- 1School of Resource and Environmental Management, Simon Fraser University, Burnaby, BC, Canada
- 2Parks Canada, Office of the Chief Ecosystem Scientist, Protected Areas Establishment and Conservation Directorate, Vancouver, BC, Canada
- 3School of Environmental Science, Simon Fraser University, Burnaby, BC, Canada
In the coming century, climate variability is projected to increase in Northeast Pacific coastal areas, increasing the need for land managers to understand how ecosystems are expected to change in response to new or enhanced disturbances. Previous research indicates that the Pacific coast of Canada, southern British Columbia (BC) experienced warmer and drier climate conditions than present, with higher than modern fire activity during the early Holocene xerothermic interval (9.5 – 7.0 kcal BP). In this study, we reconstructed past climate-fire-vegetation changes from a 13,000-year sediment record from Lost Lake in Vancouver’s Lower Seymour Conservation Reserve, BC. Contrary to other sites, the coastal western hemlock forest at this site remained cool and moist with low fire activity throughout the xerothermic period. Instead, peak fire frequencies were observed during the cool and moist Neoglacial period (4.5 kcal BP – present), when human activity became prevalent. These results have implications for the managed watershed’s resilience to fire and response to future warming conditions.
1 Introduction
Understanding past changes in climate and ecology is a useful component of managing the environmental shifts associated climate, land management, and anthropogenic climate change. By the year 2050, temperatures in British Columbia are estimated to increase by 1.3 – 2.4°C under the Shared Socioeconomic Pathway 5-8.5 (SSP 5-8.5) future climate scenario from the sixth phase of the Coupled Model Intercomparison Project (CMIP6) (Pacific Climate Impacts Consortium, 2024), and regional wildfire activity and severity are expected to intensify (Mote and Salathé, 2010). While impacts of wildfires on contemporary forest and water resources along the northwest coast of North America have been well documented (Green et al., 1999; Carignan et al., 2000; Zwolinksi, 2000; Gavin et al., 2007; Sankey et al., 2017), the effects of changing climate-fire-vegetation interactions in South Coastal BC in response to increasing temperatures and wildfire severity are less clear.
Paleoecology is a well-regarded method of understanding past interactions between climate and vegetation. Quantifying past disturbances and assessing how ecosystems respond to change is a means of identifying and evaluating disturbance regimes, helps with our understanding of the timing and significance of ecosystem shifts, and supports planning for future land management and restoration projects (Landres et al., 1999; Swetnam et al., 1999; Davies and Bunting, 2010; Whitlock et al., 2015; Kidwell, 2015; Pellatt et al., 2015; Murphy et al., 2019). The Holocene epoch (11.7 kcal BP – present; Gervais (1867-1869)) is of interest to the understanding of long-term changes in climate and wildfire activity throughout western North America because the scales of temperature and precipitation variability may have been larger than those seen in the past 200 years (Gifford et al., 2022; NASA, 2024). Two commonly used proxies that have been applied to better understand changes in vegetation and fire behavior are palynomorphs (Mathewes, 1973; Mathewes and King, 1989; Hebda, 1983; Cwynar, 1987; Hebda, 1995; Heusser, 1983; Allen, 1995; Barnosky, 1981; Pellatt et al., 2000; Gavin et al., 2001; Pellatt et al., 2001a; Brown and Hebda, 2002a, b; Gavin and Brubaker, 1999; Brown et al., 2019) and charcoal particles, respectively (Cwynar, 1987; McLachlan and Brubaker, 1995; Gavin et al., 2001; Gavin et al., 2003; Brown and Hebda, 2002a, b; Sugimura et al., 2008; Hallett et al., 2003; Derr, 2014; Murphy et al., 2019; Pellatt et al., 2015; Lucas and Lacourse, 2013; Brown et al., 2019; Prichard et al., 2009 (Supplementary Table S1; Figure 1).
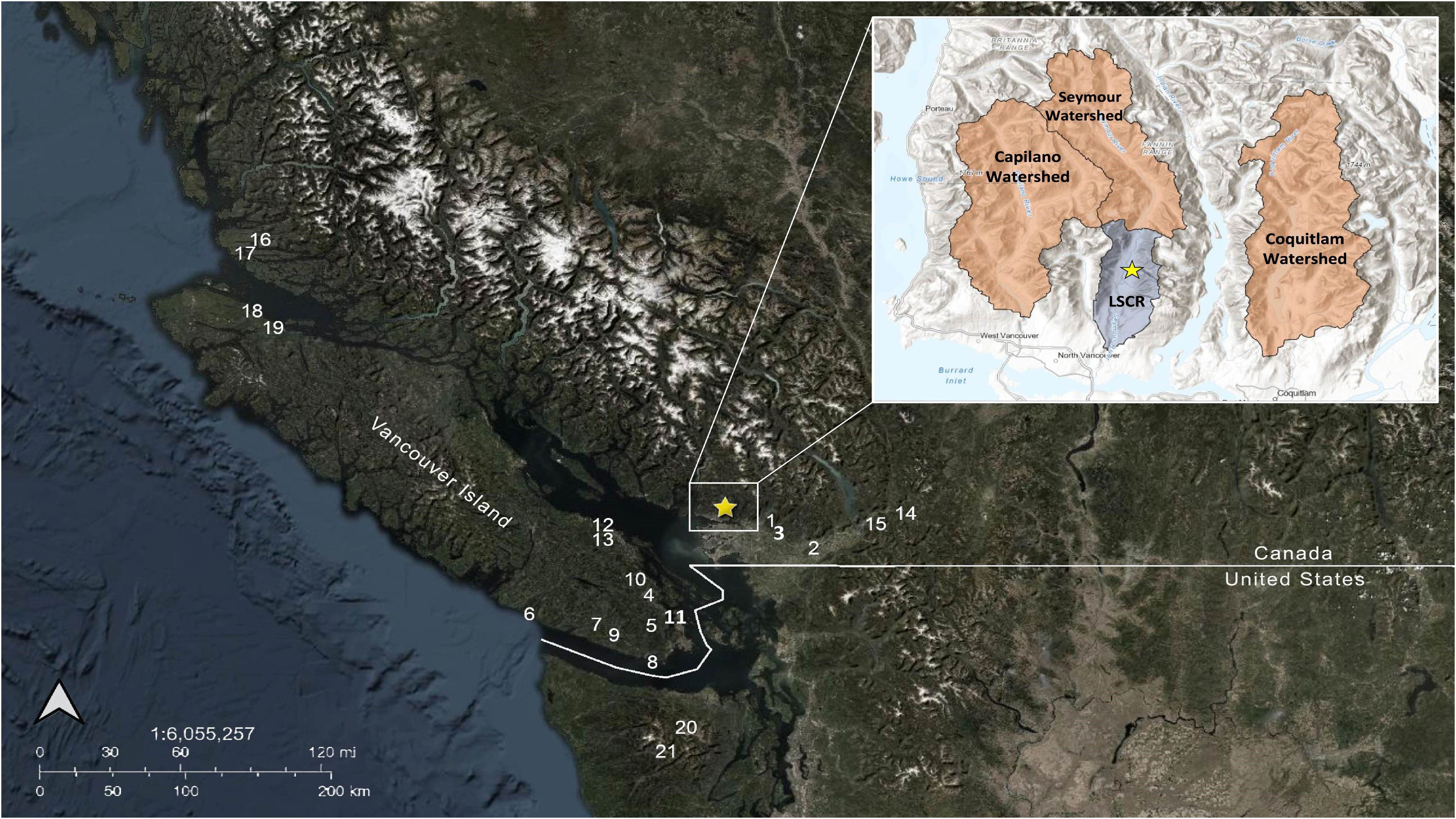
Figure 1. Study region of Western North America showing Lost Lake (yellow star) and previous paleoecological studies conducted in the Coastal Western Hemlock (CWH) and Coastal Douglas Fir (CDF) biogeoclimatic zones using pollen and/or charcoal as proxies (Supplementary Material. Appendix A). Study sites include: (Star) Lost Lake (this study); (1) Marion Lake (Wainman and Mathewes, 1987); (2) Chadsey Lake (Murphy et al., 2019); (3) Mike Lake (Pellatt et al., 2001b); (4) Somenos Lake (Murphy et al., 2019); (5) Begbie Lake (Brown et al., 2019); (6) Whyac Lake (Brown and Hebda, 2002b); (7) Pixie Lake (Brown and Hebda, 2002b); (8) East Sooke Fen (Brown and Hebda, 2002b); (9) Walker Lake (Brown & Hebda, 2003); (10) Porphyry Lake (Brown & Hebda, 2003); (11) ODP hole 1034B (Pellatt et al., 2001a); (12) Enos Lake (Brown and Hebda, 2002a); (13) Boomerang Lake (Brown and Hebda, 2002a); (14) Frozen Lake (Hallett et al., 2003); (15) Mt. Barr Cirque Lake (Hallett et al., 2003); (16) Tiny Lake (Galloway et al., 2007); (17) Two Frog Lake (Galloway et al., 2009); (18) Bear Cove Bog (Hebda, 1983); (19) Misty Lake (Lacourse, 2005); (20) Moose Lake (Gavin et al., 2001); and (21) Martins Lake (Gavin et al., 2001). Base layer and inset map sources are Esri, Maxar, Earthstar Geographics, CNES/Airbus DS, USDA, USGS, AeroGRID, IGN, NOAA NGDC, and the GIS User Community.
Regional Holocene records of the past ~12,000 years indicate that the forested ecosystems in and around the Fraser Valley of British Columbia (BC) experienced large disturbances over relatively short (centennial to several thousand year) timescales (Walker and Pellatt, 2003). Previous paleoclimatic studies of British Columbia have divided the Holocene into major periods of climatic change. The Younger Dryas, occurring approximately between 12.9 – 11.6 kcal BP, marked a period of cooling in much of the Northern Hemisphere around the North Atlantic Ocean (Cheng et al., 2020), with moderate cooling effects observed on Canada’s Pacific coast as well (Mathewes, 1993). The early Holocene xerothermic period (ca. 9.5 – 7.0 kcal BP) was drier and up to 2°C warmer than present in southwestern BC (Mathewes and Heusser, 1981). A gradual transition to cooler, moist conditions began on the southern BC coast between 7.5 and 6.0 kcal BP (Mathewes, 1973; Mathewes and Heusser, 1981; Pellatt et al., 2001a). At ~5.0 kcal BP, summer solar insolation was continuing to decline (Figure 2), and regional records indicate that the intensity of the Aleutian low-pressure system increased (Barron and Anderson, 2011; Heusser, 1983), causing a decline in summer temperatures, wetter conditions, and mid-Holocene cooling in southwestern BC (Walker and Pellatt, 2003). The Neoglacial period (ca. 4.5 kcal BP to present) was cool and wet, instigated by the combined effects of multiple glacial advances across much of British Columbia and the continued intensification of the Aleutian low pressure system (Heusser, 1983; Hebda, 1995; Pellatt and Mathewes, 1994; Clague et al., 1997).
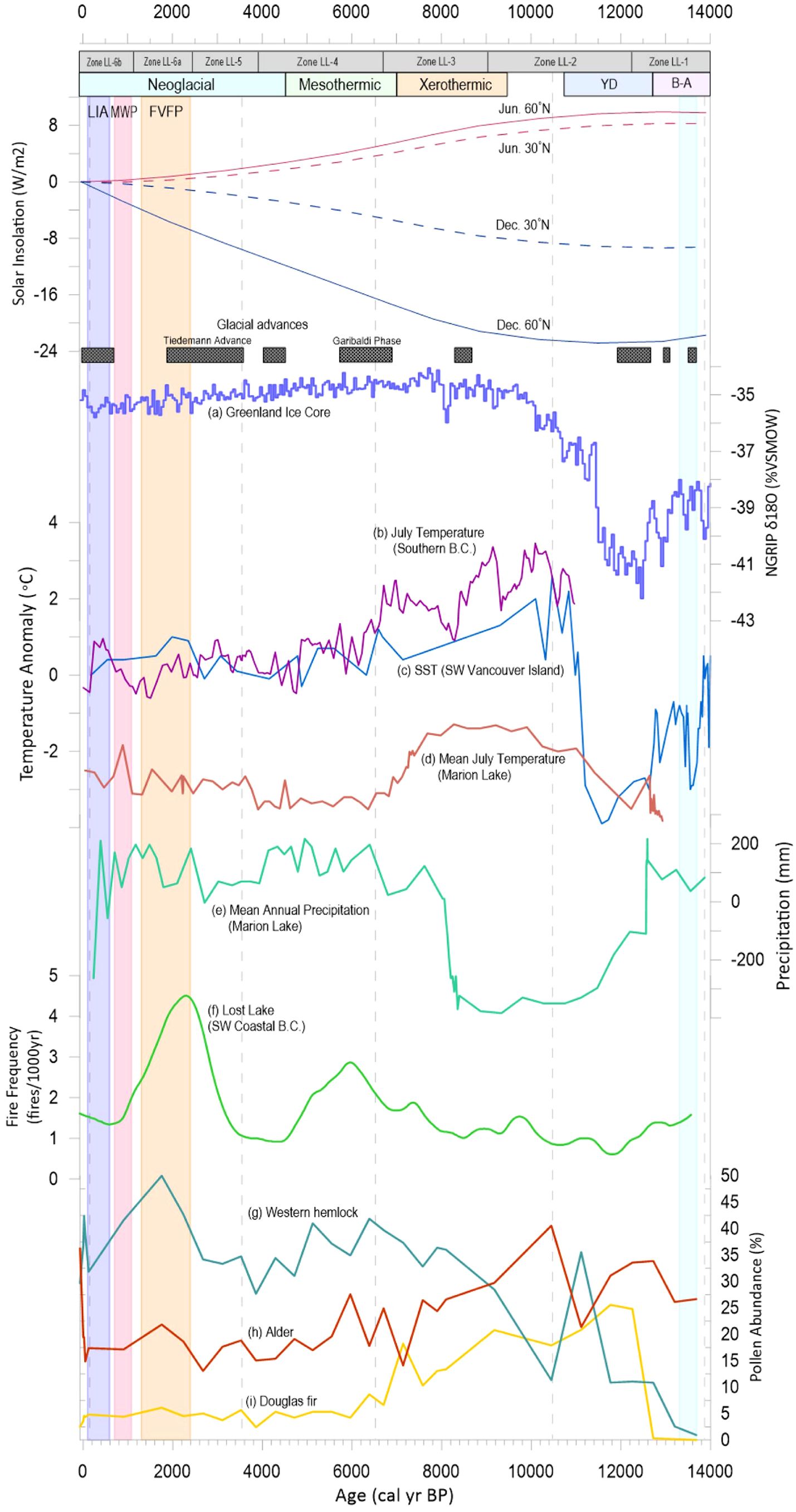
Figure 2. Synthesis of regional climatic data in the Northern Hemisphere from 14,000 kcal BP to present. Grey bars represent timing of Lost Lake Pollen Zones LL-1 to LL-6b. Colored bars represent timing of major climatic events: Bølling-Allerød (B-A, purple, Ivanovic et al., 2016), Younger Dryas (YD, blue, McManus et al., 2004; Renssen et al., 2015), Xerothermic (orange, Hebda, 1995), Mesothermic (green, Hebda, 1995), Neoglacial (light blue, Walker and Pellatt, 2003). Vertical bars represent timing of the Fraser Valley Fire Period (FVFP, light orange, Hallett et al., 2003), Medieval Warm Period (MWP, pink, Mann et al., 2009), and Little Ice Age (LIA, dark blue, Grove, 2001). Dashed grey vertical lines represent drive breaks in the Lost Lake core. Solar insolation records are from Berger and Loutre (1991) and NOAA/NGDC Paleoclimatology Program. Red solid line represents June insolation at 60˚N; red dashed line represents June insolation at 30˚N; blue solid line represents December insolation at 60˚N; blue dashed line represents December insolation at 30˚N. Historic solar insolation values were subtracted from modern values. Glacial advance periods are from Ryder and Thomson (1986), Friele & Clague (2002), Menounos et al. (2009), and Gavin et al. (2011). NGRIP δ18O record (A) is from North Greenland Ice Core Project Members (2007); “VSMOW” acronym is Vienna Standard Mean Ocean Water. Temperature anomaly records are from (B) a composite record of July temperature anomalies from four chironomid records in southern BC [Frozen Lake, Rosenberg et al. (2004); North Crater Lake and Lake of the Woods, Palmer et al. (2003); Windy Lake, Chase et al. (2008)], (C) alkenone-derived SSTs from core JT96-09PC off the southwestern coast of Vancouver Island (Kienast and McKay, 2001) and (D) a pollen-based transfer function from the Marion Lake record (Mathewes, 1973; Mathewes and Heusser, 1981). Modern mean annual SST temperature (acquired from DFO Amphitrite Point Lightstation SST Data Archives) was subtracted from SST values, and modern mean July temperature (acquired from Government of Canada (2022) Haney UBC Research Forest Station) was subtracted from the Marion Lake record. Precipitation record (E) was derived from pollen-based transfer function from the Marion Lake record (Mathewes, 1973; Mathewes and Heusser, 1981). Modern mean annual precipitation (acquired from Government of Canada’s Haney UBC Research Forest Station) was subtracted from all values. Fire frequency record (F) and CONISS-derived pollen abundance records (G), Coastal western hemlock; (H), Alder; (I) Douglas fir were taken from Lost Lake (this study).
From ca. 2.4 kcal BP to present, multiple shorter, discrete climate intervals have been observed in coastal BC. An increase in fire activity and drought frequency between 2.4 and 1.3 kcal BP has been referred to as the Fraser Valley Fire Period (FVFP) (Hallett et al., 2003). From 1.3 kcal BP onwards, the climate in the Fraser Valley remained relatively similar to modern conditions, with short, location-dependent intervals of climatic variability, including the Medieval Warm Period (MWP; ~1000 – 600 cal yr BP) and the Little Ice Age (LIA; 600-150 cal yr BP) (Walker and Pellatt, 2003; Hallett et al., 2003).
In addition to climate driven change in ecosystem structure throughout the Holocene, Indigenous people have managed their traditional lands to improve foraging capacity, and for gardening, hunting, and habitation for millennia (Boyd, 1999; Turner and Peacock, 2005; Turner, 2017). Indigenous knowledge (IK), archaeology and paleoecological investigations have documented long-term use of prescribed fire to manage food resources in southwest British Columbia (McCune et al., 2013; Pellatt and Gedalof, 2014), woolly dog domestication (McCormick et al., 2021), shellfish cultivation (Williams, 2006; Lepofsky et al., 2015), and numerous other forms of marine and terrestrial harvesting (Turner, 2017). The establishment of coastal BC by Indigenous people is well documented since deglaciation (Josenhans et al., 1997), utilizing wood products such as western redcedar (Thuja plicata) (Hebda & Mathewes, 1984) and establishing large communities throughout what now is referred as southwest British Columbia.
In this study, we use palynological and charcoal analyses to understand climate-fire-vegetation changes within the Lower Seymour Conservation Reserve (LSCR) in North Vancouver, BC, from the very end of the Pleistocene period to the present day (approximately 13,900 years). We place this record in the context of regional climatic changes throughout the Holocene to interpret long-term ecosystem changes in the LSCR. Investigating the mechanisms behind ecosystem changes during the Holocene provides insight into how local watersheds may respond to climate change in the coming decades and can be used to inform adaptation strategies within the Metro Vancouver water supply area (WSA).
2 Materials and methods
2.1 Study area
Lost Lake is a small (3.8 ha) lake located within the Metro Vancouver-governed Lower Seymour Conservation Reserve (LSCR), situated in the lowlands of the southernmost range of the Coast Mountains in North Vancouver, British Columbia (Figure 1). Late fall and winter (October to March) in this region are characterized by northeast Pacific storms which deposit a cumulative average of 112 cm of precipitation between October and March (Government of Canada, 2024). Less commonly, Arctic outflow winds blow southward through river valleys and bring bursts of cold and wind to the Puget-Georgia basin (Walker and Pellatt, 2003). Summers are generally less rainy with warm, dry conditions more recently exacerbated by uncharacteristically long heat waves (Eyquem and Feltmate, 2022). Lost Lake sits at an elevation of 235 meters above sea level in the Coastal Western Hemlock (CWH) biogeoclimatic zone, which occurs at low to mid-elevations along much of BC’s coast and is characterized by a wet, mild climate (Meidinger and Pojar, 1991). Lost Lake is located in the very wet maritime submontane (CWHvm1) subzone of the CWH zone (Meidinger and Pojar, 1991; Green and Klinka, 1994), and is surrounded by mixed stands of Tsuga heterophylla, Thuja plicata, and Pseudotsuga menziesii. To the south of Lost Lake the Coastal Douglas Fir (CDF) biogeoclimatic zone occupies a small section of the southern mainland coast, and is characterized by a drier, warmer climate than the CWH zone (Meidinger and Pojar, 1991).
Although no archaeological evidence of pre-Colonial habitation has been directly observed at Lost Lake, the Lower Mainland and surrounding areas lie on the unceded territories of several Coast Salish First Nations, including those of the Musqueam, Squamish, and Tsleil-Waututh. Archaeological evidence dating as far back as 9.5 kcal BP has been found in the Fraser River Delta, which lies directly to the south of Lost Lake (Matson and Coupland, 1995; Lepofsky et al., 2009). To the northwest of Lost Lake, archaeological evidence has dated settlements along the coast between Vancouver Island and Haida Gwaii to 14 – 13 kcal BP (McLaren et al., 2018; Gauvreau et al., 2023), while to the east, the Stó:lō-Coast Salish were active along the Fraser River corridor for millennia prior to settler arrival (Lepofsky et al., 2000, 2009). The well-documented use of the Fraser River as a transportation corridor by Stó:lō-Coast Salish (Blake, 2004; Schaepe, 2009) suggests that travel to Lost Lake was very possible despite a current lack of archaeological evidence or oral history. Land-use practices in the watersheds of North Vancouver were frequently altered throughout the 1800s and 1900s as settler activity intensified disturbance via logging, mining, slash burning, and sediment redeposition.
2.2 Field methods
In September 2020, we retrieved a 3.64 m piston core from the deepest part of Lost Lake (12 m) in six separate drives using a modified Livingston piston corer (Wright et al., 1984). We also collected a 0.41m surface core from the same location in November 2020 using a Glew gravity corer (Glew, 1988). The surface core was extruded in the field at 1 cm intervals. The piston core was photographed on-site, wrapped in PVC tubing for transport, and split longitudinally in the laboratory.
2.3 Chronology
To estimate the sediment mass accumulation rate and age of the core, we created an age-depth model using tie points based on 210Pb and AMS-14C dates and regional stratigraphic markers (i.e., the Mazama tephra). Eleven samples were selected from the surface core and sent to Flett Research Ltd. in Winnipeg, Manitoba, for 210Pb analysis (Supplementary Table S1). Additional 137Cs analyses were conducted on four samples, and 226Ra analyses were conducted on three samples (Supplementary Table S1). The age model for the Lost Lake surface core was created based on a constant rate of supply (CRS) model (Appleby and Oldfield, 1977) applied to eleven 210Pb age determinations.
Five macrofossil samples from the Livingston core (Supplementary Table S2) were sent to Beta Analytic in Marathon, Florida, for AMS-14C dating. In preparation for dating, the macrofossil samples were washed with distilled water and dried in an oven overnight at 30°C. As per Beta Analytic’s standard pretreatment protocol for plant material, the samples underwent a hot acid (HCl) wash to remove carbonates, then an alkali wash (NaOH) to eliminate secondary organic acids, followed by a final acid rinse (Beta Analytic, 2022). The AMS-14C ages were converted to calendar years before present (cal yr BP) via the program CALIB 8.2.0 (Stuiver and Reimer, 1993) and the IntCal20 dataset (Reimer et al., 2020). Finally, we established a tie-point associated with a 2-cm layer of ash found at a depth of 207-209 cm in the piston core, which we associate with the eruption of Mount Mazama in southwestern Oregon ca. 7.6 kcal BP (Zdanowicz et al., 1999).
The age model for the composite core (surface core + piston core) was constructed using the Bacon modelling program in R (Blaauw and Christen, 2011), which uses Bayesian analysis to reconstruct historical accumulation rates by combining contemporary dating with prior information. The calibrated calendar ages of four AMS-14C ages plus the date of the Mazama tephra were used to construct a representative age-depth model for the composite core (Figure 3). The 210Pb CRS model which was applied to the initial 40.5 cm of the core was joined to the top of the Bacon age model to create a composite age model.
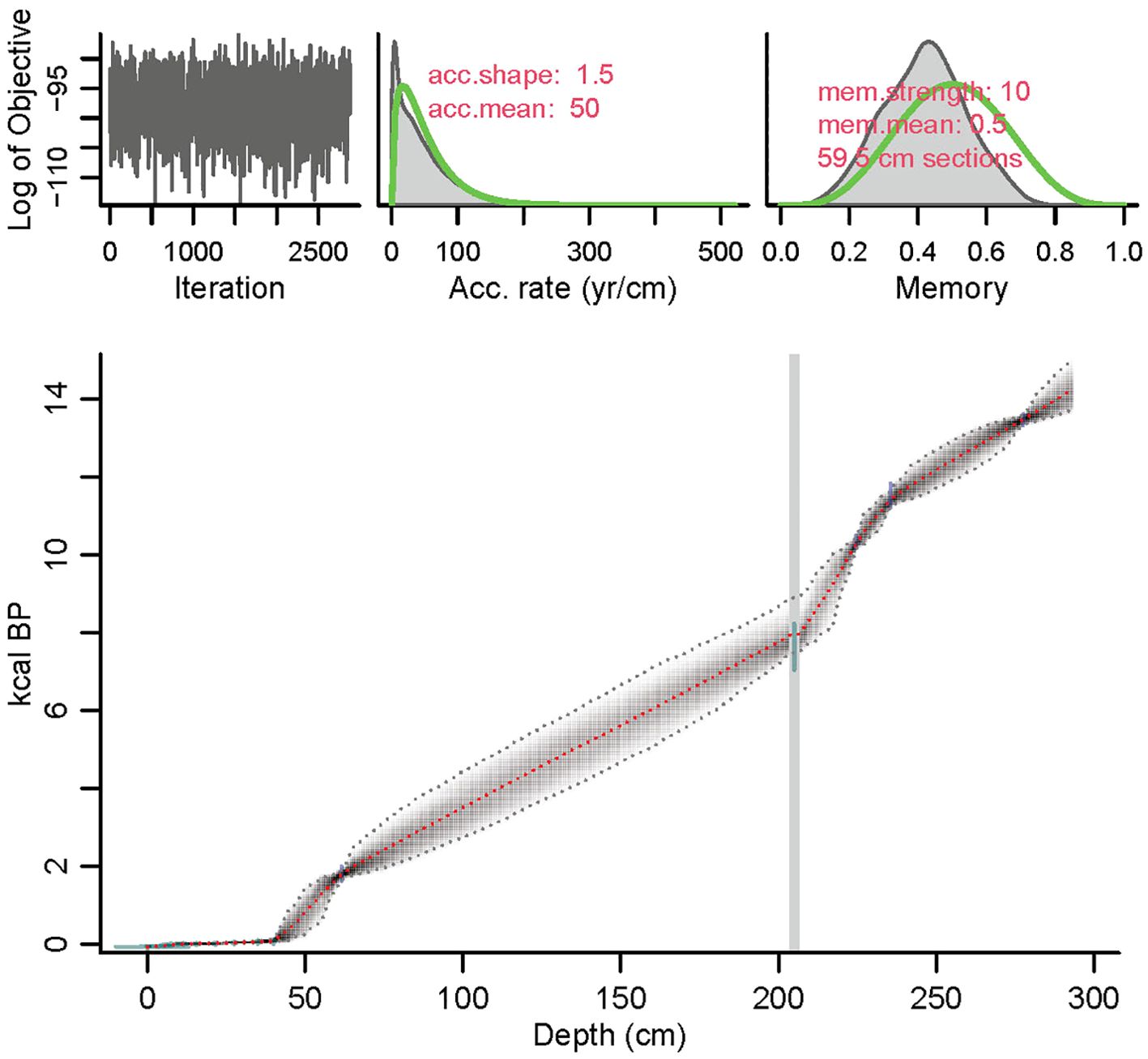
Figure 3. Bacon age model of the Lost Lake Core. Grey dotted lines represent error envelope at 95% confidence interval. Grey fill represents all likely age-depth models calculated by Bacon. Red line represents mean age (the “best” model selected by Bacon). Blue points represent AMS-14C samples (2σ probability distributions of calibrated 14C ages). Green points represent locked in 210Pb dates and Mazama ash date. Gray vertical line represents slump point at Mazama tephra to indicate instantaneous deposition.
The mean sample resolution for the composite core was 48.8 yr/cm, with a much higher resolution (5.3 yr/cm) in the first 41 cm of the core. The mean sedimentation rate for the composite core was 0.086 cm/yr, with a maximum of 1.2 cm/yr between 12 and 15 cm of the composite core.
2.4 Pollen analysis
The Lost Lake composite core was sub-sectioned for pollen analysis at 10 cm intervals along the entire length of the core, with a 1-cm3 sample being removed at each sample point. Pollen preparation methods were adapted from standard recovery techniques (Faegri and Iversen, 1989; Moore et al., 1991) at the Parks Canada Vancouver Ecology Laboratory. Lycopodium (clubmoss) marker tablets (10,679 ± 191 spores/tablet; Batch No. 938934) were added to each sample to calculate pollen concentration and accumulation rates. A minimum of 500 grains per sample were counted at 400x to 1000x magnification. Taxa were identified using reference slides from the Mathewes Pollen Laboratory at Simon Fraser University, published morphological keys (Faegri and Iversen, 1989; Moore et al., 1991), and The Global Pollen Project webpage (globalpollenproject.org) (Figure 4). Raw pollen data were archived in the open-access PANGAEA data repository. A pollen and spore percentage diagram based on the terrestrial sum was created using TILIA version 2.6.1 (Grimm, 1990). Pollen assemblage zones (Figure 5) were established using stratigraphically constrained cluster analysis conducted using incremental sum of squares (CONISS, Grimm, 1987). A pollen influx diagram was also created in TILIA using sediment accumulation rates calculated using the Bacon age model (Supplementary Figure S1).
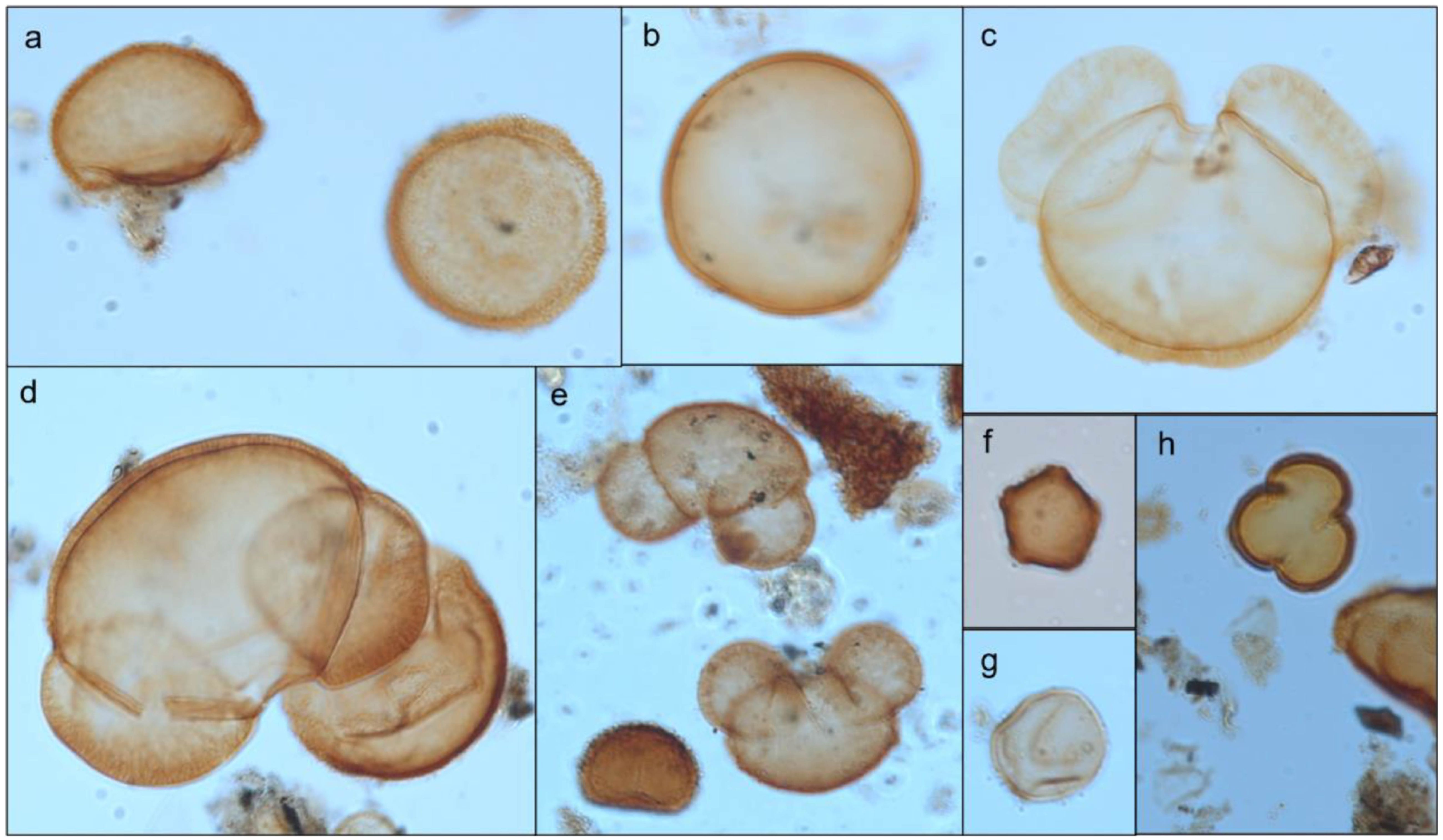
Figure 4. Pollen and spore grains from the Lost Lake core. Pollen grains are identified as: (A) Tsuga heterophylla (western hemlock); (B) Pseudotsuga menziesii (Douglas fir); (C) Abies (true fir); (D) Picea (spruce) in foreground and T. heterophylla behind; (E) Pinus (diploxylon) (vesicular grains) and Polypodium (polypody fern); (F); Alnus (alder); (G) Cupressaceae (cedar); (H) Acer (Maple, top center) and folded T. heterophylla. All grains identified using keys from Faegri and Iversen (1989); Moore et al. (1991), and type slides from the collection of Dr. Rolf Mathewes.
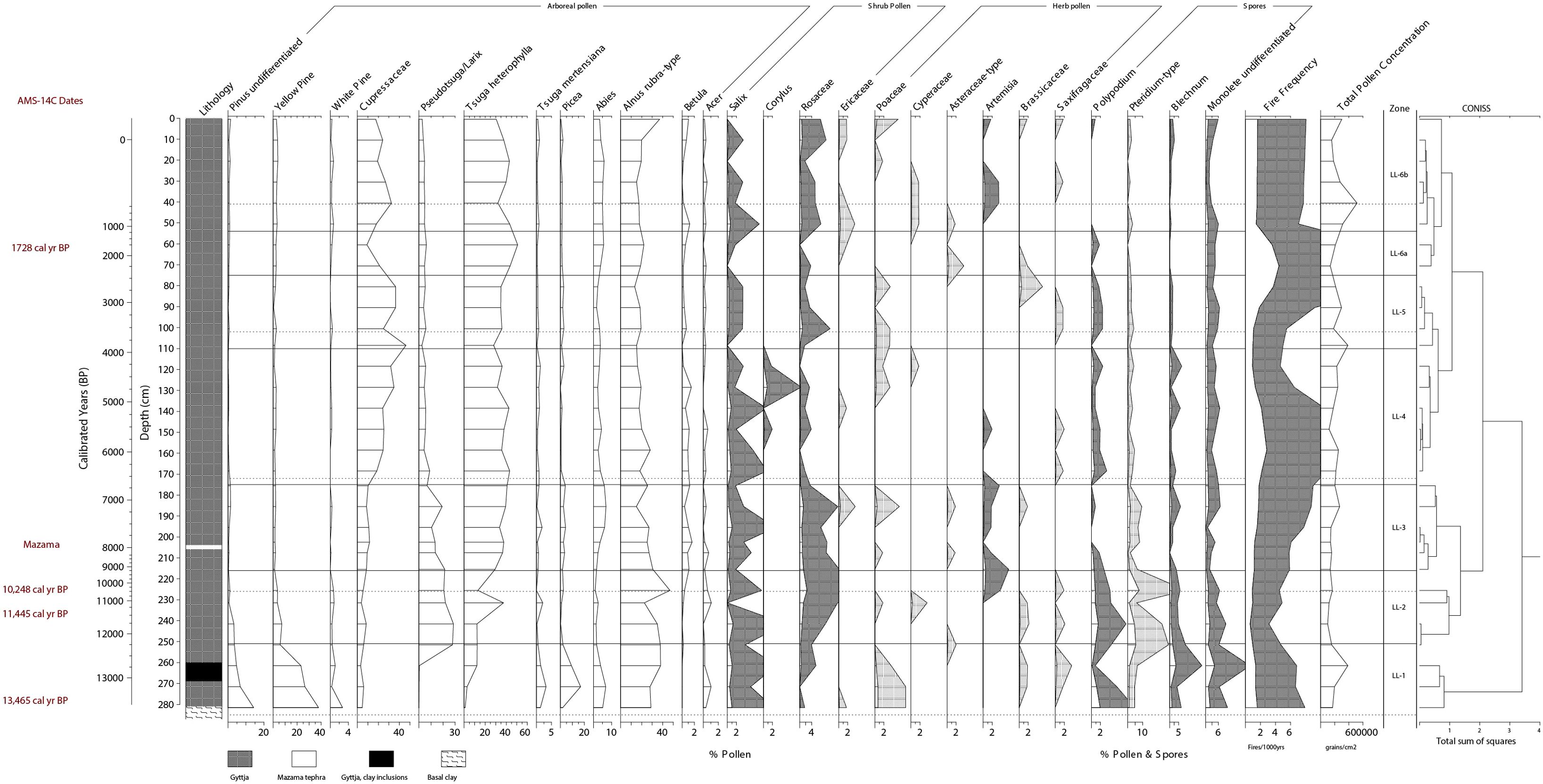
Figure 5. Pollen percent diagram of Lost Lake. Radiocarbon dates and lithology are shown on the left, fire frequency and total terrestrial pollen concentration are shown on the right. Tenfold exaggeration curves (light grey) are shown to highlight abundance of infrequent pollen and spores. Zones were delineated using constrained cluster analysis (CONISS). Solid horizontal lines represent zonal breaks. Dotted horizontal lines represent breaks in the core.
2.5 Charcoal analysis
Analysis of macroscopic charcoal followed methods commonly used in western North America modified from Hallett et al. (2003), Long et al. (1998) and Murphy et al. (2019). Contiguous 1-cm3 samples of sediment were taken at 1-cm intervals along the length of the composite core. Samples were first soaked for 24 hours in 20 ml of 5% Na(PO3)6 to facilitate disaggregation, then for 1 hour in a 6% H2O2 solution to lighten non-charcoal organic material. Samples were gently washed through a 125-μm sieve, backwashed into a 1-cm gridded petri dish, and dried for 24 hours at 30-40 °C. Samples >125 μm were then counted under a Leica® M205C stereomicroscope and identified based on the characteristics defined by Clark and Royall (1995). Recent research has demonstrated that use of oxidants (i.e., H2O2) in charcoal isolation can bleach charcoal formed at low burn temperatures (≦̸400°C), potentially leading to low-temperature particles becoming unquantifiable during optical microscope counts (Constantine and Mooney, 2022; Constantine et al., 2023). The paleorecord at Lost Lake suggests a conifer-dominated temperate rainforest was present for much of the Holocene and provides little evidence of low-intensity fires, indicating there may not be a significant decoupling between fire background component and vegetation composition. However, it must be acknowledged that the use of peroxide as a lightening agent may cause high-intensity fires to be overrepresented and low-intensity burns to be overlooked in the resultant fire record (Constantine and Mooney, 2022).
Charcoal analysis was conducted using the free software package CharAnalysis (Higuera et al., 2007) to measure the charcoal accumulation rate associated with fire episodes (CHAR, particles/cm2/year), background charcoal influx (particles/cm2/year), fire episode (peak) magnitude (charcoal pieces/cm2/peak), fire event frequency (# fires/1000yrs), and mean fire return interval (mFRI, measured in years). The median temporal resolution of the Lost Lake core (44 years) was used to interpolate charcoal counts, sample volume, and sample depths to evenly-spaced time intervals. The resulting interpolated sediment accumulation rates, which used mean ages from the Bacon age model, and interpolated charcoal concentrations were multiplied to calculate CHAR along the length of the core and for each of the individual pollen assemblage zones that were established from the constrained cluster analysis (i.e., LL-1 to LL-6b).
Fire episodes were represented by the high-frequency CHAR (Cpeak) component of the record that exceeded a threshold value which isolated fire-related peaks from non-fire related peaks. To separate fire-related peaks from non-fire related peaks, a Gaussian mixture model was used to define the noise distribution, with threshold values limited to the locally defined 99th percentile of the noise distribution. The cut-off probability for minimum counts was set to 0.05, indicating that the minimum charcoal count within 75 years before a given peak was required to have a less than 5% chance of coming from the same Poisson distribution as the maximum count associated with said peak, otherwise the peak was removed (Higuera et al., 2010). Fire frequency was estimated by summing the total number of fires using a 1000-year moving smoothing window on the fire-related peaks component of CHAR (Higuera, 2009). The mFRI was calculated by average the time periods between fire episodes along the length of the composite core (Agee, 1993). The mFRI and fire frequencies were also calculated for each of the seven pollen assemblage zones delineated in TILIA (Supplementary Table S4).
3 Results
3.1 Lithology
We identified four lithological units along the 294 cm length of the composite Lost Lake core. The deepest seven centimeters of the core (294 – 287 cm) was composed of gyttja. A fine-grained, light-gray clay from 287 to 281 cm was inferred to be a result of glacial scouring, and indicated that the 294 – 287 cm section was likely re-cored younger sediment caused by shifting during extraction of the Livingston corer. An inverted radiocarbon date from this section supports this hypothesis, and the 7-cm section was removed from the final lithology. Undifferentiated, dark brown gyttja was present from 281 to 269 cm, and was overlain by a 5-cm layer of lighter brown gyttja intermingled with small (1 – 5 mm) angular clay inclusions from 269 to 260 cm. From 260 to 206 cm, the sediment was composed dominantly of dark brown gyttja, with a mottled, inconsistent layer of presumably displaced Mazama tephra observed from 233 to 228 cm. Sediment for the depth interval of 206 – 204 cm consists of a consolidated light brown tephra from the Mount Mazama eruption. From 204 – 0 cm, the sediment was an undifferentiated dark brown gyttja containing few macrofossils.
3.2 Pollen analysis
Six pollen assemblage zones were identified in the Lost Lake composite core (LL-1 to LL-6, Figure 5) based on a constrained cluster analysis using CONISS total sum of squares (Grimm, 1987). The results of the pollen and charcoal analyses are presented in the context of these pollen assemblage zones.
3.2.1 Zone LL-1 (287 - 251 cm; >12.2 kcal BP)
Pinus reached its highest pollen percentage (>50%) at the beginning of Zone LL-1 (13.6 kcal BP) and then decreased substantially over the subsequent ca. 1400 years, reaching 9% by the end of the zone. Pseudotsuga/Larix pollen abundance remained at <1% from the base of the core until 12.7 kcal BP when it rapidly increased to its maximum value (~28%) during the transition between LL-1 and LL-2 at ca. 12.2 kcal BP. Picea (inferred to be Picea sitchensis) pollen gradually increased to its maximum percentage of 16% at 13.1 kcal BP and then steadily decreased to 2% by the end of Zone LL-1. Tsuga heterophylla abundance increased from <1% during the oldest part of the core to 12% at 12.7 kcal BP.
3.2.2 Zone LL-2 (251 – 216 cm; 12.2 – 9.1 kcal BP)
Tsuga heterophylla increased from 12% at the beginning of Zone LL-2 to ~29% by 9.1 kcal BP. Alnus decreased from 37% to 30%. Pinus continued to decline to ~1.5% of the assemblage by 9.1 kcal BP. Abies and Picea pollen abundances remained steady but low between 0.5 and 2%, respectively.
3.2.3 Zone LL-3 (216 – 175 cm; 9.1 – 6.7 kcal BP)
Pinus percentages remained below 3.5% for the duration of Zone LL-3. Tsuga heterophylla and Cupressaceae increased to 40% and 10%, respectively. Pseutotsuga/Larix decreased to 7% by the end of Zone LL-3. Shrub and herb pollen species experienced their highest percentages, with Rosaceae, Salix, and Artemisia reaching 1.3%, 1% and 0.55%, respectively.
3.2.4 Zone LL-4 (175 – 110 cm; 6.7 – 3.9 kcal BP)
Tsuga heterophylla abundances decreased from ~40% to 28 – 34% during Zone LL-4. Cupressaceae increased from 10% to nearly 40% by 3.9 kcal BP. Alnus pollen fluctuated between 15 and 20%. Pseudotsuga/Larix declined to <5% by the conclusion of Zone LL-4. Pinus, still dominated by P. contorta, remained below 3.5% of the pollen assemblage.
3.2.5 Zone LL-5 (110 – 75 cm; 3.9 – 2.4 kcal BP)
Tsuga heterophylla increased slightly to ~39% by ca. 2.4 kcal BP. Cupressaceae declined briefly to 24% before again rising to >30%, while Alnus fluctuated between 13 and 19%. Pinus and Pseudotsuga/Larix abundances fluctuated between 0.5-4% and 2-5%, respectively.
3.2.6 Zone LL-6a (75 – 54 cm; 2.4 – 1.2 kcal BP)
Tsuga heterophylla reached its maximum contribution of 50% of the assemblage at 1.7 kcal BP, at the expense of Cupressaceae, which decreased from >30% to 9% during Zone LL-6a. Alnus abundances remained between 17 and 22% of the assemblage. Pinus percentage steadily increased from 2.5 to 4% by 1.2 kcal BP, while Pseudotsuga/Larix contributed 4-6% of the pollen assemblage during Zone LL-6a.
3.2.7 Zone LL-6b (54 – 0 cm; 1,200 – -71 cal yr BP)
Alnus abundance increased from 17-22% to 36% at ca. -35 cal yr BP (~1985 AD). Cupressaceae sharply increased to 32% at ca. 140 cal yr BP at the expense of Tsuga heterophylla, which decreased to 32% before climbing back to a peak of 42% at approximately 20 cal yr BP (1930 AD). Pinus and Pseudotsuga/Larix abundance remained between 3- 6% and 2.5-5%, respectively. In all arboreal species and the majority of herb and shrub species pollen accumulation rates (Supplementary Figure S1) appear to emphasize rapid increases in influx in the final 200 years of the record. This pattern was not observed in the pollen percentage records of most species, but the percentages of Alnus rubra and Poaceae display moderate increases that follow the trend of their PARs.
3.3 Charcoal analysis
A total of 23 significant fire episodes were detected during the 13,900 years of the Lost Lake charcoal record (Figure 6; Supplementary Table S4). The mean CHAR for the Lost Lake composite record, interpolated to 44-year sample intervals, is 14 pieces/cm2/year. The period of highest CHAR occurred from -71 cal yr BP to 61 cal yr BP during Zone LL-6b (Figure 6). The current time since last fire (TSLF) is 144 years, and the most recent detected fire event occurred in 1845 AD. The longest interval of no recorded fire activity was approximately 1230 years between 12.5 and 11.2 kcal BP in Zone LL-2, while the mean fire-free interval was 555 years. The mean fire return interval (mFRI) for the composite core was 598 years with a natural range of variability of 466 - 735 years.
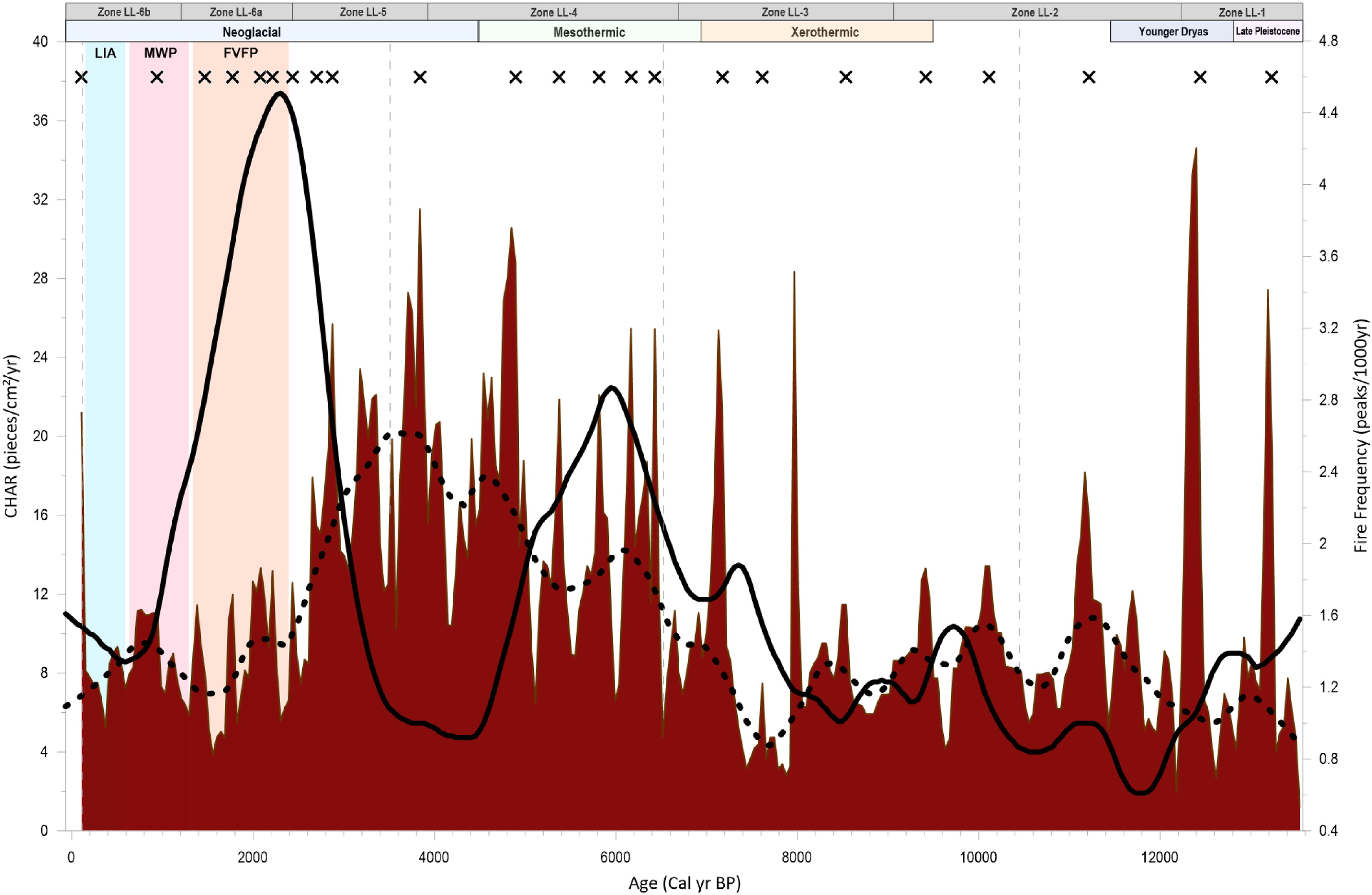
Figure 6. Synthesis of Lost Lake charcoal-inferred fire activity and major anthropogenic and climatic changes in southwestern British Columbia since the Late Glacial. Red fill represents interpolated CHAR; black solid line represents fire frequency; dotted black line represents interpolated background CHAR; X symbols represent fire events; Vegetation Zones LL-1 – LL-6b and major climatic intervals are shown at the top of the figure. Vertical colored bars represent the FVFP (orange), MWP (pink) and LIA (blue). Dotted grey vertical lines represent breaks in the Lost Lake core.
3.3.1 Zonal charcoal record
The charcoal record of Lost Lake was assessed in greater detail by using the CharAnalysis output data to calculate the fire characteristics for the individual pollen assemblage Zones LL-1 to LL-6 (Supplementary Table S4).
Fire frequency in the deepest section of the Lost Lake core was moderate (approximately 1.3 fires/1000 years) and remained low during the post-glacial, pine-dominated assemblage of Zone LL-1. Fire Frequency began increasing ca. 11.6 kcal BP and displayed a generally increasing trend throughout Zones LL-2 to LL-4. Fire frequency peaked at ca. 6.0 kcal BP when T. heterophylla and Cupressaceae dominated the pollen assemblage. A rapid decrease in fire frequency occurred between ca. 6.0 and 3.4 kcal BP during Zone LL-4 and was followed by a similarly rapid increase. Fire frequencies reached maximum values at 2.3 kcal BP. Fire frequency subsequently declined after 2.3 kcal BP with a minor increase from 550 cal yr BP to the present.
CHAR values have fluctuated substantially throughout the record but generally remained low-to-moderate in the earliest half of the core, with intermittent peaks throughout. CHAR began noticeably increasing in Zone LL-3 at approximately 7.3 kcal BP and remained elevated until ca. 4.0 kcal BP. Following 4.0 kcal BP, CHAR declined until its final small increase between approximately 550 cal yr BP and the present. Background CHAR generally aligns with the CHAR trend: it remained moderate-to-low during the earliest part of the Lost Lake core until approximately 7.5 kcal BP, at which point it increased substantially. Background CHAR levels peaked at approximately 3.6 kcal BP and then declined and remained low for the remainder of the core.
4 Discussion
4.1 Zonal interpretations
4.1.1 Zone LL-1 (287 - 251 cm; >12.2 kcal BP)
With an estimated basal age of 13.9 kcal BP, Zone LL-1 records the transition from the last glacial period into the Holocene period (11.7 kcal BP– present) (Gervais, 1867-1869), covering multiple climatic events including the Bølling-Allerød event, the Sumas glacial event, and the Younger Dryas period (Mathewes, 1993; Clague et al., 1997; Ivanovic et al., 2016). The presence of Salix and Poaceae in the deepest part of the Lost Lake core (>13.0 kcal BP) along with abundant diploxylon Pinus (yellow pine) suggest that an open, shrubby environment existed directly following deglaciation, which was quickly colonized by Pinus. We infer that the landscape was an open-canopy woodland dominated by shade-intolerant P. contorta until ca. 13,400 kcal BP.
As the climate moistened ca. ~13.0 kcal BP (Walker and Pellatt, 2003; Galloway et al., 2007; Hebda, 1995), the more shade-tolerant Tsuga mertensiana, Picea, and Abies [likely P. sitchensis and A. amabilis, based on the results of similar regional studies by Brown and Hebda (2002a); Lacourse (2005), and Wainman and Mathewes (1987)] increased in abundance at Lost Lake, likely indicating that coniferous forests began overtaking the landscape.
The Younger Dryas period (YD; 12.8 – 11.5 kcal BP) was a period of cooling in the Northern Hemisphere which was first observed in northwestern Europe (Rind et al., 1986), and later linked to coeval ecosystem-scale shifts in vegetation in the Rocky Mountains (Reasoner et al., 1994; Reasoner and Jodry, 2000), western North America (Kennett et al., 2008) and Pacific coastal North America (Mathewes, 1993; Walker and Pellatt, 2003; Galloway et al., 2009). At Lost Lake, the vegetation regime was dominated by pine with elevated fir (Abies) and spruce (Picea) until approximately 12.5 kcal BP, similar to nearby sites in in southwestern BC (Mathewes, 1973), in the Fraser Lowlands (Wainman and Mathewes, 1987), and in the CWHvm1 zone of northern Vancouver Island (Lacourse, 2005). The cool, boreal climate was not conducive to fire activity, with only two recorded fire episodes during Zone LL-1 at ca. 13.3 and 12.5 kcal BP. Elevated levels of background CHAR during fire events (Figure 6) may be indicative of fires that were occurring regionally outside the Lost Lake watershed (Clark et al., 1996; Long et al., 1998; Higuera et al., 2007), or to human activity, as the inferred boreal forest composition, cool climate and newly established forests at Lost Lake would not have been conducive to generating fuel loads capable of producing such high CHARs (Krawchuk & Moritz, 2011). Evidence of late Pleistocene-early Holocene aged human footprints on the central BC coast (Calvert Island, McLaren et al., 2018) and projectile points at a site approximately 50 km east of Lost Lake (Stave Lake, McLaren, 2017) suggest that southwestern BC was populated to some degree by the start of the Lost Lake record. Anthropogenic burning at this time could therefore be a cause of the decoupling of fire activity and climate.
4.1.2 Zone LL-2 (251 – 216 cm; 12.2 – 9.1 kcal BP)
At the end of the Younger Dryas chronozone, temperature began to increase across much of the Northern Hemisphere (Alley et al., 2000; Jennings et al., 2006; Brauer et al., 2008; Lynch-Stieglitz et al., 2011). At Lost Lake, this transition to warmer, dry conditions began ca. 12.0 kcal BP (± 580 years) and was indicated by increases in Pseudtosuga menziesii pollen abundances while Pinus, Picea, and Abies abundances remained very low. High abundances of Pteridium and Alnus indicate increased disturbance in the understory. Fire frequency also increased slightly during this interval but did not surpass 2 fires/1000yrs, indicating that the warming climate at Lost Lake was not accompanied by marked increases in fire within the watershed.
The inferred shift to warmer, drier conditions around Lost Lake after 12.0 kcal BP aligns with rising solar insolation values, which peaked at approximately 12.0 kcal BP (Figure 2), and increasing SST in the northeast Pacific between ca. 12.0 and 10.0 kcal BP (Figure 2; Kienast and McKay, 2001). The shade intolerance of the coastal variety of P. menziesii indicates that the ecosystem during this time was most likely a warmth-adapted P. menziesii-dominated forest with a shrubby understory.
The fire-sensitive species Tsuga heterophylla gradually increased to near-modern levels beginning ca. 10.0 kcal BP (Figure 5). This increase aligns temporally with a decline in summer insolation values and increase in winter insolation values at 60°N (Figure 2), and is supported by similarly timed T. heterophylla increases further north along the coast of BC (Lacourse, 2005; Galloway et al., 2009). A decrease in seasonality brought about by less extreme summer and winter solar insolation may have caused milder winter temperatures and a longer growing season, allowing T. heterophylla an opportunity to expand (Gavin et al., 2011; Meidinger and Pojar, 1991), replacing the less shade-tolerant Picea (Fastie, 1995).
4.1.3 Zone LL-3 (216 – 175 cm; 9.1 – 6.7 kcal BP)
The early Holocene xerothermic period (ca. 9.5 – 7.0 kcal BP, Mathewes, 1973) was a period of rapid warming in the western Canada, resulting in summers up to 2-4°C warmer than present in interior BC (Hebda, 1995; Walker and Pellatt, 2003; Rosenberg et al., 2004), and 1-2°C warmer than present in Pacific coastal North America (Hebda, 1995). The decline of P. menziesii and increase in T. heterophylla, Cupressaceae, and Picea abundances between ca. 9.0 and 8.0 kcal BP indicate that the climate around Lost Lake shifted toward moister conditions during the xerothermic period and likely remained relatively cool rather than warming significantly. Furthermore, following the Mazama ash horizon at ca. 7.6 kcal BP, Abies and Betula (likely swamp birch) percentages increased, indicating continued wetness and the beginning of cooler conditions at Lost Lake.
These results indicate that the xerothermic interval was not felt as strongly at Lost Lake in comparison to other sites in the Fraser Valley (Mathewes and Heusser, 1981; Wainman and Mathewes, 1987), on southern Vancouver Island (Brown et al., 2019), along the Pacific coast of Washington (Heusser et al., 1980) and at higher elevations in southwestern British Columbia (Hallett et al., 2003; Shea et al., 2022). In contrast, the interpreted cool, moist climate and mixed-species forest during Zone LL-3 at Lost Lake appears more similar to sites on the central coast of BC (Tiny Lake, Doherty, 2005; Galloway et al., 2009) and on northern Vancouver Island (Bear Cove Bog, Hebda, 1983; Misty Lake, Lacourse, 2005), which tended to experience a phase of warming earlier in the Holocene (between ca. 11.0 and 7.5 kcal BP), followed by a longer phase of cooling throughout the mid-Holocene.
4.1.4 Zone LL-4 (175 – 110 cm; 6.7 – 3.9 kcal BP)
During Zone LL-4, the inferred vegetation assemblage was dominated by T. heterophylla and Cupressaceae, with lesser amounts of Betula (swamp birch) and Salix (willow), indicating a moist climate continued to dominate from 6.7 to 3.9 kcal BP. Pseudotsuga declined to near modern levels by ca. 6.0 kcal BP, indicating that the climate was no longer dry enough to support its growth.
A local peak in fire frequency of ~3 fires/1000yrs, with an average mFRI of 308 years, occurred ca. 6.0 kcal BP (Figure 6; Supplementary Table S4), and was accompanied by elevated values of background CHAR between 6.5 and 4.0 kcal BP (Figure 6). These changes in fire frequency, mFRI, and background CHAR indicate that biomass burning increased locally around Lost Lake. Evidence of human activity has been abundant along the coast during the early-to-mid Holocene (Carlson, 1994, Carlson, 1996; Schaepe, 1998; Boyd, 1999; Lepofsky et al., 2005, 2009; Kenady et al., 2011; Walsh et al., 2015). This, in combination with little to no change in vegetation assemblage at Lost Lake, provides a basis for the idea that fire disturbance during this interval was not solely caused by climatic factors, and that human burning likely contributed as well.
While fire frequency decreased during the last half of Zone LL-4, CHAR remained high despite the inferred cool, moist climate. A potential cause for this elevated CHAR is burning that occurred either upwind of the lake or outside the watershed entirely (Gardner & Whitlock, 2001).
4.1.5 Zone LL-5 (110 – 75 cm; 3.9 – 2.4 kcal BP)
Zone LL-5 occurred shortly after the beginning of the Neoglacial period (ca. 4.5 kcal BP), during which several glacial advances occurred in the Coast Mountains likely in response to declining summer insolation in the Northern Hemisphere (Figure 2; Ryder and Thomson, 1986; Pellatt and Mathewes, 1997; Clague et al., 2009). At Lost Lake, moisture indicators Cupressaceae and T. heterophylla continued to dominate the vegetation assemblage and fire frequency remained below 1 fire/1000yrs. We infer that a hemlock-dominated assemblage was well established during Zone LL-5, and a wet moisture regime was present (Walker and Pellatt, 2003; Hallett and Hills, 2006).
Fire activity began to increase at Lost Lake at ca. 3.5 kcal BP, which coincided with the intensification of the positive (El Niño) phase of El Niño Southern Oscillation (ENSO) that is believed to have developed between 3.5 and 2.5 kcal BP (Moy et al., 2002). This time period was also characterized by an enhanced positive phase of the Pacific Decadal Oscillation (PDO) in the North Pacific Ocean after ca. 3.2 kcal BP (Barron and Anderson, 2011). The SST warming associated with the positive phases of both climate oscillations in the late Holocene (post- ~4.0 kcal BP) would have reduced the effective moisture (precipitation-evaporation) on the southern BC coast and caused drier winter conditions, likely contributing to increased fire activity.
4.1.6 Zone LL-6a (75 – 54 cm; 2.4 – 1.2 kcal BP)
The increase in fire frequency at Lost Lake also characterizes the beginning of Zone LL-6a. These increases in fire activity between ca. 3.5 and 2.3 kcal BP has similarly been observed in other paleoclimate studies in southwestern BC (Wainman and Mathewes, 1987; Hallett et al., 2003; Lepofsky et al., 2005) and northwestern Washington (Rorig and Ferguson, 1999; Prichard et al., 2009). Proposed causes include a period of regional climatic drying (the Fraser Valley Fire Period, 2.4 – 1.3 kcal BP; Hallett, 2001; Hallett et al., 2003) coinciding with a period of growing cultural interconnectedness along the Fraser River (the Marpole Phase, 2.4 – 1.2 kcal BP), during which archaeological evidence suggests social and economic networks amongst Coast Salish peoples expanded, increasing trade and thus buffering communities against ecological changes caused by the increased fire activity of the period (Lepofsky et al., 2005; Ritchie and Lepofsky, 2020).
During Zone LL-6a, pollen abundances at Lost Lake show little change, but a decline in T. plicata percentage at ca. 1.8 kcal BP is consistent with a slightly offset vegetational response to the increased wildfire and drought conditions inferred between ca. 3.0 and 2.0 kcal BP. From ca. 2.0 kcal BP until the end of Zone LL-6a at ca. 1.2 kcal BP, the pollen assemblage remained indicative of cool, wet conditions while fire frequencies declined. Moisture indicators Cupressaceae, Betula and Salix increased at approximately ca. 1.3 kcal BP, while T. heterophylla and P. menziesii remained stable, signaling only minor change to the overall forest composition.
4.1.7 Zone LL-6b (54 – 0 cm; 1,200 – -71 cal yr BP)
Lost Lake’s vegetation composition did not experience any significant changes between 1200 and 100 cal yr BP, indicating a relatively consistent environmental controls. In the past ~170 years (i.e., ca. 100 cal yr BP to present), fire activity at Lost Lake increased substantially, as evidenced by the large increases in CHAR, rising fire frequency, and high levels of disturbance in the pollen record.
The high CHAR beginning at 105 cal yr BP (AD 1845) was likely due to the increased disturbance caused by settler logging, mining and slash burning in the Lower Mainland. Increases in Pinus, Alnus, Pteridium, and shrub growth are likely due to higher levels of disturbance and stand gaps allowing increased understory growth. Cupressaceae, P. menziesii and T. heterophylla abundances decreased between ca. AD 1920 and AD 1940, most likely a direct result of logging activity.
4.2 Management implications
Looking ahead to the next century, watershed management in the LSCR has the potential to change substantially as vegetation, fire activity, and natural ranges of variability are altered by climate change (Hallett and Walker, 2000). Future climate simulations under RCP4.5 for the Metro Vancouver region project temperature increases of up to 3°C by the 2050s with rates of warming between 0.1°C to 0.6°C per decade (Wang et al., 2016; Metro Vancouver, 2016). Summer months are projected to have the highest rates of warming and up to a 20% decrease in precipitation (Metro Vancouver, 2018). Climate warming has been shown to produce longer, drier fire seasons (Abatzoglou and Williams, 2016).
An important implication of this warming and drying is a potential vegetational shift away from the moisture-loving T. plicata and T. heterophylla and an increase in fire-adapted species such as P. menziesii, perhaps moving towards a composition more similar to the drier subvariants of the CWH zone or the coastal Douglas fir (CDF) zones that currently exist in the rain shadow of Vancouver Island. Sites in the cool, moist variants of the CWH zone, such as the current conditions at our site, became more Douglas-fir dominated during the xerothermic period (Hebda, 1995). Currently, western redcedar is abundant on southern Vancouver Island in the CWHdm, CWHxm and CDF zones (Meidinger and Pojar, 1991; Hebda, 1997; Brown et al., 2019), confirming that it fares well in mixed species stands with western hemlock and coastal Douglas fir. Thus, while western redcedar may experience a decrease in abundance if precipitation decreases and/or fire activity increases, it is unlikely to disappear from the assemblage altogether. The dominant species in the current assemblage, western hemlock, has been present at Lost Lake at near modern levels since ca. 11.0 kcal BP, indicating it is highly resilient to the disturbances experienced in the watershed throughout the Holocene and is unlikely to shift a great amount. It must be noted that the timing of P. menziesii dominance at Lost Lake (ca. 12.2 – 9.1 kcal BP) was during a period of much higher summer solar insolation than present (Figure 2), which was a dominant factor in early Holocene warmth in southwestern BC (Gavin et al., 2011).
The current closed canopy forests surrounding Lost Lake indicate a higher fuel load and potential for high-severity, stand-replacing crown fires. Decreasing precipitation in the coming decades will likely dry fuels out, resulting in higher burn likelihood in the event of an ignition. Alternatively, a transition to intermediate precipitation and moderate to high fuel loads could result in mixed-severity fire regimes, causing a patchwork distribution of ground and crown fires that results in variable tree mortality, similar to what is seen in montane forests today (Agee, 1993).
We consider multiple hypotheses for the cause of Lost Lake’s perceived resilience to fire during its warmest period. The first is that the coastal setting of the LSCR created a climatic buffering effect as the cool, moist air of the Pacific Ocean dampened the insolation-driven summer temperature variations, somewhat protecting the site from extreme temperature and drought (Cwynar, 1987; Renssen et al., 2005; Galloway et al., 2009). The resulting wetter conditions along the coast may have allowed the vegetation around Lost Lake to withstand warmer temperatures due to the continuous moist climate, and this maritime influence is likely the reason drought-intolerant taxa such as T. heterophylla and Alnus were able to grow during the past warm period from ~9.5 – 7.0 kcal BP (Krajina, 1969). A second possibility is that high winter precipitation, coupled with the ability of root systems to access groundwater sources, provided a hydrologic buffer during periods of drought. As such, the vegetation around Lost Lake had ample groundwater sources even during warm, dry summers (Hahm et al., 2019; McCormick et al., 2021). We also note that during the late Holocene, the timing of peak fires in our record occur during the Neoglacial period at ca. 2.5 – 1.5 kcal BP, indicating that human activity, which is known to have been extensive during this time, may have contributed to high fire frequency in an otherwise cool climate (Lepofsky et al., 2009).
Similar to the inferred anthropogenic burning during the FVFP, the potentially intensive effects of human activity on fire frequency may have implications for watershed management as population density around the watersheds continues to increase. Housing developments in North Vancouver already border the southern boundary of the LSCR, and as the population of the Lower Mainland is projected to increase to 4.1 million by the 2040s (Ip and Lavoie, 2019), the risk of human-caused ignitions will increase around the watersheds.
5 Conclusion
This study presents new sedimentary pollen and charcoal records from Lost Lake that fill an information gap in the paleoclimate history of CWHvm1 forests in the coastal Lower Mainland of British Columbia. Our pollen record indicates that the period of highest temperature at Lost Lake occurred when P. menziesii was at its highest abundance (18-26% between ca. 12.2 and 9.2 kcal BP; Figure 5), which was several thousand years before the commonly described xerothermic interval (9.5 – 7.0 kcal BP). P. menziesii forest likely propagated at this time due to a combination of the inferred dry climate in the early Holocene (Walker and Pellatt, 2003) and the strong summer insolation (Figure 2) causing very warm summer conditions suitable to growth of the species. The low fire frequency and CHAR values during this time (Figure 6) can perhaps be explained by (a) lower overall biomass following deglaciation, or by (b) Lost Lake’s coastal proximity giving it a “moisture buffer” (Cwynar, 1987; Galloway et al., 2009) that prevented it from being as fire prone as more inland sites. The appearance of T. heterophylla beginning ca. 12.0 kcal BP, and its expansion beginning at ca. 11.5 kcal BP, provides additional evidence of coastal moisture moderation, as it indicates that even during a time of high summer insolation the Lost Lake site remained able to sustain a species that requires cool and/or moist conditions (Meidinger and Pojar, 1991).
Climate at Lost Lake during the mid-Holocene was cool and moist, producing western hemlock-western redcedar closed forests. Higher fire frequency was likely due to a combination of increased anthropogenic landscape modification and elevated charcoal influx due to increased precipitation. In the late Holocene (3.0 kcal BP onward), the vegetation assemblage at Lost Lake signaled a continuous temperate and moist climate, but fire frequency peaked during the Fraser Valley Fire Period at ca. 2.4 – 1.3 kcal BP. Plant assemblages were likely similar to modern day, but prolonged and frequent droughts may have occurred in summer due to a stronger summer Pacific High and weakened winter Aleutian Low (Fritz, 1996). This disconnect between fire and vegetation assemblage indicates that non-climatic factors such as increased anthropogenic burning were influencing fire activity, or that subsurface water storage allowed for increased resilience to summer drought conditions (Lepofsky et al., 2005; Hahm et al., 2019).
The interpreted climatic changes of this study indicate that natural vegetational succession, disturbance, broad-scale climatic changes, and human impact all contributed to the development of the current conditions found at Lost Lake. Based on our pollen and charcoal data and the province’s projected shift towards drier conditions within the century, the future vegetation and fire regime at Lost Lake may change in the following ways: (1) the fire season could lengthen, potentially with less available moisture during summers; (2) fire-adapted, drought-tolerant species such as P. menziesii and Pteridium may increase in abundance, and the ecosystem could shift towards a drier variant of the CWH zone; (3) fuel availability may rise due to increased mortality of arboreal species, potentially increasing fire risk if not managed; (4) continued population growth in the Greater Vancouver Area may increase the risk of ignitions in the WSA.
In conclusion, understanding the role of past climatic changes and ranges of variability provides a valuable perspective when considering future changes to the watershed that may occur as climate change progresses. Local-scale controls (i.e., topography/elevation, weather, human influence, fuel availability) undoubtedly play a part in Lost Lake’s paleoclimate history and should be considered when applying these results broadly to CWH forests on British Columbia’s southern coast. The potential effects of coastal moderation, combined with the apparent resilience of the forests around Lost Lake indicates that they are able to withstand large amounts of disturbance without major changes in assemblage, which bodes well for the future of the watershed.
Data availability statement
The datasets presented in this study can be found in online repositories. The names of the repository/repositories and accession number(s) can be found below: PANGAEA Data Repository (pollen data: https://doi.pangaea.de/10.1594/PANGAEA.968499; Charcoal data: https://doi.pangaea.de/10.1594/PANGAEA.968500).
Author contributions
MD: Conceptualization, Data curation, Formal analysis, Funding acquisition, Investigation, Project administration, Resources, Software, Validation, Visualization, Writing – original draft, Writing – review & editing, Methodology. MP: Conceptualization, Data curation, Formal analysis, Funding acquisition, Investigation, Methodology, Project administration, Resources, Software, Supervision, Validation, Visualization, Writing – review & editing. KK: Conceptualization, Data curation, Formal analysis, Funding acquisition, Investigation, Methodology, Project administration, Resources, Software, Supervision, Validation, Visualization, Writing – review & editing.
Funding
The author(s) declare financial support was received for the research, authorship, and/or publication of this article. This project was supported by joint funding from Mitacs Accelerate and the Metro Vancouver Regional District to MD, and a Natural Sciences and Engineering Research Council (Discovery Grant RGPIN342251) to KK. Parks Canada provided lab space and materials. The funders had no role in study design, data collection and analysis, decision to publish, or preparation of the manuscript.
Acknowledgments
We are thankful for the support provided by the Metro Vancouver Regional District, Parks Canada, and the Mitacs Accelerate program. Thank you to Dr. Rolf Mathewes for providing materials and assistance with pollen identification. Thanks to Hasini Basnayake, Jacqui Levy, Cass Perreira, and other members of the Climate, Oceans and Paleo-Environments Lab for assistance with laboratory and field work. We deeply appreciate the field assistance, site knowledge, discussions, and editing provided by D. Dunkley, which greatly improved the quality of the manuscript. Thank you to Dr. Scott Mooney and a reviewer for constructive comments that improved the manuscript. This manuscript is based on MD’s Masters of Resource and Environmental Management thesis (Duncan, 2022).
Conflict of interest
The authors declare that the research was conducted in the absence of any commercial or financial relationships that could be construed as a potential conflict of interest.
Generative AI statement
The author(s) declare that no Generative AI was used in the creation of this manuscript.
Publisher’s note
All claims expressed in this article are solely those of the authors and do not necessarily represent those of their affiliated organizations, or those of the publisher, the editors and the reviewers. Any product that may be evaluated in this article, or claim that may be made by its manufacturer, is not guaranteed or endorsed by the publisher.
Supplementary material
The Supplementary Material for this article can be found online at: https://www.frontiersin.org/articles/10.3389/fevo.2025.1504983/full#supplementary-material
References
Abatzoglou J. T., Williams A. P. (2016). Impact of anthropogenic climate change on wildfire across western US forests. Proc. Natl. Acad. Sci. – PNAS 113, 11770–11775. doi: 10.1073/pnas.1607171113
Allen G. B. (1995). Vegetation and climate history of southeast vancouver island, british columbia (Victoria (BC): University of Victoria).
Alley R. (2000). The younger dryas cold interval as viewed from central greenland. Quat. Sci. Rev. 19, 213–226. doi: 10.1016/S0277-3791(99)00062-1
Appleby P. G., Oldfield F. (1977). The calculation of lead-210 dates assuming a constant rate of supply of unsupported 210Pb to the sediment. Catena 5, 1–8. doi: 10.1016/S0341-8162(78)80002-2
Barnosky C. W. (1981). A record of late Quaternary vegetation from Davis Lake, southern Puget Lowland, Washington. Quat. Res. 16, 221–239. doi: 10.1016/0033-5894(81)90046-6
Barron J. A., Anderson L. (2011). Enhanced Late Holocene ENSO/PDO expression along the margins of the eastern North Pacific. Quat. Int. 235, 3–12. doi: 10.1016/j.quaint.2010.02.026
Berger A., Loutre M. F. (1991). Insolation values for the climate of the last 10 million years. Quat. Sci. Rev. 10, 297–317. doi: 10.1016/0277-3791(91)90033-Q
Beta Analytic (2022). Beta Analytic Standard Pretreatment Protocols. Available online at: https://www.radiocarbon.com/pretreatment-carbon-dating.htm (Accessed December 15, 2024).
Blake M. (2004). “Fraser Valley Trade and Prestige as Seen from Scowlitz,” in Complex Hunter-Gatherers: Evolution and Organization of Prehistoric Communities on the Plateau of Northwestern North America. Eds. Prentiss W. C., Kuijt I. (Salt Lake City: University of Utah Press), 103–112.
Blaauw M., Christen J. A. (2011). Flexible paleoclimate age-depth models using anautoregressive gamma process. Bayesian Anal. 6, 457–474. doi: 10.1214/11-BA618
Boyd R. (1999). Indians, fire, and the land in the Pacific Northwest (Corvallis, OR: Oregon State University Press).
Brauer A., Haug G., Dulski P., Sigman D., Negendank J. (2008). An abrupt wind shift in western europe at the onset of the younger dryas cold period. Nat. Geosci. 1, 520–523. doi: 10.1038/ngeo263
Brown K. J., Hebda R. J. (2002a). Ancient Fires on Southern Vancouver Island, British Columbia, Canada: A Change in Causal Mechanisms at about 2,000 ybp. Env. Archaeol: J. Hum. Palaeoecol. 7, 1–12. doi: 10.1179/env.2002.7.1.1
Brown K. J., Hebda R. J. (2002b). Origin, development, and dynamics of coastal temperate conifer rainforests of southern Vancouver Island, Canada. Can. Jour. For. Res. 32, 353–372. doi: 10.1139/x01-197
Brown K., Hebda N., Schoups G., Conder N., Smith K., Trofymow J. (2019). Long-term climate, vegetation and fire regime change in a managed municipal water supply area, British Columbia, Canada. Holocene 29, 1411–1424. doi: 10.1177/0959683619854523
Brown K. J., Hebda R. J. (2003). Coastal rainforest connections disclosed through a late quaternary vegetation, climate, and fire history investigation from the mountain hemlock zone on southern vancouver island, british colombia. Canada. Rev. Palaeobot. Palynol. 123, 247–269. doi: 10.1016/S0034-6667(02)00195-1
Carignan R., Arcy P. D., Lamontagne S. (2000). Comparative impacts of fire and forest harvesting on water quality in Boreal Shield lakes. Can. J. Fish. Aquat. Sci. 57, 105–117. doi: 10.1139/cjfas-57-S2-105
Carlson R. L. (1996). “Early Namu,” in Early Human Occupation in British Columbia. Eds. Carlson R. L., Della Bona L. (University of British Columbia Press, Vancouver).
Carlson R. L. (1994). “Trade and exchange in prehistoric british columbia,” in: Prehistoric exchange systems in North America. Interdisciplinary contributions to archaeology. Eds. Baugh T. G., Ericson J. E. (Boston, MA: Springer).
Chase M., Bleskie C., Walker I. R., Gavin D. G., Hu F. S. (2008). Midge-inferred holocene summer temperatures in southeastern british columbia, canada. Palaeogeogr. Palaeoclimatol. Palaeoecol. 257, 244–259. doi: 10.1016/j.palaeo.2007.10.020
Cheng H., Zhang H., Spötl C., Baker J., Sinha A., Dong X., et al. (2020). Timing and structure of the Younger Dryas event and its underlying climate dynamics. PNAS 117, 23408–23417. doi: 10.1073/pnas.2007869117
Clague J. J., Mathewes R. W., Guilbault J. P., Hutchinson I., Ricketts B. D. (1997). Pre-Younger Dryas resurgence of the southwestern margin of the Cordilleran ice sheet, British Columbia, Canada. Boreas 26, 261–278. doi: 10.1111/j.1502-3885.1997.tb00855.x
Clague J. J., Menounos B., Osborn G., Luckman B. H., Koch J. (2009). Nomenclature and resolution in Holocene glacial chronologies. Quat. Sci. Rev. 28, 2231–2238. doi: 10.1016/j.quascirev.2008.11.016
Clark J. S., Royall P. D. (1995). Particle-Size evidence for source areas of charcoal accumulation in late holocene sediments of Eastern North American lakes. Quat. Res. 43, 80–89. doi: 10.1006/qres.1995.1008
Clark J., Royall P., Chumbley C. (1996). The role of fire during climate change in an eastern deciduous forest at devil's bathtub, new york. Ecology. 77, 2148–2166. doi: 10.2307/2265709
Constantine M., Mooney S. (2022). Widely used charcoal analysis method in paleo studies involving NaOCl results in loss of charcoal formed below 400°C. Holocene 32, 1358–1362. doi: 10.1177/09596836211041740
Constantine M., Zhu X., Cadd H., Mooney S. (2023). Investigating the effect of oxidants on the quantification and characterization of charcoal in two southeast Australian sedimentary records. Fire (Basel Switzerland) 6, 54. doi: 10.3390/fire6020054
Cwynar L. C. (1987). Fire and the forest history of the North Cascade Range. Ecology 68, 791–802. doi: 10.2307/1938350
Davies A., Bunting M. (2010). Applications of palaeoecology in conservation. Open Ecol. J. 3, 54–67. doi: 10.2174/1874213001003020054
Derr K. (2014). Anthropogenic fire and landscape management on valdes island, southwestern BC. Can. J. Archaeol. 38, 250–279.
Doherty C. T. (2005). The Late-Glacial and Holocene relative sea-level history of the Seymour Inlet Complex, British Columbia, Canada. Queen’s University, Belfast, Belfast (Ireland).
Duncan M. E. (2022). Holocene fire and paleoclimate history of a small lake in the Lower Seymour Valley, British Columbia. Burnaby (BC): Simon Fraser University.
Eyquem J. L., Feltmate B. (2022). Irreversible Extreme Heat: Protecting Canadians and Communities from a Lethal Future (University of Waterloo: Intact Centre on Climate Adaptation).
Fastie C. L. (1995). Causes and ecosystem consequences of multiple pathways of primary succession at Glacier Bay, Alaska. Ecology 76, 1899–1916. doi: 10.2307/1940722
Friele P. A., Clague J. J. (2002). Younger dryas readvance in squamish river valley, southern coast mountains, british columbia. Quat. Sci. Rev. 21 (18), 1925–1933. doi: 10.1016/S0277-3791(02)00081-1
Fritz S. C. (1996). Paleolimnological records of climatic change in north america. Limnol. Oceanogr. 41, 882–889. doi: 10.4319/lo.1996.41.5.0882
Galloway J. M., Doherty C. T., Patterson R. T., Roe H. M. (2009). Postglacial vegetation and climate dynamics in the Seymour-Belize Inlet Complex, central coastal British Columbia, Canada: palynological evidence from Tiny Lake. J. Quat. Sci. 24, 322–335. doi: 10.1002/jqs.1232
Galloway J. M., Patterson R. T., Doherty C. T., Roe H. M. (2007). Multi-proxy evidence of postglacial climate and environmental change at Two Frog Lake, central mainland coast of British Columbia, Canada. J. Paleolim. 38, 569–588. doi: 10.1007/s10933-007-9091-4
Gardner J. J., Whitlock C. (2001). Charcoal accumulation following a recent fire in the cascade range, northwestern USA, and its relevance for fire-history studies. Holocene 11 (5), 541–549. doi: 10.1191/095968301680223495
Gauvreau A., Fedje D., Dyck A., Mackie Q., Hebda C. F. G., Holmes K., et al. (2023). Geo-archaeology and Haíɫzaqv oral history: Long-term human investment and resource use at EkTb-9, Triquet Island, N̓úláw̓itxˇv Tribal Area, Central Coast, British Columbia, Canada. J. Archaeol. Sci. Rep. 49, 103884. doi: 10.1016/j.jasrep.2023.103884
Gavin D. G., Brubaker L. B. (1999). A 6000-year soil pollen record of subalpine meadow vegetation in the Olympic mountains, Washington, USA. J. Ecol. 87, 106–122. doi: 10.1046/j.1365-2745.1999.00335.x
Gavin D., Brubaker L., Lertzman K. (2003). An 1800-year record of the spatial and temporal distribution of fire from the west coast of vancouver island, canada. Can. J. For. Res. 33, 573–586. doi: 10.1139/x02-196
Gavin D. G., Mclachlan J. S., Brubaker L. B., Young K. A. (2001). Postglacial history of subalpine forests, olympic peninsula, washington, USA. Holocene 11, 177–188. doi: 10.1191/095968301670879949
Gavin D. G., Hallett D. J., Feng S. H., Lertzman K. P., Prichard S. J., Brown K. J., et al. (2007). Forest fire and climate change in western North America: Insights from sediment charcoal records. Front. Ecol. Env. 5, 499–506. doi: 10.1890/060161
Gavin D. G., Henderson A. C. G., Westover K. S., Fritz S. C., Walker I. R., Leng M. J., et al. (2011). Abrupt Holocene climate change and potential response to solar forcing in western Canada. Quat. Sci. Rev. 30, 1243–1255. doi: 10.1016/j.quascirev.2011.03.003
Gervais P. (1867–1869). Zoologie et paléontologie générales: nouvelles recherches sur les animaux vértetebrés vivants et fossils (Paris: Arthus Bertrand), 263.
Gifford R., Brown C., Baron C., Clement D., Melnychuk N., Nelson H., et al. (2022). British Columbia Chapter in Canada in a Changing Climate: Regional Perspectives Report. Eds. Warren F. J., Lulham N., Lemmen D. S. (Government of Canada, Ottawa, Ontario).
Glew J. (1988). A portable extruding device for close interval sectioning of unconsolidated core samples. J. Paleolimnol. 1, 235–239. doi: 10.1007/BF00177769
Government of Canada (2022). Canadian Climate Normals 1981-2010 Station Data for Haney UBC RF Admin. Available online at: https://climate.weather.gc.ca/climate_normals/results_1981_2010_e.html?stnID=776&autofwd=1 (Accessed April 10, 2022).
Government of Canada (2024). Canadian Climate Normals 1991-2020 Data for Vancouver (Harbour) British Columbia. Available online at: https://climate.weather.gc.ca/climate_normals/results_1991_2020_e.html?searchType=stnProv&lstProvince=BC&txtCentralLatMin=0&txtCentralLatSec=0&txtCentralLongMin=0&txtCentralLongSec=0&stnID=359000000&dispBack=0 (Accessed December 30, 2024).
Green R. N., Blackwell B. A., Klinka K., Dobry J. (1999). Partial reconstruction of fire history in the Capilano watershed (Burnaby, B.C: Report to the Greater Vancouver Water District).
Green R. N., Klinka K. (1994). A field guide for site identification and interpretation for the vancouver forest region (Vancouver, BC: British Columbia Ministry of Forests).
Grimm E. C. (1987). CONISS: a FORTRAN 77 program for stratigraphically constrained cluster analysis by the method of incremental sum of squares. Comput. Geosci. 13, 13–35. doi: 10.1016/0098-3004(87)90022-7
Grimm E. C. (1990). Tilia and tiliagraph, PC spreadsheet and graphics software for pollen data, INQUA work. Gr. Data Handl. Methods Newsl. 4, 5–7.
Grove A. T. (2001). The “Little ice age” and its geomorphological consequences in mediterranean europe. Clim. Change 48, 121–136. doi: 10.1023/A:1005610804390
Hahm W. J., Rempe D. M., Dralle D. N., Dawson T. E., Lovill S. M., Bryk A. B., et al. (2019). Lithologically controlled subsurface critical zone thickness and water storage capacity determine regional plant community composition. Water Resour. Res. 55. doi: 10.1029/2018WR023760
Hallett D. J. (2001). Holocene fire history and climate change in southern british columbia, based on high-resolution analyses of sedimentary charcoal (Burnaby (BC): Simon Fraser University).
Hallett D. J., Hills L. V. (2006). Holocene vegetation dynamics, fire history, lake level and climate change in the Kootenay Valley, Southeastern British Columbia, Canada. J. Paleolim. 35, 351–371. doi: 10.1007/s10933-005-1335-6
Hallett D. J., Lepofsky D. S., Mathewes R. W., Lertzman K. P. (2003). 11,000 Years of fire history and climate in the mountain hemlock rain forests of southwestern British Columbia based on sedimentary charcoal. Can. J. For. Res. 33, 292–312. doi: 10.1139/x02-177
Hallett D., Walker R. (2000). Paleoecology and its application to fire and vegetation management in Kootenay National Park, British Columbia. J. Paleolim. 24, 401–414. doi: 10.1023/A:1008110804909
Hebda R. J. (1983). Late-glacial and postglacial vegetation history at Bear Cove Bog, northeast Vancouver Island, British Columbia. Can. J. Bot. 61, 3172–3192. doi: 10.1139/b83-355
Hebda R. J. (1995). British Columbia vegetation and climate history with focus on 6 ka BP. Géogr. Phys. Quater. 49, 55–79. doi: 10.7202/033030ar
Hebda R. J. (1997). “Impact of Climate Change on Biogeoclimatic Zones of British Columbia And Yukon,” in Responding to Global Climate Change in British Columbia and Yukon, vol. 1-15 . Eds. Taylor E., Taylor B. (Ottawa, Ontario: Environment Canada and British Columbia Ministry of Environment, Lands and Parks).
Hebda R. J., Mathewes R. W. (1984). Holocene history of cedar and native indian cultures of the north american pacific coast. Science 225 (4663), 711–713. doi: 10.1126/science.225.4663.711
Heusser L. E. (1983). Palynology and paleoecology of post-glacial sediment in an anoxic basin, Saanich Inlet, British Columbia. Can. J. Earth Sci. 20, 873–885. doi: 10.1139/e83-077
Heusser C. J., Heusser L. E., Streeter S. S. (1980). Quaternary temperatures and precipitation for the north-west coast of North America. Nature 286, 702–704. doi: 10.1038/286702a0
Higuera P. (2009). CharAnalysis 0.9: Diagnostic and analytical tools for sediment-charcoal analysis. Available online at: https://citeseerx.ist.psu.edu/viewdoc/download?doi=10.1.1.513.64&rep=rep1&type=pdf (Accessed December 1, 2021).
Higuera P. E., Peters M. E., Brubaker L. B., Gavin D. G. (2007). Understanding the origin and analysis of sediment-charcoal records with a simulation model. Quat. Sci. Rev. 26, 1790–1809. doi: 10.1016/j.quascirev.2007.03.010
Higuera P. E., Gavin D. G., Bartlein P. J., Hallett D. J. (2010). Peak detection in sediment–charcoal records: impacts of alternative data analysis methods on fire-history interpretations. Int. J. Wild. Fire 19, 996–1014. doi: 10.1071/WF09134
Ip F., Lavoie S. (2019). PEOPLE 2020: BC Sub-Provincial Population Projections. Available online at: https://www2.gov.bc.ca/assets/gov/data/statistics/people-population-community/population/people_population_projections_highlights.pdf (Accessed April 2022).
Ivanovic R., Gregoire L., Kageyama M., Roche D. M., Valdes P. J., Burke A., et al. (2016). Transient climate simulations of the deglaciation 21–9 thousand years before present (version 1) – PMIP4 Core experiment design and boundary conditions. Geosci. Mod. Dev. 9, 2563–2587. doi: 10.5194/gmd-9-2563-2016
Jennings A. E., Hald M., Smith M., Andrews J. T. (2006). Freshwater forcing from the Greenland ice sheet during the Younger Dryas: evidence from southeastern Greenland shelf cores. Quat. Sci. Rev. 25, 282–298. doi: 10.1016/j.quascirev.2005.04.006
Josenhans H., Fedje D., Pienetz R., Southon J. (1997). Early humans and rapidly changing Holocene sea levels in the Queen Charlotte Islands-Hecate Strait, British Columbia, Canada. Science 277, (71–74). doi: 10.1126/science.277.5322.71
Kenady S. M., Wilson M. C., Schalk R. F., Mierendorf R. R. (2011). Late Pleistocene butchered Bison antiquus from Ayer Pond, Orcas Island, Pacific Northwest: Age confirmation and taphonomy. Quat. Int. 233, 130–141. doi: 10.1016/j.quaint.2010.04.013
Kennett D. J., Kennett J. P., West G. J., Erlandson J. M., Johnson J. R., Hendy I. L., et al. (2008). Wildfire and abrupt ecosystem disruption on California’s Northern Channel Islands at the Ållerød–Younger Dryas boundary (13.0–12.9 ka). Quat. Sci. Rev. 27, 2530–2545. doi: 10.1016/j.quascirev.2008.09.006
Kidwell S. M. (2015). Biology in the Anthropocene: Challenges and insights from young fossil records. PNAS 112, 4922–4929. doi: 10.1073/pnas.1403660112
Kienast S. S., McKay J. L. (2001). Sea surface temperatures in the subarctic northeast Pacific reflect millennial-scale climate oscillations during the last 16 kyrs. Geophys. Res. Let. 28, 1563–1566. doi: 10.1029/2000GL012543
Krajina V. J. (1969). “Ecology of forest trees in British Columbia,” in Ecology of western North America. Vol. 1. Ed. V. J. Krajina (University of British Columbia, Department of Botany, Vancouver).
Krawchuk M. A., Moritz M. A. (2011). Constraints on global fire activity vary across a resource gradient. Ecology 92 (1), 121–132. doi: 10.1890/09-1843.1
Lacourse T. (2005). Late Quaternary dynamics of forest vegetation on northern Vancouver Island, British Columbia. Quat. Sci. Rev. 24, 105–121. doi: 10.1016/j.quascirev.2004.05.008
Landres P. B., Morgan P., Swanson F. J. (1999). Overview of the use of natural variability concepts in managing ecological systems. Ecol. Appl. 9 (4), 1179–1188. doi: 10.2307/2641389
Lepofsky D., Blake M., Brown D., Morrison S., Oakes N., Lyons N. (2000). The archaeology of the Scowlitz site, SW British Columbia. J. Field Archaeol. 27, 391–416. doi: 10.1179/jfa.2000.27.4.391
Lepofsky D., Lertzman K., Hallett D., Mathewes R. (2005). Climate change and culture change on the Southern coast of British Columbia 2400-1200 cal. B.P.: Hypothesis. Am. Antiq. 70, 267–293. doi: 10.2307/40035704
Lepofsky D., Schaepe D. M., Graesch A. P., Lenert M., Ormerod P., Carlson K. T., et al. (2009). Exploring Stó:Lō-coast salish interaction and identity in ancient houses and settlements in the Fraser Valley, British Columbia. Am. Antiq. 74, 595–626. doi: 10.1017/S0002731600048988
Lepofsky D., Smith N. F., Cardinal N., Harper J., Morris M., Gitla E. W., et al. (2015). Ancient shellfish mariculture on the northwest coast of North America. Am. Antiq. 80, 236–259. doi: 10.7183/0002-7316.80.2.236
Long C. J., Whitlock C., Bartlein P. J., Millspaugh S. H. (1998). A 9000-year fire history from the Oregon Coast Range, based on a high-resolution charcoal study. Can. J. For. Res. 28, 774–787. doi: 10.1139/cjfr-28-5-774
Lucas J. D., Lacourse T. (2013). Holocene vegetation history and fire regimes of pseudotsuga menziesii forests in the gulf islands national park reserve, southwestern british columbia, canada. Q. Res. 79, 366–376. doi: 10.1016/j.yqres.2013.03.001
Lynch-Stieglitz J., Schmidt M. W., Curry W. B. (2011). Evidence from the Florida Straits for Younger Dryas ocean circulation changes. Paleoceanography 26 (1), PA1205. doi: 10.1029/2010PA002032
Mann M. E., Zhang Z., Rutherford S., Bradley R. S., Hughes M. K., Shindell D., et al. (2009). Global signatures and dynamical origins of the little ice age and medieval climate anomaly. Science 326, 1256–1260. doi: 10.1126/science.1177303
Mathewes R. W., King M. (1989). Holocene vegetation, climate, and lake-level changes in the interior douglas-fir biogeoclimatic zone, british columbia. Can. J. Earth Sci. 26 (9), 1811–1825. doi: 10.1139/e89-154
Mathewes R. W. (1973). A palynological study of postglacial vegetation changes in the University Research Forest, southwestern British Columbia. Can. J. Bot. 51, 2085–2103. doi: 10.1139/b73-271
Mathewes R. W. (1993). Evidence for Younger Dryas-age cooling on the North Pacific coast of America. Quat. Sci. Rev. 12, 321–331. doi: 10.1016/0277-3791(93)90040-S
Mathewes R. W., Heusser L. E. (1981). A 12,000 year palynological record of temperature and precipitation trends in southwestern British Columbia. Can. J. Bot. 59, 707–710. doi: 10.1139/b81-100
Matson R. G., Coupland G. (1995). The Prehistory of the Northwest Coast (San Diego, CA: Academic Press), 364.
McCormick E. L., Dralle D. N., Hahm W. J., Tune A. K., Schmidt L. M., Chadwick K. D., et al. (2021). Widespread woody plant use of water stored in bedrock. Nature 597, 225–229. doi: 10.1038/s41586-021-03761-3
McCune J. L., Pellatt M. G., Vellend M. (2013). Multidisciplinary synthesis of long-term human–ecosystem interactions: a perspective from the garry oak ecosystem of british columbia. Biol. Conserv. 166, 293–300. doi: 10.1016/j.biocon.2013.08.004
McLachlan J. S., Brubaker L. B. (1995). Local and regional vegetation change on the northeastern olympic peninsula during the holocene. Can. J. Bot. 73 (10), 1618–1627. doi: 10.1139/b95-175
McLaren D. (2017). “The occupational history of the stave watershed,” in Archaeology of the lower fraser river region. Ed. Rousseau M. K. (Simon Fraser University, Burnaby, BC: Archaeology Press), 149–158.
McLaren D., Fedje D., Dyck A., Mackie Q., Gauvreau A., Cohen J. (2018). Terminal Pleistocene epoch human footprints from the Pacific coast of Canada. PloS One 13, e0193522. doi: 10.1371/journal.pone.0193522
McManus J. F., Francois R., Gherardi J. M., Keigwin L. D., Brown-Leger S. (2004). Collapse and rapid resumption of atlantic meridional circulation linked to deglacial climate changes. Nature 428, 834–837. doi: 10.1038/nature02494
Meidinger D., Pojar J. (Eds.) (1991). Ecosystems of British Columbia (Victoria, BC: Ministry of Forests and Range Research Branch).
Menounos B., Osborn G., Clague J. J., Luckman B. H. (2009). Latest Pleistocene and Holocene glacier fluctuations in western Canada. Quat. Sci. Rev. 28, 2049–2074. doi: 10.1016/j.quascirev.2008.10.018
Metro Vancouver (2016). Climate Projections for Metro Vancouver. Available online at: https://metrovancouver.org/services/air-quality-climate-action/Documents/climate-projections-for-metro-vancouver-2016.pdf (Accessed April 1, 2022).
Metro Vancouver (2018). Regional parks land acquisition 2050. (Metro Vancouver Regional District). Available at: http://www.metrovancouver.org/services/parks/ParksPublications/RegionalParksLandAcquisition2050.pdf (Accessed May 15, 2022).
Moore P. D., Webb J. A., Collinson M. E. (1991). Pollen Analysis. 2nd ed (Oxford: Blackwell Science).
Mote P. W., Salathé E. P. (2010). Future climate in the Pacific Northwest. Climatic Change 102, 29–50. doi: 10.1007/s10584-010-9848-z
Moy C. M., Seltzer G. O., Rodbell D. T., Anderson D. M. (2002). Variability of El Niño/Southern Oscillation activity at millennial timescales during the Holocene epoch. Nature 420, 162–165. doi: 10.1038/nature01194
Murphy S. F., Pellatt M. G., Kohfeld K. E. (2019). A 5,000-year fire history in the strait of Georgia Lowlands, British Columbia, Canada. Front. Ecol. Evol. 7. doi: 10.3389/fevo.2019.00090
NASA (2024). World of Change: Global Temperatures. Available online at: https://earthobservatory.nasa.gov/world-of-change/global-temperatures:~:text=According%20to%20an%20ongoing%20temperature,1.9%C2%B0%20Fahrenheit)%20since%201880 (Accessed December 30, 2024).
North Greenland Ice Core Project Members (2007). 50 year means of oxygen isotope data from ice core NGRIP. Supplement to: North Greenland Ice Core Project Members, (2004): High-resolution record of Northern Hemisphere climate extending into the last interglacial period. Nature 431, 147–151. doi: 10.1038/nature02805
Pacific Climate Impacts Consortium (2024). Available online at: https://www.pacificclimate.org/analysis-tools/plan2adapt (Accessed December 20, 2024).
Palmer S., Walker I., Heinrichs M., Hebda R., Scudder G. (2002). Postglacial midge community change and holocene palaeotemperature reconstructions near treeline, southern british columbia (Canada). J. Paleolimnol. 28, 469–490. doi: 10.1023/A:1021644122727
Pellatt M. G., Hebda R. J., Mathewes R. W. (2001a). High-resolution Holocene vegetation history and climate from Hole 1034B, ODP leg 169s, Saanich Inlet, Canada. Mar. Geol. 174, 211–226. doi: 10.1016/S0025-3227(00)00151-1
Pellatt M. G., Gedalof Z. (2014). Environmental change in garry oak (Quercus garryana) ecosystems: the evolution of an eco-cultural landscape. Biodivers. Conserv. 23, 2053–2067. doi: 10.1007/s10531-014-0703-9
Pellatt M. G., Smith M. J., Mathewes R. W., Walker I. R., Palmer S. L. (2000). Holocene treeline and climate change in the subalpine zone near stoyoma mountain, cascade mountains, southwestern british columbia, canada. Arctic Antarctic Alpine Res. 32 (1), 73–83. doi: 10.2307/1552412
Pellatt M. G., Mathewes R. W. (1994). Paleoecology of postglacial tree line fluctuations on the Queen Charlotte Islands, Canada. Écoscience 1, 71–81. doi: 10.1080/11956860.1994.11682230
Pellatt M. G., Mathewes R. W. (1997). Holocene tree line and climate change on the Queen Charlotte Islands, Canada. Quat. Res. 48, 88–99. doi: 10.1006/qres.1997.1903
Pellatt M. G., Mathewes R. W., Clague J. J. (2001b). Implications of a late-glacial pollen record for the glacial and climatic history of the Fraser Lowland, British Columbia. Paleogeog. Paleoclim. Paleoecol. 180, 147–157. doi: 10.1016/S0031-0182(01)00426-6
Pellatt M. G., McCoy M. M., Mathewes R. W. (2015). Paleoecology and fire history of Garry oak ecosystems in Canada: implications for conservation and environmental management. Biodiv. Cons. 24, 1621–1639. doi: 10.1007/s10531-015-0880-1
Prichard S. J., Gedalof Z., Oswald W. W., Peterson D. L. (2009). Holocene fire and vegetation dynamics in a montane forest, north cascade range, washington, USA. Q. Res. 72, 57–67. doi: 10.1016/j.yqres.2009.03.008
Reasoner M. A., Jodry M. A. (2000). Rapid response of alpine timberline vegetation to the younger dryas climate oscillation in the colorado rocky mountains, USA. Geology 28 (1), 51–54. doi: 10.1130/0091-7613(2000)028<0051:RROATV>2.3.CO;2
Reasoner M. A., Rutter N. W., Osborn G. (1994). Age of the crowfoot advance in the canadian rocky mountains. a glacial event coeval with the younger dryas oscillation. Geology. 22 (5), 439–442. doi: 10.1130/0091-7613(1994)022<0439:AOTCAI>2.3.CO;2
Reimer P. J., Austin W. E. N., Bard E., Bayliss A., Blackwell P. G., Ramsey C. B., et al. (2020). The Intcal20 northern hemisphere radiocarbon age calibration curve (0-55 cal kBP). Radiocarbon 62 (4), 725–757. doi: 10.1017/RDC.2020.41
Renssen H., Mairesse A., Goosse H., Mathiot P., Heiri O., Roche D., et al. (2015). Multiple causes of the younger dryas cold period. Nat. Geosci. 8, 946–949. doi: 10.1038/ngeo2557
Renssen H., Goosse H., Fichefet T., Brovkin V., Esschaert E., Wolk F. (2005). Simulating the Holocene climate evolution at northern high latitudes using a coupled atmosphere-sea ice-ocean-vegetation model. Clim. Dy. 24, 23–43. doi: 10.1007/s00382-004-0485-y
Rind D., Peteet D., Broecker W., McIntyre A., Ruddiman W. (1986). The impact of cold North Atlantic sea surface temperatures on climate: implications for the Younger Dryas cooling (11-10 k). Clim. Dy. 1, 3–33. doi: 10.1007/BF01277044
Ritchie M., Lepofsky D. (2020). From local to regional and back again: Social transformation in a Coast Salish settlement 1500–1000 BP. J. Anth. Arch. 60, 101210. doi: 10.1016/j.jaa.2020.101210
Rorig M. L., Ferguson S. A. (1999). Characteristics of lightning and wildland fire ignition in the Pacific Northwest. J. App. Meteorol. (1988) 38, 1565–1575. doi: 10.1175/1520-0450(1999)038<1565:COLAWF>2.0.CO;2
Rosenberg C. M., Walker I. R., Mathewes R. W., Hallett D. J. (2004). Midge-inferred Holocene climate history of two subalpine lakes in southern British Columbia, Canada. Holocene 14 (2), 258–271. doi: 10.1191/0959683604hl703rp
Ryder J. M., Thomson B. (1986). Neoglaciation in the southern Coast Mountains of British Columbia: chronology prior to the late Neoglacial maximum. Can. J. Ear. Sci. 23, 273–287. doi: 10.1139/e86-031
Sankey J. B., Kreitler J., Hawbaker T. J., McVay J. L., Miller M. E., Mueller E. R., et al. (2017). Climate, wildfire, and erosion ensemble foretells more sediment in western USA watersheds. Geophys. Res. Let. 44, 8884–8892. doi: 10.1002/2017GL073979
Schaepe D. M. (1998). Recycling Archaeology: Analysis of material from the 1973 excavation of an ancient house at the Mauer Site. Simon Fraser University, Burnaby (BC).
Schaepe D. M. (2009). Pre-colonial stó:lō Community organization: an archaeological perspective. University of British Columbia, Vancouver (BC).
Shea C. J., Steinman B. A., Brown E. T., Schreiner K. M. (2022). A multi-proxy lake-sediment record of middle through late Holocene hydroclimate change in southern British Columbia, Canada. J. Paleolim. 67, 163–182. doi: 10.1007/s10933-021-00231-8
Stuiver M., Reimer P. (1993). Extended 14C database and revised CALIB radiocarbon calibration program. Radiocarbon 35, 215–230. doi: 10.1017/S0033822200013904
Sugimura W. Y., Sprugel D. G., Brubaker L. B., Higuera P. E. (2008). Millennial-scale changes in local vegetation and fire regimes on mount constitution, orcas island, washington, USA, using small hollow sediments. Can. J. For. Res. 38, 539–552. doi: 10.1139/X07-186
Swetnam T. W., Allen C. D., Betancourt J. L. (1999). Applied historical ecology: using the past to manage for the future. Ecol. App. 9, 1189–1206. doi: 10.1890/1051-0761(1999)009[1189:AHEUTP]2.0.CO;2
Turner N. (2017). “Re Styecwmenúlecws-kucw: How We Look(ed) after Our Land,” in Secwépemc People, Land, and Laws: Yerí7 re Stsq’ey’s-kucw. Eds. Ignace M., Ignace R. E. (McGill-Queen’s University Press, Montreal & Kingston), 145–219.
Turner N., Peacock S. (2005). “Solving the Perennial Paradox: Ethnobotanical Evidence for Plant Resource Management on the Northwest Coast,” in Keeping it Living: Traditions of Plant Use and Cultivation on the Northwest Coast of North America. Eds. Deur D., Turner N. (UBC Press, Vancouver), 101–150.
Wainman N., Mathewes R. W. (1987). Forest history of the last 12000 years based on plant macrofossil analysis of sediment from Marion Lake, southwestern British Columbia. Can. J. Bot. 65, 2179–2187. doi: 10.1139/b87-300
Walker I. R., Pellatt M. G. (2003). Climate change in coastal British Columbia - A paleoenvironmental perspective. Can. Wat. Res. J. 28, 531–566. doi: 10.4296/cwrj2804531
Walsh M. K., Marlon J. R., Goring S. J., Brown K. J., Gavin D. G. (2015). A regional perspective on holocene fire–climate–human interactions in the Pacific Northwest of North America. Ann. Ass. Am. Geog. 105, 1135–1157. doi: 10.1080/00045608.2015.1064457
Wang T., Hamann A., Spittlehouse D., Carroll C. (2016). Locally downscaled and spatially customizable climate data for historical and future periods for North America. PloS One 11, e0156720. doi: 10.1371/journal.pone.0156720
Whitlock C., McWethy D. B., Tepley A. J., Veblen T. T., Holz A., McGlone M. S., et al. (2015). Past and present vulnerability of closed-canopy temperate forests to altered fire regimes. Bioscience 65, 151–163. doi: 10.1093/biosci/biu194
Williams J. (2006). Clam gardens: Aboriginal mariculture on Canada’s west coast (Vancouver: New Star Books).
Wright H. E., Mann D. H., Glaser P. H. (1984). Piston corers for peat and lake sediments. Ecology 65, 657–659. doi: 10.2307/1941430
Zdanowicz C. M., Zielinski G. A., Germani M. S. (1999). Mount Mazama eruption; calendrical age verified and atmospheric impact assessed. Geology 27, 621–624. doi: 10.1130/0091-7613(1999)027<0621:MMECAV>2.3.CO;2
Keywords: paleoclimate, paleoecology, wildfire, disturbance, novel ecosystems
Citation: Duncan ME, Pellatt MG and Kohfeld KE (2025) Coastal moderation of Holocene fire and vegetation change on the Pacific coast of Canada. Front. Ecol. Evol. 13:1504983. doi: 10.3389/fevo.2025.1504983
Received: 01 October 2024; Accepted: 16 January 2025;
Published: 10 February 2025.
Edited by:
Manel Leira, University of Santiago de Compostela, SpainReviewed by:
Scott David Mooney, University of New South Wales, AustraliaMaarten Blaauw, Queen’s University Belfast, United Kingdom
Copyright © 2025 Duncan, Pellatt and Kohfeld. This is an open-access article distributed under the terms of the Creative Commons Attribution License (CC BY). The use, distribution or reproduction in other forums is permitted, provided the original author(s) and the copyright owner(s) are credited and that the original publication in this journal is cited, in accordance with accepted academic practice. No use, distribution or reproduction is permitted which does not comply with these terms.
*Correspondence: Maggie E. Duncan, bWFnZ2llLmR1bmNhbkBkcmkuZWR1