan RJ, Guasti L, King P, Clark AJ, Chan LF. Localisation of the melanocortin-2-receptor and its accessory proteins in the developing and adult adrenal gland. J Mol Endocrinol (2011) 46(3):227–32. doi:10.1530/JME-11-0011
Pubmed Abstract | Pubmed Full Text | CrossRef Full Text | Google Scholar
15. Bielinska M, Kiiveri S, Parviainen H, Mannisto S, Heikinheimo M, Wilson DB. Gonadectomy-induced adrenocortical neoplasia in the domestic ferret (Mustela putorius furo) and laboratory mouse. Vet Pathol (2006) 43(2):97–117. doi:10.1354/vp.43-2-97
Pubmed Abstract | Pubmed Full Text | CrossRef Full Text | Google Scholar
16. Quinn TA, Ratnayake U, Dickinson H, Nguyen TH, McIntosh M, Castillo-Melendez M, et al. Ontogeny of the adrenal gland in the spiny mouse, with particular reference to production of the steroids cortisol and dehydroepiandrosterone. Endocrinology (2013) 154(3):1190–201. doi:10.1210/en.2012-1953
Pubmed Abstract | Pubmed Full Text | CrossRef Full Text | Google Scholar
17. Wagner S, Kiupel M, Peterson RA, Heikinheimo M, Wilson DB. Cytochrome b5 expression in gonadectomy-induced adrenocortical neoplasms of the domestic ferret (Mustela putorius furo). Vet Pathol (2008) 45(4):439–42. doi:10.1354/vp.45-4-439
Pubmed Abstract | Pubmed Full Text | CrossRef Full Text | Google Scholar
18. Bland ML, Desclozeaux M, Ingraham HA. Tissue growth and remodeling of the embryonic and adult adrenal gland. Ann N Y Acad Sci (2003) 995:59–72. doi:10.1111/j.1749-6632.2003.tb03210.x
Pubmed Abstract | Pubmed Full Text | CrossRef Full Text | Google Scholar
19. Naffin-Olivos JL, Auchus RJ. Human cytochrome b5 requires residues E48 and E49 to stimulate the 17,20-lyase activity of cytochrome P450c17. Biochemistry (2006) 45(3):755–62. doi:10.1021/bi051623y
Pubmed Abstract | Pubmed Full Text | CrossRef Full Text | Google Scholar
20. Pattison JC, Abbott DH, Saltzman W, Conley AJ, Bird IM. Plasticity of the zona reticularis in the adult marmoset adrenal cortex: voyages of discovery in the New World. J Endocrinol (2009) 203(3):313–26. doi:10.1677/JOE-08-0554
Pubmed Abstract | Pubmed Full Text | CrossRef Full Text | Google Scholar
21. Topor LS, Asai M, Dunn J, Majzoub JA. Cortisol stimulates secretion of dehydroepiandrosterone in human adrenocortical cells through inhibition of 3betaHSD2. J Clin Endocrinol Metab (2011) 96(1):E31–9. doi:10.1210/jc.2010-0692
Pubmed Abstract | Pubmed Full Text | CrossRef Full Text | Google Scholar
22. Hirokawa N, Ishikawa H. Electron microscopic observations on the castration-induced X zone in the adrenal cortex of male mice. Cell Tissue Res (1975) 162(1):119–30. doi:10.1007/BF00223267
Pubmed Abstract | Pubmed Full Text | CrossRef Full Text | Google Scholar
23. Beuschlein F, Looyenga BD, Bleasdale SE, Mutch C, Bavers DL, Parlow AF, et al. Activin induces x-zone apoptosis that inhibits luteinizing hormone-dependent adrenocortical tumor formation in inhibin-deficient mice. Mol Cell Biol (2003) 23(11):3951–64. doi:10.1128/MCB.23.11.3951-3964.2003
Pubmed Abstract | Pubmed Full Text | CrossRef Full Text | Google Scholar
24. Laufer E, Kesper D, Vortkamp A, King P. Sonic hedgehog signaling during adrenal development. Mol Cell Endocrinol (2012) 351(1):19–27. doi:10.1016/j.mce.2011.10.002
Pubmed Abstract | Pubmed Full Text | CrossRef Full Text | Google Scholar
25. Bandiera R, Vidal VP, Motamedi FJ, Clarkson M, Sahut-Barnola I, von Gise A, et al. WT1 maintains adrenal-gonadal primordium identity and marks a population of AGP-like progenitors within the adrenal gland. Dev Cell (2013) 27(1):5–18. doi:10.1016/j.devcel.2013.09.003
Pubmed Abstract | Pubmed Full Text | CrossRef Full Text | Google Scholar
26. Val P, Martinez-Barbera JP, Swain A. Adrenal development is initiated by cited2 and Wt1 through modulation of Sf-1 dosage. Development (2007) 134(12):2349–58. doi:10.1242/dev.004390
Pubmed Abstract | Pubmed Full Text | CrossRef Full Text | Google Scholar
27. Wood MA, Acharya A, Finco I, Swonger JM, Elston MJ, Tallquist MD, et al. Fetal adrenal capsular cells serve as progenitor cells for steroidogenic and stromal adrenocortical cell lineages in M. musculus. Development (2013) 140(22):4522–32. doi:10.1242/dev.092775
Pubmed Abstract | Pubmed Full Text | CrossRef Full Text | Google Scholar
28. Schulte DM, Shapiro I, Reincke M, Beuschlein F. Expression and spatio-temporal distribution of differentiation and proliferation markers during mouse adrenal development. Gene Expr Patterns (2007) 7(1–2):72–81. doi:10.1016/j.modgep.2006.05.009
Pubmed Abstract | Pubmed Full Text | CrossRef Full Text | Google Scholar
29. Paul A, Laufer E. Endogenous biotin as a marker of adrenocortical cells with steroidogenic potential. Mol Cell Endocrinol (2011) 336(1–2):133–40. doi:10.1016/j.mce.2011.01.015
Pubmed Abstract | Pubmed Full Text | CrossRef Full Text | Google Scholar
30. King P, Paul A, Laufer E. Shh signaling regulates adrenocortical development and identifies progenitors of steroidogenic lineages. Proc Natl Acad Sci U S A (2009) 106(50):21185–90. doi:10.1073/pnas.0909471106
Pubmed Abstract | Pubmed Full Text | CrossRef Full Text | Google Scholar
31. Mitani F, Ogishima T, Miyamoto H, Ishimura Y. Localization of P450aldo and P45011 beta in normal and regenerating rat adrenal cortex. Endocr Res (1995) 21(1–2):413–23. doi:10.3109/07435809509030457
Pubmed Abstract | Pubmed Full Text | CrossRef Full Text | Google Scholar
32. Mitani F, Mukai K, Miyamoto H, Suematsu M, Ishimura Y. Development of functional zonation in the rat adrenal cortex. Endocrinology (1999) 140(7):3342–53. doi:10.1210/en.140.7.3342
Pubmed Abstract | Pubmed Full Text | CrossRef Full Text | Google Scholar
33. Monticone S, Auchus RJ, Rainey WE. Adrenal disorders in pregnancy. Nat Rev Endocrinol (2012) 8(11):668–78. doi:10.1038/nrendo.2012.155
34. Goto M, Piper HK, Marcos J, Wood PJ, Wright S, Postle AD, et al. In humans, early cortisol biosynthesis provides a mechanism to safeguard female sexual development. J Clin Invest (2006) 116(4):953–60. doi:10.1172/JCI25091
Pubmed Abstract | Pubmed Full Text | CrossRef Full Text | Google Scholar
35. Morley SD, Viard I, Chung BC, Ikeda Y, Parker KL, Mullins JJ. Variegated expression of a mouse steroid 21-hydroxylase/beta-galactosidase transgene suggests centripetal migration of adrenocortical cells. Mol Endocrinol (1996) 10(5):585–98. doi:10.1210/me.10.5.585
Pubmed Abstract | Pubmed Full Text | CrossRef Full Text | Google Scholar
36. Freedman BD, Kempna PB, Carlone DL, Shah MS, Guagliardo NA, Barrett PQ, et al. Adrenocortical zonation results from lineage conversion of differentiated zona glomerulosa cells. Dev Cell (2013) 26(6):666–73. doi:10.1016/j.devcel.2013.07.016
Pubmed Abstract | Pubmed Full Text | CrossRef Full Text | Google Scholar
37. Sahut-Barnola I, de Joussineau C, Val P, Lambert-Langlais S, Damon C, Lefrancois-Martinez AM, et al. Cushing’s syndrome and fetal features resurgence in adrenal cortex-specific Prkar1a knockout mice. PLoS Genet (2010) 6(6):e1000980. doi:10.1371/journal.pgen.1000980
Pubmed Abstract | Pubmed Full Text | CrossRef Full Text | Google Scholar
38. de Joussineau C, Sahut-Barnola I, Levy I, Saloustros E, Val P, Stratakis CA, et al. The cAMP pathway and the control of adrenocortical development and growth. Mol Cell Endocrinol (2012) 351(1):28–36. doi:10.1016/j.mce.2011.10.006
Pubmed Abstract | Pubmed Full Text | CrossRef Full Text | Google Scholar
39. de Joussineau C, Sahut-Barnola I, Tissier F, Dumontet T, Drelon C, Batisse-Lignier M, et al. mTOR pathway is activated by PKA in adrenocortical cells and participates in vivo to apoptosis resistance in primary pigmented nodular adrenocortical disease (PPNAD). Hum Mol Genet (2014) 23(20):5418–28. doi:10.1093/hmg/ddu265
Pubmed Abstract | Pubmed Full Text | CrossRef Full Text | Google Scholar
40. Berthon A, Sahut-Barnola I, Lambert-Langlais S, de Joussineau C, Damon-Soubeyrand C, Louiset E, et al. Constitutive beta-catenin activation induces adrenal hyperplasia and promotes adrenal cancer development. Hum Mol Genet (2010) 19(8):1561–76. doi:10.1093/hmg/ddq029
Pubmed Abstract | Pubmed Full Text | CrossRef Full Text | Google Scholar
41. Huang CC, Miyagawa S, Matsumaru D, Parker KL, Yao HH. Progenitor cell expansion and organ size of mouse adrenal is regulated by sonic hedgehog. Endocrinology (2010) 151(3):1119–28. doi:10.1210/en.2009-0814
Pubmed Abstract | Pubmed Full Text | CrossRef Full Text | Google Scholar
42. Tetteh PW, Farin HF, Clevers H. Plasticity within stem cell hierarchies in mammalian epithelia. Trends Cell Biol (2015) 25(2):100–8. doi:10.1016/j.tcb.2014.09.003
Pubmed Abstract | Pubmed Full Text | CrossRef Full Text | Google Scholar
43. Guasti L, Candy Sze WC, McKay T, Grose R, King PJ. FGF signalling through Fgfr2 isoform IIIb regulates adrenal cortex development. Mol Cell Endocrinol (2013) 371(1–2):182–8. doi:10.1016/j.mce.2013.01.014
Pubmed Abstract | Pubmed Full Text | CrossRef Full Text | Google Scholar
44. Parviainen H, Schrade A, Kiiveri S, Prunskaite-Hyyrylainen R, Haglund C, Vainio S, et al. Expression of Wnt and TGF-beta pathway components and key adrenal transcription factors in adrenocortical tumors: association to carcinoma aggressiveness. Pathol Res Pract (2013) 209(8):503–9. doi:10.1016/j.prp.2013.06.002
Pubmed Abstract | Pubmed Full Text | CrossRef Full Text | Google Scholar
45. Stone DM, Hynes M, Armanini M, Swanson TA, Gu Q, Johnson RL, et al. The tumour-suppressor gene patched encodes a candidate receptor for Sonic hedgehog. Nature (1996) 384(6605):129–34. doi:10.1038/384129a0
Pubmed Abstract | Pubmed Full Text | CrossRef Full Text | Google Scholar
46. Finco I, LaPensee CR, Krill KT, Hammer GD. Hedgehog signaling and steroidogenesis. Annu Rev Physiol (2015) 77:105–29. doi:10.1146/annurev-physiol-061214-111754
47. Pan Y, Bai CB, Joyner AL, Wang B. Sonic hedgehog signaling regulates Gli2 transcriptional activity by suppressing its processing and degradation. Mol Cell Biol (2006) 26(9):3365–77. doi:10.1128/MCB.26.9.3365-3377.2006
Pubmed Abstract | Pubmed Full Text | CrossRef Full Text | Google Scholar
48. Vokes SA, Ji H, McCuine S, Tenzen T, Giles S, Zhong S, et al. Genomic characterization of Gli-activator targets in sonic hedgehog-mediated neural patterning. Development (2007) 134(10):1977–89. doi:10.1242/dev.001966
Pubmed Abstract | Pubmed Full Text | CrossRef Full Text | Google Scholar
49. Ching S, Vilain E. Targeted disruption of Sonic hedgehog in the mouse adrenal leads to adrenocortical hypoplasia. Genesis (2009) 47(9):628–37. doi:10.1002/dvg.20532
Pubmed Abstract | Pubmed Full Text | CrossRef Full Text | Google Scholar
50. Sul HS. Minireview: Pref-1: role in adipogenesis and mesenchymal cell fate. Mol Endocrinol (2009) 23(11):1717–25. doi:10.1210/me.2009-0160
Pubmed Abstract | Pubmed Full Text | CrossRef Full Text | Google Scholar
51. Halder SK, Takemori H, Hatano O, Nonaka Y, Wada A, Okamoto M. Cloning of a membrane-spanning protein with epidermal growth factor-like repeat motifs from adrenal glomerulosa cells. Endocrinology (1998) 139(7):3316–28. doi:10.1210/endo.139.7.6081
Pubmed Abstract | Pubmed Full Text | CrossRef Full Text | Google Scholar
52. Zhang P, Greendorfer JS, Jiao J, Kelpke SC, Thompson JA. Alternatively spliced FGFR-1 isoforms differentially modulate endothelial cell activation of c-YES. Arch Biochem Biophys (2006) 450(1):50–62. doi:10.1016/j.abb.2006.03.017
Pubmed Abstract | Pubmed Full Text | CrossRef Full Text | Google Scholar
53. Katoh M. Network of WNT and other regulatory signaling cascades in pluripotent stem cells and cancer stem cells. Curr Pharm Biotechnol (2011) 12(2):160–70. doi:10.2174/138920111794295710
Pubmed Abstract | Pubmed Full Text | CrossRef Full Text | Google Scholar
54. Chu Y, Ho WJ, Dunn JC. Basic fibroblast growth factor delivery enhances adrenal cortical cellular regeneration. Tissue Eng Part A (2009) 15(8):2093–101. doi:10.1089/ten.tea.2008.0305
Pubmed Abstract | Pubmed Full Text | CrossRef Full Text | Google Scholar
55. Crickard K, Ill CR, Jaffe RB. Control of proliferation of human fetal adrenal cells in vitro. J Clin Endocrinol Metab (1981) 53(4):790–6. doi:10.1210/jcem-53-4-790
Pubmed Abstract | Pubmed Full Text | CrossRef Full Text | Google Scholar
56. Gospodarowicz D, Ill CR, Hornsby PJ, Gill GN. Control of bovine adrenal cortical cell proliferation by fibroblast growth factor. Lack of effect of epidermal growth factor. Endocrinology (1977) 100(4):1080–9. doi:10.1210/endo-100-4-1080
Pubmed Abstract | Pubmed Full Text | CrossRef Full Text | Google Scholar
57. Lepique AP, Moraes MS, Rocha KM, Eichler CB, Hajj GN, Schwindt TT, et al. c-Myc protein is stabilized by fibroblast growth factor 2 and destabilized by ACTH to control cell cycle in mouse Y1 adrenocortical cells. J Mol Endocrinol (2004) 33(3):623–38. doi:10.1677/jme.1.01485
Pubmed Abstract | Pubmed Full Text | CrossRef Full Text | Google Scholar
58. Basile DP, Holzwarth MA. Basic fibroblast growth factor receptor in the rat adrenal cortex: effects of suramin and unilateral adrenalectomy on receptor numbers. Endocrinology (1994) 134(6):2482–9. doi:10.1210/en.134.6.2482
Pubmed Abstract | Pubmed Full Text | CrossRef Full Text | Google Scholar
59. Revest JM, Spencer-Dene B, Kerr K, De Moerlooze L, Rosewell I, Dickson C. Fibroblast growth factor receptor 2-IIIb acts upstream of Shh and Fgf4 and is required for limb bud maintenance but not for the induction of Fgf8, Fgf10, Msx1, or Bmp4. Dev Biol (2001) 231(1):47–62. doi:10.1006/dbio.2000.0144
Pubmed Abstract | Pubmed Full Text | CrossRef Full Text | Google Scholar
60. Kim Y, Bingham N, Sekido R, Parker KL, Lovell-Badge R, Capel B. Fibroblast growth factor receptor 2 regulates proliferation and Sertoli differentiation during male sex determination. Proc Natl Acad Sci U S A (2007) 104(42):16558–63. doi:10.1073/pnas.0702581104
Pubmed Abstract | Pubmed Full Text | CrossRef Full Text | Google Scholar
61. Kim AC, Reuter AL, Zubair M, Else T, Serecky K, Bingham NC, et al. Targeted disruption of beta-catenin in Sf1-expressing cells impairs development and maintenance of the adrenal cortex. Development (2008) 135(15):2593–602. doi:10.1242/dev.021493
Pubmed Abstract | Pubmed Full Text | CrossRef Full Text | Google Scholar
62. Berthon A, Martinez A, Bertherat J, Val P. Wnt/beta-catenin signalling in adrenal physiology and tumour development. Mol Cell Endocrinol (2012) 351(1):87–95. doi:10.1016/j.mce.2011.09.009
Pubmed Abstract | Pubmed Full Text | CrossRef Full Text | Google Scholar
63. Christofer Juhlin C, Goh G, Healy JM, Fonseca AL, Scholl UI, Stenman A, et al. Whole-exome sequencing characterizes the landscape of somatic mutations and copy number alterations in adrenocortical carcinoma. J Clin Endocrinol Metab (2014) 9:jc20143282. doi:10.1210/jc.2014-3282
Pubmed Abstract | Pubmed Full Text | CrossRef Full Text | Google Scholar
64. Benhamouche S, Decaens T, Godard C, Chambrey R, Rickman DS, Moinard C, et al. Apc tumor suppressor gene is the “zonation-keeper” of mouse liver. Dev Cell (2006) 10(6):759–70. doi:10.1016/j.devcel.2006.03.015
Pubmed Abstract | Pubmed Full Text | CrossRef Full Text | Google Scholar
65. Drelon C, Berthon A, Ragazzon B, Tissier F, Bandiera R, Sahut-Barnola I, et al. Analysis of the role of Igf2 in adrenal tumour development in transgenic mouse models. PLoS One (2012) 7(8):e44171. doi:10.1371/journal.pone.0044171
Pubmed Abstract | Pubmed Full Text | CrossRef Full Text | Google Scholar
66. Berthon A, Drelon C, Ragazzon B, Boulkroun S, Tissier F, Amar L, et al. WNT/beta-catenin signalling is activated in aldosterone-producing adenomas and controls aldosterone production. Hum Mol Genet (2014) 23(4):889–905. doi:10.1093/hmg/ddt484
Pubmed Abstract | Pubmed Full Text | CrossRef Full Text | Google Scholar
67. Drelon C, Berthon A, Mathieu M, Martinez A, Val P. Adrenal cortex tissue homeostasis and zonation: a WNT perspective. Mol Cell Endocrinol (2015). doi:10.1016/j.mce.2014.12.014
Pubmed Abstract | Pubmed Full Text | CrossRef Full Text | Google Scholar
68. Walczak EM, Kuick R, Finco I, Bohin N, Hrycaj SM, Wellik DM, et al. Wnt signaling inhibits adrenal steroidogenesis by cell-autonomous and non-cell-autonomous mechanisms. Mol Endocrinol (2014) 28(9):1471–86. doi:10.1210/me.2014-1060
Pubmed Abstract | Pubmed Full Text | CrossRef Full Text | Google Scholar
69. Heikkila M, Peltoketo H, Leppaluoto J, Ilves M, Vuolteenaho O, Vainio S. Wnt-4 deficiency alters mouse adrenal cortex function, reducing aldosterone production. Endocrinology (2002) 143(11):4358–65. doi:10.1210/en.2002-220275
Pubmed Abstract | Pubmed Full Text | CrossRef Full Text | Google Scholar
70. Suwa T, Chen M, Hawks CL, Hornsby PJ. Zonal expression of dickkopf-3 and components of the Wnt signalling pathways in the human adrenal cortex. J Endocrinol (2003) 178(1):149–58. doi:10.1677/joe.0.1780149
Pubmed Abstract | Pubmed Full Text | CrossRef Full Text | Google Scholar
71. Röhrig T, Pihlajoki M, Ziegler R, Cochran RS, Schrade A, Schillebeeckx M, et al. Toying with fate: redirecting the differentiation of adrenocortical progenitor cells into gonadal-like tissue. Mol Cell Endocrinol (2015). doi:10.1016/j.mce.2014.12.003
Pubmed Abstract | Pubmed Full Text | CrossRef Full Text | Google Scholar
72. Hsu SY, Liang SG, Hsueh AJ. Characterization of two LGR genes homologous to gonadotropin and thyrotropin receptors with extracellular leucine-rich repeats and a G protein-coupled, seven-transmembrane region. Mol Endocrinol (1998) 12(12):1830–45. doi:10.1210/mend.12.12.0211
Pubmed Abstract | Pubmed Full Text | CrossRef Full Text | Google Scholar
73. Assié G, Letouze E, Fassnacht M, Jouinot A, Luscap W, Barreau O, et al. Integrated genomic characterization of adrenocortical carcinoma. Nat Genet (2014) 46(6):6. doi:10.1038/ng.2953
Pubmed Abstract | Pubmed Full Text | CrossRef Full Text | Google Scholar
74. Bernichtein S, Petretto E, Jamieson S, Goel A, Aitman TJ, Mangion JM, et al. Adrenal gland tumorigenesis after gonadectomy in mice is a complex genetic trait driven by epistatic loci. Endocrinology (2007) 149(2):651–61. doi:10.1210/en.2007-0925
Pubmed Abstract | Pubmed Full Text | CrossRef Full Text | Google Scholar
75. El Wakil A, Bandulik S, Guy N, Bendahhou S, Zennaro MC, Niehrs C, et al. Dkk3 is a component of the genetic circuitry regulating aldosterone biosynthesis in the adrenal cortex. Hum Mol Genet (2012) 21(22):4922–9. doi:10.1093/hmg/dds333
Pubmed Abstract | Pubmed Full Text | CrossRef Full Text | Google Scholar
76. Fottner C, Hoeflich A, Wolf E, Weber MM. Role of the insulin-like growth factor system in adrenocortical growth control and carcinogenesis. Horm Metab Res (2004) 36(6):397–405. doi:10.1055/s-2004-814563
Pubmed Abstract | Pubmed Full Text | CrossRef Full Text | Google Scholar
77. Drelon C, Berthon A, Val P. Adrenocortical cancer and IGF2: is the game over or our experimental models limited? J Clin Endocrinol Metab (2013) 98(2):505–7. doi:10.1210/jc.2012-3310
78. Belgorosky A, Baquedano MS, Guercio G, Rivarola MA. Expression of the IGF and the aromatase/estrogen receptor systems in human adrenal tissues from early infancy to late puberty: implications for the development of adrenarche. Rev Endocr Metab Disord (2009) 10(1):51–61. doi:10.1007/s11154-008-9105-1
Pubmed Abstract | Pubmed Full Text | CrossRef Full Text | Google Scholar
79. Pitetti JL, Calvel P, Romero Y, Conne B, Truong V, Papaioannou MD, et al. Insulin and IGF1 receptors are essential for XX and XY gonadal differentiation and adrenal development in mice. PLoS Genet (2013) 9(1):e1003160. doi:10.1371/journal.pgen.1003160
Pubmed Abstract | Pubmed Full Text | CrossRef Full Text | Google Scholar
80. Penhoat A, Rainey WE, Viard I, Saez JM. Regulation of adrenal cell-differentiated functions by growth factors. Horm Res (1994) 42(1–2):39–43. doi:10.1159/000184143
Pubmed Abstract | Pubmed Full Text | CrossRef Full Text | Google Scholar
81. Itoh F, Watabe T, Miyazono K. Roles of TGF-beta family signals in the fate determination of pluripotent stem cells. Semin Cell Dev Biol (2014) 32:98–106. doi:10.1016/j.semcdb.2014.05.017
Pubmed Abstract | Pubmed Full Text | CrossRef Full Text | Google Scholar
82. Kumar TR, Donehower LA, Bradley A, Matzuk MM. Transgenic mouse models for tumour-suppressor genes. J Intern Med (1995) 238(3):233–8. doi:10.1111/j.1365-2796.1995.tb00928.x
Pubmed Abstract | Pubmed Full Text | CrossRef Full Text | Google Scholar
83. Vanttinen T, Liu J, Kuulasmaa T, Kivinen P, Voutilainen R. Expression of activin/inhibin signaling components in the human adrenal gland and the effects of activins and inhibins on adrenocortical steroidogenesis and apoptosis. J Endocrinol (2003) 178(3):479–89. doi:10.1677/joe.0.1780479
Pubmed Abstract | Pubmed Full Text | CrossRef Full Text | Google Scholar
84. Looyenga BD, Hammer GD. Genetic removal of Smad3 from inhibin-null mice attenuates tumor progression by uncoupling extracellular mitogenic signals from the cell cycle machinery. Mol Endocrinol (2007) 21(10):18. doi:10.1210/me.2006-0402
Pubmed Abstract | Pubmed Full Text | CrossRef Full Text | Google Scholar
85. Looyenga BD, Wiater E, Vale W, Hammer GD. Inhibin-A antagonizes TGFbeta2 signaling by down-regulating cell surface expression of the TGFbeta coreceptor betaglycan. Mol Endocrinol (2010) 24(3):608–20. doi:10.1210/me.2008-0374
Pubmed Abstract | Pubmed Full Text | CrossRef Full Text | Google Scholar
86. Schillebeeckx M, Pihlajoki M, Gretzinger E, Yang W, Thol F, Hiller T, et al. Novel markers of gonadectomy-induced adrenocortical neoplasia. Mol Cell Endocrinol (2015) 399:122–30. doi:10.1016/j.mce.2014.09.029
Pubmed Abstract | Pubmed Full Text | CrossRef Full Text | Google Scholar
87. Baba T, Otake H, Sato T, Miyabayashi K, Shishido Y, Wang CY, et al. Glycolytic genes are targets of the nuclear receptor Ad4BP/SF-1. Nat Commun (2014) 5:3634. doi:10.1038/ncomms4634
Pubmed Abstract | Pubmed Full Text | CrossRef Full Text | Google Scholar
88. Ruggiero C, Doghman M, Lalli E. How genomic studies have improved our understanding of the mechanisms of transcriptional regulation by NR5A nuclear receptors. Mol Cell Endocrinol (2015). doi:10.1016/j.mce.2014.10.022
Pubmed Abstract | Pubmed Full Text | CrossRef Full Text | Google Scholar
89. Urs AN, Dammer E, Kelly S, Wang E, Merrill AH Jr, Sewer MB. Steroidogenic factor-1 is a sphingolipid binding protein. Mol Cell Endocrinol (2007) 265-266:174–8. doi:10.1016/j.mce.2006.12.016
90. Blind RD, Suzawa M, Ingraham HA. Direct modification and activation of a nuclear receptor-PIP2 complex by the inositol lipid kinase IPMK. Sci Signal (2012) 5(229):ra44. doi:10.1126/scisignal.2003111
Pubmed Abstract | Pubmed Full Text | CrossRef Full Text | Google Scholar
91. Parker KL. The roles of steroidogenic factor 1 in endocrine development and function. Mol Cell Endocrinol (1998) 145(1–2):15–20. doi:10.1016/S0303-7207(98)00164-6
Pubmed Abstract | Pubmed Full Text | CrossRef Full Text | Google Scholar
92. Lichtenauer UD, Duchniewicz M, Kolanczyk M, Hoeflich A, Hahner S, Else T, et al. Pre-B-cell transcription factor 1 and steroidogenic factor 1 synergistically regulate adrenocortical growth and steroidogenesis. Endocrinology (2007) 148(2):693–704. doi:10.1210/en.2006-0681
Pubmed Abstract | Pubmed Full Text | CrossRef Full Text | Google Scholar
93. Beuschlein F, Mutch C, Bavers DL, Ulrich-Lai YM, Engeland WC, Keegan C, et al. Steroidogenic factor-1 is essential for compensatory adrenal growth following unilateral adrenalectomy. Endocrinology (2002) 143(8):3122–35. doi:10.1210/endo.143.8.8944
Pubmed Abstract | Pubmed Full Text | CrossRef Full Text | Google Scholar
94. Figueiredo BC, Cavalli LR, Pianovski MA, Lalli E, Sandrini R, Ribeiro RC, et al. Amplification of the steroidogenic factor 1 gene in childhood adrenocortical tumors. J Clin Endocrinol Metab (2005) 90(2):615–9. doi:10.1210/jc.2004-0942
Pubmed Abstract | Pubmed Full Text | CrossRef Full Text | Google Scholar
95. Doghman M, Karpova T, Rodrigues GA, Arhatte M, De MJ, Cavalli LR, et al. Increased steroidogenic factor-1 dosage triggers adrenocortical cell proliferation and cancer. Mol Endocrinol (2007) 21(12):2968–87. doi:10.1210/me.2007-0120
Pubmed Abstract | Pubmed Full Text | CrossRef Full Text | Google Scholar
96. Latre de Late P, Wakil AE, Jarjat M, de Krijger RR, Heckert LL, Naquet P, et al. Vanin-1 inactivation antagonizes the development of adrenocortical neoplasia in Sf-1 transgenic mice. Endocrinology (2014) 155(7):16. doi:10.1210/en.2014-1088
Pubmed Abstract | Pubmed Full Text | CrossRef Full Text | Google Scholar
97. Lee FY, Faivre EJ, Suzawa M, Lontok E, Ebert D, Cai F, et al. Eliminating SF-1 (NR5A1) sumoylation in vivo results in ectopic hedgehog signaling and disruption of endocrine development. Dev Cell (2011) 21(2):315–27. doi:10.1016/j.devcel.2011.06.028
Pubmed Abstract | Pubmed Full Text | CrossRef Full Text | Google Scholar
98. Lalli E, Melner MH, Stocco DM, Sassone-Corsi P. DAX-1 blocks steroid production at multiple levels. Endocrinology (1998) 139(10):4237–43. doi:10.1210/en.139.10.4237
Pubmed Abstract | Pubmed Full Text | CrossRef Full Text | Google Scholar
99. Achermann JC, Meeks JJ, Jameson JL. Phenotypic spectrum of mutations in DAX-1 and SF-1. Mol Cell Endocrinol (2001) 185(1–2):17–25. doi:10.1016/S0303-7207(01)00619-0
Pubmed Abstract | Pubmed Full Text | CrossRef Full Text | Google Scholar
100. Scheys JO, Heaton JH, Hammer GD. Evidence of adrenal failure in aging Dax1-deficient mice. Endocrinology (2011) 152(9):3430–9. doi:10.1210/en.2010-0986
Pubmed Abstract | Pubmed Full Text | CrossRef Full Text | Google Scholar
101. Zazopoulos E, Lalli E, Stocco DM, Sassone-Corsi P. DNA binding and transcriptional repression by DAX-1 blocks steroidogenesis. Nature (1997) 390(6657):311–5. doi:10.1038/36899
Pubmed Abstract | Pubmed Full Text | CrossRef Full Text | Google Scholar
102. Cui S, Ross A, Stallings N, Parker KL, Capel B, Quaggin SE. Disrupted gonadogenesis and male-to-female sex reversal in Pod1 knockout mice. Development (2004) 131(16):4095–105. doi:10.1242/dev.01266
Pubmed Abstract | Pubmed Full Text | CrossRef Full Text | Google Scholar
103. Kim AC, Hammer GD. Adrenocortical cells with stem/progenitor cell properties: recent advances. Mol Cell Endocrinol (2007) 26(5–266):10–6. doi:10.1016/j.mce.2006.12.028
Pubmed Abstract | Pubmed Full Text | CrossRef Full Text | Google Scholar
104. Bandiera R, Sacco S, Vidal VP, Chaboissier MC, Schedl A. Steroidogenic organ development and homeostasis: a WT1-centric view. Mol Cell Endocrinol (2015). doi:10.1016/j.mce.2015.01.009
Pubmed Abstract | Pubmed Full Text | CrossRef Full Text | Google Scholar
105. Kiiveri S, Liu J, Westerholm-Ormio M, Narita N, Wilson DB, Voutilainen R, et al. Differential expression of GATA-4 and GATA-6 in fetal and adult mouse and human adrenal tissue. Endocrinology (2002) 143(8):3136–43. doi:10.1210/endo.143.8.8939
Pubmed Abstract | Pubmed Full Text | CrossRef Full Text | Google Scholar
106. Pihlajoki M, Gretzinger E, Cochran R, Kyrönlahti A, Schrade A, Hiller T, et al. Conditional mutagenesis of Gata6 in SF1-positive cells causes gonadal-like differentiation in the adrenal cortex of mice. Endocrinology (2013) 154(5):1754–67. doi:10.1210/en.2012-1892
Pubmed Abstract | Pubmed Full Text | CrossRef Full Text | Google Scholar
107. Padua MB, Jiang T, Morse DA, Fox SC, Hatch HM, Tevosian SG. Combined loss of the GATA4 and GATA6 transcription factors in male mice disrupts testicular development and confers adrenal-like function in the testes. Endocrinology (2015). doi:10.1210/en.2014-1907
Pubmed Abstract | Pubmed Full Text | CrossRef Full Text | Google Scholar
108. Zhang Y, Goss AM, Cohen ED, Kadzik R, Lepore JJ, Muthukumaraswamy K, et al. A Gata6-Wnt pathway required for epithelial stem cell development and airway regeneration. Nat Genet (2008) 40(7):862–70. doi:10.1038/ng.157
Pubmed Abstract | Pubmed Full Text | CrossRef Full Text | Google Scholar
109. Tian Y, Zhang Y, Hurd L, Hannenhalli S, Liu F, Lu MM, et al. Regulation of lung endoderm progenitor cell behavior by miR302/367. Development (2011) 138(7):1235–45. doi:10.1242/dev.061762
Pubmed Abstract | Pubmed Full Text | CrossRef Full Text | Google Scholar
110. Beuling E, Baffour-Awuah NY, Stapleton KA, Aronson BE, Noah TK, Shroyer NF, et al. GATA factors regulate proliferation, differentiation, and gene expression in small intestine of mature mice. Gastroenterology (2011) 140(4):e1–2. doi:10.1053/j.gastro.2011.01.033
Pubmed Abstract | Pubmed Full Text | CrossRef Full Text | Google Scholar
111. Beuling E, Aronson BE, Tran LM, Stapleton KA, Ter Horst EN, Vissers LA, et al. GATA6 is required for proliferation, migration, secretory cell maturation, and gene expression in the mature mouse colon. Mol Cell Biol (2012) 32(17):3392–402. doi:10.1128/MCB.00070-12
Pubmed Abstract | Pubmed Full Text | CrossRef Full Text | Google Scholar
112. Whissell G, Montagni E, Martinelli P, Hernando-Momblona X, Sevillano M, Jung P, et al. The transcription factor GATA6 enables self-renewal of colon adenoma stem cells by repressing BMP gene expression. Nat Cell Biol (2014) 16(7):695–707. doi:10.1038/ncb2992
Pubmed Abstract | Pubmed Full Text | CrossRef Full Text | Google Scholar
113. Tsuji S, Kawasaki Y, Furukawa S, Taniue K, Hayashi T, Okuno M, et al. The miR-363-GATA6-Lgr5 pathway is critical for colorectal tumourigenesis. Nat Commun (2014) 5:3150. doi:10.1038/ncomms4150
Pubmed Abstract | Pubmed Full Text | CrossRef Full Text | Google Scholar
114. Lancaster MA, Knoblich JA. Organogenesis in a dish: modeling development and disease using organoid technologies. Science (2014) 345(6194):1247125. doi:10.1126/science.1247125
Pubmed Abstract | Pubmed Full Text | CrossRef Full Text | Google Scholar
Keywords: adrenal cortex, hormone, plasticity, stem cell, steroid, steroidogenesis
Citation: Pihlajoki M, Dörner J, Cochran RS, Heikinheimo M and Wilson DB (2015) Adrenocortical zonation, renewal, and remodeling. Front. Endocrinol. 6:27. doi: 10.3389/fendo.2015.00027
Received: 18 December 2014; Accepted: 16 February 2015;
Published online: 05 March 2015.
Edited by:
Pierre Val, Centre National de la Recherche Scientifique, FranceReviewed by:
David Breault, Boston Children’s Hospital, USAGary Hammer, University of Michigan, USA
Copyright: © 2015 Pihlajoki, Dörner, Cochran, Heikinheimo and Wilson. This is an open-access article distributed under the terms of the Creative Commons Attribution License (CC BY). The use, distribution or reproduction in other forums is permitted, provided the original author(s) or licensor are credited and that the original publication in this journal is cited, in accordance with accepted academic practice. No use, distribution or reproduction is permitted which does not comply with these terms.
*Correspondence: David B. Wilson, Washington University School of Medicine, Box 8208, 660 South Euclid Avenue, St. Louis, MO 63110, USA e-mail:d2lsc29uX2RAd3VzdGwuZWR1