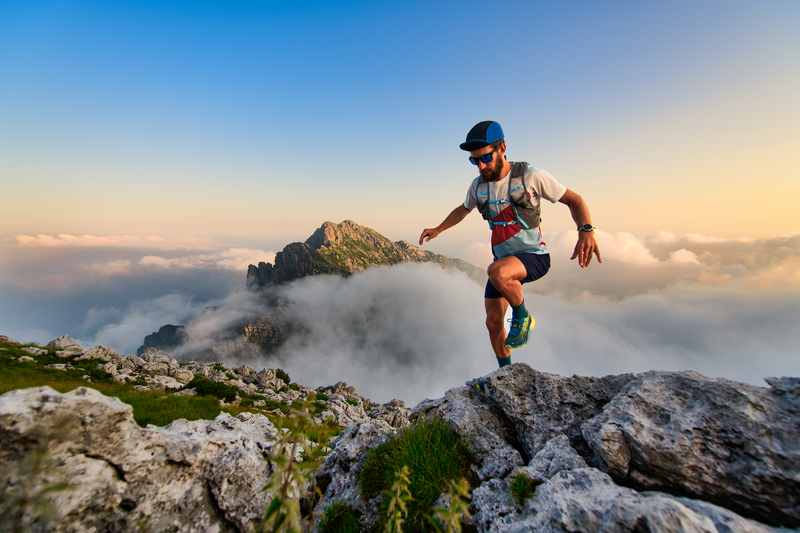
95% of researchers rate our articles as excellent or good
Learn more about the work of our research integrity team to safeguard the quality of each article we publish.
Find out more
PERSPECTIVE article
Front. Endocrinol. , 14 March 2012
Sec. Cellular Endocrinology
Volume 3 - 2012 | https://doi.org/10.3389/fendo.2012.00035
This article is part of the Research Topic Biology and therapeutic potential of brown adipose tissue View all 16 articles
Recent identification of active brown fat reserves in adult humans has re-stimulated interest in the role of brown adipocytes in energy homeostasis. In addition, there is accumulating evidence to support the concept of an alteration in energy balance through acquisition of brown fat features in traditional white fat depots. We recently described an important role played by the TGF-β/Smad3 signaling pathway in modulating the appearance of brown adipocytes in traditional white fat, and its implications to thermogenesis, mitochondrial energetics, energy expenditure, and protection from diabetes and obesity. Here we review the data supporting this phenomenon and put into perspective the promise of conversion of white fat to a brown fat state as a potential therapeutic option for obesity and diabetes.
The rise in the worldwide incidence of obesity and, by a tight correlation, diabetes is proof that we are failing as a populace to obey the energy balance equation. Obesity is principally characterized by accumulation of fat in adipose tissue (Gesta et al., 2007; Park et al., 2008). In conditions of energy excess, the white adipose tissue accumulates fat in the form of triglycerides, whilst brown adipose tissue has the potential stimulate energy expenditure by dissipation of fat to produce heat and maintain body temperature. White fat comprises of large unilocular lipid-containing adipocytes with few mitochondria. In contrast, brown fat comprises of small multilocular cells with abundant mitochondria. Brown adipocytes are uniquely characterized by the expression of uncoupling protein-1 (UCP1). Copious amount of brown fat exists in rodents and human infants and it was considered to be non-existent in adult humans. Recent findings that metabolically active brown fat exists in humans (Nedergaard et al., 2007; Cypess et al., 2009; van Marken Lichtenbelt et al., 2009; Virtanen et al., 2009) have stimulated interest concerning the therapeutic potential of augmenting brown fat to combat metabolic diseases (Enerback, 2010; Nedergaard and Cannon, 2010). Further, it appears that brown fat shares its developmental origin with muscle, and not white fat as it was long presumed (Atit et al., 2006; Timmons et al., 2007). Seale et al. (2008, 2009) provided the formal proof that brown fat is related to skeletal muscle and further showed that the transcription factor PRDM16 determines the fate of Myf5+-precursor cells toward brown fat lineage.
Brown adipocytes are also found interspersed within the white adipose tissue, in response to chemical or hormonal stimulation, environmental changes, cold exposure, and defined genetic manipulation (Langin, 2009; Lefterova and Lazar, 2009; Frontini and Cinti, 2010). The most well studied models whereby brown adipocytes appear in white fat are upon cold exposure or after stimulation of the beta(3)-adrenoceptor pathways. Cold exposure of mice results in expression of the brown adipocyte marker, UCP1, in inguinal white adipose tissue (Loncar, 1991) and in mesenteric, epididymal, retroperitoneal, inguinal, and periovarian adipose depots upon exposure to cold or to treatment with a beta-adrenoceptor agonist (Cousin et al., 1992). In agreement, beta 3-adrenoceptor knockout mice show suppressed occurrence of brown adipocytes in white fat upon cold exposure (Jimenez et al., 2003). In contrast, transgenic mice overexpressing the beta 1-adrenergic receptor in adipose tissue exhibit abundant appearance of brown fat cells in subcutaneous white adipose tissue and are resistant to obesity (Soloveva et al., 1997). Chronic treatment with the beta3-adrenoceptor agonist, CL 316,243, (Bloom et al., 1992), promotes thermogenesis, and the appearance of multilocular adipocytes in white fat while protecting from high-fat diet-induced obesity (Himms-Hagen et al., 1994). Infusion of CL 316,243 reduced abdominal fat, increased resting metabolic rate, and abundant multilocular brown adipocytes expressing uncoupling protein (UCP) appeared in retroperitoneal white fat (Ghorbani et al., 1997). Similarly, appearance of brown adipocytes in white adipose tissue during CL 316,243-treatment correlated with reversal of obesity and diabetes in Zucker fa/fa rats (Ghorbani and Himms-Hagen, 1997). Also, beta3-adrenergic receptors mediate CL 316,243 agonist-induced effects on energy expenditure, insulin secretion, and food intake (Grujic et al., 1997).
Interestingly, genetic background modulates the relative degree of browning of white adipose tissue. CL 316,243 prevented the development of diet-induced obesity in A/J animals, but not in C57BL/6J animals. In agreement, CL 316,243-treated A/J mice, but not B/6J mice, showed abundant UCP1 expression in white adipose depots (Collins et al., 1997). Also, significant stain-specific differences in UCP1 transcript levels were seen in various white fat depots derived from A/J and C57BL/6J strains of mice after stimulation of adrenergic signaling (Guerra et al., 1998). Further, cold exposure induced brown adipocytes in retroperitoneal fat of adult A/J mice but not in C57BL/6J mice. In contrast, induction of UCP1 in interscapular brown adipose tissue showed no such strain dependence (Xue et al., 2007).
Tissue plasticity which allows efficient conversion of white adipocyte to brown adipocyte and vice versa has been proposed as a potential mechanism (Frontini and Cinti, 2010). Thus, cold exposure conditions would promote white-to-brown conversion to fulfill the demand for thermogenesis, whereas, high-fat diet would promote conversion of brown-to-white fat to enable energy storage (Cinti, 2011). It is believed that multilocular fat cells that appear in white fat upon CL 316243-treatment derive from convertible unilocular adipocytes that become multilocular (Himms-Hagen et al., 2000) and that emergence of brown adipocytes in white fat upon cold exposure reflects beta(3)-adrenoceptor-mediated transdifferentiation (Barbatelli et al., 2010). The retinoblastoma (Rb) protein family acts as a molecular switch determining white versus brown adipocyte differentiation. Thus, inactivation of pRB results in the expression of UCP1 in the white adipose tissue and down-regulation of pRB expression is associated with white into brown adipocyte transdifferentiation in response to beta3-adrenergic receptor agonist treatment (Hansen et al., 2004). Further, mice lacking the Rb-family member, p107, possess white adipose tissue with multilocular adipocytes that express elevated levels of UCP1 and PGC-1α, a transcriptional co-activator implicated in mitochondrial biogenesis and adaptive thermogenesis and pRb binds and represses PGC-1α transcription (Scime et al., 2005).
Multilocular fat cells that appear in white fat upon CL 316243-treatment may derive from a precursor cell that gives rise to more typical brown adipocytes (Himms-Hagen et al., 2000). To date, such a precursor pool is unidentified. The evidence that the dermis, muscle, and brown fat all originate from the central dermomyotome (Atit et al., 2006) and that immature undifferentiated brown fat cells harbor muscle-specific transcripts and brown preadipocytes exhibit a myogenic signature (Timmons et al., 2007) stimulated a search for a common brown fat/muscle progenitor. Indeed, Seale et al. (2008, 2009) provided evidence that brown fat shares developmental ontogeny with skeletal muscle with the transcription factor PRDM16 promoting the differentiation of skeletal muscle-derived Myf5+ precursor cells toward a classical brown adipocyte lineage. However, they did not detect Myf5+ precursor cell derivatives in the examined white fat. These findings suggest that, either (i) Myf5+ precursor cells do not promote the browning phenomenon in white fat, or (ii) a context dependent role for Myf5+ precursor cells may yet exist with respect to induction of brown adipocytes in white fat, or (iii) other distinct precursor cell pools resident in white fat or other tissue/s may drive the browning phenotype in white fat. Lineage tracing methods with the availability of unique gene expression markers for the three major types of adipose tissue depots – the brown (Zic1), the beige, or brite (Hoxc9), and the white (Tcf21; Walden et al., 2011) – would help identify the potential precursor pool that drives browning of white fat.
Autocrine or paracrine factors – classic examples being insulin and leptin – play critical roles in regulating not only the cells from which they derive, but also play overarching roles in regulating the biology of other cells that orchestrate glucose homeostasis. This is possible via these factors engaging their specific receptors in the cell type where the action is elicited. Several facts support a notion that a similar such secretagogue might regulate the appearance of brown adipocytes in white fat: (i) the white fat is a reservoir that synthesizes and secretes multiple cytokines and hormones such as leptin, (ii) classical brown fat might produce factors that promote brown adipocyte induction locally as well as at a distance in white fat, and (iii) considering the muscle-specific origin of brown adipocytes, it is logical to assume that a muscle-specific factor might serve as a inducer of brown adipocytes. Indeed, a muscle-derived hormone, irisin, has been identified that promotes induction of brown adipocytes in white fat (Bostrom et al., 2012). Irisin, whose receptor is presently unidentified, is induced in the muscle by PGC-1α expression. It is induced with exercise and a rise in plasma irisin levels correlate with increased energy expenditure, enhanced glucose homeostasis, and protection from obesity. The observation that irisin has paracrine effects on white fat to stimulate induction of a brown fat program has utility in not only furthering the knowledge about browning of white fat but also has obvious therapeutic potential. It is plausible that other factors such as irisin, produced locally in white fat or acting in a paracrine manner, may stimulate the browning of white fat.
Neural circuits are also recognized as critical modifiers of the white fat to brown fat phenomenon. An enriched environment induced browning of white fat associated with expression of genes involved in brown adipogenesis, thermogenesis, and beta-adrenergic signaling pathways along with improved energy homeostasis and glucose tolerance (Cao et al., 2011). Hypothalamic overexpression of the brain-derived neurotrophic factor (BDNF) promoted a lean phenotype accompanied with acquisition of brown fat features in the white fat, whereas, inhibition of BDNF signaling reversed this phenotype (Cao et al., 2011). Also, hypothalamic neuropeptide Y (NPY) has been implicated in promoting brown fat features in white fat (Chao et al., 2011). Knockdown of NPY expression in the dorsomedial hypothalamus (DMH) reduced white fat depots, increased energy expenditure, and suppressed high-fat diet-induced obesity. Interestingly, these phenomena were accompanied by appearance of brown adipocytes in inguinal white adipose tissue (Chao et al., 2011).
Increased FOXC2 expression in adipocytes results in a lean phenotype and protects from hypertriglyceridemia and diet-induced insulin resistance and obesity primarily by increasing the beta-adrenergic–cAMP–protein kinase A (PKA) signaling pathway (Cederberg et al., 2001). PPARα stimulated the expression of the PRDM16 gene in brown adipocytes and PPARα activation in white adipocytes induced expression of brown fat markers (Hondares et al., 2011). C/EBPα and the corepressors CtBP1 and CtBP2 repress visceral white adipose genes and activate UCP1 transcription during PPARγ agonist-mediated induction of the brown phenotype in white adipocytes (Vernochet et al., 2009). Induction of UCP1 expression in white adipose tissue, but not in classic interscapular brown adipose tissue, is dependent on cyclooxygenase activity (Madsen et al., 2010). Cyclooxygenase (COX)-2, a rate-limiting enzyme in prostaglandin synthesis, is a downstream effector of beta-adrenergic signaling in white adipose tissue and is required for appearance of brown fat features in white fat. Prostaglandin induced differentiation of mesenchymal progenitors toward a brown adipocyte phenotype and overexpression of COX-2 in white fat induced brown adipogenesis in white fat, increased energy expenditure, and protected mice against high-fat diet-induced obesity (Vegiopoulos et al., 2010). Retinoic acid reduces body weight, increases body temperature and adiposity in rodent models and stimulates UCP1 expression in brown adipose tissue and skeletal muscle. Administration of all-trans retinoic acid results in reduced adiposity and adipocyte cell size with a rise in multilocular adipocytes expressing brown fat markers in white fat depots (Mercader et al., 2006). Wnt10b blocks beta3-agonist-induced brown adipose tissue differentiation and suppresses UCP1 expression through repression of PGC-1α, while promoting the appearance of unilocular lipid droplets and expression of white adipocyte genes consistent with conversion of brown fat to white fat (Kang et al., 2005). AMP-kinase (AMPK) signaling is also implicated in the brown fat to white fat conversion. Adipose-specific ablation of an AMP-kinase substrate, desnutrin/ATGL, converts brown fat to a white fat-like tissue where the mice exhibit severely impaired thermogenesis, increased expression of white fat genes and decreased brown fat genes (Ahmadian et al., 2011).
While it is clear that many signals can promote the browning of white fat, the mechanistic details are poorly understood. Further, some of the prior observations utilized cell culture models like primary mouse embryonic fibroblasts differentiated fat cells that harbor both lineages of “brown” fat cells. Also, many of the mouse studies were not performed at thermo-neutral temperature, and instead at an adapted colder temperature of 22–24°C which complicates the role of these molecules in thermogenesis and energy expenditure. Further, whether the repressing of white fat phenotype of these molecules is executed via functional regulation of adaptive thermogenesis or determination of cell fate and it remains unclear how many of these signals regulate the Myf5+ lineage brown fat.
We recently elucidated the importance of TGF-β signaling in the appearance of brown adipocytes within the white adipose tissue (Crunkhorn, 2011; Yadav et al., 2011). The TGF-β superfamily member, BMP7, has been implicated in brown adipogenesis (Tseng et al., 2008). Whether, there exists cross-talk between TGF-β and BMP signaling in mediating brown adipogenesis remains to be determined. Similarly, whether BMP signals, or signals emanating from other TGF-β superfamily members, play a role in promoting browning of white fat remains unclear. Moreover, it is also not known if there exists a region-specific importance of TGF-β signaling as it pertains to brown adipocyte development within the different white fat depots, such as the mesenteric, epididymal, retroperitoneal, inguinal, and periovarian adipose depots.
BMP proteins promote differentiation to either white adipocytes or brown adipocytes (Schulz et al., 2011). BMP7 triggers commitment of mesenchymal progenitor cells to a brown adipocyte lineage, and implantation of these cells into nude mice results in development of adipose tissue containing mostly brown adipocytes (Tseng et al., 2008). Subpopulation of adipogenic progenitors (termed ScaPCs) residing in murine brown fat, white fat, and skeletal muscle were isolated and it was shown that muscle and white fat derived Sca-1(+) cells were able to differentiate into brown-like adipocytes upon stimulation with BMP7 (Schulz et al., 2011). Also, Bmp7 knockout embryos show a marked reduction of brown fat, whereas, adenoviral-mediated expression of BMP7 in mice results in a significant increase in brown fat mass and leads to an increase in energy expenditure and a reduction in weight gain (Tseng et al., 2008). However, the role of BMP7 in promoting browning of white fat remains unclear. A more recent study identified Zfp423, a BMP-Smad signaling effector, as a transcriptional regulator of both brown and white preadipocyte differentiation (Gupta et al., 2010), although whether Zfp423 plays a role in promoting brown fat features in white fat is unknown.
The molecular signature that we obtain in white fat derived from Smad3-deficient mice and mice treated with the TGF-β neutralization antibody, 1D11, provides interesting insight into the development of brown adipocytes within the white fat milieu. Thus, white fat from Smad3−/− mice and mice treated with 1D11 antibodies, expresses a preponderance of genes that represent brown fat, mitochondrial, and skeletal muscle biology (Yadav et al., 2011). These findings are in contrast to studies where PPAR γ agonist rosiglitazone promotes norepinephrine augmentable UCP1 gene expression in a subset of white adipocyte cells, without concurrent expression of brown fat/muscle-specific markers (Petrovic et al., 2010). Thus, chronic treatment with rosiglitazone promotes the expression of brown fat genes, mitochondriogenesis, and thermogenesis capacity in a significant subset of white fat cells. However, the cells, referred to as “brite” adipocytes, are devoid of transcripts for classic brown adipocytes markers (including PRDM16) or for myocyte-associated genes. We reconcile these observations as evidence of at least two separate pathways that promote browning in the white fat. Rosiglitazone might promote a pool of brown adipocyte precursors that does not share a muscle origin, while the TGFβ1 effect could act on a pool of cells that might potentially represent a common progenitor for white, brown, and muscle cells. Smad3 cooccupies the genome with cell type specific master transcription factors, including Myod1 in myotubes, PU.1 in pro-B cells, and Oct4 in ESCs (Mullen et al., 2011). It is conceivable that in white fat cells, Smad2/3 interacts with factor/s that specify and maintain cell identity and cellular function. This would also provide plausible explanation for the increased expression of brown fat and skeletal muscle-specific genes in Smad3−/− white fat.
Discovering approaches to either prevent fat storage or promote fat dissipation will have a major clinical impact (Tseng et al., 2010). Human white adipocytes have the potential to acquire brown fat cell features (Tiraby et al., 2003). Our findings support the notion that promoting brown adipocyte like features in white fat might favorably alter energy balance and protect from obesity and diabetes (Tiraby and Langin, 2003). Further, our anti-TGF-β antibody studies strongly suggest that this strategy has translational potential in treatment of human obesity and diabetes. Similarly, the role of neuronal signaling regulators, NPY, and BDNF, have been elucidated in the promotion of browning of white fat (Cao et al., 2011; Chao et al., 2011) making these factors amenable for consideration as therapeutic targets. Importantly, the fact that simple changes in the environment promotes hypothalamic BDNF expression and leads to browning of white fat and increased energy dissipation (Cao et al., 2011) is of particular relevance with respect to behavioral modulation as a means to manage and treat obesity and diabetes. Interestingly, nicotine induces browning of white fat, along with associated histological and molecular characteristics, decreases food intake and protects from obesity (Yoshida et al., 1999) thus presenting a potential anti-obesity modality. Furthermore, evidence that prostaglandins, cyclooxygenase 2, and retinoic acid mediated pathways promote the accumulation of brown adipocyte like cells in traditional white fat depots (Madsen et al., 2010; Vegiopoulos et al., 2010; Mercader et al., 2006) argues for utility of these molecules and their downstream effectors as candidate therapeutic targets. Finally, considering its ability to induce browning of white fat and improving glucose homeostasis, the hormone irisin can now be added to list of therapeutic candidates for metabolic disease (Bostrom et al., 2012).
As elevated TGF-β levels are common in many disease conditions, there is a push to develop TGF-β antagonist therapies, including TGF-β neutralization antibodies and small molecule TGF-β receptor antagonists (Yingling et al., 2004). Indeed, such regimens are being clinically evaluated for diseases, such as cancer, fibrosis, scarring, diabetic nephropathy, where elevated TGF-β levels are implicated (Massague et al., 2000; Rane et al., 2006; Massague, 2008). Findings that TGF-β1 levels are elevated with obesity, taken together with our illustration of beneficial effects of the anti-TGFβ neutralization in mouse models of obesity and diabetes, provide a rationale to consider anti-TGF-β modalities for these diseases. The efficacy of 1D11 (α-TGF-β) has been tested in preclinical disease models (Ling et al., 2003; Nam et al., 2008) and a closely related human version of this antibody, designated Fresolimumab, is currently under evaluation in human clinical studies of pulmonary fibrosis, renal disease, and cancer. However, the TGF-β family proteins engage specific receptors in virtually every cell type (Roberts and Sporn, 1985). In addition to the canonical TGF-β/Smad signaling node, cross-talk with other signaling networks is a common feature of TGF-β signals (Derynck and Zhang, 2003) and inhibiting the TGF-β pathway at the ligand–receptor level may damage essential signaling networks that cross-talk with TGF-β.
Although we propose that modulation of TGF-β/Smad3 signaling activates a brown adipocyte like phenotype in rodent white fat, its implication in browning of human white fat is unclear at this time. Further, understanding the molecular pathways of TGF-β/Smad3 signaling as it pertains to appearance of brown adipocytes in white fat depots is essential to decipher mechanistic details. Does TGF-β/Smad3 signaling affect transdifferentiation or precursor cell dynamics as it induces browning of white fat? Which white fat depots are the primary targets, and how do the TGF-β/Smad3 signals harmonize with other molecules, hormones, and pathways that also regulate browning of white fat? Answers to these questions, while yielding greater mechanistic insight, will uncover novel targets for development of rational therapeutics to counter obesity and diabetes.
The authors declare that the research was conducted in the absence of any commercial or financial relationships that could be construed as a potential conflict of interest.
We apologize to authors whose contribution to this field of research have not been cited or have only been indirectly cited due to space limitations. Support for this work came from funds from the NIDDK, NIH intramural program.
Ahmadian, M., Abbott, M. J., Tang, T., Hudak, C. S., Kim, Y., Bruss, M., Hellerstein, M. K., Lee, H. Y., Samuel, V. T., Shulman, G. I., Wang, Y., Duncan, R. E., Kang, C., and Sul, H. S. (2011). Desnutrin/ATGL is regulated by AMPK and is required for a brown adipose phenotype. Cell Metab. 13, 739–748.
Atit, R., Sgaier, S. K., Mohamed, O. A., Taketo, M. M., Dufort, D., Joyner, A. L., Niswander, L., and Conlon, R. A. (2006). Beta-catenin activation is necessary and sufficient to specify the dorsal dermal fate in the mouse. Dev. Biol. 296, 164–176.
Barbatelli, G., Murano, I., Madsen, L., Hao, Q., Jimenez, M., Kristiansen, K., Giacobino, J. P., de Matteis, R., and Cinti, S. (2010). The emergence of cold-induced brown adipocytes in mouse white fat depots is determined predominantly by white to brown adipocyte transdifferentiation. Am. J. Physiol. Endocrinol. Metab. 298, E1244–E1253.
Bloom, J. D., Dutia, M. D., Johnson, B. D., Wissner, A., Burns, M. G., Largis, E. E., Dolan, J. A., and Claus, T. H. (1992). Disodium (R,R)-5-[2-[[2-(3-chlorophenyl)-2-hydroxyethyl]-amino] propyl]-1,3-benzodioxole-2,2-dicarboxylate (CL 316,243). A potent beta-adrenergic agonist virtually specific for beta 3 receptors. A promising antidiabetic and antiobesity agent. J. Med. Chem. 35, 3081–3084.
Bostrom, P., Wu, J., Jedrychowski, M. P., Korde, A., Ye, L., Lo, J. C., Rasbach, K. A., Bostrom, E. A., Choi, J. H., Long, J. Z., Kajimura, S., Zingaretti, M. C., Vind, B. F., Tu, H., Cinti, S., Hojlund, K., Gygi, S. P., and Spiegelman, B. M. (2012). A PGC1-alpha-dependent myokine that drives brown-fat-like development of white fat and thermogenesis. Nature 481, 463–468.
Cao, L., Choi, E. Y., Liu, X., Martin, A., Wang, C., Xu, X., and During, M. J. (2011). White to brown fat phenotypic switch induced by genetic and environmental activation of a hypothalamic-adipocyte axis. Cell Metab. 14, 324–338.
Cederberg, A., Gronning, L. M., Ahren, B., Tasken, K., Carlsson, P., and Enerback, S. (2001). FOXC2 is a winged helix gene that counteracts obesity, hypertriglyceridemia, and diet-induced insulin resistance. Cell 106, 563–573.
Chao, P. T., Yang, L., Aja, S., Moran, T. H., and Bi, S. (2011). Knockdown of NPY expression in the promotes development of brown adipocytes and prevents diet-induced obesity. Cell Metab. 13, 573–583.
Cinti, S. (2011). Between brown and white: novel aspects of adipocyte differentiation. Ann. Med. 43, 104–115.
Collins, S., Daniel, K. W., Petro, A. E., and Surwit, R. S. (1997). Strain-specific response to beta 3-adrenergic receptor agonist treatment of diet-induced obesity in mice. Endocrinology 138, 405–413.
Cousin, B., Cinti, S., Morroni, M., Raimbault, S., Ricquier, D., Penicaud, L., and Casteilla, L. (1992). Occurrence of brown adipocytes in rat white adipose tissue: molecular and morphological characterization. J. Cell. Sci. 103(Pt 4), 931–942.
Crunkhorn, S. (2011). Metabolic disease: turning ‘bad’ fat into ‘good.’ Nat. Rev. Drug Discov. 10, 659.
Cypess, A. M., Lehman, S., Williams, G., Tal, I., Rodman, D., Goldfine, A. B., Kuo, F. C., Palmer, E. L., Tseng, Y. H., Doria, A., Kolodny, G. M., and Kahn, C. R. (2009). Identification and importance of brown adipose tissue in adult humans. N. Engl. J. Med. 360, 1509–1517.
Derynck, R., and Zhang, Y. E. (2003). Smad-dependent and Smad-independent pathways in TGF-beta family signalling. Nature 425, 577–584.
Frontini, A., and Cinti, S. (2010). Distribution and development of brown adipocytes in the murine and human adipose organ. Cell Metab. 11, 253–256.
Gesta, S., Tseng, Y. H., and Kahn, C. R. (2007). Developmental origin of fat: tracking obesity to its source. Cell 131, 242–256.
Ghorbani, M., Claus, T. H., and Himms-Hagen, J. (1997). Hypertrophy of brown adipocytes in brown and white adipose tissues and reversal of diet-induced obesity in rats treated with a beta3-adrenoceptor agonist. Biochem. Pharmacol. 54, 121–131.
Ghorbani, M., and Himms-Hagen, J. (1997). Appearance of brown adipocytes in white adipose tissue during CL 316,243-induced reversal of obesity and diabetes in Zucker fa/fa rats. Int. J. Obes. Relat. Metab. Disord. 21, 465–475.
Grujic, D., Susulic, V. S., Harper, M. E., Himms-Hagen, J., Cunningham, B. A., Corkey, B. E., and Lowell, B. B. (1997). Beta3-adrenergic receptors on white and brown adipocytes mediate beta3-selective agonist-induced effects on energy expenditure, insulin secretion, and food intake. A study using transgenic and gene knockout mice. J. Biol. Chem. 272, 17686–17693.
Guerra, C., Koza, R. A., Yamashita, H., Walsh, K., and Kozak, L. P. (1998). Emergence of brown adipocytes in white fat in mice is under genetic control. Effects on body weight and adiposity. J. Clin. Invest. 102, 412–420.
Gupta, R. K., Arany, Z., Seale, P., Mepani, R. J., Ye, L., Conroe, H. M., Roby, Y. A., Kulaga, H., Reed, R. R., and Spiegelman, B. M. (2010). Transcriptional control of preadipocyte determination by Zfp423. Nature 464, 619–623.
Hansen, J. B., Jorgensen, C., Petersen, R. K., Hallenborg, P., de Matteis, R., Boye, H. A., Petrovic, N., Enerback, S., Nedergaard, J., Cinti, S., te Riele, H., and Kristiansen, K. (2004). Retinoblastoma protein functions as a molecular switch determining white versus brown adipocyte differentiation. Proc. Natl. Acad. Sci. U.S.A. 101, 4112–4117.
Himms-Hagen, J., Cui, J., Danforth, E. Jr., Taatjes, D. J., Lang, S. S., Waters, B. L., and Claus, T. H. (1994). Effect of CL-316,243, a thermogenic beta 3-agonist, on energy balance and brown and white adipose tissues in rats. Am. J. Physiol. 266, R1371–R1382.
Himms-Hagen, J., Melnyk, A., Zingaretti, M. C., Ceresi, E., Barbatelli, G., and Cinti, S. (2000). Multilocular fat cells in WAT of CL-316243-treated rats derive directly from white adipocytes. Am. J. Physiol. Cell Physiol. 279, C670–C681.
Hondares, E., Rosell, M., Diaz-Delfin, J., Olmos, Y., Monsalve, M., Iglesias, R., Villarroya, F., and Giralt, M. (2011). PPARalpha induces PGC-1alpha gene expression and contributes to the thermogenic activation of brown fat; involvement of PRDM16. J. Biol. Chem. 296, 43112–43122.
Jimenez, M., Barbatelli, G., Allevi, R., Cinti, S., Seydoux, J., Giacobino, J. P., Muzzin, P., and Preitner, F. (2003). Beta 3-adrenoceptor knockout in C57BL/6J mice depresses the occurrence of brown adipocytes in white fat. Eur. J. Biochem. 270, 699–705.
Kang, S., Bajnok, L., Longo, K. A., Petersen, R. K., Hansen, J. B., Kristiansen, K., and Macdougald, O. A. (2005). Effects of Wnt signaling on brown adipocyte differentiation and metabolism mediated by PGC-1alpha. Mol. Cell. Biol. 25, 1272–1282.
Langin, D. (2009). Recruitment of brown fat and conversion of white into brown adipocytes: strategies to fight the metabolic complications of obesity? Biochim. Biophys. Acta 1801, 372–376.
Lefterova, M. I., and Lazar, M. A. (2009). New developments in adipogenesis. Trends Endocrinol. Metab. 20, 107–114.
Ling, H., Li, X., Jha, S., Wang, W., Karetskaya, L., Pratt, B., and Ledbetter, S. (2003). Therapeutic role of TGF-beta-neutralizing antibody in mouse cyclosporine A nephropathy: morphologic improvement associated with functional preservation. J. Am. Soc. Nephrol. 14, 377–388.
Madsen, L., Pedersen, L. M., Lillefosse, H. H., Fjaere, E., Bronstad, I., Hao, Q., Petersen, R. K., Hallenborg, P., Ma, T., de Matteis, R., Araujo, P., Mercader, J., Bonet, M. L., Hansen, J. B., Cannon, B., Nedergaard, J., Wang, J., Cinti, S., Voshol, P., Doskeland, S. O., and Kristiansen, K. (2010). UCP1 induction during recruitment of brown adipocytes in white adipose tissue is dependent on cyclooxygenase activity. PLoS ONE 5, e11391. doi:10.1371/journal.pone.0011391
Massague, J., Blain, S. W., and Lo, R. S. (2000). TGFbeta signaling in growth control, cancer, and heritable disorders. Cell 103, 295–309.
Mercader, J., Ribot, J., Murano, I., Felipe, F., Cinti, S., Bonet, M. L., and Palou, A. (2006). Remodeling of white adipose tissue after retinoic acid administration in mice. Endocrinology 147, 5325–5332.
Mullen, A. C., Orlando, D. A., Newman, J. J., Loven, J., Kumar, R. M., Bilodeau, S., Reddy, J., Guenther, M. G., Dekoter, R. P., and Young, R. A. (2011). Master transcription factors determine cell-type-specific responses to TGF-beta signaling. Cell 147, 565–576.
Nam, J. S., Terabe, M., Mamura, M., Kang, M. J., Chae, H., Stuelten, C., Kohn, E., Tang, B., Sabzevari, H., Anver, M. R., Lawrence, S., Danielpour, D., Lonning, S., Berzofsky, J. A., and Wakefield, L. M. (2008). An anti-transforming growth factor beta antibody suppresses metastasis via cooperative effects on multiple cell compartments. Cancer Res. 68, 3835–3843.
Nedergaard, J., Bengtsson, T., and Cannon, B. (2007). Unexpected evidence for active brown adipose tissue in adult humans. Am. J. Physiol. Endocrinol. Metab. 293, E444–E452.
Nedergaard, J., and Cannon, B. (2010). The changed metabolic world with human brown adipose tissue: therapeutic visions. Cell Metab. 11, 268–272.
Park, K. W., Halperin, D. S., and Tontonoz, P. (2008). Before they were fat: adipocyte progenitors. Cell Metab. 8, 454–457.
Petrovic, N., Walden, T. B., Shabalina, I. G., Timmons, J. A., Cannon, B., and Nedergaard, J. (2010). Chronic peroxisome proliferator-activated receptor gamma (PPARgamma) activation of epididymally derived white adipocyte cultures reveals a population of thermogenically competent, UCP1-containing adipocytes molecularly distinct from classic brown adipocytes. J. Biol. Chem. 285, 7153–7164.
Rane, S. G., Lee, J. H., and Lin, H. M. (2006). Transforming growth factor-beta pathway: role in pancreas development and pancreatic disease. Cytokine Growth Factor Rev. 17, 107–119.
Schulz, T. J., Huang, T. L., Tran, T. T., Zhang, H., Townsend, K. L., Shadrach, J. L., Cerletti, M., Mcdougall, L. E., Giorgadze, N., Tchkonia, T., Schrier, D., Falb, D., Kirkland, J. L., Wagers, A. J., and Tseng, Y. H. (2011). Identification of inducible brown adipocyte progenitors residing in skeletal muscle and white fat. Proc. Natl. Acad. Sci. U.S.A. 108, 143–148.
Scime, A., Grenier, G., Huh, M. S., Gillespie, M. A., Bevilacqua, L., Harper, M. E., and Rudnicki, M. A. (2005). Rb and p107 regulate preadipocyte differentiation into white versus brown fat through repression of PGC-1alpha. Cell Metab. 2, 283–295.
Seale, P., Bjork, B., Yang, W., Kajimura, S., Chin, S., Kuang, S., Scime, A., Devarakonda, S., Conroe, H. M., Erdjument-Bromage, H., Tempst, P., Rudnicki, M. A., Beier, D. R., and Spiegelman, B. M. (2008). PRDM16 controls a brown fat/skeletal muscle switch. Nature 454, 961–967.
Seale, P., Kajimura, S., and Spiegelman, B. M. (2009). Transcriptional control of brown adipocyte development and physiological function – of mice and men. Genes Dev. 23, 788–797.
Soloveva, V., Graves, R. A., Rasenick, M. M., Spiegelman, B. M., and Ross, S. R. (1997). Transgenic mice overexpressing the beta 1-adrenergic receptor in adipose tissue are resistant to obesity. Mol. Endocrinol. 11, 27–38.
Timmons, J. A., Wennmalm, K., Larsson, O., Walden, T. B., Lassmann, T., Petrovic, N., Hamilton, D. L., Gimeno, R. E., Wahlestedt, C., Baar, K., Nedergaard, J., and Cannon, B. (2007). Myogenic gene expression signature establishes that brown and white adipocytes originate from distinct cell lineages. Proc. Natl. Acad. Sci. U.S.A. 104, 4401–4406.
Tiraby, C., and Langin, D. (2003). Conversion from white to brown adipocytes: a strategy for the control of fat mass? Trends Endocrinol. Metab. 14, 439–441.
Tiraby, C., Tavernier, G., Lefort, C., Larrouy, D., Bouillaud, F., Ricquier, D., and Langin, D. (2003). Acquirement of brown fat cell features by human white adipocytes. J. Biol. Chem. 278, 33370–33376.
Tseng, Y. H., Cypess, A. M., and Kahn, C. R. (2010). Cellular bioenergetics as a target for obesity therapy. Nat. Rev. Drug Discov. 9, 465–482.
Tseng, Y. H., Kokkotou, E., Schulz, T. J., Huang, T. L., Winnay, J. N., Taniguchi, C. M., Tran, T. T., Suzuki, R., Espinoza, D. O., Yamamoto, Y., Ahrens, M. J., Dudley, A. T., Norris, A. W., Kulkarni, R. N., and Kahn, C. R. (2008). New role of bone morphogenetic protein 7 in brown adipogenesis and energy expenditure. Nature 454, 1000–1004.
van Marken Lichtenbelt, W. D., Vanhommerig, J. W., Smulders, N. M., Drossaerts, J. M., Kemerink, G. J., Bouvy, N. D., Schrauwen, P., and Teule, G. J. (2009). Cold-activated brown adipose tissue in healthy men. N. Engl. J. Med. 360, 1500–1508.
Vegiopoulos, A., Muller-Decker, K., Strzoda, D., Schmitt, I., Chichelnitskiy, E., Ostertag, A., Berriel Diaz, M., Rozman, J., Hrabe de Angelis, M., Nusing, R. M., Meyer, C. W., Wahli, W., Klingenspor, M., and Herzig, S. (2010). Cyclooxygenase-2 controls energy homeostasis in mice by de novo recruitment of brown adipocytes. Science 328, 1158–1161.
Vernochet, C., Peres, S. B., Davis, K. E., Mcdonald, M. E., Qiang, L., Wang, H., Scherer, P. E., and Farmer, S. R. (2009). C/EBPalpha and the corepressors CtBP1 and CtBP2 regulate repression of select visceral white adipose genes during induction of the brown phenotype in white adipocytes by peroxisome proliferator-activated receptor gamma agonists. Mol. Cell. Biol. 29, 4714–4728.
Virtanen, K. A., Lidell, M. E., Orava, J., Heglind, M., Westergren, R., Niemi, T., Taittonen, M., Laine, J., Savisto, N. J., Enerback, S., and Nuutila, P. (2009). Functional brown adipose tissue in healthy adults. N. Engl. J. Med. 360, 1518–1525.
Walden, T. B., Hansen, I. R., Timmons, J. A., Cannon, B., and Nedergaard, J. (2011). Recruited versus non-recruited molecular signatures of brown, “brite” and white adipose tissues. Am. J. Physiol. Endocrinol. Metab. 302, E19–E31.
Xue, B., Rim, J. S., Hogan, J. C., Coulter, A. A., Koza, R. A., and Kozak, L. P. (2007). Genetic variability affects the development of brown adipocytes in white fat but not in interscapular brown fat. J. Lipid Res. 48, 41–51.
Yadav, H., Quijano, C., Kamaraju, A. K., Gavrilova, O., Malek, R., Chen, W., Zerfas, P., Zhigang, D., Wright, E. C., Stuelten, C., Sun, P., Lonning, S., Skarulis, M., Sumner, A. E., Finkel, T., and Rane, S. G. (2011). Protection from obesity and diabetes by blockade of TGF-beta/Smad3 signaling. Cell Metab. 14, 67–79.
Yingling, J. M., Blanchard, K. L., and Sawyer, J. S. (2004). Development of TGF-beta signalling inhibitors for cancer therapy. Nat. Rev. Drug Discov. 3, 1011–1022.
Keywords: TGF-beta, Smad3, obesity, brown fat, white fat
Citation: Yadav H and Rane SG (2012) TGF-β/Smad3 signaling regulates brown adipocyte induction in white adipose tissue. Front. Endocrin. 3:35. doi: 10.3389/fendo.2012.00035
Received: 17 November 2011; Accepted: 13 February 2012;
Published online: 14 March 2012.
Edited by:
Patrick Seale, University of Pennsylvania, USAReviewed by:
Maria Christina Werneck Avellar, Universidade Federal de São Paulo, BrazilCopyright: © 2012 Yadav and Rane. This is an open-access article distributed under the terms of the Creative Commons Attribution Non Commercial License, which permits non-commercial use, distribution, and reproduction in other forums, provided the original authors and source are credited.
*Correspondence: Sushil G Rane, Diabetes, Endocrinology and Obesity Branch, National Institute of Diabetes and Digestive and Kidney Diseases, National Institutes of Health, Bethesda, MD 20892, USA. e-mail:cmFuZXNAbWFpbC5uaWguZ292
Disclaimer: All claims expressed in this article are solely those of the authors and do not necessarily represent those of their affiliated organizations, or those of the publisher, the editors and the reviewers. Any product that may be evaluated in this article or claim that may be made by its manufacturer is not guaranteed or endorsed by the publisher.
Research integrity at Frontiers
Learn more about the work of our research integrity team to safeguard the quality of each article we publish.