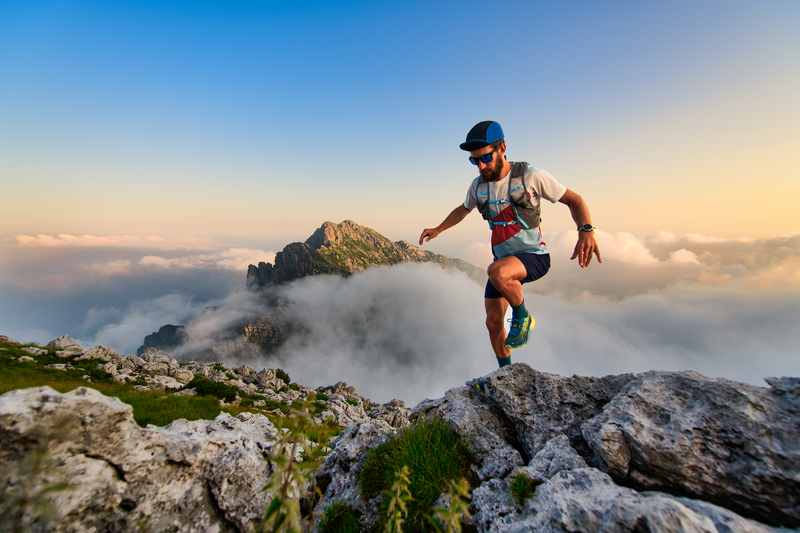
95% of researchers rate our articles as excellent or good
Learn more about the work of our research integrity team to safeguard the quality of each article we publish.
Find out more
REVIEW article
Front. Electron. Mater , 21 June 2023
Sec. Semiconducting Materials and Devices
Volume 3 - 2023 | https://doi.org/10.3389/femat.2023.1174159
This article is part of the Research Topic Celebrating 1 Year of Frontiers in Electronic Materials View all 11 articles
In recent years, perovskite material-based photovoltaic devices have attracted great attention of researchers because of an expeditious improvement in their efficiency from 3.8% to over 25%. The electron transport layer (ETL), which functions for the extraction and transportation of photogenerated electrons from active perovskite material to the electrodes, is a vital part of these perovskite solar cells (PSCs). The optoelectronic properties of these electron transport layer materials also have an impact on the performance of these perovskite solar cells, and for commercialized flexible perovskite solar cells, low-temperature and solution-processable electron transport layers having high stability and suitable optoelectronic properties are needed. In this regard, the solution-processable films of different metal oxides have been largely investigated by many research groups. So, this review summarizes the optoelectronic properties of the different metal oxide-based electron transport layers and the development in the performance of the perovskite solar cells, which have solution-processable metal oxides as electron transport layers.
Solar energy, a green energy source, is the demand of the time as conventional energy sources cannot deal with the present and future energy crises in the world. For harvesting solar energy, bulk silicon wafer-based solar cells (SCs), which are the first-generation SCs, have successfully been developed and commercialized (Liu et al., 2018). However, they have limited power conversion efficiency (PCE), and the cost of these is also high as the material is used in bulk (Andreani et al., 2019). To address the high cost of solar cells, there has been research into the second-generation solar cell technologies, such as thin-film silicon solar cells and thin-film heterojunction-based solar cells (Shah et al., 1995). However, the PCE of these SCs also remains limited. So, a solar cell that has high stability, high PCE, and low cost needs to be developed and commercialized. In this respect, third-generation solar cells like organic solar cells (OSCs), perovskite solar cells (PSCs), and quantum dot solar cells (SCs) are of great interest in research because of their potential for high PCE (Brown and Wu, 2009; Tvrdy and Kamat, 2011; Khatibi et al., 2019). In these, the PSCs have attracted the eye of the researchers more because of a significant increase in the PCE from 3.8% to 25.5% (as certified by the Best Cell-Efficiency Chart in 2022 shown in Figure 1) (Best Cell-Efficiency Chart NREL, 2021). PSCs have a basic construction in which an active perovskite layer is placed between a hole-transporting layer (HTL) and an electron-transporting layer (ETL) (Hussain et al., 2018). The active layer is used to absorb the light and create the hole–electron pairs (i.e., the excitons) in the devices. Although the carrier (i.e., electron or hole) transport layers (CTLs) are used for the efficient extraction and transportation of these carriers from the active layer to the electrodes. The performance of PSCs depends upon the stability of active material and the stability of these CTLs, i.e., these CTLs also play a vital role in achieving high stability and the high PCE of SCs (Wang et al., 2019a). For a high PCE, these CTLs should have many optical and electrical properties like high electron/hole mobility, high blocking ability to the counter carriers, a wide band gap, and high transparency to ensure the light passes through them with minimal absorbance (Ansari et al., 2018). These CTLs should also show a high compatibility and ohmic contact with the adjacent active layer and the electrode. In addition to these, for the better extraction of the electrons, the conduction band (C.B.) of the ETL should match the lower unoccupied molecular orbit (LUMO) of the active layer and for the better extraction of holes; the valence band (V.B.) of the HTL should match the higher occupied molecular orbit (HOMO) of the active layer (Ren et al., 2019). As the cost of solar is also a major factor for its commercialization, the CTLs should be processable via solution process methods that are cost-effective and highly compatible with large-scale printing of flexible solar cells. Metal oxides like TiO2, ZnO, SnO2, Nb2O5, CeOx, WO3, and In2O3 for ETLs are the ideal materials that meet such requirements (Kojima et al., 2009; Kumar et al., 2013; Song et al., 2015; Singh et al., 2016; Wang et al., 2019b). These metal oxides show high stability in the ambient environment, and these can be processable via solution process methods, which make these useful for large-scale and flexible solar cell devices. For the ETL, TiO2 has been investigated a lot as it has a wide band gap and high transparency. However, it also has low carrier mobility and also needs a high temperature (∼500 °C) for better crystallinity, leading to better performance of SCs. So, the materials like SnO2 and ZnO are emerging as an alternative to TiO2 because these can be processed at a relatively low temperature, and these materials possess a wider band gap, higher electron mobility, and higher transparency. In addition to these binary metal oxides, other inorganic materials like BaSnO3, SrTiO3, SrSnO3, ZrSnO4, and (CH3)2Sn(COOH)2 have also been developed and investigated as an ETL for futuristic PSCs (Bera et al., 2014; Shin et al., 2017; Guo et al., 2019a; Li et al., 2020; Noh et al., 2020). The aim of this review is to present a comprehensive analysis of binary metal oxide-based solution-processable ETLs used in PSCs.
FIGURE 1. Achieved efficiencies of different SCs. Adapted from the National Renewable Energy Laboratory (Best Cell-Efficiency Chart NREL, 2021).
In PSCs, perovskite materials (having a general chemical formula ABX3, where A and B are cations and X is an anion) such as CH3NH3PbI3, CH3NH3PbCl3 CH3NH3PbBr3, and CsPbBr3 are used as an absorber layer for harvesting the light (Mesquita et al., 2017). This is why the term “perovskite solar cell” is being used for this type of solar cell. The crystal structure of these perovskite materials is shown in Figure 2.
The organic-metal halide perovskites consist of an organic cation [i.e., CH3NH3+ (methyl-ammonium), CH3CH2NH3+ (ethyl-ammonium), and NH2CH = NH2+ (formamidinium)], a divalent metal cation (i.e., Pb2+, Sn2+, and Ge2+), and a monovalent halogen anion (i.e., F-, Cl-, Br-, and I) (Ono et al., 2017). For photovoltaic applications, perovskite materials have first been investigated by Kojima et al. (2009) who fabricated the two different PSCs with CH3NH3PbBr3 and CH3NH3PbI3 as absorbing layers, having PCE of 3.1% and 3.81%, respectively. After this, CH3NH3PbI3 (methylammonium lead iodide or MAPbI3) has largely been investigated in PSCs because it has a small band gap (1.5–1.6 eV) and a wide absorption spectrum up to 800 nm.
The perovskite solar cell has a general structure, in which the active perovskite material film is sandwiched between the ETL and HTL, and the transparent conducting oxide (TCO) contact layer with a backing of the glass substrate and metal contacts have been attached with the corresponding ETL and HTL based on the structure. If the TCO layer exists adjacent to the ETL, the configuration is known as the n–i–p configuration, or if the TCO exists adjacent to the HTL, it is known as the p–i–n configuration or the inverted structure. The PSCs have been further divided into a planar and mesoporous structure. In the planar structure, only the compact layer of the transporting material is used. If the configuration is n-i-p, then it is a planar heterojunction PSC; otherwise, it is an inverted planar heterojunction solar cell, as shown in Figures 3A,B respectively. However, in the mesoporous (n-i-p) structure, the mesoporous scaffold layer either of n-type material or the inert Al2O3 is added, as shown in Figure 3C, and in the mesoporous (p-i-n) structure, the mesoporous scaffold layer either of p-type material or the inert Al2O3 is added, as shown in Figure 3D. These scaffold layers help in the formation of the pin-hole-free film of perovskite material and also in better extraction of the carriers.
FIGURE 3. Different configuration of PSCs: (A) planar (n-i-p) heterojunction structure, (B) inverted planar (p-i-n) heterojunction structure, (C) mesoporous (n-i-p) heterojunction structure, and (D) mesoporous (p-i-n) heterojunction structure.
When the light falls on a PSC and enters into the perovskite layer either passing through the ETL or HTL, the perovskite material absorbs the light and creates the pairs of electron–hole carriers, i.e., excitons, as shown in Figures 4A,B. Then, these carriers get diffused into the absorber layer and collected via ETL and HTL materials, respectively, at the boundaries and have been transported to the respective electrodes, and the external circuit connected to it gets the power (Jung and Park, 2015). For the efficient collection of carriers, the diffusion length of excitons should be high, and also, the thickness of the absorber layer should be less than the diffusion length of excitons so that the high number of carriers can be collected by transporting materials before their recombination happens.
For collecting the carriers from the absorber and transporting them to the corresponding electrodes, the ETL plays a crucial role. Therefore, for a better outcome of the solar cell, these ETLs should also possess some important properties like high carrier mobility, large-band gap, better energy level alignment with the absorber layer, high transparency to the light, high conductivity, high stability, and better adhesivity to the absorber layer. In addition to these, for the low cost and flexible device, these can also be processable via vacuum-free and low-temperature solution process techniques. The metal oxides like TiO2, ZnO, SnO2, CeOx, WOx, InOx, and NbOx, have optoelectronic properties suitable for ETLs, and these can also be processed via solution-processable methods like spin coating, chemical bath deposition, electro-spray, spray pyrolysis, and screen-printing methods. Some advantages and disadvantages of these metal oxides as an ETL have been summarized in Table 1. Furthermore, the development in PCE and the other properties of the PSCs based on these solution-processable metal oxide ETLs have been discussed.
As an ETL material in PSCs, TiO2 is a promising material as its optical and electrical properties favor electron extraction. In PSCs, TiO2 has been investigated in the form of a compact layer (c-TiO2) and a mesoscopic layer (m-TiO2). TiO2 has the properties like a wider band gap (∼3.1 eV), the electron mobility of the order of 1 cm2/V-sec, a conduction band minimum (CBM, i.e., LUMO level) of ∼ −4.1 eV, and a valence band maximum (VBM, i.e., HOMO level) of ∼ −7.2 eV with reference to the vacuum level (Seo et al., 2005; Green et al., 2014; Jung and Park, 2015). These properties favor the efficient extraction of electrons from the active material. The favorable conduction band minimum alignment of the TiO2 helps in the efficient extraction of the electrons, and the deeper valance band maximum blocks the movement of the holes from the perovskite layer to TiO2. Because of these, TiO2 has been used widely as an ETL material. In addition to these favorable properties, TiO2 has some drawbacks too like the low electron mobility and the need for a high temperature for the crystalline film. For the better electrical conductivity of TiO2, the nano-crystalline film is required and for this, there is a need for high-temperature (∼500 °C) annealing (Green et al., 2014). This requirement of high-temperature annealing limits the use of TiO2 for flexible photovoltaic devices. The performance of some TiO2 ETL based PSCs have been summarized in Table 2.
In 2009, when Kojima et al. (2009) introduced the first perovskite-based photovoltaic device, TiO2 had been used as the ETL material. In this work, the TiO2 film was fabricated by using the TiO2 paste post-treated at 480 °C temperature for 1 h. The TiO2/MAPbI3 heterojunction-based cell gave the best PCE of 3.81%. In the solution-processed methods, the most common precursor for the fabrication of the compact TiO2 film is titanium diisopropoxide bis(acetylacetonate). In 2012, Kim et al. (2012) first introduced the all-solid-state PSCs having the structure of FTO/TiO2/m-TiO2/MAPbI3/spiro-OMeTAD/Au, where the spiro-OMeTAD has been used as the HTL. In these PSCs, the c-TiO2 has been fabricated by the spin coating method with titanium diisopropoxide bis(acetylacetonate) as a precursor, while the m-TiO2 was fabricated by the doctor blade coating methods using the nanocrystalline TiO2 paste. These PSCs resulted in a champion PCE of 9.7%. Lee et al. (2012) had fabricated mesoporous PSCs of the structure of FTO/TiO2/m-Al2O3/MAPbI2Cl/spiro-OMeTAD/Ag, which resulted in a champion efficiency of 10.9%. In these PSCs, the c-TiO2 layer was deposited by the spray pyrolysis method. Kim et al. (2013a) used the nanorods of the rutile-phase TiO2 prepared via a hydrothermal method to form the m-TiO2 layer in PSCs, and the corresponding PSCs resulted in the champion PCE of 9.4% for 0.6-μm length of nanorods, and it decreased as the length of the nanorods increased. Liu et al. (2013) used the spin-coated TiO2 films on the FTO substrate to fabricate the planar PSCs having MAPbI3-xClx as an active material, which was deposited using the vapor deposition method, and these simple planar structured cells gave the best PCE of 15.4% without any complex structure. Docampo et al. (2013) had investigated the TiOx film prepared via spin coating and a low temperature (∼130 °C) post-annealing in the inverted structure PSCs. These inverted configuration cells delivered the best PCE of 9.8% and 6.4% on FTO-coated glass substrates and an ITO-coated flexible substrates, respectively. In addition to the spin coating and the spray pyrolysis method, the TiO2 ETLs have also been fabricated the via chemical bath deposition (CBD) method (Liu et al., 2013; Liang et al., 2017). Liang et al. (2017) fabricated the low-temperature (100 °C) annealed TiO2 film via the CBD method using TiCl4 as a precursor for the PSCs having the structure of FTO/TiO2/MAPbI3/spiro-OMeTAD/Au. The device resulted in a PCE of 11.18%, and the treatment of TiO2 films with UV-ozone improved the PCE to 12.62%. Lu et al. (2020) had also opted for the same CBD method and the same device structure, but they had delicately treated the TiCl4 precursor via KOH, which changes the morphology of the film, and hence, the device performance with a champion PCE of 19.1% have been achieved.
Yang et al. (2021a) had opted for a low-temperature (∼150 °C) annealing process for anatase TiO2 film growth for fabricating flexible PSCs with the PEN/ITO/TiO2/MAPbI3/spiro-OMeTAD/Au structure. For the deposition of the TiO2 film, they synthesized the colloidal TiO2 solution via the sol–gel method, which was used as a precursor for the TiO2 layer. The concentration of the precursor has been optimized to 2 mol/L for full coverage and aggregation-free films, which resulted in 16.11% efficiency. Recently, Bao et al. (2023) had investigated a honey-comb (Hc) type TiO2 film as an ETL in PSCs with FTO/TiO2/Hc-TiO2/MAPbI3/spiro-OMeTAD/MoO3/Ag structure, as shown in Figure 5. To fabricate the interconnecting Hc-TiO2 network, the sacrificial template method has been opted. This Hc-TiO2 network has a periodicity of around 450 nm, resulting in an additional light trapping at this wavelength scale. Hc-TiO2 showed a better band alignment with perovskite than the compact TiO2, and the perovskite crystal film has a preferred orientation along the (110) plane due to the confinement in the Hc-TiO2 pores, which reduced the trap density. The corresponding PSCs have yielded a PCE of 20.85% and showed a high tolerance toward the change in the optical path with changing the incident angle of the light.
FIGURE 5. (A) Schematic representation of Hc-TiO2 deposition, (B) structure of FTO/TiO2/Hc-TiO2/MAPbI3/spiro-OMeTAD/MoO3/Ag PSCs, (C) band structure of corresponding PSCs, (D) SEM image of perovskite/c-TiO2 (i), perovskite/Hc-TiO2(ii), perovskite/pore (iii), and cross-sectional structure of corresponding PSCs, (E) J-V curve and parameter, and (F) stability of corresponding PSCs. Reproduced with permission from Bao et al. (2023).
TiO2 needs a high annealing temperature for suitable morphological, electrical, and optical properties to show high performance of the PSCs, which limits its use for a flexible photovoltaic device. So, an alternative that exhibits the same or better characteristics with low temperature treatment is needed to develop for highly efficient new-generation flexible photovoltaic devices. In this regard, ZnO is the first material as an alternative to TiO2, which has been investigated by the researchers. It has a large band gap of ∼3.4 eV, higher electron mobility (200–205 cm2V−1 s −1), and the HOMO and LUMO energy levels are also similar to TiO2 (Sun et al., 2011). In addition to these, it can crystallize at a lower temperature, which makes it a potential alternative to TiO2 for flexible PSC devices. ZnO-based PSCs have some drawbacks as well like the presence of hydroxyl (−OH) radicals at the ZnO film surface, which reacts with perovskite materials and leads to its degradation (Yang et al., 2015). It limits the performance and stability of the corresponding PSCs. Still, many research groups have investigated it as an ETL in PSCs and also tried to tackle these challenges. The performance of some ZnO ETL based PSCs have been summarized in Table 3. Kumar et al. (2013) had investigated the low-temperature (˂100 °C) processed ZnO film for the PSC application. For preparing the compact film of ZnO, the electro-deposition technique was opted and a film of the nanorod ZnO was fabricated over the compact layer by the CBD method; corresponding PSCs resulted in the best PCE of 8.9% on a rigid substrate, while only 2.62% on a flexible substrate. Liu and Kelly (2014) had used the ZnO nanoparticle dispersion solution in a mixture of butanol, chloroform, and methanol solvents in a 70:5:5 ratio as a precursor for spin coating the ZnO ETL to fabricate the cell of ITO/ZnO/MAPbI3/spiro-OMeTAD/Ag structures and achieved a 15.7% efficiency. Ahmadi et al. (2020) had investigated the suitable solvent to prepare the precursor solution for the deposition of ZnO ETL and found that the ZnO film that was prepared with the 2-methoxyethanol solvent showed a high PCE of 10.39% and also a good reproducibility in terms of performance. ZnO/MAPbI3 films prepared by 2-methoxyethanol demonstrate higher absorbance in the range of 400–600 nm, lower PL emission intensity, and larger grain sizes than their IPA and ethanol counterparts.
Chavan et al. (2022) had developed a new organic ligand-free organometallic approach to synthesize the ZnO quantum dots (QDs) for the ZnO ETL. The developed ZnO QDs were capped by easily removable dimethyl sulfoxide (DMSO) molecules. Furthermore, the developed QDs were spin-coated and needed only a 150 °C post-annealing treatment up to 10 min only for the growth of the ZnO ETL layer. The developed ZnO ETL showed better uniformity, better charge extraction, and better band alignment with perovskite than the ETL deposited by usual sol–gel-processed ZnO. The device based on these newly developed ZnO QDs resulted in a champion PCE of 20.05%. Leng et al. (2021) treated the ZnO nanoparticle-processed ETL via 4,7-dichloro-1,10-phenanthroline (Cl-phen) and 1,10-bathophenanthroline (BPhen). This can suppress the -OH group content on the ZnO surface. Furthermore, the Cl-phen modification reduced the energy gap between CBM OF ZnO and LUMO of perovskite from 0.29eV to 0.06eV, as shown in Figure 6. This enhanced the charge extraction and resulted in a champion PCE of 21.15%.
FIGURE 6. (A) Device structure of the phenanthroline derivatives/ZnO bilayer-based PSC. (B) Schematic representation of additive treatment and its effect on the perovskite grain size. (C) Energy-level diagram of the materials layer. (D) J-V curve of corresponding PSCs. Reproduced with permission from Leng et al. (2021).
Tin (Ⅱ) oxide (SnO2) is also an emerging material for ETL purposes and as a replacement of TiO2 in PSCs. It has a large band gap of around 3.6 eV, higher bulk electron mobility (100–200 cm2V−1sec-1), and high transparency to the visible light spectrum (∼90%) (Sun et al., 2011; Wang et al., 2020a). In addition, the deposition and crystallization of the SnO2 film can be carried out at a comparatively lower processing temperature (≤200 °C) than TiO2. The performance of some SnO2 ETL based PSCs have been summarized in Table 4. Song et al. (2015) had also reported that SnO2 has better environmental stability than TiO2. SnO2 is widely used in the planar heterojunction PSCs as compared to the mesoscopic structure, i.e., the compact SnO2 (c-SnO2) has been investigated widely for ETL purposes. Ke et al. (2015) first investigated the solution-processed SnO2 films in PSCs, which were deposited via spin coating of the precursor of SnCl2 (dissolved in ethanol) with annealing at 180 °C, and the corresponding devices (i.e., FTO/SnO2/MAPbI3/spiro-OMeTAD/Au) gave the best PCE of 17.21% with small hysteresis, as shown in Figure 7.
FIGURE 7. Schematic representation of (A) structure and (B) band diagram. FTO/SnO2/MAPbI3/spiro-OMeTAD/Au cells, (C) transmittance of FTO, TiO2/FTO, and SnO2/FTO, and (D) J-V curve of corresponding SnO2 ETL-based PSCs. Reproduced with permission from Ke et al. (2015).
Anaraki et al. (2016) had opted for the chemical bath deposition (CBD) method for the deposition of the SnO2 ETL in mixed perovskite-based PSCs, which result in the best PCE of 20.8%. Liu et al. (2016) had used the dual combustion method for preparing the precursor of SnO2. In this, SnCl2 and ammonium nitrate (NH4NO3) were used as the combined oxidizer, while urea and acetylacetone as the fuel to the reaction. This method lowers the annealing temperature of SnO2 to 140 °C, but the corresponding PSCs show severe hysteresis and the PCE remains only 12.93%, which further increases to 15.18% via passivation of SnO2 with C60-SAM (self-assembled monolayer). Jiang et al. (2017) had used the SnO2 nanoparticles as the precursor solution and 150 °C annealing temperature for the SnO2 ETL in mixed perovskite ((FAPbI3)1−x (MAPbBr3)x)-based SCs, which resulted in the best PCE of 21.6% in the 0.0737 cm2 area device and the 20.1% in 1 cm2 area device. Huang et al. (2017b) investigated the low temperature (∼70 °C) UV-sintered SnO2 ETL in CH3NH3PbI3-xClx-based PSCs with the highest PCE of 16.21%. Barbé et al. (2017) had deposited the amorphous SnO2 via CBD at only 55 °C without any further annealing for MAPbI3-based PSCs, which result in the best PCE of 14.28%. Dong et al. (2019) had investigated the low-temperature (∼50 °C)-processed and UV-treated SnO2 ETL for mixed perovskite [Cs0.05(FA0.85MA0.15)0.95Pb(I0.85Br0.15)3]-based PSCs. The PSCs yielded the best PCEs of 20.5% and 17.5% on rigid and flexible substrates, respectively. A bilayer SnO2 of amorphous-crystalline hetero phase has been studied by Lee et al. (2020) in the triple cation perovskite-based PSCs, which yield a best PCE of 20.39% and 14.93% for smaller (∼0.09 cm2) and larger (3.55 cm2) active device areas, respectively. Recently, Wang et al. (2021) had modified the precursor of SnO2 with an addition of hydrogen peroxide (H2O2). In comparison to the unmodified precursor-based SnO2 film, the resultant modified precursor-based SnO2 film exhibits less trap density, improved electrical conductivity, outstanding film fabrication repeatability, and better energy level alignment, leading to significantly higher charge carrier extraction. The corresponding PSCs yield a best and a stabilized PCE of 22.15% and 21.9%, respectively, having a reduced hysteresis effect as compared to the devices based on an un-modified SnO2 precursor.
In addition to the compact layer SnO2, mesoscopic SnO2 (m-SnO2)-based PSCs have also been reported. Li et al. (2015) first reported PSCs based on m-SnO2 annealed at 450 °C, which resulted in a best PCE of 6.50% without any treatment of the SnO2 surface and a PCE of 10.18% with surface treatment via TiCl4. Roose et al. (2016) had investigated the m-SnO2-based PSCs on FTO and AZO substrates having the structures FTO/SnO2/m-SnO2/perovskite/spiro-OMeTAD/Au and AZO/SnO2/m-SnO2/perovskite/spiro-OMeTAD/Au, respectively. For this, the compact SnO2 has been synthesized via spray pyrolysis, while m-SnO2 has been synthesized via spin coating of the precursor, followed by annealing at 450 °C. The PSCs on AZO substrates show better performance than those on FTO substrates with the best PCE of 13.1%. The aforementioned mesoscopic scaffold SnO2 ETLs require a high temperature for growth, which hinders the growth of flexible PSCs. So a low-temperature (∼180 °C) processable m-SnO2-based PSCs have been reported by Wang et al. (2020a). To optimize the m-SnO2 layer, polyethylene glycol has been introduced in the colloidal SnO2 precursor and the PEG can be removed from the film through low-temperature annealing, which resulted in a mesoporous SnO2 film. The corresponding mixed perovskite [(Cs0.05FA0.95PbI3)0.97 (MAPbBr3)0.03]-based PSCs show a best PCE of 20.82%. Xiong et al. (2021) introduced polyethylene glycol diacrylate (PEGDA) in SnO2 dispersion solution to avoid agglomeration in the SnO2 film for ITO/SnO2/FAPbI3/Spiro-OMeTAD/MoO3/Ag PSCs. The PEGDA-modified SnO2 films were more uniform than the pristine SnO2 films, and the energy level of the modified film matched well with the perovskite, which improves the carrier transfer and reduces the energy loss. This resulted in a higher Voc of 1.14 V and a high PCE of 23.31%. Yoo et al. (2021) had opted the CBD route for the SnO2 ETL deposition in FTO/SnO2/mixed perovskite/spiro-OMeTAD/Au PSCs. They studied the changes in SnO2 film deposition with reaction time. During the SnO2 film deposition, the decomposition pathway of the SnO2 precursor (i.e., SnCl2) depends on the pH of the solution, which changes with the reaction time as urea present in the precursor decomposes and releases OH−. Initially, for pH ∼1–1.5, the dominant product of deposition was SnO2, and later on at pH = 3, the incomplete oxidation of Sn2+ to Sn4+ occurred due to the reduced amount of oxygen in the precursor solution, resulting in the SnO2-x phase. For higher pH∼6, (Sn6O4(OH)4 and SnO are produced, which are non-electron transporting phases. So, by optimizing the time of deposition, these phases can be avoided. The PSCs based on the ETL with SnO2 and SnO2-x phases yielded a high PCE of up to 24.4% with a certified PCE of 25.2%. Min et al. (2021) had reported a certified champion PCE of 25.5%, introducing an interlayer between the SnO2 ETL and FAPbI3 perovskite layer. They used the Cl− ion-based precursor for the SnO2 layer and also introduced the Cl− ions in FAPbI3. This introduced an interlayer of FASnClx between SnO2 and FAPbI3, which can enhance the charge extraction and reduce the recombination, leading to a high PCE.
In PSCs, WOx has also been investigated by many research groups as an alternative to TiO2. It shows a band gap of 2.6–3.1 eV varying according to its crystal structure (Berak and Sienko, 1970; Wang et al., 2012; Wang et al., 2017a). Although its band gap is smaller than TiO2, it shows high stability, and it also has a high electron mobility (10–20 cm2 V−1 s−1) in comparison to TiO2 (Wang et al., 2016). The performance of some WOx ETL based PSCs have been summarized in Table 5. Mahmood et al. (2015) had explored hydrothermally grown tungsten trioxide (WO3)-based nanostructures like 0-D nanoparticles, 1-D nanorods, and 3-D nanosheet arrays to fabricate porous ETL by using the electrospray method. In these, the 2-D nanosheet-WO3 film yielded enhanced carrier transport as compared to the nanorods or nanoparticles, which resulted in a PCE of 11.24% in FTO/WO3-nanosheet/TiO2/MAPbI3/spiro-OMeTAD/Ag configuration devices. Wang et al. (2016) had prepared amorphous WOx at 150 °C as the ETL for PSCs by using the spin coating method. The pristine WOx-based cell yielded a PCE of 10.42%, and with a composite of WOx-TiOx, the performance increased to a PCE of 14.47%. When the annealing temperature of WOx-TiOx layer decreased to 70 °C, corresponding PSCs yielded a best PCE of 13.45%. Wang et al. (2017a) had investigated the low-temperature (120 °C) annealed film of WOx and Nb-doped WOx, i.e., W(Nb)Ox in the flexible PSCs. The cell with pristine WOx yielded a PCE of 14.59%, and the introduction of Nb had increased the PCE to 15.65%. Chen et al. (2020) had prepared a solution-processed nano-crystalline WOx film at ultra-low temperature (50 °C) by using the precursor of WCl6 dissolved in different alcohols like ethanol, butanol, and hexanol named as E-WOx, B-WOx, and H-WOx. The PSCs produced by using the hexanol solvent and mixed perovskite have resulted in the best performance with a higher PCE of 20.77%, less charge recombination sites, and higher carrier mobility than the film produced by using other solvents.
Indium oxide (In2O3), having a wide band gap (∼3.75 eV) and a high carrier mobility (∼20 cm2 V−1 s −1), is also a promising alternative to TiO2 (Korotcenkov et al., 2005), (Lau et al., 2015). It also shows high transparency and good thermal stability. The performance of some In2O3 ETL based PSCs have been summarized in Table 6. In 2016, Qin et al. (2016) optimized the precursor (In(NO3)3· 4.5H2O+ ethanol) concentration and the annealing temperature (200 °C) of the solution-processed film of In2O3 for the MAPbI3-based PSCs. The PSCs with only In2O3 as an ETL have exceeded a PCE of 13%, and when it was used in combination with PCBM as other ETL, the cell yielded a best PCE of 14.83%. Chen et al. (2017) had also used the spin coating method to prepare the film of In2O3 for the PSCs, but the precursor of [In(NO3)3·4.5H2O+ ethanol] has been modified by adding acetylacetone to chelate In3+ and stabilize the precursor, which yielded the PCE of the cell (ITO/In2O3/MAPbI3/Spiro-OMeTAD/Ag) to be 15.3%. This modified precursor film of In2O3 has strongly quenched the photoluminescence (PL) emission peak intensity of perovskite, which indicates the efficient extraction of the carriers (i.e., the electrons) from the perovskite material. Guo et al. (2019b) used the fuel combustion method to prepare the precursor for spin coating the film of In2O3, and these films resulted in an electron mobility of 0.65 cm2V−1s -1, and after modification with PCBM, the device having a structure of ITO/In2O3/PCBM/mixed perovskite/Spiro-OMeTAD/Ag resulted in the best PCE of 18.12%. Yang et al. (2021b) had demonstrated a gradient potential structure for the ETL by using the bilayer of pristine In2O3 and Sn-doped In2O3. This bilayer provided a gradient potential to the charge carrier, which enhanced the charge separation, extraction, and suppressed the charge recombination. This, as a result, enhanced the Voc of the device (1.05 V for pristine In2O3 to 1.10 V for the bilayer), leading to a device efficiency of 20.65% in the ITO/NiOx/perovskite/Sn: In2O3/In2O3/Ag structure.
Having a wide optical band gap (∼4.1-eV), high transmission, higher carrier mobility, and a suitable conduction band energy level, Nb2O5 has also been investigated as an ETL and hole-blocking layer in PSCs (Yokoyama et al., 2020). The performance of some NbOx ETL based PSCs have been summarized in Table 7. Kogo et al. (2015) had studied solution-processed Nb2O5 as the hole-blocking layer in mesoporous Al2O3-based PSCs. The cells show a VOC up to 1.13 V, but the average PCE remains at only 8.8%. In this study, niobium ethoxide was used as a precursor to spin coat the film of niobium oxide. Zhang et al. (2018) investigated the different precursors like NbCl5 in ethanol and niobium ethoxide in isopropanol and in ethanol to prepare the amorphous NbOx film for PSCs, which was treated with UV-ozone without any further annealing. It has been found that it is too obstinate to remove many counter ions and solvent molecules like the chloride ion, which have a strong ionic interaction with Nb5+ and the IPA molecule by UVO treatment only. These impurities show an impact on the electrical properties like conductivity, carrier mobility, and defect concentration of the film, which further reduce the photovoltaic performance of the device. Furthermore, the ethanol molecules have a shorter molecular length, and the C2H5O− anion has a weak interaction with the Nb5+ ion. So, these can easily be escaped from the film by UV treatment only. This is why niobium ethoxide and ethanol are suitable precursors to prepare the film of amorphous NbOx. The NbOx film annealed at 500 °C has also been investigated, and it showed that high-temperature post-treatment is not required to achieve the high efficiency. The UVO-treated amorphous NbOx ETL-based PSCs with a FTO/NbOx/mixed perovskite/spiro-OMeTAD/Ag structure have shown the best PCE of 19.09%. Furthermore, the amorphous NbOx ETL-based PSCs showed resistance against UV light because of the large band gap (∼4.0 eV) of NbOx, thus leading to enhancement of the stability of these PSCs. Wang et al. (2019b) had used the colloidal Nb2O5 solution to prepare the spin-coated film of Nb2O5 at low temperature (150 °C) for PSCs having an FTO/Nb2O5/mixed perovskite/spiro-OMeTAD/Au structure. The device displayed good stability, and the highest PCE of 20.22% and also a higher VOC of 1.19 V.
Cerium oxide (CeOx) is a rare-earth oxide that has a wide band gap (∼3.5 eV), high ionic conductivity, large dielectric constant, and high thermal and chemical stability (Wang et al., 2017b). The performance of some CeOx ETL based PSCs have been summarized in Table 8. In 2017, Wang et al. (2017b) investigated a low temperature (∼150 °C) and sol–gel-processable CeOx films as an ETL in PSCs having an FTO/CeOx/MAPbI3/spiro-OMeTAD/Ag structure. These solar cells show a best PCE of 14.32% with better stability than the devices with TiO2 as the ETL, and when in addition to CeOx, a PC61BM layer was introduced in PSCs; the PCE was improved to 17.04%. The introduction of PC61BM has improved the charge extraction and the transmittance of the ETL and hence the device performance. The HOMO and LUMO energy levels of CeOx were found to be −7.5 and −4.0, respectively, by this group, which is suitable for electron extraction and transportation, as well as blocking of holes in PSCs.
Hu et al. (2018) had investigated CeOx as an ETL in the inverted structure (ITO/NiOx/Perovskite/CeOx/Ag) PSCs. They prepared the CeOx film via solution process with the cerium (III) acetylacetonate hydrate as a precursor, followed by low-temperature (100 °C) annealing, and the devices based on these showed the best PCE of 17.1%. In a nitrogen environment, the devices based on CeOx retained more than 90% of their performance after 200 h, which was greater than their PCBM-based counterparts, in which 80% was retained after 160 h. This is because the CeOx film resists the pinhole formation from the Ag electrode side, which isolates the perovskite film, and its degradation gets slow. Fang et al. (2018) had opted for a precursor of CeOx nanocrystals in chlorobenzene to fabricate high-quality nanocrystalline films of CeOx, which showed highly compact, pin-hole-free morphology and comparatively higher conductivity (∼10−4 S cm-1). This method has been used in fabricating the devices having the inverted device structure, i.e., FTO/NiMgLiO/MAPbI3/CeOx/Ag, which showed a best PCE of 16.65%. When a PC61BM layer has been introduced in addition to the CeOx film, the best cell PCE improved to 18.69%. Pang et al. (2020) had demonstrated the low-temperature (80 °C) and UV-O3 treated CeOx film in flexible PSCs having a PEN/ITO/CeOx/MAPbI3/spiro-OMeTAD/Au structure, which resulted in the best PCE of 14.63%.
In summary, we have provided a focused review of the different solution-processable metal oxide-based ETLs for PSCs. In metal oxides, TiO2 has largely been investigated as an ETL material, but the necessity of a high temperature for film growth and crystallization, which leads to superior performance of the corresponding PSCs, hampers the development of flexible photovoltaic devices. For these, other materials like ZnO and SnO2 have been investigated by researchers as an alternative to TiO2. ZnO-based PSCs show lower stability because of the degradation of the perovskite material due to the existence of the hydroxyl (-OH) group on the ZnO surface. In addition, SnO2 has emerged as a suitable low-temperature-processable ETL that has excellent optoelectronic properties, and the SnO2 ETL-based PSCs achieved a remarkable PCE of ∼25.5%. In addition to these, other metal oxides such as CeOx, NbOx, InOx, and WOx have also been investigated as a solution-processable ETL in PSCs. These have shown many favorable improvements relating to the efficiency and stability of the PSCs, but these have not been investigated largely by the photovoltaic research community. These can also emerge as the facile ETL for PSCs in the future when these will be investigated as ETLs in the PSCs largely, and more attention will be given to engineering the optoelectronic properties of these in favor of the emergence of excellent ETLs for PSCs.
All authors listed have made a substantial, direct, and intellectual contribution to the work and approved it for publication.
One of the authors HS, acknowledges UGC for grant of SRF. Authors are also very thankful to Dr. Venu Gopal Achanta, Director CSIR-NPL, INDIA for his constant encouragement and support to carry out these studies.
The authors declare that the research was conducted in the absence of any commercial or financial relationships that could be construed as a potential conflict of interest.
All claims expressed in this article are solely those of the authors and do not necessarily represent those of their affiliated organizations, or those of the publisher, the editors, and the reviewers. Any product that may be evaluated in this article, or claim that may be made by its manufacturer, is not guaranteed or endorsed by the publisher.
Aamir, M., Adhikari, T., Sher, M., Revaprasadu, N., Khalid, W., Akhtar, J., et al. (2018). Fabrication of planar heterojunction CsPbBr 2 I perovskite solar cells using ZnO as an electron transport layer and improved solar energy conversion efficiency. New J. Chem. 42 (17), 14104–14110. doi:10.1039/C8NJ02238K
Ahmadi, S. H., Ghaffarkani, M., Ameri, M., Safari, N., and Mohajerani, E. (2020). Solvent selection for fabrication of low temperature ZnO electron transport layer in perovskite solar cells. Opt. Mater (Amst) 106, 109977. doi:10.1016/j.optmat.2020.109977
Anaraki, E. H., Kermanpur, A., Steier, L., Domanski, K., Matsui, T., Tress, W., et al. (2016). Highly efficient and stable planar perovskite solar cells by solution-processed tin oxide. Energy Environ. Sci. 9 (10), 3128–3134. doi:10.1039/C6EE02390H
Andreani, L. C., Bozzola, A., Kowalczewski, P., Liscidini, M., and Redorici, L. (2019). Silicon solar cells: Toward the efficiency limits. Adv. Phys. X 4 (1), 1548305. doi:10.1080/23746149.2018.1548305
Ansari, M. I. H., Qurashi, A., and Nazeeruddin, M. K. (2018). Frontiers, opportunities, and challenges in perovskite solar cells: A critical review. J. Photochem. Photobiol. C Photochem. Rev. 35, 1–24. doi:10.1016/j.jphotochemrev.2017.11.002
Bao, Y., Wang, D., Hui, W., Gu, L., Chao, L., and Song, L. (2023). Honeycomb-type TiO 2 films toward a high tolerance to optical paths for perovskite solar cells. ChemSusChem 16 (2), e202201749. doi:10.1002/cssc.202201749
Barbé, J., Tietze, M. L., Neophytou, M., Murali, B., Alarousu, E., Labban, A. E., et al. (2017). Amorphous tin oxide as a low-temperature-processed electron-transport layer for organic and hybrid perovskite solar cells. ACS Appl. Mater Interfaces 9 (13), 11828–11836. doi:10.1021/acsami.6b13675
Bera, A., Wu, K., Sheikh, A., Alarousu, E., Mohammed, O. F., and Wu, T. (2014). Perovskite oxide SrTiO 3 as an efficient electron transporter for hybrid perovskite solar cells. J. Phys. Chem. C 118 (49), 28494–28501. doi:10.1021/jp509753p
Berak, J. M., and Sienko, M. J. (1970). Effect of oxygen-deficiency on electrical transport properties of tungsten trioxide crystals. J. Solid State Chem. 2 (1), 109–133. doi:10.1016/0022-4596(70)90040-X
Best Cell-Efficiency Chart (NREL) (2021). Best cell-efficiency Chart (NREL). Available at: https://www.nrel.gov/pv/cell-efficiency.html (accessed Feb. 01 2021).
Bi, D., Boschloo, G., Schwarzmüller, S., Yang, L., Johansson, E. M. J., and Hagfeldt, A. (2013). Efficient and stable CH3NH3PbI3-sensitized ZnO nanorod array solid-state solar cells. Nanoscale 5 (23), 11686. doi:10.1039/c3nr01542d
Brown, G. F., and Wu, J. (2009). Third generation photovoltaics. Laser Phot. Rev. 3 (4), 394–405. doi:10.1002/lpor.200810039
Chavan, R. D., Wolska-Pietkiewicz, M., Prochowicz, D., Jędrzejewska, M., Tavakoli, M. M., Yadav, P., et al. (2022). Organic ligand-free ZnO quantum dots for efficient and stable perovskite solar cells. Adv. Funct. Mater 32 (49), 2205909. doi:10.1002/adfm.202205909
Chen, C.-M., Lin, Z.-K., Huang, W.-J., and Yang, S.-H. (2016). WO3 nanoparticles or nanorods incorporating Cs2CO3/PCBM buffer bilayer as carriers transporting materials for perovskite solar cells. Nanoscale Res. Lett. 11 (1), 464. doi:10.1186/s11671-016-1670-8
Chen, C., Jiang, Y., Wu, Y., Guo, J., Kong, X., Wu, X., et al. (2020). Low-temperature-processed WO x as electron transfer layer for planar perovskite solar cells exceeding 20% efficiency. Sol. RRL 4 (4), 1900499. doi:10.1002/solr.201900499
Chen, P., Yin, X., Que, M., Liu, X., and Que, W. (2017). Low temperature solution processed indium oxide thin films with reliable photoelectrochemical stability for efficient and stable planar perovskite solar cells. J. Mater Chem. A Mater 5 (20), 9641–9648. doi:10.1039/C7TA00183E
Conings, B., Baeten, L., Jacobs, T., Dera, R., D’Haen, J., Manca, J., et al. (2014). An easy-to-fabricate low-temperature TiO 2 electron collection layer for high efficiency planar heterojunction perovskite solar cells. Apl. Mater 2 (8), 081505. doi:10.1063/1.4890245
Culu, A., Kaya, I. C., and Sonmezoglu, S. (2022). Spray-pyrolyzed tantalium-doped TiO 2 compact electron transport layer for UV-photostable planar perovskite solar cells exceeding 20% efficiency. ACS Appl. Energy Mater 5 (3), 3454–3462. doi:10.1021/acsaem.1c03848
Docampo, P., Ball, J. M., Darwich, M., Eperon, G. E., and Snaith, H. J. (2013). Efficient organometal trihalide perovskite planar-heterojunction solar cells on flexible polymer substrates. Nat. Commun. 4 (1), 2761. doi:10.1038/ncomms3761
Dong, Q., Li, J., Shi, Y., Chen, M., Ono, L. K., Zhou, K., et al. (2019). Improved SnO 2 electron transport layers solution-deposited at near room temperature for rigid or flexible perovskite solar cells with high efficiencies. Adv. Energy Mater 9, 1900834. doi:10.1002/aenm.201900834
Eze, V. O., Seike, Y., and Mori, T. (2017). Efficient planar perovskite solar cells using solution-processed amorphous WO x/fullerene C 60 as electron extraction layers. Org. Electron 46, 253–262. doi:10.1016/j.orgel.2017.04.024
Fang, R., Wu, S., Chen, W., Liu, Z., Zhang, S., Chen, R., et al. (2018). [6,6]-Phenyl-C 61 -butyric acid methyl ester/cerium oxide bilayer structure as efficient and stable electron transport layer for inverted perovskite solar cells. ACS Nano 12 (3), 2403–2414. doi:10.1021/acsnano.7b07754
GarcÃa-Sã¡nchez, M. F., Ortiz, A., Santana, G., Bizarro, M., Peña, J., Cruz-Gandarilla, F., et al. (2010). Synthesis and characterization of nanostructured cerium dioxide thin films deposited by ultrasonic spray pyrolysis. J. Am. Ceram. Soc. 93 (1), 155–160. doi:10.1111/j.1551-2916.2009.03374.x
Gheno, A., Thu Pham, T. T., Di Bin, C., Bouclé, J., Ratier, B., and Vedraine, S. (2017). Printable WO3 electron transporting layer for perovskite solar cells: Influence on device performance and stability. Sol. Energy Mater. Sol. Cells 161, 347–354. doi:10.1016/j.solmat.2016.10.002
Gil, B., Yun, A. J., Lim, J., Cho, J., Kim, B., Ryu, S., et al. (2023). Design of SnO 2 electron transport layer in perovskite solar cells to achieve 2000 h stability under 1 sun illumination and 85 °C. Adv. Mater Interfaces 10 (11). doi:10.1002/admi.202202148
Green, M. A., Ho-Baillie, A., and Snaith, H. J. (2014). The emergence of perovskite solar cells. Nat. Photonics 8 (7), 506–514. doi:10.1038/nphoton.2014.134
Gu, B., Zhu, Y., Lu, H., Tian, W., and Li, L. (2018). Efficient planar perovskite solar cells based on low-cost spin-coated ultrathin Nb2O5 films. Sol. Energy 166, 187–194. doi:10.1016/j.solener.2018.03.054
Guo, H., Chen, H., Zhang, H., Huang, X., Yang, J., Wang, B., et al. (2019). Low-temperature processed yttrium-doped SrSnO3 perovskite electron transport layer for planar heterojunction perovskite solar cells with high efficiency. Nano Energy 59, 1–9. doi:10.1016/j.nanoen.2019.01.059
Guo, X., Lin, Z., Ma, J., Hu, Z., Su, J., Zhang, C., et al. (2019). Low temperature combustion synthesized indium oxide electron transport layer for high performance and stable perovskite solar cells. J. Power Sources 438, 226981. doi:10.1016/j.jpowsour.2019.226981
Guo, Y., Tao, J., Jiang, J., Zhang, J., Yang, J., Chen, S., et al. (2018). Low temperature solution deposited niobium oxide films as efficient electron transport layer for planar perovskite solar cell. Sol. Energy Mater. Sol. Cells 188, 66–72. doi:10.1016/j.solmat.2018.08.020
Habisreutinger, S. N., Wenger, B., Snaith, H. J., and Nicholas, R. J. (2017). Dopant-free planar n–i–p perovskite solar cells with steady-state efficiencies exceeding 18%. ACS Energy Lett. 2 (3), 622–628. doi:10.1021/acsenergylett.7b00028
Han, D., Xin, Y., Yuan, Q., Yang, Q., Wang, Y., Yang, Y., et al. (2019). Solution-processed 2D Nb 2 O 5 (001) nanosheets for inverted CsPbI 2 Br perovskite solar cells: Interfacial and diffusion engineering. Sol. RRL 3 (7), 1900091. doi:10.1002/solr.201900091
Han, W., Wang, Y., Wan, J., and Wang, D. (2022). Eliminating hysteresis of perovskite solar cells with hollow TiO2 mesoporous electron transport layer. Chem. Res. Chin. Univ. 38 (1), 117–122. doi:10.1007/s40242-022-1401-x
Hou, Y., Quiroz, C. O. R., Scheiner, S., Chen, W., Stubhan, T., Hirsch, A., et al. (2015). Low-temperature and hysteresis-free electron-transporting layers for efficient, regular, and planar structure perovskite solar cells. Adv. Energy Mater 5 (20), 1501056. doi:10.1002/aenm.201501056
Hu, T., Xiao, S., Yang, H., Chen, L., and Chen, Y. (2018). Cerium oxide as an efficient electron extraction layer for p–i–n structured perovskite solar cells. Chem. Commun. 54 (5), 471–474. doi:10.1039/C7CC08657A
Huang, A., Zhu, J., Zhou, Y., Yu, Y., Liu, Y., Yang, S., et al. (2017). One step spray-coated TiO 2 electron-transport layers for decent perovskite solar cells on large and flexible substrates. Nanotechnology 28 (1), 01LT02. doi:10.1088/0957-4484/28/1/01LT02
Huang, L., Sun, X., Li, C., Xu, J., Xu, R., Du, Y., et al. (2017). UV-sintered low-temperature solution-processed SnO 2 as robust electron transport layer for efficient planar heterojunction perovskite solar cells. ACS Appl. Mater Interfaces 9 (26), 21909–21920. doi:10.1021/acsami.7b04392
Hussain, I., Tran, H. P., Jaksik, J., Moore, J., Islam, N., and Uddin, M. J. (2018). Functional materials, device architecture, and flexibility of perovskite solar cell. Emergent Mater 1 (3–4), 133–154. doi:10.1007/s42247-018-0013-1
Im, J.-H., Jang, I.-H., Pellet, N., Grätzel, M., and Park, N.-G. (2014). Growth of CH3NH3PbI3 cuboids with controlled size for high-efficiency perovskite solar cells. Nat. Nanotechnol. 9 (11), 927–932. doi:10.1038/nnano.2014.181
Ito, S., Tanaka, S., Vahlman, H., Nishino, H., Manabe, K., and Lund, P. (2014). Carbon-Double-bond-free printed solar cells from TiO 2/CH 3 NH 3 PbI 3/CuSCN/Au: Structural control and photoaging effects. ChemPhysChem 15 (6), 1194–1200. doi:10.1002/cphc.201301047
Jarwal, D. K., Mishra, A. K., Baral, K., Kumar, A., Kumar, C., Rawat, G., et al. (2022). Performance optimization of ZnO nanorods ETL based hybrid perovskite solar cells with different seed layers. IEEE Trans. Electron Devices 69 (5), 2494–2499. doi:10.1109/TED.2022.3162552
Jeong, I., Park, Y. H., Bae, S., Park, M., Jeong, H., Lee, P., et al. (2017). Solution-processed ultrathin TiO 2 compact layer hybridized with mesoporous TiO 2 for high-performance perovskite solar cells. ACS Appl. Mater Interfaces 9 (42), 36865–36874. doi:10.1021/acsami.7b11901
Jiang, Q., Chu, Z., Wang, P., Yang, X., Liu, H., Wang, Y., et al. (2017). Planar-structure perovskite solar cells with efficiency beyond 21%. Adv. Mater. 29 (46), 1703852. doi:10.1002/adma.201703852
Jung, H. S., and Park, N.-G. (2015). Perovskite solar cells: From materials to devices. Small 11 (1), 10–25. doi:10.1002/smll.201402767
Ke, W., Fang, G., Liu, Q., Xiong, L., Qin, P., Tao, H., et al. (2015). Low-temperature solution-processed tin oxide as an alternative electron transporting layer for efficient perovskite solar cells. J. Am. Chem. Soc. 137 (21), 6730–6733. doi:10.1021/jacs.5b01994
Khambunkoed, N., Homnan, S., Gardchareon, A., Chattrapiban, N., Songsiriritthigul, P., Wongratanaphisan, D., et al. (2021). Fully-covered slot-die-coated ZnO thin films for reproducible carbon-based perovskite solar cells. Mater Sci. Semicond. Process 136, 106151. doi:10.1016/j.mssp.2021.106151
Khatibi, A., Razi Astaraei, F., and Ahmadi, M. H. (2019). Generation and combination of the solar cells: A current model review. Energy Sci. Eng. 7 (2), 305–322. doi:10.1002/ese3.292
Kim, H.-S., Lee, C. R., Im, J. H., Lee, K. B., Moehl, T., Marchioro, A., et al. (2012). Lead iodide perovskite sensitized all-solid-state submicron thin film mesoscopic solar cell with efficiency exceeding 9%. Sci. Rep. 2 (1), 591. doi:10.1038/srep00591
Kim, H.-S., Lee, J. W., Yantara, N., Boix, P. P., Kulkarni, S. A., Mhaisalkar, S., et al. (2013). High efficiency solid-state sensitized solar cell-based on submicrometer rutile TiO 2 nanorod and CH 3 NH 3 PbI 3 perovskite sensitizer. Nano Lett. 13 (6), 2412–2417. doi:10.1021/nl400286w
Kim, H.-S., Mora-Sero, I., Gonzalez-Pedro, V., Fabregat-Santiago, F., Juarez-Perez, E. J., Park, N. G., et al. (2013). Mechanism of carrier accumulation in perovskite thin-absorber solar cells. Nat. Commun. 4 (1), 2242. doi:10.1038/ncomms3242
Kim, H.-S., and Park, N.-G. (2014). Parameters affecting I – V hysteresis of CH 3 NH 3 PbI 3 perovskite solar cells: Effects of perovskite crystal size and mesoporous TiO 2 layer. J. Phys. Chem. Lett. 5 (17), 2927–2934. doi:10.1021/jz501392m
Kim, J., Kim, G., Kim, T. K., Kwon, S., Back, H., Lee, J., et al. (2014). Efficient planar-heterojunction perovskite solar cells achieved via interfacial modification of a sol–gel ZnO electron collection layer. J. Mat. Chem. A 2 (41), 17291–17296. doi:10.1039/C4TA03954H
Kogo, A., Numata, Y., Ikegami, M., and Miyasaka, T. (2015). Nb 2 O 5 blocking layer for high open-circuit voltage perovskite solar cells. Chem. Lett. 44 (6), 829–830. doi:10.1246/cl.150167
Kojima, A., Teshima, K., Shirai, Y., and Miyasaka, T. (2009). Organometal halide perovskites as visible-light sensitizers for photovoltaic cells. J. Am. Chem. Soc. 131 (17), 6050–6051. doi:10.1021/ja809598r
Korotcenkov, G., Brinzari, V., Ivanov, M., Cerneavschi, A., Rodriguez, J., Cirera, A., et al. (2005). Structural stability of indium oxide films deposited by spray pyrolysis during thermal annealing. Thin Solid Films 479 (1–2), 38–51. doi:10.1016/j.tsf.2004.11.107
Kumar, M. H., Yantara, N., Dharani, S., Graetzel, M., Mhaisalkar, S., Boix, P. P., et al. (2013). Flexible, low-temperature, solution processed ZnO-based perovskite solid state solar cells. Chem. Commun. 49 (94), 11089. doi:10.1039/c3cc46534a
Kumar, N., Lee, H. B., Sahani, R., Tyagi, B., Cho, S., Lee, J., et al. (2022). Room-temperature spray deposition of large-area SnO 2 electron transport layer for high performance, stable FAPbI 3 -based perovskite solar cells. Small Methods 6 (2), 2101127. doi:10.1002/smtd.202101127
Lau, L. N., Ibrahim, N. B., and Baqiah, H. (2015). Influence of precursor concentration on the structural, optical and electrical properties of indium oxide thin film prepared by a sol–gel method. Appl. Surf. Sci. 345, 355–359. doi:10.1016/j.apsusc.2015.03.129
Lee, H. B., Kumar, N., Ovhal, M. M., Kim, Y. J., Song, Y. M., and Kang, J. (2020). Dopant-free, amorphous–crystalline heterophase SnO 2 electron transport bilayer enables >20% efficiency in triple-cation perovskite solar cells. Adv. Funct. Mater 30 (24), 2001559. doi:10.1002/adfm.202001559
Lee, J.-W., Lee, T.-Y., Yoo, P. J., Grätzel, M., Mhaisalkar, S., and Park, N.-G. (2014). Rutile TiO2-based perovskite solar cells. J. Mater Chem. A Mater 2 (24), 9251. doi:10.1039/c4ta01786b
Lee, M. M., Teuscher, J., Miyasaka, T., Murakami, T. N., and Snaith, H. J. (2012). Efficient hybrid solar cells based on meso-superstructured organometal halide perovskites. Science 338 (6107), 643–647. doi:10.1126/science.1228604
Leng, S., Wang, L., Wang, X., Zhang, Z., Liang, J., Zheng, Y., et al. (2021). Bottom interfacial engineering for methylammonium-free regular-structure planar perovskite solar cells over 21%. Sol. RRL 5 (8), 2100285. doi:10.1002/solr.202100285
Li, F., Shen, Z., Weng, Y., Lou, Q., Chen, C., Shen, L., et al. (2020). Novel electron transport layer material for perovskite solar cells with over 22% efficiency and long-term stability. Adv. Funct. Mater 30 (45), 2004933. doi:10.1002/adfm.202004933
Li, T., Rui, Y., Wang, X., Shi, J., Wang, Y., Yang, J., et al. (2021). Grain size and interface modification via cesium carbonate post-treatment for efficient SnO 2 -based planar perovskite solar cells. ACS Appl. Energy Mater 4 (7), 7002–7011. doi:10.1021/acsaem.1c01055
Li, Y., Zhu, J., Huang, Y., Liu, F., Lv, M., Chen, S., et al. (2015). Mesoporous SnO 2 nanoparticle films as electron-transporting material in perovskite solar cells. RSC Adv. 5 (36), 28424–28429. doi:10.1039/C5RA01540E
Liang, C., Wu, Z., Li, P., Fan, J., Zhang, Y., and Shao, G. (2017). Chemical bath deposited rutile TiO 2 compact layer toward efficient planar heterojunction perovskite solar cells. Appl. Surf. Sci. 391, 337–344. doi:10.1016/j.apsusc.2016.06.171
Lin, S., Yang, B., Qiu, X., Yan, J., Shi, J., Yuan, Y., et al. (2018). Efficient and stable planar hole-transport-material-free perovskite solar cells using low temperature processed SnO2 as electron transport material. Org. Electron 53, 235–241. doi:10.1016/j.orgel.2017.12.002
Liu, B.-T., Guo, B.-W., and Balamurugan, R. (2020). Effect of polyethylene glycol incorporation in electron transport layer on photovoltaic properties of perovskite solar cells. Nanomaterials 10 (9), 1753. doi:10.3390/nano10091753
Liu, D., and Kelly, T. L. (2014). Perovskite solar cells with a planar heterojunction structure prepared using room-temperature solution processing techniques. Nat. Photonics 8 (2), 133–138. doi:10.1038/nphoton.2013.342
Liu, J., Yao, Y., Xiao, S., and Gu, X. (2018). Review of status developments of high-efficiency crystalline silicon solar cells. J. Phys. D. Appl. Phys. 51 (12), 123001. doi:10.1088/1361-6463/aaac6d
Liu, M., Johnston, M. B., and Snaith, H. J. (2013). Efficient planar heterojunction perovskite solar cells by vapour deposition. Nature 501 (7467), 395–398. doi:10.1038/nature12509
Liu, X., Tsai, K.-W., Zhu, Z., Sun, Y., Chueh, C.-C., and Jen, A. K.-Y. (2016). A low-temperature, solution processable tin oxide electron-transporting layer prepared by the dual-fuel combustion method for efficient perovskite solar cells. Adv. Mater Interfaces 3 (13), 1600122. doi:10.1002/admi.201600122
Lu, H., Zhong, J., Ji, C., Zhao, J., and Zhao, R. (2020). Fabricating an optimal rutile TiO2 electron transport layer by delicately tuning TiCl4 precursor solution for high performance perovskite solar cells. Nano Energy 68, 104336. doi:10.1016/j.nanoen.2019.104336
Mahmood, K., Swain, B. S., and Amassian, A. (2014). Double-layered ZnO nanostructures for efficient perovskite solar cells. Nanoscale 6 (24), 14674–14678. doi:10.1039/C4NR04383A
Mahmood, K., Swain, B. S., and Jung, H. S. (2014). Controlling the surface nanostructure of ZnO and Al-doped ZnO thin films using electrostatic spraying for their application in 12% efficient perovskite solar cells. Nanoscale 6 (15), 9127. doi:10.1039/C4NR02065K
Mahmood, K., Swain, B. S., Kirmani, A. R., and Amassian, A. (2015). Highly efficient perovskite solar cells based on a nanostructured WO 3 –TiO 2 core–shell electron transporting material. J. Mater Chem. A Mater 3 (17), 9051–9057. doi:10.1039/C4TA04883K
Mahmud, M. A., Elumalai, N. K., Upama, M. B., Wang, D., Chan, K. H., Wright, M., et al. (2017). Low temperature processed ZnO thin film as electron transport layer for efficient perovskite solar cells. Sol. Energy Mater. Sol. Cells 159, 251–264. doi:10.1016/j.solmat.2016.09.014
Mandati, S., Dileep, R. K., Veerappan, G., and Ramasamy, E. (2022). Large area bar coated TiO2 electron transport layers for perovskite solar cells with excellent performance homogeneity. Sol. Energy 240, 258–268. doi:10.1016/j.solener.2022.04.060
Masi, S., Mastria, R., Scarfiello, R., Carallo, S., Nobile, C., Gambino, S., et al. (2018). Room-temperature processed films of colloidal carved rod-shaped nanocrystals of reduced tungsten oxide as interlayers for perovskite solar cells. Phys. Chem. Chem. Phys. 20 (16), 11396–11404. doi:10.1039/C8CP00645H
Méndez, P. F., Muhammed, S. K. M., Barea, E. M., Masi, S., and Mora-Seró, I. (2019). Analysis of the UV–Ozone-Treated SnO 2 electron transporting layer in planar perovskite solar cells for high performance and reduced hysteresis. Sol. RRL 3 (9), 1900191. doi:10.1002/solr.201900191
Mesquita, I., Andrade, L., and Mendes, A. (2017). Perovskite solar cells: Materials, configurations and stability. Renew. Sustain. Energy Rev. 82, 2471–2489. doi:10.1016/j.rser.2017.09.011
Min, H., Lee, D. Y., Kim, J., Kim, G., Lee, K. S., Kim, J., et al. (2021). Perovskite solar cells with atomically coherent interlayers on SnO2 electrodes. Nature 598 (7881), 444–450. doi:10.1038/s41586-021-03964-8
Murugadoss, G., Kanda, H., Tanaka, S., Nishino, H., Ito, S., Imahori, H., et al. (2016). An efficient electron transport material of tin oxide for planar structure perovskite solar cells. J. Power Sources 307, 891–897. doi:10.1016/j.jpowsour.2016.01.044
Nguyen, M. H., Yoon, S.-H., and Kim, K.-S. (2022). Hydrothermally fabricated TiO2 heterostructure boosts efficiency of MAPbI3 perovskite solar cells. J. Industrial Eng. Chem. 106, 382–392. doi:10.1016/j.jiec.2021.11.013
Noh, Y. W., Jin, I. S., Park, S. H., and Jung, J. W. (2020). Room-temperature synthesis of ZrSnO4 nanoparticles for electron transport layer in efficient planar hetrojunction perovskite solar cells. J. Mater Sci. Technol. 42, 38–45. doi:10.1016/j.jmst.2019.11.008
Ono, L. K., Juarez-Perez, E. J., and Qi, Y. (2017). Progress on perovskite materials and solar cells with mixed cations and halide anions. ACS Appl. Mater Interfaces 9 (36), 30197–30246. doi:10.1021/acsami.7b06001
Orel, Z. C., and Orel, B. (1994). Optical properties of pure CeO2 and mixed CeO2/SnO2 thin film coatings. Phys. status solidi (b) 186 (1), K33–K36. doi:10.1002/pssb.2221860135
Pang, A., Li, J., Wei, X.-F., Ruan, Z.-W., Yang, M., and Chen, Z.-N. (2020). UV–O 3 treated annealing-free cerium oxide as electron transport layers in flexible planar perovskite solar cells. Nanoscale Adv. 2 (9), 4062–4069. doi:10.1039/D0NA00367K
Qin, M., Ma, J., Ke, W., Qin, P., Lei, H., Tao, H., et al. (2016). Perovskite solar cells based on low-temperature processed indium oxide electron selective layers. ACS Appl. Mater Interfaces 8 (13), 8460–8466. doi:10.1021/acsami.5b12849
Qin, P., Tanaka, S., Ito, S., Tetreault, N., Manabe, K., Nishino, H., et al. (2014). Inorganic hole conductor-based lead halide perovskite solar cells with 12.4% conversion efficiency. Nat. Commun. 5 (1), 3834. doi:10.1038/ncomms4834
Qiu, J., Qiu, Y., Yan, K., Zhong, M., Mu, C., Yan, H., et al. (2013). All-solid-state hybrid solar cells based on a new organometal halide perovskite sensitizer and one-dimensional TiO2 nanowire arrays. Nanoscale 5 (8), 3245. doi:10.1039/c3nr00218g
Ren, X., Wang, Z. S., and Choy, W. C. H. (2019). Device Physics of the carrier transporting layer in planar perovskite solar cells. Adv. Opt. Mater 7 (20), 1900407–1900423. doi:10.1002/adom.201900407
Rong, Y., Ku, Z., Mei, A., Liu, T., Xu, M., Ko, S., et al. (2014). Hole-Conductor-free mesoscopic TiO 2/CH 3 NH 3 PbI 3 heterojunction solar cells based on anatase nanosheets and carbon counter electrodes. J. Phys. Chem. Lett. 5 (12), 2160–2164. doi:10.1021/jz500833z
Roose, B., Baena, J. P. C., Gödel, K. C., Graetzel, M., Hagfeldt, A., Steiner, U., et al. (2016). Mesoporous SnO2 electron selective contact enables UV-stable perovskite solar cells. Nano Energy 30, 517–522. doi:10.1016/j.nanoen.2016.10.055
Seo, Y. S., Lee, C., Lee, K. H., and Yoon, K. B. (2005). 1:1 and 2:1 charge-transfer complexes between aromatic hydrocarbons and dry Titanium dioxide. Angew. Chem. Int. Ed. 44 (6), 910–913. doi:10.1002/anie.200461972
Shah, A. V., Platz, R., and Keppner, H. (1995). Thin-film silicon solar cells: A review and selected trends. Sol. Energy Mater. Sol. Cells 38 (1–4), 501–520. doi:10.1016/0927-0248(94)00241-X
Shen, D., Zhang, W., Li, Y., Abate, A., and Wei, M. (2018). Facile deposition of Nb 2 O 5 thin film as an electron-transporting layer for highly efficient perovskite solar cells. ACS Appl. Nano Mater 1 (8), 4101–4109. doi:10.1021/acsanm.8b00859
Shin, S. S., Yeom, E. J., Yang, W. S., Hur, S., Kim, M. G., Im, J., et al. (2017). Colloidally prepared La-doped BaSnO 3 electrodes for efficient, photostable perovskite solar cells. Science 356 (6334), 167–171. doi:10.1126/science.aam6620
Singh, T., Singh, J., and Miyasaka, T. (2016). Role of metal oxide electron-transport layer modification on the stability of high performing perovskite solar cells. ChemSusChem 9 (18), 2559–2566. doi:10.1002/cssc.201601004
Son, D.-Y., Im, J.-H., Kim, H.-S., and Park, N.-G. (2014). 11% efficient perovskite solar cell based on ZnO nanorods: An effective charge collection system. J. Phys. Chem. C 118 (30), 16567–16573. doi:10.1021/jp412407j
Song, J., Zheng, E., Bian, J., Wang, X. F., Tian, W., Sanhiera, Y., et al. (2015). Low-temperature SnO 2 -based electron selective contact for efficient and stable perovskite solar cells. J. Mater Chem. A Mater 3 (20), 10837–10844. doi:10.1039/C5TA01207D
Sun, Y., Seo, J. H., Takacs, C. J., Seifter, J., and Heeger, A. J. (2011). Inverted polymer solar cells integrated with a low-temperature-annealed sol-gel-derived ZnO film as an electron transport layer. Adv. Mater. 23 (14), 1679–1683. doi:10.1002/adma.201004301
Suzuki, T., Kosacki, I., Anderson, H. U., and Colomban, P. (2004). Electrical conductivity and lattice defects in nanocrystalline cerium oxide thin films. J. Am. Ceram. Soc. 84 (9), 2007–2014. doi:10.1111/j.1151-2916.2001.tb00950.x
Tian, W., Song, P., Zhao, Y., Shen, L., Liu, K., Zheng, L., et al. (2022). Monolithic bilayered in 2 O 3 as an efficient interfacial material for high-performance perovskite solar cells. Interdiscip. Mater. 1 (4), 526–536. doi:10.1002/idm2.12047
Tvrdy, K., and Kamat, P. V. (2011). Quantum dot solar cells. Compr. Nanosci. Technol. 1 (5), 257–275. doi:10.1016/B978-0-12-374396-1.00129-X
Wang, E., Chen, P., Yin, X., Wu, Y., and Que, W. (2020). Novel ethanol vapor annealing treatment of SnO2 quantum dots film for highly efficient planar heterojunction perovskite solar cells. Org. Electron 84, 105751. doi:10.1016/j.orgel.2020.105751
Wang, F., Di Valentin, C., and Pacchioni, G. (2012). Rational band gap engineering of WO3 photocatalyst for visible light water splitting. ChemCatChem 4 (4), 476–478. doi:10.1002/cctc.201100446
Wang, H., Liu, H., Ye, F., Chen, Z., Ma, J., Liang, J., et al. (2021). Hydrogen peroxide-modified SnO2 as electron transport layer for perovskite solar cells with efficiency exceeding 22%. J. Power Sources 481, 229160. doi:10.1016/j.jpowsour.2020.229160
Wang, K., Shi, Y., Dong, Q., Li, Y., Wang, S., Yu, X., et al. (2015). Low-temperature and solution-processed amorphous WOX as electron-selective layer for perovskite solar cells. J. Phys. Chem. Lett. 6 (5), 755–759. doi:10.1021/acs.jpclett.5b00010
Wang, K., Shi, Y., Gao, L., Chi, R., Shi, K., Guo, B., et al. (2017). W(Nb)O x -based efficient flexible perovskite solar cells: From material optimization to working principle. Nano Energy 31, 424–431. doi:10.1016/j.nanoen.2016.11.054
Wang, K., Shi, Y., Li, B., Zhao, L., Wang, W., Wang, X., et al. (2016). Amorphous inorganic electron-selective layers for efficient perovskite solar cells: Feasible strategy towards room-temperature fabrication. Adv. Mater. 28 (9), 1891–1897. doi:10.1002/adma.201505241
Wang, Q., Peng, C., Du, L., Li, H., Zhang, W., Xie, J., et al. (2020). Enhanced performance of perovskite solar cells via low-temperature-processed mesoporous SnO 2. Adv. Mater Interfaces 7 (4), 1901866. doi:10.1002/admi.201901866
Wang, R., Mujahid, M., Duan, Y., Wang, Z. K., Xue, J., and Yang, Y. (2019). A review of perovskites solar cell stability. Adv. Funct. Mater 29 (47), 1808843. doi:10.1002/adfm.201808843
Wang, X., Deng, L. L., Wang, L. Y., Dai, S. M., Xing, Z., Zhan, X. X., et al. (2017). Cerium oxide standing out as an electron transport layer for efficient and stable perovskite solar cells processed at low temperature. J. Mater Chem. A Mater 5 (4), 1706–1712. doi:10.1039/C6TA07541J
Wang, Z., Lou, J., Zheng, X., Zhang, W.-H., and Qin, Y. (2019). Solution processed Nb 2 O 5 electrodes for high efficient ultraviolet light stable planar perovskite solar cells. ACS Sustain Chem. Eng. 7 (7), 7421–7429. doi:10.1021/acssuschemeng.9b00991
Wojciechowski, K., Saliba, M., Leijtens, T., Abate, A., and Snaith, H. J. (2014). Sub-150 °C processed meso-superstructured perovskite solar cells with enhanced efficiency. Energy Environ. Sci. 7 (3), 1142–1147. doi:10.1039/C3EE43707H
Wu, Y., Yang, X., Chen, H., Zhang, K., Qin, C., Liu, J., et al. (2014). Highly compact TiO 2 layer for efficient hole-blocking in perovskite solar cells. Appl. Phys. Express 7 (5), 052301. doi:10.7567/APEX.7.052301
Xing, Z., Li, S. H., Wu, B. S., Wang, X., Wang, L. Y., Wang, T., et al. (2018). Photovoltaic performance and stability of fullerene/cerium oxide double electron transport layer superior to single one in p-i-n perovskite solar cells. J. Power Sources 389, 13–19. doi:10.1016/j.jpowsour.2018.03.079
Xiong, Z., Lan, L., Wang, Y., Lu, C., Qin, S., Chen, S., et al. (2021). Multifunctional polymer framework modified SnO 2 enabling a photostable α-FAPbI 3 perovskite solar cell with efficiency exceeding 23. ACS Energy Lett. 6 (11), 3824–3830. doi:10.1021/acsenergylett.1c01763
Xu, Z., Huang, L., Jiang, Y., Li, Z., Chen, C., He, Z., et al. (2022). Thermal annealing-free SnO 2 for fully room-temperature-processed perovskite solar cells. ACS Appl. Mater Interfaces 14 (36), 41037–41044. doi:10.1021/acsami.2c11488
Xu, Z., Jiang, Y., Li, Z., Chen, C., Kong, X., Chen, Y., et al. (2021). Rapid microwave-assisted synthesis of SnO 2 quantum dots for efficient planar perovskite solar cells. ACS Appl. Energy Mater 4 (2), 1887–1893. doi:10.1021/acsaem.0c02992
Yang, B., Ma, R., Wang, Z., Ouyang, D., Huang, Z., Lu, J., et al. (2021). Efficient gradient potential top electron transport structures achieved by combining an oxide family for inverted perovskite solar cells with high efficiency and stability. ACS Appl. Mater Interfaces 13 (23), 27179–27187. doi:10.1021/acsami.1c05284
Yang, G., Chen, C., Yao, F., Chen, Z., Zhang, Q., Zheng, X., et al. (2018). Effective carrier-concentration tuning of SnO 2 quantum dot electron-selective layers for high-performance planar perovskite solar cells. Adv. Mater. 30 (14), 1706023. doi:10.1002/adma.201706023
Yang, J., Siempelkamp, B. D., Mosconi, E., De Angelis, F., and Kelly, T. L. (2015). Origin of the thermal instability in CH 3 NH 3 PbI 3 thin films deposited on ZnO. Chem. Mater. 27 (12), 4229–4236. doi:10.1021/acs.chemmater.5b01598
Yang, J., Zhang, Q., Xu, J., Liu, H., Qin, R., Zhai, H., et al. (2019). All-inorganic perovskite solar cells based on CsPbIBr2 and metal oxide transport layers with improved stability. Nanomaterials 9 (12), 1666. doi:10.3390/nano9121666
Yang, Z., Chen, W., Mei, A., Li, Q., and Liu, Y. (2021). Flexible MAPbI3 perovskite solar cells with the high efficiency of 16.11% by low-temperature synthesis of compact anatase TiO2 film. J. Alloys Compd. 854, 155488. doi:10.1016/j.jallcom.2020.155488
Ye, X., Ling, H., Zhang, R., Wen, Z., Hu, S., Akasaka, T., et al. (2020). Low-temperature solution-combustion-processed Zn-Doped Nb2O5 as an electron transport layer for efficient and stable perovskite solar cells. J. Power Sources 448, 227419. doi:10.1016/j.jpowsour.2019.227419
Yella, A., Heiniger, L.-P., Gao, P., Nazeeruddin, M. K., and Grätzel, M. (2014). Nanocrystalline rutile electron extraction layer enables low-temperature solution processed perovskite photovoltaics with 13.7% efficiency. Nano Lett. 14 (5), 2591–2596. doi:10.1021/nl500399m
Yokoyama, T., Nishitani, Y., Miyamoto, Y., Kusumoto, S., Uchida, R., Matsui, T., et al. (2020). Improving the open-circuit voltage of Sn-based perovskite solar cells by band alignment at the electron transport layer/perovskite layer interface. ACS Appl. Mater Interfaces 12 (24), 27131–27139. doi:10.1021/acsami.0c04676
Yoo, J. J., Seo, G., Chua, M. R., Park, T. G., Lu, Y., Rotermund, F., et al. (2021). Efficient perovskite solar cells via improved carrier management. Nature 590 (7847), 587–593. doi:10.1038/s41586-021-03285-w
Yoon, S., Kim, S. J., Kim, H. S., Park, J. S., Han, I. K., Jung, J. W., et al. (2017). Solution-processed indium oxide electron transporting layers for high-performance and photo-stable perovskite and organic solar cells. Nanoscale 9 (42), 16305–16312. doi:10.1039/C7NR05695H
Zhang, C., Shi, Y., Wang, S., Dong, Q., Feng, Y., Wang, L., et al. (2018). Room-temperature solution-processed amorphous NbO x as an electron transport layer in high-efficiency photovoltaics. J. Mater Chem. A Mater 6 (37), 17882–17888. doi:10.1039/C8TA06436A
Zhang, J., Shi, C., Chen, J., Wang, Y., and Li, M. (2016). Preparation of ultra-thin and high-quality WO3 compact layers and comparision of WO3 and TiO2 compact layer thickness in planar perovskite solar cells. J. Solid State Chem. 238, 223–228. doi:10.1016/j.jssc.2016.03.033
Zhang, X., Li, J., Bi, Z., He, K., Xu, X., Xiao, X., et al. (2020). Stable and efficient air-processed perovskite solar cells employing low-temperature processed compact In2O3 thin films as electron transport materials. J. Alloys Compd. 836, 155460. doi:10.1016/j.jallcom.2020.155460
Zhou, Y., Li, X., and Lin, H. (2020). To Be higher and stronger—metal oxide electron transport materials for perovskite solar cells. Small 16 (15), 1902579. doi:10.1002/smll.201902579
Zhu, G., Shen, Y., Xu, K., Huangfu, M., Cao, M., Gu, F., et al. (2016). Preparation of ZnO electron transport layers by spray technology for perovskite solar cells. J. Alloys Compd. 689, 192–198. doi:10.1016/j.jallcom.2016.07.182
Keywords: electron transport layers, metal oxides, perovskite solar cell, sol–gel process, hybrid perovskite materials
Citation: Sharma H and Srivastava R (2023) Solution-processed pristine metal oxides as electron-transporting materials for perovskite solar cells. Front. Electron. Mater. 3:1174159. doi: 10.3389/femat.2023.1174159
Received: 28 February 2023; Accepted: 19 May 2023;
Published: 21 June 2023.
Edited by:
Jie Su, Xidian University, ChinaReviewed by:
Chong Chen, Chinese Academy of Sciences (CAS), ChinaCopyright © 2023 Sharma and Srivastava. This is an open-access article distributed under the terms of the Creative Commons Attribution License (CC BY). The use, distribution or reproduction in other forums is permitted, provided the original author(s) and the copyright owner(s) are credited and that the original publication in this journal is cited, in accordance with accepted academic practice. No use, distribution or reproduction is permitted which does not comply with these terms.
*Correspondence: Harshit Sharma, aGFyc2hpdC5ucGwxOWFAYWNzaXIucmVzLmlu
Disclaimer: All claims expressed in this article are solely those of the authors and do not necessarily represent those of their affiliated organizations, or those of the publisher, the editors and the reviewers. Any product that may be evaluated in this article or claim that may be made by its manufacturer is not guaranteed or endorsed by the publisher.
Research integrity at Frontiers
Learn more about the work of our research integrity team to safeguard the quality of each article we publish.