- 1Department of Biochemistry, National Cerebral and Cardiovascular Center Research Institute, Osaka, Japan
- 2National Cerebral and Cardiovascular Center Research Institute, Osaka, Japan
The growth hormone secretagogue-receptor (GHS-R) was discovered in humans and pigs in 1996. The endogenous ligand, ghrelin, was discovered 3 years later, in 1999, and our understanding of the physiological significance of the ghrelin system in vertebrates has grown steadily since then. Although the ghrelin system in non-mammalian vertebrates is a subject of great interest, protein sequence data for the receptor in non-mammalian vertebrates has been limited until recently, and related biological information has not been well organized. In this review, we summarize current information related to the ghrelin receptor in non-mammalian vertebrates.
General Introduction
As implied by their name, growth hormone secretagogues (GHSs), which are artificial derivatives of enkephalin, exhibit growth hormone (GH)-releasing activity (1). Some of these GHSs also stimulate appetite in mammals (2). In 1996, Howard et al. (3) discovered a G-protein-coupled receptor (GPCR) with seven transmembrane domains (TMDs) in humans and pigs, and found that GHSs bound to this receptor and elicited an increase in the intracellular Ca2+ concentration of cells in which it was stably expressed. They named this receptor the GHS-receptor type-1a (GHS-R1a); in addition, they found an alternative splice variant of the receptor that lacked the Ca2+ signaling capacity and named it GHS-R type-1b (GHS-R1b). The mammalian GHS-R gene (ghsr) comprises two exons separated by one intron (4, 5). GHS-R1a comprises 366 amino acids (AAs), where the first exon (exon 1) encodes the first 265 AAs from TMD 1–5, and the second exon (exon 2) encodes the remaining 101 AAs from TMD 6 and 7. In contrast, the alternative splice variant of ghsr, GHS-R1b, is formed from the first exon and part of the intron. Thus, the protein sequence of the entire 289-AA GHS-R1b is identical to GHS-R1a from the N-terminal end to TMD 5.
Extensive investigations were performed to identify the endogenous ligand for the orphan GHS-R1a following discovery of the receptor, and reverse pharmacology facilitated the identification of a natural ligand in 1999 by Kojima et al. (6). The peptide ligand, which contains 28 AAs, was isolated from stomach extracts of rats and named “ghrelin.” Ghrelin has a unique fatty acid modification on its N-terminal third serine (Ser3), with an n-octanoyl group linked to the hydroxyl group of Ser3. This modification is essential for the binding of ghrelin to the receptor (7) and for eliciting various physiological actions. After the discovery of its endogenous ligand, GHS-R1a was found to mediate various physiological functions of ghrelin: neuroendocrine function; appetite regulation; cardiovascular function; gastro-entero-pancreatic function; glucose metabolism; and cell functions including apoptosis, proliferation, and differentiation (8–10).
In non-mammalian vertebrates, GHSs affect the regulation of GH release and of appetite in fish and birds (11–14), suggesting the presence of an endogenous ghrelin-like substance and a corresponding receptor system. We first isolated ghrelin from a non-mammalian vertebrate, the bullfrog (15). Subsequently, ghrelin was determined to be present in various non-mammalian vertebrates, and its physiological effects were gradually revealed [for reviews, see Ref. (16, 17)]. However, investigations of non-mammalian ghrelin receptors still lag behind those on mammalian ghrelin receptors. In this review, we summarize our recent work and those of others on ghrelin receptors in non-mammalian vertebrates and provide a comprehensive discussion of their general features.
Classification and Nomenclature of Ghrelin Receptors
We begin by describing the nomenclature for the ghrelin receptors in mammals, because the nomenclature for the receptors in non-mammalian vertebrates is more complicated and various names have been used based on the presence of splice variants, paralogs, and different AA lengths. In the first description provided by Howard et al. (3), GHS-R1a was defined as a functional receptor induced by agonist-dependent intracellular Ca2+, and GHS-R1b as a splice variant of unknown function. They classified them simply as “a” and “b” because their sequences and functions differed. Thus the names are based on the sequence and structure: “GHS-R1” refers to the receptor with a “type-1” AA sequence, “a” signifies “activated by ghrelin or GHSs,” and “b” indicates “a splice variant of ghsr” which contains the first exon and an unspliced intron that continues the coding sequence in the mRNA and terminates at a stop codon within the intron. The International Union of Pharmacology Committee on Receptor Nomenclature and Drug Classification has accepted “GHS-R1a” as the name for the functional ghrelin receptor (18). Hence, two GHS-Rs exist in mammals: GHS-R1a, which is derived from regular splicing of the gene; and GHS-R1b, which originates from alternative splicing of the gene (Figure 1). On the basis of these names, we describe the naming of the receptors in non-mammalian vertebrates as follows.
The non-mammalian GHS-Rs are also roughly divided into two types: (i) an isoform that arises from regular splicing of the gene and (ii) an isoform derived from alternative splicing of the gene (Figure 1). The former is further classified into two isoforms (Figure 1): one denotes an isoform that we designated “GHS-Ra,” which has structural properties similar to those of the mammalian GHS-R1a and is activated by ghrelin and GHSs. GHS-Ra is further divided into two paralogs “1a” and “2a,” where “GHS-R2a” refers to the receptor with a “type-2” AA sequence distinct from that of GHS-R1a and whose existence is confirmed only in specific fish. The other denotes another isoform that we designated “GHS-R1a-like receptor (GHS-R1a-LR),” which has structural features that differ from those of GHS-Ra and for which intracellular Ca2+ increase in response to ghrelin or GHS treatment is either small or not confirmed. This distinction between GHS-Ra and GHS-R1a-LR is evident in the phylogenetic analysis based on the AA sequences of ghrelin receptors (Figure 2).
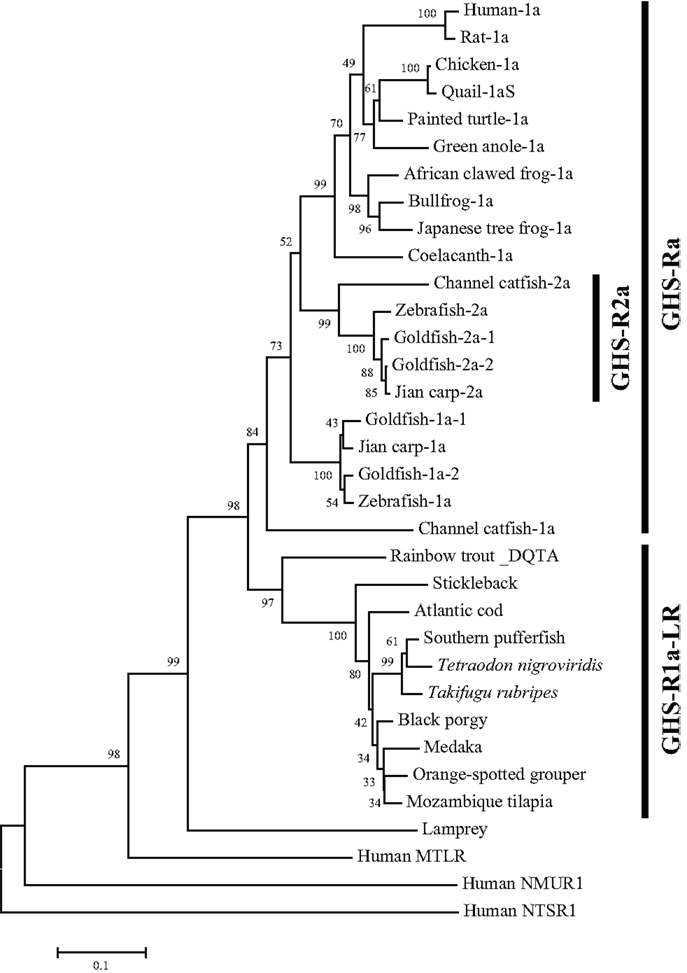
Figure 2. Phylogenetic tree of GHS-Ra and GHS-R1a-LR in non-mammalian vertebrates. The phylogenetic tree was constructed by using the neighbor-joining method with MEGA4 (http://www.megasoftware.net/). The numbers on the branch points are the bootstrap values (as percentages based on 2000 replicates). The scale bar indicates the average number of substitutions per position (a relative measure of evolutionary distance). Receptors for human motilin (MTLR), neuromedin-U (NMUR1), and neurotensin (NTSR1) were used as the outgroup.
The isoforms derived from alternative splicing of the gene are divided into five types: 1b, 1aV (1c), 1bV, tv, and tv-like receptors. These receptors are formed by different modes of alternative splicing and have distinct structures.
Non-Mammalian Vertebrate Species with Sequenced Ghrelin Receptors
We have summarized the non-mammalian vertebrates for which the cDNA or genes of GHS-R have been identified and made available in public databases in Table 1 (fish) and Table 2 (reptiles, amphibians, and birds). The AA sequences of GHS-R1a, 2a; GHS-R1a-LR; and their multiple alignments are shown in Figure 3.
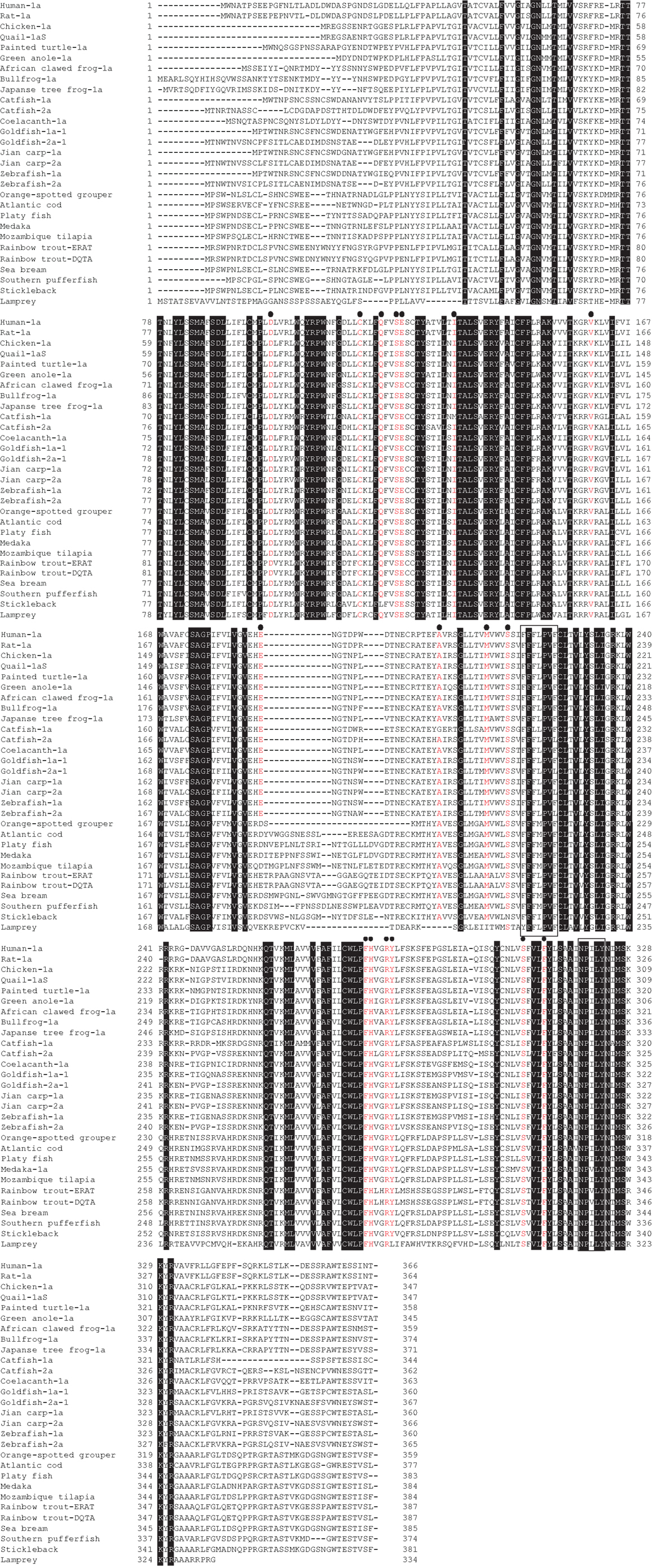
Figure 3. GHS-R1a, 2a and GHS-R1a-LR proteins in non-mammalian vertebrates. Dense shading indicates common amino acids (AAs) in all species. Red letters under black circles represent specific AAs related to ligand binding, selectivity, and constitutive activity of GHS-R1a and 2a. Boxes show the typical motifs of the G-protein-coupled receptor transmembrane domains 5 and 7. Sequences were aligned using GENETYX-Mac version 15.0.1.
Many GHS-Rs have been identified in non-mammalian vertebrates, and the most of the GHS-R types that have been found are present in fish (19 species). With the recent identification of a GHS-R in bullfrog and Japanese tree frog (19), we now know the GHS-Rs for three kinds of frogs, including African clawed frogs. In reptiles, there are no reports about GHS-Rs at present, although the Ensembl genome database search (http://www.ensembl.org/index.html) yields the GHS-R1a gene for the green anole (Anolis carolinensis) and painted turtle (Chrysemys picta bellii). Very recently, massive numbers of partial nucleotide sequences (approximately 450-bp encoding a 150-AA protein) of GHS-R have been registered for 124 species of Squamata, including snakes and Iguanidae, by Wiens et al. (98) at Stony Brook University in the NCBI database. In birds, GHS-Rs have been found in five species.
Structural Features of the Ghrelin Receptor in Non-Mammalian Vertebrates
Three features are prominent in non-mammalian GHS-Rs: (1) the presence of paralogs in a few species of teleosts; (2) two isoforms, GHS-Ra and GHS-R1a-LR; and (3) avian-specific alternative splice forms of GHS-R (Figure 1). Further details are provided below (see also Classification and Nomenclature of Ghrelin Receptors).
Presence of Paralogs in Only a Few Species of Teleosts
The GHS-Ra paralog GHS-R2a is found only in a limited number of teleosts, and little is known about the presence of GHS-R paralogs in other vertebrates. GHS-R2a has an AA sequence that is approximately 70% identical to that of GHS-R1a. At present, this receptor has been identified in Cypriniformes such as goldfish, zebrafish, and carp, and in channel catfish in the order Siluriformes (Figures 2 and 3). The two isoforms are encoded by different genes (i.e., the zebrafish GHS-R1a and 2a genes are located separately on chromosomes 4 and 24, respectively), which are considered to have diverged via the third round of whole-genome duplication (3R-WGD) that occurred in the ray-finned fish lineage (20, 21).
In addition, isoforms with approximately 95% identity have been found in goldfish (Cypriniformes) and rainbow trout (Salmoniformes). In goldfish, there are two paralogs each for GHS-R1a and 2a: GHS-R1a-1, 1a-2, 2a-1, and 2a-2 (Figures 2, 3, and 5). Each receptor originated from a separate gene demonstrated to have a different intron sequence (22). In the rainbow trout, two paralogous sequences, namely the DQTA/LN-type and ERAT/IS-type, have been identified (23) (Figure 3). Their names indicate AA substitutions at D20E, Q32R, T54A, A62T, L168I, and N264S. These two receptor sequences are known to be derived from at least three distinct genes (the DQTA/LN-type derives from two genes and the ERAT/IS-type originates from one gene), on the basis of analyses of an intron sequence of each receptor (23). These paralogs of goldfish and rainbow trout are considered to have originated from polyploidization events that occurred after 3R-WGD (24) and tandem duplication of the genes, which also affected the opsin gene in these species (25). The presence of multiple paralogs may be a peculiar characteristic of Ostariophysi and Protacanthopterygii in euteleosts (20, 21).
Two Ghrelin Receptor Isoforms: GHS-Ra and GHS-R1a-LR
As shown in Figure 1, there are two isoforms in non-mammalian vertebrates: GHS-Ra and GHS-R1a-LR. GHS-Ra includes GHS-R1a and 2a. Tetrapods including mammals, birds, reptiles, and amphibians have GHS-R1a, whereas some bony fish such as Coelacanthiformes, Cypriniformes (e.g., goldfish, carp, and zebrafish), and Siluriformes (e.g., channel catfish) have both GHS-R1a and 2a. GHS-R1a-LRs show considerable AA identity to GHS-R1a, but have a unique structural feature not found in any tetrapod: the second extracellular loop (ECL2) that connects TMD 4 and 5 is notably longer than that of GHS-R1a (Figure 4). In addition, GHS-R1a-LRs have the characteristic that ghrelin or GHS treatment either does not increase intracellular Ca2+ (23, 26) or requires pharmacological doses to activate the receptor (27, 28). This type of receptor is seen in a limited number of fish classified as Percomorpha within the superorder Acanthopterygii, which is the most evolutionally advanced group of teleosts, including Perciformes such as black porgy and tilapia, Gasterosteiformes such as stickleback and medaka, Tetraodontiformes such as pufferfish, and Salmoniformes such as rainbow trout (Figure 3). An exception is the orange-spotted grouper, which belongs to Perciformes but has an ECL2 that is not long (Figure 3). These species have some morphological characteristics such as a highly mobilized upper jaw, a respiratory tract not linked to the swim bladder, and a splinter article in their fins. Salmoniformes belong to Protacanthopterygii, which contains a number of moderately advanced teleosts. This evolutionary background may be reflected in the molecular evolution and structure of the ghrelin receptor.
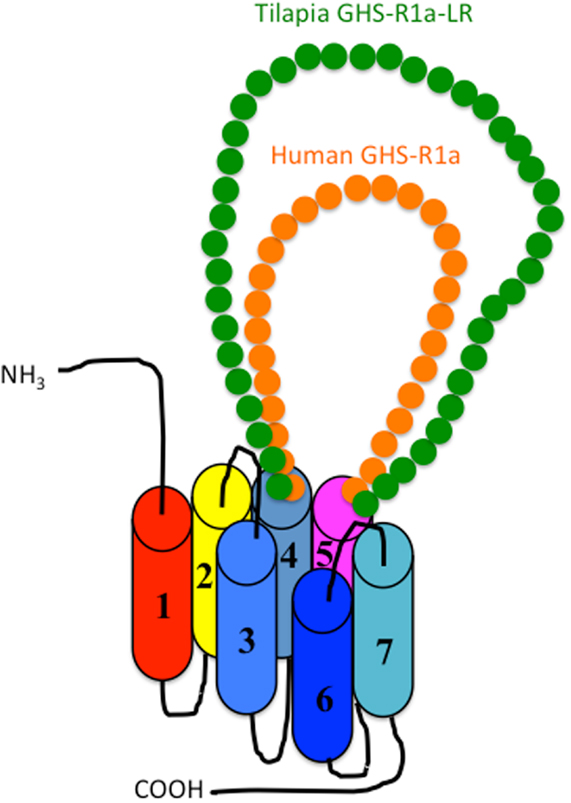
Figure 4. Schematic diagram of the second extracellular loop (ECL2) in the GHS-R1a and GHS-R1a-like receptors. Human GHS-R1a, which has a short ECL2 (orange), and tilapia GHS-R1a-like receptor (GHS-R1a-LR), which has a long ECL2 (green), are shown as representative examples. The length of ECL2 in human GHS-R1a is 28 amino acids (AAs), whereas the ECL2 in tilapia GHS-R1a-LR is 43 AAs. Each receptor is classified in a different branch of the phylogenetic tree (Figure 2). GHS-Ra, which includes GHS-R1a or 2a, is found in tetrapods including chickens (birds), mammals, reptiles, and amphibians, as well as some bony fishes such as Coelacanthiformes, Cypriniformes (e.g., goldfish, carp, and zebrafish), and Siluriformes (channel catfish). These animal species have the receptor with the short ECL2. In contrast, GHS-R1a-LR is found only in a fish group that includes Perciformes such as tilapia, Gasterosteiformes such as stickleback and medaka, Tetraodontiformes such as pufferfish, and Salmoniformes such as rainbow trout.
A partial sequence similar to that of the ghrelin receptor was found in a database for the sea lamprey (Petromyzon marinus). This receptor could not be placed at the branch of GHS-Ra or GHS-R1a-LR in the phylogenetic analysis (Figure 2). The sea lamprey belongs to the group Cyclostomata in the class Agnatha, which is a class of fish with the characteristics of ancient basal vertebrates. Therefore, the receptor in the sea lamprey may contain ancestral characteristics of the ghrelin receptor.
Avian-Specific GHS-Rs
Birds have specific alternative spliced forms of GHS-R other than GHS-R1b, i.e., 1aV (or 1c), 1bV, tv, and tv-like receptor (29–32), which are generated by differential modes of splicing from GHS-R1b. GHS-R1aV (30) and GHS-R1c (29) are identical receptors found in chickens. Here, “V” is considered to mean “variant” (30), whereas Geelissen et al. (29) used the designation “c” to indicate an isoform different from “a” or “b.” We proposed that GHS-R1c should be referred to as GHS-R1aV because the receptor is identical to GHS-R1a with the exception that it lacks 16 AAs (46 bp) in TMD 6 (16). GHS-R1bV is found in quail. Its C-terminal part differs from that of GHS-R1b, and an AA sequence that differs from 1b is translated from the intermediate intron by a frame-shift due to an 8-bp deletion of the intermediate intron of ghsr. GHS-Rtv is found in chickens (31). The signature “tv” was first used by Sirotkin et al. (31), although its meaning is unclear. The composition of GHS-Rtv is complex: two distinct parts of the intermediate intron sequence of ghsr lie between the exon 1 and exon 2 sequences of GHS-R1a [see Ref. (33)]. Kitazawa et al. (32) reported a receptor similar to chicken GHS-Rtv in the Japanese quail. Because the composition was different from that of GHS-Rtv, it was designated as a GHS-Rtv-like receptor and considered to be a possible ortholog of GHS-Rtv. The functions of these avian variants are completely unknown.
Kitazawa et al. (32) reported five isoforms of GHS-Rs in the Japanese quail: GHS-R1a-L, 1a-S, 1aV-L, 1b-L, and 1bV-L. The “L” and “S” appended to GHS-R1a signify the long-type (354 AAs) and short-type (347 AAs) receptors for GHS-R1a, respectively. GHS-R1a-S is a receptor that lacks 7 AAs at the N-terminus of GHS-R1a-L. Two ATG initiation codons are present in the cDNA and the functional codon is unknown.
Tissue Expression of Ghrelin Receptor mRNAs and Their Isoforms
Expression of GHS-Ra and GHS-R1a-LR
In agreement with a wide range of physiological functions of ghrelin, GHS-R1a transcripts have been detected in human tissues such as the brain, heart, lung, liver, kidney, pancreas, stomach, intestines, and adipose tissue (34, 35). In particular, high expression levels have been detected in the pituitary gland (36), which is consistent with the role of ghrelin in regulating GH release. In the brain, where expression levels are relatively high, GHS-R1a mRNA is widely distributed in regions linked to energy homeostasis such as the arcuate nuclei of the hypothalamus; area postrema; nucleus of the solitary tract; the dorsal motor nucleus of the vagus; hippocampus; dopaminergic neurons in the ventral tegmental area and substantia nigra; parasympathetic preganglionic neurons; the dorsal and medial raphe nuclei; and the dentate gyrus (9, 34, 37, 38).
In non-mammalian vertebrates, GHS-R1a or GHS-R1a-LR transcripts have been found in the central nervous system and various peripheral organs. As in humans, predominant expression occurs in the pituitary in channel catfish (39), chickens (29, 30, 40–43), and ducks (44) for GHS-R1a, as well as in the black porgy (28), orange-spotted grouper (45), and rainbow trout (23) for GHS-R1a-LR. However, expression in the pituitary gland is not dominant in all species. In Mozambique tilapia, GHS-R1a-LR mRNA is mainly detected in the brain. The distribution of the ghrelin receptor in other tissues also differs among animal species.
In fish, GHS-R transcripts have been detected in most organs. The genes are expressed in all regions of the brain, including the olfactory bulbs and tracts, telencephalon, diencephalon, optic tectum, vagal lobe, hypothalamus, cerebellum and medulla, and spinal cord. Gene expression has also been detected in the eyes, heart, thymus, liver, stomach, intestine, spleen, gill, gall bladder, muscle, kidney, head kidney, Brockmann bodies, skin, muscle, and gonads (23, 26, 28, 39, 45, 46). In rainbow trout, GHS-R1a-LR mRNA expression has been detected in blood leukocytes and head kidney leukocytes (47). Cypriniformes such as goldfish and zebrafish, as well as Siluriformes such as channel catfish, possess paralogs of GHS-Ra, each of which has different levels and patterns of expression (22, 39, 46).
In amphibians, strong GHS-R1a mRNA expression has been found in brain regions such as the diencephalon and mesencephalon; the stomach and testis; and to a lesser extent in the small and large intestines, adrenal gland, and kidney in the bullfrog (19). In the Japanese tree frog, GHS-R1a transcripts have been detected in almost all tissues examined, although relatively high expression was detected in the duodenum, small and large intestines, and ovary. However, unlike in other animals, pituitary expression was absent in both species (19).
In birds, GHS-R1a mRNA has also been detected in almost all tissues examined. GHS-R1a mRNA is expressed in chicken tissues such as the hypothalamus, telencephalon, cerebrum, cerebellum, optic lobes, brainstem, heart, lung, thymus, liver, spleen, pancreas, proventriculus, gizzard, duodenum, adrenal gland, kidney, gonads, breast muscle, subcutaneous fat, leg muscle, abdominal fat, and uropygial gland (29, 30, 40, 41, 44). Comparing the relative expression levels in these tissues is difficult; nonetheless, the expression levels in the brain, gastrointestinal tract, liver, and spleen appear to be relatively high compared with other tissues, although strain differences may exist (29, 30, 33). In ducks, mRNA expression has been detected in the subcutaneous fat, hypothalamus, small intestine, testis, cerebellum, and cerebrum (44). In the Japanese quail, GHS-R1a mRNA expression was examined only in the gastrointestinal tract (32), where region-specific expression was detected at relatively high levels in the upper and lower intestines such as the esophagus, crop, and colon, but weak levels in the middle portions of the gastrointestinal tract (e.g., the proventriculus, duodenum, gizzard, jejunum, and ileum).
Expression of Ghrelin Receptor Isoforms Other than GHS-Ra and GHS-R1a-LR
Growth hormone secretagogue-receptor type-1b is a splice variant of the mammalian GHS-R. In humans, its mRNA distribution is more widespread than that of GHS-R1a, and varies spatially and quantitatively from that of GHS-R1a (34). This suggests the possibility that GHS-R1b is involved in specific GHS-R1a-independent physiological activities, although these remain unknown.
In non-mammalian vertebrates, there are a few reports on the mRNA distribution of GHS-R1b. First, GHS-R1b mRNA has been detected in the brain of fish. In the black porgy, the level of expression was highest in the telencephalon, followed by the hypothalamus, pituitary, optic tectum, thalamus, and spinal cord, whereas little was detected in peripheral tissues (28). In Mozambique tilapia, the brain is the site with the highest expression of GHS-R1b mRNA, although transcripts were also detected in the stomach, adipose tissue, gill, liver, intestine, spleen, kidney, and muscle (26). In orange-spotted grouper, the expression levels of GHS-R1b mRNA were high in the pituitary, hypothalamus, cerebellum, medulla, spinal cord, gill filament, spleen, liver, stomach, head kidney, kidney, gonad, red muscle, skin, and fat body (45). In rainbow trout, GHS-R1b mRNA was strongly expressed in the pituitary, whereas weak expression was observed in the hypothalamus, pyloric appendage, middle intestine, spleen, and head kidney (23). In channel catfish, the expression level of GHS-R1b mRNA was highest in the pituitary, but it was approximately 400 times lower in most peripheral tissues compared with the expression level of GHS-R1a (39).
In birds, GHS-R1aV or GHS-Rtv mRNA expression was detected in almost all tissues examined, a pattern almost identical to that of GHS-R1a mRNA expression, although expression levels of each isoform differed (29, 30, 33). GHS-Rtv transcripts were first detected in chicken ovaries (31). In Japanese quail, the expression of the GHS-Rtv-like receptor was detected in the gastrointestinal tract but only in the proventriculus and gizzard (32). The function of these avian variants is entirely unknown.
Regulation of Ghrelin Receptor Expression
Satiation and hunger signals regulate ghsr expression. A condition of negative energy balance such as fasting increases GHS-R1a mRNA expression in the hypothalamus and pituitary of rats, while re-feeding restores the increased expression level to a normal level (48, 49). The gene expression of ghsr is affected by various hormonal factors, it is stimulated by ghrelin (5, 49–51), GH-releasing hormone (GHRH) (52), thyroid hormone (53), and glucocorticoid (dexamethasone) (54, 55). In contrast, it is inhibited by GH (56–58), leptin (49), glucocorticoid (50), and insulin-like growth factor-I (IGF-I) (59). These are summarized in Table 3.
Acute or chronic changes in the energy status or environmental conditions appear to have varying effects on ghsr expression in non-mammalian vertebrates (Table 3). In Mozambique tilapia, GHS-R1a-LR mRNA levels in the brain are unaffected by fasting, whereas GHS-R1b mRNA expression is increased (60). Peddu et al. (61) reported acute pre- and post-prandial changes in GHS-R1a-LR and GHS-R1b mRNA expression, whereas pre-GHS-R mRNA levels (immature mRNA, hetero-nuclear RNA) did not reflect changes in feeding status. Riley et al. (62) showed that acute increased blood glucose reduced GHS-R1a-LR mRNA levels in the brain and increased gastric ghrelin mRNA expression as well as plasma ghrelin levels. This change in plasma ghrelin levels is the opposite of that seen in humans or goldfish, where a glucose load decreases plasma ghrelin levels (63, 64). In conditions of chronic negative energy balance, there was no change in the GHS-R1a-LR expression levels in the brains of Atlantic salmon fasted for 14 days (65). In contrast, goldfish GHS-R1a-1 mRNA levels decreased in the vagal lobe and GHS-R1a-2 mRNA levels increased in the liver after 7 days of fasting (22). In bullfrogs, GHS-R1a mRNA expression was up-regulated in the stomach and ventral skin, whereas that in the brain did not change after 10 days of starvation (19). These results suggest that the nutritional condition of the body affects ghrelin receptor expression. Furthermore, GHS-R1a mRNA expression was up-regulated in the brain, stomach, and ventral skin after 10 days of dehydration of tree frog (19). This result may support the view that ghrelin is involved in the regulation of water balance in frogs, as seen in rats (66) and chicks (67).
Hormonal control of ghsr expression has been reported. Ghrelin appears to have a stimulatory effect on ghsr expression in non-mammalian vertebrates, as it does in mammals. However, the effects differ depending on the ghrelin form, receptor isoform, and target tissue. In channel catfish, the C-terminal structure of ghrelin affects ghsr expression (39). In the pituitary, catfish ghrelin-Gly (this is naturally occurring 23-AA ghrelin where Gly is extended at the C-terminus) increased the levels of GHS-R1a mRNA but not of GHS-R2a mRNA. In contrast, catfish ghrelin-amide (22-AA ghrelin with an amide structure at the C-terminus) had no effect on either receptor. In the Brockmann bodies, catfish ghrelin-amide or ghrelin-Gly dramatically increased the GHS-R2a mRNA expression levels with different time courses. In zebrafish, goldfish ghrelin12-amide stimulated the mRNA expression of both GHS-R1a and 2a in the brain, but with different time courses (46). In orange-spotted grouper, rat ghrelin (10−5 M) inhibited the expression of GHS-R1a-LR and GHS-R1b mRNA in the hypothalamus and pituitary (45). In chickens, Geelissen et al. (29) reported that ghrelin down-regulated GHS-R1a and GHS-R1aV mRNA expression in the pituitary in vitro. In another in vitro study, GHRP-6 stimulated the promoter activity of black porgy GHS-R1a-LR expressed in HEK293 cells (68).
The effects of GH or glucocorticoids on non-mammalian ghsr expression also vary depending on the GH species used, target tissue, and GHS-R isoform. In orange-spotted grouper, sea bream GH (10−7 M) did not affect GHS-R1a-LR levels in the hypothalamus but reduced them in the pituitary, whereas it decreased GHS-R1b mRNA levels in both the hypothalamus and pituitary (45). In chickens, bovine GH and corticosterone decreased mRNA expression of both GHS-R1a and GHS-R1aV, but human GHRH1-29 reduced only GHS-R1a mRNA expression in the pituitary in vitro (29).
Yeung et al. (68) analyzed the 5′-flanking region of ghsr in black porgy and identified a number of putative binding sites for transcription factors such as AP1, NF-1, Oct-1, and USF. Changes in ghsr expression during embryogenesis have been reported in orange-spotted grouper (45) and channel catfish (39). In both species, ghsr expression fluctuates depending on the embryonic stage, and the expression levels of GHS-R isoforms are separately regulated.
Signaling Pathways of the Ghrelin Receptor
Howard et al. (3) observed increases in intracellular Ca2+ levels in cells transfected with GHS-R1a. The intracellular signaling of GHS-R1a is mediated by the activation of a G-protein subtype, Gaq/11, which induces the production of inositol triphosphate (IP3), release of Ca2+, and activation of protein kinase C (PKC) (69). These events are seen in cells transfected with GHS-R1a as well as in somatotrophs (70–74).
In addition, GHS-R1a functions in an agonist-independent manner and causes high basal IP3 production in the absence of agonists, indicating that GHS-R1a is a constitutively active receptor (71, 74, 75). This activity in turn triggers phospholipase C (PLC)–PKC-dependent Ca2+ mobilization, which is associated with the L-type voltage-gated calcium channel via PKC. Furthermore, extracellular signal-regulated kinase 1 and 2 (ERK1/2) are activated by GHRP-6. A GHS-R antagonist (d-Lys3)-GHRP-6, was shown to inhibit basal PLC and ERK1/2 activity (76).
When a non-mammalian ghrelin receptor was expressed in mammalian cells, a rise in intracellular Ca2+ was observed with ghrelin or GHSs (19, 22, 27, 28, 32, 77, 78). A similar Ca2+ mobilization was also induced by ghrelin in the primary culture of goldfish pituitary cells (79, 80), which was important for inducing the release of GH and luteinizing hormone (LH) from goldfish somatotrophs (79) and gonadotrophs (80), respectively. Little is known about the intracellular signaling pathways involved.
In addition to binding ghrelin, non-mammalian ghrelin receptors are capable of binding GHSs such as GHRP-2 and GHRP-6; ipamorelin; and L163,255, L692,585, and L163,540, although the agonistic activity varies according to the receptor present in each animal (19, 22, 27, 28, 32, 77). In addition, a GHS-R1a antagonist (d-Lys3)-GHRP-6, is also capable of inhibiting ghrelin binding to the receptor (22). These results indicate that the structural interactions between the ligand and the AAs of the receptor essential for ligand binding and receptor activation are conserved among vertebrates. However, ligand selectivity has been found in the case of GHRP-6 and hexarelin for goldfish GHS-R1a-1, 1a-2, and 2a-2 (Figure 5) (22).
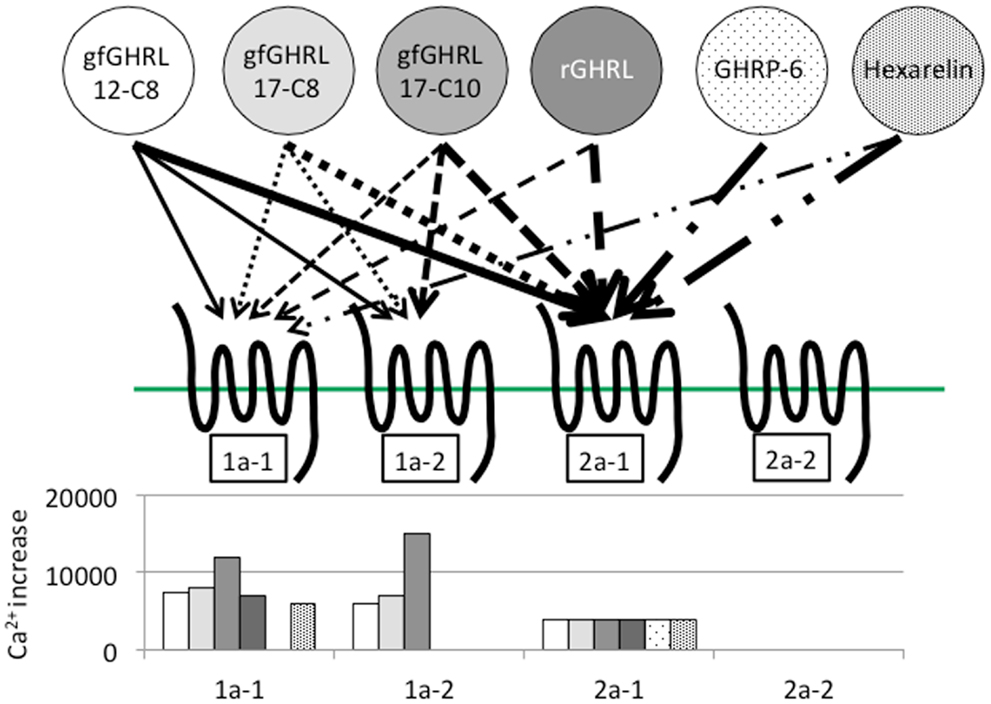
Figure 5. Ligand selectivity and intracellular Ca2+ signaling in four goldfish ghrelin receptors. Four goldfish ghrelin receptors exhibited different ligand selectivity. The schematic figures above show the strength of the ligand-receptor affinity based on the thickness of the arrow, while the bar graphs below show the maximum value of the stimulated increase in the intracellular Ca2+ signal. Goldfish ghrelin (gfGHRL) 12-C8 (octanoylated ghrelin with 12 amino acids, AAs), 17-C8 (octanoylated ghrelin with 17 AAs), and 17-C10 (decanoylated ghrelin with 17 AAs); rat ghrelin (rGHRL); and two GHSs, GHRP-6 and hexarelin, were used in the experiment. For example, the arrows indicate that the intracellular Ca2+ increased in cells expressing GHS-R1a-1 after exposure to gfGHRL12-C8, 17-C8, and 17-C10; rat ghrelin; and hexarelin, but not after exposure to GHRP-6 at a similar dose. The corresponding bar graph shows that gfGHRL17-C10 increased Ca2+ much more strongly than the other agonists. Furthermore, although GHS-R2a-2 was capable of binding all of the agonists examined at a low dose, none of the agonists increased the intracellular Ca2+ level.
In fish-specific GHS-R1a-LRs, particularly of the pufferfish and black porgy, pharmacological doses of receptor agonists are required in some cases to activate the receptors (27, 28), whereas no reaction was found at all in the receptors in tilapia and rainbow trout, even with homologous ghrelin (23, 26). The reason behind this phenomenon remains to be elucidated.
Receptor functionality has not been examined in the African clawed frog or teleosts such as channel catfish, zebrafish, and Jian carp where GHS-Ra has been identified. We expect that these receptors will be responsive to ghrelin or GHS because of their structural properties, such as the short ECL2 loop (Figure 4). However, confirmation of these receptor activities will be required to test this hypothesis in the future.
Key Amino Acids Related to Ligand Selectivity and Receptor Functionality in the Ghrelin Receptor Structure
Feighner et al. (81) reported key AAs that play essential roles in GHS-R1a activation on the basis of the structure of human GHS-R1a and three types of GHSs with different structures, i.e., MK-0677, GHRP-6, and L692,585. Their results showed that D99, C116, E124, M213, S217, and H280 in human GHS-R1a have crucial roles in receptor activation. In particular, M213 is required for the binding of GHRP-6 and L692,585. S217 and H280 are specifically involved with the binding of GHRP-6. In ghrelin receptors identified in non-mammalian vertebrates, all of the AAs listed above are conserved, with the exception of an AA that is equivalent to S217 in the stickleback receptor (Figure 3). This may suggest that the GHS-Ra and GHS-R1a-LR identified in non-mammalian vertebrates have the ability to bind GHSs. However, as described earlier, goldfish GHS-Ra has ligand selectivity (22). In addition, the GHS-R1a-LR in rainbow trout and tilapia shows no Ca2+ response in receptor-expressing mammalian cells (23, 26). Although AAs equivalent to M213, S217, and H280, which are essential for binding of GHRP-6 to the receptor, are all conserved in goldfish GHS-Ra, GHRP-6 does not increase the intracellular Ca2+ in HEK 293 cells expressing goldfish GHS-R1a-1 and 1a-2. Thus, the interaction between the ligand and key AAs in the receptor related to ligand binding may be more complicated than anticipated.
Holst et al. (82) found that the ghrelin receptor elicited strong, ligand-independent signaling in transfected COS-7 or HEK293 cells. Independent of ligand selectivity, the relationship between constitutive receptor activity and the AA composition of the receptor has also been examined (83–85). These studies suggest that of the AAs in human GHS-R1a, V160, F279, A204, I134, and A204 are important for controlling constitutive receptor activity (Figure 3). These AAs are conserved in the GHS-Ra and GHS-R1a-LR identified in non-mammalian vertebrates (Figure 3); therefore, all of them may be constitutively active receptors, although their activity has been confirmed only in the black porgy receptor (86).
Physiological Function of GHS-Rs
GHS-R1a mediates the information conveyed by ghrelin and elicits various physiological functions. In addition to its hypophysiotropic effects and regulation of appetite, ghrelin affects many physiological functions, including gastrointestinal motility, cardiovascular performance, cell proliferation, immune function, bone metabolism, sleep, and the promotion of learning and memory (9, 87, 88). Recent evidence suggests that ghrelin functions as a blood glucose regulator (89).
Roles of GHS-R1a and 2a
Growth hormone secretagogue-receptor type-1a or 2a is thought to mediate various physiological functions of ghrelin, although direct evidence in non-mammalian vertebrates remains sparse. Recently, Yahashi et al. (90) reported that the peripheral effects of ghrelin on food intake and locomotor activity in goldfish are mediated via one of the four ghrelin receptor isoforms, GHS-R2a-1. In addition, ghrelin has the ability to stimulate GH and LH release from goldfish pituitary (64, 79, 80, 91). GHS-R1a-2 mRNA shows the most abundant expression in this structure, suggesting that the receptor is involved in the regulation of pituitary hormone release. Changes in GHS-R1a or 2a expression depending on the energy state suggest the involvement of ghrelin in energy homeostasis, as observed in frogs and goldfish (19, 22). However, no change was observed in the case of tilapia (60). In chickens and quails, the distributions of the receptor are consistent with its role in gut contraction (32). However, although the ghrelin receptor is expressed throughout the intestinal tracts of goldfish and rainbow trout, ghrelin has no effects on intestinal motility (92). This result is in contrast to that seen in zebrafish, in which rat and human ghrelin stimulate gut contraction (93). Further studies are necessary to determine the nature of the relationship between ghrelin receptors and physiological function.
Roles of GHS-R1b
In contrast with GHS-R1a, little is known about the functions of the GHS-R1b isoform. Mammalian and non-mammalian GHS-R1b show no apparent intracellular Ca2+ signaling response to ghrelin or GHSs (32, 86). Co-expression of GHS-R1a and 1b reduces the signaling capacity of GHS-R1a via hetero-dimerization (28, 86, 94), suggesting that GHS-R1b acts as a dominant-negative mutant during signaling via GHS-R1a (86). Intriguingly, GHS-R1b forms heterodimeric associations with other GPCRs such as neurotensin receptor 1 (NTSR1) (95). This heterodimeric receptor binds to peptide hormones other than ghrelin and affects intracellular signaling, i.e., the GHS-R1b/NTSR1 heterodimer binds neuromedin-U and induces cAMP production instead of Ca2+ signaling.
Although GHS-R1b exists in the same gene as GHS-R1a, the sites, patterns, levels, and regulation of GHS-R1b expression differ from those of GHS-R1a. Therefore, elucidation of the physiological function of the receptor is awaited.
Perspective
In this review, we assembled current knowledge about ghrelin receptors in non-mammalian vertebrates. Many questions remain unanswered because receptor genes have been identified only in a limited number of species. However, the functional importance of the ghrelin system is gradually becoming understood in species where the receptor distribution is clear. Presence of unique GHS-Rs such as GHS-R2a, GHS-R1a-LR, or variants found only in non-mammalian vertebrates are interesting in the divergence of the ghrelin system; therefore, examining the structural relationship and function of non-mammalian GHS-Rs based on comparisons with mammalian GHS-Rs is important for understanding the significance of the ghrelin system in vertebrates. However, the ghrelin system of an animal studied may also need to be considered without preconceptions or making comparisons with mammalian data. Thus, the study of non-mammalian GHS-Rs should be interesting and attract many researchers in the future.
Conflict of Interest Statement
The authors declare that the research was conducted in the absence of any commercial or financial relationships that could be construed as a potential conflict of interest.
Acknowledgments
We thank Dr. Christopher A. Loretz (University of Buffalo, Buffalo, NY, USA) for valuable comments on this manuscript. We thank Mrs. Azumi Ooyama for excellent technical assistance. Hiroyuki Kaiya, Mikiya Miyazato, and Kenji Kangawa were supported by a Grant-in-Aid for Scientific Research from the Ministry of Education, Culture, Science, Sports, and Technology (MEXT, KAKENHI) of Japan and by the Takeda Science Foundation.
References
1. Tannenbaum GS, Bowers CY. Interactions of growth hormone secretagogues and growth hormone-releasing hormone/somatostatin. Endocrine (2001) 14:21–7. doi:10.1385/ENDO:14:1:021
2. Laferrère B, Abraham C, Russell CD, Bowers CY. Growth hormone releasing peptide-2 (GHRP-2), like ghrelin, increases food intake in healthy men. J Clin Endocrinol Metab (2005) 90:611–4. doi:10.1210/jc.2004-1719
3. Howard AD, Feighner SD, Cully DF, Arena JP, Liberator PA, Rosenblum CI, et al. A receptor in pituitary and hypothalamus that functions in growth hormone release. Science (1996) 273:974–7. doi:10.1126/science.273.5277.974
4. McKee KK, Palyha OC, Feighner SD, Hreniuk DL, Tan CP, Phillips MS, et al. Molecular analysis of rat pituitary and hypothalamic growth hormone secretagogue receptors. Mol Endocrinol (1997) 11:415–23. doi:10.1210/me.11.4.415
5. Petersenn S. Structure and regulation of the growth hormone secretagogue receptor. Minerva Endocrinol (2002) 27:243–56.
6. Kojima M, Hosoda H, Date Y, Nakazato M, Matuso H, Kangawa K. Ghrelin is a growth-hormone-releasing acylated peptide. Nature (1999) 402:656–60. doi:10.1038/45230
7. Großauer J, Kosol S, Schrank E, Zangger K. The peptide hormone ghrelin binds to membrane-mimetics via its octanoyl chain and an adjacent phenylalanine. Bioorg Med Chem (2010) 18:5483–8. doi:10.1016/j.bmc.2010.06.062
8. Hosoda H, Kojima M, Kangawa K. Biological, physiological, and pharmacological aspects of ghrelin. J Pharmacol Sci (2006) 100:398–410. doi:10.1254/jphs.CRJ06002X
9. Chen CY, Asakawa A, Fujimiya M, Lee SD, Inui A. Ghrelin gene products and the regulation of food intake and gut motility. Pharmacol Rev (2009) 61:430–81. doi:10.1124/pr.109.001958
10. Kojima M, Kangawa K. Ghrelin: more than endogenous growth hormone secretagogue. Ann N Y Acad Sci (2010) 1200:140–8. doi:10.1111/j.1749-6632.2010.05516.x
11. Bowers CY, Momany FA, Reynolds GA, Hong A. On the in vitro and in vivo activity of a new synthetic hexapeptide that acts on the pituitary to specifically release growth hormone. Endocrinology (1984) 114:1537–45. doi:10.1210/endo-114-5-1537
12. Geris KL, Hickey GJ, Berghman LR, Visser TJ, Kühn ER, Darras VM. Pituitary and extrapituitary action sites of the novel nonpeptidyl growth hormone (GH) secretagogue L-692,429 in the chicken. Gen Comp Endocrinol (1998) 111:186–96. doi:10.1006/gcen.1998.7102
13. Geris KL, Hickey GJ, Vanderghote A, Kühn ER, Darras VM. Synthetic growth hormone secretagogues control growth hormone secretion in the chicken at pituitary and hypothalamic levels. Endocrine (2001) 14:67–72. doi:10.1385/ENDO:14:1:067
14. Shepherd BS, Eckert SM, Parhar IS, Vijayan MM, Wakabayashi I, Hirano T, et al. The hexapeptide KP-102 (D-Ala-D-beta-Nal-Ala-Trp-D-Phe-Lys-NH(2)) stimulates growth hormone release in a cichlid fish (Oreochromis mossambicus). J Endocrinol (2000) 167:R7–10. doi:10.1677/joe.0.167R007
15. Kaiya H, Kojima M, Hosoda H, Koda A, Yamamoto K, Kitajima Y, et al. Bullfrog ghrelin is modified by n-octanoic acid at its third threonine residue. J Biol Chem (2001) 276:40441–8. doi:10.1074/jbc.M105212200
16. Kaiya H, Miyazato M, Kangawa K, Peter RE, Unniappan S. Ghrelin: a multifunctional hormone in non-mammalian vertebrates. Comp Biochem Physiol A Mol Integr Physiol (2008) 149:109–28. doi:10.1016/j.cbpa.2007.12.004
17. Kaiya H, Miyazato M, Kangawa K. Recent advances in the phylogenetic study of ghrelin. Peptides (2011) 32:2155–74. doi:10.1016/j.peptides.2011.04.027
18. Davenport AP, Bonner TI, Foord SM, Harmar AJ, Neubig RR, Pin JP, et al. International Union of Pharmacology. LVI. Ghrelin receptor nomenclature, distribution, and function. Pharmacol Rev (2005) 57:541–6. doi:10.1124/pr.57.4.1
19. Kaiya H, Koizumi Y, Konno N, Yamamoto K, Uchiyama M, Kangawa K, et al. Ghrelin receptor in two species of anuran amphibian, bullfrog (Rana catesbeiana), and Japanese tree frog (Hyla japonica). Front Endocrinol (Lausanne) (2011) 2:31. doi:10.3389/fendo.2011.00031
20. Meyer A, Schartl M. Gene and genome duplications in vertebrates: the one-to-four (-to-eight in fish) rule and the evolution of novel gene functions. Curr Opin Cell Biol (1999) 11:699–704. doi:10.1016/S0955-0674(99)00039-3
21. Jaillon O, Aury JM, Brunet F, Petit JL, Stange-Thomann N, Mauceli E, et al. Genome duplication in the teleost fish Tetraodon nigroviridis reveals the early vertebrate proto-karyotype. Nature (2004) 431:946–57. doi:10.1038/nature03025
22. Kaiya H, Miura T, Matsuda K, Miyazato M, Kangawa K. Two functional growth hormone secretagogue receptor (ghrelin receptor) type 1a and 2a in goldfish, Carassius auratus. Mol Cell Endocrinol (2010) 327:25–39. doi:10.1016/j.mce.2010.06.004
23. Kaiya H, Mori T, Miyazato M, Kangawa K. Ghrelin receptor (GHS-R)-like receptor and its genomic organisation in rainbow trout, Oncorhynchus mykiss. Comp Biochem Physiol A Mol Integr Physiol (2009) 153:438–50. doi:10.1016/j.cbpa.2009.04.612
24. Leggatt RA, Iwama GK. Occurrence of polyploidy in the fishes. NULL (2003) 13:237–46. doi:10.1023/B:RFBF.0000033049.00668.fe
25. Rennison DJ, Owens GL, Taylor JS. Opsin gene duplication and divergence in ray-finned fish. Mol Phylogenet Evol (2012) 62:986–1008. doi:10.1016/j.ympev.2011.11.030
26. Kaiya H, Riley LG, Janzen W, Hirano T, Grau EG, Miyazato M, et al. Identification and genomic sequence of a ghrelin receptor (GHS-R)-like receptor in the Mozambique tilapia, Oreochromis mossambicus. Zool Sci (2009) 26:330–7. doi:10.2108/zsj.26.330
27. Palyha OC, Feighner SD, Tan CP, McKee KK, Hreniuk DL, Gao YD, et al. Ligand activation domain of human orphan growth hormone (GH) secretagogue receptor (GHS-R) conserved from pufferfish to humans. Mol Endocrinol (2000) 14:160–9. doi:10.1210/me.14.1.160
28. Chan CB, Cheng CH. Identification and functional characterization of two alternatively spliced growth hormone secretagogue receptor transcripts from the pituitary of black seabream, Acanthopagrus schlegeli. Mol Cell Endocrinol (2004) 214:81–95. doi:10.1016/j.mce.2003.11.020
29. Geelissen SM, Beck IM, Darras VM, Kühn ER, Van der Geyten S. Distribution and regulation of chicken growth hormone secretagogue receptor isoforms. Gen Comp Endocrinol (2003) 134:167–74. doi:10.1016/S0016-6480(03)00250-8
30. Tanaka M, Miyazaki T, Yamamoto I, Nakai N, Ohta Y, Tsushima N, et al. Molecular characterization of chicken growth hormone secretagogue receptor gene. Gen Comp Endocrinol (2003) 134:198–202. doi:10.1016/S0016-6480(03)00247-8
31. Sirotkin AV, Grossmann R, María-Peon MT, Roa J, Tena-Sempere M, Klein S. Novel expression and functional role of ghrelin in chicken ovary. Mol Cell Endocrinol (2006) 257–258:15–25. doi:10.1016/j.mce.2006.06.004
32. Kitazawa T, Maeda Y, Kaiya H. Molecular cloning of growth hormone secretagogue-receptor and effect of quail ghrelin on gastrointestinal motility in Japanese quail. Regul Pept (2009) 158:132–42. doi:10.1016/j.regpep.2009.07.005
33. Richards MP, McMurtry JP. The avian proghrelin system. Int J Pept (2010) 2010:749401. doi:10.1155/2010/749401
34. Gnanapavan S, Kola B, Bustin SA, Morris DG, McGee P, Fairclough P, et al. The tissue distribution of the mRNA of ghrelin and subtypes of its receptor, GHS-R, in humans. J Clin Endocrinol Metab (2002) 87:2988–91. doi:10.1210/jc.87.6.2988
35. Sun Y, Garcia JM, Smith RG. Ghrelin and growth hormone secretagogue receptor expression in mice during aging. Endocrinology (2007) 148:1323–9. doi:10.1210/en.2006-0782
36. Ueberberg B, Unger N, Saeger W, Mann K, Petersenn S. Expression of ghrelin and its receptor in human tissues. Horm Metab Res (2009) 1:814–21. doi:10.1055/s-0029-1233462
37. Guan XM, Yu H, Palyha OC, McKee KK, Feighner SD, Sirinathsinghji DJ, et al. Distribution of mRNA encoding the growth hormone secretagogue receptor in brain and peripheral tissues. Brain Res Mol Brain Res (1997) 48:23–9. doi:10.1016/S0169-328X(97)00071-5
38. Zigman JM, Jones JE, Lee CE, Saper CB, Elmquist JK. Expression of ghrelin receptor mRNA in the rat and the mouse brain. J Comp Neurol (2006) 494:528–48. doi:10.1002/cne.20823
39. Small BC, Quiniou SM, Kaiya H. Sequence, genomic organization and expression of two channel catfish, Ictalurus punctatus, ghrelin receptors. Comp Biochem Physiol A Mol Integr Physiol (2009) 154:451–64. doi:10.1016/j.cbpa.2009.07.027
40. Saito E-S, Kaiya H, Tachibana T, Tomonaga S, Denbow DM, Kangawa K, et al. Inhibitory effect of ghrelin on food intake is mediated by the corticotropin-releasing factor system in neonatal chicks. Regul Pept (2005) 125:201–8. doi:10.1016/j.regpep.2004.09.003
41. Richards MP, Poch SM, McMurtry JP. Characterization of turkey and chicken ghrelin genes, and regulation of ghrelin and ghrelin receptor mRNA levels in broiler chickens. Gen Comp Endocrinol (2006) 145:298–310. doi:10.1016/j.ygcen.2005.09.013
42. Yamamoto I, Kaiya H, Tsutsui C, Sakai T, Tsukada A, Miyazato M, et al. Primary structure, tissue distribution, and biological activity of chicken motilin receptor. Gen Comp Endocrinol (2008) 156:509–14. doi:10.1016/j.ygcen.2008.03.007
43. Yamamoto I, Yoshimura Y, Tsukada A, Kansaku N, Tsushima N, Tanaka M. Predominant expression of growth hormone secretagogue receptor in the caudal lobe of chicken pituitary and parallel developmental increase in expression of pituitary GH and proventriculus ghrelin mRNA. NULL (2008) 79:116–21. doi:10.1111/j.1740-0929.2007.00506.x
44. Nie Q, Fang M, Xie L, Peng X, Xu H, Luo C, et al. Molecular characterization of the ghrelin and ghrelin receptor genes and effects on fat deposition in chicken and duck. J Biomed Biotechnol (2009) 2009:567120. doi:10.1155/2009/567120
45. Chen T, Tang Z, Yan A, Li W, Lin H. Molecular cloning and mRNA expression analysis of two GH secretagogue receptor transcripts in orange-spotted grouper (Epinephelus coioides). J Endocrinol (2008) 199:253–65. doi:10.1677/JOE-08-0325
46. Cruz SA, Tseng YC, Kaiya H, Hwang PP. Ghrelin affects carbohydrate-glycogen metabolism via insulin inhibition and glucagon stimulation in the zebrafish (Danio rerio) brain. Comp Biochem Physiol A Mol Integr Physiol (2010) 156:190–200. doi:10.1016/j.cbpa.2010.01.019
47. Yada T, Kaiya H, Mutoh K, Azuma T, Hyodo S, Kangawa K. Ghrelin stimulates phagocytosis and superoxide production in fish leucocytes. J Endocrinol (2006) 189:57–65. doi:10.1677/joe.1.06187
48. Kim MS, Yoon CY, Park KH, Shin CS, Park KS, Kim SY, et al. Changes in ghrelin and ghrelin receptor expression according to feeding status. Neuroreport (2003) 4:1317–20. doi:10.1097/00001756-200307180-00006
49. Nogueiras R, Tovar S, Mitchell SE, Rayner DV, Archer ZA, Dieguez C, et al. Regulation of growth hormone secretagogue receptor gene expression in the arcuate nuclei of the rat by leptin and ghrelin. Diabetes (2004) 53:2552–8. doi:10.2337/diabetes.53.10.2552
50. Kaji H, Kishimoto M, Kirimura T, Iguchi G, Murata M, Yoshioka S, et al. Hormonal regulation of the human ghrelin receptor gene transcription. Biochem Biophys Res Commun (2001) 284:660–6. doi:10.1006/bbrc.2001.5035
51. Barreiro ML, Suominen JS, Gaytán F, Pinilla L, Chopin LK, Casanueva FF, et al. Developmental, stage-specific, and hormonally regulated expression of growth hormone secretagogue receptor messenger RNA in rat testis. Biol Reprod (2003) 68:1631–40. doi:10.1095/biolreprod.102.008862
52. Kineman RD, Kamegai J, Frohman LA. Growth hormone (GH)-releasing hormone (GHRH) and the GH secretagogue (GHS), L692,585, differentially modulate rat pituitary GHS receptor and GHRH receptor messenger ribonucleic acid levels. Endocrinology (1999) 140:3581–6. doi:10.1210/en.140.8.3581
53. Kamegai J, Tamura H, Ishii S, Sugihara H, Wakabayashi I. Thyroid hormones regulate pituitary growth hormone secretagogue receptor gene expression. J Neuroendocrinol (2001) 13:275–8. doi:10.1046/j.1365-2826.2001.00623.x
54. Tamura H, Kamegai J, Sugihara H, Kineman RD, Frohman LA, Wakabayashi I. Glucocorticoids regulate pituitary growth hormone secretagogue receptor gene expression. J Neuroendocrinol (2000) 12:481–5. doi:10.1046/j.1365-2826.2000.00446.x
55. Thomas GB, Bennett PA, Carmignac DF, Robinson IC. Glucocorticoid regulation of growth hormone (GH) secretagogue-induced growth responses and GH secretagogue receptor expression in the rat. Growth Horm IGF Res (2000) 10:45–52. doi:10.1054/ghir.1999.0138
56. Bennett PA, Thomas GB, Howard AD, Feighner SD, van der Ploeg LH, Smith RG, et al. Hypothalamic growth hormone secretagogue-receptor (GHS-R) expression is regulated by growth hormone in the rat. Endocrinology (1997) 138:4552–7. doi:10.1210/en.138.11.4552
57. Kamegai J, Wakabayashi I, Miyamoto K, Unterman TG, Kineman RD, Frohman LA. Growth hormone-dependent regulation of pituitary GH secretagogue receptor (GHS-R) mRNA levels in the spontaneous dwarf rat. Neuroendocrinology (1998) 68:312–8. doi:10.1159/000054379
58. Nass R, Gilrain J, Anderson S, Gaylinn B, Dalkin A, Day R, et al. High plasma growth hormone (GH) levels inhibit expression of GH secretagogue receptor messenger ribonucleic acid levels in the rat pituitary. Endocrinology (2000) 141:2084–9. doi:10.1210/en.141.6.2084
59. Kamegai J, Tamura H, Shimizu T, Ishii S, Sugihara H, Oikawa S. Insulin-like growth factor-I down-regulates ghrelin receptor (growth hormone secretagogue receptor) expression in the rat pituitary. Regul Pept (2005) 127:203–6. doi:10.1016/j.regpep.2004.12.001
60. Riley LG, Fox BK, Breves JP, Kaiya H, Dorough CP, Hirano T, et al. Absence of effects of short-term fasting on plasma ghrelin and brain expression of ghrelin receptors in the tilapia, Oreochromis mossambicus. Zool Sci (2008) 25:821–7. doi:10.2108/zsj.25.821
61. Peddu SC, Breves JP, Kaiya H, Gordon GE, Riley LG Jr. Pre- and postprandial effects on ghrelin signaling in the brain and on the GH/IGF-I axis in the Mozambique tilapia (Oreochromis mossambicus). Gen Comp Endocrinol (2009) 161:412–8. doi:10.1016/j.ygcen.2009.02.008
62. Riley LG Jr., Walker AP, Dorough CP, Schwandt SE, Grau EG. Glucose regulates ghrelin, neuropeptide Y, and the GH/IGF-I axis in the tilapia, Oreochromis mossambicus. Comp Biochem Physiol A Mol Integr Physiol (2009) 154:541–6. doi:10.1016/j.cbpa.2009.08.018
63. Shiiya T, Nakazato M, Mizuta M, Date Y, Mondal MS, Tanaka M, et al. Plasma ghrelin levels in lean and obese humans and the effect of glucose on ghrelin secretion. J Clin Endocrinol Metab (2002) 87:240–4. doi:10.1210/jc.87.1.240
64. Unniappan S, Canosa LF, Peter RE. Orexigenic actions of ghrelin in goldfish: feeding-induced changes in brain and gut mRNA expression and serum levels, and responses to central and peripheral injections. Neuroendocrinology (2004) 79:100–8. doi:10.1159/000076634
65. Hevrøy EM, Azpeleta C, Shimizu M, Lanzén A, Kaiya H, Espe M, et al. Effects of short-term starvation on ghrelin, GH-IGF system, and IGF-binding proteins in Atlantic salmon. Fish Physiol Biochem (2011) 37:217–32. doi:10.1007/s10695-010-9434-3
66. Hashimoto H, Ueta Y. Central effects of ghrelin, a unique peptide, on appetite and fluid/water drinking behavior. Curr Protein Pept Sci (2011) 12:280–7. doi:10.2174/138920311795906727
67. Tachibana T, Kaiya H, Denbow DM, Kangawa K, Furuse M. Central ghrelin acts as an anti-dipsogenic peptide in chicks. Neurosci Lett (2006) 405:241–5. doi:10.1016/j.neulet.2006.07.019
68. Yeung CM, Chan CB, Cheng CH. Isolation and characterization of the 5’-flanking region of the growth hormone secretagogue receptor gene from black seabream Acanthopagrus schlegeli. Mol Cell Endocrinol (2004) 223:5–15. doi:10.1016/j.mce.2004.06.005
69. Wettschureck N, Moers A, Wallenwein B, Parlow AF, Maser-Gluth C, Offermanns S. Loss of Gq/11 family G proteins in the nervous system causes pituitary somatotroph hypoplasia and dwarfism in mice. Mol Cell Biol (2005) 25:1942–8. doi:10.1128/MCB.25.5.1942-1948.2005
70. Cheng K, Chan WW, Butler B, Barreto A Jr., Smith RG. Evidence for a role of protein kinase-C in His-D-Trp-Ala-Trp-D-Phe-Lys-NH2-induced growth hormone release from rat primary pituitary cells. Endocrinology (1991) 129:3337–42. doi:10.1210/endo-129-6-3337
71. Herrington J, Hille B. Growth hormone-releasing hexapeptide elevates intracellular calcium in rat somatotropes by two mechanisms. Endocrinology (1994) 135:1100–8. doi:10.1210/en.135.3.1100
72. Lei T, Buchfelder M, Fahlbusch R, Adams EF. Growth hormone releasing peptide (GHRP-6) stimulates phosphatidylinositol (PI) turnover in human pituitary somatotroph cells. J Mol Endocrinol (1995) 14:135–8. doi:10.1677/jme.0.0140135
73. Bresson-Bépoldin L, Dufy-Barbe L. GHRP6-stimulated hormone secretion in somatotrophs: involvement of intracellular and extracellular calcium sources. J Neuroendocrinol (1996) 8:309–14. doi:10.1046/j.1365-2826.1996.04608.x
74. Lania A, Ballaré E, Corbetta S, Filopanti M, Persani L, Spada A. Growth hormone-releasing hexapeptide (GHRP-6) increases intracellular calcium concentrations in cultured cells from human pituitary adenomas of different types. Eur J Endocrinol (1998) 139:343–8. doi:10.1530/eje.0.1390343
75. Holst B, Brandt E, Bach A, Heding A, Schwartz TW. Nonpeptide and peptide growth hormone secretagogues act both as ghrelin receptor agonist and as positive or negative allosteric modulators of ghrelin signaling. Mol Endocrinol (2005) 19:2400–11. doi:10.1210/me.2005-0059
76. Chu KM, Chow KB, Leung PK, Lau PN, Chan CB, Cheng CH, et al. Over-expression of the truncated ghrelin receptor polypeptide attenuates the constitutive activation of phosphatidylinositol-specific phospholipase C by ghrelin receptors but has no effect on ghrelin-stimulated extracellular signal-regulated kinase 1/2 activity. Int J Biochem Cell Biol (2007) 39:752–64.
77. Chan CB, Leung PK, Wise H, Cheng CH. Signal transduction mechanism of the seabream growth hormone secretagogue receptor. FEBS Lett (2004) 577:147–53. doi:10.1016/j.febslet.2004.08.088
78. Tachibana T, Tanaka M, Kaiya H. Central injection of des-acyl chicken ghrelin does not affect food intake in chicks. Gen Comp Endocrinol (2011) 171:183–8. doi:10.1016/j.ygcen
79. Grey CL, Chang JP. Ghrelin-induced growth hormone release from goldfish pituitary cells involves voltage-sensitive calcium channels. Gen Comp Endocrinol (2009) 160:148–57. doi:10.1016/j.ygcen.2008.11.006
80. Grey CL, Grayfer L, Belosevic M, Chang JP. Ghrelin stimulation of gonadotropins (LH) release from goldfish pituitary cells: presence of the growth hormone secretagogue receptor (GHS-R1a) and involvement of voltage-sensitive Ca2+ channels. Mol Cell Endocrinol (2010) 317:64–77. doi:10.1016/j.mce.2009.12.024
81. Feighner SD, Howard AD, Prendergast K, Palyha OC, Hreniuk DL, Nargund R, et al. Structural requirements for the activation of the human growth hormone secretagogue receptor by peptide and nonpeptide secretagogues. Mol Endocrinol (1998) 2:137–45.
82. Holst B, Cygankiewicz A, Jensen TH, Ankersen M, Schwartz TW. High constitutive signaling of the ghrelin receptor – identification of a potent inverse agonist. Mol Endocrinol (2003) 217:2201–10. doi:10.1210/me.2003-0069
83. Holst B, Holliday ND, Bach A, Elling CE, Cox HM, Schwartz TW. Common structural basis for constitutive activity of the ghrelin receptor family. J Biol Chem (2004) 279:53806–17. doi:10.1074/jbc.M407676200
84. Liu G, Fortin JP, Beinborn M, Kopin AS. Four missense mutations in the ghrelin receptor result in distinct pharmacological abnormalities. J Pharmacol Exp Ther (2007) 322:1036–43. doi:10.1124/jpet.107.123141
85. Rediger A, Piechowski CL, Yi CX, Tarnow P, Strotmann R, Grüters A, et al. Mutually opposite signal modulation by hypothalamic heterodimerization of ghrelin and melanocortin-3 receptors. J Biol Chem (2011) 86:39623–31. doi:10.1074/jbc.M111.287607
86. Leung PK, Chow KB, Lau PN, Chu KM, Chan CB, Cheng CH, et al. The truncated ghrelin receptor polypeptide (GHS-R1b) acts as a dominant-negative mutant of the ghrelin receptor. Cell Signal (2007) 19:1011–22. doi:10.1016/j.cellsig.2006.11.011
87. Diano S, Farr SA, Benoit SC, McNay EC, da Silva I, Horvath B, et al. Ghrelin controls hippocampal spine synapse density and memory performance. Nat Neurosci (2006) 9:381–8. doi:10.1038/nn1656
88. Carlini VP, Perez MF, Salde E, Schiöth HB, Ramirez OA, de Barioglio SR. Ghrelin induced memory facilitation implicates nitric oxide synthase activation and decrease in the threshold to promote LTP in hippocampal dentate gyrus. Physiol Behav (2010) 101:117–23. doi:10.1016/j.physbeh.2010.04.026
89. Verhulst PJ, Depoortere I. Ghrelin’s second life: from appetite stimulator to glucose regulator. World J Gastroenterol (2012) 18:3183–95. doi:10.3748/wjg.v18.i25.3183
90. Yahashi S, Kang KS, Kaiya H, Matsuda K. GHRP-6 mimics ghrelin-induced stimulation of food intake and suppression of locomotor activity in goldfish. Peptides (2012) 34:324–8. doi:10.1016/j.peptides.2012.01.025
91. Unniappan S, Peter RE. In vitro and in vivo effects of ghrelin on luteinizing hormone and growth hormone release in goldfish. Am J Physiol Regul Integr Comp Physiol (2004) 286:R1093–101. doi:10.1152/ajpregu.00669.2003
92. Kitazawa T, Itoh K, Yaosaka N, Maruyama K, Matsuda K, Teraoka H, et al. Ghrelin does not affect gastrointestinal contractility in rainbow trout and goldfish in vitro. Gen Comp Endocrinol (2012) 178:539–45. doi:10.1016/j.ygcen
93. Olsson C, Holbrook JD, Bompadre G, Jönsson E, Hoyle CH, Sanger GJ, et al. Identification of genes for the ghrelin and motilin receptors and a novel related gene in fish, and stimulation of intestinal motility in zebrafish (Danio rerio) by ghrelin and motilin. Gen Comp Endocrinol (2008) 155:217–26. doi:10.1016/j.ygcen.2007.05.016
94. Chow KB, Sun J, Chu KM, Tai Cheung W, Cheng CH, Wise H. The truncated ghrelin receptor polypeptide (GHS-R1b) is localized in the endoplasmic reticulum where it forms heterodimers with ghrelin receptors (GHS-R1a) to attenuate their cell surface expression. Mol Cell Endocrinol (2012) 348:247–54. doi:10.1016/j.mce.2011.08.034
95. Takahashi K, Furukawa C, Takano A, Ishikawa N, Kato T, Hayama S, et al. The neuromedin U-growth hormone secretagogue receptor 1b/neurotensin receptor 1 oncogenic signaling pathway as a therapeutic target for lung cancer. Cancer Res (2006) 66:9408–19. doi:10.1158/0008-5472.CAN-06-1349
96. Wang H, Gao FY, Huang ZH, Lu MX. Cloning and expression analysis of tilapia growth hormone secretagogue receptor (Unpublished, database only).
97. Wang H, Gao FY, Lu MX, Zhu HP, Huang ZH. Isolation and characterization of the 5′-flanking region of the growth hormone secretagogue receptor gene from O. hornorum. (Unpublished, database only).
98. Wiens JJ, Hutter CR, Mulcahy DG, Noonan BP, Townsend TM, Sites JW Jr., et al. Resolving the phylogeny of lizards and snakes (Squamata) with extensive sampling of genes and species. Biol Lett (2012) 8:1043–6. doi:10.1098/rsbl.2012.0703
Keywords: ghrelin, ghrelin receptor, GHS-R, GHS-R-like receptor, fishes, amphibians, reptiles, birds
Citation: Kaiya H, Kangawa K and Miyazato M (2013) Ghrelin receptors in non-mammalian vertebrates. Front. Endocrinol. 4:81. doi: 10.3389/fendo.2013.00081
Received: 27 November 2012; Paper pending published: 10 December 2012;
Accepted: 20 June 2013; Published online: 17 July 2013.
Edited by:
Hubert Vaudry, University of Rouen, FranceReviewed by:
Hélène Volkoff, Memorial University of Newfoundland, CanadaCatharina Olsson, University of Gothenburg, Sweden
Copyright: © 2013 Kaiya, Kangawa and Miyazato. This is an open-access article distributed under the terms of the Creative Commons Attribution License, which permits use, distribution and reproduction in other forums, provided the original authors and source are credited and subject to any copyright notices concerning any third-party graphics etc.
*Correspondence: Hiroyuki Kaiya, Department of Biochemistry, National Cerebral and Cardiovascular Center Research Institute, 5-7-1 Fujishirodai, Suita, Osaka 565-8565, Japan e-mail: kaiya@ncvc.go.jp