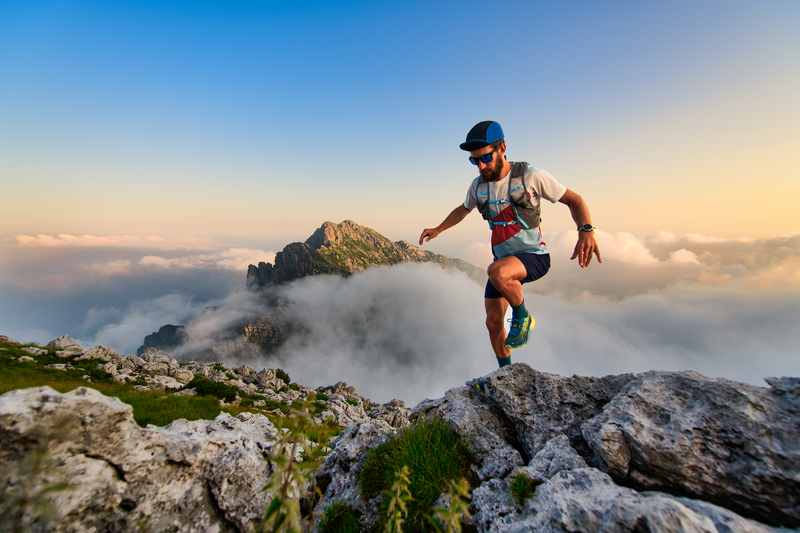
95% of researchers rate our articles as excellent or good
Learn more about the work of our research integrity team to safeguard the quality of each article we publish.
Find out more
PERSPECTIVE article
Front. Electron. , 04 April 2025
Sec. Flexible Electronics
Volume 6 - 2025 | https://doi.org/10.3389/felec.2025.1528802
Flexible electronic skin has garnered significant interest due to its promising applications in underwater robotics, aircraft monitoring systems, and human healthcare systems. A critical requirement for flexible electronic skin is to exhibit high sensitivity, stretchability, and stability. Functional materials, as essential components of flexible electronic skin, significantly influence the overall performance of the device. Consequently, a variety of material and structural designs have been developed to enhance the performance of functional materials. This perspective delves into recent advances in the development of functional materials and engineering strategies that endow electronic skin with sensitivity, stretchability, and stability. The applications of the smart electronic skin for precise decoding of flow field are highlighted. Finally, a forward-looking perspective is provided on the future of flexible electronic skin for flow field decoding, which outlines the challenges and opportunities for ongoing research and innovation in this field.
Flexible electronic skin is an innovative technology that emulates the properties of human skin, offering remarkable sensitivity and adaptability. Composed of advanced functional materials, electronic skin enables real-time monitoring of environmental and physiological signals, making it crucial for applications in healthcare, robotics, and wearable devices (Zarei et al., 2023; Yang et al., 2024).
To replicate the properties of the natural skin, two approaches are employed from the perspective of materials consideration and engineering strategies. A rich set of high-performance functional materials are integrated with soft substrate for tactile sensation, including piezoelectric materials (Qiu et al., 2024), metal nanowires (Raman and Arunagirinathan, 2022), carbon-configured materials (Min et al., 2023), hydrogels (Hu L. et al., 2023), etc. Owing to their intrinsically flexibility, multifunctionality, ultrahigh stretchability, and long-term stability, these materials endow the electronic skin with superior capability to detect multiplexed and broad-range of stimuli such as pressure (Li J. et al., 2023), strain (Dai W. et al., 2024), temperature (Liao et al., 2024; Mao et al., 2024) and so on.
However, simply employing high-performance functional materials tend to exhibit insufferable hysteresis and response time, which is insufficient to meet the increasingly complex demands of electronic skin (Liu et al., 2021). Therefore, a preferred tactic is to introduce engineering strategies to further modify the structures of functional materials, resulting in further enhancements to the performance of electronic skin. Techniques such as ultra-thin films, serpentine or island bridge design and nano-micro structures (Niu et al., 2023) have been employed. These advancements have resulted in the creation of smart electronic skin, which seamlessly integrates flexible electronic technology with machine learning-supported smart systems (Kim et al., 2019; Jung et al., 2020).
Flow fields are of utmost importance in numerous domains, including water flow, airflow, and blood circulation. The rapid evolution of underwater exploration, aerospace, and medical surveillance has heightened the demand for precise flow field decoding, notably transformed by the advent of flexible electronic skin (Wu et al., 2020). The flexible electronics enable profound understanding and accurate quantification of the role of flow fields in diverse environments and biological systems, thereby propelling technological advancements and sparking innovations in these fields.
In this perspective, we summarize the advances in the development of functional materials and engineering strategies that give electronics high sensitivity, stretchability, and stability. We highlight the applications of smart electronic skin in precisely decoding flow fields (Figure 1). Lastly, we provide a forward-looking perspective on the challenges and opportunities for future research and innovation in the field of flexible electronic skin for flow field decoding.
Figure 1. Schematic of the overall contents of this perspective. Reprinted with permission from refs. (Wu et al., 2017; Li et al., 2020; Wang F. et al., 2021; Hu X. et al., 2023; Yang et al., 2023; Dai H. et al., 2024; Gong et al., 2024; Shao et al., 2024; Wang X. et al., 2024). Copyright 2017 American Chemical Society; Copyright 2020 Oxford University Press; Copyright 2021 The American Association for the Advancement of Science; Copyright 2024 John Wiley and Sons; Copyright 2023 John Wiley and Sons; Copyright 2023 Springer Nature; Copyright 2024 John Wiley and Sons; Copyright 2024 Springer Nature.
For flexible electronic skin, the structure typically consists of three parts: stretchable substrate, sensitive functional component, and stable packaging layer. In practical applications, polydimethylsiloxane (PDMS) (Nan et al., 2024), ecoflex (Li N. et al., 2024), dragon skin (Liu et al., 2024) and styrene-ethylene/butylene-styrene (SEBS) (Shao et al., 2023) films are widely used as the stretchable substrates. To enhance the sensitivity of the electronic skin, a prevalent approach is to integrate the sensitive functional component. In this regard, flexible electronic skin based on highly sensitive piezoelectric polymers and piezoelectric ceramics have been widely employed, facilitating the accurate detection of diverse stimuli (Ha et al., 2019; Han M. et al., 2019; Mahapatra et al., 2021). In addition to piezoelectric materials, most of the functional materials are used as conductors, either by penetrating within the elastic matrix or being dispersed on its surface to form piezoresistive sensors, such as carbon-framed materials (especially carbon nanotubes (CNTs) (Lin et al., 2022), graphene (Zheng et al., 2020), carbon black (CB) (Shao et al., 2023), and carbides (Mxene) (Guo et al., 2021)), metal-based nanomaterials (Raman and Arunagirinathan, 2022), inorganic semiconductor materials (Hu et al., 2024) and hydrogels (Tang et al., 2024).
Among these materials, graphene exhibits exceptional electrical conductivity and is widely employed. For instance, Figure 2A illustrates the graphite nanoplates penetrate with polyurethane (PU) to form nanocomposite film, which demonstrates superior electrical conductivity and extremely high flexibility (Wu et al., 2017). However, the percolation-based sensor rarely exhibits good linear performance on a larger scale due to the uneven mixing and the uncontrollable disruption of conductive filler network. Therefore, surface modification of the mentioned material is required to gain desirable mechanical properties (Lin et al., 2022). In addition, laser-induced graphene combined with an elastic ecoflex polymer forms a stretchable electronic skin that demonstrates high sensitivity and stability (Li Y. et al., 2024). Han Z. et al. (2019) drop casted the carbon black (CB) solutions onto airlaid paper to obtain an ultrahigh sensitivity and flexible pressure sensor. Song et al. (2023) presented a 3D-printed pressure sensor consisting of an interdigital MXene electrode and a porous CNT-PDMS active layer, which yields the highest sensitivity due to the increased contact area, as shown in Figure 2B.
Figure 2. Engineering strategies of functional materials for flexible electronic skin development. Materials considerations: (A) Graphite (Wu et al., 2017). Reprinted with permission. Copyright 2017 American Chemical Society. (B) Carbon nanotubes (Song et al., 2023). Reprinted with permission. Copyright 2023 The American Association for the Advancement of Science. (C) Monocrystalline silicon (Hu et al., 2024). Copyright 2024 The American Association for the Advancement of Science. (D) AgNWs (Liu et al., 2022). Copyright 2022 The American Association for the Advancement of Science. (E) Hydrogel (Liao et al., 2024). Reprinted with permission. Copyright 2024 John Wiley and Sons. Engineering strategies: (F) Ultrathin film (Hu X. et al., 2023). Reprinted with permission. Copyright 2023 John Wiley and Sons. (G) Serpentine structure (Jang et al., 2022). Reprinted with permission. Copyright 2022 Springer Nature. (H) Island-bridge structure (Zhu et al., 2018). Reprinted with permission. Copyright 2018 The American Association for the Advancement of Science. (I) Pyramid (Yang et al., 2023). Reprinted with permission. Copyright 2023 Springer Nature. (J) Crack (Kim et al., 2020). Reprinted with permission. Copyright 2020 Springer Nature. Applications of smart electronic skin for precise decoding of flow field. (K) Underwater flow decoding (Dai H. et al., 2024). Reprinted with permission. Copyright 2024 John Wiley and Sons. (L) Air flow decoding (Gong et al., 2024). Reprinted with permission. Copyright 2024 Springer Nature. (M) Respiration flow decoding (Li Y. et al., 2023). Reprinted with permission. Copyright 2023 Elsevier. (N) Arteries blood flow detection (Wang F. et al., 2021). (O) Hemodynamic detection (Ma et al., 2023). Reprinted with permission. Copyright 2023 Elsevier.
Although promising, ongoing challenges for these carbon-framed functional materials-based devices typically suffer from device-to-device variation. In contrast, well-established inorganic semiconductor-based strain sensor exhibits uniformity and consistency in the device performance, demonstrating the omnidirectional capability (Figure 2C) (Hu et al., 2024).
Flexible electronic skin using metal nanowires have attracted notable attention, attributed to their high electrical conductivity and mechanical flexibility. Figure 2D illustrates a micromolding-based method for printing silver nanowires (AgNWs), which demonstrates potential applications in soft electronics (Liu et al., 2022).
As a promising alternative, hydrogels have gained widespread attention due to their exceptional high stretchability, good conductivity, biocompatibility, and mechanical properties. Figure 2E shows a programmable microfluidic-assisted hydrogel patch that features high stretchability and impressive conductivity (Liao et al., 2024). In addition, Wang et al. developed a stretchable hydrogel-based multimodal electronic skins that can self-calibrate the sensing of any two of three stimuli: strain, temperature, and humidity (Wang W. et al., 2024).
Stable packaging materials play a pivotal role in the performance of electronic skin, providing long-term durability under various conditions (Zhou et al., 2024). The materials such as parylene (Mariello et al., 2021), polyimide (Kim et al., 2018), PDMS (Li J. et al., 2024) and SEBS (Yi et al., 2023) are often employed as the packaging materials for 2D electronic device. For instance, Wang X. et al. (2024) employed PDMS silicone gel to package the flexible pressure sensor array, which serves to isolate the air. This isolation effectively safeguards oxidative behavior of the electrode and sensitive layers within the sensor, thereby ensuring the long-term usability of the sensor. For three-dimensionally (3D) architected electronic skin, Liu et al. (2024) proposed a heterogeneous encapsulation strategy that encapsulates functional components with different layers and materials, which ensures that the surrounding soft materials have similar mechanical properties to the human skin.
In addition to the material point of view, the property of flexible electronic skin can be improved from the engineering strategies, including ultra-thin films, serpentine design, micro-nano structures. The ultra-thin film ensures low modulus mechanics, which endows the sensor with good conformability to curved surfaces, avoiding any significant constraint on natural motions of the skin, when undergoing pressing or stretching (Wu et al., 2020). A dual-sacrificial-layer method has been employed to produce a CNT-based thinnest pressure sensor featuring a thickness of ≈850 nm, as shown in Figure 2F (Hu X. et al., 2023). The sensor achieves superior sensitivity and perfect conformability simultaneously. Most of the existing hydrogels are relatively thick and have poor air permeability. Zhang et al. (2024) presents a ∼10-μm-thick hydrogel sensor, which exhibits great skin compliance.
The electronic skins demonstrate certain stretchability on highly elastic substrates; however, the conductive path is susceptible to fracture when subjected to high strain. To enhance the stretchability of the electronic skin, designs incorporating serpentine patterns and island-bridge structures are proposed. These designs possess low stiffness and good stretchability, avoiding the generation of large or irreversible cracks in a certain strain region and ensure good electrical interconnection between the sensing units (Jo et al., 2024). By analyzing various stretchable 2D and 3D sensing units, including zigzag, rhomb, serpentine, net, wave and spring, the results show that 2D structures have the superiority in thickness and attachable comfort as wearable devices attached on tendons (Shu et al., 2021). Taking advantage of the serpentine structure, Jang et al. (2022) proposed heterogeneous serpentine ribbons, which enable ambulatory electrodermal activity monitoring on the palm in free-living conditions, as shown in Figure 2G. Cai et al. (2021) reported a multifunctional electronic skin based on a patterned serpentine metal film for tactile sensing of pressure and temperature, which exhibited excellent flexibility and wearability. A stretchable ultrasound probe that exploits an island-bridge layout with multilayer electrodes, showing excellent stretchability (Zhu et al., 2018) (Figure 2H). In addition to serpentine patterns and island-bridge structures, spiral form, kirigami-inspired structures and watch-chain shapes (Li et al., 2020; Meng et al., 2022; Che et al., 2024) have also been used.
Micro-nano structures located on the surface or inside the sensing layers have demonstrated their effectiveness in enhancing the sensitivity, stretchability, and stability of the flexible electronic skin. Various forms of micro-nano structures have been employed, such as porous (Xiao et al., 2024), micro-pyramids (Hu X. et al., 2023), nanofibers (Bai et al., 2023), cracks (Zhang et al., 2023), nanowires (Won et al., 2019), interlocking structure (Ilami et al., 2021), interface non-uniform structure (yarn structure) (Liang et al., 2020) and so on (Niu et al., 2021).
Compared with ordinary planar films, the porous structure can achieve higher tensile and perceptual properties (Park et al., 2012). Unlike the salt-templating method (Wang H. et al., 2021), the laser ablation can produce thinner porous structures. The porous structure of the laser-scribed graphene and PU nanomesh-based electronic skin can be attached on finger with clear fingerprint morphology (Qiao et al., 2022). The flexible electronic skin, which utilizes pressure-induced changes in the contact area of microstructures, typically demonstrates superior sensitivity and faster response times compared to alterations in the conductive network of porous structures (Liu et al., 2021). Figure 2I (Yang et al., 2023) presents a flexible electronic skin with gradient pyramidal microstructures fabricated by a multiple laser ablations method. The sensor allows the applications in subtle pulse detection, interactive robotic hand, and ultrahigh-resolution smart weight scale/chair. Besides, the all-fibrous based sensitive mechanoacoustic sensor demonstrates excellent heart signal detection ability (Zhi et al., 2023).
The spider-inspired cracked multifunctional electronic skin has been developed by employing a location-programmable uniaxial stretching method (Zhang et al., 2023). Owing to the strain sensitive cracked straight electrode, the device exhibits a high sensitivity. By employing a laser-induced nanoscale cracking method, Kim et al. (2020) combined the aforementioned engineering strategies and developed a novel flexible electronic skin featuring an ultra-thin crack-based layer and serpentine patterned structure with a thickness of ∼50 μm, as shown in Figure 2J. The sensor allows conformal contact with the epidermis, enabling a more direct measurement of skin deformation.
The flow field perception has been widely adopted in various fields, such as underwater robotics, aircrafts, and human healthcare monitoring. By incorporating engineering strategies for functional materials from the development of flexible electronic skin into flow field sensors, enhanced performance can be achieved. For example, the flexible polyvinylidene fluoride−trifluoroethylen/barium titanate [P(VDF-TrFE)/BTO] nanofiber mat-based flow sensor can be used for water flow detection (Hu et al., 2019). To enhance the sensitivity of the P(VDF-TrFE)/BTO nanofiber-based flow sensor, the involvement of hydrogel cupula is a viable approach (Ma et al., 2020). In addition, thermoresponsive hydrogel cupula can be designed to tune the sensitivity (Jiang et al., 2021). The harbor seal possesses ultrasensitive hydrodynamic trail-following capabilities, for the undulated geometric structure and material property of the whiskers (Kamat et al., 2023; Zheng et al., 2023). Inspired by the unique undulating morphology of harbor seal whiskers, the flow sensor featuring a 3D magnetic-based force-decoupling perception capability and a bionic seal whisker array structure proficient at detecting diverse hydrodynamic information, as shown in Figure 2K (Dai H. et al., 2024).
The flexible electronic skin can be used for air flow field perception in a non-destructive manner. Xiong et al. (2021) proposed a smart flexible sensing skin with a thickness of ∼80 μm, which used serpentine pattern Platinum (Pt) and Constantan alloy electrodes as temperature and strain sensors for multi-function flight perception. Gong et al. (2024) reported a flexible calorimetric sensor, less than 90 μm thick featuring a spiral heater and a thermistors array, which can be attached to a wing model to detect the angles of attack and slip, as shown in Figure 2L.
The flexible electronic skin inspired flow sensors demonstrate great potential in human breath detection. Li Y. et al. (2023) developed an integrated wearable smart respiratory monitoring sensor to analyze breath status, as shown in Figure 2M. Combined with artificial intelligence technology, the sensor facilitated the decoding of human respiratory flow and outperformed existing respiratory sensors in multi-parameter extraction. Meanwhile, the blockage of blood circulation will lead to serious cardiovascular disease. By employing advanced smart electronic skin technology, the dynamics of blood flow can be monitored in a non-invasive, continuous and accurate evaluation of vascular health (Wang et al., 2018; Fortin et al., 2021; Huang et al., 2024). Flexible Doppler ultrasound device with a 3 by 3 array of oblique 1–3 composite piezoelectric transducers, serpentine electrode structure and a soft silicone package can be used for the monitoring of carotid blood flow velocity, as shown in Figure 2N (Wang F. et al., 2021). The ultrasound patch with five layers of serpentine interconnections are used for continuous monitoring of cerebral hemodynamics, which enables screening and diagnosing brain disorders as well as understanding neurovascular functions (Zhou et al., 2024). Direct detection of blood flow provides critical insights into cardiovascular situation. Notably, changes in blood flow can significantly affect superficial blood pressure, making it possible to reflect blood flow through hemodynamic detection. The highly sensitive FlexiPulse with laser-engraved serpentine pattern, is capable of detecting subtle changes in strain induced by blood flow and enables independent clinical assessments of cardiovascular disease, as shown in Figure 2O (Ma et al., 2023). The kirigami-inspired highly sensitive and conformal pulse-wave sensor demonstrate accurate pulse waveforms compared with commercial medical device (Meng et al., 2022).
By leveraging the innovations in smart electronic skin, advanced flow sensing technologies hold the promise of providing improved robotic sensory systems, and more efficient aerodynamics in aircraft and real-time, precise monitoring capabilities that can lead to earlier detection of medical conditions.
In conclusion, the recent advancements in functional materials have significantly propelled the development of flexible electronic skin. By integrating functional materials with structural strategies, remarkable progress has been achieved in enhancing the sensitivity, stretchability, and stability of this technology. Furthermore, the emergence of smart electronic skin has enabled precise decoding of flow fields, marking a significant milestone in this field. Looking ahead, future research should prioritize the development of even more adaptable and multifunctional materials to expand the capabilities of flow sensors. Interdisciplinary collaborations between materials science, bioengineering, and artificial intelligence could unlock new possibilities for real-time analysis and automated decision-making in robotics and medicine. Furthermore, exploring the miniaturization and energy efficiency of these sensors will be crucial for their integration into portable and long-term monitoring systems. Lastly, the integration of artificial intelligence in smart electronic skin will enable real-time data analysis and interpretation of flow fields. This will not only enhance the accuracy and efficiency of data acquisition but also facilitate the development of intelligent systems that can respond dynamically to their surroundings. Overall, the future of flexible electronic skin is promising and will continue to revolutionize various industries with its advancements in materials, engineering strategies, and integration with smart technologies.
The original contributions presented in the study are included in the article/supplementary material, further inquiries can be directed to the corresponding authors.
XH: Conceptualization, Formal Analysis, Funding acquisition, Supervision, Writing – original draft, Writing – review and editing. SG: Conceptualization, Formal Analysis, Funding acquisition, Supervision, Writing – original draft, Writing – review and editing. YC: Writing – review and editing. FZ: Writing – review and editing.
The author(s) declare that financial support was received for the research and/or publication of this article. This work was supported by the Fundamental Research Funds for the Central Universities, China (Grant No. 2023JBMC019), the National Natural Science Foundation of China (Grant No. 50305035 and 52275004), and Beijing Natural Science Foundation (Grant No.3242012).
The authors declare that the research was conducted in the absence of any commercial or financial relationships that could be construed as a potential conflict of interest.
The handling editor ZM declared past co-authorship with the author XH.
The author(s) declare that no Generative AI was used in the creation of this manuscript.
All claims expressed in this article are solely those of the authors and do not necessarily represent those of their affiliated organizations, or those of the publisher, the editors and the reviewers. Any product that may be evaluated in this article, or claim that may be made by its manufacturer, is not guaranteed or endorsed by the publisher.
Bai, Y., Yin, L., Hou, C., Zhou, Y., Zhang, F., Xu, Z., et al. (2023). Response regulation for epidermal fabric strain sensors via mechanical strategy. Adv. Funct. Mater. 33, 1–13. doi:10.1002/adfm.202214119
Cai, M., Jiao, Z., Nie, S., Wang, C., Zou, J., and Song, J. (2021). A multifunctional electronic skin based on patterned metal films for tactile sensing with a broad linear response range. Sci. Adv. 7, eabl8313–10. doi:10.1126/sciadv.abl8313
Che, Z., Wan, X., Xu, J., Duan, C., Zheng, T., and Chen, J. (2024). Speaking without vocal folds using a machine-learning-assisted wearable sensing-actuation system. Nat. Commun. 15, 1873–1938. doi:10.1038/s41467-024-45915-7
Dai, H., Zhang, C., Hu, H., Hu, Z., Sun, H., Liu, K., et al. (2024a). Biomimetic hydrodynamic sensor with whisker array architecture and multidirectional perception ability. Adv. Sci. 11, 24052766–e2405312. doi:10.1002/advs.202405276
Dai, W., Lei, M., Dai, Z., Ding, S., Wang, F., Fang, D., et al. (2024b). Self-Adhesive electronic skin with bio-inspired 3D architecture for mechanical stimuli monitoring and human-machine interactions. Small 2406564 20, 24065644–e2406614. doi:10.1002/smll.202406564
Fortin, J., Rogge, D. E., Fellner, C., Flotzinger, D., Grond, J., Lerche, K., et al. (2021). A novel art of continuous noninvasive blood pressure measurement. Nat. Commun. 12, 1387. doi:10.1038/s41467-021-21271-8
Gong, Z., Di, W., Jiang, Y., Dong, Z., Yang, Z., Ye, H., et al. (2024). Flexible calorimetric flow sensor with unprecedented sensitivity and directional resolution for multiple flight parameter detection. Nat. Commun. 15, 3091. doi:10.1038/s41467-024-47284-7
Guo, Y., Wei, X., Gao, S., Yue, W., Li, Y., and Shen, G. (2021). Recent advances in carbon material-based multifunctional sensors and their applications in electronic skin systems. Adv. Funct. Mater. 31, 1–35. doi:10.1002/adfm.202104288
Ha, T., Tran, J., Liu, S., Jang, H., Jeong, H., Mitbander, R., et al. (2019). A chest-laminated Ultrathin and stretchable E-tattoo for the measurement of electrocardiogram, seismocardiogram, and cardiac time intervals. Adv. Sci. 6, 1900290. doi:10.1002/advs.201900290
Han, M., Wang, H., Yang, Y., Liang, C., Bai, W., Yan, Z., et al. (2019a). Three-dimensional piezoelectric polymer microsystems for vibrational energy harvesting, robotic interfaces and biomedical implants. Nat. Electron. 2, 26–35. doi:10.1038/s41928-018-0189-7
Han, Z., Li, H., Xiao, J., Song, H., Li, B., Cai, S., et al. (2019b). Ultralow-cost, highly sensitive, and flexible pressure sensors based on carbon black and airlaid paper for wearable electronics. ACS Appl. Mater. Interfaces 11, 33370–33379. doi:10.1021/acsami.9b12929
Hu, B., Xu, D., Shao, Y., Nie, Z., Liu, P., Li, J., et al. (2024). Ultrathin crystalline silicon-based omnidirectional strain gauges for implantable/wearable characterization of soft tissue biomechanics. Sci. Adv. 10, eadp8804. doi:10.1126/sciadv.adp8804
Hu, L., Chee, P. L., Sugiarto, S., Yu, Y., Shi, C., Yan, R., et al. (2023a). Hydrogel-based flexible electronics. Adv. Mater. 35, e2205326–e2205332. doi:10.1002/adma.202205326
Hu, X., Jiang, Y., Ma, Z., Xu, Y., and Zhang, D. (2019). Bio-inspired flexible lateral line sensor based on p(Vdf-trfe)/bto nanofiber mat for hydrodynamic perception. Sensors Switz. 19, 5384. doi:10.3390/s19245384
Hu, X., Wu, M., Che, L., Huang, J., Li, H., Liu, Z., et al. (2023b). Nanoengineering Ultrathin flexible pressure sensor with superior sensitivity and perfect conformability. Small 19, 22080155–e2208112. doi:10.1002/smll.202208015
Huang, H., Wu, R. S., Lin, M., and Xu, S. (2024). Emerging wearable ultrasound technology. IEEE Trans. Ultrason. Ferroelectr. Freq. Control 71, 713–729. doi:10.1109/TUFFC.2023.3327143
Ilami, M., Bagheri, H., Ahmed, R., Skowronek, E. O., and Marvi, H. (2021). Materials, actuators, and sensors for soft bioinspired robots. Adv. Mater. 33, e2003139–e2003147. doi:10.1002/adma.202003139
Jang, H., Sel, K., Kim, E., Kim, S., Yang, X., Kang, S., et al. (2022). Graphene e-tattoos for unobstructive ambulatory electrodermal activity sensing on the palm enabled by heterogeneous serpentine ribbons. Nat. Commun. 13, 6604–6613. doi:10.1038/s41467-022-34406-2
Jiang, Y., Wang, N., Zhuo, S., He, Q., Ma, Z., Liu, M., et al. (2021). Hydrodynamic pressure sensors with tunable sensitivity based on thermoresponsive hydrogels. J. Appl. Polym. Sci. 138, 1–8. doi:10.1002/app.50023
Jo, H., Song, Y., Lee, D., Kang, Y. J., Ahn, J., and Song, J. H. (2024). Lego-Like model reconfigurable and transparent stretchable strain sensor for wearable and biomedical applications. Adv. Funct. Mater. 34, 1–15. doi:10.1002/adfm.202306160
Jung, Y. H., Hong, S. K., Wang, H. S., Han, J. H., Pham, T. X., Park, H., et al. (2020). Flexible piezoelectric acoustic sensors and machine learning for speech processing. Adv. Mater. 32, 19040200–e1904118. doi:10.1002/adma.201904020
Kamat, A. M., Zheng, X., Bos, J., Cao, M., Triantafyllou, M. S., and Kottapalli, A. G. P. (2023). Undulating seal whiskers evolved optimal wavelength-to-diameter ratio for efficient reduction in vortex-induced vibrations. Adv. Sci. 11, e2304304–e2304315. doi:10.1002/advs.202304304
Kim, K., Ha, I., Kim, M., Choi, J., Won, P., Jo, S., et al. (2020). A deep-learned skin sensor decoding the epicentral human motions. Nat. Commun. 11, 2149. doi:10.1038/s41467-020-16040-y
Kim, T., Lee, T., Lee, G., Choi, Y. W., Kim, S. M., Kang, D., et al. (2018). Polyimide encapsulation of spider-inspired crack-based sensors for durability improvement. Appl. Sci. 8, 367. doi:10.3390/app8030367
Kim, Y. S., Mahmood, M., Lee, Y., Kim, N. K., Kwon, S., Herbert, R., et al. (2019). All-in-One, wireless, stretchable hybrid electronics for smart, connected, and ambulatory physiological monitoring. Adv. Sci. 6, 1900939. doi:10.1002/advs.201900939
Li, H., Ma, Y., Liang, Z., Wang, Z., Cao, Y., Xu, Y., et al. (2020). Wearable skin-like optoelectronic systems with suppression of motion artifacts for cuff-less continuous blood pressure monitor. Natl. Sci. Rev. 7, 849–862. doi:10.1093/nsr/nwaa022
Li, J., Jia, H., Zhou, J., Huang, X., Xu, L., Jia, S., et al. (2023a). Thin, soft, wearable system for continuous wireless monitoring of artery blood pressure. Nat. Commun. 14, 5009–5012. doi:10.1038/s41467-023-40763-3
Li, J., Jia, S., Li, D., Chow, L., Zhang, Q., Yang, Y., et al. (2024a). Wearable bio-adhesive metal detector array (BioMDA) for spinal implants. Nat. Commun. 15, 7800. doi:10.1038/s41467-024-51987-2
Li, N., Liu, L., Liu, Y., and Leng, J. (2024b). Metamaterial-based electronic skin with conformality and multisensory integration. Adv. Funct. Mater. 34, 1–12. doi:10.1002/adfm.202406789
Li, Y., Liu, C., Zou, H., Che, L., Sun, P., Yan, J., et al. (2023b). Integrated wearable smart sensor system for real-time multi-parameter respiration health monitoring. Cell Rep. Phys. Sci. 4, 101191. doi:10.1016/j.xcrp.2022.101191
Li, Y., Matsumura, G., Xuan, Y., Honda, S., and Takei, K. (2024c). Stretchable electronic skin using laser-induced graphene and liquid metal with an action recognition system powered by machine learning. Adv. Funct. Mater. 34, 1–10. doi:10.1002/adfm.202313824
Liang, X., Li, H., Dou, J., Wang, Q., He, W., Wang, C., et al. (2020). Stable and biocompatible carbon nanotube ink mediated by silk protein for printed electronics. Adv. Mater. 32, 20001655–e2000210. doi:10.1002/adma.202000165
Liao, J., Ma, Z., Liu, S., Li, W., Yang, X., Hilal, M. E., et al. (2024). Programmable microfluidic-assisted highly conductive hydrogel patches for customizable soft electronics. Adv. Funct. Mater. 34, 1–13. doi:10.1002/adfm.202401930
Lin, M., Zheng, Z., Yang, L., Luo, M., Fu, L., Lin, B., et al. (2022). A high-performance, sensitive, wearable multifunctional sensor based on rubber/CNT for human motion and skin temperature detection. Adv. Mater. 34, e2107309–e2107313. doi:10.1002/adma.202107309
Liu, M., Hang, C., Zhao, X., Zhu, L. Y., Ma, R. G., Wang, J. C., et al. (2021). Advance on flexible pressure sensors based on metal and carbonaceous nanomaterial. Nano Energy 87, 106181. doi:10.1016/j.nanoen.2021.106181
Liu, Y., Zheng, M., O’Connor, B., Dong, J., and Zhu, Y. (2022). Curvilinear soft electronics by micromolding of metal nanowires in capillaries. Sci. Adv. 8, eadd6996. doi:10.1126/sciadv.add6996
Liu, Z., Hu, X., Bo, R., Yang, Y., Cheng, X., Pang, W., et al. (2024). A three-dimensionally architected electronic skin mimicking human mechanosensation. Sci. (80) 384, 987–994. doi:10.1126/science.adk5556
Ma, Z., Hua, H., You, C., Ma, Z., Guo, W., Yang, X., et al. (2023). FlexiPulse: a machine-learning-enabled flexible pulse sensor for cardiovascular disease diagnostics. Cell Rep. Phys. Sci. 4, 101690. doi:10.1016/j.xcrp.2023.101690
Ma, Z., Xu, Y., Jiang, Y., Hu, X., and Zhang, D. (2020). BTO/P(VDF-TrFE) nanofiber-based artificial lateral line sensor with drag enhancement structures. J. Bionic Eng. 17, 64–75. doi:10.1007/s42235-020-0005-8
Mahapatra, D., Mohapatra, P., Aria, A., Christie, G., Mishra, Y. K., Hofmann, S., et al. (2021). Piezoelectric materials for energy harvesting and sensing applications: roadmap for future smart materials. Adv. Sci. 8, 2100864. doi:10.1002/advs.202100864
Mao, Q., Liao, Z., Yuan, J., and Zhu, R. (2024). Multimodal tactile sensing fused with vision for dexterous robotic housekeeping. Nat. Commun. 15, 6871–6912. doi:10.1038/s41467-024-51261-5
Mariello, M., Fachechi, L., Guido, F., and De Vittorio, M. (2021). Conformal, ultra-thin skin-contact-actuated hybrid piezo/triboelectric wearable sensor based on AlN and parylene-encapsulated elastomeric blend. Adv. Funct. Mater. 31, 1–19. doi:10.1002/adfm.202101047
Meng, K., Xiao, X., Liu, Z., Shen, S., Tat, T., Wang, Z., et al. (2022). Kirigami-inspired pressure sensors for wearable dynamic cardiovascular monitoring. Adv. Mater. 34, 22024788–e2202510. doi:10.1002/adma.202202478
Min, J. K., Jung, Y., Ahn, J., Lee, J. G., Lee, J., and Ko, S. H. (2023). Recent advances in biodegradable green electronic materials and sensor applications. Adv. Mater. 35, 22112733–e2211328. doi:10.1002/adma.202211273
Nan, K., Wong, K., Li, D., Ying, B., McRae, J. C., Feig, V. R., et al. (2024). An ingestible, battery-free, tissue-adhering robotic interface for non-invasive and chronic electrostimulation of the gut. Nat. Commun. 15, 6749. doi:10.1038/s41467-024-51102-5
Niu, H., Wei, X., Li, H., Yin, F., Wang, W., Seong, R. S., et al. (2023). Micropyramid array bimodal electronic skin for intelligent material and surface shape perception based on capacitive sensing. Adv. Sci. 11, 23055288–e2305613. doi:10.1002/advs.202305528
Niu, H., Zhang, H., Yue, W., Gao, S., Kan, H., Zhang, C., et al. (2021). Micro-nano processing of active layers in flexible tactile sensors via template methods: a review. Small 17, e2100804–e2100830. doi:10.1002/smll.202100804
Park, J., Wang, S., Li, M., Ahn, C., Hyun, J. K., Kim, D. S., et al. (2012). Three-dimensional nanonetworks for giant stretchability in dielectrics and conductors. Nat. Commun. 3, 916–918. doi:10.1038/ncomms1929
Qiao, Y., Li, X., Wang, J., Ji, S., Hirtz, T., Tian, H., et al. (2022). Intelligent and multifunctional graphene nanomesh electronic skin with high comfort. Small 18, e2104810–e2104811. doi:10.1002/smll.202104810
Qiu, Y., Wang, F., Zhang, Z., Shi, K., Song, Y., Lu, J., et al. (2024). Quantitative softness and texture bimodal haptic sensors for robotic clinical feature identification and intelligent picking. 0348 10, eadp0348–14. doi:10.1126/sciadv.adp0348
Raman, S., and Arunagirinathan, R. S. (2022). Silver nanowires in stretchable resistive strain sensors. Nanomaterials 12, 1932. doi:10.3390/nano12111932
Shao, B., Lu, M. H., Wu, T. C., Peng, W. C., Ko, T. Y., Hsiao, Y. C., et al. (2024). Large-area, untethered, metamorphic, and omnidirectionally stretchable multiplexing self-powered triboelectric skins. Nat. Commun. 15, 1238–1314. doi:10.1038/s41467-024-45611-6
Shao, W., Cui, T., Li, D., Jian, J., Li, Z., Ji, S., et al. (2023). Carbon-based textile sensors for physiological-signal monitoring. Mater. (Basel) 16, 3932. doi:10.3390/ma16113932
Shu, S., An, J., Chen, P., Liu, D., Wang, Z., Li, C., et al. (2021). Active-sensing epidermal stretchable bioelectronic patch for noninvasive conformal, and wireless tendon monitoring, 9783432. doi:10.34133/2021/9783432
Song, Y., Tay, R. Y., Li, J., Xu, C., Min, J., Sani, E. S., et al. (2023). 3D-printed epifluidic electronic skin for machine learning–powered multimodal health surveillance. Sci. Adv. 9, eadi6492–13. doi:10.1126/sciadv.adi6492
Tang, Y., Wu, B., Li, J., Lu, C., Wu, J., and Xiong, R. (2024). Biomimetic structural hydrogels reinforced by gradient twisted plywood architectures. Adv. Mater. 37, 24113722–e2411411. doi:10.1002/adma.202411372
Wang, C., Li, X., Hu, H., Zhang, L., Huang, Z., Lin, M., et al. (2018). Monitoring of the central blood pressure waveform via a conformal ultrasonic device. Nat. Biomed. Eng. 2, 687–695. doi:10.1038/s41551-018-0287-x
Wang, F., Jin, P., Feng, Y., Fu, J., Wang, P., Liu, X., et al. (2021a). Flexible Doppler ultrasound device for the monitoring of blood flow velocity. Sci. Adv. 7, eabi9283–10. doi:10.1126/sciadv.abi9283
Wang, H., Zhou, R., Li, D., Zhang, L., Ren, G., Wang, L., et al. (2021b). High-performance foam-shaped strain sensor based on carbon nanotubes and Ti3C2Tx MXene for the monitoring of human activities. ACS Nano 15, 9690–9700. doi:10.1021/acsnano.1c00259
Wang, W., Yao, D., Wang, H., Ding, Q., Luo, Y., Ding, H., et al. (2024a). A breathable, stretchable, and self-calibrated multimodal electronic skin based on hydrogel microstructures for wireless wearables. Adv. Funct. Mater. 34, 1–15. doi:10.1002/adfm.202316339
Wang, X., Wu, G., Zhang, X., Lv, F., Yang, Z., Nan, X., et al. (2024b). Traditional Chinese medicine (TCM)-Inspired fully printed soft pressure sensor array with self-adaptive pressurization for highly reliable individualized long-term pulse diagnostics. Adv. Mater. 37, e2410312–e2410313. doi:10.1002/adma.202410312
Won, P., Park, J. J., Lee, T., Ha, I., Han, S., Choi, M., et al. (2019). Stretchable and transparent kirigami conductor of nanowire percolation network for electronic skin applications. Nano Lett. 19, 6087–6096. doi:10.1021/acs.nanolett.9b02014
Wu, J., Wang, H., Su, Z., Zhang, M., Hu, X., Wang, Y., et al. (2017). Highly flexible and sensitive wearable E-skin based on graphite nanoplatelet and polyurethane nanocomposite films in mass industry production available. ACS Appl. Mater. Interfaces 9, 38745–38754. doi:10.1021/acsami.7b10316
Wu, S., Peng, S., Yu, Y., and Wang, C. H. (2020). Strategies for designing stretchable strain sensors and conductors. Adv. Mater. Technol. 5, 1–25. doi:10.1002/admt.201900908
Xiao, A., Jiang, X., Hu, Y., Li, H., Jiao, Y., Yin, D., et al. (2024). A degradable bioelectronic scaffold for localized cell transfection toward enhancing wound healing in a 3D space. Adv. Mater. 36, 24045344–e2404615. doi:10.1002/adma.202404534
Xiong, W., Zhu, C., Guo, D., Hou, C., Yang, Z., Xu, Z., et al. (2021). Bio-inspired, intelligent flexible sensing skin for multifunctional flying perception. Nano Energy 90, 106550. doi:10.1016/j.nanoen.2021.106550
Yang, R., Dutta, A., Li, B., Tiwari, N., Zhang, W., Niu, Z., et al. (2023). Iontronic pressure sensor with high sensitivity over ultra-broad linear range enabled by laser-induced gradient micro-pyramids. Nat. Commun. 14, 2907. doi:10.1038/s41467-023-38274-2
Yang, X., Chen, W., Fan, Q., Chen, J., Chen, Y., Lai, F., et al. (2024). Electronic skin for health monitoring systems: properties, functions, and applications. Adv. Mater. 36, 24025422–e2402628. doi:10.1002/adma.202402542
Yi, J., Zou, G., Huang, J., Ren, X., Tian, Q., Yu, Q., et al. (2023). Water-responsive supercontractile polymer films for bioelectronic interfaces. Nature 624, 295–302. doi:10.1038/s41586-023-06732-y
Zarei, M., Lee, G., Lee, S. G., and Cho, K. (2023). Advances in biodegradable electronic skin: material progress and recent applications in sensing, robotics, and human–machine interfaces. Adv. Mater. 35, 22031933–e2203236. doi:10.1002/adma.202203193
Zhang, C., Wu, M., Cao, S., Liu, M., Guo, D., Kang, Z., et al. (2023). Bioinspired environment-adaptable and ultrasensitive multifunctional electronic skin for human healthcare and robotic sensations. Small 19, e2304004–e2304012. doi:10.1002/smll.202304004
Zhang, Z., Yang, J., Wang, H., Wang, C., Gu, Y., Xu, Y., et al. (2024). A 10-micrometer-thick nanomesh-reinforced gas-permeable hydrogel skin sensor for long-term electrophysiological monitoring. Sci. Adv. 10, eadj5389–12. doi:10.1126/sciadv.adj5389
Zheng, Q., Lee, J. hun, Shen, X., Chen, X., and Kim, J. K. (2020). Graphene-based wearable piezoresistive physical sensors. Mater. Today 36, 158–179. doi:10.1016/j.mattod.2019.12.004
Zheng, X., Kamat, A. M., Cao, M., and Kottapalli, A. G. P. (2023). Wavy whiskers in wakes: explaining the trail-tracking capabilities of whisker arrays on seal muzzles. Adv. Sci. 10, 22030622–e2203121. doi:10.1002/advs.202203062
Zhi, C., Shi, S., Zhang, S., Si, Y., Yang, J., Meng, S., et al. (2023). Bioinspired all-fibrous directional moisture-wicking electronic skins for biomechanical energy harvesting and all-range health sensing. Nano-Micro Lett. 15, 60–17. doi:10.1007/s40820-023-01028-2
Zhou, S., Gao, X., Park, G., Yang, X., Qi, B., Lin, M., et al. (2024). Transcranial volumetric imaging using a conformal ultrasound patch. Nature 629, 810–818. doi:10.1038/s41586-024-07381-5
Keywords: flexible electronic skin, functional materials, engineering strategy, flow field decoding, sensitivity
Citation: Hu X, Guo S, Chen Y and Zhao F (2025) Functional materials-enabled flexible electronic skin for flow field decoding. Front. Electron. 6:1528802. doi: 10.3389/felec.2025.1528802
Received: 15 November 2024; Accepted: 21 March 2025;
Published: 04 April 2025.
Edited by:
Zhiqiang Ma, City University of Hong Kong, Hong Kong SAR, ChinaReviewed by:
Manmatha Mahato, Korea Advanced Institute of Science and Technology (KAIST), Republic of KoreaCopyright © 2025 Hu, Guo, Chen and Zhao. This is an open-access article distributed under the terms of the Creative Commons Attribution License (CC BY). The use, distribution or reproduction in other forums is permitted, provided the original author(s) and the copyright owner(s) are credited and that the original publication in this journal is cited, in accordance with accepted academic practice. No use, distribution or reproduction is permitted which does not comply with these terms.
*Correspondence: Xiaohe Hu, eGhodUBianR1LmVkdS5jbg==; Sheng Guo, c2hndW9AYmp0dS5lZHUuY24=
Disclaimer: All claims expressed in this article are solely those of the authors and do not necessarily represent those of their affiliated organizations, or those of the publisher, the editors and the reviewers. Any product that may be evaluated in this article or claim that may be made by its manufacturer is not guaranteed or endorsed by the publisher.
Research integrity at Frontiers
Learn more about the work of our research integrity team to safeguard the quality of each article we publish.