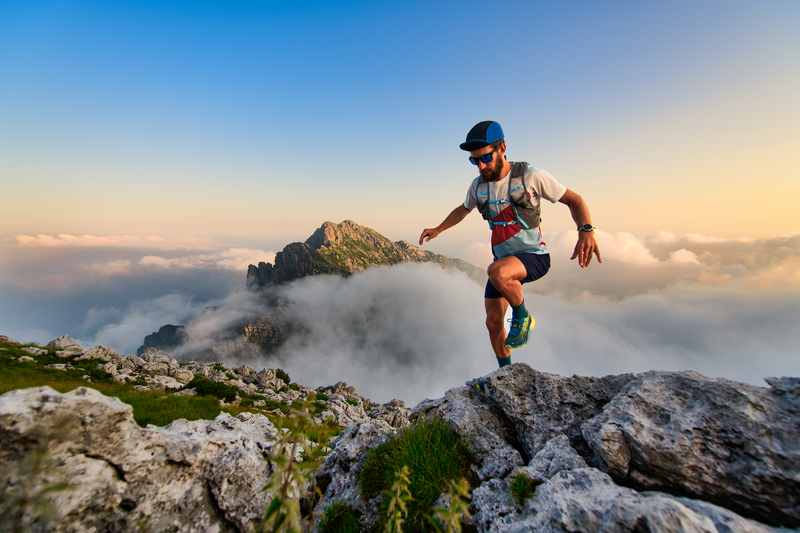
95% of researchers rate our articles as excellent or good
Learn more about the work of our research integrity team to safeguard the quality of each article we publish.
Find out more
REVIEW article
Front. Electron. , 06 September 2022
Sec. Flexible Electronics
Volume 3 - 2022 | https://doi.org/10.3389/felec.2022.985681
Biodegradable electronics have great potential to reduce the environmental footprint of electronic devices and to avoid secondary removal of implantable health monitors and therapeutic electronics. Benefiting from the intensive innovation on biodegradable nanomaterials, current transient electronics can realize full components’ degradability. However, design of materials with tissue-comparable flexibility, desired dielectric properties, suitable biocompatibility and programmable biodegradability will always be a challenge to explore the subtle trade-offs between these parameters. In this review, we firstly discuss the general chemical structure and degradation behavior of polymeric biodegradable materials that have been widely studied for various applications. Then, specific properties of different degradable polymer materials such as biocompatibility, biodegradability, and flexibility were compared and evaluated for real-life applications. Complex biodegradable electronics and related strategies with enhanced functionality aimed for different components including substrates, insulators, conductors and semiconductors in complex biodegradable electronics are further researched and discussed. Finally, typical applications of biodegradable electronics in sensing, therapeutic drug delivery, energy storage and integrated electronic systems are highlighted. This paper critically reviews the significant progress made in the field and highlights the future prospects.
The emergence of flexible electronic devices offers unlimited possibilities for their application in many areas of smart electronics, including health monitors (Li et al., 2020a), therapeutic devices (Long et al., 2018; Liu et al., 2021; Long et al., 2021), wireless power supply components (Li et al., 2018a; Li et al., 2020b; Li et al., 2020c; Li et al., 2021a) and artificial electronic skin (Chou et al., 2015; Miyamoto et al., 2017), etc. Flexible electronic devices should be developed with good mechanical deformability and integrated functionality, designed to adapt to the specific tissues with which they interact and to move with minimal damage to tissue properties, significantly reducing the immune response of human tissues (Wang et al., 2020a). However, prolonged exposure to non-biological components in the vicinity of the implanted organ will inevitably lead to a negative immune response in the short and/or long term (Li et al., 2020d; Wang et al., 2022a). As a result, surgical removal of chronic implants is often required after the intended use has been achieved, but this additional secondary surgery can be physically and financially taxing for the patient.
Over the past decade, biodegradable electronic devices (transient electronics) that naturally degrade or completely dissolve in the physiological environment have emerged as attractive alternatives in invasive and non-invasive biomedical fields. Arterial pulse sensors for blood flow monitoring (Boutry et al., 2019), bioresorbable pacemakers without leads or batteries (Choi et al., 2021), biodegradable supercapacitors (Hu et al., 2019), and multifunctional integrated electronic skins represent flexible (Kang et al., 2016), stretchable biodegradable electronics that can be safely absorbed by the body after they have completed their therapeutic and diagnostic functions, marking a tremendous advancement of transient electronics in biomedicine (Figure 1). More importantly, these efforts benefit from the vast chemical design space of various organic polymers allowing great tunability of electronic, mechanical and degradable properties. This tunability circumvents the use of complex structures and patterns to achieve the desired properties and facilitates the development of advanced biodegradable electronics.
FIGURE 1. Applications of biodegradable electronics include implantable sensors, therapeutic electronics, implantable power devices, and integrated electronic systems. These achievements have been made possible by the vast chemical design space of various organic polymers, allowing for great tuning of electronic, mechanical and degradable properties. Images adapted with permission from refs (Son et al., 2015; Zhang et al., 2018; Boutry et al., 2019; Li et al., 2020e; Koo et al., 2020; Choi et al., 2021; Sheng et al., 2021; Yue et al., 2021). Copyright 2019 Springer Nature, 2020 Springer Nature, 2021 Springer Nature, 2020 AAAS, 2021 AAAS, 2018 Wiley, 2015 American Chemical Society, and 2021 Wiley.
For many applications, complete breakdown of the polymer into monomeric structural units (Type I degradable polymers) (Feig et al., 2018) is not required, and the breakdown equipment alone is sufficient to alleviate the need for invasive and expensive recycling procedures (Feig et al., 2018). In addition to macroscopic degradation, the molecular cleavage of the polymer backbone into oligomers and monomers can be further broken down by immune mechanisms in vivo or through microorganisms in the environment. This more complete chemical degradation (called Type II) (Feig et al., 2018) may help alleviate the environmental problems of discarded e-waste as electronics become more prevalent (Li et al., 2018b).
This review provides an overview of the chemical structure and degradation behavior of degradable polymers used in a wide range of electronic products, comparing and evaluating the mechanical and degradation properties of different degradable polymeric materials, focusing on the tunable electronic properties of polymeric materials. Finally, typical applications of biodegradable polymers materials in sensing, therapeutic drug delivery, energy storage and integrated electronic systems are presented, and the main challenges and future prospects for stretchable and bioresorbable electronics are indicated.
Biodegradable polymers include both naturally derived materials and synthetic polymers, which are classified in the manner shown in the Figure 2. Among the naturally derived materials, plant-based polysaccharides (e.g., cellulose, alginate, dextran) and animal-derived polymers (e.g., collagen, silk, chitosan) are used for transient electronic applications due to their inherent enzymatic degradability (Sun et al., 2018). However, these materials, despite their better biocompatibility and degradable properties, may be more complex to extract than synthetic polymers (Diouf-Lewis et al., 2017; Kumar et al., 2018; Chang et al., 2020). Since the simplest linear, aliphatic and thermoplastic polyester polyglycolide (PGA) was marketed as the first biodegradable suture in the 1960s, many advances in the development of synthetic biodegradable polymers have been made in a range of synthetic biodegradable polymers (Schmitt et al., 1969; Middleton and Tipton, 2000; Nair and Laurencin, 2007). Various biodegradable poly (α-ester) cross-linked elastomers such as poly (1,8-octanediol-citrate) (POC) citrate and poly (glycerol sebacate) (PGS), polycarbonate, polyurethane (PU), polydioxanone, and polyhydroxyalkanoate (PHA) have emerged. Of these, poly (lactic acid) or poly (propylene glycol) (PLA/PLLA), poly (co-glycolic acid) or poly (propylene glycol) (co-glycol) (PLGA), POC, PGS, polycaprolactone (PCL), and more recently, polyhydroxybutyrate (PHB) and polyhydroxyvalerate (PHV) have attracted the most attention (Can et al., 2011; Vey et al., 2011; Bonartsev et al., 2012; Tian et al., 2012; Yang et al., 2014; Goonoo et al., 2015; Regazzoli et al., 2017; Wang et al., 2022b; Vlachopoulos et al., 2022).
FIGURE 2. Classification of natural and synthetic biodegradable polymeric materials and the corresponding chemical structures.
Polymeric materials are highly tunable in terms of their chemical structure, morphology and dissolution time scales, and their rates can be adjusted by changing the intrinsic properties of the polymer, including molecular weight, crystal structure, chemical composition, hydrophilic or hydrophobic properties and erosion mechanisms (Nair and Laurencin, 2007; Feig et al., 2018). In addition to flexibility and biocompatibility, this ability to modulate the intrinsic properties of polymeric materials makes them promising candidates for compliant, customizable and biodegradable device components (Amass et al., 1998; Nair and Laurencin, 2007).
Polymers are attractive for their scalability, solution processability, ability to be rationally tuned by synthetic design, and a variety of material properties (e.g., stretchability, toughness, compliance, electrical conductivity) (Chiong et al., 2021). In designing polymers that are eco- and human-friendly as well as impart desirable properties (e.g., self-healing, stimulus responsiveness, adhesion) for electronic applications. Next, we will discuss how to design polymers to impart biocompatible, biodegradable, flexible, conductive or other specific functional properties.
Generally, a material is considered biocompatible if it produces an acceptable host response when exposed to body or body fluids (Vert et al., 2012). These adverse reactions are triggered by chemical or physical reactions of the material and include chronic inflammation, production of cytotoxic substances, and corrosion of the implanted material and so on (Irimia-Vladu, 2014; Cao and Uhrich, 2018). When considering wearable and implantable electronics, degradable polymer materials must not only be chemically compatible with their surroundings (i.e., hydrophilic, non-contaminating, non-toxic), but also mechanically compatible (i.e., flexible, stretchable) (Vert et al., 2012). Rigorous evaluation through in vitro culture experiments or in vivo implantation is critical before materials can be classified as biocompatible.
Natural polymers derived from biological systems, including polymers derived from proteins and polysaccharides (e.g., sericin, collagen, gelatin, elastin) are readily available, inexpensive, and often biocompatible (i.e., non-toxic and non-inflammatory) (Xu et al., 2018; Chen et al., 2019; Wang et al., 2020b). Synthetic polymers such as PLA, PLGA, and PEG are often used in implantable electronics due to their typical lubricity, sterilization capabilities, broad temperature tolerance and minimal chemical reactivity in vivo (Fukai et al., 2004; Teng, 2012; Huang, 2017).
In recent scientific studies, many representative biocompatibility studies of degradable polymers have been widely reported (Hosseini et al., 2021). For example, the biocompatibility of PLA and PLGA microspheres implanted in rats was verified by human histological and immunological analyses (Anderson and Shive, 2012). The biocompatibility of POC scaffolds was confirmed by their lack of effect on the morphology and phenotype of porcine chondrocytes (Jeong and Hollister, 2010). The biocompatibility of PGS films was demonstrated in a similar manner (Rai et al., 2012). The biocompatibility of PCL films was confirmed by examining the effect of their exposure on the viability of L929 mouse fibroblasts (Serrano et al., 2004). Finally, the in vitro and in vivo biocompatibility of silk has been widely demonstrated, and it is widely used as a substrate for degradable electronics and in many biomedical applications (Vepari and Kaplan, 2007).
In contrast to polymeric materials containing superhydrophilic groups, cationic polymers, particularly poly (ethyleneimine) (PEI), have recently begun to be used in biocompatible polymer research (Englert et al., 2015). Although this cationic polymer has less potential as a substrate and encapsulant, it achieves complete biocompatibility and degradability of the device, which is important to facilitate the development of implantable transient electronic devices. These recent examples demonstrate the importance of considering charge density when designing and modifying the molecular structure of polymers (Bus et al., 2017). Most current polymer-based electronics do not use charged polymers, thus leaving much potential for the application of these emerging polymers in implantable electronics.
The time that a biodegradable polymer is able to maintain its designed function under conditions of use is the most important indicator of its performance. This duration is referred to as the “functional time” (Hosseini et al., 2021). The time it takes for a polymer material to completely degrade and lose weight is also important. This time is referred to as the “disappearance time” (Chiong et al., 2021; Hosseini et al., 2021; Manousiouthakis et al., 2022). Depending on the chemical and physical properties and the rate of release, the degradation products can cause biocompatibility problems. For the successful use of polymers, the functional time, the time of disappearance, the degradation products and the release rate must be well characterized and controlled. For this purpose, the degradation mechanisms must be understood.
The main degradation mechanisms of the materials used in all these applications are similar, but differ kinetically due to different processing conditions and use environments. Polymers used in biomedical devices have four main degradation mechanisms: hydrolysis (reaction with water in the tissue), oxidation (due to oxidants produced by the tissue), enzymatic degradation, and physical degradation (e.g., water swelling and mechanical loading and wear) (Brannigan and Dove, 2017). Widely used biodegradable synthetic polymers often contain ester bonds that promote hydrolytic degradation under acidic or alkaline conditions. Amide, sulfonamide, anhydride, carbonate, ether, acyl, imide, phosphonate, thioester, urea and carbamate bonds are also unstable sites susceptible to hydrolytic degradation (Brannigan and Dove, 2017; Chiong et al., 2021) (Figure 3A). The mechanism of polymer hydrolysis has been extensively studied and more detailed review articles can be found (Lyu and Untereker, 2009; Brannigan and Dove, 2017) (Figures 3C,D).
FIGURE 3. Chemical structures of fractions susceptible to hydrolysis (A) and oxidation (B) are shown. Hydrolysable bonds and oxidative attack sites are highlighted in red and marked with an asterisk. Images adapted with permission from 2018, American Chemical Society (Feig et al., 2018). (C) Scheme of water-soluble, temperature-responsive polyacetals and their degradation by acid hydrolysis. (D) Chemical structures of the fully degradable semiconductor polymer p (DPP-PPD) and its monomeric by-products after cleavage and the biodegradable elastomer e-PCL. Images adapted with permission from 2021, Wiley (Chiong et al., 2021).
Oxidation is another fundamental process that degrades polymers. The oxidation mechanism usually involves the formation, proliferation and movement of free radicals within the polymer prior to final termination (George and Celina, 2000) (Figure 3B). These reactions may also involve catalysts, including natural enzymes produced by the body. Enzymatic degradation is also due to defense against implanted foreign substances. Collagen, polysaccharides (hyaluronic acid), some polyesters (e.g., polyhydroxyalkanoates, PHA), synthetic polycarbonates, and proteins are degraded primarily due to these types of reactions (Casas et al., 1999).
An important issue to consider is the effect of polymer degradation products on humans. Hydrolysis produces carboxylic acids and/or hydroxyl chain ends, and the hydroxyl groups can later be further oxidized. The products of the oxidation reaction are usually aldehydes, ketones or carboxylic acids. Implants of smaller size and slower degradation may trigger a milder immune response than larger implants made of the same material (Lyu and Untereker, 2009; Ilyas et al., 2022a).
Flexibility and stretchability are desirable mechanical characteristics for biomedical devices and are critical when attaching materials to the body. The flexible surfaces of organs and tissues greatly limit the application of rigid electronic products, placing higher demands on the flexibility of new biodegradable electronic products, especially the flexibility and stretchability of substrate materials (Feiner and Dvir, 2018).
Cellulose biopolymers and their derivatives have shown high performance (e.g., high Young’s modulus, excellent thermal stability, low coefficient of thermal expansion as well as high strength, stiffness and adsorption ability, excellent degradation properties and biocompatibility) (Fu et al., 2016; Yu et al., 2018) and become the most widely used synthetic degradable polymer substrate materials (Kamel and Khattab, 2020). Diverse modifications of cellulose biopolymers for water solubility, electrical conductivity, optical transparency, and gas tightness have led to successful applications in a variety of emerging fields such as bioresorbable electronics (Zhang and Bellan, 2017), energy storage devices (Yu et al., 2018), and optoelectronic devices (Morsada et al., 2021).
Widely used synthetic polymers and their copolymers (e.g., PVA, PCL, PGA, PLA, and PLGA) have adjustable degradation rates and biocompatibility, but lack tensile properties and flexibility that are compatible with human tissues (Liu et al., 2012; Ilyas et al., 2022b). Unlike traditional biodegradable polymers, degradable bioelastomers require chemical and/or physical cross-linking to form a three-dimensional cross-linked network structure which allows them to typically have a glass transition temperature (Tg) below body temperature, high elongation at break, and a fully reversible stress-strain curve (Drotleff et al., 2004). Physically cross-linked degradable bioelastomers are known as thermoplastic degradable bioelastomers, and chemically cross-linked degradable bioelastomers are also known as thermosetting degradable bioelastomers, which mainly include thermosetting and light-curing bioelastomers. Polyglyceryl sebacate (PGS) provides biodegradability and elasticity as a thermosetting polyol/dicarboxylic acid-based bioelastomer with excellent mechanical properties (Shin et al., 2003). Another thermosetting elastomeric polymer, POC, offers excellent mechanical properties (higher tensile strength, modulus of elasticity) and adjustable degradability (Krook et al., 2020). More physical and chemical properties of typical degradable elastomers are shown in Table 1.
TABLE 1. Representative physicochemical properties of typical thermosetting, photocuring, thermoplastic degradable bioelastomers.
The basic material types for basic electronic components including diodes, transistors, capacitors and inductors are substrates, dielectrics, semiconductors and conductors. Organic polymer chemistry can be processed to meet the electronic needs of these materials while adjusting for the desired degradation kinetics, stretchability and scalability of production (Feig et al., 2018). Since most polymers are natural insulators, they have been developed as substrates and dielectrics. Whereas conjugated conducting polymers act as semiconductors or conductors. Designing stretchable semiconductor materials, especially conducting polymer materials, has become increasingly challenging because the polymer backbone capable of facilitating electron transport through alternating double and single bonds is usually rigid (Liu et al., 2020). However, recent advances in controlling the chemistry and morphology of conjugated polymers have made it possible to develop stretchable semiconductors and conductors.
Substrate and packaging materials typically make up the majority of the weight of the device, and as such, they largely determine the overall degradation behavior of the device (Hwang et al., 2014; Hadidi et al., 2022). Clearly, the selection of substrate and package with the desired degradation characteristics is critical to the design of biodegradable devices. The range of biodegradable insulating materials that can be used as substrates is limited by compatibility with the device fabrication process steps, requiring consideration of thermal stability, solvent compatibility, and mechanical robustness (Hwang et al., 2014). To circumvent these conditions, the target substrate can be separated from the processing step by transferring the device to the substrate after fabrication.
As an example of this strategy (Figure 4A), Hwang and his colleagues demonstrated a versatile transfer printing method to fabricate complementary metal oxide semiconductor (CMOS) arrays on a variety of synthetic biodegradable substrates (Hwang et al., 2014), such as PLGA, PCL, and rice paper. The authors used this technique to fabricate a transient hydration sensor made of soluble silicon, SiO2, and Mg components on PLGA that can be used to monitor wound healing, Similar transfer-based strategies have been reported for transient silicon-based devices on PVA, silk, and cellulose (Jin et al., 2014; Jung et al., 2015; Fu et al., 2016). In another work, Hwang and his colleagues used transfer printing methods to fabricate silicon-based micro heaters on silk that degrade after 15 days for transient heat therapy to prevent post-surgical infection (Figure 4B) (Hwang et al., 2012). Bao and colleagues recently fabricated an ultrathin flexible CMOS circuit using trimethylsilyl-functionalized cellulose as a substrate and exhibited complete degradation properties within 1 month (Figure 4C) (Lei et al., 2017).
FIGURE 4. Degradable polymer substrates and packaging used for application demonstration. (A) Scheme of the general transfer process of devices fabricated on a temporary silicon substrate to a final degradable substrate. This transfer method enables a wide choice of substrate materials. Reproduced permission from 2014, Wiley (Hwang et al., 2014). (B) (i) Silicon-based microheater on a thin silk substrate. (ii) Exploded view with top view in the lower right inset. (iii) Image shows the time sequence of dissolution in deionized water, with complete decomposition at about 10 min. Reproduced permission from 2012, AAAS (Hwang et al., 2012). (C) (i) Flexible device using a degradable polymer as the active material or substrate. (ii) Photographs of devices in various stages of disintegration. Reproduced permission from 2017, National Academy of Sciences (Lei et al., 2017).
Considering that implanted devices need to adhere tightly around the brain or heart and make stable conformal contact with dynamic biological tissues, more flexible and stretchable substrate materials are required. Stretchable transient Si-based pH and electrophysiological sensors are manufactured from POC using transfer printing, and complete dissolution is observed after immersion in PBS (pH 10)12 at room temperature (Hwang et al., 2015). For such elastomers, photocrosslinking by the introduction of maleic anhydride can also be used to avoid prolonged heat shrinkage and curing (Tran et al., 2010). Similar strategies can be used with other synthetic degradable polymers to expand the range of materials that can be used to make stretchable and degradable substrates.
Many stretchable and biodegradable materials used as sealants and substrates may also qualify as dielectrics due to their insulating properties, but the dielectric properties of many polymers have not been fully explored. Currently, the general strategy for creating biodegradable dielectrics is to incorporate high-κ fillers into biodegradable polymeric matrices (Deshmukh et al., 2017). Common high-κ materials include metal oxides (Al2O3, HfO2), SiO2 and carbon nanotube materials (Figure 5A) (Gupta and Gupta, 2009; Zeng et al., 2016), etc. These composites exhibit general transients through matrix degradation while achieving tunable dielectric constants.
FIGURE 5. Design and functional realization of biodegradable dielectrics, semiconductors and conductors. (A) The dielectric constants of degradable composites can be increased by adding high κ-additive carbon nanotubes (CNTs). Reproduced permission from 2016, Royal Society of Chemistry (Zeng et al., 2016). (B) A fully biodegradable capacitive pressure sensor consisting of PGS’s pyramidal microstructure. Reproduced permission from 2015, Wiley (Boutry et al., 2015). (C) Topographical and current AFM image for 40: 60 TPU: P3TMA. Reproduced permission from 2014, Royal Society of Chemistry (Pérez-Madrigal et al., 2014). (D) DPP polymers with imine bonds and PCL-based elastomers. Reproduced permission from 2019, American Chemical Society (Tran et al., 2019). (E) SEM images of PPy-coated PLGA nanofiber network. Reproduced permission from 2009, Elsevier (Lee et al., 2009). (F) Undoped conductive polyurethane (DCPU) containing aniline oligomers. Reproduced permission from 2016, Springer Nature (Xu et al., 2016).
Plant-based fibers (e.g., cotton, jute, bamboo) and sugars such as glucose and lactose are natural polymers that inherently have practical dielectric properties. OFETs fabricated with sugar dielectrics and fullerenes as semiconductors show a small hysteresis phenomenon (Irimia-Vladu et al., 2010). In addition to natural materials, synthetic biodegradable polymers, such as PGS, also exhibit useful dielectric properties. As shown in Figure 5B, Bao and her colleagues reported the implementation of fully degradable capacitive pressure sensors using pyramidal microstructures of PGS with in vivo degradation rates of approximately 0.2–1.5 mm per month (Boutry et al., 2015). Additionally, it should be noted that most of the aforementioned dielectrics have been studied at relatively low operating frequencies (<1 kHz). For eventual use in more complex electronic devices, additional research and optimization of the high frequency performance of biodegradable dielectrics is needed.
Conductive polymers (CPs) are synthetic macromolecules with highly discrete π-conjugated backbone structures and configurable side chains. Some of the most common CPs include polypyrene (PPy), polyaniline (PANi), polythiophene (PTh), poly (3,4-ethylenedioxythiophene) (PEDOT) and their derivatives (Kenry and Liu, 2018; Bao et al., 2022). Similar to dielectric composites, methods for achieving degradable conductive polymers can be divided into two main categories. The first category of degradable conductors includes composites of non-degradable conductive polymers with electrically insulating degradable polymers. The second category of degradable conductors includes conductive oligomers, or degradable conductive polymers by incorporation based on the incorporation of modified monomers, degradable monomer units and conjugated joints.
Biodegradable conductive scaffolds are formed by electrostatically spinning biodegradable polymers and subsequently polymerizing conductive monomers in situ (Figure 5E) (Lee et al., 2009). Alternatively, conductive polymers can be co-spun with biodegradable polymers to form conductive fibers. For example, co-spinning of camphorsulfonic acid (CPSA)-doped polyaniline with gelatin yields fiber sheets with conductivity up to 2.1 × 10–2 S/cm (Li et al., 2006). Similarly, CPSA-doped PANI co-spun with poly (L-lactide-co-ε-caprolactone) (PLCL) exhibited electrical conductivity up to 1.38 × 10–2 S/cm at 30 wt% PANI (Jeong et al., 2008).
To confer better degradability and faster body clearance to conductive biodegradable polymers, small-sized short chains of conductive monomers, i.e., conductive oligomers, are increasingly being explored as viable alternatives to CPs as conductive components of electroactive biodegradable copolymers (Wei and Faul, 2008). In fact, studies on pyrrole, aniline and thiophene oligomers have shown that in addition to having similar, these oligomers can be processed and copolymerized more readily with biodegradable polymers and show better biodegradability (Wang et al., 2011; Guo et al., 2013). Most importantly, these oligomers can be more readily absorbed and internalized by macrophages and then excreted by the kidneys. Thus, conductive oligomers have emerged as a viable option for achieving fully biodegradable and conductive polymer structures (Kenry and Liu, 2018).
In addition to biocompatibility issues, the mobility of the dopant can be a problem for applications requiring relatively long-term stability, as the conductivity decreases significantly when the dopant leaches. To address this issue, Xu and coworkers synthesized a multi-segmented undoped conductive polyurethane (DCPU) elastomer consisting of an aniline trimer linked to biodegradable PCL and the dopant dihydroxymethylpropionic acid (DMPA) (Figure 5F) (Xu et al., 2016). Immersion in PBS further increases the conductivity up to 4.7 × 10–3 S/cm. Crucially, although their polymers degraded to 75.8% of their original mass in PBS after 14 days in the presence of lipase, the electrical conductivity did not decrease by more than one order of magnitude.
In addition to biodegradable conductive polymer blends and oligomer-based conductive approaches, strategies for the modification of conductive monomers and the integration of degradable monomer units or conjugated joints to produce bioerodible CPs are receiving increasing attention. Instead of partial degradation based on conventional chemical bond breaking, these modified CPs can be gradually eroded to achieve enhanced and complete biodegradation. One of the first studies demonstrating this concept showed that pyrrole monomers could be modified and then polymerized to achieve readily erodible PPy (Zelikin et al., 2002). The conductive films made from acid-functionalized PPy show a low average resistance of about 300 Ω. Importantly, the modified PPy is capable of progressive degradation under physiological conditions. This suggests that the degradation rate of these erodible CPs can be modified by introducing and adjusting the amount of different functional groups.
In addition to monomer modification and integration of biodegradable units, a recent example shows that fully erodible CPs can be prepared by incorporating degradable conjugate linkages. Amine bonds (-C=N-) were used as conjugate linkages to synthesize biodegradable conductive PDPP-PD polymers with an average hole mobility of about 4.2 × 10−2 –3.4 × 10−1 cm2/Vs (Lei et al., 2017). More significantly, the PDPP-PD solutions decomposed to the monomer DPP-CHO after 10 days and completely after 40 days under acidic conditions, based on their absorption spectra and physical colors.
So far, the conductivity of type II conductive polymers may be lower than that of type I conductive polymers (Table 2). Better control of the chemistry, doping, and morphology of these materials could help improve the conductivity of type II conducting polymers for high-performance electronics.
Semiconductors are critical to the switching mechanism of organic transistors and therefore to complex electronic circuits. They are usually characterized by the charge carrier mobility, which indicates the rate of free charge movement through the material when pulled by an electric field. Typical semiconductor polymers are polythiophene (e.g., poly (3-hexylthiophene), P3HT) (Bao et al., 1996) and donor-acceptor copolymers originally developed for organic photovoltaics (e.g., diketopyrrolopyrrole, DPP) (Bao et al., 1996). In organic systems, charge transport occurs between and among the conjugated backbones. As with conducting polymers, copolymerization has been used to produce semiconductors that exhibit type I degradation. As shown in Figure 5C (Pérez-Madrigal et al., 2014), poly (3-thiophene methyl acetate) (P3TMA), a P3HT derivative with a carboxylic acid substituent, was selected for use with thermoplastic hybrid polyurethanes (TPUs) showing enhanced semiconductor behavior and electrochemical degradability. Lipomi reported a stretchable and biodegradable semiconductor-a block copolymer based on repeating rigid, conjugated DPP and soft poly (s-caprolactone) (PCL) chain segments-demonstrating improved strain tolerance and a near hole mobility of 0.1 cm2/Vs (Sugiyama et al., 2018). The charge mobility was correlated with up to PCL polyester chains provide hydrolysis sites for chain breakage under physiological conditions, with no detectable PCL content after 12 weeks.
A combination of polymer chemistry and physics was used to develop an intrinsically stretchable and fully degradable semiconductor polymer system. As shown in Figure 5D (Tran et al., 2019), The semiconductor part is the DPP polymer with imine bonds, while the elastomer is based on PCL, where both matrix and semiconductor are designed to completely degrade into monomeric components. Nano-constrained p (DPP-PPD) showed no change in mobility (∼0.03 cm2/Vs) at up to 100% strain and subsequent release.
To avoid the potential problems of non-degradable implantable electronics in biomedical applications that interfere with imaging, act as foci for bacterial infection, or migrate and displace, bioresorbable implantable electronics have been extensively investigated as an alternative to permanent implants (Wang et al., 2020a). System-level absorbable devices for biomedical applications can be divided into four main application scenarios: sensing and monitoring, therapeutic drug delivery, wireless power supply, and integrated electronics (e.g., electronic skin). Each category is discussed in detail below.
Bioresorbable sensors have been widely reported because physicians often need to track various physiological indicators of patients, especially after surgery, for a short period of time (Salvatore et al., 2017; Lee et al., 2019a; Ashammakhi et al., 2021). Recently reported examples include bioresorbable temperature sensors, chemical/mechanical sensors, and electrophysiological signal sensors. For example, Kim’s group (Figure 6A) (Lee et al., 2019a) reports a biodegradable, integrated drug delivery platform consisting of a temperature sensor, a polymeric drug reservoir, and a heater for controlled intracranial drug delivery via thermal stimulation, achieving enhanced therapeutic efficacy for brain tumor treatment. The device dissolved after a sustained 10-weeks in vivo drug release, leaving no detectable debris or causing side effects. A recent study demonstrated two types of bioresorbable fiber optic pressure sensors for monitoring intracranial pressure and temperature with optical interfaces made of PLGA fibers (Shin et al., 2019), and in vitro solubility studies and histopathological evaluations confirmed the biodegradability of these complete systems.
FIGURE 6. Typical applications of transient electronics in the field of sensors. (A) (i) Bioabsorbable Electron Patch (BEP) image with temperature sensor. (ii) Wireless heater degradation images at 37°C (0–14 days). Reproduced permission from 2019, Springer Nature (Lee et al., 2019a). (B) (i) Illustration and equivalent circuit of the capacitive pressure sensor. (ii) Results at 3 months post-implantation. Scale bar. 100 μm. Reproduced permission from 2019, Springer Nature (Boutry et al., 2019). (C) (i) Schematic diagram of a transient NO sensor consisting of a bioresorbable PLLA-PTMC substrate, Au nanomembrane electrode, and poly (eugenol) film. (ii) The manufacturing process of the NO sensor. (iii) Time varied degradation images. Reproduced permission from 2020, Springer Nature (Li et al., 2020e).
Another fully biodegradable pressure sensor aimed for measuring arterial blood flow in both contact and non-contact modes, as shown in Figure 6B (i) were also recently reported by Bao’s group (Boutry et al., 2019). One week after implantation around the femoral artery in rats, stable operation of the biodegradable sensor was observed. Twelve weeks after implantation, the rats were able to move without any apparent limb damage, after which the sensors were retrieved and it was observed that all sensor components, including the poly [octamethylene maleate (anhydride) citrate] (POMaC) sealing layer, PGS microstructure pyramid, magnesium wire and PLLA membrane, had degraded and only the PHB/HV remained Figure 6B (ii).
Pressure and temperature sensors represent the most studied bioresorbable physical sensors to date. However, several new hot research areas have recently emerged, including biodegradable sensors targeting dopamine, ROS/RNS, and RNA (Tao et al., 2012; Kim et al., 2018; Lee et al., 2019a). For applications in the central nervous system, biodegradable soft neurotransmitter sensors have been explored for continuous and real-time monitoring of dopamine levels in the brain and have demonstrated their usefulness as electrochemical sensors (Kim et al., 2018). All components of the sensors were gradually dissolved in PBS at 37°C over 15 h. Real-time monitoring of ROS and RNS in organisms has attracted a lot of attention. A flexible physical transient electrochemical sensor has been used for real-time wireless nitric oxide monitoring Figure 6C (i) (Li et al., 2020e). The combination of a highly stretchable matrix, ultrathin electrodes, and selective membranes gives the sensor ideal flexibility and stretchability and resistive stability, and these materials are fully bioresorbable. The NO sensor completely disappeared after 8 weeks of implantation into the joint cavity. Hematoxylin-eosin (HE) staining of the implantation site tissue showed no obvious signs of inflammation or any residual PLLA-PTMC matrix and gold nanomembrane electrodes, successfully achieving complete physical transient.
Biodegradable therapeutic devices have gained tremendous momentum in the last decade, thanks to advances in modulation processes and novel processing technologies for flexible, stretchable degradable materials (Long et al., 2018; Cha et al., 2019; La Mattina et al., 2020; Wei et al., 2021). In 2014, Omenetto and colleagues demonstrated a programmable transient thermal therapy system for surgical site disinfection and anti-infection using a silk substrate and encapsulation with magnesium metal as the heating resistor (Figure 7A) (Tao et al., 2014). The lifetime of the entire device can be further tuned by the encapsulation material, allowing the device to dissolve completely within minutes to weeks. In 2015, a thermal actuator, consisting of Mo electrodes (Lee et al., 2015a), thin PLGA scaffolds, and biolipid membranes, was fully degradable in vivo and successfully implemented to stimulate target tissues or drive drug delivery devices in response to dynamic changes monitored by integrated sensors (Figure 7B). As shown in Figure 7E (Lee et al., 2019a), to treat cancer in organs with special blood barriers, such as the brain, peritoneum, and eye, Kim et al. (2018) reported a flexible, adhesive Bioabsorbable Electron Patch (BEP) consisting of a hydrophilic drug-carrying oxidized starch (OST) membrane and a hydrophobic PLA encapsulated membrane. The synergistic effects and degradable properties for brain tumor treatment by integrating all these materials and device components were demonstrated in vivo mouse subcutaneous and canine brain GBM models.
FIGURE 7. Typical applications for biodegradable therapeutic electronics. (A) Silk-based device for wirelessly activated drug release. Reproduced permission from 2014, National Academy of Sciences (Tao et al., 2014). (C) Thermally responsive drug nanocarriers induced by the integrated flexible heater. Reproduced permission from 2017, Springer Nature (Tamayol et al., 2017). (E) Bioabsorbable electronic patch (BEP) consists of OST film and PLA encapsulated film. Reproduced permission from 2019, Springer Nature (Lee et al., 2019a). (B) (D) (F–H) A series of fully bioresorbable implantable therapeutic devices from Rogers’ team within the past few years, including radio stimulators for recovery of peripheral nerve injury, drug delivery systems, and pacemakers. Reproduced with permission (Lee et al., 2015a; Koo et al., 2018; Choi et al., 2020; Koo et al., 2020; Choi et al., 2021). Copyright 2015 Springer Nature, 2018 Springer Nature, 2020 Springer Nature, 2020 AAAS and 2021 Springer Nature.
Rogers and colleagues have reported a series of fully bioresorbable implantable therapeutic devices within the last few years, including a fully degradable radiostimulator for accelerated functional recovery from peripheral nerve injury (Figures 7C,D,F) (Koo et al., 2018; Choi et al., 2020), a drug delivery system consisting of mechanically stabilized PBTPA containers (Figure 7G) (Koo et al., 2020) and a recently reported fully implantable and bioresorbable pacemaker without leads or batteries (Figure 7H) (Choi et al., 2021), which are in vitro and in vivo complete degradability have been demonstrated, thus attracting widespread interest and attention.
The pollution caused by e-waste and the toxic effects of electronic materials on humans should draw attention to the sustainable development of flexible electronics and the environment. Bioresorbable energy storage devices are integral components of bioresorbable electronic implants because they eliminate the need for external power lines (Li et al., 2018c; Li et al., 2020f). Arranging and integrating efficient power sources for biodegradable and bioresorbable devices is a daunting step. In general, materials and structural design are the primary methods for redesigning typical power devices into biocompatible, miniaturized systems.
Batteries provide high energy density and immediate readiness and are therefore the most widely used power source (Li et al., 2018a; Chao et al., 2021). Transient primary cells, as representative primary cells, can generate currents with spontaneous redox reactions through the redox potential difference between the biodegradable anode and cathode. However, the need for frequent replacement or recharging, the great sacrifice of performance to reduce size, and possible biological toxicity pose significant challenges for biodegradable implantable batteries (Fu et al., 2015). Among various energy storage devices, compared with batteries, high-performance supercapacitors are of great interest because of their high-power density, good cycling stability, fast charge and discharge rates, and simple structure.
As shown in Figure 8A Lee et al., (2017a), reported a transient micro-supercapacitor consisting of a biodegradable Mo film, a biopolymer hydrogel electrolyte containing NaCl salts (agarose gel), and a PLGA substrate with a PA encapsulation layer. The performance of the device is comparable to that of non-transient devices. The molybdenum forked finger electrode was completely degraded after 9 days in PBS at pH 7.4 at 37°C and showed stable performance before degradation. Chen et al. (2019) demonstrated an all-wood asymmetric supercapacitor (ASC) wood carbon (MnO2 @WC) (Figure 8B) (Zhao et al., 2017). The all-wood ASC device can biodegrade in the environment and provide a significantly high capacitance of 3.6 F cm−2, mainly due to the carbonized and direct-channel wood with high electronic and ionic conductivity and low curvature.
FIGURE 8. Typical applications of biodegradable power supply equipment. (A) NaCl/agarose gel electrolyte planar supercapacitor on a glass substrate. Reproduced permission from 2017, Wiley (Lee et al., 2017a). (B) Schematic diagram of a stretchable serpentine wire supercapacitor. Reproduced permission from 2017, Wiley (Zhao et al., 2017). (C) Optical photographs of mesoporous cellulose membrane-based planar-type micro-supercapacitors. Reproduced permission from 2019, Elsevier (Lee et al., 2019b). (D) Schematic and electrostatic immunity mechanism of fully degradable rechargeable Zn-Mxene capacitor. Reproduced permission from 2019, American Chemical Society (Yang et al., 2019). (E) Biodegradable PLA/PVA and/or starch paper are used for the package edges and exterior. Reproduced permission from 2021, AAAS (Sheng et al., 2021). (F) Milestones of TENG using degradable materials as frictional electrical layers. Reproduced permission from 2020, Wiley (Chao et al., 2021). (G) (i) Schematic and photos of BD-TENG; (ii) Photographs of BD-TENG at different stages of the degradation timeline. Reproduced permission from 2016, AAAS (Zheng et al., 2016). (H) (i) Schematic diagram of T2ENGs; (ii) T2ENG gradually dissolved in DI water. Reproduced permission from 2016, Wiley (Zheng et al., 2016).
As shown in Figure 8C (Lee et al., 2019b), highly flexible biodegradable POC can also be used as an encapsulation layer for supercapacitors, providing good mechanical and electrochemical stability in aqueous solutions. The introduction of microstructure engineering strategies can greatly improve the cycling performance of Zn-Mxene supercapacitors and facilitate the controlled adjustment of device degradation time Figure 8D (Yang et al., 2019). Similarly, as shown in Figure 8E (Sheng et al., 2021), fully biodegradable supercapacitor implants with two-dimensional, defective amorphous MoOx flakes grown in situ on water-soluble molybdenum foil as electrodes and sodium alginate (Alg-Na) gel as electrolyte have high area capacitance (112.5 mF cm−2 at 1 mA cm−2) and excellent energy density (15.64 μWh cm−2)/high power density (2.53 mW cm−2), and their lifetimes can be designed for a few days to several weeks (Sheng et al., 2021).
In recent years, nanogenerators (NGs) have begun to emerge as a new technology for converting biomechanical energy into electrical energy (Figure 8F) (Li and Wang, 2017; Li et al., 2018d; Long et al., 2019; Li et al., 2020b; Chao et al., 2021). Figure 8G Shows a transient friction nanogenerator consisting of PLGA, PVA, PCL, and poly (3-hydroxybutyric-co-3-hydroxyvaleric acid) (PHB/V) (Zheng et al., 2016). The friction generator loses its structural integrity and degrades after 50 days at pH 7.4 PBS and 37°C. Tao et al. reported a suite of silk-based multifunctional implantable transient triboelectric nanogenerators (T2ENGs) for remote in vivo biomedical sensing/monitoring and “smart” therapies automatically triggered by specific symptoms (i.e., epilepsy monitoring) (Figure 8H) (Zhang et al., 2018). Due to the remarkable frictional electrical properties of the silk, the implanted T2ENGs device provides a stable output of ∼6 V for a relatively long period of 6 h. Local injection of 10 ml of saline into the implanted area triggers rapid degradation and loss of frictional electrical function within 30 min.
By integrating suitable bioresorbable device components, bioresorbable integrated electronic systems can be fabricated as promising solutions to various clinical challenges (Choi et al., 2016a; Choi et al., 2016b; Lin et al., 2016; Li et al., 2021b). Bioresorbable stents have been developed as a potential solution to address in-stent restenosis (Bai et al., 2019). Recently, (Figure 9A) Son et al. (2015) proposed a multifunctional bioresorbable electronic stent that provides postoperative monitoring and therapeutic functions in addition to the original functions of conventional bioresorbable stents (Son et al., 2015). The proposed bioresorbable electronic stent is equipped with a bioresorbable flow sensor for monitoring blood flow and a bioresorbable heater and temperature sensor for controlling postoperative drug delivery. The entire system consists of bioresorbable materials, so the complete biodegradation of the stent reduces the possibility of restenosis. Future research directions for biodegradable integrated electronic systems, including bioresorbable electric scaffolds and postoperative monitoring systems, may lie in the integration of various sensors, memories, wirelessly powered devices, and even therapeutic nanoparticles (Lee et al., 2015b; Lee et al., 2017b; Cha et al., 2019).
FIGURE 9. (A)Schematic diagram of a bioresorbable stent for endovascular disease. Reproduced permission from 2015, American Chemical Society (Son et al., 2015). (B) Schematic diagram of a dual bionic multifunctional self-powered e-skin with layered nanostructures of spider webs and ant tentacles. Reproduced permission from 2021, Wiley (Yue et al., 2021).
The human skin has a variety of functions, such as sensing external stimuli, giving flexibility to joint or muscle movement, protecting vital organs from injury, and performing autonomous self-repair (Wang et al., 2018). Further research in skin-inspired integrated electronics will contribute to the great development of electronic skin for prosthetics (Wang et al., 2018; Zou et al., 2018). In 2021, self-powered, sensitive, flexible, breathable, biodegradable, intelligent, and integrated e-skin with a multi-layered, all-nanofibers (NFs) structure was successfully developed (Figure 9B) (Yue et al., 2021). In addition, the e-skin is used to efficiently harvest biomechanical energy and monitor whole-body physiological signals of pressure, temperature, and humidity. Thanks to the complete NFs structure, the e-skin has a breathability of 20.87 ms−1, guaranteeing comfort when worn. This study combines versatile, sensitive and self-powered materials to ensure an intelligent and integrated e-skin to detect various physiological or environmental signals. It even ensures permeability and biodegradability, thus helping to promote more practical and environmentally friendly applications of e-skins in human-machine interfaces and artificial intelligence (Yue et al., 2021).
The rapid development of bioresorbable materials and the understanding of their dissolution behavior have led to the invention of numerous high-performance bioresorbable electronic devices with multiple applications in the last few years. Despite the rapid development and remarkable achievements in bioresorbable electronics, there are some potential problems and challenges, and the main challenges and possible solutions are listed below.
1. Degradation mechanism studies and modulation of degradation behavior. Although a large set of organic and inorganic biomaterials have been studied over the past 30 years, the diversity of degradation behaviors of materials has limited the progress of research. The majority of degradable implantable devices currently utilize simple hydrolysis decomposition mechanisms, and the influence of multiple chemical components in the in vivo microenvironment on the decomposition of implantable materials should be investigated in more depth to expand the application of degradable materials.
2. Innovative chemistry and processing technologies. First, functional polymers from organic sources should be emphasized in future research in order to develop fully organic flexible, stretchable and biocompatible bioelectronics. Further, natural abundance, renewable and intrinsic biocompatible natural polymers should be explored to enrich the field of sustainable healthcare monitoring and green electronics.
There are more areas of concern in the existing research on degradable electronics. In terms of biosafety, attention needs to be paid to the effects of degradation byproducts on humans, which requires relevant clinical studies to investigate potential concomitant chronic or acute diseases from degradation byproducts. In terms of device processing, there are often difficulties in combining new materials with existing technologies or products, and the more critical challenge is also to achieve integration of multiple components in a device without compromising system functionality and device size. Finally, wirelessly powered components should also be considered for inclusion in the overall system considerations.
A systematic research approach could accelerate the progress of future biodegradable bioelectronics research. For designing polymeric materials with specific electronic properties (semiconductors, conductors), new possibilities exist to utilize unconventional degradable connections. Since most degradable materials are studied from different geographic environments in nature, for future work it would be interesting to consider specific microenvironments in the human body that favor decomposition mechanisms other than simple hydrolysis. For example, mechanisms that degrade polymers using highly reactive oxygen and nitrogen radical species produced by macrophages upon encountering foreign bodies. Further understanding of the chemical composition of different natural and physiological environments could open the door to biodegradable polymeric materials that are currently not fully explored in flexible bioresorbable electronic systems.
ZZ researched the data and wrote the manuscript. YL wrote parts of the manuscript and edited the manuscript. XD and HZ reviewed and supervised the writing of the manuscript. All authors contributed to the article and approved the submitted version.
This work was supported by the Sichuan Provincial Health Commission (Grant No. 17PJ108).
The authors declare that the research was conducted in the absence of any commercial or financial relationships that could be construed as a potential conflict of interest.
All claims expressed in this article are solely those of the authors and do not necessarily represent those of their affiliated organizations, or those of the publisher, the editors and the reviewers. Any product that may be evaluated in this article, or claim that may be made by its manufacturer, is not guaranteed or endorsed by the publisher.
Amass, W., Amass, A., and Tighe, B. (1998). A review of biodegradable polymers: Uses, current developments in the synthesis and characterization of biodegradable polyesters, blends of biodegradable polymers and recent advances in biodegradation studies. Polym. Int. 47 (2), 89–144. doi:10.1002/(sici)1097-0126(1998100)47:2<89::aid-pi86>3.0.co;2-f
Anderson, J. M., and Shive, M. S. (2012). Biodegradation and biocompatibility of PLA and PLGA microspheres. Adv. Drug Deliv. Rev. 64, 72–82. doi:10.1016/j.addr.2012.09.004
Ashammakhi, N., Hernandez, A. L., Unluturk, B. D., Quintero, S. A., Barros, N. R., Hoque Apu, E., et al. (2021). Biodegradable implantable sensors: Materials design, fabrication, and applications. Adv. Funct. Mat. 31 (49), 2104149. doi:10.1002/adfm.202104149
Bai, W., Shin, J., Fu, R., Kandela, I., Lu, D., Ni, X., et al. (2019). Bioresorbable photonic devices for the spectroscopic characterization of physiological status and neural activity. Nat. Biomed. Eng. 3 (8), 644–654. doi:10.1038/s41551-019-0435-y
Bao, Y. Y., Paunovic, N., and Leroux, J. C. (2022). Challenges and opportunities in 3D printing of biodegradable medical devices by emerging photopolymerization techniques. Adv. Funct. Mat. 32 (15), 2109864. doi:10.1002/adfm.202109864
Bao, Z., Dodabalapur, A., and Lovinger, A. J. (1996). Soluble and processable regioregular poly(3-hexylthiophene) for thin film field-effect transistor applications with high mobility. Appl. Phys. Lett. 69 (26), 4108–4110. doi:10.1063/1.117834
Bonartsev, A. P., Boskhomodgiev, A. P., Iordanskii, A. L., Bonartseva, G. A., Rebrov, A. V., Makhina, T. K., et al. (2012). Hydrolytic degradation of poly(3-hydroxybutyrate), polylactide and their derivatives: Kinetics, crystallinity, and surface morphology. Mol. Cryst. Liq. Cryst. 556, 288–300. doi:10.1080/15421406.2012.635982
Boutry, C. M., Beker, L., Kaizawa, Y., Vassos, C., Tran, H., Hinckley, A. C., et al. (2019). Biodegradable and flexible arterial-pulse sensor for the wireless monitoring of blood flow. Nat. Biomed. Eng. 3 (1), 47–57. doi:10.1038/s41551-018-0336-5
Boutry, C. M., Nguyen, A., Lawal, Q. O., Chortos, A., Rondeau-Gagne, S., and Bao, Z. (2015). A sensitive and biodegradable pressure sensor array for cardiovascular monitoring. Adv. Mat. 27 (43), 6954–6961. doi:10.1002/adma.201502535
Brannigan, R. P., and Dove, A. P. (2017). Synthesis, properties and biomedical applications of hydrolytically degradable materials based on aliphatic polyesters and polycarbonates. Biomater. Sci. 5 (1), 9–21. doi:10.1039/c6bm00584e
Bruggeman, J. P., de Bruin, B. J., Bettinger, C. J., and Langer, R. (2008). Biodegradable poly(polyol sebacate) polymers. Biomaterials 29 (36), 4726–4735. doi:10.1016/j.biomaterials.2008.08.037
Bus, T., Englert, C., Reifarth, M., Borchers, P., Hartlieb, M., Vollrath, A., et al. (2017). 3rd generation poly(ethylene imine)s for gene delivery. J. Mat. Chem. B 5 (6), 1258–1274. doi:10.1039/c6tb02592g
Can, E., Udenir, G., Kanneci, A. I., Kose, G., and Bucak, S. (2011). Investigation of PLLA/PCL blends and paclitaxel release profiles. AAPS PharmSciTech 12 (4), 1442–1453. doi:10.1208/s12249-011-9714-y
Cao, Y., and Uhrich, K. E. (2018). Biodegradable and biocompatible polymers for electronic applications: A review. J. Bioact. Compatible Polym. 34 (1), 3–15. doi:10.1177/0883911518818075
Casas, J., Zhao, Q., Donovan, M., Schroeder, P., Stokes, K., and Untereker, D. (1999). In vitro modulation of macrophage phenotype and inhibition of polymer degradation by dexamethasone in a human macrophage/Fe/stress system. J. Biomed. Mat. Res. 46 (4), 475–484. doi:10.1002/(sici)1097-4636(19990915)46:4<475::aid-jbm5>3.0.co;2-j
Cha, G. D., Kang, D., Lee, J., and Kim, D. (2019). Bioresorbable electronic implants: History, materials, fabrication, devices, and clinical applications. Adv. Healthc. Mat. 8 (11), 1801660. doi:10.1002/adhm.201801660
Chang, M. M., Song, T., Liu, X., Lin, Q., He, B., and Ren, J. (2020). Cellulose-based biosensor for bio-molecules detection in medical diagnosis: A mini-review. Curr. Med. Chem. 27 (28), 4593–4612. doi:10.2174/0929867327666200221145543
Chao, S. Y., Ouyang, H., Jiang, D., Fan, Y., and Li, Z. (2021). Triboelectric nanogenerator based on degradable materials. Ecomat 3 (1), 19. doi:10.1002/eom2.12072
Chen, Y., Yu, L., Zhang, B., Feng, W., Xu, M., Gao, L., et al. (2019). Design and synthesis of biocompatible, hemocompatible, and highly selective antimicrobial cationic peptidopolysaccharides via click chemistry. Biomacromolecules 20 (6), 2230–2240. doi:10.1021/acs.biomac.9b00179
Chiong, J. A., Tran, H., Lin, Y., Zheng, Y., and Bao, Z. (2021). Integrating emerging polymer chemistries for the advancement of recyclable, biodegradable, and biocompatible electronics. Adv. Sci. (Weinh). 8 (14), 2101233. doi:10.1002/advs.202101233
Choi, C., Choi, M. K., Hyeon, T., and Kim, D. H. (2016). Nanomaterial-based soft electronics for healthcare applications. Chemnanomat 2 (11), 1006–1017. doi:10.1002/cnma.201600191
Choi, S., Lee, H., Ghaffari, R., Hyeon, T., and Kim, D. H. (2016). Recent advances in flexible and stretchable bio-electronic devices integrated with nanomaterials. Adv. Mat. 28 (22), 4203–4218. doi:10.1002/adma.201504150
Choi, Y. S., Hsueh, Y. Y., Koo, J., Yang, Q., Avila, R., Hu, B., et al. (2020). Stretchable, dynamic covalent polymers for soft, long-lived bioresorbable electronic stimulators designed to facilitate neuromuscular regeneration. Nat. Commun. 11 (1), 5990. doi:10.1038/s41467-020-19660-6
Choi, Y. S., Yin, R. T., Pfenniger, A., Koo, J., Avila, R., Benjamin Lee, K., et al. (2021). Fully implantable and bioresorbable cardiac pacemakers without leads or batteries. Nat. Biotechnol. 39 (10), 1228–1238. doi:10.1038/s41587-021-00948-x
Chou, H.-H., Nguyen, A., Chortos, A., To, J. W., Lu, C., Mei, J., et al. (2015). A chameleon-inspired stretchable electronic skin with interactive colour changing controlled by tactile sensing. Nat. Commun. 6 (1), 8011. doi:10.1038/ncomms9011
Deshmukh, K., Ahamed, M. B., Deshmukh, R. R., Pasha, S. K. K., Sadasivuni, K. K., Polu, A. R., et al. (2017). Newly developed biodegradable polymer nanocomposites of cellulose acetate and Al2O3 nanoparticles with enhanced dielectric performance for embedded passive applications. J. Mat. Sci. Mat. Electron. 28 (1), 973–986. doi:10.1007/s10854-016-5616-9
Ding, T., Liu, Q., Shi, R., Tian, M., Yang, J., and Zhang, L. (2006). Synthesis, characterization and in vitro degradation study of a novel and rapidly degradable elastomer. Polym. Degrad. Stab. 91 (4), 733–739. doi:10.1016/j.polymdegradstab.2005.06.007
Diouf-Lewis, A., Commereuc, S., and Verney, V. (2017). Toward greener polyolefins: Antioxidant effect of phytic acid from cereal waste. Eur. Polym. J. 96, 190–199. doi:10.1016/j.eurpolymj.2017.09.014
Drotleff, S., LUngwitz, U., BreunigM., , Dennis, A., Blunk, T., Tessmar, J., et al. (2004). Biomimetic polymers in pharmaceutical and biomedical sciences. Eur. J. Pharm. Biopharm. 58 (2), 385–407. doi:10.1016/j.ejpb.2004.03.018
Englert, C., Hartlieb, M., Bellstedt, P., Kempe, K., Yang, C., Chu, S. K., et al. (2015). Enhancing the biocompatibility and biodegradability of linear poly(ethylene imine) through controlled oxidation. Macromolecules 48 (20), 7420–7427. doi:10.1021/acs.macromol.5b01940
Feig, V. R., Tran, H., and Bao, Z. (2018). Biodegradable polymeric materials in degradable electronic devices. ACS Cent. Sci. 4 (3), 337–348. doi:10.1021/acscentsci.7b00595
Feiner, R., and Dvir, T. (2018). Tissue-electronics interfaces: From implantable devices to engineered tissues. Nat. Rev. Mat. 3 (1), 17076. doi:10.1038/natrevmats.2017.76
Feng, Z.-Q., Wu, J., Cho, W., Leach, M. K., Franz, E. W., Naim, Y. I., et al. (2013). Highly aligned poly(3, 4-ethylene dioxythiophene) (PEDOT) nano- and microscale fibers and tubes. Polymer 54 (2), 702–708. doi:10.1016/j.polymer.2012.10.057
Fu, K. K., Wang, Z., Dai, J., Carter, M., and Hu, L. (2016). Transient electronics: Materials and devices. Chem. Mat. 28 (11), 3527–3539. doi:10.1021/acs.chemmater.5b04931
Fu, K., Liu, Z., Yao, Y., Wang, Z., Zhao, B., Luo, W., et al. (2015). Transient rechargeable batteries triggered by cascade reactions. Nano Lett. 15 (7), 4664–4671. doi:10.1021/acs.nanolett.5b01451
Fukai, R., Dakwa, P. H. R., and Chen, W. (2004). Strategies toward biocompatible artificial implants: Grafting of functionalized poly(ethylene glycol)s to poly(ethylene terephthalate) surfaces. J. Polym. Sci. A. Polym. Chem. 42 (21), 5389–5400. doi:10.1002/pola.20352
George, G. A., and Celina, M. (2000). “Homogeneous and heterogeneous oxidation of polypropylene,” in Handbook of polymer degradation (CRC Press), Boca Raton, Florida, United States, 299–302.
Goonoo, N., Bhaw-Luximon, A., Rodriguez, I. A., Wesner, D., Schonherr, H., Bowlin, G. L., et al. (2015). Poly(ester-ether)s: III. Assessment of cell behaviour on nanofibrous scaffolds of PCL, PLLA and PDX blended with amorphous PMeDX. J. Mat. Chem. B 3 (4), 673–687. doi:10.1039/c4tb01350f
Guo, B., Finne-Wistrand, A., and Albertsson, A.-C. (2011). Degradable and electroactive hydrogels with tunable electrical conductivity and swelling behavior. Chem. Mat. 23 (5), 1254–1262. doi:10.1021/cm103498s
Guo, B., Finne-Wistrand, A., and Albertsson, A.-C. (2011). Facile synthesis of degradable and electrically conductive polysaccharide hydrogels. Biomacromolecules 12 (7), 2601–2609. doi:10.1021/bm200389t
Guo, B., Glavas, L., and Albertsson, A.-C. (2013). Biodegradable and electrically conducting polymers for biomedical applications. Prog. Polym. Sci. 38 (9), 1263–1286. doi:10.1016/j.progpolymsci.2013.06.003
Gupta, T. (2009). “Dielectric materials,” in Copper interconnect technology. Editor T. Gupta (New York, NY: Springer New York), 67–110.
Gyawali, D., Tran, R. T., Guleserian, K. J., Tang, L., and Yang, J. (2010). Citric-acid-derived photo-cross-linked biodegradable elastomers. J. Biomaterials Sci. Polym. Ed. 21 (13), 1761–1782. doi:10.1163/092050609x12567178204169
Hadidi, M., Jafarzadeh, S., Forough, M., Garavand, F., Alizadeh, S., Salehabadi, A., et al. (2022). Plant protein-based food packaging films; recent advances in fabrication, characterization, and applications. Trends Food Sci. Technol. 120, 154–173. doi:10.1016/j.tifs.2022.01.013
Heijkants, R. G. J. C., Calck, R. V. v., van Tienen, T. G., de Groot, J. H., Buma, P., Pennings, A. J., et al. (2005). Uncatalyzed synthesis, thermal and mechanical properties of polyurethanes based on poly(ε-caprolactone) and 1, 4-butane diisocyanate with uniform hard segment. Biomaterials 26 (20), 4219–4228. doi:10.1016/j.biomaterials.2004.11.005
Hosseini, E. S., Dervin, S., Ganguly, P., and Dahiya, R. (2021). Biodegradable materials for sustainable health monitoring devices. ACS Appl. Bio Mat. 4 (1), 163–194. doi:10.1021/acsabm.0c01139
Hou, Q., Grijpma, D. W., and Feijen, J. (2009). Creep-resistant elastomeric networks prepared by photocrosslinking fumaric acid monoethyl ester-functionalized poly(trimethylene carbonate) oligomers. Acta Biomater. 5 (5), 1543–1551. doi:10.1016/j.actbio.2008.12.012
Hu, M. M., Wang, J., Liu, J., Wang, P., Feng, Y., Wang, H., et al. (2019). A flour-based one-stop supercapacitor with intrinsic self-healability and stretchability after self-healing and biodegradability. Energy Storage Mater. 21, 174–179. doi:10.1016/j.ensm.2018.12.013
Huang, X. (2017). Bioresorbable materials and their application in electronics. Cambridge: Cambridge University Press.
Hwang, S.-W., Lee, C. H., Cheng, H., Jeong, J. W., Kang, S. K., Kim, J. H., et al. (2015). Biodegradable elastomers and silicon nanomembranes/nanoribbons for stretchable, transient electronics, and biosensors. Nano Lett. 15 (5), 2801–2808. doi:10.1021/nl503997m
Hwang, S.-W., Tao, H., Kim, D. H., Cheng, H., Song, J. K., Rill, E., et al. (2012). A physically transient form of silicon electronics. Science 337 (6102), 1640–1644. doi:10.1126/science.1226325
Hwang, S. W., Song, J. K., Huang, X., Cheng, H., Kang, S. K., Kim, B. H., et al. (2014). High-performance biodegradable/transient electronics on biodegradable polymers. Adv. Mat. 26 (23), 3905–3911. doi:10.1002/adma.201306050
Ilyas, R. A., Zuhri, M. Y. M., Aisyah, H. A., Asyraf, M. R. M., Hassan, S. A., Zainudin, E. S., et al. (2022). Natural fiber-reinforced polylactic acid, polylactic acid blends and their composites for advanced applications. Polymers 14 (1), 202. doi:10.3390/polym14010202
Ilyas, R. A., Zuhri, M. Y. M., Norrrahim, M. N. F., Misenan, M. S. M., Jenol, M. A., Samsudin, S. A., et al. (2022). Natural fiber-reinforced polycaprolactone green and hybrid biocomposites for various advanced applications. Polymers 14 (1), 182. doi:10.3390/polym14010182
Irimia-Vladu, M. (2014). Green” electronics: Biodegradable and biocompatible materials and devices for sustainable future. Chem. Soc. Rev. 43 (2), 588–610. doi:10.1039/c3cs60235d
Irimia-Vladu, M., Troshin, P. A., Reisinger, M., Shmygleva, L., Kanbur, Y., Schwabegger, G., et al. (2010). Biocompatible and biodegradable materials for organic field-effect transistors. Adv. Funct. Mat. 20 (23), 4069–4076. doi:10.1002/adfm.201001031
Jeong, C. G., and Hollister, S. J. (2010). Mechanical, permeability, and degradation properties of 3D designed poly(1, 8 octanediol-co-citrate) scaffolds for soft tissue engineering. J. Biomed. Mat. Res. B Appl. Biomater. 93B (1), 141–149. doi:10.1002/jbm.b.31568
Jeong, S. I., Jun, I. D., Choi, M. J., Nho, Y. C., Lee, Y. M., and Shin, H. (2008). Development of electroactive and elastic nanofibers that contain polyaniline and poly(L-lactide-co-epsilon-caprolactone) for the control of cell adhesion. Macromol. Biosci. 8 (7), 627–637. doi:10.1002/mabi.200800005
Jia, X. T., Wang, C., Zhao, C., Ge, Y., and Wallace, G. G. (2016). Toward biodegradable Mg-air bioelectric batteries composed of silk fibroin-polypyrrole film. Adv. Funct. Mat. 26 (9), 1454–1462. doi:10.1002/adfm.201503498
Jin, S. H., Shin, J., Cho, I. T., Han, S. Y., Lee, D. J., Lee, C. H., et al. (2014). Solution-processed single-walled carbon nanotube field effect transistors and bootstrapped inverters for disintegratable, transient electronics. Appl. Phys. Lett. 105 (1), 013506. doi:10.1063/1.4885761
Jung, Y. H., Chang, T. H., Zhang, H., Yao, C., Zheng, Q., Yang, V. W., et al. (2015). High-performance green flexible electronics based on biodegradable cellulose nanofibril paper. Nat. Commun. 6 (1), 7170. doi:10.1038/ncomms8170
Kamel, S., and Khattab, T. A. (2020). Recent advances in cellulose-based biosensors for medical diagnosis. Biosens. (Basel) 10 (6), 67. doi:10.3390/bios10060067
Kang, S.-K., Murphy, R. K. J., Hwang, S. W., Lee, S. M., Harburg, D. V., Krueger, N. A., et al. (2016). Bioresorbable silicon electronic sensors for the brain. Nature 530 (7588), 71–76. doi:10.1038/nature16492
Kenry, and B. Liu (2018). Recent advances in biodegradable conducting polymers and their biomedical applications. Biomacromolecules 19 (6), 1783–1803. doi:10.1021/acs.biomac.8b00275
Kim, H. S., Yang, S. M., Jang, T. M., Oh, N., and Hwang, S. W. (2018). Bioresorbable silicon nanomembranes and iron catalyst nanoparticles for flexible, transient electrochemical dopamine monitors. Adv. Healthc. Mat. 7 (24), 1801071. doi:10.1002/adhm.201801071
Koo, J., Kim, S. B., Choi, Y. S., Xie, Z., Bandodkar, A. J., Khalifeh, J., et al. (2020). Wirelessly controlled, bioresorbable drug delivery device with active valves that exploit electrochemically triggered crevice corrosion. Sci. Adv. 6 (35), eabb1093. doi:10.1126/sciadv.abb1093
Koo, J., MacEwan, M. R., Kang, S. K., Won, S. M., Stephen, M., Gamble, P., et al. (2018). Wireless bioresorbable electronic system enables sustained nonpharmacological neuroregenerative therapy. Nat. Med. 24 (12), 1830–1836. doi:10.1038/s41591-018-0196-2
Krook, N. M., Jaafar, I. H., Sarkhosh, T., LeBlon, C., Coulter, J. P., and Jedlicka, S. S. (2020). In vitro examination of poly(glycerol sebacate) degradation kinetics: Effects of porosity and cure temperature. Int. J. Polym. Mater. Polym. Biomaterials 69 (8), 535–543. doi:10.1080/00914037.2019.1596907
Kumar, M., Sundaram, S., Gnansounou, E., Larroche, C., and Thakur, I. S. (2018). Carbon dioxide capture, storage and production of biofuel and biomaterials by bacteria: A review. Bioresour. Technol. 247, 1059–1068. doi:10.1016/j.biortech.2017.09.050
La Mattina, A. A., Mariani, S., and Barillaro, G. (2020). Bioresorbable materials on the rise: From electronic components and physical sensors to in vivo monitoring systems. Adv. Sci. (Weinh). 7 (4), 1902872. doi:10.1002/advs.201902872
Lee, C. H., Kim, H., Harburg, D. V., Park, G., Ma, Y., Pan, T., et al. (2015). Biological lipid membranes for on-demand, wireless drug delivery from thin, bioresorbable electronic implants. NPG Asia Mat. 7 (11), e227. doi:10.1038/am.2015.114
Lee, G., Kang, S. K., Won, S. M., Gutruf, P., Jeong, Y. R., Koo, J., et al. (2017). Fully biodegradable microsupercapacitor for power storage in transient electronics. Adv. Energy Mat. 7 (18), 1700157. doi:10.1002/aenm.201700157
Lee, H., Lee, G., Yun, J., Keum, K., Hong, S. Y., Song, C., et al. (2019). Facile fabrication of a fully biodegradable and stretchable serpentine-shaped wire supercapacitor. Chem. Eng. J. 366, 62–71. doi:10.1016/j.cej.2019.02.076
Lee, H., Lee, Y., Song, C., Cho, H. R., Ghaffari, R., Choi, T. K., et al. (2015). An endoscope with integrated transparent bioelectronics and theranostic nanoparticles for colon cancer treatment. Nat. Commun. 6 (1), 10059. doi:10.1038/ncomms10059
Lee, J., Cho, H. R., Cha, G. D., Seo, H., Lee, S., Park, C. K., et al. (2019). Flexible, sticky, and biodegradable wireless device for drug delivery to brain tumors. Nat. Commun. 10 (1), 5205. doi:10.1038/s41467-019-13198-y
Lee, J. Y., Bashur, C. A., Goldstein, A. S., and Schmidt, C. E. (2009). Polypyrrole-coated electrospun PLGA nanofibers for neural tissue applications. Biomaterials 30 (26), 4325–4335. doi:10.1016/j.biomaterials.2009.04.042
Lee, J., Yoo, B., Lee, H., Cha, G. D., Lee, H. S., Cho, Y., et al. (2017). Ultra-wideband multi-dye-sensitized upconverting nanoparticles for information security application. Adv. Mat. 29 (1), 1603169. doi:10.1002/adma.201603169
Lei, T., Guan, M., Liu, J., Lin, H. C., Pfattner, R., Shaw, L., et al. (2017). Biocompatible and totally disintegrable semiconducting polymer for ultrathin and ultralightweight transient electronics. Proc. Natl. Acad. Sci. U. S. A. 114 (20), 5107–5112. doi:10.1073/pnas.1701478114
Li, C., Guo, C., Fitzpatrick, V., Ibrahim, A., Zwierstra, M. J., Hanna, P., et al. (2020). Design of biodegradable, implantable devices towards clinical translation. Nat. Rev. Mat. 5 (1), 61–81. doi:10.1038/s41578-019-0150-z
Li, J., Hacker, T. A., Wei, H., Long, Y., Yang, F., Ni, D., et al. (2021). Long-term in vivo operation of implanted cardiac nanogenerators in swine. Nano Energy 90, 106507. doi:10.1016/j.nanoen.2021.106507
Li, J., Kang, L., Long, Y., Wei, H., Yu, Y., Wang, Y., et al. (2018). Implanted battery-free direct-current micro-power supply from in vivo breath energy harvesting. ACS Appl. Mat. Interfaces 10 (49), 42030–42038. doi:10.1021/acsami.8b15619
Li, J., Kang, L., Yu, Y., Long, Y., Jeffery, J. J., Cai, W., et al. (2018). Study of long-term biocompatibility and bio-safety of implantable nanogenerators. Nano Energy 51, 728–735. doi:10.1016/j.nanoen.2018.07.008
Li, J., Long, Y., and Wang, X. (2020). Polymer-based nanogenerator for biomedical applications. Chem. Res. Chin. Univ. 36 (1), 41–54. doi:10.1007/s40242-020-9085-6
Li, J., Long, Y., Yang, F., and Wang, X. (2020). Degradable piezoelectric biomaterials for wearable and implantable bioelectronics. Curr. Opin. Solid State Mater. Sci. 24 (1), 100806. doi:10.1016/j.cossms.2020.100806
Li, J., Long, Y., Yang, F., and Wang, X. (2020). Respiration-driven triboelectric nanogenerators for biomedical applications. Ecomat 2 (3), e12045. doi:10.1002/eom2.12045
Li, J., Long, Y., Yang, F., Wei, H., Zhang, Z., Wang, Y., et al. (2020). Multifunctional artificial artery from direct 3D printing with built-in ferroelectricity and tissue-matching modulus for real-time sensing and occlusion monitoring. Adv. Funct. Mat. 30 (39), 2002868. doi:10.1002/adfm.202002868
Li, J., and Wang, X. (2017). Research Update: Materials design of implantable nanogenerators for biomechanical energy harvesting. Apl. Mat. 5 (7), 073801. doi:10.1063/1.4978936
Li, J., Yang, F., Long, Y., Dong, Y., Wang, Y., and Wang, X. (2021). Bulk ferroelectric metamaterial with enhanced piezoelectric and biomimetic mechanical properties from additive manufacturing. ACS Nano 15 (9), 14903–14914. doi:10.1021/acsnano.1c05003
Li, L., Ge, J., Guo, B., and Ma, P. X. (2014). In situ forming biodegradable electroactive hydrogels. Polym. Chem. 5 (8), 2880–2890. doi:10.1039/c3py01634j
Li, M., Guo, Y., Wei, Y., Macdiarmid, A., and Lelkes, P. (2006). Electrospinning polyaniline-contained gelatin nanofibers for tissue engineering applications. Biomaterials 27 (13), 2705–2715. doi:10.1016/j.biomaterials.2005.11.037
Li, R. F., Wang, L., and Yin, L. (2018). Materials and devices for biodegradable and soft biomedical electronics. Materials 11 (11), 2108. doi:10.3390/ma11112108
Li, R., Qi, H., Ma, Y., Deng, Y., Liu, S., Jie, Y., et al. (2020). A flexible and physically transient electrochemical sensor for real-time wireless nitric oxide monitoring. Nat. Commun. 11 (1), 3207. doi:10.1038/s41467-020-17008-8
Li, R., Wang, L., Kong, D., and Yin, L. (2018). Recent progress on biodegradable materials and transient electronics. Bioact. Mater. 3 (3), 322–333. doi:10.1016/j.bioactmat.2017.12.001
Lin, S. T., Yuk, H., Zhang, T., Parada, G. A., Koo, H., Yu, C., et al. (2016). Stretchable hydrogel electronics and devices. Adv. Mat. 28 (22), 4497–4505. doi:10.1002/adma.201504152
Liu, A., Long, Y., Li, J., Gu, L., Karim, A., Wang, X., et al. (2021). Accelerated complete human skin architecture restoration after wounding by nanogenerator-driven electrostimulation. J. Nanobiotechnology 19 (1), 280. doi:10.1186/s12951-021-01036-7
Liu, K., Tran, H., Feig, V. R., and Bao, Z. (2020). Biodegradable and stretchable polymeric materials for transient electronic devices. MRS Bull. 45 (2), 96–102. doi:10.1557/mrs.2020.24
Liu, Q., Jiang, L., Shi, R., and Zhang, L. (2012). Synthesis, preparation, in vitro degradation, and application of novel degradable bioelastomers—a review. Prog. Polym. Sci. 37 (5), 715–765. doi:10.1016/j.progpolymsci.2011.11.001
Liu, Q. Y., Tian, M., Ding, T., Shi, R., and Zhang, L. (2005). Preparation and characterization of a biodegradable polyester elastomer with thermal processing abilities. J. Appl. Polym. Sci. 98 (5), 2033–2041. doi:10.1002/app.22397
Long, Y., Li, J., Yang, F., Wang, J., and Wang, X. (2021). Wearable and implantable electroceuticals for therapeutic electrostimulations. Adv. Sci. (Weinh). 8 (8), 2004023. doi:10.1002/advs.202004023
Long, Y., Wei, H., Li, J., Yao, G., Yu, B., Ni, D., et al. (2018). Effective wound healing enabled by discrete alternative electric fields from wearable nanogenerators. ACS Nano 12 (12), 12533–12540. doi:10.1021/acsnano.8b07038
Long, Y., Yu, Y., Yin, X., Li, J., Carlos, C., Du, X., et al. (2019). Effective anti-biofouling enabled by surface electric disturbance from water wave-driven nanogenerator. Nano Energy 57, 558–565. doi:10.1016/j.nanoen.2018.12.069
Lyu, S., and Untereker, D. (2009). Degradability of polymers for implantable biomedical devices. Int. J. Mol. Sci. 10 (9), 4033–4065. doi:10.3390/ijms10094033
Manousiouthakis, E., Park, J., Hardy, J. G., Lee, J. Y., and Schmidt, C. E. (2022). Towards the translation of electroconductive organic materials for regeneration of neural tissues. Acta Biomater. 139, 22–42. doi:10.1016/j.actbio.2021.07.065
Mawad, D., Gilmore, K., Molino, P., Wagner, K., Wagner, P., Officer, D. L., et al. (2011). An erodible polythiophene-based composite for biomedical applications. J. Mat. Chem. 21 (15), 5555–5560. doi:10.1039/c1jm10259a
Middleton, J. C., and Tipton, A. J. (2000). Synthetic biodegradable polymers as orthopedic devices. Biomaterials 21 (23), 2335–2346. doi:10.1016/s0142-9612(00)00101-0
Migneco, F., Huang, Y. C., Birla, R. K., and Hollister, S. J. (2009). Poly(glycerol-dodecanoate), a biodegradable polyester for medical devices and tissue engineering scaffolds. Biomaterials 30 (33), 6479–6484. doi:10.1016/j.biomaterials.2009.08.021
Miyamoto, A., Lee, S., Cooray, N. F., Lee, S., Mori, M., Matsuhisa, N., et al. (2017). Inflammation-free, gas-permeable, lightweight, stretchable on-skin electronics with nanomeshes. Nat. Nanotechnol. 12 (9), 907–913. doi:10.1038/nnano.2017.125
Morsada, Z., Hossain, M. M., Islam, M. T., Mobin, M. A., and Saha, S. (2021). Recent progress in biodegradable and bioresorbable materials: From passive implants to active electronics. Appl. Mater. Today 25, 101257. doi:10.1016/j.apmt.2021.101257
Nair, L. S., and Laurencin, C. T. (2007). Biodegradable polymers as biomaterials. Prog. Polym. Sci. 32 (8), 762–798. doi:10.1016/j.progpolymsci.2007.05.017
Nijst, C. L. E., Bruggeman, J. P., Karp, J. M., Ferreira, L., Zumbuehl, A., Bettinger, C. J., et al. (2007). Synthesis and characterization of photocurable elastomers from poly(glycerol-co-sebacate). Biomacromolecules 8 (10), 3067–3073. doi:10.1021/bm070423u
Pérez-Madrigal, M. M., Giannotti, M. I., Armelin, E., Sanz, F., and Aleman, C. (2014). Electronic, electric and electrochemical properties of bioactive nanomembranes made of polythiophene:thermoplastic polyurethane. Polym. Chem. 5 (4), 1248–1257. doi:10.1039/c3py01313h
Pomerantseva, I., Krebs, N., Hart, A., Neville, C. M., Huang, A. Y., and Sundback, C. A. (2009). Degradation behavior of poly(glycerol sebacate). J. Biomed. Mat. Res. A 91A (4), 1038–1047. doi:10.1002/jbm.a.32327
Rai, R., Tallawi, M., Grigore, A., and Boccaccini, A. R. (2012). Synthesis, properties and biomedical applications of poly(glycerol sebacate) (PGS): A review. Prog. Polym. Sci. 37 (8), 1051–1078. doi:10.1016/j.progpolymsci.2012.02.001
Regazzoli, D., Leone, P. P., Colombo, A., and Latib, A. (2017). New generation bioresorbable scaffold technologies: An update on novel devices and clinical results. J. Thorac. Dis. 2017, S979–S985. doi:10.21037/jtd.2017.07.104
Salvatore, G. A., Sulzle, J., Dalla Valle, F., Cantarella, G., Robotti, F., Jokic, P., et al. (2017). Biodegradable and highly deformable temperature sensors for the internet of things. Adv. Funct. Mat. 27 (35), 1702390. doi:10.1002/adfm.201702390
Schmitt, E. E., Epstein, M., and Polistina, R. A. (1969). Process for polymerizing a glycolide. Google Patents.
Serrano, M. C., Pagani, R., Vallet-Regí, M., Peña, J., Rámila, A., and Izquierdo, I. (2004). In vitro biocompatibility assessment of poly($epsiv;-caprolactone) films using L929 mouse fibroblasts. Biomaterials 25 (25), 5603–5611. doi:10.1016/j.biomaterials.2004.01.037
Sheng, H., Zhou, J., Li, B., He, Y., Zhang, X., Liang, J., et al. (2021). A thin, deformable, high-performance supercapacitor implant that can be biodegraded and bioabsorbed within an animal body. Sci. Adv. 7 (2), eabe3097. doi:10.1126/sciadv.abe3097
Shin, H., Jo, S., and Mikos, A. G. (2003). Biomimetic materials for tissue engineering. Biomaterials 24 (24), 4353–4364. doi:10.1016/s0142-9612(03)00339-9
Shin, J., Liu, Z., Bai, W., Liu, Y., Yan, Y., Xue, Y., et al. (2019). Bioresorbable optical sensor systems for monitoring of intracranial pressure and temperature. Sci. Adv. 5 (7), eaaw1899. doi:10.1126/sciadv.aaw1899
Son, D., Lee, J., Lee, D. J., Ghaffari, R., Yun, S., Kim, S. J., et al. (2015). Bioresorbable electronic stent integrated with therapeutic nanoparticles for endovascular diseases. ACS Nano 9 (6), 5937–5946. doi:10.1021/acsnano.5b00651
Sugiyama, F., Kleinschmidt, A. T., Kayser, L. V., Alkhadra, M. A., Wan, J. M. H., Chiang, A. S. C., et al. (2018). Stretchable and degradable semiconducting block copolymers. Macromolecules 51 (15), 5944–5949. doi:10.1021/acs.macromol.8b00846
Sun, Q. Q., Qian, B., Uto, K., Chen, J., Liu, X., and Minari, T. (2018). Functional biomaterials towards flexible electronics and sensors. Biosens. Bioelectron. X. 119, 237–251. doi:10.1016/j.bios.2018.08.018
Tamayol, A., Hassani Najafabadi, A., Mostafalu, P., Yetisen, A. K., Commotto, M., Aldhahri, M., et al. (2017). Biodegradable elastic nanofibrous platforms with integrated flexible heaters for on-demand drug delivery. Sci. Rep. 7 (1), 9220. doi:10.1038/s41598-017-04749-8
Tao, H., Hwang, S. W., Marelli, B., An, B., Moreau, J. E., Yang, M., et al. (2014). Silk-based resorbable electronic devices for remotely controlled therapy and in vivo infection abatement. Proc. Natl. Acad. Sci. U. S. A. 111 (49), 17385–17389. doi:10.1073/pnas.1407743111
Tao, H., Kainerstorfer, J. M., Siebert, S. M., Pritchard, E. M., Sassaroli, A., Panilaitis, B. J. B., et al. (2012). Implantable, multifunctional, bioresorbable optics. Proc. Natl. Acad. Sci. U. S. A. 109 (48), 19584–19589. doi:10.1073/pnas.1209056109
Teng, H. (2012). Overview of the development of the fluoropolymer industry. Appl. Sci. (Basel).Applied Sci. 2 (2), 496–512. doi:10.3390/app2020496
Tian, H. Y., Tang, Z., Zhuang, X., Chen, X., and Jing, X. (2012). Biodegradable synthetic polymers: Preparation, functionalization and biomedical application. Prog. Polym. Sci. 37 (2), 237–280. doi:10.1016/j.progpolymsci.2011.06.004
Tran, H., Feig, V. R., Liu, K., Wu, H. C., Chen, R., Xu, J., et al. (2019). Stretchable and fully degradable semiconductors for transient electronics. ACS Cent. Sci. 5 (11), 1884–1891. doi:10.1021/acscentsci.9b00850
Tran, R. T., Thevenot, P., Gyawali, D., Chiao, J. C., Tang, L., and Yang, J. (2010). Synthesis and characterization of a biodegradable elastomer featuring a dual crosslinking mechanism. Soft Matter 6 (11), 2449–2461. doi:10.1039/c001605e
Vepari, C., and Kaplan, D. L. (2007). Silk as a biomaterial. Prog. Polym. Sci. 32 (8), 991–1007. doi:10.1016/j.progpolymsci.2007.05.013
Vert, M., Doi, Y., Hellwich, K. H., Hess, M., Hodge, P., Kubisa, P., et al. (2012). Terminology for biorelated polymers and applications (IUPAC Recommendations 2012). Pure Appl. Chem. 84 (2), 377–410. doi:10.1351/pac-rec-10-12-04
Vey, E., Rodger, C., Booth, J., Claybourn, M., Miller, A. F., and Saiani, A. (2011). Degradation kinetics of poly(lactic-co-glycolic) acid block copolymer cast films in phosphate buffer solution as revealed by infrared and Raman spectroscopies. Polym. Degrad. Stab. 96 (10), 1882–1889. doi:10.1016/j.polymdegradstab.2011.07.011
Vlachopoulos, A., Karlioti, G., Balla, E., Daniilidis, V., Kalamas, T., Stefanidou, M., et al. (2022). Poly(Lactic acid)-based microparticles for drug delivery applications: An overview of recent advances. Pharmaceutics 14 (2), 359. doi:10.3390/pharmaceutics14020359
Wang, B., Cheng, C., Jin, M., He, J., Zhang, H., Ren, W., et al. (2022). A site distance effect induced by reactant molecule matchup in single-atom catalysts for fenton-like reactions. Angew. Chem. Int. Ed. Engl. 134 (33), e202207268. in English. doi:10.1002/ange.202207268
Wang, J., Ayari, M. A., Khandakar, A., Chowdhury, M. E. H., Uz Zaman, S. A., Rahman, T., et al. (2022). Estimating the relative crystallinity of biodegradable polylactic acid and polyglycolide polymer composites by machine learning methodologies. Polymers 14 (3), 527. doi:10.3390/polym14030527
Wang, L., Lou, Z., Wang, K., Zhao, S., Yu, P., and Wei, W. (2020). Biocompatible and biodegradable functional polysaccharides for flexible humidity sensors. Res. (Wash D C) 2020, 8716847. doi:10.34133/2020/8716847
Wang, P. P., Hu, M., Wang, H., Chen, Z., Feng, Y., Wang, J., et al. (2020). The evolution of flexible electronics: From nature, beyond nature, and to nature. Adv. Sci. (Weinh). 7 (20), 2001116. doi:10.1002/advs.202001116
Wang, S., Guan, S., Wang, J., Liu, H., Liu, T., Ma, X., et al. (2017). Fabrication and characterization of conductive poly (3, 4-ethylenedioxythiophene) doped with hyaluronic acid/poly (l-lactic acid) composite film for biomedical application. J. Biosci. Bioeng. 123 (1), 116–125. doi:10.1016/j.jbiosc.2016.07.010
Wang, S., Oh, J. Y., Xu, J., Tran, H., and Bao, Z. (2018). Skin-inspired electronics: An emerging paradigm. Acc. Chem. Res. 51 (5), 1033–1045. doi:10.1021/acs.accounts.8b00015
Wang, Y., Tran, H. D., and Kaner, R. B. (2011). Applications of oligomers for nanostructured conducting polymers. Macromol. Rapid Commun. 32 (1), 35–49. doi:10.1002/marc.201000280
Wei, Z. X., and Faul, C. F. J. (2008). Aniline oligomers - architecture, function and new opportunities for nanostructured materials. Macromol. Rapid Commun. 29 (4), 280–292. doi:10.1002/marc.200700741
Wei, Z., Xue, Z., and Guo, Q. (2021). Recent progress on bioresorbable passive electronic devices and systems. Micromachines (Basel). 12 (6), 600. doi:10.3390/mi12060600
Xu, C., Huang, Y., Yepez, G., Wei, Z., Liu, F., Bugarin, A., et al. (2016). Development of dopant-free conductive bioelastomers. Sci. Rep. 6 (1), 34451. doi:10.1038/srep34451
Xu, W., Xiao, M., Yuan, L., Zhang, J., and Hou, Z. (2018). Preparation, physicochemical properties and hemocompatibility of biodegradable chitooligosaccharide-based polyurethane. Polym. (Basel). 10 (6), 580. doi:10.3390/polym10060580
Yang, J., Webb, A. R., and Ameer, G. A. J. A. M. (2004). Novel citric acid-based biodegradable elastomers for tissue engineering. Adv. Mat. 16 (6), 511–516. doi:10.1002/adma.200306264
Yang, Q., Huang, Z., Li, X., Liu, Z., Li, H., Liang, G., et al. (2019). A wholly degradable, rechargeable Zn–Ti3C2 MXene capacitor with superior anti-self-discharge function. ACS Nano 13 (7), 8275–8283. doi:10.1021/acsnano.9b03650
Yang, Y., Ko, T. P., Liu, L., Li, J., Huang, C. H., Chan, H. C., et al. (2014). Structural insights into enzymatic degradation of oxidized polyvinyl alcohol. Chembiochem 15 (13), 1882–1886. doi:10.1002/cbic.201402166
Yu, X. W., Shou, W., Mahajan, B. K., Huang, X., and Pan, H. (2018). Materials, processes, and facile manufacturing for bioresorbable electronics: A review. Adv. Mat. 30 (28), 1707624. doi:10.1002/adma.201707624
Yue, O., Wang, X., Liu, X., Hou, M., Zheng, M., Wang, Y., et al. (2021). Spider-web and ant-tentacle doubly bio-inspired multifunctional self-powered electronic skin with hierarchical nanostructure. Adv. Sci. 8 (15), 2004377. doi:10.1002/advs.202004377
Zelikin, A. N., Lynn, D. M., Farhadi, J., Martin, I., Shastri, V., and Langer, R. (2002). Erodible conducting polymers for potential biomedical applications. Angew. Chem. Int. Ed. 41 (1), 141–144. doi:10.1002/1521-3773(20020104)41:1<141::aid-anie141>3.0.co;2-v
Zeng, X., Deng, L., Yao, Y., Sun, R., Xu, J., and Wong, C. P. (2016). Flexible dielectric papers based on biodegradable cellulose nanofibers and carbon nanotubes for dielectric energy storage. J. Mat. Chem. C Mat. 4 (25), 6037–6044. doi:10.1039/c6tc01501h
Zhang, Q., Yan, Y., Li, S., and Feng, T. (2010). The synthesis and characterization of a novel biodegradable and electroactive polyphosphazene for nerve regeneration. Mater. Sci. Eng. C 30 (1), 160–166. doi:10.1016/j.msec.2009.09.013
Zhang, X., and Bellan, L. M. (2017). Composites formed from thermoresponsive polymers and conductive nanowires for transient electronic systems. ACS Appl. Mat. Interfaces 9 (26), 21991–21997. doi:10.1021/acsami.7b04748
Zhang, Y. J., Zhou, Z., Fan, Z., Zhang, S., Zheng, F., Liu, K., et al. (2018). Self-powered multifunctional transient bioelectronics. Small 14 (35), 1802050. doi:10.1002/smll.201802050
Zhao, D. W., Chen, C., Zhang, Q., Chen, W., Liu, S., Wang, Q., et al. (2017). High performance, flexible, solid-state supercapacitors based on a renewable and biodegradable mesoporous cellulose membrane. Adv. Energy Mat. 7 (18), 1700739. doi:10.1002/aenm.201700739
Zheng, Q., Zou, Y., Zhang, Y., Liu, Z., Shi, B., Wang, X., et al. (2016). Biodegradable triboelectric nanogenerator as a life-time designed implantable power source. Sci. Adv. 2 (3), e1501478. doi:10.1126/sciadv.1501478
Zhou, J.-f., Peng, J., Wang, Y. g., Cheng, L., and Wu, Z. (2016). Preparation of polypyrrole-embedded electrospun poly(lactic acid) nanofibrous scaffolds for nerve tissue engineering. Neural Regen. Res. 11 (10), 1644. doi:10.4103/1673-5374.193245
Keywords: degradation, polymers, flexible electronics, biomedical devices., transient electronics, bioresorbable materials, implantable devices
Citation: Zhai Z, Du X, Long Y and Zheng H (2022) Biodegradable polymeric materials for flexible and degradable electronics. Front. Electron. 3:985681. doi: 10.3389/felec.2022.985681
Received: 07 July 2022; Accepted: 01 August 2022;
Published: 06 September 2022.
Edited by:
Jun Li, University of Wisconsin-Madison, United StatesReviewed by:
Ruosen Xie, University of Wisconsin-Madison, United StatesCopyright © 2022 Zhai, Du, Long and Zheng. This is an open-access article distributed under the terms of the Creative Commons Attribution License (CC BY). The use, distribution or reproduction in other forums is permitted, provided the original author(s) and the copyright owner(s) are credited and that the original publication in this journal is cited, in accordance with accepted academic practice. No use, distribution or reproduction is permitted which does not comply with these terms.
*Correspondence: Yin Long, eWlubG9uZzU2QHVlc3RjLmVkdS5jbg==; Heng Zheng, ZmVuZ3dlaS1iY0AxNjMuY29t
Disclaimer: All claims expressed in this article are solely those of the authors and do not necessarily represent those of their affiliated organizations, or those of the publisher, the editors and the reviewers. Any product that may be evaluated in this article or claim that may be made by its manufacturer is not guaranteed or endorsed by the publisher.
Research integrity at Frontiers
Learn more about the work of our research integrity team to safeguard the quality of each article we publish.