- School of Mechanical Engineering, University of Shanghai for Science and Technology, Shanghai, China
With the increasing demand in seamless interface between artificial devices and biological structures, flexible bioelectronics has been developed rapidly in recent years. Compared with traditional rigid bioelectronics, flexible devices are more adaptable to the integration for various parts both inside and outside of the organism. Significant achievements have been made in biomedical devices, neuroelectronics and wearable devices. With the development of flexible bioelectronics, electromagnetics is becoming a crucial part in signal interference reduction and information transmission or feedback, taking advantages of strong penetration and rapid response in a variety of biological materials. In this review, we focus on the latest developments in electromagnetic based flexible bioelectronics, involving materials, sensation, seamless integration, and power supply, as well as the latest achievements in the fields of external wearables, internal implants, soft robotics and drug delivery system. Based on these, the main challenges facing flexible bioelectronics, are analyzed, including stretchability caused by mismatch between mechanical properties of soft and hard components, biocompatibility, environmental stability, to facilitate the further development of flexible bioelectronics.
1 Introduction
Flexible bioelectronics is an important branch and future direction of bioelectronics, which mainly focuses on developing flexible and stretchable conductors to eliminate the mismatch between organisms and machines, couple flexible electrodes with biological tissues and provide better direction for the development and application of wearable devices, implantable devices and soft robots. Flexible wearable devices can achieve more flexible integration on the soft, curved or dynamically deformed skin or organisms, providing comfortable, portable and continuous health monitoring and treatment in a non-invasive and wireless manner (Wu et al., 2017; Gao et al., 2019). The biocompatibility of implantable flexible bioelectronic devices has been greatly improved, and with the development of integrated circuits, the size of components can reach the nanometer level, which provides great convenience for neural regulation and cell drug transport to achieve precise local treatment (Joshipura et al., 2017; Phillips et al., 2022). The performance of materials, sensors, communication, driving, power source, and integration technology can directly affect the overall performance of flexible bioelectronic devices. Among these components, electromagnetic technology has been a promising choice and can be widely applied in flexible bioelectronic devices.
Taking great advantages of high permeability, precise feedback and fast response, electromagnetic based flexible bioelectronics has been widely applied in biomedical field (Dagdevirren et al., 2017; Ye et al., 2023; Dolete et al., 2022; Zhang et al., 2021). It can be utilized to monitor various human physiological signals and biomolecules, including blood sugar, blood pressure, electrocardiogram, etc., providing doctors with real-time monitoring and timely treatment decisions; Furthermore, medical imaging such as magnetic resonance imaging (MRI) and magnetic resonance spectroscopy (MRS) can be performed using this technology to aid in disease diagnosis and treatment. Additionally, flexible bioelectronics can be applied to treatment and rehabilitation methods like magnetic field stimulation, nerve stimulation and physical therapy to help patients recover quickly. Lastly, flexible bioelectronics can also facilitate human-computer interactions such as brain-computer interfaces and motion control (Fu et al., 2022; Li et al., 2023; Wan et al., 2020), making it easier and more efficient for humans to interact with machines.
Here, we aim to introduce the latest advancements in flexible bioelectronics based on electromagnetic technology. In the following of the paper include:
- Section 2 describes the system composition of flexible bioelectronics and highlights the importance of electromagnetic technology;
- Section 3 discusses the key technologies in flexible bioelectronics fabrication, involving design method and material selection for soft integration and sensor fabrication techniques;
- Section 4 presents the current applications of flexible bioelectronics, including wearable devices, implantable devices, soft robots and drug delivery system;
- Section 5 discuss the challenges facing flexible bioelectronics based on electromagnetic technology;
- Section 6 summarizes the entire article and provide insight into the future development of this field.
2 Magnetism based flexible bioelectronic devices
2.1 Working principle
Electromagnetic based flexible bioelectronic devices typically consist of sensors, communications, driving devices and energy supply devices. According to different use scenarios, materials with different properties are used as substrates to integrate all required devices onto the substrate to achieve the required functions. As integration technology advances, the size of bioelectronic devices has decreased to the nanometer scale, allowing for the development of both external wearable devices and internal implantable devices. It can be found that integration efficiency improvement will accelerate the advancement of bioelectronics in various application areas. Working principle and applications of electromagnetic based flexible bioelectronics are shown in Figure 1.
2.2 System composition
The electromagnetic based flexible bioelectronic device comprises several key components, including bio integration, substrate, sensor, communication, driving device and energy supplying device. These components work in tandem to create a flexible electronic device that can be employed both in vivo and in vitro, performing a range of measurement and control tasks. The bio integration module is designed to interface with biological organisms and collect physiological data, while the substrate provides the structural framework for the device. The sensor detects changes in both environmental and physiological conditions, while the communication enables data transmission with external devices. The driving device controls the movements of the device, and the energy supplying device ensures the device has sufficient power to operate effectively. By working together seamlessly, these components create a highly versatile and functional flexible bioelectronic device. Figure 2 (Dai et al., 2019) demonstrates the basic components of flexible bioelectronics based on electromagnetism. Moreover, Table 1 presents the classification, characteristics and application scenarios of sensor, communication and driving device.
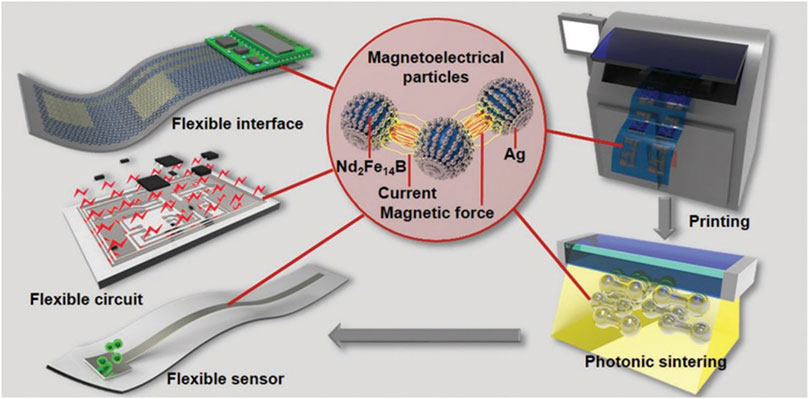
Figure 2. A schematic of electromagnetic based flexible bioelectronics (Dai et al., 2019).
2.2.1 Bio integration
Bio integration refers to wearable and implantable devices that detect physiological activities, perceive or regulate biochemical and metabolic processes, or deliver drugs (Kalantar-zadeh et al., 2017). These devices integrate sensors, communicators, driving devices and energy supply devices onto specific substrates according to different usage purposes and scenarios, in order to achieve the expected work in or out of the organism. Bio integrated systems are also providing inspiration for new sensing and driving mechanisms. For example, mechanoreceptors on the skin can transform pressure into exchange signals, which are received in the brain as tactile. Epidermal bioelectronic devices are very thin and can detect a series of physical and chemical signals. Bio integration and its various components complement each other, providing new ideas for the development direction of various devices, and the characteristics of each device also provide possibilities for the diversity of bio integration. In addition, the development of integrated circuits also plays a critical role in bio integration, with their small size, low power consumption and few solder joints being essential in chip design. With the development of integrated circuits to the nanometer level (Kesama et al., 2023), the volume of bioelectronic devices will also become smaller to a certain extent, which is more conducive to external wear and internal implantation. What’s more, higher integration efficiency facilitates the versatility of future bioelectronics, improving their utility in the medical industry.
2.2.2 Substrate
In general, the materials used in bioelectronic devices should be non-toxic, prevent direct harm to the body, and reduce the immune activity as much as possible. Biocompatibility is crucial, particularly for flexible bioelectronic devices, which require a substrate with good flexibility and extensibility to adapt to deformation on the organism. The substrate refers to a carrier or protective membrane, as most functional devices have poor biocompatibility in order to achieve their ideal functions, which would cause damage, inflammation, and tissue or organ damage if directly assembled and operated. Implantable devices face the issue of biological fouling. When the implantable device activates the biological immune system, macrophages and foreign giant cells will grow on the implantable device, inevitably disrupting the normal operation of the device (Sheikh et al., 2015). Therefore, when the substrate is used as a carrier or protective film, flexible substrates are made of flexible materials as a flexible substrate, which is separated from the organism through external encapsulation to achieve biocompatibility. Moreover, implantable devices require substrates with appropriate elastic modulus to achieve compatible bioelectronics, as different organs or parts of the organism have different softness. To realize the function and signal transmission, stretchable electronic components and substrates must have high flexibility, which means they must have appropriate elastic modulus (Ware et al., 2012). Therefore, the substrate type depends on the material properties, will be mentioned in the next section when introducing biomaterials.
2.2.3 Sensor
Bioelectronics relies on sensors for wearable and implantable devices. Currently, there are several commonly used types of sensors: capacitance sensors, piezoresistive sensors, piezoelectric sensors and electromagnetic sensors.
Capacitive sensors sense pressure and strain by adjusting the front area and parallel plate spacing, and use the capacitance changes caused by the deformation of pressure-sensitive mechanical components to change the separation gap of capacitors (Liu et al., 2021). Capacitance sensors have high sensitivity and can detect small changes in strain, commonly used in wearable electronics applications. A tactile pressure capacitance sensor made of carbon nanotubes can even sense the tactile sensation caused by insects staying on the surface (Lipomi et al., 2011).
Piezoresistive sensors achieve indirect detection of pressure and strain by converting external physical stimuli into changes in the conductive path between conductive materials. By utilizing changes in resistance, they can be easily achieved through electrical testing systems (Abolpour et al., 2020). Piezoresistive sensors are mainly used in biomedical applications such as cardiovascular, intracranial, and intraocular pressure measurements (Han et al., 2019; Kim et al., 2018; Park et al., 2019).
Piezoelectric sensors utilize piezoelectric materials with high sensitivity, fast response, and high voltage characteristics, such as P-based lanthanum-doped zirconate titanates (PZT) and polyvinylidene fluoride (PVDF) (Liu et al., 2021), to detect external stimuli. Piezoelectric sensors excel in biomechanical technology, measuring dynamics and kinematics for precise force measurement on body parts and surfaces (Pons et al., 2007). In medicine, they aid surface detection and are advancing for intrabody use, as seen in an implantable device monitoring vital signs and intestinal ingestion (Dagdeviren et al., 2017). Flexible bioelectronics leverage piezoelectric materials on stretchable substrates, creating sensors for curved surfaces and electronic tattoos for vibration sensing (Ha et al., 2019; Sun et al., 2019).
Electromagnetic sensors, though slower in development, show promise in bioelectronics for their simple structure, substantial output power, and stability. Electromagnetic sensors use the principle of electromagnetic induction to detect the position, shape, or motion status of target objects. When the target object approaches or moves away from the sensor, the magnetic field distribution changes, resulting in induced current or induced voltage. The generated current or voltage signals can be measured and analyzed to determine the position and motion status of the target object. Overcoming size limitations, especially in implantable devices, is crucial for their potential as the preferred choice in flexible bioelectronic devices. Compared with piezoelectric sensors, electromagnetic sensors have less impact on temperature changes and mechanical vibrations, and have the characteristics of high sensitivity and fast response, enabling high-precision measurements. Compared with ultrasonic sensing, electromagnetic sensors have the advantages of non-contact measurement, low requirements for the material and shape of the target object, and low interference with environmental noise. Therefore, considering all factors, electromagnetic sensors may be a more ideal choice in flexible bioelectronics.
2.2.4 Communication
With the rapid progress of technology, the demand for various new types of wireless communication is also increasing. Integrating them into polymers to assist in progressive detection and tasks in the medical field, and the communication capabilities of devices vary depending on their usage location. When dealing with tasks in the form of wireless communication, flexibility is a crucial feature that enables systems to adjust and adapt more flexibly to different communication needs and environmental conditions. There are two types of communication devices used in bioelectronics. One is wearable antenna, which is used to transmit and receive soft radio frequency when wearing; The other is the use of wireless devices for communication and information reception, and the significance of this application in medical technology is increasing. In addition to causing problems in processing information and collecting data, wired communication will also limit the scope of communication. Therefore, in the process of developing wireless communication in bioelectronics, general costs and high communication efficiency have been studied and created. With the overall development of bioelectronics towards flexibility, these communication devices are also developing from bulky models to flexible deformable models, in order to provide users with low costs and high comfort. Flexible bioelectronic devices can better fit biological curves, and flexibility enables wireless communication technology to be better integrated into bioelectronic devices, achieving more stable data transmission. In addition, flexible bioelectronic devices can adapt well to biological movements and morphological changes, thereby improving the reliability of wireless communication technology during movement. Due to the current vigorous development of wireless communication, a stretchable conductive elastomer has been created, which can not only provide wireless communication but also low-cost manufacturing, instead of using bulky wires (Chen et al., 2017).
2.2.5 Driving device
Flexible implantable bioelectronic devices rely heavily on effective driving devices that can move to designated positions after implantation, such as for drug transportation. Pneumatic actuators driven by pressurized air, exothermic reactions from pressure sources provided by combustion, biological hybrid drives, and electromagnetic drives are some of the most commonly used driving methods. To ensure the success of flexible electronic devices, soft driving methods are essential. Choosing the right driving device is crucial for achieving effective and accurate movement to the designated position.
Taking advantages of high response, high working density and large strain capacity (Manns et al., 2018; El-Atab et al., 2020), pneumatic actuators are usually used as the best method for biological robots to move and manufacture grasping devices (Mosadegh et al., 2014). However, for built-in pumps, high power input is required, and batteries with high power input cannot realize long-term independent operation, which greatly limits the application of this driving method in implantable devices.
A large amount of heat and pressure are generated by combustion, which is usually used in the driving of explosive robots (Bartlett et al., 2015). Soft robots can distribute the pressure generated by combustion to different pneumatic chambers through the logic board. However, if this driving is combined with bioelectronics, it is essential to ensure the safety of organisms in the combustion process and extend the service life of the system.
Thermal response driving remotely activates shape memory alloys or polymer materials by heating them through infrared, near-infrared, thermal radiation, and other methods (EI Atab et al., 2020). The driving is reversible and can restore the original shape, making it safer than ultraviolet or electric driving. However, its driving efficiency is often lower, and it is often used in scenarios such as micro actuators and micro pumps.
Driven by light stimulation response, it has wireless advantages and can be controlled even in small target sizes (Wei et al., 2012). Light responsive actuators can be classified based on the required spectrum, visible light, or near-infrared light for actuation (EI Atab et al., 2020). Soft robots that use visible light to stimulate actuators operate in natural environments without consuming excessive energy. Photo-responsive materials require high intensity light and exhibit simple and small deformations, leading to low motion speeds.
The electromagnetic drive is a relatively suitable driving mode at present. Among all the known soft robot driving stimuli, the magnetic field has unparalleled advantages in the ability to penetrate into various materials, and magnetized materials have relatively rapid changes in the magnetic field (Hines et al., 2016). Polymers, gels, papers, and fluids have been used as the medium to incorporate magnetic particles and fillers such that they can be actuated when an external magnetic field is applied. The insertion of discrete magnetic fillers into soft compounds results in a magnetization profile with variable magnitude and direction (Qiu et al., 2014). When an external magnetic field is applied, the magnetic particles align with the magnetic field, resulting in different modes of motion, such as bending, stretching, and twisting. An array of multiple electromagnets or moving permanent magnets can generate spatial gradients within a closed volume, which can be precisely controlled. Currently, the application of magnetic drive is extensive, such as using a magnetic field to drive soft robot for minimally invasive surgery and targeted drug transportation. What’s more, controlled magnetic fields can be applied to achieve precise programming and application of magnetic soft actuators.
Therefore, magnetic drive is a suitable drive mode for flexible implantable bioelectronic device, which can reduce interference and has high accuracy.
2.2.6 Energy supplying device
In addition to sensing and communication modes, the development of stretchable power supply has played a prominent role in manufacturing flexible bioelectronic devices. The energy supply components used in flexible electronic devices need to meet several conditions, including material compliance, mechanical durability under repeated loads, the ability to provide energy that meets the requirements for the device and safety issues. The commonly used energy collection components at present are based on piezoelectric, frictional, photovoltaic and thermal electric systems to collect energy. Piezoelectricity converts kinetic energy generated by the human body into electrical energy, while friction converts energy generated by friction into electrical energy. Photovoltaic cells convert light energy into electrical energy.
Compared with energy collectors, batteries and supercapacitors are the most widely used power supplies in bioelectronics. Batteries store energy through electrochemical processes, and some batteries can be designed flexibly to store large amounts of energy and have a long cycle life (Song et al., 2018). However, due to the unique structure of the battery, its mechanical properties are poor and cannot be easily stretched. Serpentine batteries overcome this problem and can be easily stretched, making them suitable for flexible electronic devices. While compared with batteries, supercapacitors can withstand millions of charging cycles and charge and discharge quickly. When discharged, they can produce huge energy bursts, and the cost of materials is low (Zhou et al., 2020). Many researchers have further optimized supercapacitors, such as Chen et al. (2014) developed a universal method for highly stretchable and conductive electrodes. High performance and stretchable linear supercapacitors are prepared by wrapping continuous carbon nanotube films around pre stretched elastic lines. Cao and Zhou’s team demonstrated a simple method for manufacturing supercapacitors on elastic substrates, which has a porous structure, short ion transfer time, low ion diffusion resistance, and can maintain superior electrochemical performance under large deformation of 800% area strain (Zhou et al., 2020). Therefore, for the application of flexible bioelectronics, the supercapacitor that can adapt to various deformation conditions is a good energy supply component.
2.3 Data collection system and machine learning
With the continuous progress of the Internet of Things (IoT) and information technology, bioelectronics has various applications in data collection systems, artificial intelligence, and machine learning.
Bioelectronics devices can collect biological signals (such as electroencephalography, electromyography, electrocardiogram, etc.) and transmit them to mobile phones or computer terminals for real-time health monitoring and other activities, to improve treatment effectiveness and reduce medical costs. The author of (Yousuf et al., 2023) developed a skin-inspired bimodal flexible and sensitive capacitive sensor, which could track the movement patterns precisely under different bending curvatures based on changes in capacitance, providing guidance in neurophysiology and kinematics for fields such as ergonomics and intelligent robots. The author of (Kim et al., 2020) proposed a wireless and continuous monitoring method for daily stress through bioelectronics devices. The device is worn on the inner wrist of the user’s hand and monitors three daily activities: office work, household chores, and meditation, verifying that both office work and household chores could bring pressure to users.
After collecting data from bioelectronic devices, combined with machine learning algorithms, it can be used to analyze biological signal data, classify gestures, signal types, disease features, and predict physical conditions.
The author of (Ban et al., 2023) designed a headband, soft, and wearable bioelectronics system for continuous monitoring of EOG signals, and trained and modeled EOG data through the machine learning interface, successfully classifying real-time eye movements (including blinking, up, down, left, and right). The author of (Kwon et al., 2020) designed a soft bioelectronic system using an additive nanomanufacturing technology for recording biological potentials on human skin. The device is mounted on the forearm to record EMG signals of different gestures (open hand, closed hand, index finger bend, and wrist bend), and a deep learning algorithm based on neural networks has been developed. The algorithm can recognize subtle emissivity patterns while considering amplitude, with an accuracy of up to 98.5% for hand posture classification.
3 Key technologies
In Chapter 3, we introduce key technologies for electromagnetic based flexible sensors, which typically include the following steps:
- Selection of substrate: Flexible substrate materials should be chosen to ensure the flexibility and bendability of the sensor;
- The selection of magnetic materials, such as magnetic particles, ferrite, etc;
- Composite methods: coating method, chemical deposition method, sputtering method;
- Patterned and structured methods: Use photolithography, 3D printing methods and moulds to customize specific surface textures or structures for sensors;
- Integration: Using technologies such as hot pressing and packaging to integrate and package sensors.
Section 3.1 introduces flexible magnetic materials, including flexible substrates and magnetic particles; Section 3.2 introduces the composite method of magnetic particles and flexible substrates, as well as how to pattern and structure them; Section 3.3 introduces the integration methods of electronic devices.
3.1 Magnetic soft materials
Magnetic soft materials are mainly composed of magnetic particles and flexible substrates. The magnetism of the material is determined by the size, mass, and concentration of the dispersed magnetic particles, while the mechanical properties of the material largely depend on the composition of flexible polymers. By combining different materials, magnetic soft materials with unique properties can be created, making them ideal for building soft and stretchable devices for various applications such as sensing monitoring, medical diagnosis, and drug transportation.
This section offers a comprehensive overview of the different types of magnetic particles and flexible substrates commonly used in magnetic soft materials, along with their mechanical and magnetized properties.
3.1.1 Magnetic particles
There are many types of magnetic particles, mainly including iron (Fe), cobalt (Co), nickel (Ni), neodymium iron boron (NdFeB), ferrite and iron oxide. The important magnetic properties of magnetic particles include saturation magnetization, remanence and coercivity, magnetic diameter, magnetocrystalline anisotropy constant and magnetic relaxation mechanism. Types and characteristics of magnetic materials are summarized in Table 2.
The magnetic properties of iron particles largely depend on their particle size and oxidation amount. The magnetization decreases with the decrease of particle size, which is related to the higher surface volume ratio of smaller particles, resulting in a much higher contribution of the surface oxide layer.
Fe2O3 magnetic particles have the characteristics of biocompatibility, strong superparamagnetism, low toxicity, etc. They are widely used because they can manipulate particle movement and provide imaging contrast in the presence of external magnetic fields.
As a kind of commonly used magnetic nanoparticles, Fe3O4 magnetic particles have stable chemical properties, high catalytic activity, good magnetic responsiveness and biocompatibility. They can reduce their particle size to several nanometers by changing reaction conditions, so they’re widely used in high magnetic recording materials, adsorbents, biosensors, magnetic resonance imaging and other fields (Zhao et al., 2008).
NdFeB permanent magnetic materials have excellent comprehensive hard magnetic properties such as high residual magnetization, high coercivity and high magnetic energy product. There are many chemical methods to synthesize NdFeB nanoparticles, including sol-gel method, spontaneous combustion method, microwave assisted combustion method, thermal decomposition method and mechanochemical method. The magnetic properties of NdFeB magnetic particles prepared by different chemical methods vary. The NdFeB nanoparticles prepared by the sol-gel method have massive and flaky grains gathered together, and the grain size and direction are uneven, which may lead to the formation of reverse magnetic chips and reduce the residual magnetization of the magnet (Meng et al., 2022); The coercivity of NdFeB nanoparticles synthesized by microwave assisted combustion process is 8.0 kOe, the saturation magnetization is 40 emu g-1, the saturation magnetization increases, and the energy product of 3.57 MGOe is obtained (Swaminathan et al., 2013). NdFeB nanoparticles were prepared by mechanochemical method, and the synthesized sample temperature was increased from 800°C to 900°C. The average size of NdFeB particles increased from 100 nm to 158 nm, and they exhibited a high coercivity of 21 kOe (Koylu-Alkan et al., 2016).
Barium ferrite is widely used in permanent magnetic materials because of its low cost, excellent chemical stability and corrosion resistance. In order to obtain high-performance barium ferrite, people are trying to obtain pure crystal BaFe12O19 single domain particles, and have developed different synthesis technologies, such as sol-gel technology, micro-emulsion, hydrothermal reaction, etc. The synthesis of barium hexaferrite powder by sol-gel combustion technology is a new method, which uniquely combines the chemical sol-gel process and the combustion process. The combustion process is based on gel and then burns the aqueous solution containing the required metal salts and some organic fuels to produce products with large volume, bulk and large surface area. The size and magnetic properties of microcrystals can be controlled according to demand (Li et al., 2009).
3.1.2 Flexible substrates
Bioelectronics are typically made from flexible substrates and encapsulated to protect nearby tissues (Chitrakar et al., 2022). Flexible substrates are soft and bendable material used as the base for bioelectronic devices, are the main source of flexibility and stretchability of electronic devices (Liu et al., 2018), allowing for better compatibility with biological tissues and achieving comfortable wearability, which are favored in the manufacturing of modern robotic systems due to their elasticity, resilience and conformation. The micro and macro structures determine its ultimate degree of viscoelasticity, which is a characteristic of materials that exhibit both viscous and elastic mechanical responses. Soft electronic devices must have Young’s modulus similar to biomaterials in order to achieve smooth interaction and seamless integration. The ideal substrate should exhibit Young’s modulus between 1 kPa and 100 MPa. This section lists three common substrates, namely silicone, polydimethylsiloxane, hydrogel and MXene (Phillips et al., 2022). Types and characteristics of flexible substrates are summarized in Table 3.
Silicone, a common substrate for bioelectronics, can have a modulus of about 130 GPa (Chitrakar et al., 2022). Silicone is polymeric siloxane with a wide variety of use, which is widely used in medical devices, implants, and household products. Medical grade silicones and silicone rubbers are bioinert, water resistant, and biocompatible. Low-density silicone can be given elasticity and flexibility through simple processing methods. Silicone can be used as a material for encapsulation. This material is most common for flexible and stretchable bioelectronics due to its diverse uses and easy fabrication method. Additionally, it can also be purchased commercially as Ecoflex, which has better stretchability and lower modulus, suitable for coating bioelectronic devices (Tao et al., 2016).
Polydimethylsiloxane (PDMS) is a silicone elastomer formed by siloxane bonds. Due to its high elasticity, biocompatibility, high dielectric strength, breathability, low chemical reactivity, biocompatibility and other characteristics (Phillips et al., 2022). PDMS has a stretchability of up to 1,000% (Liu et al., 2018). Its flexibility is determined by its Si–O siloxane backbone. The average Young’s modulus is ∼1–3 MPa. Super soft PDMS has a lower modulus of 0.1 kPa–2.3 MPa, which is favorable for soft tissues (Chitrakar et al., 2022). PDMS has been used in numerous fields of biological robots and bioelectronics. It is used to design high-sensitivity capacitive sensors for measuring pressure and oxygen content in heart and blood vessels, and to measure the interface pressure between nerve stem and cuff electrodes for monitoring the health of nerve tissue. PDMS polymers are also conducive to the fabrication of microfluidic channels in bioelectronic devices due to their optical transparency, flexibility and applicability to soft lithography.
Polyethylene terephthalate (PET) is an aromatic thermoplastic polyester commonly used as a substrate for flexible bioelectronic devices (Lee et al., 2014). PET has a polymeric backbone linked by ester bonds between aromatic group monomers, which gives it chemical stability and forms a stable semi-crystalline hydrophobic domain that resists biodegradation. Each aromatic ring and two adjacent aromatic rings in PET have a direct effect on the glass transition temperature (about 75–85°C), crystallinity and Young’s modulus (about 2∼4 GPa), respectively (Tonelli et al., 2002). As a thermoplastic, PET produces a film by a combination of extrusion and cold rolling to achieve the desired thickness. Though it cannot be stretched for its relatively high modulus, PET features good transparency (>85%), high creep resistance and excellent printability (Liu et al., 2018).
The biomedical use of polyurethane (PU), specifically ether based (Burke et al., 2004), has gained significant popularity in the 21st century and has emerged as a prevalent material for vascular grafts, owing to its exceptional tear and abrasion resistance. PU contains three components: a diisocyanate or triocyanate, a chain extender, and a macrodiol (Kanyanta et al., 2010). PU is highly tunable when mixed with other polymers. Pure PU has a Young’s modulus of ∼91 MPa and a stretchability of 290% (Shi et al., 2020). This elastomer has a superior flex-fatigue life and mechanical degradation resistance when compared to silicone elastomers. Additionally, its mechanical properties can be adjusted by altering the temperature and humidity, making it highly versatile. This further proves that PU is another organic material with multiple applications for flexible and stretchable bioelectronics (Ying et al., 2021).
Hydrogel is cross-linked polymer networks with high water content. Due to its similarity with biological tissues, it has been widely studied in tissue engineering and biomedical fields. It can serve as structural materials and active components, providing conductivity, sensing ability, and mechanical drive (Phillips et al., 2022). The flexible nature of hydrogel allows to minimize the mechanical mismatch with biological tissues. The high water content of hydrogels provides a humid and ion rich physiological environment. In addition, hydrogel has extraordinary flexibility in its electrical, mechanical and biological design, making it a unique bridge material in the biological world. Due to these special advantages, hydrogel has recently attracted more and more attention in the field of bioelectronics, which is part of the ongoing efforts to seamlessly connect biology and electronics.
MXene, as a new type of two-dimensional layered material, possesses hydrophilicity due to its rich functional groups on the surface, excellent metal conductivity, high capacitance, good biocompatibility and superior mechanical properties (Venkateshalu et al., 2020). Mainly used for biosensing, diagnostic imaging, cancer treatment, and nerve implantation.
Although neural tissues are highly sensitive to environmental conditions and interactions, MXene does not affect their biological activity. Neuronal tissue grows, forms a neural network, and adhere to MXene substrates, indicating that MXene can effectively interface with neural tissue. MXene coating implanted on metal electrodes can inhibit surface oxidation and provide a mechanically compatible neural interface between the implant and surrounding nerve tissue (Xu et al., 2016). In addition, MXene can easily form composites with other materials such as polymers, oxides and carbon nanotubes, which further provides an effective method to adjust the properties of MXene for various applications.
3.2 Fabrication technique
Section 3.2 introduces the composite methods of magnetic particles and flexible substrates, including coating method, chemical deposition method, and sputtering method; And methods of patterning and structuring, including the use of photolithography, 3D printing methods to moulds to customize specific surface textures or structures of sensors.
3.2.1 Composite methods
3.2.1.1 Coating method
Coating is the use of tools such as scrapers, brushes, or coating machines to evenly coat a liquid mixture of magnetic particles onto a flexible substrate surface. After coating, the particles are fixed on the substrate through heat treatment or drying, forming a magnetic composite flexible material.
Spin coating is a fast and common method for depositing thin films on substrates, which can easily form uniform films.
The author of (Zhu et al., 2023) prepared FeSiAl/WS2 soft magnetic composite materials, where WS2 was peeled off into micrometer sized thin sheets and uniformly coated on the surface of FeSiAl grains to form an efficient coating. The material has low eddy current loss and moderate effective magnetic permeability, making it suitable for high-frequency applications.
3.2.1.2 Chemical deposition method
Chemical deposition method usually deposits metals from a chemical solution onto a flexible substrate in vacuum, forming a thin film. This method can be used to deposit magnetic materials, such as metals or oxides, on flexible substrates. Chemical deposition methods have been used to deposit thin conductive materials onto various substrate materials.
The author of (Tang et al., 2018) studied flexible magnetic nanocomposite PDMA hydrogel by chemical precipitation method, immersed the prepared hydrogel in Fe2+/Fe3+solution to load ferrous and ferric ions, and then went through a series of post-treatment to obtain flexible hydrogel film with uniformly distributed magnetic nanoparticles.
3.2.1.3 Sputtering method
The sputtering method combines thin films by bombarding the surface of the target material with high-energy particles in a vacuum environment, thereby removing surface atoms or molecules of the target material and depositing them on the substrate. This technology can easily deposit different metals, metal alloys, and compounds (Rao et al., 2023).
The author of (Li R. et al., 2017) grew a 5 nm Ta layer on a pre stretched PDMS film using a magnetron sputtering system in a vacuum environment. After releasing the pre tension force, the Young’s modulus between the PDMS substrate and the metal layer did not match, resulting in surface wrinkles. By using sputtering method, a 150 nm thick FeCoTa film was tilted and sputtered onto a wrinkled surface, resulting in the final magnetic flexible film.
3.2.2 Patterned and structured methods
3.2.2.1 Photolithography
Photolithography is a commonly used micro and nano processing technology. The pattern on the chip is transferred to the substrate surface by photoresist and stepper, and then the required microstructure is prepared by etching and other processes. This approach is utilized to fabricate precise patterns ranging from nanometer to micrometer sizes on the underlying substrate. It has been extended to fabricate soft and stretchable electronics for biomedical applications, such as skin-mountable and wearable electronics. Photolithography, soft lithography, nanoimprint lithography, e-beam lithography, focused ion beam lithography, scanning probe lithography, etc., are some of the widely used lithography techniques (Chitrakar et al., 2022). Generally, the photolithography process comprises of several steps, such as photoresist application, prebake, mask alignment and exposure, development, post bake, etching, and resist stripping (Acikgoz et al., 2011). Soft photolithography allows for the utilization of elastic impressions, molds, or photomasks to create structures or transfer materials. Especially for using in flexible devices, process compatibility, device scale, and photolithography precision should be taken into account (Zschieschang et al., 2023).
The author of (Jumril et al., 2014) proposed a novel photosensitive magnetic film and a simple hybrid film fabrication process. The film is -made of soft and elastic material, capable of optical patterning and response to magnetic fields. Using a spin-on-glass (SOG) spin coating method, a sacrificial oxide layer was deposited on a glass substrate; the first layer of polymer (non-magnetic) serving as the actuator was deposited on the oxide film glass substrate to create the base film layer and increase the adhesion of the layer. A chamber was created on the silicon substrate. Photoresist was coated on the silicon substrate with a Si3N4 coating to create the chamber pattern, followed by photolithography. A silicon cavity is formed by anisotropic etching of silicon substrate using bulk micromachining process.
The author of (D’Imperio et al., 2018) described the fabrication and performance of optically transmitted 3D nanocylinders and nanocoaxes on independent, flexible films made of negative photoresist. The fabrication mainly consists of standard lithography techniques and the separation of the film from the substrate by a stripping technique. The chemical and mechanical stability of the polymer film and release layer enables them to produce microstructures through multilayer lithography and subsequent deposition of metal-insulator-metal layers, resulting in nanoscale coaxial columns. The robustness and flexibility of the sample are conducive to possible applications in wearable electronics and opto-bioelectronic interfaces.
The author of (Ko et al., 2017) constructed silver nanowires (AgNW) micropatterns on a variety of substrates in a simple, economical, and environmentally friendly way without the need for aggressive etching or stripping processes. AgNW patterns of different shapes and sizes were constructed on a glass substrate using polyethylene glycol (PEG) photolithography. Based on the second hydrogel gelation process, the AgNW pattern on the glass substrate is directly transferred to the synthetic/natural hydrogel substrate. The resulting AgNW micropattern exhibits high electrical conductivity (ca. 8.40 × 103 S cm−1), low sheet resistance (7.51 ± 1.11Ω sq−1), and excellent bending durability (resistance increases by only ∼3 and ∼13% after 40 and 160 bending cycles, respectively) on the hydrogel. And good stability under wet conditions (resistance increases by only ∼6% after 4 h). The AgNW micropatterned hydrogels will serve as highly efficient and mechanically stable microelectrodes for the development of next-generation bioelectronic devices, particularly implantable biomedical devices.
3.2.2.2 3D printing methods
Another method for patterning and structuring is to use 3D printing technology to deposit conductive and resistive materials directly on the surface of mechanical flexible polymers, paper and silk. High speed inkjet printing is very accurate and can produce high-resolution features. Moreover, inkjet printing consumes little material and eliminates the need for environmentally harmful photoresist chemicals used in lithography (Sinar et al., 2013).
Fused Deposition Modeling (FDM) is the most common 3D printing technology at present, which extrudes thermoplastic polymer filament through heating nozzle. The resolution depends on the size of the print nozzle. The author of (Zhou et al., 2023) used fused deposition modeling 3D printing technology to fabricate flexible multifunctional sensors. Polyvinylidene fluoride and cobalt ferrite (CoFe2O4) were used to prepare (0–3) composite silk. These filaments can be prepared into various shapes and have good mechanical and electrical properties. It has reference value for the future development of personalized wearable sensors. The author of (Nelson et al., 2019) used thermoplastic polyurethane (TPU) to create transparent, flexible, and biocompatible microfluidic devices with channel sizes consistently below 100 μm and as small as 40 μm through fusion deposition modeling 3D printing. The channels consistently print out about 100 μm smaller than the design size, but are repeatable and predictable. They demonstrate that TPU has properties that can be used in microfluidic applications while remaining cost effective (about $0.01 per device) and best suited for rapid prototyping (fabrication time <25 min).
Direct Ink Write (DIW)is also a common additive manufacturing method, which extrudes pressurized liquid polymer precursors through nozzles. After extrusion, continuous or intermittent stimulation will cure the material. When exposure or heating is used to promote crosslinking, DIW can be used to prepare hydrogels, allowing anisotropic binding into the resulting hydrogel structure. Multiple nozzles can be used for printing at the same time to produce objects with multiple materials. Like FDM, its print resolution is limited by nozzle size. The disadvantage is that the low viscosity of its ink does not allow the printing of hollow or suspended structures, and it needs to sacrifice materials as support in the printing process. The fabrication of flexible metal circuits is investigated using polymer/metal precursor ink and interfacial reaction direct writing technology (Sun et al., 2023). PVA and AgNO3 are thoroughly mixed as polymer/metal precursors. PVA acts as an adsorption reducing agent and adhesion layer in the metal circuit, serving as a flexible composite material to connect the flexible metal circuit with the substrate. AgNO3 is added to the ink as a metal source. Through controlled REDOX reaction at the interface of the nano direct writing structure and the reducing agent VC aqueous solution, a uniform layer of AgNP conductive particles is formed on the EVA skeleton, creating a patterned conductive path on the flexible substrate. The resistivity of the composite silver layer can reach 10−6 Ω·m without any post-processing. Additionally, after 10,000 cycles of 180° bending, the resistance change is maintained within 20%. Direct writing technology enables the easy production of patterned flexible metal circuits with good electrical conductivity and flexibility. MXene inks are promising candidates for manufacturing conductive circuits and flexible devices. The author of (Kong et al., 2022) utilized MXene ink to write electronic circuits on flexible substrates. This circuit exhibits a fast and accurate capacitive response in touchpads and water level measurements, demonstrating the outstanding potential of these MXene inks in electrical equipment manufacturing.
Stereo Lithography Apparatus (SLA), a series of polymerization technologies, involves the polymerization of objects on the surface of liquid prepolymers. Using photopolymerization, it allows the formation of thin features and geometric shapes in the resin. It is the most useful for manufacturing hard components and rarely used in soft robots (Phillips et al., 2022). The author of (Heo et al., 2019) reported the first 3D printable conductive hydrogel that can be photocrosslinked while maintaining high conductivity. In addition, they prepared a gelatin methacryloyl (GelMA) hydrogel encapsulated in dorsal root ganglion (DRG) cells and pattered the conductive hydrogel onto the substrate by using a desktop SLA 3D printer. The 3D-printed conductive hydrogel provides excellent structural support for the systematic transfer of ES to encapsulated DRG cells to enhance neuronal differentiation. The results show that conductive hydrogels can be used as 3D printing materials for electrical applications, and hydrogel-based electronic devices are considered promising for biological signal recording and stimulation of living tissues.
Selective Laser Sintering (SLS) builds objects by selectively melting powder particles and then fusing them together. The substrate particles are sintered layer by layer until the structure is completed. The print resolution is determined by the size of the particles, and no support structure is required. The author of (Ding et al., 2022) employed an environmentally friendly method to prepare a novel mixed powder composed of laboratory-prepared thermoplastic polyether block amide (TPAE) and multi-walled carbon nanotubes (MWCNTs) for SLS 3D printing, and established a carbon nanotube conductive network structure in a SLS-treated nanocomposite device. In compression tests with different deformation strains and speeds, the flexible sensor machined by SLS shows a regular, fast, sensitive and stable cyclic response, showing its great potential in artificial electronic skin, human-machine interface and health monitoring.
3.2.2.3 Fabrication with moulds
Using moulds to manufacture flexible structures is a simple and effective method. Flexible materials such as polymers, silicone, or other elastic materials are injected into the mould and cured before being physically separated to control the shape and structure of the material. The mould can be reused for multiple production runs. This manufacturing method allows for the creation of a variety of flexible structure objects, such as flexible substrates and soft robots (Wang et al., 2021; Lin et al., 2023), by adjusting the shape and structure of the mould. The resulting objects possess the desired shape and size, while also maintaining their softness and elasticity to adapt to various application scenarios.
Inspired by the movement of earthworms through contraction and stretching between body segments, the author of (Lin et al., 2023) proposed an integrated mould-folding diaphragm consisting of a body segment structure with radially magnetized properties to achieve large 3D and bidirectional deformations subject to the internal volume variation capacity of a low uniform magnetic drive field (40 mT). In addition, the appearance of the magnetically driven folding diaphragm based on the proposed magnetic drive can be easily customized to the desired appearance and then implanted as a soft drive in different robotic systems.
The author of (Wang et al., 2018) developed an ultrafast response precisely controllable soft electromagnet actuator based on Ecoflex rubber film filled with NdFeB. The actuator can be used to prepare grippers of different sizes and shapes according to customer requirements, so as to realize the grasping and gripping function of spherical and cylindrical objects. By optimizing the matrix and size of the driver, it shows the advantages of ultra-fast response speed (<0.1 s), large elongation (<120%), good working stability, high working accuracy for a long time, and customizable design.
3.3 Integrated methods
3.3.1 Hot pressing
Hot pressing, as a simple and cost-effective method of preparation, can combine flexible base materials with electronic components and conductive materials to form the structure of flexible electronic devices by controlling temperature and pressure. This method enables high-precision machining while maintaining the flexibility of the material, and can also be used to process polymers and their composites to achieve improved physical properties (Liu et al., 2022), making it ideal for manufacturing flexible electronic devices. By using hot pressing, various types of flexible bioelectronic devices such as flexible substrates and flexible sensors can be manufactured, which provides new possibilities for lightweight and flexible bioelectronic products. Therefore, hot pressing technology has an important application prospect in the manufacture of flexible bioelectronic devices.
The author of (Liu et al., 2022) studies the impact of hot pressing on the electrical and thermal performance of thick conducting polymer poly (3,4-ethylene dioxythiophene):poly (styrene sulfonate) (PEDOT:PSS) films after acid treatment. The in-plane electrical conductivity of thick hot-pressed PEDOT:PSS film exceeded 1500 S/cm, which is 50% higher than that of non-hot-pressed counterparts. Its in-plane thermal conductivity reached as high as 1.11 W/mK. Such electrical and thermal enhancement via the hot-pressing process is attributed to the optimized morphology and microstructures, which provide short paths for thermal and electrical transportation. Therefore, the hot-pressed film can be used as a flexible conductor and heat sink respectively for flexible electronics and thermal management.
The author of (Cataldi et al., 2015) designed and manufactured a material that utilizes a combination of all biodegradable components (substrate and polymer matrix) and graphene nanosheets. The configured ink is sprayed on a pure cellulose sheet, which is hot pressed into a fiber network after drying. The resulting nanostructured flexible composite exhibits excellent isotropic conductivity, reaching a very low thin-layer resistance value of ≈10Ω sq-1, depending on the relative concentration between the biopolymer and the graphene nanosheets. By hot pressing, graphene nanosheets are embedded into cellulose fibers, resulting in flexible composites with high electrical conductivity. Unlike the conductive paper technology, the proposed paper-like flexible conductor exhibits bilateral isotropic conductivity due to pressure-induced impregnation.
3.3.2 Packaging
Packaging is the final step in the integration process of flexible electromagnetic biosensors, aimed at protecting critical components of the equipment. Generally, materials with flexibility and chemical corrosion resistance are used to protect biosensing materials from external factors such as pollution, oxidation, and damage, thereby ensuring the sensitivity, reliability, and stability of sensors and extending their service life.
4 Application
This section introduces the application fields of flexible bioelectronics, mainly from four directions, namely wearable devices, implantable devices, soft robots and drug delivery system.
4.1 Wearable devices
The author of (Wan et al., 2020) developed a flexible hybrid electromagnetic triboelectric nanogenerator composed of polydimethylsiloxane (PDMS), multi-walled carbon nanotubes (MWCNTs) and NdFeB particles. The magnetic and conductive polydimethylsiloxane (MC-PDMS) has certain flexibility, which can be adhered to rough fabrics and human skin through tape, or even sewn on cloth. It can not only be used as a flexible magnetic polymer of EMG to provide electromagnetic induction in the copper coil, but also as an electrode of TENG to conduct friction electricity. In addition, the MC-PDMS material can be used for self-powered 3D trajectory sensing, which involves high information detection capability above the coil array. The device has great application potential in wearable electronics and man-machine fields. Structure schematic of the hybridized electromagnetic-triboelectric nanogenerator is shown in Figure 3A. And Figure 3B illustrates the working principle of TENG. When Kapton comes into contact with MC-PDMS, due to the difference in electronegativity, Kapton is positively charged while MC-PDMS is negatively charged. When Kapton slides outward, it generates a positive current signal; Stop output when fully sliding out. When Kapton slides backwards, it generates a negative current signal; Contact again to stop output. Through this cycle, stable current is generated from Kapton motion.
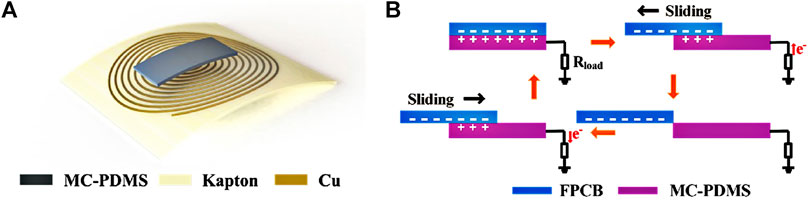
Figure 3. (A) Structure schematic of the hybridized electromagnetic-triboelectric nanogenerator, and (B) Schematic diagram of working principle of TENG.
The author of (Li et al., 2023) invented a flexible wearable device that can be combined with injected Fe3O4 nanoparticles to capture circulating tumor cells and thus block metastasis. The flexible device contains origami-like magnetic membranes that attract Fe3O4@Au nanoparticles (NPs) that are surface modified with specific aptamers, these NPs are injected intravenously into the blood vessels, Decoy devices are formed to capture circulating tumor cells. The death of circulating tumor cells is triggered by light energy from the flexible AlGaAs LEDs in the device. After 10 cycles, the device showed a capture efficiency of 72.31%. The convergence of nanomaterials and flexible electronics reveals an emerging field that uses wearable and flexible stimulators to activate the biological effects of nanomaterials to improve the therapeutic efficacy and postoperative outcomes of diseases. A schematic of a flexible wearable device capturing circulating tumor cells and triggering death is shown in Figure 4.
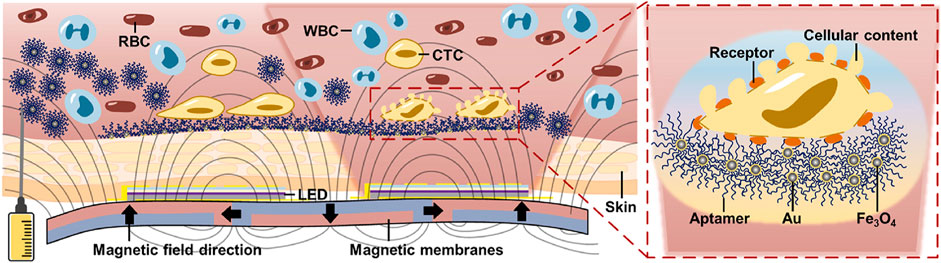
Figure 4. A schematic of the mechanism by which a wearable device captures and triggers the death of circulating tumor cells.
The author of (Fu et al., 2022) proposed a lightweight polymer porous sponge capable of detecting external magnetic fields and strain excitation. The sponge is composed of sodium alginate/chitosan, micron carbonyl iron and nanoscale Fe3O4 magnetic particles. It has a dual network structure. It shows superior mechanical strength and hydrophilicity. The sponge sensor shows significant electrical response to external magnetic fields and mechanical stimulation, while maintaining relatively stable recoverability and repeatability. Its sensing properties are highly dependent on the microstructure changes of magnetic particles and conductive multi-walled carbon nanotube networks. This sensor shows high potential in wearable multi-sensor electronics and intelligent transportation devices. Preparation process of porous sponge and the ΔR/R0 plot of the sensor attached to the wrist at different bending angles are shown in Figure 5.
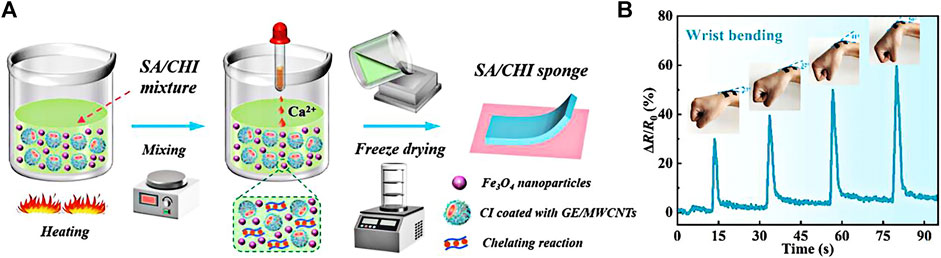
Figure 5. (A) Fabrication procedures of the porous sponge, and (B) The ΔR/R0 plot of the sensor attached to the wrist at different bending angles.
The author of (Pan et al., 2023) proposed a flexible magnetic field sensor with PZT/CFO double-layer structure that can be integrated on wearable devices. The sensor was used on a mica substrate via van der Waals oxide heteroepitaxy, which reduced the substrate clamping effect and demonstrated a high electromagnetic response. The flexible sensor has high sensitivity and stable response to AC and DC weak magnetic fields. With the increase of bending degree, its electromagnetic output and detection limit tend to decrease. The flexible electromagnetic sensor can be integrated into wearable devices and significantly broadens the potential applications of flexible magnetic field sensors.
4.2 Implantable devices
The author of (Zhang et al., 2021) proposed a flexible implantable polyimide catheter device for targeted treatment of cardiovascular diseases by aggregating magnetic nanoparticles (as shown in Figure 6A). Specific locations can be reached, such as narrow blood vessels and complex joint locations. Accurate treatment and its effective, controlled and sustainable drug supplementation are achieved through repeated injections of nanoparticles. The magnetic field generated by the Cu micro coils fabricated on the PI surface can precisely control the nanoparticles. In addition, the selected catheter has excellent flexibility and biocompatibility, and the manufactured device exhibits good biocompatibility in the carotid artery, with only mild biological immune response in implanted devices in rats. This technology has significant implications for cardiovascular treatment strategies.
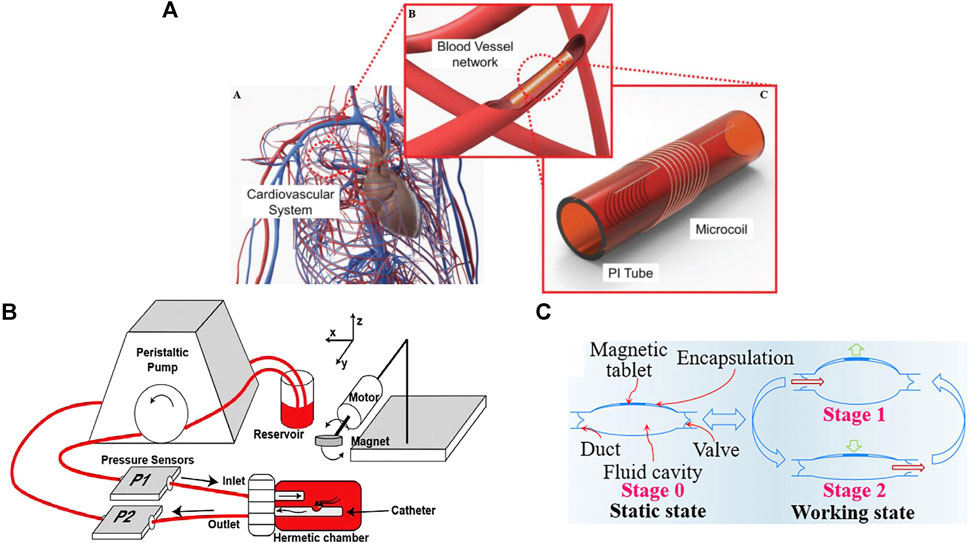
Figure 6. Schematic of Implantable devices. (a) Schematic of flexible implantable catheter device and application scenario. (A) Complex cardiovascular system; (B) Prefabricated devices placed in blood vessels; (C) Schematic diagram of a device manufactured on the surface of a flexible conduit. (b) Schematic of the bench-top blood circulation and magnetic actuation setup; (c) Working principle: The internal electromagnet is driven by an alternating magnetic field and discharge the ascites from the pump chamber.
The author of (Yang et al., 2022) developed a magnetically actuated self-clearing catheter that uses magnetic micro actuated smart catheter (as shown in Figure 6B), IVH is treated in situ by dissolving the obstructive thrombus through an externally applied magnetic field. It can speed up the expulsion of blood from the ventricles, maintain lower ventricular volume, and improve the survival rate of patients with IVH. The catheter is seven times longer than conventional catheters and has a higher survival rate (86%) after treatment, playing a vital role in neurology, urology, cardiovascular and application.
The author of (Wang et al., 2023), inspired by lymph nodes, proposed a soft artificial ascites metastasis system with good biocompatibility. The implantable system consists of a pump chamber, valves, and pipelines, which are made of soft and flexible silicone, making them adaptable to surrounding tissues. The chamber is equipped with a magnetic plate that is driven by an external magnetic field, as shown in Figure 6C, which is the working principle of the system. Subsequently, the system can expel ascites at a maximum speed of 23 mL min-1, much higher than the natural lymphatic system and existing technology equipment. The liver function of the experimental subjects was improved, and there were no serious complications within 4 weeks, proving the safety and effectiveness of the treatment. This artificial lymphatic system paves the way for further clinical research.
4.3 Soft robots
The author of (Reghu et al., 2020) developed a high-performance artificial insect millirobot driven by laser and magnetic field, which is based on an insect like functional nanocomposite material based on magnetite, zeolitic imidazolate framework-8, and polytetrafluoroethylene. The millirobot has the characteristics of effective CO2 gas release, strong magnetic properties and strong light and heat, and uses near-infrared laser and magnets to anesthetize drosophila in a non-contact manner, and spatially and spatially control the release of matrix from nanocomposites at the desired position on the water surface. Photograph of a prepared MAG–ZIF-8–PTFE nanocomposite disk is shown in Figure 7.
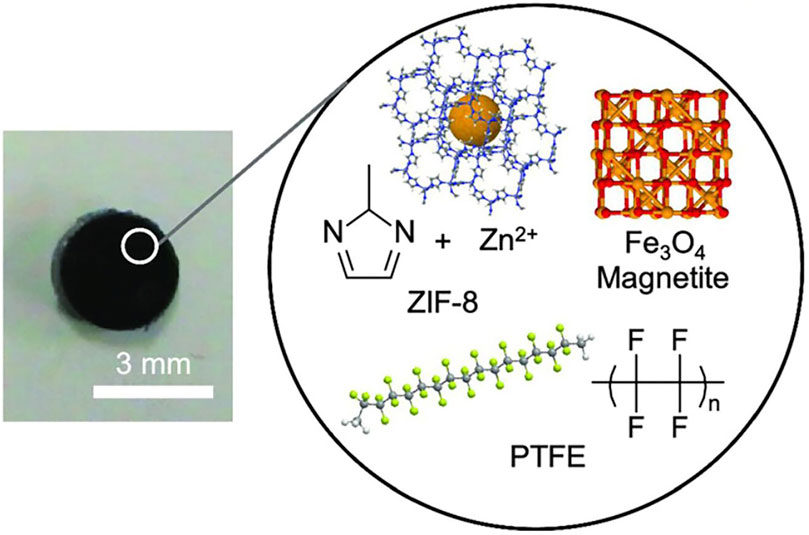
Figure 7. Photograph of a prepared MAG–ZIF-8–PTFE nanocomposite disk (left) and a schematic illustration of chemicals in the nanocomposite (right).
The author of (Lin et al., 2023) proposed bio-inspired magnetic-driven folded diaphragm for biomimetic robot. The magnetic film is composed of the structure of body segments with radial magnetization property, to achieve deformation with inside-volume change capability subjected to the low homogeneous magnetically driving field (40 mT). The bio-earthworm crawling robot and bio-squid swimming robot are designed using this integrated mold folding magnetic film (as shown in Figure 8). The former can achieve crawling, turning, and climbing over triangular slopes under a single uniform magnetic field, with a speed of 30 mm s-1. Its crawling speed can be controlled by adjusting the strength and frequency of the magnetic field. The latter can achieve swimming movements such as snorkeling, vertical diving, and horizontal diving at a speed of 24 mm/s under a magnetic field with a frequency of 2 Hz.
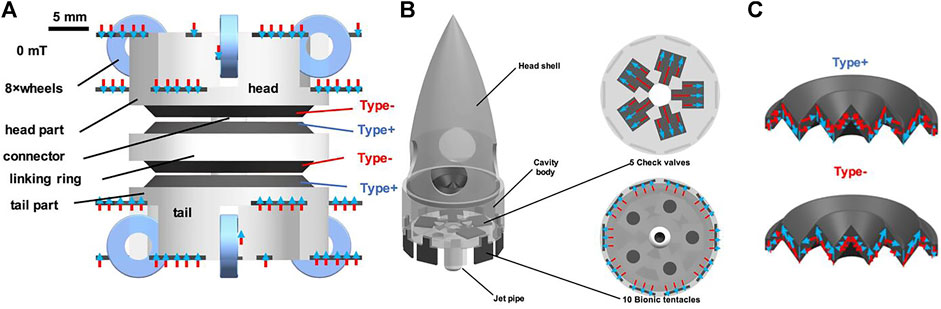
Figure 8. (A) Schematic diagram of the single-section crawling robot; (B) Schematic diagram of a bionic squid swimming robot; (C) magnetization characteristics of crawling robot component and swimming robot component.
The author of (Sun et al., 2022) developed a magnetic soft millimeter robot with joint structure by 3D printing hydrogel. The joint structure can transform bending deformation into folding deformation, leading to local deformation in the joint area. Unlike uniform bending deformation, this local deformation allows the robot to move more, while reducing the overall energy consumption. Experiments and numerical simulations show that the magnetic arthropod micro robot can perform multi-mode motion and programmed shape transformation, such as moving, flipping, capturing, carrying and releasing. The robot has been successfully tested in pig organs, including the aorta, stomach, and intestine, for foreign body removal, showing potential application in surgery. Fabrication, magnetization, and actuation of bioinspired magnetic arthropod millirobots made by magnetic hydrogels are illustrated in Figure 9.
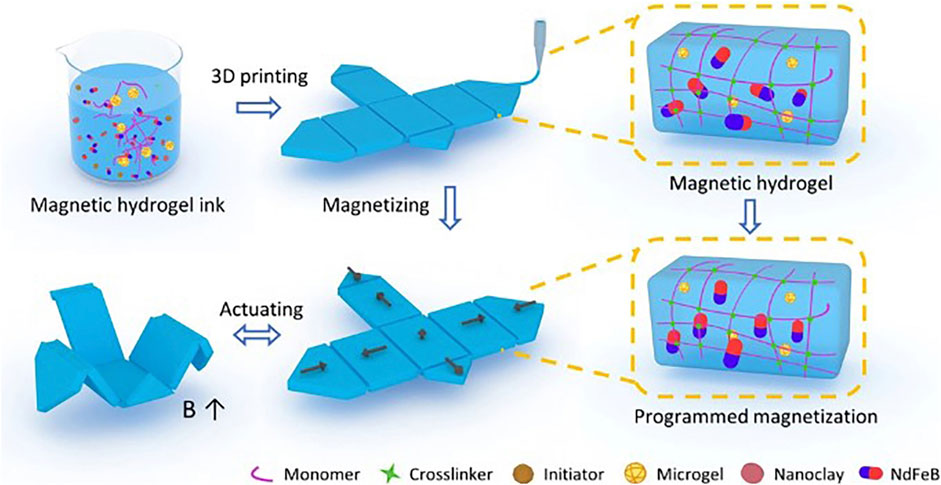
Figure 9. Fabrication, magnetization, and actuation of bioinspired magnetic arthropod millirobots made by magnetic hydrogels.
The author of (Joyee et al., 2020) proposed an untethered fully 3D printed soft robot capable of multi-modal locomotion. The robot’s main body structure is made of magnetic particle polymer composites, which can be 3D printed with multiple materials. The robot contains two functional parts, anterior and posterior legs, with embedded magnetic materials. It has three degrees of freedom and can bend bi-directionally in the XY plane and Z direction. In addition, the robot integrates a magnetically controlled drug carrier reservoir in its front leg, which can store liquid drugs and release them after reaching the target. The drug delivery process was simulated in both a human stomach and lung model. This soft robot has immense potential in drug delivery applications and can navigate through narrow and complex pathways to deliver drugs precisely to the target. The process of integrating drug reservoir into robot anterior leg is illustrated in Figure 10.
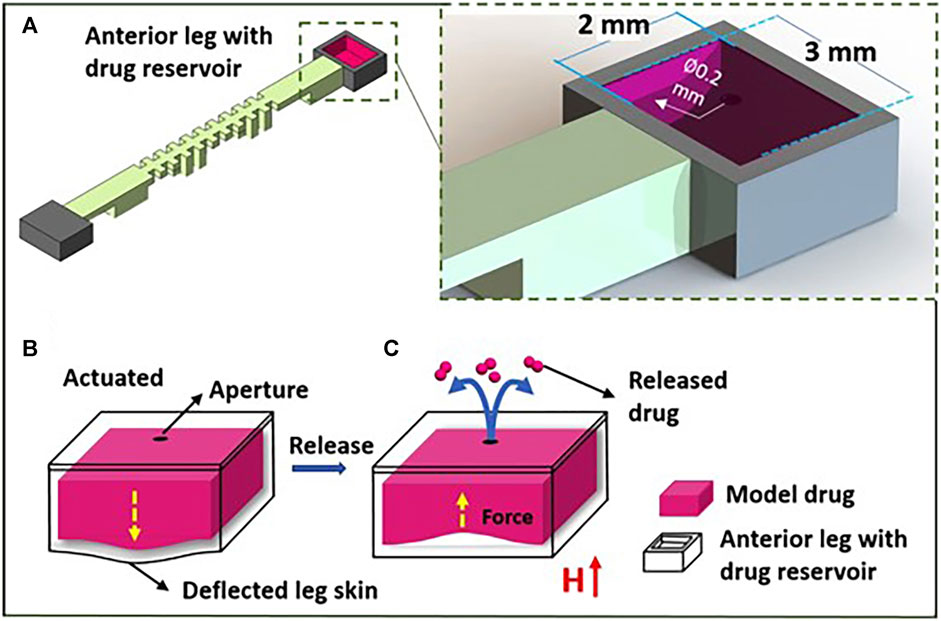
Figure 10. The process of integrating drug reservoir into robot anterior leg. (A) Design of drug reservoir in the anterior leg; (B) Magnetic field from the anterior magnet creating deflection of the leg skin towards the magnet; (C) Drug released through the small aperture after the anterior magnetic field is turned off.
4.4 Drug delivery system
The author of (Ye et al., 2023) developed a magnetic microrobots with folate targeting (as shown in Figure 11A), which can be used for cancer treatment. The researchers used the hydrogel network of biodegradable gelatin methacryloyl (GeIMA) and the porous structure of the magnetic metal-organic framework (MOF) to load enough anticancer folic acid (FA) and anticancer drug doxorubicin (DOX) respectively, so that the magnetic navigation targeting robot can successfully navigate around the lesion site through the magnetic field. Under the constant temperature of 37°C, the release of DOX gradually increased in the first 96 h, and then the release rate began to slow down. The magnetic microrobot has the body length of 140 μm and the screw diameter of 50 μm. With the control of a rotating magnetic field (20mT, 2 Hz), the microrobot can flexibly reach the narrow space between cancer cells and achieve precise positioning. What’s more, the inhibitory rate of FA containing microrobot on cancer cells can reach 93%. It has the characteristics of good biocompatibility, long internal circulation time, high magnetic response sensitivity, and stable properties.
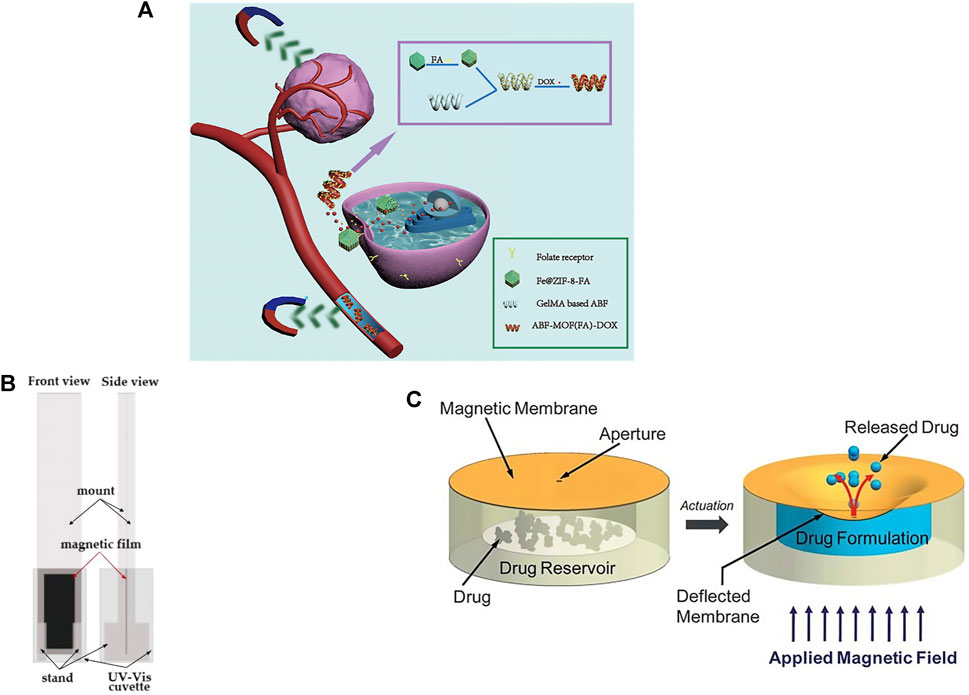
Figure 11. Schematic of the drug delivery system. (A) The illustration of magnetically controlled microrobots for folate-targeted cancer therapy: A folatetargeting magnetic microrobot system that consists of biodegradable GelMAbased ABF microhelix and FAloaded Fe@ZIF8 nanoparticles was developed, for which therapeutic drugs can be loaded into the hydrogel net work of the microrobots for cancer therapy; (B) Schematic diagram of drug delivery micropump triggered by magneto mechanical triggering: The film was fixed inside the mounts so that a constant pressure was applied on all four sides of the films, while exposure to the release medium was achieved through the slot. The total width of the mount was designed so that it fitted a 1 cm quartz cell in order to directly assess UV-Vis measurements for the release environment; (C) Schematic illustration of the MEMS drug delivery device and its operation: the device consists of a drug-loaded micro reservoir, sealed by an elastic magnetic PDMS membrane with a laser-drilled aperture. Once the device is actuated in an external magnetic field, the magnetic membrane deforms and discharges the drug solution out of the reservoir.
The author of (Dolete et al., 2022) developed a drug delivery micropump based on magnetic alginate film (as shown in Figure 11B), which drives the deformation of magnetic film through external magnetic field. Which triggers the release of the drug. Sodium alginate was chosen as the polymer matrix because the structure of sodium alginate allows for the binding of different types of molecules. Moreover, magnetite nanoparticles (Fe3O4) and sodium alginate both have excellent biocompatibility. Therefore, magnetite nanoparticles are incorporated into an sodium alginate matrix to prepare a magnetic film. Under the action of alternating magnetic field, the micro-pump can achieve 72.91 ± 3.99% drug release within 60 min, and can control the dose of drug directly released to the site of action.
The author of (Pirmoradi et al., 2011) investigated micropumps based on a magnetic membrane provided with a centered laser-drilled aperture that could trigger drug release from a reservoir in the presence of a magnetic field (as shown in Figure 11C). And the micropump is mainly prepared from biocompatible PDMS materials. The concentration of its drug release can be controlled by the strength of the magnetic field, and the desired dose can be achieved through repeated actuating cycles. According to their studies, 3.4 ng of docetaxel could be released every minute in the presence of a 0.25 T magnetic field. Taxane for the treatment of proliferative retinopathy.
5 Challenges
This section discusses some of the challenges facing the current electromagnetic based flexible bioelectronics, including biological integration, material selection, power supply and packaging, sensing and control, etc.
5.1 Bio integration
The first is bio integration. The electromagnetic based flexible bioelectronics are mainly composed of sensor, communication, driving device and energy supplying device. In application scenarios such as medical monitoring and invasive surgery, flexible bioelectronic devices need to have the function of long-term or continuous monitoring. In addition, they should be tested in an environment free of electromagnetic interference to accurately transmit signals to the monitor and provide corresponding treatment methods for patients.
The integration of flexible bioelectronic devices is an unresolved issue. Different from traditional rigid electronic devices, flexible bioelectronic devices have low modulus and high flexibility, which will lead to poor connection of the interface between the sensor and the circuit. Therefore, more efforts are needed to ensure the mechanical stability and performance uniformity of flexible bioelectronic devices (Li et al., 2020).
In addition, these devices usually need to be miniaturized, and while reducing size, some integrated components need to be reduced or eliminated, which creates some limitations in the printing, driving, control and transportation of robots to the workplace. Therefore, adopting appropriate manufacturing technologies is crucial (Eshaghi et al., 2021).
5.2 Material selection
Flexible bioelectronic devices need to be compatible with living organisms to avoid causing immune reactions or tissue damage. However, there are still some issues with flexible bioelectronic materials and manufacturing processes. Some materials may cause allergic reactions or cytotoxicity.
Good chemical stability and magnetic responsiveness shall be ensured for magnetic particles; Flexible polymer materials should: 1) Require soft and stretchable materials; 2) Biocompatibility and non-toxicity; 3) Recoverability. In addition, the arrangement and distribution of magnetic particles within flexible polymers also have an impact on the performance of electronic devices.
How magnetic particles are evenly distributed in a flexible substrate is also a problem, and uneven distribution can affect magnetic properties and work stability; In addition, how to repeatedly produce flexible films with uniformly distributed magnetic particles in bulk is also a concern for researchers.
In addition, flexible bioelectronic devices need to be able to adapt to various bending and twisting deformations, and must be able to withstand long-term mechanical stress and wear. Therefore, the selected materials should have sufficient stability and reliability to ensure their long-term use.
For example, although hydrogels have many excellent properties, there are still many challenges and unmet needs. When hydrogel loses water or freezes at low temperature, it will become hard and brittle, without biocompatibility and flexibility, or unable to maintain conductivity. Secondly, the weak strength and toughness of hydrogels need to be improved (Li Y. et al., 2021).
5.3 Power and packaging
Then there is the power issue. The power supply used in flexible electronic devices should be stretchable, sustainable, self-healing, green, and low-carbon.
If electronic devices are implanted into organisms, the challenge of recharging the power supply needs to be considered. Therefore, more efficient energy management technologies are needed to extend battery life or achieve energy recovery. Flexible bioelectronic devices typically require power supply through wireless energy transmission, but current wireless energy transmission technologies still face some challenges in terms of distance, efficiency, and reliability. In addition, if magnetic drive is used, it can effectively solve the power problem, but it is necessary to consider issues such as the applied magnetic field strength and magnetization method. We also need to consider how to design small-sized, high-performance, and low-power electromagnetic devices that can work in complex and tiny organisms.
What’s more, it is also necessary to consider the long-term stability of flexible biosensors in actual working environments. Due to the environmental factors encountered by implantable electronic devices in daily activities, such as electromagnetic interference, multiple signal cross interferenc, humidity, sweat and water infiltration, or the need to operate in the environment of biological fluids, further consideration should be given to the chemical and environmental reliability of human interaction systems, as well as biocompatibility packaging technology when designing systems. The packaging layer is indispensable for ensuring the functional stability of the device (Pyo et al., 2021). The volume of its equipment should also be as small as possible, so dense filling materials can be used to make encapsulation, including polymer and inorganic materials (Hines et al., 2016).
5.4 Sensing and control
Flexible bioelectronic devices need reliable sensors and control systems to accurately collect and process biological signals and achieve biofeedback control. For example, flexible bioelectronic devices need to be able to detect and recognize signals within organisms, such as electrical signals, chemical signals, biomechanical signals, and optical signals and convert these signals into digital signals for processing and analysis.
Spatial accuracy. Although electromagnetic based flexible bioelectronics can achieve invasive surgery in the human body and work on complex surfaces such as organs, the spatial accuracy of its operation is a challenge. Flexible materials may exhibit nonlinear behavior during deformation, which poses challenges to the control prediction of the system.
Drive method. The movement of electronic devices can be achieved through magnetic drive, and through hybrid drives such as magnetothermal, magneto-optical, and magnetochemical, multifunctional operations can be achieved, such as grasping, rolling, and other actions in software robots.
Finally, the flexible bioelectronic devices can be worn on the human body at present, and the soft characteristics can enable the devices to be implanted into the human body or animal body, but they are still in the initial stage, and the operation of flexible robots is far from being as flexible and elegant as the natural soft creatures.
6 Conclusion
Here, we provide an overview of the current status of flexible bioelectronics, including the basic composition and working principle of electromagnetic based flexible bioelectronic. We discuss the key technologies in flexible bioelectronics fabrication, involving design method and material selection for soft integration and sensor fabrication techniques. Additionally, the application fields of flexible bioelectronics are reviewed, including wearable devices, implantable devices and soft robots. Finally, we discuss the challenges facing flexible bioelectronics, such as the need for the development of new materials with improved mechanical properties and better production efficiency.
Materials play a key role in flexible bioelectronics. At present, the flexible materials used are still traditional and common types of materials. When combined with other materials, their mechanical properties are often not ideal, such as small stretchable range. Therefore, there are still many new organic materials that need to be developed and developed by scientists. In addition, the preparation efficiency of materials also needs to be improved, and better and stable processes should be developed to achieve large-scale production.
On the other hand, the electromagnetic based flexible bioelectronic also needs further optimization design. By optimizing the surface structure of the equipment, operations can be carried out on more complex object surfaces. In addition, the control accuracy of the device can be improved by combining deep learning and other methods, so as to design flexible biological electronic device with more accurate and stable control.
Overall, with the continuous development of flexible bioelectronic system integration and magnetic soft material preparation technology, electromagnetic based flexible bioelectronic is expected to become an extremely attractive direction in the fields of biology, medical devices and other fields.
Author contributions
SP and MZ conceived and designed the project. SP wrote the main draft of the manuscript. MZ wrote the sections of the manuscript. LL collected the data and results. HS provided the guidance and reviewed the manuscript. All authors contributed to the article and approved the submitted version.
Funding
This work was supported by National Natural Science Foundation of China: project No. 52175055.
Conflict of interest
The authors declare that the research was conducted in the absence of any commercial or financial relationships that could be construed as a potential conflict of interest.
Publisher’s note
All claims expressed in this article are solely those of the authors and do not necessarily represent those of their affiliated organizations, or those of the publisher, the editors and the reviewers. Any product that may be evaluated in this article, or claim that may be made by its manufacturer, is not guaranteed or endorsed by the publisher.
References
Abolpour Moshizi, S., Azadi, S., Belford, A., Razmjou, A., Wu, S., Han, Z. J., et al. (2020). Development of an ultra-sensitive and flexible piezoresistive flow sensor using vertical graphene nanosheets. Nano-Micro Lett. 12, 109–118. doi:10.1007/s40820-020-00446-w
Acikgoz, C., Hempenius, M. A., Huskens, J., and Vancso, G. J. (2011). Polymers in conventional and alternative lithography for the fabrication of nanostructures. Eur. Polym. J. 47 (11), 2033–2052. doi:10.1016/j.eurpolymj.2011.07.025
Ban, S., Lee, Y. J., Kwon, S., Kim, Y. S., Chang, J. W., Kim, J. H., et al. (2023). Soft wireless headband bioelectronics and electrooculography for persistent human–machine interfaces. ACS Applied Electronic Materials 5 (2), 877–886. doi:10.1021/acsaelm.2c01436
Banerjee, M., Sachdev, P., and Mukherjee, G. S. (2012). Preparation of PVA/Co/Ag film and evaluation of its magnetic and microstructural properties. J. Appl. Phys. 111 (9), 094302. doi:10.1063/1.4708058
Bartlett, N. W., Tolley, M. T., Overvelde, J. T. B., Weaver, J. C., Mosadegh, B., Bertoldi, K., et al. (2015). A 3D-printed, functionally graded soft robot powered by combustion. Science 349 (6244), 161–165. doi:10.1126/science.aab0129
Breger, J. C., Yoon, C. K., Xiao, R., Kwag, H. R., Wang, M. O., Fisher, J. P., et al. (2015). Self-folding thermo-magnetically responsive soft microgrippers. ACS Appl. Mater. interfaces 7 (5), 3398–3405. doi:10.1021/am508621s
Burke, A., and Hasirci, N. (2004). Polyurethanes in biomedical applications. Biomaterials Mol. Eng. Tissue 553, 83–101. doi:10.1007/978-0-306-48584-8_7
Cai, Y., Shen, J., Ge, G., Zhang, Y., Jin, W., Huang, W., et al. (2018). Stretchable Ti3C2Tx MXene/carbon nanotube composite based strain sensor with ultrahigh sensitivity and tunable sensing range. ACS Nano 12 (1), 56–62. doi:10.1021/acsnano.7b06251
Cataldi, P., Bayer, I. S., Bonaccorso, F., Pellegrini, V., Athanassiou, A., and Cingolani, R. (2015). Foldable conductive cellulose fiber networks modified by graphene nanoplatelet-bio-based composites. Adv. Electron. Mater. 1 (12), 1500224. doi:10.1002/aelm.201500224
Chen, S., Qi, J., Fan, S., Qiao, Z., Yeo, J. C., and Lim, C. T. (2023). Flexible wearable sensors for cardiovascular health monitoring. Adv. Healthc. Mater. 10, e2100116. doi:10.1002/adhm.202100116
Chen, T., Hao, R., Peng, H., and Dai, L. (2014). High-performance, stretchable, wire-shaped supercapacitors. Angew. Chem. Int. Ed. 54, 618–622. n/a–n/a. doi:10.1002/anie.201409385
Chen, Y., Zhang, Y., Liang, Z., Cao, Y., Han, Z., and Feng, X. (2020). Flexible inorganic bioelectronics. Npj Flex. Electron. 4 (1), 2. doi:10.1038/s41528-020-0065-1
Chen, Z., Xi, J., Huang, W., and Yuen, M. M. F. (2017). Stretchable conductive elastomer for wireless wearable communication applications. Sci. Rep. 7 (1), 10958. doi:10.1038/s41598-017-11392-w
Chitrakar, C., Hedrick, E., Adegoke, L., and Ecker, M. (2022). Flexible and stretchable bioelectronics. Materials 15 (5), 1664. doi:10.3390/ma15051664
Dagdeviren, C., Javid, F., Joe, P., von Erlach, T., Bensel, T., Wei, Z., et al. (2017). Flexible piezoelectric devices for gastrointestinal motility sensing. Nat. Biomed. Eng. 1 (10), 807–817. doi:10.1038/s41551-017-0140-7
Dai, W., Xu, H., Zhang, C., Li, Y., Pan, H., Wang, H., et al. (2019). Flexible magnetoelectrical devices with intrinsic magnetism and electrical conductivity. Adv. Electron. Mater. 5 (6), 1900111. doi:10.1002/aelm.201900111
Diller, E., and Sitti, M. (2014). Three-dimensional programmable assembly by untethered magnetic robotic micro-grippers. Adv. Funct. Mater. 24 (28), 4397–4404. doi:10.1002/adfm.201400275
D’Imperio, L. A., McCrossan, A. F., Naughton, J. R., Merlo, J. M., Calm, Y. M., Burns, M. J., et al. (2018). Arrays of electrically-addressable, optically-transmitting 3D nanostructures on free-standing, flexible polymer films. Flexible Print. Electron. 3 (2), 025007. doi:10.1088/2058-8585/aac8fc
Ding, B., Zhang, Y., Wang, J., Mei, S., Chen, X., Li, S., et al. (2022). Selective laser sintering 3D-Printed conductive thermoplastic polyether-block-amide elastomer/carbon nanotube composites for strain sensing system and electro-induced shape memory. Compos. Commun. 35, 101280. doi:10.1016/j.coco.2022.101280
Dolete, G., Chircov, C., Motelica, L., Ficai, D., Oprea, O. C., Gheorghe, M., et al. (2022). Magneto-mechanically triggered thick films for drug delivery micropumps. Nanomaterials 12 (20), 3598. doi:10.3390/nano12203598
El-Atab, N., Mishra, R. B., Al-Modaf, F., Joharji, L., Alsharif, A. A., Alamoudi, H., et al. (2020). Soft actuators for soft robotic applications: a review. Adv. Intell. Syst. 2 (10), 2070102. doi:10.1002/aisy.202070102
Eshaghi, M., Ghasemi, M., and Khorshidi, K. (2021). Design, manufacturing and applications of small-scale magnetic soft robots. Extreme Mech. Lett. 44, 101268. doi:10.1016/j.eml.2021.101268
Fonseca, F. C., Goya, G. F., Jardim, R. F., Muccillo, R., Carreño, N. L. V., Longo, E., et al. (2002). Superparamagnetism and magnetic properties of Ni nanoparticles embedded in SiO2. Phys. Rev. B 66 (10), 104406. doi:10.1103/PhysRevB.66.104406
Fu, Y., Zhao, S., Wan, Z., Tian, Y., and Wang, S. (2022). Investigation into a lightweight polymeric porous sponge with high magnetic field and strain sensitivity. Nanomaterials 12 (16), 2762. doi:10.3390/nano12162762
Gao, D., Parida, K., and Lee, P. S. (2019). Emerging soft conductors for bioelectronic interfaces. Adv. Funct. Mater. 1907184. doi:10.1002/adfm.201907184
Guan, R., Zheng, H., Liu, Q., Ou, K., Li, D. s., Fan, J., et al. (2022). DIW 3D printing of hybrid magnetorheological materials for application in soft robotic grippers. Compos. Sci. Technol. 223, 109409. doi:10.1016/j.compscitech.2022.109409
Guldiken, R., and Onen, O. (2012). MEMS ultrasonic transducers for biomedical applications. MEMS Biomed. Appl., 120–149. doi:10.1533/9780857096272.2.120
Ha, T., Tran, J., Liu, S., Jang, H., Jeong, H., Mitbander, R., et al. (2019). A chest-laminated ultrathin and stretchable E-tattoo for the measurement of electrocardiogram, seismocardiogram, and cardiac time intervals. Adv. Sci. 6, 1900290. doi:10.1002/advs.201900290
Han, Z., Li, H., Xiao, J., Song, H., Li, B., Cai, S., et al. (2019). Ultralow-cost, highly sensitive, and flexible pressure sensors based on carbon black and airlaid paper for wearable electronics. ACS Appl. Mater. interfaces 11 (36), 33370–33379. doi:10.1021/acsami.9b12929
Heo, D. N., Lee, S. J., Timsina, R., Qiu, X., Castro, N. J., and Zhang, L. G. (2019). Development of 3D printable conductive hydrogel with crystallized PEDOT: PSS for neural tissue engineering. Mater. Sci. Eng. C 99, 582–590. doi:10.1016/j.msec.2019.02.008
Hines, L., Petersen, K., Lum, G. Z., and Sitti, M. (2016). Soft actuators for small-scale robotics. Adv. Mater. 29 (13), 1603483. doi:10.1002/adma.201603483
Hu, X., Ge, Z., Wang, X., Jiao, N., Tung, S., and Liu, L. (2022). Multifunctional thermo-magnetically actuated hybrid soft millirobot based on 4D printing. Compos. Part B Eng. 228, 109451. doi:10.1016/j.compositesb.2021.109451
Hui, S., Zhang, Y. D., Xiao, T. D., Wu, M., Ge, S., Hines, W. A., et al. (2001). Fe/SiO 2 nanocomposite soft magnetic materials. MRS Online Proc. Libr. 703, V6.3–6. doi:10.1557/PROC-703-V6.3
Ji, Z., Yan, C., Yu, B., Wang, X., and Zhou, F. (2017). Multimaterials 3D printing for free assembly manufacturing of magnetic driving soft actuator. Adv. Mater. Interfaces 4 (22), 1700629. doi:10.1002/admi.201700629
Joshipura, I., Finn, M., Tan, S., Dickey, M., and Lipomi, D. (2017). Stretchable bioelectronics—current and future. MRS Bull. 42 (12), 960–967. doi:10.1557/mrs.2017.270
Joyee, E. B., and Pan, Y. (2020). Additive manufacturing of multi-material soft robot for on-demand drug delivery applications. J. Manuf. Process. 56, 1178–1184. doi:10.1016/j.jmapro.2020.03.059
Jumril, Y., Muzalifah, M. S., Burhanuddin, Y. M., and Bais, B. (2014). Finite element analysis and preliminary fabrication of flexible membrane with embedded magnetic nanoparticles. Adv. Mater. Res. 1024, 147–150. doi:10.4028/www.scientific.net/amr.1024.147
Kalantar-zadeh, K., Ha, N., Ou, J. Z., and Berean, K. J. (2017). Ingestible sensors. ACS Sensors 2 (4), 468–483. doi:10.1021/acssensors.7b00045
Kanyanta, V., and Ivankovic, A. (2010). Mechanical characterisation of polyurethane elastomer for biomedical applications. J. Mech. Behav. Biomed. Mater. 3 (1), 51–62. doi:10.1016/j.jmbbm.2009.03.005
Kesama, M. R., and Kim, S. (2023). DNA-Nanocrystal assemblies for environmentally responsive and highly efficient energy harvesting and storage. Adv. Sci. 10 (14), 2206848. doi:10.1002/advs.202206848
Kim, H., Kim, Y.-S., Mahmood, M., Kwon, S., Epps, F., Rim, Y. S., et al. (2020). Wireless, continuous monitoring of daily stress and management practice via soft bioelectronics. Biosens. Bioelectron. 112764, 112764. doi:10.1016/j.bios.2020.112764
Kim, Y., Chortos, A., Xu, W., Liu, Y., Oh, J. Y., Son, D., et al. (2018). A bioinspired flexible organic artificial afferent nerve. Science 360, 998–1003. doi:10.1126/science.aao0098
Ko, H. C., Stoykovich, M. P., Song, J., Malyarchuk, V., Choi, W. M., Yu, C.-J., et al. (2008). A hemispherical electronic eye camera based on compressible silicon optoelectronics. Nature 454 (7205), 748–753. doi:10.1038/nature07113
Ko, Y., Kim, J., Kim, D., Yamauchi, Y., and You, J. (2017). A simple silver nanowire patterning method based on poly (ethylene glycol) photolithography and its application for soft electronics. Sci. Rep. 7 (1), 2282. doi:10.1038/s41598-017-02511-8
Kong, N., Zhang, J., Hegh, D., Usman, K. A. S., Qin, S., Lynch, P. A., et al. (2022). Environmentally stable MXene ink for direct writing flexible electronics. Nanoscale 14 (17), 6299–6304. doi:10.1039/D1NR07387G
Koylu-Alkan, O., Barandiaran, J. M., Salazar, D., and Hadjipanayis, G. C. (2016). Submicron R2Fe14B particles. AIP Adv. 6 (5), 056027. doi:10.1063/1.4944771
Kwon, Y. T., Kim, H., Mahmood, M., Kim, Y. S., Demolder, C., and Yeo, W. H. (2020). Printed, wireless, soft bioelectronics and deep learning algorithm for smart human–machine interfaces. ACS Applied Materials & Interfaces 12 (44), 49398–49406. doi:10.1021/acsami.0c14193
Lee, S., Inoue, Y., Kim, D., Reuveny, A., Kuribara, K., Yokota, T., et al. (2014). A strain-absorbing design for tissue–machine interfaces using a tunable adhesive gel. Nat. Commun. 5 (1), 5898. doi:10.1038/ncomms6898
Li, J., Zhan, Q., Zhang, S., Wei, J., Wang, J., Pan, M., et al. (2017a). Magnetic anisotropy and high-frequency property of flexible FeCoTa films obliquely deposited on a wrinkled topography. Sci. Rep. 7 (1), 2837. doi:10.1038/s41598-017-03288-6
Li, Q., Nan, K., Le Floch, P., Lin, Z., Sheng, H., Blum, T. S., et al. (2019). Cyborg organoids: implantation of nanoelectronics via organogenesis for tissue-wide electrophysiology. Nano Lett. 19, 5781–5789. doi:10.1021/acs.nanolett.9b02512
Li, R., Zhang, L., Shi, L., and Wang, P. (2017b). MXene Ti3C2: an effective 2D light-to-heat conversion material. ACS Nano 11 (4), 3752–3759. doi:10.1021/acsnano.6b08415
Li, S., Cong, Y., and Fu, J. (2021b). Tissue adhesive hydrogel bioelectronics. J. Mater. Chem. B 9 (22), 4423–4443. doi:10.1039/d1tb00523e
Li, S., Zhou, X., Dong, Y., and Li, J. (2020). Flexible self-repairing materials for wearable sensing applications: elastomers and hydrogels. Macromol. Rapid Commun. 2000444, e2000444. doi:10.1002/marc.202000444
Li, Y., Li, N., De Oliveira, N., and Wang, S. (2021a). Implantable bioelectronics toward long-term stability and sustainability. Matter 4 (4), 1125–1141. doi:10.1016/j.matt.2021.02.001
Li, Y., Liu, X., Zhang, Y., Wu, Z., Ling, W., Zhang, X., et al. (2023). A flexible wearable device coupled with injectable Fe3O4 nanoparticles for capturing circulating tumor cells and triggering their deaths. Biosens. Bioelectron. 235, 115367. doi:10.1016/j.bios.2023.115367
Li, Y., Wang, Q., and Yang, H. (2009). Synthesis, characterization and magnetic properties on nanocrystalline BaFe12O19 ferrite. Curr. Appl. Phys. 9 (6), 1375–1380. doi:10.1016/j.cap.2009.03.002
Liao, M., Liao, H., Ye, J., Wan, P., and Zhang, L. (2019). Polyvinyl alcohol-stabilized liquid metal hydrogel for wearable transient epidermal sensors. ACS Appl. Mater. Interfaces 11, 47358–47364. doi:10.1021/acsami.9b16675
Lin, D., Yang, F., Gong, D., and Li, R. (2023). Bio-inspired magnetic-driven folded diaphragm for biomimetic robot. Nat. Commun. 14 (1), 163. doi:10.1038/s41467-023-35905-6
Lipomi, D., Vosgueritchian, M., Tee, B. K., Hellstrom, S. L., Lee, J. A., Fox, C. H., et al. (2011). Skin-like pressure and strain sensors based on transparent elastic films of carbon nanotubes. Nat. Nanotech 6, 788–792. doi:10.1038/nnano.2011.184
Liu, W., Lei, Z., Yang, R., Xing, W., Tao, P., Shang, W., et al. (2022). Facile approach to enhance electrical and thermal performance of conducting polymer PEDOT: PSS films via hot pressing. ACS Appl. Mater. Interfaces 14 (8), 10605–10615. doi:10.1021/acsami.1c19397
Liu, X., Wei, Y., and Qiu, Y. (2021). Advanced flexible skin-like pressure and strain sensors for human health monitoring. Micromachines 12, 695. doi:10.3390/mi12060695
Liu, Y., Wang, H., Zhao, W., Zhang, M., Qin, H., and Xie, Y. (2018). Flexible, stretchable sensors for wearable health monitoring: sensing mechanisms, materials, fabrication strategies and features. Sensors 18 (2), 645. doi:10.3390/s18020645
Lockett, M., Sarmiento, V., Balingit, M., Oropeza-Guzmán, M. T., and Vázquez-Mena, O. (2020). Direct chemical conversion of continuous CVD graphene/graphite films to graphene oxide without exfoliation. Carbon 158, 202–209. doi:10.1016/j.carbon.2019.10.076
Lu, H., Zhang, M., Yang, Y., Huang, Q., Fukuda, T., Wang, Z., et al. (2018). A bioinspired multilegged soft millirobot that functions in both dry and wet conditions. Nat. Commun. 9 (1), 3944. doi:10.1038/s41467-018-06491-9
Manns, M., Morales, J., and Frohn, P. (2018). Additive manufacturing of silicon based PneuNets as soft robotic actuators. Procedia CIRP 72, 328–333. doi:10.1016/j.procir.2018.03.186
Mirzanejad, H., and Agheli, M. (2019). Soft force sensor made of magnetic powder blended with silicone rubber. Sensors Actuators A Phys. 293, 108–118. doi:10.1016/j.sna.2019.04.021
Mosadegh, B., Polygerinos, P., Keplinger, C., Wennstedt, S., Shepherd, R. F., Gupta, U., et al. (2014). Pneumatic networks for soft robotics that actuate rapidly. Adv. Funct. Mater. 24 (15), 2163–2170. doi:10.1002/adfm.201303288
Nagahama, S., Migita, K., and Sugano, S. (2019). Soft magnetic powdery sensor for tactile sensing. Sensors 19 (12), 2677. doi:10.3390/s19122677
Nelson, M. D., Ramkumar, N., and Gale, B. K. (2019). Flexible, transparent, sub-100 µm microfluidic channels with fused deposition modeling 3D-printed thermoplastic polyurethane. J. Micromechanics Microengineering 29 (9), 095010. doi:10.1088/1361-6439/ab2f26
Ongaro, F., Scheggi, S., Yoon, C. K., den Brink, F. v., Oh, S. H., Gracias, D. H., et al. (2017). Autonomous planning and control of soft untethered grippers in unstructured environments. J. micro-bio robotics 12, 45–52. doi:10.1007/s12213-016-0091-1
Ozbolat, V., Dey, M., Ayan, B., Povilianskas, A., Demirel, M. C., and Ozbolat, I. T. (2018). 3D printing of PDMS improves its mechanical and cell adhesion properties. ACS Biomaterials Sci. Eng. 4 (2), 682–693. doi:10.1021/acsbiomaterials.7b00646
Pan, W., Ao, Y., Zhou, P., Fetisov, L., Fetisov, Y., Zhang, T., et al. (2023). A Flexible Magnetic Field Sensor Based on PZT/CFO Bilayer via van der Waals Oxide Heteroepitaxy. Sensors 23 (22), 9147. doi:10.3390/s23229147
Pang, D., and Chang, C. (2017). Development of a novel transparent flexible capacitive micromachined ultrasonic transducer. Sensors 17, 1443. doi:10.3390/s17061443
Park, S.-J., Jeon, J.-Y., Kang, B.-C., and Ha, T.-J. (2020). Wearable temperature sensors based on lanthanum-doped aluminum-oxide dielectrics operating at low-voltage and high-frequency for healthcare monitoring systems. Ceram. Int. 47, 4579–4586. doi:10.1016/j.ceramint.2020.10.023
Park, Y. J., Sharma, B. K., Shinde, S. M., Kim, M. S., Jang, B., Kim, J. H., et al. (2019). All MoS2-based large area, skin-attachable active-matrix tactile sensor. ACS Nano 13 (3), 3023–3030. doi:10.1021/acsnano.8b07995
Phillips, J. W., Prominski, A., and Tian, B. (2022). Recent advances in materials and applications for bioelectronic and biorobotic systems. View 3 (3), 20200157. doi:10.1002/viw.20200157
Pirmoradi, F. N., Jackson, J. K., Burt, H. M., and Chiao, M. (2011). On-demand controlled release of docetaxel from a battery-less MEMS drug delivery device. Lab a Chip 11 (16), 2744–2752. doi:10.1039/c1lc20134d
Pons, J. L. L., Rocon, E., Forner-Cordero, A., and Moreno, J. (2007). Biomedical instrumentation based on piezoelectric ceramics. J. Eur. Ceram.Soc. 27, 4191–4194. doi:10.1016/j.jeurceramsoc.2007.02.126
Pyo, S., Lee, J., Bae, K., Sim, S., and Kim, J. (2021). Recent progress in flexible tactile sensors for human-interactive systems: from sensors to advanced applications. Adv. Mater. 33, 2005902. doi:10.1002/adma.202005902
Qi, S., Guo, H., Fu, J., Xie, Y., Zhu, M., and Yu, M. (2020). 3D printed shape-programmable magneto-active soft matter for biomimetic applications. Compos. Sci. Technol. 188, 107973. doi:10.1016/j.compscitech.2019.107973
Qingyu, M., Junhua, Y., Jifeng, Z., Ziyu, L., et al. (2022). Review on chemical synthesis of Nd-FeB magnetic nanoparticles: microstructure and magnetic properties. Rare Metal Mater. Eng. 51 (04), 1253–1262. doi:10.12442/j.issn.1002-185X.20210180
Qiu, T., Lee, T. C., Mark, A., Morozov, K. I., Münster, R., Mierka, O., et al. (2014). Swimming by reciprocal motion at low Reynolds number. Nat. Commun. 5, 5119. doi:10.1038/ncomms6119
Rao, A. U., Tiwari, S. K., Goyat, M. S., and Chawla, A. K. (2023). Recent developments in magnetron-sputtered silicon nitride coatings of improved mechanical and tribological properties for extreme situations. J. Mater. Sci. 58, 9755–9804. doi:10.1007/s10853-023-08575-4
Reghu, S., You, H., Seenivasan, K., Nishimura, S., Taniike, T., and Miyako, E. (2020). Design and control of bioinspired millibots. Adv. Intell. Syst. 2, 2000059. doi:10.1002/aisy.202000059
Regtien, P., and Dertien, E. (2018). Piezoelectric sensors. Sensors Mechatronics, 245–265. doi:10.1016/b978-0-12-813810-6.00008-2
Reiser, A., Lindén, M., Rohner, P., Marchand, A., Galinski, H., Sologubenko, A. S., et al. (2019). Multi-metal electrohydrodynamic redox 3D printing at the submicron scale. Nat. Commun. 10 (1), 1853. doi:10.1038/s41467-019-09827-1
Sheikh, Z., Brooks, P., Barzilay, O., Fine, N., and Glogauer, M. (2015). Macrophages, foreign body giant cells and their response to implantable biomaterials. Materials 8 (9), 5671–5701. doi:10.3390/ma8095269
Shi, C. Y., Zhang, Q., Yu, C. Y., Rao, S., Yang, S., Tian, H., et al. (2020). An ultrastrong and highly stretchable polyurethane elastomer enabled by a zipper-like ring-sliding effect. Adv. Mater. 32 (23), 2000345. doi:10.1002/adma.202000345
Sinar, D., Knopf, G. K., and Nikumb, S. (2013). Graphene-based inkjet printing of flexible bioelectronic circuits and sensors. Micromach. Microfabr. Process Technol. 108. doi:10.1117/12.2003936
Song, W.-J., Park, J., Kim, D. H., Bae, S., Kwak, M.-J., Shin, M., et al. (2018). Jabuticaba-inspired hybrid carbon filler/polymer electrode for use in highly stretchable aqueous Li-ion batteries. Adv. Energy Mater. 8 (10), 1702478. doi:10.1002/aenm.201702478
Sun, B., Jia, R., Yang, H., Chen, X., Tan, K., Deng, Q., et al. (2022). Magnetic arthropod millirobots fabricated by 3D-printed hydrogels. Adv. Intell. Syst. 4 (1), 2100139. doi:10.1002/aisy.202100139
Sun, R., Carreira, S. C., Chen, Y., Xiang, C., Xu, L., Zhang, B., et al. (2019). Stretchable piezoelectric sensing systems for self-powered and wireless health monitoring. Adv. Mater. Technol. 4 (5), 1900100. doi:10.1002/admt.201900100
Sun, R., Ma, M., Ma, X., Kang, H., Wang, S., and Sun, J. (2023). Direct-writing flexible metal circuit with polymer/metal precursor ink and interfacial reaction. Langmuir 39, 7426–7433. doi:10.1021/acs.langmuir.3c00642
Swaminathan, V., Deheri, P. K., Bhame, S. D., and Ramanujan, R. V. (2013). Novel microwave assisted chemical synthesis of Nd2Fe14B hard magnetic nanoparticles. Nanoscale 5 (7), 2718–2725. doi:10.1039/c3nr33296a
Tang, J., Tong, Z., Xia, Y., Liu, M., Lv, Z., Gao, Y., et al. (2018). Super tough magnetic hydrogels for remotely triggered shape morphing. J. Mater. Chem. B 6 (18), 2713–2722. doi:10.1039/C8TB00568K
Tao, L. Q., Wang, D. Y., Tian, H., Ju, Z. Y., Liu, Y., Chen, Y. Q., et al. (2016). “Tunable and wearable high performance strain sensors based on laser patterned graphene flakes,” in 2016 IEEE International Electron Devices Meeting (IEDM), San Francisco, California, USA, December 3 - 7, 2016 (IEEE), 18.3. 1–18.3. 4. doi:10.1109/IEDM.2016.7838445
Tognato, R., Armiento, A. R., Bonfrate, V., Levato, R., Malda, J., Alini, M., et al. (2019). A stimuli-responsive nanocomposite for 3D anisotropic cell-guidance and magnetic soft robotics. Adv. Funct. Mater. 29 (9), 1804647. doi:10.1002/adfm.201804647
Tonelli, A. E. (2002). PET versus PEN: what difference can a ring make? Polymer 43 (2), 637–642. doi:10.1016/S1089-3156(00)00028-3
Venkateshalu, S., and Grace, A. N. (2020). MXenes—a new class of 2D layered materials: synthesis, properties, applications as supercapacitor electrode and beyond. Appl. Mater. Today 18, 100509. doi:10.1016/j.apmt.2019.100509
Wan, J., Wang, H., Miao, L., Chen, X., Song, Y., Guo, H., et al. (2020). A flexible hybridized electromagnetic-triboelectric nanogenerator and its application for 3D trajectory sensing. Nano Energy 74, 104878. doi:10.1016/j.nanoen.2020.104878
Wang, C., Li, X., Hu, H., Zhang, L., Huang, Z., Lin, M., et al. (2018). Monitoring of the central blood pressure waveform via a conformal ultrasonic device. Nat. Biomed. Eng. 2, 687–695. doi:10.1038/s41551-018-0287-x
Wang, P., Fu, J., Jin, P., Zeng, J., Miao, X., Wang, H., et al. (2023). A soft, bioinspired artificial lymphatic system for interactive ascites transfer. Bioeng. Transl. Med. 8 (5), e10567. doi:10.1002/btm2.10567
Wang, X., Zhang, Q., Liu, P., Zhu, X., Wu, C., Wang, J., et al. (2021). An ultrafast response and precisely controllable soft electromagnet actuator based on Ecoflex rubber film filled with neodymium-iron-boron. J. Micromechanics Microengineering 31 (2), 025010. doi:10.1088/1361-6439/abd222
Ware, T., Simon, D., Arreaga-Salas, D. E., Reeder, J., Rennaker, R., Keefer, E. W., et al. (2012). Fabrication of responsive, softening neural interfaces. Adv. Funct. Mater. 22 (16), 3470–3479. doi:10.1002/adfm.201200200
Wei, J., and Yu, Y. (2012). Photodeformable polymer gels and crosslinked liquid-crystalline polymers. Soft Matter 8 (31), 8050. doi:10.1039/c2sm25474c
Wu, H., Gao, W., and Yin, Z. (2017). Materials, devices and systems of soft bioelectronics for precision therapy. Adv. Healthc. Mater. 6 (10), 1700017. doi:10.1002/adhm.201700017
Xu, B., Zhu, M., Zhang, W., Zhen, X., Pei, Z., Xue, Q., et al. (2016). Ultrathin MXene-micropattern-based field-effect transistor for probing neural activity. Adv. Mater. 28 (17), 3333–3339. doi:10.1002/adma.201504657
Yang, Q., Enríquez, Á., Devathasan, D., Thompson, C. A., Nayee, D., Harris, R., et al. (2022). Application of magnetically actuated self-clearing catheter for rapid in situ blood clot clearance in hemorrhagic stroke treatment. Nat. Commun. 13 (1), 520. doi:10.1038/s41467-022-28101-5
Ye, M., Zhou, Y., Zhao, H., and Wang, X. (2023). Magnetic microrobots with folate targeting for drug delivery. Cyborg and Bionic Systems 4, 0019. doi:10.34133/cbsystems.0019
Ying, W. B., Wang, G., Kong, Z., Yao, C. K., Wang, Y., Hu, H., et al. (2021). A biologically muscle-inspired polyurethane with super-tough, thermal reparable and self-healing capabilities for stretchable electronics. Adv. Funct. Mater. 31 (10), 2009869. doi:10.1002/adfm.202009869
Yousuf, M., Garg, M., Arya, D. S., and Singh, P. (2023). Skin-Inspired Bimodal Magneto-Dielectric-Based Flexible and Sensitive Capacitive Sensor for Limb Motion Tracking. IEEE Transactions on Electron Devices 70 (3), 1338–1343. doi:10.1109/TED.2022.3232585
Yuk, H., and Zhao, X. (2018). A new 3D printing strategy by harnessing deformation, instability, and fracture of viscoelastic inks. Adv. Mater. 30 (6), 1704028. doi:10.1002/adma.201704028
Zhang, H., Li, Y., Zhang, P., Ren, C., Xu, X., Sun, B., et al. (2021). A flexible implantable polyimide catheter device for targeted treatment of cardiovascular diseases by aggregating magnetic nanoparticles. IEEE Trans. Components, Packag. Manuf. Technol. 11 (6), 911–917. doi:10.1109/TCPMT.2021.3082923
Zhang, P., and Travas-Sejdic, J. (2021). Fabrication of conducting polymer microelectrodes and microstructures for bioelectronics. J. Mater. Chem. C 9 (31), 9730–9760. doi:10.1039/d1tc01618k
Zhao, Y., Qiu, Z., and Huang, J. (2008). Preparation and analysis of Fe3O4 magnetic nanoparticles used as targeted-drug carriers. Chin. J. Chem. Eng. 16 (3), 451–455. doi:10.1016/s1004-9541(08)60104-4
Zhou, D., Yang, Y., and Rao, W. F. (2023). 3D printed magnetoelectric composites for personalized wearable multifunctional sensors. 3D Print. Addit. Manuf. doi:10.1089/3dp.2022.0396
Zhou, Y., Cao, C., Cao, Y., Han, Q., Parker, C. B., and Glass, J. T. (2020). Robust and high-performance electrodes via crumpled Au-cnt forests for stretchable supercapacitors. Matter 2, 1307–1323. doi:10.1016/j.matt.2020.02.024
Keywords: flexible bioelectronic, electromagnetic, magnetic sensation, magnetic drive, wearable device, implantable device
Citation: Pan S, Zhou M, Liu L and Shen H (2024) Electromagnetic based flexible bioelectronics and its applications. Front. Electron. 5:1240603. doi: 10.3389/felec.2024.1240603
Received: 15 June 2023; Accepted: 02 January 2024;
Published: 25 April 2024.
Edited by:
Geng Yang, Zhejiang University, ChinaReviewed by:
Ramendra Pal, Birla Institute of Technology and Science, IndiaXin Xia, Hong Kong University of Science and Technology (Guangzhou), China
Copyright © 2024 Pan, Zhou, Liu and Shen. This is an open-access article distributed under the terms of the Creative Commons Attribution License (CC BY). The use, distribution or reproduction in other forums is permitted, provided the original author(s) and the copyright owner(s) are credited and that the original publication in this journal is cited, in accordance with accepted academic practice. No use, distribution or reproduction is permitted which does not comply with these terms.
*Correspondence: Huimin Shen, hmshen@usst.edu.cn