- 1State Key Laboratory of Superhard Materials, College of Physics, Jilin University, Changchun, China
- 2School of Physical Science and Technology, Ningbo University, Ningbo, China
Room-temperature superconductors have long been the ultimate goal of scientists. Pressure-stabilized hydrides are a new rapidly growing class of high-temperature superconductors and are believed to be a new superconducting system, undoubtedly leading to a surge in the discovery of new hydrogen-rich materials. They are the forefront of physics and material science. Lanthanide polyhydrides formed under pressure are promising conventional superconductors. Especially, both the theoretical and experimental reports on lanthanum superhydrides under pressure, exhibiting superconductivity at temperatures as high as 250 K, have further stimulated an intense search for room-temperature superconductors in hydrides. This review focuses on the recent advances of crystal structures, stabilities, and superconductivity of lanthanide polyhydrides at high pressures, including the experimental results from our group. By using in situ four-probe electrical measurements and the synchrotron X-ray diffraction technique, we have identified several high-temperature superconducting phases: a lanthanum superhydride and two cerium superhydrides. The present work indicates that superconductivity declines along the La–Ce–Pr–Nd series, while magnetism becomes more and more pronounced. These discoveries have enriched the binary system of clathrate superhydrides and provided more hints for studying the role of rare earth metal elements having high-temperature superconductivity.
Introduction
Since Kamerlingh Onnes first discovered superconductivity with Tc∼4.2 K in mercury (Onnes, 1911), room-temperature superconductivity has been one of the most challenging projects in multiple fields such as physics and material science. In 1957, Bardeen, Cooper, and Schrieffer established the microscopic BCS theory to provide an explanation for conventional superconductors (Bardeen et al., 1957), which was also considered a road map to high-temperature superconductors. For these years, the search for superconducting materials with higher Tc has been ongoing. The famous and representative conventional superconductor MgB2 had a Tc of 39 K at ambient pressure (Nagamatsu et al., 2001), which was far lower than the Tc for cuprates with 133 K (Iqbal et al., 1994). By the application of hydrostatic pressure, Tc of the HgBaCaCuO compound was increased to ∼164 K (Gao et al., 1994). However, cuprate-based superconductors are beyond the conventional BCS theory, and there is shortage of a generally accepted theory to explain the microscopic mechanism of them.
Back in 1935, Winger and Huntington proposed that hydrogen could be metallized under high pressure (Winger et al., 1935). According to the BCS theory, high phonon frequencies, large electronic density of states (DOS) near the Fermi level, and strong electron phonon coupling (EPC) can result in high Tc. In 1968, Ashcroft predicted that the realization of solid metallic hydrogen would open the pathway to high Tc superconductors (Ashcroft., 1968). Achieving a metallic state of hydrogen, referred to as metallic hydrogen, is dubbed as the Holy Grail in the high-pressure community. Experimental evidences on the transformation of hydrogen to the atomic state were reported close to 500 GPa (Loubeyre et al., 2020; Dias et al., 2017; Eremets et al., 2019), but such higher pressure limited its properties and application research. In 2004, Ashcroft suggested an alternative approach that metallization and superconductivity of hydrogen-rich compounds existed at much lower pressures (Ashcroft., 2004). Only 10 years later, Ashcroft’s idea found its experimental proofs, and extraordinarily, high-temperature superconductivities were demonstrated in compressed H3S and LaH10 (Drozdov et al., 2015; Einaga et al., 2016; Troyan et al., 2016; Huang et al., 2019; Somayazulu et al., 2019; Drozdov et al., 2019). It has been found that binary hydride LaH10 can achieve high temperature superconductivity of 250–260 K, representing a new breakthrough in the binary system until now. These encouraging results have spurred a flurry of interests in other compressed hydrides, particularly for the ones from the same group. In this review, we are going to summarize the recent progresses on the lanthanide polyhydrides under high pressure. Through the discussions, we hope that some hints could be concluded for future studies in new hydrides with high-temperature superconductivity.
Dicussions
Lanthanum Superhydride
Lanthanum superhydride LaH10 was first theoretically reported as a good candidate for high-Tc superconductivity (Peng et al., 2017; Liu et al., 2017), before experiments confirmed its high-temperature superconductivity (Somayazulu et al., 2019). Peng et al. and Liu et al. have independently performed systematic density functional theory (DFT) studies and proposed that the high-temperature superconducting phase fcc-LaH10 with Tc reaching 280 K. fcc-LaH10 showed a clathrate-like structure constituted by H cages, with La atoms occupying the fcc lattice positions. The high Tc has been explained as the strong electron -phonon coupling, which is associated with the strongly hybridized La f and H s orbitals (Liu et al., 2019; Wang et al., 2020). These theoretical predictions that lanthanum superhydride has great potential to be a near room-temperature superconductor have motivated experimental verifications. However, such experiments are very challenging, limited by hydrogen permeability and sample size.
Several experimental groups have successfully synthesized the predicted fcc-LaH10 phase by laser heating La metal with pure hydrogen or ammonia borane (NH3BH3) at high pressure in a diamond anvil cell. The initial experimental observation of LaHx with Tc at 215 K and 150 GPa was claimed by Drozdov et al. (Drozdov et al., 2018). They have obtained the target sample by heating the sample of La and pure hydrogen below 1,000 K at 170 GPa. The sample had a Tc of 209 K at 170 GPa, which increased to 215 K when the pressure reduced to 150 GPa. Then, Somayazulu et al. reported the superconducting lanthanum superhydride with Tc ∼ 260 K at 180–200 GPa (Somayazulu et al., 2019). Different from Drozdov et al., they chose ammonia borane as the hydrogen source. In addition, they also found that Tc decreased with increasing measuring current. Subsequently, Drozdov et al. changed the synthesized conditions and reported that LaH10 had a highest Tc of 250 K at 170 GPa and decreased linearly as pressure increased (Drozdov et al., 2019). In their work, they also confirmed the crystal structure of LaH10 as Fm
During this time, we have also explored the superconductivity in the lanthanum superhydride. We used NH3BH3 as the hydrogen source considering the heating decompression reaction: NH3BH3→3H2+c-BN (Chen et al., 2021; Shao et al., 2021b; Kondrat’ev et al., 2015). The La and NH3BH3 samples were loaded inside the hole of the gasket. We have applied the four-probe method to study the electrical resistance, and four Mo electrodes were sputtered onto the diamonds with a top flat of 60 μm beveled 300 μm, which have been successfully used in our previous work (Chen et al., 2021). The Raman shift of diamond was measured to calibrate the pressure (Akahama et al., 2006). By laser heating a mixture of La and NH3BH3 to about 1,400 K at 171 GPa, a resistance drop was triggered at about 238.4 K during the cooling cycle (Figure 1A). To further confirm this superconductivity, we have also measured the temperature-dependent electrical resistance under a series of applied magnetic fields from 0–6 T. Tc decreased along with the increasing magnetic fields, and the superconducting state was suppressed by the external magnetic fields, further proving the superconductivity. The upper critical magnetic fields were extrapolated based on the single-band Werthamer–Helfand–Hohenberg (WHH) (Werthamer et al., 1966) and Ginzburg–Landau (GL) (Ginzburg et al., 1950) models. The two models yielded the Hc2(0) as 158.1 and 114.9 T, respectively. These values are consistent with the reported experimental data (Drozdov et al., 2019). The coherence length can be calculated based on
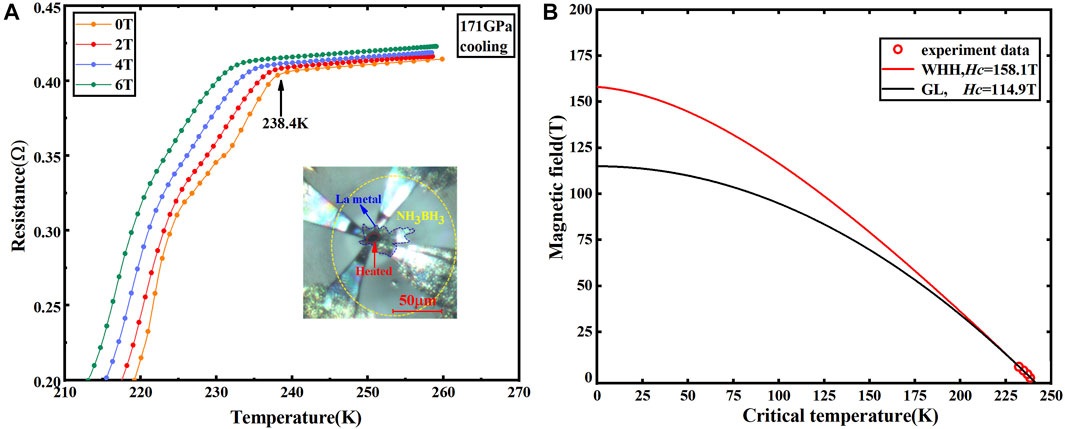
FIGURE 1. Our work measured the superconductivity of lanthanum superhydride at 171 GPa. (A) Temperature-dependent electrical resistance at 171 GPa during the cooling process and upon the external magnetic fields (0–6 T), measured by the four-probe technique. The inset shows that the sample chamber consists of La and NH3BH3 after laser heating. (B) Upper critical magnetic fields estimated by the single-band Werthamer–Helfand–Hohenberg (WHH) (Werthamer et al., 1966) and Ginzburg–Landau (GL) (Ginzburg et al., 1950) models.
Cerium Polyhydrides
From 2018, our group has carried out a series of work on cerium polyhydrides, particularly the discovery of two high-temperature superconducting cerium polyhydrides. Several new hydrides are studied by the treatment of different compression pathways (Li X. et al., 2019; Li et al., 2021). We have successfully synthesized a series of cerium polyhydrides by the direct reaction of Ce and H2 upon cold compression up to 159 GPa (Li X. et al., 2019). The Ce polyhydrides (CeH3, CeH3+x, CeH4, CeH9-δ, and CeH9) presented an increase of hydrogen content as pressure increased. The formed CeH9 had a unique clathrate-like structure consisting of H29 cages surrounding Ce atoms occupying the hexagonal P63/mmc symmetry (see Figure 2A). CeH9 was also with the nearest-neighbor H–H distances closest to predictions for solid atomic metallic hydrogen in all synthesized hydrides. The electron localization function of CeH9 indicated an ionic bonding between Ce and H atoms, and the band structure confirmed its metallic character. The density of electronic-state calculations indicated the significant contribution of H at the Fermi level. Our cold-compression experiment provides a facile route to potential superconductors in superhydrides. The discovery of CeH9 with atomic-like hydrogen sub-lattice suggests a low-pressure route for bulk dense atomic hydrogen stabilized by other element atoms. Independently, Salke et al. synthesized the clathrate hydride CeH9 at 80–100 GPa by laser heating the mixture of the Ce sample and H2 gas up to 2,000 K (Salke et al., 2019). They decompressed the sample and observed that the hexagonal CeH9 became unstable below 93 GPa.
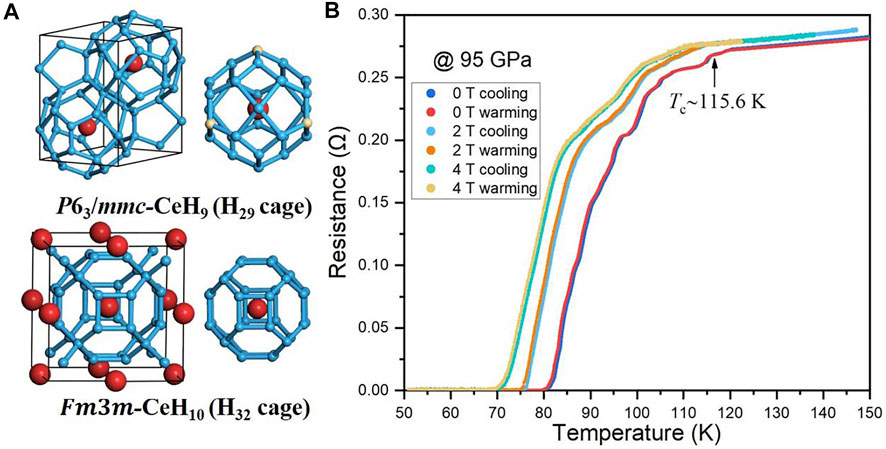
FIGURE 2. Crystal structures and superconducting transitions in the Ce–H system (Chen et al., 2021). (A) Crystal structures and hydrogen sub-lattice of P63/mmc-CeH9 and Fm
Recently, we have also further conducted deep experiments to explore the possible superconducting phases, and two phases were acquired: P63/mmc-CeH9 and Fm
The isotope effects were also experimentally observed by substituting the hydrogen in CeH9 with heavier deuterium and the decreased Tc was measured, which substantiated the conventional electron–phonon coupling mechanism of the BCS theory. According to the reported theoretical calculations (Li B. et al., 2019; Peng et al., 2017), the much heavier and larger cerium atoms provided sufficient electrons to hydrogen and stabilized the H29 and H32 fascinating cages. The predicted Ef and electron–phonon coupling coefficient λ were both at high level, which contributed to an optimistic superconducting Tc. Presently, our result enriches the binary system of clathrate superhydrides and provides more evidence for studying the role of rare earth metal elements. This work also verifies that Ce is a potential choice for designing ternary or even more complex high-temperature superconducting hydrides.
Praseodymium and Neodymium Polyhydrides
We also performed a similar experimental method on the praseodymium and neodymium polyhydrides (Zhou et al., 2020a; Zhou et al., 2020b). Under high-pressure and high-temperature conditions, the introduction of lanthanide heavy metal atoms stabilized the hydrogen structure, and new superhydrides with high hydrogen content were obtained. We have adopted NH3BH3 as the hydrogen source, which greatly improved the success rate of experimental studies on superhydrides. At 130 GPa, we synthesized two novel superhydrides F
Our group has obtained new hydrides PrH9 and NdH9 in experiment. The theoretical calculations found that PrH9 possessed weak superconductivity, while NdH9 displayed strong magnetism (Zhou et al., 2020a; Zhou et al., 2020b). With the increase of f electrons, the outer f electrons had a significant effect on the superconductivity of these new superhydrides. The present results on lanthanide superhydrides show that superconductivity declines along the La–Ce–Pr–Nd series, while magnetism becomes more and more pronounced (see Figure 3). Generally, based on the BCS theory, the electron–phonon coupling directly affected the superconductivity of the conventional superconductors. The existence of magnetism prevented the formations of cooper pairs and then was not favorable to the superconductivity. Therefore, compared with lanthanum hydride, the increased number of f electrons in neodymium displayed pronounced magnetic properties, at the same time suppressing the conventional superconductivity based on electron–phonon coupling. It was further clarified that magnetism is an important factor to affect the conventional superconductivity in superhydrides.
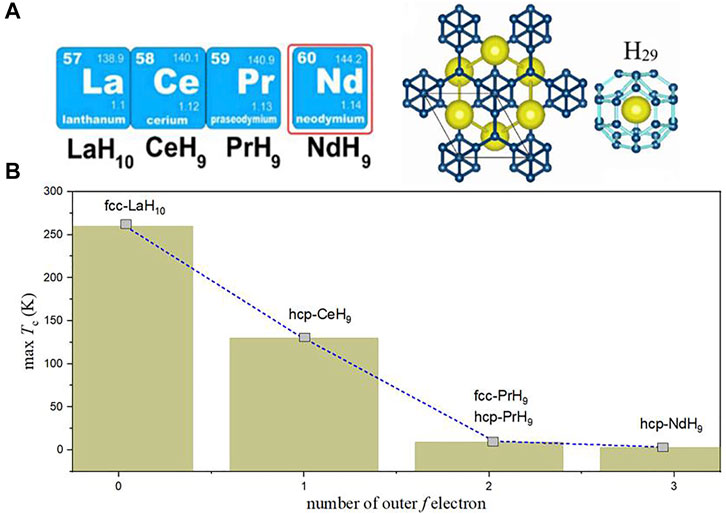
FIGURE 3. Crystal structure and superconducting transition temperature Tc of novel lanthanide metal hydrides synthesized experimentally. (A) Crystal structures and hydrogen cage configurations of CeH9, PrH9, and NdH9 found in this work; (B) Relationship of maximum Tc and outer f electrons of these new superhydrides.
Europium Polyhydrides
Europium as an active metal is a divalent element in general and reacts with hydrogen under pressure. Our previous work has obtained a series of Eu hydrides (F
Other Heavy Lanthanide Polyhydrides
The heavy lanthanide hydrides are predicted as promising superconductors under pressure. Several groups have carried on the theoretical predictions of heavy lanthanide hydrides with high hydrogen content (Peng et al., 2017; Sun et al., 2020; Hai et al., 2021; Song et al., 2021). Peng et al. reported a serious of stable rare earth (RE) hydrides REH6, REH9, and REH10 with unusual H clathrate structures at high pressure. The H-rich RE hydrides usually exhibited high-temperature superconductivity related to the H clathrate structures with strong EPC and large H-derived DOS near the Fermi surface, but the heavy lanthanide polyhydrides had much lower Tc due to heavier atoms which reduced the superconductivity (Peng et al., 2017). Subsequently, Sun et al. performed the EPC calculations for lanthanide hydrides with high hydrogen content and summarized the Tc for them. For the RE elements with half-filled 4f states (Eu, Gd, Dy, and Ho), they had the lowest DOS H-s values, resulting in a weak EPC and low Tc below 10 K. As the number of outer electrons increased, the Tcs for YbH10 and LuH8 reached as high as 102 K at 250 GPa and 86 K at 300 GPa, respectively (Sun et al., 2020). A series of stable superconducting Tb hydrides under pressure have been studied by Hai et al. TbHn (n = 1, 2, 3) were predicted to be stable under low pressure, while the terbium hydrides with high hydrogen content were stable at high pressure. The terbium polyhydrides exhibited high-temperature superconductivity especially for Fm
Perspective
It is important to find the high-temperature superconductors; at the same time, it is essential that the pressures required are reduced. Merit index S reflects the relationship between Tc and pressure (Pickard et al., 2020). We set S(MgB2) as 1. A superconductor with a higher value of S means it has a higher critical temperature at mild pressure. As Figure 4 shows, LaH10 and CeH10 have high S values 1.35 and 1.12, which indicates that these hydrides have a stronger superconductivity under similar pressure. Thus, lanthanide elements have played a key role in forming high-temperature superconductors.
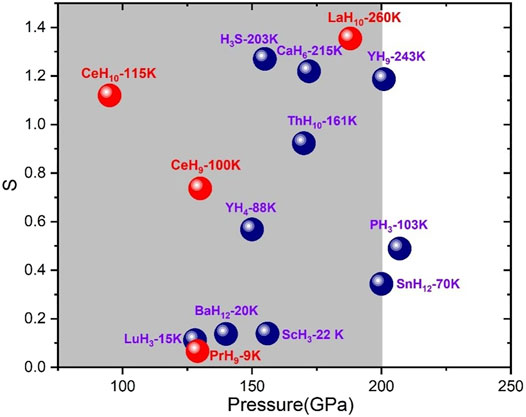
FIGURE 4. S for different superconducting hydrides reported from the experiment. Merit index S, can be explained as follows: S=Tc/(T2c,MgB2+P2)1/2 (Pickard et al., 2020; Shipley et al., 2021; Wang et al., 2021), where the temperature is expressed in Kelvin and the pressure is expressed in GPa. Tc,MgB2 is the superconductivity critical temperature of MgB2 at ambient pressures, which equals to 39 K.
In conclusion, we summarize the experimental results of lanthanide hydrides at high pressure. The lanthanide hydrides with high hydrogen content have clathrate structures with hydrogen cages surrounding metal atoms and show cubic or hexagonal symmetries in general. Although several high-temperature superconducting lanthanum hydrides have been discovered, the extremely high synthetic pressures limit their practical applications. Therefore, there is still a long way to obtain a high-temperature superconductor at ambient pressure.
Author Contributions
XH conceived this project. SC and WC performed the experiment. JG analyzed the experimental data. XH and JG wrote and revised the article. All authors discussed the results and offered the useful discussions.
Funding
This work was supported by the National Key R&D Program of China (Grant No. 2018YFA0305900), the National Natural Science Foundation of China (Grant Nos. 11974133, 52072188, 51632002, and 51720105007), and the Program for Changjiang Scholars and Innovative Research Team in University (Grant No. IRT_15R23).
Conflict of Interest
The authors declare that the research was conducted in the absence of any commercial or financial relationships that could be construed as a potential conflict of interest.
Publisher’s Note
All claims expressed in this article are solely those of the authors and do not necessarily represent those of their affiliated organizations, or those of the publisher, the editors, and the reviewers. Any product that may be evaluated in this article, or claim that may be made by its manufacturer, is not guaranteed or endorsed by the publisher.
References
Akahama, Y., and Kawamura, H. (2006). Pressure Calibration of Diamond Anvil Raman Gauge to 310GPa. J. Appl. Phys. 100, 043516. doi:10.1063/1.2335683
Ashcroft, N. W. (2004). Hydrogen Dominant Metallic Alloys: High Temperature Superconductors? Phys. Rev. Lett. 92, 187002. doi:10.1103/physrevlett.92.187002
Ashcroft, N. W. (1968). Metallic Hydrogen: a High-Temperature Superconductor? Phys. Rev. Lett. 21, 1748–1749. doi:10.1103/physrevlett.21.1748
Bardeen, J., Cooper, L. N., and Schrieffer, J. R. (1957). Microscopic Theory of Superconductivity. Phys. Rev. 106, 162–164. doi:10.1103/physrev.106.162
Chen, W., Semenok, D. V., Huang, X., Shu, H., Li, X., Duan, D., et al. (2021). High-Temperature Superconducting Phases in Cerium Superhydride with a Tc up to 115 K below a Pressure of 1 Megabar. Phys. Rev. Lett. 127, 117001. doi:10.1103/physrevlett.127.117001
Dias, R. P., and Silvera, I. F. (2017). Observation of the Wigner-Huntington Transition to Metallic Hydrogen. Science 355 (6326), 715–718. doi:10.1126/science.aal1579
Drozdov, A. P., Eremets, M. I., Troyan, I. A., Ksenofontov, V., and Shylin, S. I. (2015). Conventional Superconductivity at 203 Kelvin at High Pressures in the Sulfur Hydride System. Nature 525, 73–76. doi:10.1038/nature14964
Drozdov, A. P., Kong, P. P., Minkov, V. S., Besedin, S. P., Kuzovnikov, M. A., Mozaffari, S., et al. (2019). Superconductivity at 250 K in Lanthanum Hydride under High Pressures. Nature 2019, 569528e531. doi:10.1038/s41586-019-1201-8
Drozdov, A. P., Minkov, V. S., Besedin, S. P., Kong, P. P., Kuzovnikov, M. A., Knyazev, D. A., et al. (2018). Superconductivity at 215 K in Lanthanum Hydride at High Pressures. arXiv:1808.07039. doi:10.48550/arXiv.1808.07039
Einaga, M., Sakata, M., Ishikawa, T., Shimizu, K., Eremets, M. I., Drozdov, A. P., et al. (2016). Crystal Structure of the Superconducting Phase of Sulfur Hydride. Nat. Phys. 12, 835–838. doi:10.1038/nphys3760
Eremets, M. I., Drozdov, A. P., Kong, P. P., and Wang, H. (2019). Semimetallic Molecular Hydrogen at Pressure above 350 GPa. Nat. Phys. 15, 1246–1249. doi:10.1038/s41567-019-0646-x
Gao, L., Xue, Y. Y., Chen, F., Xiong, Q., Meng, R. L., Ramirez, D., et al. (1994). Superconductivity up to 164 K inHgBa2Cam−1CumO2m+2+δ(m=1, 2, and 3) under Quasihydrostatic Pressures. Phys. Rev. B 50 4260, 4263. doi:10.1103/physrevb.50.4260
Ginzburg, V. L., and Landau, L. D. (1950). On the Theory of Superconductivity. Zh. Eksp. Teor. Fiz. 20, 1064. doi:10.1007/978-3-540-68008-6_4
Hai, Y.-L., Lu, N., Tian, H.-L., Jiang, M.-J., Yang, W., Li, W.-J., et al. (2021). Cage Structure and Near Room-Temperature Superconductivity in TbHn (N=1-12). J. Phys. Chem. C 125, 3640–3649. doi:10.1021/acs.jpcc.1c00645
Hong, F., Yang, L., Shan, P., Yang, P., Liu, Z., Sun, J., et al. (2020). Superconductivity of Lanthanum Superhydride Investigated Using the Standard Four-Probe Configuration under High Pressures. Chin. PHY Lett. 37 (10), 107401. doi:10.1088/0256-307x/37/10/107401
Huang, X., Wang, X., Duan, D., Sundqvist, B., Li, X., Huang, Y., et al. (2019). High-temperature Superconductivity in Sulfur Hydride Evidenced by Alternating-Current Magnetic Susceptibility. Natl. Sci. Rev. 6, 713–718. doi:10.1093/nsr/nwz061
Iqbal, Z., Datta, T., Kirven, D., Lungu, A., Barry, J. C., Owens, F. J., et al. (1994). Superconductivity above 130 K in the Hg-Pb-Ba-Ca-Cu-O System. Phys. Rev. B 49, 12322–12325. doi:10.1103/physrevb.49.12322
Kondrat’ev, Y. V., Butlak, A. V., Kazakov, I. V., and Timoshkin, A. Y. (2015). Sublimation and Thermal Decomposition of Ammonia Borane: Competitive Processes Controlled by Pressure. Thermochim. Acta. 622, 642. doi:10.1016/j.tca.2015.08.021
Li, B., Miao, Z., Ti, L., Liu, S., Chen, J., Shi, Z., et al. (2019). Predicted High-Temperature Superconductivity in Cerium Hydrides at High Pressures. J. Appl. Phys. 126, 235901. doi:10.1063/1.5130583
Li, X., Huang, X., Chen, W., Zhou, D., Xie, H., Zhuang, Q., et al. (2021). New Cage-like Cerium Trihydride Stabilized at Ambient Conditions. CCS Chem. 4, 825–831. doi:10.31635/ccschem.021.202100799
Li, X., Huang, X., Duan, D., Pickard, C. J., Zhou, D., Xie, H., et al. (2019). Polyhydride CeH9 with an Atomic-like Hydrogen Clathrate Structure. Nat. Commun. 10, 3461. doi:10.1038/s41467-019-11330-6
Liu, H., Naumov, I. I., Hoffmann, R., Ashcroft, N. W., and Hemley, R. J. (2017). Potential High-Tc Superconducting Lanthanum and Yttrium Hydrides at High Pressure. Proc. Natl. Acad. Sci. U. S. A. 114, 6990–6995. doi:10.1073/pnas.1704505114
Liu, L., Wang, C., Yi, S., Kim, K. W., Kim, J., and Cho, J.-H. (2019). Microscopic Mechanism of Room-Temperature Superconductivity in Compressed LaH10. Phys. Rev. B 99, 140501. doi:10.1103/physrevb.99.140501
Loubeyre, P., Occelli, F., and Dumas, P. (2020). Synchrotron Infrared Spectroscopic Evidence of the Probable Transition to Metal Hydrogen. Nature 577, 631–635. doi:10.1038/s41586-019-1927-3
Ma, L., Zhou, M., Wang, Y., Kawaguchi, S., Ohishi, Y., Peng, F., et al. (2021). Experimental Clathrate Superhydrides EuH6 and EuH9 at Extreme Pressure Conditions. Phys. Rev. Res. 3, 043107. doi:10.1103/physrevresearch.3.043107
Nagamatsu, J., Nakagawa, N., Muranaka, T., Zenitani, Y., and Akimitsu, J. (2001). Superconductivity at 39 K in Magnesium Diboride. Nature 410, 63–64. doi:10.1038/35065039
Peña-Alvarez, M., Binns, J., Hermann, A., Kelsall, L. C., Dalladay-Simpson, P., Gregoryanz, E., et al. (2019). Praseodymium Polyhydrides Synthesized at High Temperatures and Pressures. Phys. Rev. B 100, 184109. doi:10.1103/physrevb.100.184109
Peng, F., Sun, Y., Pickard, C. J., Needs, R. J., Wu, Q., and Ma, Y. (2017). Hydrogen Clathrate Structures in Rare Earth Hydrides at High Pressures: Possible Route to Room-Temperature Superconductivity. Phys. Rev. Lett. 119, 107001. doi:10.1103/physrevlett.119.107001
Pickard, C. J., Errea, I., and Eremets, M. I. (2020). Superconducting Hydrides under Pressure. Annu. Rev. Condens. Matter Phys. 11, 57–76. doi:10.1146/annurev-conmatphys-031218-013413
Salke, N. P., Davari Esfahani, M. M., Zhang, Y., Kruglov, I. A., Zhou, J., Wang, Y., et al. (2019). Synthesis of Clathrate Cerium Superhydride CeH9 at 80-100 GPa with Atomic Hydrogen Sublattice. Nat. Commun. 10, 4453. doi:10.1038/s41467-019-12326-y
Semenok, D. V., Zhou, D., Kvashnin, A. G., Huang, X., Galassox, M., Kruglov, I. A., et al. (2021). Novel Strongly Correlated Europium Superhydrides. J. Phys. Chem. Lett. 12, 32–40. doi:10.1021/acs.jpclett.0c03331
Shao, M., Chen, S., Chen, W., Zhang, K., Huang, X., and Cui, T. (2021a). Superconducting ScH3 and LuH3 at Megabar Pressures. Inorg. Chem. 60, 15330–15335. doi:10.1021/acs.inorgchem.1c01960
Shao, M., Chen, W., Zhang, K., Huang, X., and Cui, T. (2021b). High-pressure Synthesis of Superconducting Clathratelike YH4. Phys. Rev. B 104, 174509. doi:10.1103/physrevb.104.174509
Shipley, A. M., Hutcheon, M. J., and Needs, R. J. (2021). High-throughput Discovery of High-Temperature Conventional Superconductors. arXiv:2105.02296. doi:10.1103/PhysRevB.104.054501
Shukor, R. A. (2021). Coherence Length versus Transition Temperature of Hydride-Based and Room Temperature Superconductors. Results Phys. 25, 104219. doi:10.1016/j.rinp.2021.104219
Somayazulu, M., Ahart, M., Mishra, A. K., Geballe, Z. M., Baldini, M., Meng, Y., et al. (2019). Evidence for Superconductivity above 260 K in Lanthanum Superhydride at Megabar Pressures. Phys. Rev. Lett. 122, 027001. doi:10.1103/PhysRevLett.122.027001
Song, H., Zhang, Z., Cui, T., Pickard, C. J., Kresin, V. Z., and Duan, D. (2021). High Tc Superconductivity in Heavy Rare Earth Hydrides. Chin. Phys. Lett. 38 (10), 107401. doi:10.1088/0256-307x/38/10/107401
Sun, D., Minkov, V. S., Mozaffari, S., Sun, Y., Ma, Y., Chariton, S., et al. (2021). High-temperature Superconductivity on the Verge of a Structural Instability in Lanthanum Superhydride. Nat. Commun. 12:6863. doi:10.1038/s41467-021-26706-w
Sun, W., Kuang, X., Keen, H. D. J., Lu, C., and Hermann, A. (2020). Second Group of High-Pressure High-Temperature Lanthanide Polyhydride Superconductors. Phys. Rev. B 102, 144524. doi:10.1103/physrevb.102.144524
Troyan, I., Gavriliuk, A., Rüffer, R., Chumakov, A., Mironovich, A., Lyubutin, I., et al. (2016). Observation of Superconductivity in Hydrogen Sulfide from Nuclear Resonant Scattering. SCIENCE 351 (6279), 1303–1306. doi:10.1126/science.aac8176
Wang, C., Yi, S., and Cho, J.-H. (2020). Multiband Nature of Room-Temperature Superconductivity in LaH10 at High Pressure. Phys. Rev. B 101, 104506. doi:10.1103/physrevb.101.104506
Wang, D., Ding, Y., and Mao, H.-K. (2021). Future Study of Dense Superconducting Hydrides at High Pressure. Materials 14 (24), 7563. doi:10.3390/ma14247563
Werthamer, N. R., Helfand, E., and Hohenberg, P. C. (1966). Temperature and Purity Dependence of the Superconducting Critical Field,Hc2. III. Electron Spin and Spin-Orbit Effects. Phys. Rev. 147, 295–302. doi:10.1103/physrev.147.295
Wigner, E., and Huntington, H. B. (1935). On the Possibility of a Metallic Modification of Hydrogen. J. Chem. Phys. 3, 764–770. doi:10.1063/1.1749590
Zhou, D., Semenok, D. V., Duan, D., Xie, H., Chen, W., Huang, X., et al. (2020a). Superconducting Praseodymium Superhydrides. Sci. Adv. 6, eaax6849. doi:10.1126/sciadv.aax6849
Keywords: high pressure, lanthanide hydrides, superconductivity, crystal structure, magnetic properties
Citation: Guo J, Chen S, Chen W, Huang X and Cui T (2022) Advances in the Synthesis and Superconductivity of Lanthanide Polyhydrides Under High Pressure. Front. Electron. Mater. 2:906213. doi: 10.3389/femat.2022.906213
Received: 28 March 2022; Accepted: 19 April 2022;
Published: 25 May 2022.
Edited by:
Sheng Ran, Washington University in St. Louis, United StatesReviewed by:
Dong Qian, Shanghai Jiao Tong University, ChinaCopyright © 2022 Guo, Chen, Chen, Huang and Cui. This is an open-access article distributed under the terms of the Creative Commons Attribution License (CC BY). The use, distribution or reproduction in other forums is permitted, provided the original author(s) and the copyright owner(s) are credited and that the original publication in this journal is cited, in accordance with accepted academic practice. No use, distribution or reproduction is permitted which does not comply with these terms.
*Correspondence: Xiaoli Huang, aHVhbmd4aWFvbGlAamx1LmVkdS5jbg==