- 1 Department of Cell Biology, Physiology and Immunology, University of Cordoba, Cordoba, Spain
- 2 Instituto Maimónides de Investigación Biomédica, Biomedical Research Center in Red Physiopathology of Obesity and Nutrition, Cordoba, Spain
The secretory pathway is a process characteristic of cells specialized in secretion such as endocrine cells and neurons. It consists of different stages that are dependent on specific transport of proteins in vesicular-tubular carriers. Biochemical analyses have unveiled a number of protein families that confer identity to carrier vesicles and specificity to their transport. Among them is the family of Rab proteins, Ras-like small GTPases that anchor to the surface of transport vesicles and participate in vesicle formation from the donor compartment, transport along cytoskeletal tracks, and docking and fusion with the acceptor compartment. All of these functions are accomplished through the recruitment of effector proteins, such as sorting adaptors, tethering factors, kinases, phosphatases, and motors. The numerous Rab proteins have distinct subcellular distributions throughout the endomembrane system, which ensures efficient cargo transfer. Rab proteins act as molecular switches that alternate between a cytosolic GDP-bound, inactive form and a membrane-associated GTP-bound, active conformation. Cycling between inactive and active states is a highly regulated process that enables Rabs to confer spatio-temporal precision to the different stages through which a vesicle passes during its lifespan. This review focuses on our current knowledge on Rab functioning, from their structural features to the multiple regulatory proteins and effectors that control Rab activity and translate Rab function. Furthermore, we also summarize the information available on a particular Rab protein, Rab18, which has been linked to the control of secretory granule traffic in neuroendocrine cells.
The secretory pathway is an intricate, multi-step process that comprises the sequential participation of numerous subcellular mechanisms, such as synthesis, modification, sorting, and delivery of proteins that are secreted to the extracellular milieu or that are components of a variety of intracellular membrane compartments and the cell surface (Bonifacino and Glick, 2004). Proteins transported along the secretory pathway are synthesized by ribosomes associated to the ER-membrane and are translocated into the lumen of this organelle. Then, proteins are packed into vesicles and transferred to the Golgi complex passing through the ER/Golgi intermediate compartment (i.e., ERGIC). At the more distal region of this organelle, the trans-Golgi network, proteins are sorted into different types of vesicles according to their final destinations (Gerdes, 2008). Some proteins traffic to the constitutive secretory pathway, which is common to all cell types and responsible for the continuous delivery of protein and lipid components to the plasma membrane and extracellular matrix. The regulated secretory pathway is a landmark of specialized secretory cells, such as endocrine cells and neurons, that form and store secretory vesicles containing specific secretory cargoes to be exocytosed in a regulated manner upon cell surface receptor stimulation (Park and Loh, 2008). The trans-Golgi network is also the intracellular compartment wherein the secretory and endocytic pathways converge. In the latter pathway, molecules are internalized from the cell surface in endocytic vesicles and transported to early endosomes, wherein they undergo extensive sorting. From there, endocytosed molecules can then be recycled to the plasma membrane, transported to the trans-Golgi network, or addressed to lysosomes via late endosomes for degradation (Seaman, 2008).
Shuttle vesicles mediate most of the transfer of proteins between the different compartments of the secretory pathway. This process comprises several sequential steps: first of all, coat complexes are responsible for both, the formation of transport vesicles, which occurs by budding and fission from the donor compartment, and the specific incorporation of cargo into the newly formed vesicles (Brett and Traub, 2006). After uncoating, vesicles move to the acceptor compartment, normally by means of their association with motor proteins that interact with and move along cytoskeletal tracks (Brett and Traub, 2006). In the proximity of the target compartment, vesicles are transiently linked to the acceptor membrane by a multifactorial complex in a process referred to as tethering. Finally, vesicles dock and fuse with the acceptor membrane allowing cargo unload to the acceptor compartment. A plethora of proteins that ensure the specificity and efficiency of cargo selection, vesicle targeting, and fusion, control tightly each one of these stages. These proteins include tethering factors, v-SNARE and t-SNARE complexes, and Rab GTPase proteins (Figure 1; Bonifacino and Glick, 2004; Jahn and Scheller, 2006; Takamori et al., 2006). This review focuses on the latter group of proteins. First, it provides a general picture on the common molecular characteristics and mechanisms of action of Rab GTPases in relation to the secretory pathway. Then, it discusses the information available on a particular member of this family of proteins that regulates secretory granule traffic in neuroendocrine cells, Rab18 (Vazquez-Martinez et al., 2007).
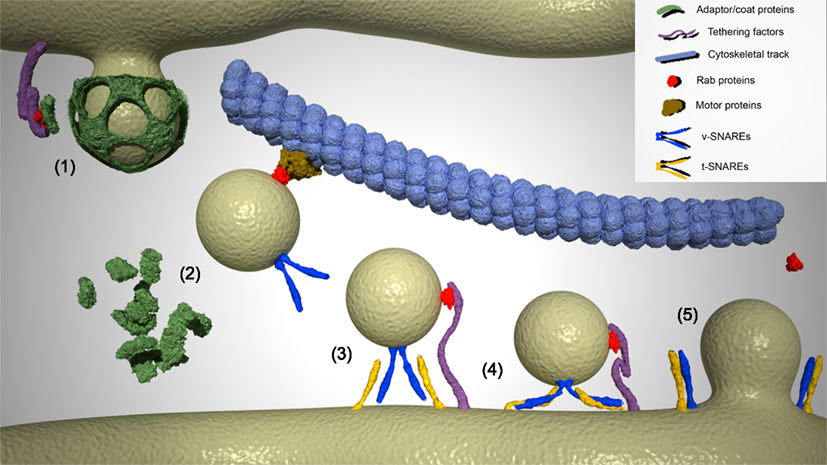
Figure 1. Different stages of vesicle budding from the donor compartment and fusion with the acceptor compartment. (1) Vesicle formation and initiation of coat and adaptor proteins assembly. Specific Rab proteins interact with tethering factors anchored to the donor membrane to form multi-subunit Rab tethers that address coat proteins such as COPI, COPII and clathrin, and adaptor proteins that determine the specificity of cargo to the surface of the budding vesicle. (2) Uncoating and specific transport of carrier vesicles along cytoskeletal tracks. Newly formed vesicles lose their coat by inactivation of particular Rab GTPases and activation of uncoating enzymes. Motor protein complexes recognize and recruit uncoated vesicles to cytoskeletal tracks to transport the cargo to the corresponding acceptor compartment. These motor protein complexes include Rab proteins that modulate the processivity and direction of the motor protein movement. During transport, specific v-SNARE complexes are added to the surface of vesicles, thus conferring specificity to their fusion with the corresponding target compartment. (3) Tethering of vesicles to the acceptor compartment. Vesicles in the proximity of the target compartment become tethered to its membrane in a process driven by a Rab protein/tether factor complex. (4) Docking of vesicles to the acceptor compartment. v-SNARE and t-SNAREs assemble into a four-helix bundle and vesicles are approached to the target membrane. (5) Membrane fusion and release of cargo into the target compartment. Trans-SNARE complexes promote fusion of the vesicle and acceptor lipid bilayers. Cargo is transferred to the acceptor compartment, and the SNAREs are recycled. Rab proteins are inactivated and released into the cytosol, wherein they remain until a new cycle of activation is initiated.
The Rab GTPase Family
Monomeric Rab proteins, members of the Ras superfamily of small GTPases, control multitude of vital cellular processes including protein exocytosis and endocytosis, intracellular signaling, differentiation, and development. These general functions are accomplished via regulation of intracellular vesicle dynamics, from their formation to their fusion with the correct target membrane. Rab proteins control vesicle genesis from donor compartments by forming part of the so-called multi-subunit Rab tethers, which are regulatory complexes that interact with coat proteins, such as COPI, COPII, and clathrin, influencing their recruitment to vesicle budding zones and, ultimately, defining the newly formed vesicle identity (Angers and Merz, 2010). Rab proteins also determine the specificity of carrier-vesicle addressing and transport to acceptor compartments by interacting directly or indirectly with molecular motors, recruiting them to the surface of transport vesicles and even acting as modulators of the motor processivity along cytoskeletal tracks (Hammer III and Wu, 2002; Echard et al., 1998; Jordens et al., 2001; Kuroda and Fukuda, 2005). Finally, Rab GTPases also participate in vesicle tethering, docking, and fusion events. They associate with v-SNARE and/or t-SNARE proteins forming trans-SNARE complexes, thus organizing fusion-competent microdomains in the acceptor membrane (Wickner and Schekman, 2008). Figure 1 illustrates the involvement of Rab proteins in the different steps of the life cycle of carrier vesicles from vesicle formation to fusion.
Besides direct functions in vesicle dynamics, Rab proteins also serve as intermediates in the integration of extracellular signals into the molecular machinery accountable for intracellular membrane traffic (Miaczynska et al., 2004). They act as regulated molecular switches, cycling between an inactive, GDP-bound cytosolic state and an active, GTP-bound membrane associate state (Pfeffer and Aivazian, 2004; Seabra and Wasmeier, 2004; Pfeffer, 2005). Thus, the control of this activity cycle, which is dependent on a wide spectrum of signal transduction-related proteins, is key to conferring spatial and temporal coordination to the cellular response. Altogether, this array of functions has led to the widely accepted view that Rab proteins are core constituents needed for orchestrating the intracellular organization of endomembrane systems. Consequently, dysfunctions affecting synthesis and/or activity of these proteins entail the appearance of an important number of severe human diseases (Zerial and McBride, 2001).
A number of genome analyses have revealed that in the yeast Saccharomyces cerevisiae Rab family is composed of 11 members, but that in humans there are almost 70 different Rab proteins (Zerial and McBride, 2001; Colicelli, 2004). The fact that there exist almost seven times more members in mammals than in yeasts has been attributed to the necessity of fulfilling the specificity demands of an endomembrane-trafficking system highly increased in complexity during evolution (Dacks and Field, 2004, 2007). Thus, different Rab GTPases localize to distinct subcompartments and, although in some instances several Rabs may associate to the same organelle, they always segregate in specific spatio-temporal microdomains (Sonnichsen et al., 2000). The complexity of the Rab protein system increases enormously when considering their mode of action. Rab GTPases always act in conjunction with an ample range of different proteins that either regulate Rab activity or are regulated by Rab activation. Proteins comprising the latter group, the so-called Rab effector proteins, are the ultimate responsible for translating Rab function into the molecular machinery that drives intracellular vesicle positioning (Stenmark, 2009).
Structural and Functional Features of Rab Proteins
To date, crystal structures are available for at least 16 Rab GTPases in the GTP-bound conformation and 17 in the GDP-bound one, which has allowed some generalization about the structural organization of Rab proteins (Eathiraj et al., 2005; Lee et al., 2009). The tertiary structure of this group of proteins is very similar to the structural folding of other GTPases of the Ras family. It consists of a central barrel composed of six β-sheets surrounded by five α-helices. In α1 resides a region broadly conserved among all GTPases (Ostermeier and Brunger, 1999), which includes the GTP-binding platform and the element for binding Mg2+, a cofactor required for high-affinity nucleotide binding and hydrolysis (Vetter and Wittinghofer, 2001). Further structural analysis of Rab proteins has defined two regions, termed switches I and II, which are located between α1/β2 loop and β3/β4 loop, respectively. These regions are critical for conferring functional specificity to Rabs, as they contact the γ-phosphate of GTP and, together with the interswitch region, exhibit large conformational differences between the inactive and active states (Dumas et al., 1999; Stroupe and Brunger, 2000). Similarities and dissimilarities in the tertiary structure adopted by these regions have led to classification of the Rab family into eight phylogenetic groups composed of clusters of related Rabs with similar active conformations (Pereira-Leal and Seabra, 2001). For example, in Rab5 and Rab22, which both belong to subfamily V, switches I and II adopt nearly identical active conformations (Eathiraj et al., 2005).
The region exhibiting highest evolutionary divergence in terms of amino acid sequence of Rab proteins constitutes the C-terminal domain, the so-called hypervariable domain. Hypervariable domains have been proposed to be responsible for Rab protein localization (Chavrier et al., 1991). This was first supported by subcellular fractionation studies using a hybrid protein consisting of 166 N-terminal residues of Rab2, which localizes to the ERGIC, fused with either the 35 C-terminal amino acids of Rab5, a protein that targets the early endosomal compartment, or the 35 C-terminal amino acids of Rab7, which specifically binds to late endosomes. These substitutions were sufficient to address Rab2/Rab5 and Rab2/Rab7 chimera proteins to the early or late endosomal compartments, respectively (Chavrier et al., 1991). However, subsequent studies suggested that the membrane targeting of Rab proteins is more complex, involving non-hypervariable domain determinants as well (Stenmark et al., 1994; Ali et al., 2004). The particular signatures of the Rab C-terminal domain comprise CC, CXC (i.e., Rab-CXC proteins), or CAAX motifs (i.e., Rab-CAAX proteins), where C is cysteine, A is usually an aliphatic residue, and X is any amino acid. Rab-CXC proteins, the most abundant group, undergo posttranslational modification by covalent addition of two geranylgeranyl (20-carbon) isoprenoids onto the C-terminal cysteines, which is necessary for these Rabs to associate with cellular membranes to carry out intracellular functions (Lane and Beese, 2006; Leung et al., 2006). This enzymatic reaction is catalyzed by geranylgeranyltransferase type II (i.e., RGGT; also known as RabRGGT) but only when Rab proteins are in complex with an accessory protein known as Rab escort protein (i.e., REP; Farnsworth et al., 1994). Two possible mechanisms for Rab prenylation have been proposed. The first suggests that newly translated Rabs bind REP and, then, the complex is recognized by RGGT. After addition of the geranylgeranyl moieties to the cysteine residues, RGGT dissociates from REP, which remains bound to the Rab protein and delivers it to target membranes to be, finally, released into the cytosol for a new prenylation cycle. In the alternative hypothesis, RGGT and REP would already form a complex prior to binding Rab proteins. After prenylation, RGGT dissociates from REP/Rab, and REP escorts the prenylated Rab to membranes (Andres et al., 1993; Alexandrov et al., 1994; Thoma et al., 2001a,b). In the case of Rab-CAAX proteins, which are the less represented group of Rab proteins (e.g., Rab8, Rab13, Rab18, Rab23, and Rab38), after protein translation only one geranylgeranyl group is added to the C-terminal domain by RGGT in the presence of REP. Then, mono-geranylgeranylated Rabs need further processing to be re-directed to their target membranes, which involves addition of a carboxyl–methyl group in the prenylated cysteine by sequential action of two methyltransferases located in the ER-membrane (Leung et al., 2007). Although di-geranylgeranylated Rab-CXC proteins also require methylation for correct membrane targeting, only one methyltransferase is involved in catalyzing this reaction (Leung et al., 2007).
As mentioned earlier, activation of Rab GTPases by exchange of GDP for GTP entails major conformational changes in the tertiary structure of the protein that define the specificity of Rabs. This reaction is catalyzed by guanine nucleotide exchange factors (i.e., GEFs), which weaken GDP interaction with the switch regions and facilitate GDP release and the subsequent GTP binding (Vetter and Wittinghofer, 2001). The large number of GTPases requires a multitude of GEFs to ensure signaling specificity. GEF proteins characterized so far share distinctive hallmarks in their sequences, such as Vsp9- Sec2- or Mss4-like domains (Yu and Schreiber, 1995; Delprato et al., 2004; Mizuno-Yamasaki et al., 2010). Regarding the mechanisms of action whereby GEFs facilitate GDP/GTP exchange, although different proteins use diverse strategies, in all cases, GEF interaction with the Rab protein perturbs the molecular conformation of switches I and II. This sterically occludes the Mg2+-binding site essential for the high-affinity binding of the GDP. The high GTP/GDP ratio present in the cytosol allows rapid binding of GTP and the subsequent activation and translocation of the Rab protein to the corresponding target compartment. Activity of GEF proteins is strictly regulated by protein–protein and protein–lipid interactions, binding of second messengers and posttranslational modifications, which are thus key control points of the Rab-driven spatio-temporal coordination of endomembrane transport. Among a long list of Rab GEFs, we can cite as examples Mon1 that has been shown to act as GEF of Rab7 and its yeast homolog Ypt7 after activation by Ccz1 (Nordmann et al., 2010), TRAPP-I that promotes activation of Ypt1p in yeast and Rab1 in mammalian cells at the surface of ER-Golgi transport vesicles (Sacher et al., 2008), Sec2p that acts as sequential GEF for the yeast Rabs Ypt32p and Sec4p in the early stages of the secretory pathway (Mizuno-Yamasaki et al., 2010), and Rabex-5 that activates Rab5 in early endosomes, which is necessary for membrane fusion (Delprato et al., 2004).
Although Rab proteins are considered GTPases, their capacity to hydrolyze GTP is in fact very low and, thereby, they require help from a group of proteins referred to as GTPase-activating proteins (i.e., GAPs) to accelerate and to make this process efficient. GAPs belong to a very heterogeneous protein family whose members only share a common TBC1 domain, although the Rab substrate specificity of GAPs does not appear to reside in this sequence (Barr and Lambright, 2010). Rather, GAPs have been proposed to bind particular Rabs either due to their specific location or to spatial-dependent interactions with regulatory proteins. For example, TBC1D20 is an ER-membrane protein and, consequently, it is only able to target Rab proteins that share this location (Haas et al., 2007). On the other hand, Gyp7p only acts on the yeast Rab Ypt7p in concert with a protein kinase (Brett et al., 2008). As GEFs, GAPs are also highly regulated proteins. Their activity is also tightly controlled by interaction with many regulatory proteins and lipids, by second messengers and by posttranslational modifications, which highlights the importance of these proteins in the coordination of the Rab-driven cellular responses (Barr and Lambright, 2010).
Finally, other proteins also play important roles in modulating Rab activity, the so-called Rab GDP dissociation inhibitor (i.e., GDI) and GDI displacement factor (i.e., GDF). Whereas GDI prevents GDP release, thereby stabilizing the Rab protein in its inactive form (Pfeffer and Aivazian, 2004), GDF recognizes specific Rab–GDI complexes and promote GDI release and the subsequent binding of a GEF to the Rab protein (Pfeffer and Aivazian, 2004). Regarding GDI, there exist two isoforms in human cells: GDIα, whose expression is restricted to brain tissue, and the ubiquitously expressed GDIβ (Alory and Balch, 2001). RabGDI and REP molecules have regions of high sequence homology, and partially shared properties: they both bind inactive Rabs but, whereas RabGDI show very high-affinity for prenylated, GDP-bound Rabs, REP only interacts with unprenylated Rabs (Goody et al., 2005). On the other hand, the first GDF identified was the yeast protein Yip1, which interacts with the Rab proteins Ypt1 and Ypt31 (Yang et al., 1998). In later studies, other members of the Yip protein family have been discovered. All of them are transmembrane proteins that present the C- and N-terminal domains facing the cytosol (Calero and Collins, 2002; Calero et al., 2002). A gene database survey has identified 16 putative Yips in humans, which supports the fact that each Yip protein can interact with numerous Rab proteins (Pfeffer and Aivazian, 2004).
Protein Structure, Subcellular Localization and Function of Rab18
Rab18 was initially cloned from mouse pituitary AtT20 cells in the context of a PCR-based strategy aimed at cloning members of the Rab family from rodent pituitary (Yu et al., 1993). The cDNA sequence isolated coded for a 23.5-kDa protein with a consensus GTP-binding domain and sequence motifs that are characteristic of the Rab family of proteins (Yu et al., 1993). Northern blot analysis showed that Rab18 is ubiquitously expressed, but expression is higher in neuroendocrine tissues, including brain and pituitary (Yu et al., 1993). In 2005, Kukimoto-Niino and coworkers released the first X-ray diffraction crystal of human Rab18 (DOI: 10.2210/pdb1x3s/pdb), which confirmed the high structural homology of Rab18 with other members of the Rab family.
The hypervariable C-terminal domain of Rab18 contains a CAAX amino acid motif, which supports that this GTPase is monoprenylated by RGGT in the presence of a REP (likely REP-1). It has been shown that, after prenylation, Rab18 is post-translationally modified by incorporation of carboxyl–methyl groups in its C-terminal domain, a reaction that takes place in specialized subdomains of the ER-membrane enriched in the methyltransferases Icmt and Rce1 (Leung et al., 2007). After methylation, Rab18 is released into the cytosol and it can begin cycling between its inactive, cytosolic GDP-bound conformation and its active membrane-associated, GTP-bound form. By analogy with data from other members of the Rab family, it seems conceivable that this GDP-to-GTP cycle could also be regulated in the case of Rab18 by specific GEFs, GTPase activator proteins (i.e., GAPs), guanine dissociation inhibitors (i.e., GDIs) and GDI displacement factors (i.e., GDFs), although none of these proteins have been identified so far.
Regarding the intracellular localization of Rab18, pioneering confocal and electron microscopy studies by Lutcke et al. (1994) in kidney and intestinal epithelial cells showed that Rab18 immunolabeling is predominantly localized to vesicular-tubular structures below the apical brush border of proximal tubule cells. Given that these structures closely resemble the dense apical tubules implicated in membrane recycling to the apical surface and in transcytosis, the authors suggested that Rab18 might play a role in endosome recycling. However, a subsequent study aimed at elucidating how Salmonella escapes transport to lysosomes during endocytosis in macrophages showed that the bacteria bypasses the endocytic route by accumulating in a specialized compartment enriched in Rab18 but lacking early or late endosomal markers, such as transferrin and Rab7, respectively (Hashim et al., 2000). Later studies carried out in adipocytes, fibroblasts and neuroendocrine cells have also failed to localize Rab18 to early or late endosomes (Martin et al., 2005; Ozeki et al., 2005; Vazquez-Martinez et al., 2007; Dejgaard et al., 2008). Instead, Rab18 immunoreactivity colocalized with specific markers of lipid droplets in 3T3-L1 adipocytes (Martin et al., 2005; Ozeki et al., 2005), secretory granules in PC12, AtT20, and melanotrope cells (Vazquez-Martinez et al., 2007) and cis-Golgi and ER compartments in NRK, Vero, and COS7 fibroblasts (Dejgaard et al., 2008). Together, these results indicate that the subcellular distribution of Rab18 depends strongly on the cell type considered and this, in turn, suggests that this GTPase may subserve distinct, specific roles depending on cell specialization and its interaction with different effectors.
In relation to the participation of Rab18 in the secretory pathway, a differential display analysis of genes expressed in pituitary melanotropes enabled us to isolate the RAB18 gene by virtue of its preferential expression in a subset of cells exhibiting low hormone secretory activity (Malagon et al., 2005). Further subcellular localization studies of Rab18 in (neuro)endocrine cells lines, such as the rat pheochromocytoma PC12 cell line, the adrenocorticotropin (ACTH)-secreting AtT20 cell line, and α-melanotropin stimulating hormone-producing melanotrope cells showed that this GTPase mainly distributed diffuse in the cytosol under non-stimulated conditions, whereas after specific stimulation of the cell secretory activity it colocalized with the secretory granule markers secretogranin II, chromogranin A, and NPY. However, Rab18 did not colocalize with another member of the Rab family known to associate with secretory vesicles in their docking stage, Rab3A (Fischer von Mollard et al., 1991; Holz et al., 1994; Johannes et al., 1994, 1998; Regazzi et al., 1996; Martelli et al., 2000; Schluter et al., 2004). These findings suggested that in neuroendocrine cells Rab18 cycles between the cytosol and the surface of a discrete population of secretory granules upon activation of the regulated secretory pathway (Vazquez-Martinez et al., 2007). This was confirmed by investigating the intracellular distribution of Rab18 mutants. We engineered two Rab18 mutants by substitution of specific amino acid residues in the GTP-binding platform and tagged them with GFP: one, Rab18(S22N), is deficient in GTP binding and, therefore, constitutively inactive, and the second, Rab18(Q67L), is unable to hydrolyze GTP even with help from GAPs and, therefore, constitutively active. Transfection experiments carried out in PC12 and AtT20 cells demonstrated that whereas the constitutively active mutant Rab18(Q67L) was always found in association with secretory granules under basal and stimulated conditions, the constitutively inactive mutant Rab18(S22N) remained cytosolic even after stimulation of hormone secretion (Vazquez-Martinez et al., 2007). In sum, as for other Rab proteins, replacement of GDP by GTP is also required for Rab18 to be translocated to its target compartment.
An important functional insight into the specific role of Rab18 in (neuro)endocrine cells came from the observation that Rab18 translocation to secretory granules was accompanied by a reduction in the secretory response of cells to stimulatory challenges. Specifically, when wild-type Rab18 protein or the constitutively active mutant was overexpressed in PC12 cells, secretion in response to a depolarizing pulse of K+ was significantly lower than in control cells (Vazquez-Martinez et al., 2007). Thus, HPLC quantification of the amount of dopamine released into the medium revealed that Rab18-overexpressing PC12 cells secreted less dopamine than non-transfected cells or cells transfected with a control plasmid. This inhibitory effect was also observed at single-cell level by using a fluorescent styryl dye (i.e., FM5-95) that is endocytosed by the cells after each exocytotic event, the amount of internalized fluorophore being proportional to the number of secretory granules fused during the exocytotic event (Cochilla et al., 1999; Brumback et al., 2004). In the case of ACTH-secreting AtT20 cells, the inhibitory effect of Rab18 overexpression on the secretory rate was also observed in cells treated with the hypothalamic secretagog CRH at the population level, quantifying ACTH release by ELISA, and at single-cell level, using FM5-95 (Vazquez-Martinez et al., 2007). Altogether, these findings were consistent with the idea that interaction of Rab18 with secretory granules may function as a brake of granule traffic to control the amount of product released upon reception of a stimulatory input. Indeed, time-lapse video microscopy experiments supported this view, as they demonstrated that the motility of vesicles bearing Rab18 is reduced as compared with the averaged movement of the granule population. Thus, in cells overexpressing the secretory neuropeptide NPY fused to GFP, the majority of NPY-containing secretory granules displayed long and directed trajectories, whereas in Rab18-overexpressing cells, most granules bearing the GTPase exhibited short and confined trajectories (Vazquez-Martinez et al., 2007). This suggests that the presence of Rab18 in the surface of secretory granule impairs secretory granule transport. Rab18 could accomplish this function by modulating the activity of motor proteins either by direct interaction or indirectly by forming protein complexes with yet unknown effectors that would ultimately determine the processivity of motor proteins. In support of this, other members of the Rab family of GTPases have been reported to affect the secretory pathway in such a way. For example, Rab6 has been shown to associate with the microtubule motor Rabkinesin-6, thus promoting the delivery of vesicles from the Golgi to the ER (Echard et al., 1998), Rab4A interacts with dynein light chain and modulates its activity (Bielli et al., 2001), and Rab27A binds to the effector protein melanophilin, which links this GTPase to the actin motor myosin-Va and promotes melanosome secretion (Fukuda et al., 2002b).
To date, other Rab proteins, including Rab27A, Rab3A, and Rab11, have been reported to modulate Ca2+-triggered exocytosis in neuroendocrine cells by interacting directly with secretory granules. Specifically, Rab27A promotes stimulated secretion in PC12 cells (Fukuda et al., 2002a), whereas Rab3A (Fischer von Mollard et al., 1991; Johannes et al., 1994, 1998) and Rab11 (Khvotchev et al., 2003) have been shown to negatively regulate secretion in neurons. Rab3A has also been reported to decrease secretory granule release in pancreatic β-cells (Regazzi et al., 1996) and adrenal chromaffin cells (Holz et al., 1994). However, although Rab3A and Rab18 are coexpressed in (neuro)endocrine cells and appear to display similar functions restraining secretory granule release, as mentioned above, they do not coexist on the surface of the same secretory granule population, which supports the view that these two GTPases act at different steps of the secretory process. Rab3A has been found to be associated with secretory granules that are in close apposition to the plasma membrane, suggesting a role for this GTPase in the final steps of granule exocytosis (i.e., docking and fusion; Martelli et al., 2000; Schluter et al., 2004). Yet, ultrastructural analysis of the secretory granules immunolabeled with Rab18 revealed that they are located at a distance from the plasma membrane that is unlikely to correspond to secretory granules in the docking stage, which suggests that Rab18 acts at earlier steps of the secretory process (Vazquez-Martinez et al., 2007). However, to date the molecular mechanism whereby Rab18 negatively modulates secretory granule transport and, therefore, granule release remains to be elucidated. Future studies aimed at characterizing the Rab18 effector proteins and their relationship to the vesicle transport machinery will be necessary in order to obtain insight into the function of Rab18 in relation to the regulated secretory pathway.
Rabs in Human Disease
Alterations in Rab proteins and their associated proteins or effectors are being increasingly implicated in human disease. Griscelli syndrome type 1 was the first human disease discovered to be directly related to a Rab protein, namely a loss-of-function mutation in a Rab protein, Rab27A (Menasche et al., 2000). This disease is a rare, autosomal recessive disorder that results in pigmentary dilution of the skin and the hair, due to the accumulation of melanosomes in melanocytes, and different degrees of immunodeficiency, due to uncontrolled T-cell and macrophage activation (Menasche et al., 2000). Another type of Griscelli syndrome, GS type 2, is due to a mutation in the Rab27A effector and motor protein myosin-Va. These patients exhibit primary neurological defects but, unlike patients with GS type 1, normal immune function (Menasche et al., 2000). Charcot–Marie–Tooth type 2B neuropathy represents a further Rab-related human disease. This is an autosomal dominant axonal disease characterized by sensory loss, distal muscle weakness and frequent infections in feet and toes (Young and Suter, 2003). Four different mutations in RAB7 gene have been identified as causative of this disease. All of them give rise to constitutively active mutants unable to hydrolyze GTP that have been proposed to alter the retrograde axonal transport and, in particular, neurotrophin trafficking and signaling (Cogli et al., 2009).
Mutations in Rab regulatory proteins also affect the activity of Rabs and, as a consequence, have profound effects on the cell functioning. For instance, loss-of-function mutations in REP-1 that lead to choroideremia, an X-linked form of retinal degeneration characterized by an excess of unprenylated Rab27A in retinal pigment epithelial cells (Seabra, 1996). Furthermore, a mutation in RabGDIa has been related to X-linked non-specific mental retardation (D’Adamo et al., 1998). This mutant leads to a reduced Rab3A recycling in synapses and, therefore, impaired neurotransmitter release that profoundly affects development of the central nervous system (D’Adamo et al., 1998). RabGGT has also been linked to human disease. A mutation in the α-subunit has been proposed to cause Hermansky–Pudlak syndrome, which is a rare autosomal recessive disorder characterized by albinism, bleeding, and lysosomal lipofuscin accumulation (Huizing et al., 2000).
Altered expression of a number of Rab proteins is also associated with different types of cancer. For example, expression of Rab3 is elevated in cancers of the nervous system and neuroendocrine cells (Culine et al., 1992), as well as insulinomas (Lankat-Buttgereit et al., 1994), Rab5 expression appears to be upregulated in relation to the degree of malignancy and metastasis of human lung adenocarcinoma (Li et al., 1999), while Rab20 levels in pancreatic carcinoma samples are abnormally enhanced (Amillet et al., 2006), etc. Rab25 is perhaps the Rab family member best known in terms of its involvement in cancer. Extreme levels of Rab25 transcripts have been found in diverse epithelial cancers, such as ovarian and breast cancers (Cheng et al., 2004; Agarwal et al., 2009). In addition, Rab25 expression in these tissues is positively correlated with cancer aggressiveness and progression. Physical interaction of Rab25 with the adhesion protein integrin has been reported and proposed as the driving force for increased tumor invasion (Cheng et al., 2004; Subramani and Alahari, 2010).
Regarding Rab18, to date a number of microarray analyses have shown that RAB18 gene expression is deregulated in neuroendocrine gastrointestinal tumors (Hofsli et al., 2005), prostate adenocarcinoma (True et al., 2006), breast cancer (Yan et al., 2008), and pancreatic adenocarcinoma (Kalinina et al., 2010). These findings suggest that Rab18 may constitute a valuable target as a diagnostic, prognostic, and/or therapeutic tool for these types of cancers. However, these studies do not provide further characterization of the precise relationship of this GTPase with the pathophysiology of these tumors. As mentioned before, we have demonstrated that Rab18 negatively modulate the secretory activity of neuroendocrine cells. Therefore, one could envisage that alterations in Rab18 expression and/or activity would yield malfunctions of the secretory process, such as those exhibited by many endocrine disorders characterized by hypersecretion of a particular hormone. This is the case for acromegaly, a pituitary tumor associated to high circulating levels of growth hormone (GH; Melmed et al., 2002). We recently demonstrated that in patients with acromegaly elevated GH plasma levels arise from both somatotrope hyperplasia and altered secretory activity of individual cells (Vazquez-Martinez et al., 2008). These findings suggested that the pathological alterations leading to GH hypersecretion might also have a secretory pathway-related molecular component intrinsic to each individual somatotropinoma cell. In fact, all acromegalic tissues analyzed exhibited an abnormally reduced level of Rab18 expression as compared to healthy pituitary glands or glands from patients with non-functioning adenomas that presented normal GH circulating levels. These findings pointed to the lack of this protein as one of the possible causatives of the hypersecretory activity of somatotropinoma cells. Interestingly, double immunoelectron microscopy revealed that the proportion of Rab18-immunolabeled secretory granules was consistently lower in somatotropes from acromegalic patients than in those from patients with non-functioning adenomas. Accordingly, Rab18 protein content observed by immunofluorescence was also lower in single pituitary cells from the former patients. Furthermore, the hypersecretory activity shown by these somatotropinoma cells could be reverted substantially by overexpression of Rab18, thereby suggesting that modulation of Rab18 production may represent an important point of control within the process of hormone secretion in these tumor cells. Altogether, these results suggest that low Rab18 content in somatotropinoma cells could result in the partial loss of control on the molecular mechanism that retains a particular subset of the secretory granules from being released under basal conditions and/or on stimulatory inputs. Figure 2 presents a theoretical model of the contribution of Rab18 to the hypersecretory state of somatotropinoma cells in acromegaly. Finally, it is worth to highlight that these data are the first report directly linking a member of the Rab GTPase family with dysfunctions of endocrine cells in humans.
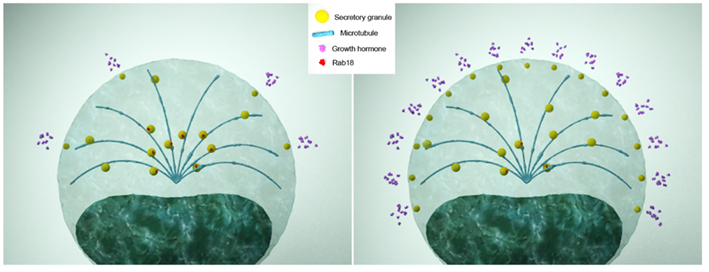
Figure 2. Theoretical model of the contribution of Rab18 to the hypersecretory state of somatotropinoma cells in acromegalic patients. In physiological conditions (left panel), somatotrope cells express normal levels of Rab18, which retains a number of GH-containing secretory granules from being released upon stimulatory inputs, thus ensuring the maintenance of the secretory response of cells within non-pathological limits. In acromegalic patients (right panel), somatotropinoma cells express lower levels of Rab18, which would cause uncontrolled secretory granule transport toward the plasma membrane and, consequently, an abnormally enhanced secretory response.
Conclusion
In the last two decades a large contribution to ascertain the specific participation of each Rab protein in the endomembrane organization and the secretory process has been made. Nevertheless, when taking into account the diversity of Rabs in humans and the high number of Rab regulators and effectors, it is evident that there is still a long way until we get a unifying view to better understand the profound implications of Rab proteins on the cell physiology under both normal and pathological conditions. Once the specific location and possible function of Rab proteins in the secretory process have been established, the next major challenge will be to determine their spatio-temporal interactomes. Next, we must face the endeavor of understanding the workings of the Rab/effector trafficking machineries in relation to the different stages of the secretory pathway. For this, we should address the following questions. How and when do Rab/effector complexes interact with cargo carriers? What are the molecular mechanisms whereby these complexes govern secretory vesicle movement? How do they interact with cytoskeleton networks? How is specific vesicle addressing achieved? This information will definitely contribute to gain further knowledge on the pathophysiology of a number of (neuro)endocrine diseases and forms of cancer that appear as a consequence of a malfunctioning in the control of the secretory pathway.
Conflict of Interest Statement
The authors declare that the research was conducted in the absence of any commercial or financial relationships that could be construed as a potential conflict of interest.
Acknowledgments
This work was supported by FEDER (BFU2007-60180/BFI, HF2007-0002), FIS/ISCIII (CIBERobn CB03/06), and J. Andalucia (CVI-0139, P07-CTS-03039), Spain.
References
Agarwal, R., Jurisica, I., Mills, G. B., and Chen, K. W. (2009). The emerging role of the Rab25 small GTPase in cancer. Traffic 10, 1561–1568.
Alexandrov, K., Horiuchi, H., Steele-Mortimer, O., Seabra, M. C., and Zerial, M. (1994). Rab escort protein-1 is a multifunctional protein that accompanies newly prenylated rab proteins to their target membranes. EMBO J. 13, 5262–5273.
Ali, B. R., Wasmeier, C., Lamoreux, L., Strom, M., and Seabra, M. C. (2004). Multiple regions contribute to membrane targeting of Rab GTPases. J. Cell Sci. 117, 6401–6412.
Alory, C., and Balch, W. E. (2001). Organization of the Rab-GDI/CHM superfamily: the functional basis for choroideremia disease. Traffic 2, 532–543.
Amillet, J. M., Ferbus, D., Real, F. X., Antony, C., Muleris, M., Gress, T. M., and Goubin, G. (2006). Characterization of human Rab20 overexpressed in exocrine pancreatic carcinoma. Hum. Pathol. 37, 256–263.
Andres, D. A., Seabra, M. C., Brown, M. S., Armstrong, S. A., Smeland, T. E., Cremers, F. P., and Goldstein, J. L. (1993). cDNA cloning of component A of Rab geranylgeranyl transferase and demonstration of its role as a Rab escort protein. Cell 73, 1091–1099.
Angers, C. G., and Merz, A. J. (2010). New links between vesicle coats and Rab-mediated vesicle targeting. Semin. Cell Dev. Biol. doi:10.1016/j.semcdb.2010.07.003. [Epub ahead of print].
Bielli, A., Thornqvist, P. O., Hendrick, A. G., Finn, R., Fitzgerald, K., and McCaffrey, M. W. (2001). The small GTPase Rab4A interacts with the central region of cytoplasmic dynein light intermediate chain-1. Biochem. Biophys. Res. Commun. 281, 1141–1153.
Bonifacino, J. S., and Glick, B. S. (2004). The mechanisms of vesicle budding and fusion. Cell 116, 153–166.
Brett, C. L., Plemel, R. L., Lobinger, B. T., Vignali, M., Fields, S., and Merz, A. J. (2008). Efficient termination of vacuolar Rab GTPase signaling requires coordinated action by a GAP and a protein kinase. J. Cell Biol. 182, 1141–1151.
Brett, T. J., and Traub, L. M. (2006). Molecular structures of coat and coat-associated proteins: function follows form. Curr. Opin. Cell Biol. 18, 395–406.
Brumback, A. C., Lieber, J. L., Angleson, J. K., and Betz, W. J. (2004). Using FM1-43 to study neuropeptide granule dynamics and exocytosis. Methods 33, 287–294.
Calero, M., and Collins, R. N. (2002). Saccharomyces cerevisiae Pra1p/Yip3p interacts with Yip1p and Rab proteins. Biochem. Biophys. Res. Commun. 290, 676–681.
Calero, M., Winand, N. J., and Collins, R. N. (2002). Identification of the novel proteins Yip4p and Yip5p as Rab GTPase interacting factors. FEBS Lett. 515, 89–98.
Chavrier, P., Gorvel, J. P., Stelzer, E., Simons, K., Gruenberg, J., and Zerial, M. (1991). Hypervariable C-terminal domain of rab proteins acts as a targeting signal. Nature 353, 769–772.
Cheng, K. W., Lahad, J. P., Kuo, W. L., Lapuk, A., Yamada, K., Auersperg, N., Liu, J., Smith-McCune, K., Lu, K. H., Fishman, D., Gray, J. W., and Mills, G. B. (2004). The RAB25 small GTPase determines aggressiveness of ovarian and breast cancers. Nat. Med. 10, 1251–1256.
Cochilla, A. J., Angleson, J. K., and Betz, W. J. (1999). Monitoring secretory membrane with FM1-43 fluorescence. Annu. Rev. Neurosci. 22, 1–10.
Cogli, L., Piro, F., and Bucci, C. (2009). Rab7 and the CMT2B disease. Biochem. Soc. Trans. 37, 1027–1031.
Culine, S., Honore, N., Tavitian, A., and Olofsson, B. (1992). Overexpression of the ras-related rab2 gene product in peripheral blood mononuclear cells from patients with hematological and solid neoplasms. Cancer Res. 52, 3083–3088.
D’Adamo, P., Menegon, A., Lo Nigro, C., Grasso, M., Gulisano, M., Tamanini, F., Bienvenu, T., Gedeon, A. K., Oostra, B., Wu, S. K., Tandon, A., Valtorta, F., Balch, W. E., Chelly, J., and Toniolo, D. (1998). Mutations in GDI1 are responsible for X-linked non-specific mental retardation. Nat. Genet. 19, 134–139.
Dacks, J. B., and Field, M. C. (2004). “Eukaryotic cell evolution from a comparative genomic perspective: the endomembrane system,” in Organelles, Genomes and Eukaryote Phylogeny An Evolutionary Synthesis in the Age of Genomics, eds R. P. Hirt and D. S. Horner (Boca Raton: CRC Press), 309–334.
Dacks, J. B., and Field, M. C. (2007). Evolution of the eukaryotic membrane-trafficking system: origin, tempo, and mode. J. Cell Sci. 120, 2977–2985.
Dejgaard, S. Y., Murshid, A., Erman, A., Kizilay, O., Verbich, D., Lodge, R., Dejgaard, K., Ly-Hartig, T. B., Pepperkok, R., Simpson, J. C., and Presley, J. F. (2008). Rab18 and Rab43 have key roles in ER-Golgi trafficking. J. Cell Sci. 121, 2768–2781.
Delprato, A., Merithew, E., and Lambright, D. G. (2004). Structure, exchange determinants, and family-wide rab specificity of the tandem helical bundle and Vps9 domains of Rabex-5. Cell 118, 607–617.
Dumas, J. J., Zhu, Z., Connolly, J. L., and Lambright, D. G. (1999). Structural basis of activation and GTP hydrolysis in Rab proteins. Structure 7, 413–423.
Eathiraj, S., Pan, X., Ritacco, C., and Lambright, D. G. (2005). Structural basis of family-wide Rab GTPase recognition by rabenosyn-5. Nature 436, 415–419.
Echard, A., Jollivet, F., Martinez, O., Lacapere, J. J., Rousselet, A., Janoueix-Lerosey, I., and Goud, B. (1998). Interaction of a Golgi-associated kinesin-like protein with Rab6. Science 279, 580–585.
Farnsworth, C. C., Seabra, M. C., Ericsson, L. H., Gelb, M. H., and Glomset, J. A. (1994). Rab geranylgeranyl transferase catalyzes the geranylgeranylation of adjacent cysteines in the small GTPases Rab1A, Rab3A, and Rab5A. Proc. Natl. Acad. Sci. U.S.A. 91, 11963–11967.
Fischer von Mollard, G., Sudhof, T. C., and Jahn, R. (1991). A small GTP-binding protein dissociates from synaptic vesicles during exocytosis. Nature 349, 79–81.
Fukuda, M., Kanno, E., Saegusa, C., Ogata, Y., and Kuroda, T. S. (2002a). Slp4-a/granuphilin-a regulates dense-core vesicle exocytosis in PC12 cells. J. Biol. Chem. 277, 39673–39678.
Fukuda, M., Kuroda, T. S., and Mikoshiba, K. (2002b). Slac2-a/melanophilin, the missing link between Rab27 and myosin Va: implications of a tripartite protein complex for melanosome transport. J. Biol. Chem. 277, 12432–12436.
Gerdes, H. H. (2008). Membrane traffic in the secretory pathway. Cell. Mol. Life Sci. 65, 2779–2780.
Goody, R. S., Rak, A., and Alexandrov, K. (2005). The structural and mechanistic basis for recycling of Rab proteins between membrane compartments. Cell. Mol. Life Sci. 62, 1657–1670.
Haas, A. K., Yoshimura, S., Stephens, D. J., Preisinger, C., Fuchs, E., and Barr, F. A. (2007). Analysis of GTPase-activating proteins: Rab1 and Rab43 are key Rabs required to maintain a functional Golgi complex in human cells. J. Cell Sci. 120, 2997–3010.
Hammer, J. A. III, and Wu, X. S. (2002). Rabs grab motors: defining the connections between Rab GTPases and motor proteins. Curr. Opin. Cell Biol. 14, 69–75.
Hashim, S., Mukherjee, K., Raje, M., Basu, S. K., and Mukhopadhyay, A. (2000). Live Salmonella modulate expression of Rab proteins to persist in a specialized compartment and escape transport to lysosomes. J. Biol. Chem. 275, 16281–16288.
Hofsli, E., Thommesen, L., Yadetie, F., Langaas, M., Kusnierczyk, W., Falkmer, U., Sandvik, A. K., and Laegreid, A. (2005). Identification of novel growth factor-responsive genes in neuroendocrine gastrointestinal tumour cells. Br. J. Cancer 92, 1506–1516.
Holz, R. W., Brondyk, W. H., Senter, R. A., Kuizon, L., and Macara, I. G. (1994). Evidence for the involvement of Rab3A in Ca(2+)-dependent exocytosis from adrenal chromaffin cells. J. Biol. Chem. 269, 10229–10234.
Huizing, M., Anikster, Y., and Gahl, W. A. (2000). Hermansky–Pudlak syndrome and related disorders of organelle formation. Traffic 1, 823–835.
Jahn, R., and Scheller, R. H. (2006). SNAREs–engines for membrane fusion. Nat. Rev. Mol. Cell Biol. 7, 631–643.
Johannes, L., Lledo, P. M., Chameau, P., Vincent, J. D., Henry, J. P., and Darchen, F. (1998). Regulation of the Ca2+ sensitivity of exocytosis by Rab3a. J. Neurochem. 71, 1127–1133.
Johannes, L., Lledo, P. M., Roa, M., Vincent, J. D., Henry, J. P., and Darchen, F. (1994). The GTPase Rab3a negatively controls calcium-dependent exocytosis in neuroendocrine cells. EMBO J. 13, 2029–2037.
Jordens, I., Fernandez-Borja, M., Marsman, M., Dusseljee, S., Janssen, L., Calafat, J., Janssen, H., Wubbolts, R., and Neefjes, J. (2001). The Rab7 effector protein RILP controls lysosomal transport by inducing the recruitment of dynein–dynactin motors. Curr. Biol. 11, 1680–1685.
Kalinina, T., Gungor, C., Thieltges, S., Moller-Krull, M., Murga-Penas, E. M., Wicklein, D., Streichert, T., Schumacher, U., Kalinin, V., Simon, R., Otto, B., Dierlamm, J., Schwarzenbach, H., Effenberger, K. E., Bockhorn, M., Izbicki J. R., and Yekebas, E. F. (2010). Establishment and characterization of a new human pancreatic adenocarcinoma cell line with high metastatic potential to the lung. BMC Cancer 10, 295–307. doi: 10.1186/1471-2407-10-295
Khvotchev, M. V., Ren, M., Takamori, S., Jahn, R., and Sudhof, T. C. (2003). Divergent functions of neuronal Rab11b in Ca2+ -regulated versus constitutive exocytosis. J. Neurosci. 23, 10531–10539.
Kuroda, T. S., and Fukuda, M. (2005). Functional analysis of Slac2-c/MyRIP as a linker protein between melanosomes and myosin VIIa. J. Biol. Chem. 280, 28015–28022.
Lane, K. T., and Beese, L. S. (2006). Thematic review series: lipid posttranslational modifications. Structural biology of protein farnesyltransferase and geranylgeranyltransferase type I. J. Lipid Res. 47, 681–699.
Lankat-Buttgereit, B., Fehmann, H. C., Hering, B. J., Bretzel, R. G., and Goke, B. (1994). Expression of the ras-related rab3a gene in human insulinomas and normal human pancreatic islets. Pancreas 9, 434–438.
Lee, M. T., Mishra, A., and Lambright, D. G. (2009). Structural mechanisms for regulation of membrane traffic by rab GTPases. Traffic 10, 1377–1389.
Leung, K. F., Baron, R., Ali, B. R., Magee, A. I., and Seabra, M. C. (2007). Rab GTPases containing a CAAX motif are processed post-geranylgeranylation by proteolysis and methylation. J. Biol. Chem. 282, 1487–1497.
Leung, K. F., Baron, R., and Seabra, M. C. (2006). Thematic review series: lipid posttranslational modifications. Geranylgeranylation of Rab GTPases. J. Lipid Res. 47, 467–475.
Li, Y., Meng, X., Feng, H., Zhang, G., Liu, C., and Li, P. (1999). Over-expression of the RAB5 gene in human lung adenocarcinoma cells with high metastatic potential. Chin. Med. Sci. J. 14, 96–101.
Lutcke, A., Parton, R. G., Murphy, C., Olkkonen, V. M., Dupree, P., Valencia, A., Simons, K., and Zerial, M. (1994). Cloning and subcellular localization of novel rab proteins reveals polarized and cell type-specific expression. J. Cell Sci. 107(Pt 12), 3437–3448.
Malagon, M. M., Cruz, D., Vazquez-Martinez, R., Peinado, J. R., Anouar, Y., Tonon, M. C., Vaudry, H., Gracia-Navarro, F., and Castano, J. P. (2005). Analysis of Rab18 and a new golgin in the secretory pathway. Ann. N. Y. Acad. Sci. 1040, 137–139.
Martelli, A. M., Baldini, G., Tabellini, G., Koticha, D., and Bareggi, R. (2000). Rab3A and Rab3D control the total granule number and the fraction of granules docked at the plasma membrane in PC12 cells. Traffic 1, 976–986.
Martin, S., Driessen, K., Nixon, S. J., Zerial, M., and Parton, R. G. (2005). Regulated localization of Rab18 to lipid droplets: effects of lipolytic stimulation and inhibition of lipid droplet catabolism. J. Biol. Chem. 280, 42325–42335.
Melmed, S., Casanueva, F. F., Cavagnini, F., Chanson, P., Frohman, L., Grossman, A., Ho, K., Kleinberg, D., Lamberts, S., Laws, E., Lombardi, G., Vance, M. L., Werder, K. V., Wass, J., and Giustina, A. (2002). Guidelines for acromegaly management. J. Clin. Endocrinol. Metab. 87, 4054–4058.
Menasche, G., Pastural, E., Feldmann, J., Certain, S., Ersoy, F., Dupuis, S., Wulffraat, N., Bianchi, D., Fischer, A., Le Deist, F., and de Saint Basile, G. (2000). Mutations in RAB27A cause Griscelli syndrome associated with haemophagocytic syndrome. Nat. Genet. 25, 173–176.
Miaczynska, M., Pelkmans, L., and Zerial, M. (2004). Not just a sink: endosomes in control of signal transduction. Curr. Opin. Cell Biol. 16, 400–406.
Mizuno-Yamasaki, E., Medkova, M., Coleman, J., and Novick, P. (2010). Phosphatidylinositol 4-phosphate controls both membrane recruitment and a regulatory switch of the Rab GEF Sec2p. Dev. Cell 18, 828–840.
Nordmann, M., Cabrera, M., Perz, A., Brocker, C., Ostrowicz, C., Engelbrecht-Vandre, S., and Ungermann, C. (2010). The Mon1–Ccz1 complex is the GEF of the late endosomal Rab7 homolog Ypt7. Curr. Biol. 20, 1654–1659.
Ostermeier, C., and Brunger, A. T. (1999). Structural basis of Rab effector specificity: crystal structure of the small G protein Rab3A complexed with the effector domain of rabphilin-3A. Cell 96, 363–374.
Ozeki, S., Cheng, J., Tauchi-Sato, K., Hatano, N., Taniguchi, H., and Fujimoto, T. (2005). Rab18 localizes to lipid droplets and induces their close apposition to the endoplasmic reticulum-derived membrane. J. Cell Sci. 118, 2601–2611.
Park, J. J., and Loh, Y. P. (2008). How peptide hormone vesicles are transported to the secretion site for exocytosis. Mol. Endocrinol. 22, 2583–2595.
Pereira-Leal, J. B., and Seabra, M. C. (2001). Evolution of the Rab family of small GTP-binding proteins. J. Mol. Biol. 313, 889–901.
Pfeffer, S., and Aivazian, D. (2004). Targeting Rab GTPases to distinct membrane compartments. Nat. Rev. Mol. Cell Biol. 5, 886–896.
Regazzi, R., Ravazzola, M., Iezzi, M., Lang, J., Zahraoui, A., Andereggen, E., Morel, P., Takai, Y., and Wollheim, C. B. (1996). Expression, localization and functional role of small GTPases of the Rab3 family in insulin-secreting cells. J. Cell Sci. 109, 2265–2273.
Sacher, M., Kim, Y. G., Lavie, A., Oh, B. H., and Segev, N. (2008). The TRAPP complex: insights into its architecture and function. Traffic 9, 2032–2042.
Schluter, O. M., Schmitz, F., Jahn, R., Rosenmund, C., and Sudhof, T. C. (2004). A complete genetic analysis of neuronal Rab3 function. J. Neurosci. 24, 6629–6637.
Seabra, M. C. (1996). New insights into the pathogenesis of choroideremia: a tale of two REPs. Ophthalmic Genet. 17, 43–46.
Seabra, M. C., and Wasmeier, C. (2004). Controlling the location and activation of Rab GTPases. Curr. Opin. Cell Biol. 16, 451–457.
Seaman, M. N. (2008). Endosome protein sorting: motifs and machinery. Cell. Mol. Life Sci. 65, 2842–2858.
Sonnichsen, B., De Renzis, S., Nielsen, E., Rietdorf, J., and Zerial, M. (2000). Distinct membrane domains on endosomes in the recycling pathway visualized by multicolor imaging of Rab4, Rab5, and Rab11. J. Cell Biol. 149, 901–914.
Stenmark, H. (2009). Rab GTPases as coordinators of vesicle traffic. Nat. Rev. Mol. Cell Biol. 10, 513–525.
Stenmark, H., Valencia, A., Martinez, O., Ullrich, O., Goud, B., and Zerial, M. (1994). Distinct structural elements of rab5 define its functional specificity. EMBO J. 13, 575–583.
Stroupe, C., and Brunger, A. T. (2000). Crystal structures of a Rab protein in its inactive and active conformations. J. Mol. Biol. 304, 585–598.
Subramani, D., and Alahari, S. K. (2010). Integrin-mediated function of Rab GTPases in cancer progression. Mol. Cancer 9, 312–320.
Takamori, S., Holt, M., Stenius, K., Lemke, E. A., Gronborg, M., Riedel, D., Urlaub, H., Schenck, S., Brugger, B., Ringler, P., Muller, S. A., Rammner, B., Grater, F., Hub, J. S., De Groot, B. L., Mieskes, G., Moriyama, Y., Klingauf, J., Grubmuller, H., Heuser, J., Wieland, F., and Jahn, R. (2006). Molecular anatomy of a trafficking organelle. Cell 127, 831–846.
Thoma, N. H., Iakovenko, A., Kalinin, A., Waldmann, H., Goody, R. S., and Alexandrov, K. (2001a). Allosteric regulation of substrate binding and product release in geranylgeranyltransferase type II. Biochemistry 40, 268–274.
Thoma, N. H., Niculae, A., Goody, R. S., and Alexandrov, K. (2001b). Double prenylation by RabGGTase can proceed without dissociation of the mono-prenylated intermediate. J. Biol. Chem. 276, 48631–48636.
True, L., Coleman, I., Hawley, S., Huang, C. Y., Gifford, D., Coleman, R., Beer, T. M., Gelmann, E., Datta, M., Mostaghel, E., Knudsen, B., Lange, P., Vessella, R., Lin, D., Hood, L., and Nelson, P. S. (2006). A molecular correlate to the Gleason grading system for prostate adenocarcinoma. Proc. Natl. Acad. Sci. U.S.A. 103, 10991–10996.
Vazquez-Martinez, R., Cruz-Garcia, D., Duran-Prado, M., Peinado, J. R., Castano, J. P., and Malagon, M. M. (2007). Rab18 inhibits secretory activity in neuroendocrine cells by interacting with secretory granules. Traffic 8, 867–882.
Vazquez-Martinez, R., Martinez-Fuentes, A. J., Pulido, M. R., Jimenez-Reina, L., Quintero, A., Leal-Cerro, A., Soto, A., Webb, S. M., Sucunza, N., Bartumeus, F., Benito-Lopez, P., Galvez-Moreno, M. A., Castano, J. P., and Malagon, M. M. (2008). Rab18 is reduced in pituitary tumors causing acromegaly and its overexpression reverts growth hormone hypersecretion. J. Clin. Endocrinol. Metab. 93, 2269–2276.
Vetter, I. R., and Wittinghofer, A. (2001). The guanine nucleotide-binding switch in three dimensions. Science 294, 1299–1304.
Yan, L. X., Huang, X. F., Shao, Q., Huang, M. Y., Deng, L., Wu, Q. L., Zeng, Y. X., and Shao, J. Y. (2008). MicroRNA miR-21 overexpression in human breast cancer is associated with advanced clinical stage, lymph node metastasis and patient poor prognosis. RNA 14, 2348–2360.
Yang, X., Matern, H. T., and Gallwitz, D. (1998). Specific binding to a novel and essential Golgi membrane protein (Yip1p) functionally links the transport GTPases Ypt1p and Ypt31p. EMBO J. 17, 4954–4963.
Young, P., and Suter, U. (2003). The causes of Charcot–Marie–Tooth disease. Cell. Mol. Life Sci. 60, 2547–2560.
Yu, H., Leaf, D. S., and Moore, H. P. (1993). Gene cloning and characterization of a GTP-binding Rab protein from mouse pituitary AtT-20 cells. Gene 132, 273–278.
Yu, H., and Schreiber, S. L. (1995). Structure of guanine-nucleotide-exchange factor human Mss4 and identification of its Rab-interacting surface. Nature 376, 788–791.
Keywords: neuroendocrine cells, Rab proteins, Rab18, secretory pathway, vesicle traffic
Citation: Vázquez-Martínez R and Malagón MM (2011) Rab proteins and the secretory pathway: the case of Rab18 in neuroendocrine cells. Front. Endocrin. 2:1. doi: 10.3389/fendo.2011.00001
Received: 28 October 2010;
Paper pending published: 15 December 2010;
Accepted: 03 January 2011;
Published online: 17 January 2011.
Edited by:
Eric W. Roubos, Radboud University Nijmegen, NetherlandsReviewed by:
Gerard Martens, Radboud University Nijmegen, NetherlandsMiguel Seabra, Imperial College London, UK
Copyright: © 2011 Vázquez-Martínez and Malagón. This is an open-access article subject to an exclusive license agreement between the authors and the Frontiers Research Foundation, which permits unrestricted use, distribution, and reproduction in any medium, provided the original authors and source are credited.
*Correspondence: Maria M. Malagón, Department of Cell Biology, Physiology and Immunology, Universidad de Córdoba, Campus de Rabanales, Edificio Severo-Ochoa, Planta 3, E-14014 Córdoba, Spain. e-mail:YmMxbWFwb21AdWNvLmVz