- 1Habitat Regeneration and Landscaping Department, Wildlife and Natural Heritage, Royal Commission for AlUla, AlUla, Saudi Arabia
- 2Independent researcher, Göttingen, Germany
- 3Department of Biology, College of Science, Princess Nourah bint Abdulrahman University, Riyadh, Saudi Arabia
- 4Section of Organic Plant Breeding and Agrobiodiversity, University of Kassel, Witzenhausen, Germany
- 5Microbiology and Immunology Unit, Natural and Health Sciences Research Center, Princess Nourah bint Abdulrahman University, Riyadh, Saudi Arabia
- 6Environmental and biomaterial Unit, Natural and Health Sciences Research Center, Princess Nourah bint Abdulrahman University, Riyadh, Saudi Arabia
Drought is considered one of the most critical abiotic environmental stresses and limits plant growth, development, and productivity. It constitutes a real threat to humanity, especially in dry areas worldwide. Plants manage the negative effects of drought through a complex set of related mechanisms. Knowledge of plant responses and adaptation is more meaningful in plant breeding and genetics for improving drought resistance species. This review will focus on drought response mechanisms and drought adaptation, providing examples from plant species differing in their life-form, including herbaceous and woody plants. Additionally, the potential role of enhancing plant drought responses will be emphasized. This review is of potential significance to researchers and those who wish to obtain a glimpse into plant behavior under drought conditions.
1 Introduction
Drought is one of the most serious abiotic factors that limits plant growth, development, and productivity (Iqbal et al., 2022). Drought is defined as an event of shortages in the water supply, whether atmospheric, surface water, or groundwater (Zia et al., 2021), in the short term or long term (Bodner et al., 2015). Meteorologists define drought as a prolonged period without significant, precipitation (Zia et al., 2021), and this includes dryland regions that are classified climatically as arid, semi-arid, and dry subhumid. Drylands account for more than one-third of the Earth’s land surface (Peguero-Pina et al., 2020). Contrary to a dry climate, agricultural drought occurs when the plant roots have limited water availability through rainfall and/or irrigation to meet excessive transpiration (Zia et al., 2021), which adversely affects their metabolism, plant growth, and development (Rai and Rai, 2020). Agricultural drought occurs particularly in drylands, in which the cultivated area accounts for 25% of dryland areas (Bodner et al., 2015). However, a periodically unexpected drought stress often occurs in most of the other land areas (Fang and Xiong, 2015).
Most herbaceous and woody plants are mainly present in semi-arid and sub-humid areas (Peguero-Pina et al., 2020; Fang and Xiong, 2015). Even over a short period, drought can markedly affect plant growth and productivity via its direct and/or indirect influences on photosynthesis, metabolism, and other physiological and cellular processes (Iqbal et al., 2022). The impact of drought depends on its duration and severity, the plant species and genotype (Bodner et al., 2015), the developmental stage of the plant (Nezhadahmadi et al., 2013), and the age of a tree (Wang et al., 1995). For instance, it was reported that yield loss reached 20% and 46.8% in wheat (Triticum aestive L.) plants exposed to drought at the booting and tillering stages, respectively, whereas it was 64.46% when the plant was subjected to drought at the anthesis stage (Nezhadahmadi et al., 2013). In a similar context, other important food and economic crops have also been affected negatively by drought, such as soybean in which reductions in pollen germination (17%), seed weight (35%), and seed number (45%) were noted under drought stress (Poudel et al., 2023). A strong negative effect of drought on rice yield and physiology has been reported, particularly at the flowering stage (Yang et al., 2019). Furthermore, popcorn maize (Zea mays L.), suffered chlorophyll degradation and early senescence under drought stress (Schmitt et al., 2024).
Besides herbaceous plants, woody plants, i.e., trees and shrubs, are markedly influenced by soil water shortages, particularly over a prolonged period in different regions (Iqbal et al., 2022). For instance, the Norway spruce (Picea abies) population in Central Europe is more sensitive to drought stress than other conifers (Schiop et al., 2017). Furthermore, severe droughts in Texas and California have resulted in the death of approximately 300 million and 102 million trees, respectively, which affected the forest carbon balance and ecosystem function (Choat et al., 2018).
Over and above that, the future food demand for a continuously growing world population (approximately 9 billion in 2050), together with limited water resources and climate change, further aggravates the effects of drought, which will become the most critical threat to global food security, particularly in tropical countries (Seleiman et al., 2021; Zia et al., 2021). It is estimated that approximately 1.8 billion people will face water shortages by 2025 (Nezhadahmadi et al., 2013). Therefore, it is imperative to improve drought tolerance in plants and utilize economical and beneficial agriculture practices to mitigate the effects of climate change and thus increase food security (Seleiman et al., 2021). To meet this purpose, intensive knowledge of the mechanisms of plant responses to drought at different levels is required. This review focuses on the response mechanisms of plants exposed to drought. These responses will be tackled in detail below, providing examples from different life-forms, i.e., herbaceous and woody plants, and the interconnections among these responses, where appropriate, will be highlighted. In addition, we focus on understanding the mechanism of tree mortality under drought. Finally, we discuss the plant adaptive responses to drought stress and their application in drought tolerance in plants.
2 Morphological and physiological drought response
2.1 Growth and productivity
Germination initiates when the seed imbibes adequate water to establish new plants, whereas under drought, seeds do not imbibe sufficient water, which ultimately reduces germination as well as the overall plant number per unit area (Zia et al., 2021). Poor seedling growth has also been reported under drought stress. This is due to the interruption of the water supply to meristematic cells at the vegetative stage, which inhibits cell elongation and reductional cell division (Zia et al., 2021). Several studies have shown a significant reduction in several growth parameters, such as the number of leaves, tillers, leaf surface area, shoot length, internode elongation, fresh and dry matter, and plant height in response to drought stress (Farooq et al., 2009; Fahad et al., 2017; Zia et al., 2021). On the other hand, the incremental growth of root and related traits (root/shoot ratio, length, density, weight, and volume) was observed in some drought-stressed plants (Fang and Xiong, 2015). Such a condition is correlated with increasing water absorption from the deep soil layer, i.e., hydrotropism (Bodner et al., 2015). Declines in root elongation, branching, and the formation of the cambium layer has been reported under severe drought (Fang and Xiong, 2015). During the reproductive growth of plants, drought stress directly influences flowering, pollination, and seed development. This causes a reduction in the number and size of seeds and fruits or the shrinking of seeds, which leads to significant yield and quality losses (Dietz et al., 2021). Table 1 presents a summary of several morphological and physiological effects of drought stress on plants, which are discussed in detail herein.
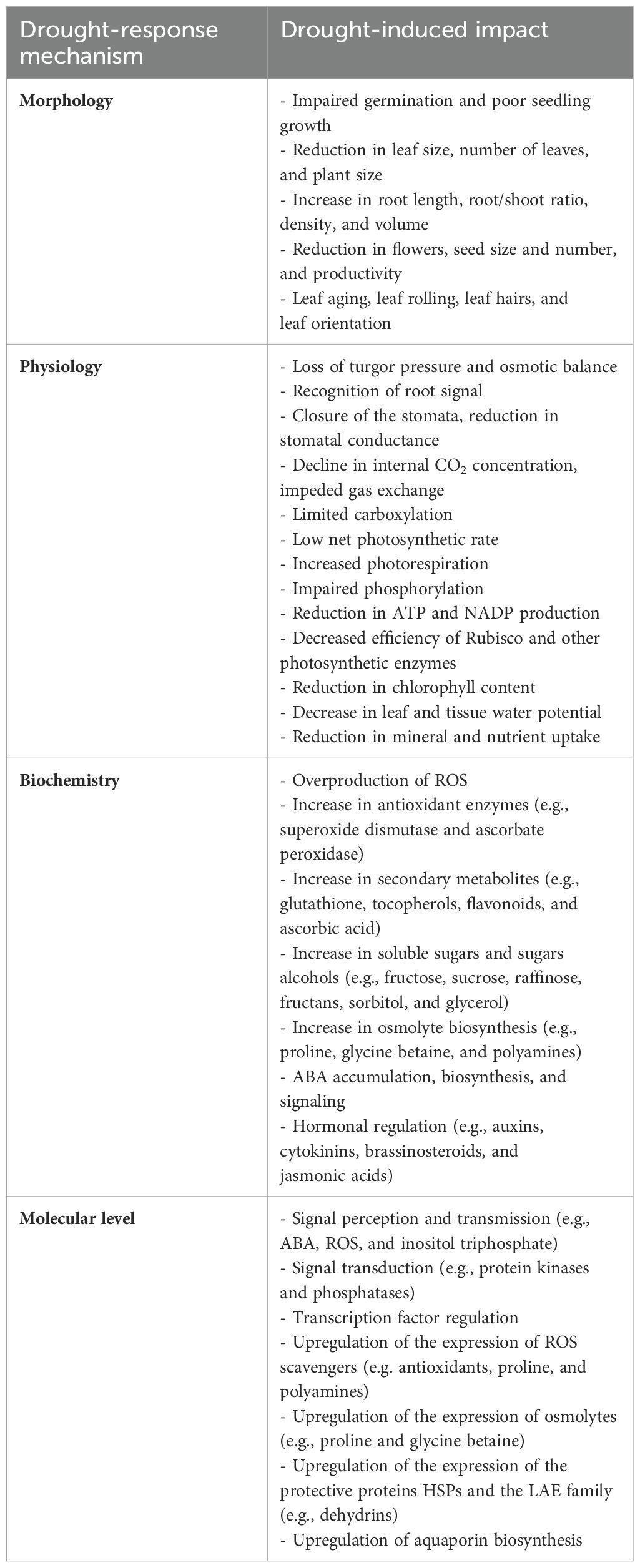
Table 1. Plant drought responses, from morphological, physiological, biochemical, and molecular perspectives.
In herbaceous plants, a significant reduction in the length and weight of both shoot and root under drought has been well detected in several Solanaceous species such as pepper (C. annum L.), potato (S. tuberosum L.), and tomato (S. lycopersicum L.) (Aghaie et al., 2018). It was reported that the percentage reduction in yield under drought stress was 63–87% in maize (Z. mays L.), 57% in wheat (T. aestivum L.), and 60% in sunflower (Helianthus annus L.) (Fahad et al., 2017). With regard to woody plants, drought damage has been detected in the seedlings and young trees of the Norway spruce (P. abies L. Karst) (Schiop et al., 2017). Furthermore, the decline in the growth efficiency (wood growth per leaf area) of the Douglas fir (Pseudotsuga menziesii) and lodgepole pine (Pinus contorta) was probably caused by hydraulic limitations on water transport in trees (Hunt et al., 1999). The production of apples (Malus domestica Borkh) (Ping and Bai, 2015) and olive fruits (Olea europaea) is markedly influenced by drought stress, particularly over a prolonged period in different regions (Brito et al., 2019).
Taken together, a reduction in plant growth is likely a consequence of reduced turgor pressure and rate of photosynthesis under drought exposure (Farooq et al., 2009; Zia et al., 2021). Biomass allocation (i.e., reduced leaf area and enlarged root system) is the main morphological modification under drought (Dietz et al., 2021).
2.2 Closure of stomata
The main role of stomatal opening and closing is to balance water vapor loss and CO2 uptake (Zargar et al., 2017). Drought induces stomatal closure to avoid water loss through transpiration to stabilize the water status of the shoot (Dietz et al., 2021) and thus increases water use efficiency (WUE, the ratio of photosynthetic and transpiration rates) in most plants (Chaves et al., 2003) under mild drought. The closure of stomata (stomatal limitation) under drought stress reduces the flow of CO2 and decreases CO2 assimilation, which in turn significantly causes low photosynthetic activity. In addition, it influences other physiological and biochemical processes, such as a reduction in leaf and tissue water content, gas exchange, ion exchange between the root and shoot, and growth limitation (Oguz et al., 2022), and makes plants susceptible to oxidative damage (Fahad et al., 2017), while it increases leaf and canopy temperature (Rai and Rai, 2020). Stomatal regulation is more closely linked to the soil moisture content than to leaf water status, and it might be the reason why stomata respond to special hormonal signals, mainly abcissic acid (ABA), transmitted from the roots via xylem flux upward to the shoot in a drying soil profile (Fahad et al., 2017).
In wheat (T. aestivum) plants subjected to drought in a controlled growth chamber, the net photosynthesis rate dramatically decreased following reductions in leaf stomatal conductance, transpiration rates, and intercellular CO2 concentration. The suppression of these gas exchange variables led to a significant yield loss (Hassan, 2006). In another study, a significant decline in photosynthetic rates in soybean (G. max L.) plants (Ohashi et al., 2006) and different wheat (T. aestivum) cultivars (Subrahmanyam et al., 2006) exposed to water stress was correlated with a reduction in stomatal conductance and transpiration rates in leaves. In orange tree (Citrus sinensis L.) the interactions between the gas exchange variables has been extensively reported under drought stress conditions, due to the reduction in stomatal conductance, which decreases leaf water potential (Gomes et al., 2004). Additionally, Kitao et al. (2003) found a reduction in the net assimilation rate in long-term drought- stressed Erman’s birch trees (Betula ermanii) as a consequence of a lower leaf intercellular CO2 concentration due to stomatal closure. Cano et al., 2013 showed that the limitations in photosynthesis of drought-stressed sessile oak (Quercus petraea) and beech (Fagus sylvatica) tree leaves were related to the reductions in stomatal conductance (the diffusion of CO2 from the air surrounding the leaf to substomatal cavities) and mesophyll conductance (the diffusion of CO2 from the substomatal cavities to chloroplastic stroma).
Taken together, decreased transpiration upon stomatal closure is an initial plant response to mild drought, which affects the net photosynthesis rate (Figure 1).
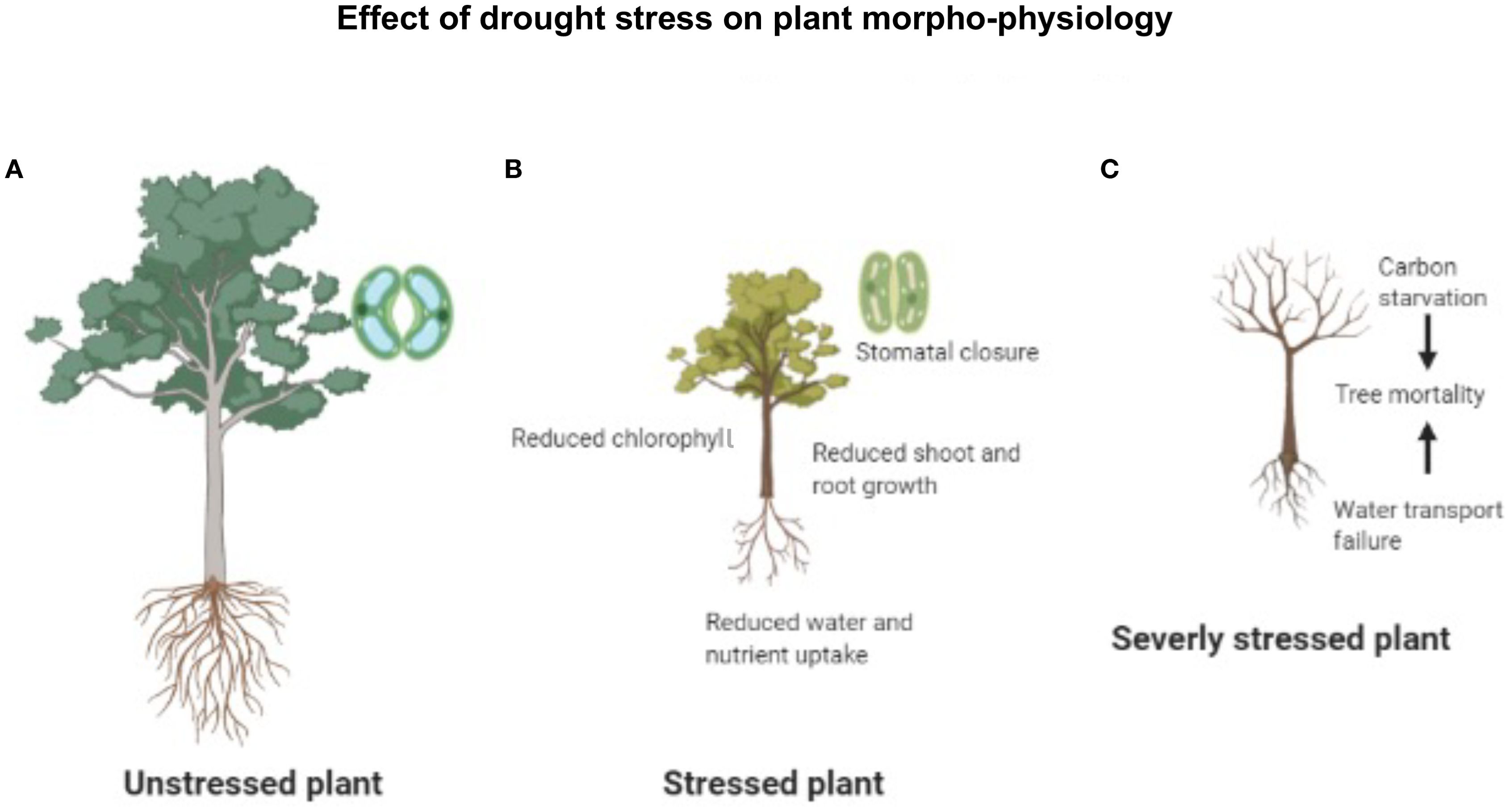
Figure 1. The morpho-physiology and appearance of the plant under different water availability conditions. (A) A plant under normal conditions (unstressed plant). (B) A plant suffering drought stress (stress plant). (C) A plant suffering severe drought stress and tree mortality (severely stressed plant).
2.3 Photosynthesis
Photosynthesis is one of the prime physiological implications of the water deficit condition (Kamanga et al., 2018), which arise from stomatal closure and metabolic impairment (Farooq et al., 2009), known also as photochemical limitations (Bodner et al., 2015). Under mild to moderate drought, the stomatal limitation is the chief factor for the dimension of the net photosynthesis rate (Fahad et al., 2017). In severe drought, photosynthesis is directly limited through the impairment of the activity of enzymes involved in photosynthesis and thus malfunctioning photosynthetic apparatus (Zargar et al., 2017). A reduction in the activation state of the CO2- binding enzyme called ribulose 1,5 bisphosphate carboxylase oxygenase (Rubisco) under severe drought conditions could impair the process of photosynthesis in many plant species (Das et al., 2020; Fahad et al., 2017). Such a reduction is accompanied by a reduction in carboxylation, the rate of ribulose bisphosphate (RuBP) regeneration, and stromal fructose-1,6-bisphosphatase activity and thus adversely affects the quantum efficiency of PS II (Kaur and Asthir, 2017). It has also been observed that a decline in CO2 assimilation is usually associated with the increase in the photorespiration rate (Brito et al., 2019). Moreover, impaired photophosphorylation and the decreased production of adenosine triphosphate (ATP) and nicotinamide adenine dinucleotide phosphate (NADP) (Bhargava and Sawant, 2013) are the major factors limiting photosynthesis under drought (Jaldhani et al., 2022). Table 2 presents the different drought-plant responses between a mild-moderate and severe drought.
In tobacco (N. tabacum), a decrease in CO2 supply to Rubisco under both mild and severe water stress was primarily responsible for the observed reduction in CO2 fixation, which leads to the inhibition of photosynthesis (Parry et al., 2002). In addition, Dias and Brueggemann (2010) showed a strong reduction in the activity of Calvin cycle enzymes, e.g., Rubisco, stromal fructose-1,6-bisphosphatase, and ribulose-5-phosphate kinase in water-stressed common bean (Phaseolus vulgaris L.), suggesting that the decline of CO2 assimilation under drought stress was probably due to the decline of enzyme activity involved in RuBP regeneration. A study by Bota et al., 2004 suggested that a dominant role of decreased stomatal conductance in photosynthesis downregulation during mild drought, and the impairment of Rubisco activity and RuBP content limits photosynthesis in severe drought. In olive leaves (O. europaea L.), a reduction in photosynthetic Calvin cycle enzymes, mainly Rubisco downregulation, was observed under drought stress (Brito et al., 2019) as well as in Fuji apple leaves (M. domestica Borkh.) (Ping and Bai, 2015). A study on a detrimental effect of drought in two species of leguminous trees, Prosopis chilensis and Prosopis tamarugo, indicated that a decrease in CO2 supply to Rubisco is primarily responsible for a reduction in CO2 fixation under mild and severe water stress (Delatorre et al., 2008).
Taken together, photochemical limitations, i.e., reductions in Rubisco activity, RuBP regeneration, and ATP synthesis, are responsible for the decline in photosynthesis during severe drought.
2.4 Chlorophyll pigment
Chlorophyll is the most important group of photosynthetic pigments responsible for light absorption and is found in the thylakoids of the chloroplasts. Both the composition and concentration of chlorophyll [chlorophyll-a, chlorophyll-b, the chlorophyll a/b ratio, and total chlorophyll (a + b)] have a direct influence on photosynthesis and dry weight (Li et al., 2019). Under drought stress, the thylakoid membranes in the chloroplast are damaged, adversely affecting the synthesis of chlorophyll (Figure 1). Moreover, the reduction in chlorophyll content of plant leaves under drought has also been reported in some species, which could directly limit photosynthesis (Oguz et al., 2022). Many studies have provided sufficient evidence that the reduction in chlorophyll content under drought stress is attributed to the inactivation of important chlorophyll biosynthetic enzymes (Rai and Rai, 2020) or oxidative damage of chloroplast lipids and chlorophyll degradation (Oguz et al., 2022).
In canola plants (Brassica napus L.), the average reduction in total chlorophyll (a + b) was 23.8% in five cultivars exposed to drought stress at the flowering stage (Din et al., 2011). In another study, a 35–60% decrease in chlorophyll synthesis and the chlorophyll a/b ratio has been reported in plant species such as cucumber (Cucumis sativus) and tomato (S. lycopersicum), which is caused by the inactivation of the 5-aminolevulinate dehydratase enzyme (Rai and Rai, 2020). However, an increased chlorophyll content and chlorophyll-a compared with chlorophyll-b was observed in cereals plants (Fahad et al., 2017) and herbaceous plants such as ajwain (Trachyspermum ammi L.) (Azhar et al., 2011). A study conducted with Norway spruce (P. abies L. Karst) seedlings under water stress showed a slight reduction in photosynthetic activity as a result of degradation in chlorophyll-a and chlorophyll-b content in the needles of water-stressed spruce seedlings (Schiop et al., 2017). In oil palm (Elaeis guineensis, tenera), the ratio of chlorophyll a/b and total chlorophyll (a + b) was significantly decreased under water stress (Azzeme et al., 2016). In contrast, the concentration of chlorophylls remained unchanged in Fuji apple leaves in a drought progression experiment (Ping and Bai, 2015).
The chlorophyll content is another critical component affected by drought stress and is plant species dependent. Therefore, the characterization of plant species that are resistant to chlorophyll degradation under drought stress is the target of plant breeders to improve abiotic resistance (Kamanga et al., 2018).
2.5 Plant-water relations
The disturbance of plant-water relations starts when the available water in soil decreases, and then water potential and turgor in the leaves become lower than that in the roots, and stomatal closure occurs, with consequent adverse effects on plant growth (Moradi, 2016). A significant reduction in relative water content (RWC, i.e., the percentage of water present at the time of sampling, in relation to the amount of water in a saturated leaf), leaf water potential, and transpiration rate, with a simultaneous increase in leaf and canopy temperature, has been observed in several plants species (Jaldhani et al., 2022; Kamanga et al., 2018; Fahad et al., 2017).
In herbaceous plants such as on triticale (X. triticosecale) and maize (Z. mays), the impact on leaf water potential was greater in drought-sensitive plants than in drought-resistant genotypes (Grzesiak et al., 2006). Similarly, Kramer and Boyer (1995) demonstrated the differential sensitivity of plant genotypes with reduced tissue RWC, concluding that the sensitive genotypes were more drastically affected than the tolerant ones. More than a 40% reduction in RWC has been reported in wheat, maize and amaranths (Amaranthus sp.) under drought stress (Zia et al., 2021). Regarding woody plants, the reduction in the total water content of Indian fig (Opuntia sp.) cladodes was 57% under drought stress. This is attributed to water loss by parenchyma of the cladodes; therefore, a lower turgor potential was observed (Nerd and Nobel, 1991). Early investigations on jack pine (Pinus banksiana Lamb.), black spruce (Picea mariana Mill.), and aspen (Populus tremuloides Michx) demonstrated that net photosynthesis, as well as mesophyll conductance, showed no response to leaf water potential until a threshold point was reached, below which they decreased linearly (Dang et al., 1997). Variation in the leaf water potential threshold point is an important physiological trait and is species-specific (Kolb and Stone, 2000). A study by Aref et al. (2013) showed that water-stressed African juniper (Juniperus procera) could photosynthesize at a water potential as low as −5.77 MPa. Such a low water potential at which African juniper sustained its photosynthetic activity might lead to speculation that the photosynthetic apparatus of J. procera is relatively stable under a limited water supply.
Among different factors influencing plant-water relations, the leaf water potential threshold point could be used as an index to compare different species in terms of their responses to water stress and in turn the sensitivity of their photosynthetic apparatus (Kolb and Stone, 2000).
2.6 Plant-nutrient relations
Drought has a negative impact on the uptake of minerals and nutrients from the soil as it negatively affects enzymes related to nutrient absorption and translocation. For example, a decline in the activity of nitrate reductase causes a lower uptake of nitrate (NO3-) from the soil (Poudel et al., 2021). A reduction in transpiration could also limit the movement and diffusion of water-soluble nutrients in the soil, such as Si, Mg, Ca, NO3-, and phosphate (PO4-3), leading to a reduction in the growth rate of plants (Poudel et al., 2021). Low soil moisture content causes a reduction in root growth that negatively affects the uptake of less mobile nutrients such as P (Fahad et al., 2017). The improper transportation of nutrients from roots to shoots leads to a reduced concentration of nutrients in plant tissues (Das et al., 2020).
In crop plants, water deficit conditions result in an increase in N content, with no change in K content (Das et al., 2020). An investigation on tea plants (Camellia sinensis, cv. O Kuntze) has demonstrated that the mineral uptake of Ca, Zn, Cu, Mn, Mg, and B is obstructed under drought stress (Das et al., 2020). The impaired C and O2 flux to the nodules, coupled with N accumulation in legumes plants such as soybean (Glycine max L. Merr.), inhibited N fixing ability under drought stress (Fahad et al., 2017). In beech seedlings (F. sylvatica L.), the total P and PO4-3 content was dramatically reduced in roots and shoots under water- deficit conditions, and the C, N, and NO3- content differed between roots and shoots under stress (Peuke and Rennenberg, 2004). A study by Schulte et al. (1998) revealed that the partitioning of S between the plant parts in young pedunculate oak (Quercus robur L.) was affected by drought. The phloem loading and/or phloem transport of reduced sulfur was inhibited after water stress.
Plant-nutrient relations under abiotic stress become more sophisticated due to the interactive effects of different nutrients on each other (Jaldhani et al., 2022).
3 Biochemical and molecular drought responses
3.1 Oxidative damage
The exposure of plants to drought stress triggers an excessive production of reactive oxygen species (ROS) and makes plant cells suffer oxidative stress. This occurs when plants absorb more light than can be utilized in carbon fixation due to stomatal closure, leading to a break in the dynamic equilibrium between intracellular generation and the removal of ROS by its scavenging system (Fang and Xiong, 2015). ROS are highly reactive compounds including non-radicals such as singlet oxygen (1O2), hydrogen peroxide (H2O2), and free radicals like superoxide radical (O2•) and hydroxyl radical (OH•) (Bandurska, 2022). The overproduction of ROS prompts oxidative stress and cellular damage through the oxidation of lipids, protein denaturation, enzyme inhibition, DNA strand breakage, and blocked photosynthesis (Yang et al., 2021), Such consequences lead to activation of the programmed cell death pathway and ultimately leading to cell death and injury (Das et al., 2020). It was found that lipid peroxidation via ROS increases the amount of malondialdehyde, which becomes an indicator of oxidative damage under drought stress (Fahad et al., 2017). ROS production is reported to be upregulated by drought stress (Foyer and Noctor, 2005), which might be related to stomatal closure associated with the limitation on CO2 fixation (Cruz de Carvalho, 2008). Therefore, the overproduction of ROS, along with ABA and Ca+2, acts as a stress signal (second messengers) that alters the transcription of genes, thereby participating in the acclimation of plants to abiotic stresses (Oguz et al., 2022). Table 1 shows the various biochemical and molecular effects of drought stress, which are described in detail here. Foyer et al. (1994) reported that the reduction of chlorophyll content under drought stress can be attributed to increased ROS, which causes lipid peroxidation, resulting in chloroplast damage and eventually chlorophyll degradation. This is consistent with the result reported by Moran et al. (1994), who found that lipid peroxidation and protein degradation were increased by four times in pea (Pisum sativum) plants under drought where drought-induced ROS generation. A study by Patanè et al. (2022) found that long- shelf-life tomatoes from cv. Vulcano had a great resistance to oxidative stress under drought because of low levels of malondialdehyde compared with the control. In woody plants, Brito et al. (2019) reported that the exposure of olive trees (O. europaea L.) to severe drought stress causes oxidative damage through the formation of ROS. These ROS cause serious damage to the cellular operation by damaging lipids, proteins, carbohydrates, pigments, and DNA, as well as increased cellular membrane damage and electrolyte leakage. A study by Carraro and Di Iorio, 2022 reported that woody plants from Citrus rootstocks cv. Carrizo citrange showed increased drought resistance due to lower malondialdehyde and H2O2, along with other attributes related to the ROS scavenging system, compared with the control. Therefore, malondialdehyde content may be considered as a biomarker for the drought stress condition.
3.2 Antioxidant defense system
Nitric oxide (NO) and ROS have significant signaling functions in plants via their interaction as they have a role in modifying proteins involved in their metabolism and homeostasis (Lindermayr and Durner, 2015). Their accumulation under stress may lead to the inactivation of vital signaling molecules and cell damage (Wani et al., 2021). To protect plants from ROS damage, plants induce endogenous antioxidant defense systems, including enzymatic and non-enzymatic antioxidants. The synergistic effect of both components is involved in the modulation of ROS and coping with oxidative stress. Antioxidant enzymes include superoxide dismutase, catalase, ascorbate peroxidase, glutathione reductase, monodehydro-ascorbate reductase, and dehydroascorbate reductase, whereas non-enzymatic antioxidants are secondary metabolites (Ahmad et al., 2023), including ascorbate, glutathione, ascorbic acid, tocopherols, flavonoids (e.g. phenolics), and carotenoids (Yang et al., 2021). Recent studies have provided evidence that soluble sugars (e.g., disaccharides, raffinose, and fructans), along with the traditional antioxidants, play a role in ROS (Zia et al., 2021). These antioxidants protect plants either by directly scavenging the ROS or by increasing non-enzymatic defense. For example, superoxide dismutase is important in catalyzing the dismutation of two molecules of superoxide (O2-) into O2 and H2O2 (Carraro and Di Iorio, 2022). Flavonoids and the carotenoids:chlorophyll ratio play a role in scavenging singlet oxygen (1O2), thereby lessening the oxidative damage (Ansari et al., 2019).
In barely plants, increased activities of catalase and superoxide dismutase were observed in drought-tolerant genotypes compared to drought-sensitive genotypes (Kaur and Asthir, 2017). Drought induced antioxidant activity by increasing the levels of anthocyanin and total flavonoids in the flowering plant Labisia pimila L., and ascorbic acid and glutathione in Mexican cotton (Gossypium hirsutum L.) and the common bean (P. vulgaris L.) (Ahmad et al., 2023). High leaf phenolic concentration and antioxidant activity with low lipid peroxidation and photochemical damage was observed in olive (O. europaea L. cv. Gaidourelia) leaves exposed to a water deficit (Brito et al., 2019). Furthermore, three antioxidant enzyme activities, superoxide dismutase, catalase, and peroxidase, were found in drought-stressed plants from Carrizo citrange, which increased drought tolerance (Carraro and Di Iorio, 2022). Therefore, the maintenance of high levels of antioxidant compounds can be a good strategy for plants to cope with oxidative stress and increases plant drought stress tolerance (Fahad et al., 2017).
3.3 Osmotic adjustment
As a response to water deficiency in the soil, plants stimulate osmotic adjustment, also named osmoregulation, which involves the accumulation and synthesis of osmotically active substances to increase the capability of cells to hold/retain water through reducing (more negative) the osmotic potential of cell cytoplasm and, consequently, increase the gradient for water influx and the maintenance of cell turgor (Yang et al., 2021; Moradi, 2016). These substances are termed as osmolytes, also named compatible solutes or osmoprotectants (Moradi, 2016). Osmolytes are highly soluble molecules, non-toxic at molar concentrations, and part of normal metabolic reactions (Oguz et al., 2022; Moradi, 2016). They can be divided into different groups depending on the character of compound: osmolytes containing ammonium compounds (e.g., glycine betaine, and polyamines); osmolytes containing sugars and sugar alcohols (e.g., fructose, glucose, sucrose, trehalose, fructan, mannitol, sorbitol, and glycerol); osmolytes containing amino acids (e.g., proline, citrulline, pipecolic acid, and ectoine); and osmolytes containing sulfonium compounds (choline o-sulfate and dimethyl sulfoniumpropironate) (Wach and Skowron, 2022). Under drought stress, these compounds contribute to the maintenance of the structural integrity of cell membranes by maintaining turgor pressure (Yang et al., 2021) and to the stabilization of enzymes and proteins by replacing neighboring water molecules and hence preventing the build-up of intramolecular hydrogen bonds that cause irreversible structural disturbances (Moradi, 2016). They also play dual roles in scavenging ROS under drought stress (Oguz et al., 2022).
Among them, proline is one of the most important osmolytes studied in drought-stressed plants. A large body of data found a positive correlation between proline accumulation in leaves and mechanisms of plant tolerance to harmful water deficits (Kamanga et al., 2018). In addition to its function as an osmolyte, proline acts as a source of energy, carbon, and nitrogen, and as an ROS scavenger, in which it plays a role in the stabilization of enzymes and proteins and in the prevention of cell death in drought-stressed plants (Wang et al., 2022).
In herbaceous plant such as watermelon (Citrullus lanatus L.), the accumulation of free proline in leaves, stems, and roots has been detected under a water deficit (Wang et al., 2022). Studies on maize (Z. mays L.) showed that proline content increased in the growing region of the primary root at a low water potential level due its increased transport, and not due to the proline biosynthesis in the roots (Verslues and Sharp, 1999). Moreover, Farooq et al. (2009) stated that free proline content was increased in drought-tolerant petunia (Petunia hybrid) varieties under drought stress. In woody plants, Norway spruce seedlings (P. abies sp.) showed an accumulation of proline levels in needles subjected to a short and severe water stress treatment in the greenhouse (Schiop et al., 2017). The accumulation of proline has also been reported in beech (F. sylvatica L.) (Leuschner, 2020), Persian walnut (Juglans regia L.), poplar (P. nigra) trees (Polle et al., 2019), and citrus cultivars (Carrizo citrange and Cleopatra mandarin) (Carraro and Di Iorio, 2022) under water deficit. Therefore, proline content is an interesting target in increasing plant tolerance (Carraro and Di Iorio, 2022).
3.4 ABA biosynthesis and signaling
Abscisic acid (ABA) is a small molecule lipophilic phytohormone that is naturally produced at a low concentration under normal conditions in plants (Iqbal et al., 2022). Upon water deficits, ABA plays a pivotal role in the signal connections in the aboveground and underground parts of plants. The roots of plants suffering drought stress produce ABA, which transmits a signal via vascular bundles to leaves, causing leaf senescence and stomatal closure (Yang et al., 2021). This, in turn, reduces the transpiration rate and increases WUE (Tenorio Berrío et al., 2022). It also triggers the expression of the ABA biosynthesis gene (Brunner et al., 2015) or is transported via xylem to leaves (Yang et al., 2021) and thus increases the ABA content in leaves. Another study demonstrated that the accumulation of ABA under drought stress plays a role in regulating hydraulic conductance in roots via increasing aquaporin activity (also named ion channel protein), a membrane protein regulator of plant-water relations (Brunner et al., 2015), which potentiates a 10- to 20-fold increase in water permeability at the soil-root surface (Farooq et al., 2009).
The accumulation of ABA acts as a stress signal of drought stress for the expression of drought-responsive genes (i.e., those expressed only under pronounced water shortages) (Iqbal et al., 2022) and promotes the synthesis of different protective proteins (Ahmad et al., 2023).
ABA induces several transduction signals, such as protein kinases (SnRK2), phosphatase, and G-proteins (Yang et al., 2021). The induction of SnRK2 occurs and activates downstream transcription factors that regulate the expression of drought-responsive genes (Ahmad et al., 2023; Iqbal et al., 2022). Generally, the ABA level is low in plants under normal conditions; however, drought-stressed plants increase their ABA level. The activation of the ABA-dependent signaling pathway occurs due to the binding of ABA with appropriate receptors, pyrabactin resistance (PYR), pyrabactin resistance-like (PYL), and the regulatory component of ABA receptors (RCAR), thus enhancing the stimulation of the signal transduction cascade under stress conditions (Fidler et al., 2022). Carraro and Di Iorio (2022) reported that ABA accumulation in leaves was detected in both herbaceous and woody plants exposed to drought. They stated that leaves from Sunki maravilha had significantly higher ABA than Rangpur lime, which showed greater drought tolerance in the former trees.
3.5 Drought-responsive gene expression
The molecular response of plants to drought stress is a complex process involving signal perception and multiple signal transduction pathways, resulting in the expression of drought-responsive genes with different functions, causing the plant to undergo physiological and biochemical changes during drought stress (Yang et al., 2021). Signal perception by receptors is a common feature of plants and other living organisms and is considered the first step in plant signal transduction under stress conditions (Lamaoui et al., 2018). In general, the external drought stimuli, i.e., the hydraulic and electrical signals, are recognized and captured by receptors on the cell membrane, and this is followed by the generation of intercellular second messengers such as inositol triphosphate, Ca+2, ROS, ABA, and phosphoglycerol. Then, signals are amplified gradually through the cascade transmission of the signals, resulting in the expression of drought-responsive genes (Yang et al., 2021; Oguz et al., 2022). Plants use two signaling pathways, named the ABA-dependent and ABA-independent pathways, to upregulate or downregulate the expression of drought-responsive genes. These genes can be divided into two groups depending on protein function. The first group compromises genes encoding functional proteins that directly contribute to drought tolerance. It includes antioxidant enzymes, osmolytes (e.g., proline, sucrose, betaine), protective proteins [e.g., heat shock protein (HSPs) and late embryogenesis abundant (LEA) protein such as dehydrins], and key enzymes involved in the biosynthesis of aquaporin genes. The crucial role of those molecules is to minimize the cellular damage caused by drought stress. The second group comprises genes encoding regulatory proteins that are required for stress signaling. It includes genes that encode for protein kinases, protein phosphatases, phospholipid metabolism-related genes, transcription factors, calmodulin, and those involved in protein degradation (Yang et al., 2021; Fang and Xiong, 2015).
In chickpea (Cicer arietinum L.) seedlings under drought stress, it was reported that 56 genes were downregulated, e.g., metabolism-related genes, whereas 36 genes were upregulated, such as ABA catabolism, stress-related, and cellular processing genes (Maqbool et al., 2017). In woody plants such as teak (Tectona grandis L.), transcriptome analysis revealed the expression levels of 977 genes from the root contigs in response to drought stress treatment (Galeano et al., 2019). A total of 5,331 transcripts were differentially expressed in Populus sp. and 2,445 transcripts were differentially expressed in Pinus sp. in the response of roots to drought. Several genes encoding HSPs (also called chaperones), the LAE family (e.g., dehydrins), and enzymes involved in ROS were upregulated in root tissues in response to cellular dehydration in poplar and pine trees (Brunner et al., 2015).
4 Drought-induced tree mortality
Although tree mortality is primarily attributed to pathogens and other factors such as competition, windthrow or tree age, recent evidence shows that extreme drought can also lead to the widespread mortality of trees and woodland plants in many forest types (Leuschner, 2020; Senf et al., 2020; Choat et al., 2018). This would be completely in agreement with a previous study by Allen et al., 2010, who reported that drought can act as a trigger that may eventually lead to mortality in trees that are already under stress from old age, poor soil features, and air pollution, and subject to root and stem damage by fungal pathogens and insects, e.g., wood-boring insects. In another study, Camarero (2021) demonstrated that severe drought in combination with elevated temperature likely triggered widespread forest dieback (a stand- level decline and gradual deterioration of tree health) and elevated mortality rates.
Typically, dying trees showed early-warning signals such as the following: shoot death, partial or complete leaf shedding, the production of epicormic shoots, the loss of fine roots (Camarero, 2021), a decline in the tree growth rate, reduced radical growth rate, and increased canopy defoliation from 1 year to several years before death, depending on the lifespan of the tree and the intensity of the drought (Leuschner, 2020). Three important mechanisms of drought-related forest dieback and mortality will be discussed below.
4.1 Hydraulic failure
The extreme drought might lead to a gradual decline in the hydraulic efficiency of the xylem by increasing water losses through the leaf, due to high cuticular permeability and a leaf-to-air vapor pressure deficit (Camarero, 2021), thereby initiating cavitation (nucleation of vaporization) and producing xylem embolism, i.e. air-filled conduit (McDowell et al., 2008). Such irreversible tree cavitation cause trees to die due to water cutoff before the non-structural carbohydrates (NSCs, soluble sugars, and sometimes starch) are fully consumed (He et al., 2020). Hydraulic failure also occurs within the soil-root system due to a reduction in soil moisture or fine root mortality, which may cause tree dehydration and death (Camarero, 2021).
Anderegg et al. (2012) suggested that the mortality of Populus tremuloides roots was a consequence of hydraulic failure in its roots and branches. In addition, a study provided evidence that the mortality of beech (F. sylvatica L.) and Populus deltoides L. was a result of xylem cavitation under extreme drought conditions (Barigah et al., 2013). Moreover, Brodribb et al. (2016) investigated embolism-induced hydraulic failure by using an optical technique to visualize the development of embolism in the leaf network.
4.2 Carbon starvation
Extended drought stress drives a carbohydrate deficit and cellular metabolic limitation that directly trigger mortality through carbon starvation (Senf et al., 2020) and a weakened defense against biotic agent attack (Leuschner, 2020). The process of carbon starvation occurs because the carbon supply from photosynthesis and the mobilization of carbon reserve autophagy is less than the carbon use by respiration, growth, and defense (McDowell, 2011). The process is initiated by stomatal closure, which reduces CO2 uptake and causes low photosynthetic activity, thereby reducing the CO2 assimilation rate (Allen et al., 2010). Consequently, trees are forced to mobilize stored carbon, i.e., NSCs, to maintain cellular metabolic demand, until carbon reserves are eventually depleted (He et al., 2020). The physiological response of carbon depletion is closely associated with carbon reserves, particularly in the root, which is a large sink for NSCs (Brunner et al., 2015). The disrupted cellular metabolism hinders the production and movement of non-structural carbohydrates (NSCs), which are essential for plant defense against biotic attacks (Allen et al., 2010) It also leads to increased emissions of volatile compounds, such as ethanol, that attract insects and changes the food quality for them (McDowell et al., 2008).
Carbon starvation has been detected as a reason for dying beech trees (F. sylvatica L.), and starch reserves in the inner annual rings are depleted (Leuschner, 2020). Under drought conditions, several beech stands in a German forest showed increased mortality due to bark infestation by the oomycetes Phythophora citricola and Phythophora cambivora (Leuschner, 2020). A reduction in the reserve accumulation in the root system was detected in a drought study of the two poplar species P. tremuloides and P. balsamifera (Brunner et al., 2015).
4.3 Biotic agents
Upon drought, biotic agents may amplify, or be amplified, by hydraulic failure and/or carbon starvation, which subsequently drive forest mortality (McDowell et al., 2008). Heat and drought in combination have an impact on the characteristics of the insect population. For example, warm droughts may increase the intensity of outbreaks of western spruce budworm as biotic mortality agents (McDowell et al., 2008). Several studies have been performed on drought- and infestation-triggered forest tree mortality as an emerging phenomenon affecting forests globally that may be linked to increasing temperatures and drought frequency and severity (Adams et al., 2012). Investigations on the effect of drought and topography on recent patterns of tree mortality in old-growth mixed conifer forests revealed that co-occurring periods of high spring and summer temperatures and low annual and seasonal precipitation triggered high tree mortality (Yuhas and Scuderi, 2009). In addition, Huang and Anderegg (2011) demonstrated that severe drought in combination with elevated temperature likely triggered the widespread forest mortality of trembling aspen (P. tremuloides).
Taken together, a longer drought duration increases tree mortality through carbon starvation, whereas more extreme drought causes tree mortality through hydraulic failure (McDowell et al., 2008).
5 Plant adaptation strategies to drought
Plants have evolved different drought-adaptive strategies that allow them to cope with drought stress for the benefit of their growth, development, and reproduction (Fang and Xiong, 2015). Plant drought adaptation is defined as the integrated capability of plants in both drought resistance and drought recovery for the adaptation to water-deficit stress and re-watering (Chen et al., 2016). Drought resistance can be further classified into three functional strategies: drought escape, drought avoidance, and drought tolerance (Bandurska, 2022). It refers to the ability of plants in natural vegetation to grow and activate appropriate coping strategies to ensure reproductive success when exposed to water deficits.
The adaptive responses to drought vary from the molecular level up to plant level, as shown in Figure 2. Many factors can affect the adaptive responses of drought-stressed plants, such as plant genotype, growth stage, the intensity and duration of stress (Oguz et al., 2022), and a combination of these factors with other environmental factors (Jaldhani et al., 2022). Table 2 shows that plant responses differed between different drought intensities.
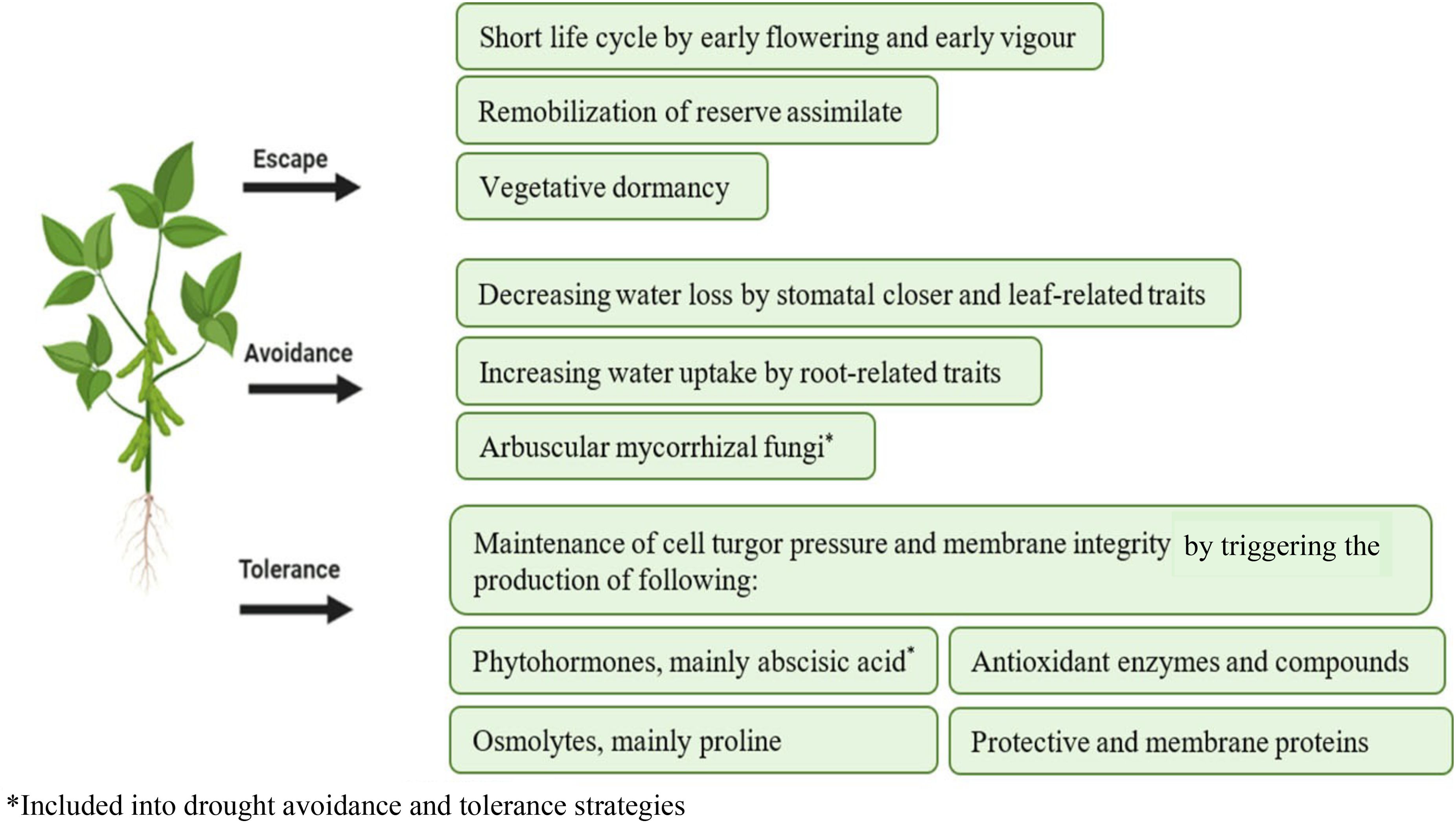
Figure 2. Plant strategies for drought resistance and their beneficial functions for plant survival and recovery: Escape, Avoidance and Tolerance.
5.1 Drought escape strategy
To escape the harmful effects of drought stress on plant productivity, plants shorten the growing season through rapid development during the vegetative stage, early flowering, and seed formation before the onset of drought stress (Seleiman et al., 2021). In this circumstance, the plant maximizes the metabolic pathways for fast growth and self-reproduction before the arrival of drought stress (Moradi, 2016). A better remobilization of NSC stored in the stems and leaves also plays an important role in mitigating the negative effects of drought during grain filling (Tuberosa, 2012).
Early vigor is another trait related to drought escape, allowing annual crops to optimize WUE and limit water loss via evaporation from the soil surface (Tuberosa, 2012) in spring drought and early summer drought (Bodner et al., 2015). It is also stated that vegetative dormancy may be considered an escape strategy in drought deciduous species (e.g. Cistus sp and Periploca sp.) and rhizomatous herbs (e.g. Brachypodium retusum and Stipa tenacissima) (Peguero-Pina et al., 2020). An escape strategy is a common feature in drought-sensitive plant species as native annuals that have a short life cycle with a high growth rate while moisture in the soil lasts (Chaves et al., 2003).
5.2 Drought avoidance strategy
In this strategy, plants avoid drought stress in plant cells through delaying or weakening that stress for a short period of time (Bandurska, 2022) by promoting water homeostasis in tissue (Polle et al., 2019) and the continuity of different physiological process (Ilyas et al., 2021). Mild drought induces a drought avoidance strategy in stressed plants, allowing the maintenance of their leaf relative water content within the limits in which the photosynthetic capacity shows no or little change (Manes et al., 2006). Plants can maintain a high water potential either by restricting water loss or increasing water absorption (Moradi, 2016). This strategy is commonly used in annuals and perennials and is associated with morphological and physiological alterations in leaf-related and root-related traits (Chaves et al., 2003).
The adaptive leaf-related traits involved in minimizing water loss are stomatal closure, fewer stomata, a thick cuticle, and a small stomatal aperture (Ilyas et al., 2021). Increased WUE is a beneficial adaptive trait in a drought avoidance strategy (Seleiman et al., 2021) that is achieved by increasing the accumulation of biomass (i.e., an optimization of carbon uptake) and decreasing evapotranspiration through stomatal closure (Jaldhani et al., 2022). Some plants reduce the canopy leaf surface area through the production of smaller leaves or shedding of older leaves (Chaves et al., 2003) to reduce canopy temperature and protect them from excess water loss (Fang and Xiong, 2015). This reduces absorbed solar radiation either through leaf rolling, an erect leaf angle (Chaves et al., 2003), a dense trichome layer, or leaf pubescence, allowing plants to decrease evapotranspiration under intense light conditions and increasing reflectance (Fang and Xiong, 2015). Epicuticular wax crystals promoting leaf glaucescence is considered an adaptive trait for coping with restricted water availability by increasing leaf reflectance (Peguero-Pina et al., 2020). Other leaf-associated traits, such as a thicker cuticle epidermis, thicker palisade tissue, higher ratio of palisade to spongy parenchyma thickness, and more developed epidermal vascular bundle sheath, help to maintain high water potentials in plant tissues (Fang and Xiong, 2015).
On the other hand, the adaptive trait that maximizes water uptake is associated with root-related traits, including long and deep roots, high density, thick roots, and high proliferation (Ilyas et al., 2021; Farooq et al., 2009). Moreover, the ability to increase root growth and the root:shoot ratio has been observed in some plants under drought stress to capture adequate subsoil water (Fang and Xiong, 2015), and this feature is mediated through the accumulation of ABA in the roots (Tuberosa, 2012). In addition, the inhibition of lateral roots is considered a drought-adaptive response and promotes the growth of the primary root, enabling the extraction of water from deeper soil layers (Bhargava and Sawant, 2013). Plant-associated mycorrhizal fungi (AMF) contribute to the drought avoidance strategy by increasing water uptake surface and also penetrate fine soil pores that are inaccessible to root hairs, which helps to redistribute water from wetter to drier soil patches via fungal hyphae (Poudel et al., 2021). These drought- adaptive responses of AMF have been documented in different trees, such as trifoliate orange (Citrus trifoliata), black locust (Robinia pseudoacacia), apple (M. hupehensis), and carob (Ceratonia siliqua L.), and agricultural crops, such as wheat (T. durum), chickpea (C. arietinum L.), maize, rice, and tomato (Mathur and Roy, 2021; Haider et al., 2020).
5.3 Drought tolerance strategy
Under severe and long-term drought, some plants induce drought tolerance as the final strategy to survive and alleviate drought damage (Bandurska, 2022) during the reproductive and vegetative phases (Moradi, 2016). This refers to the ability of plants to tolerate (resist) cellular damage by drought to maintain a certain level of their function even with a low tissue water potential (Fang and Xiong, 2015). This strategy is regulated by ABA-dependent and -independent pathways and includes thousands of genes involved in maintaining cell turgor pressure and membrane integrity (Bandurska, 2022). ABA is a major endogenous phytohormone that plays a pivotal role in the drought tolerance strategy (Ahmad et al., 2023). In addition, osmotic adjustment has been considered an important part of drought tolerance, including the synthesis and accumulation of different compatible compounds (e.g., proline, glycine-betaine, raffinose mannitol, and pinitol) under drought (Bandurska, 2022). Remarkably, it was found that osmotic adjustment is a species-specific feature, in which different plants accumulate different osmolytes. For example, increased proline is a common adaptive response for a wide range of drought-stressed plants, whereas glycine betaine is produced by certain plant species subjected to drought such as sugar beet (Beta vulgaris), spinach (Spinacia oleracea), and barley (Hordeum vulgare) (Osmolovskaya et al., 2018). The biosynthesis of protective proteins (e.g., LEA proteins, dehydrins, and chaperons) is another drought-tolerant trait that is involved in enzyme and membrane protection (Bandurska, 2022). Of these, LEA plays a central role in drought tolerance due to its high hydrophilicity. It was documented that LEA prevents the mechanical damage of cellular structures such as mitochondria and chloroplasts by forming a membrane-protecting shield, thus preventing the peroxidation of membrane lipids (Osmolovskaya et al., 2018). To protect cells against the hazardous effects of excessive ROS, plants have evolved a series of sophisticated enzymatic and non-enzymatic antioxidant systems (Bandurska, 2022). It was stated that AMF contributes to the drought tolerance strategy through enhanced osmotic adjustment, antioxidants such as carotenoids and glutathione, and the increased expression of the aquaporin gene and other drought-responsive genes (Poudel et al., 2021; Haider et al., 2020).
5.4 Drought recovery strategy
The ability of plants to resume growth, repair physiological activity (Bandurska, 2022), and overcome yield reduction after drought stress termination is defined as drought recovery (Fang and Xiong, 2015). It was believed that drought recovery plays an essential role in plant drought adaptation, along with drought resistance in herbaceous crops (Chen et al., 2016) and woody plants (Leuschner, 2020). A full or partial recovery depends on the severity and duration of drought as well as the tree species. It has been suggested that recovery after mild drought occurs through two mechanisms, if there is not a high level of cavitation. These mechanisms are the regrowth of the xylem or refilling embolized xylem conduits. The latter is well-documented in herbaceous species such as maize (Z. mays L.) (Choat et al., 2018).
It is important to empathize that plants may combine two or more strategies to grow and flower satisfactory under drought stress. Increasing our knowledge of plant adaptation under drought stress can be a crucial issue in the improvement of new methods for increasing drought resistance in herbaceous and woody plants.
6 Drought-tolerance induction
In addition to plant breeding and transgenic approaches, there are different agronomic practices that can potentially alleviate the deleterious effects of drought stress, including soil management and culture practices, irrigation, crop residues and mulching, and a selection of drought-tolerant varieties (Lamaoui et al., 2018). The application of specific compounds has drawn a great deal of attention for improving drought tolerance in plants. These compounds can be used as a foliar application (spraying or fumigating) at different growth stages of established crops, or as a seed treatment (Seleiman et al., 2021).
6.1 Nutrient and mineral application
Although several studies have revealed that fertilizers have no significant effect on drought stress as water is critical for the mobility and metabolism of these nutrients (Lamaoui et al., 2018), other studies highlighted the promising results of using N, P, S, K, selenium (SE), and boron (B) to alleviate drought stress in different crops (Seleiman et al., 2021; Zia et al., 2021; Das et al., 2020). An adequate N supply in poplar (Populus alba x P. glandulosa) improved drought tolerance through the regulation of root water uptake and increased WUE and growth performance (Song et al., 2019a). A study on maize seedlings indicated that adequate N application under certain drought stress increased the activity of antioxidant enzymes and chlorophyll content in leaves to alleviate the damage caused by drought stress (Song et al., 2019b). Si-fortified fertilizer is a beneficial nutrient that mitigates the harmful effects of drought stress in monocot or dicot plants (Das et al., 2020). The foliar application of B improves the capacity of wheat to tolerate stress and enhances growth parameters (Zia et al., 2021).
6.2 Phytohormone application
Evidence shows that synthetic phytohormone treatment improves plant growth against stress and increases the drought tolerance capability of plants (Seleiman et al., 2021). For example, the application of ABA and salicylic acid has been reported to improve drought tolerance by strengthening antioxidant enzyme activity. In addition, ABA treatment helps the marketability of horticultural crops by reducing drought-induced wilting symptoms (Jaldhani et al., 2022). A foliar gibberellic acid spray enhanced the photosynthetic machinery and stomatal conductance in cotton, wheat, and maize plants (Seleiman et al., 2021) and promoted pollen and seed cone production in Sitka spruce (Picea sitchensis) under drought stress (Farooq et al., 2009).
6.3 Osmolyte application
The application of proline to leaves increased the endogenous free proline content in onion (Allium cepa L.), which improved osmotic adjustment and protected the subcellular structure (Seleiman et al., 2021), and improved the quality and yield of maize (Ilyas et al., 2021). In addition, the application of glycine betaine on wheat leaves increased the leaf water potential and activity of antioxidant (Ilyas et al., 2021).
6.4 Plant growth-promoting microorganism application
The application of plant growth-promoting microorganisms is a promising eco-friendly approach to alleviate drought stress in many herbaceous and woody plants. It consists of numerous beneficial microorganisms that live on the planet, such as plant growth-promoting rhizobacteria, endophytes, and AMF (Mathur and Roy, 2021). Generally, these rhizospheric microbes function as biocontrol agents (pathogens) and plant growth promoting substances and enhance soil fertility and structure. In addition, they assist plant growth and development under drought stress (Ilyas et al., 2021). Although many microbes have shown beneficial results in drought tolerance in inoculated plants by enhancing the production of phytohormones, antioxidants, and osmolytes, the development of commercial biofertilizers containing drought-tolerant microbes needs further investigation.
Continuous research is being carried out on the formulation of biofertilizers that should contain selected microorganisms with the desired metabolic active and long shelf life and are easily degradable in soils (Mathur and Roy, 2021).
7 Conclusion
Plants manage the negative effects of drought on growth and productivity through a complex set of related mechanisms. Stomatal closure is the first plant response to drought, followed by morphological modifications in leaf- and root-related traits under mild stress. Metabolic limitations impair photosynthesis, and hydraulic failure causes tree mortality under severe drought. The ongoing advancements in the field of biotechnology hold great expectations for the improvement of plant tolerance to drought via breeding and the expression of stress-induced genes. In parallel, innovative breeding programs could also assist in the propagation of plants with desired genetic traits that are capable of withstanding harsh conditions. Undoubtedly, more studies are required to characterize the adaptive traits, e.g., resistance to chlorophyll degradation, antioxidant compounds, and proline content, that increase plant tolerance to drought. Although the use of specific compounds to mitigate the devastating effects of the upcoming drought is promising, there is a need for continuous research to visualize their benefits in a range of plant species and determine the suitable concentration at which to apply them at the critical growth stage or seed treatment.
Furthermore, modern day agronomists have been working extensively on developing crop varieties that can withstand drought conditions, a critical challenge due to climate change and water scarcity. The development of drought-resilient crops through these approaches has revolutionized agriculture, particularly in regions disposed to water scarcity. Native traits provide a foundation for breeding programs that can be accelerated using molecular markers and genomic selection. In conclusion, a combination of these strategies, tailored to specific environmental conditions and societal needs, is likely to be the most effective way of developing crops that can thrive in a world where droughts are becoming more frequent and severe.
Author contributions
MN: Writing – original draft, Writing – review & editing. HA: Writing – original draft, Writing – review & editing, Investigation. MA: Writing – original draft, Writing – review & editing. AA: Writing – original draft. BH: Writing – review & editing. AM: Writing – original draft, Conceptualization, Data curation, Writing – review & editing.
Funding
The author(s) declare that no financial support was received for the research, authorship, and/or publication of this article.
Conflict of interest
The authors declare that the research was conducted in the absence of any commercial or financial relationships that could be construed as a potential conflict of interest.
Publisher’s note
All claims expressed in this article are solely those of the authors and do not necessarily represent those of their affiliated organizations, or those of the publisher, the editors and the reviewers. Any product that may be evaluated in this article, or claim that may be made by its manufacturer, is not guaranteed or endorsed by the publisher.
References
Adams H. D., Luce C. H., Breshears D. D., Allen C. D., Weiler M., Hale V. C., et al. (2012). Ecohydrological consequences of drought- and infestation-triggered tree die-off: insights and hypotheses. Ecohydrology 5, 145–159. doi: 10.1002/eco.233
Aghaie P., Tafreshi S. A. H., Ebrahimi M. A., Haerinasab M. (2018). Tolerance evaluation and clustering of fourteen tomato cultivars grown under mild and severe drought conditions. Scientia Horticulturaea 232, 1–12. doi: 10.1016/j.scienta.2017.12.041
Ahmad S., Belwal V., Punia S. S., Ram M., Dalip, Rajput S. S., et al. (2023). Role of plant secondary metabolites and phytohormones in drought tolerance: A review. Gesunde Pflanzen 75, 1–18. doi: 10.1007/s10343-022-00795-z
Allen C. D., Macalady A. K., Chenchouni H., Bachelet D., McDowell N., Vennetier M., et al. (2010). A global overview of drought and heat-induced tree mortality reveals emerging climate change risks for forests. For. Ecol. Manage. 259, 660–684. doi: 10.1016/j.foreco.2009.09.001
Anderegg W. R. L., Berry J. A., Smith D. D., Sperry J. S., Anderegg L. D. L., Field C. B. (2012). The roles of hydraulic and carbon stress in a widespread climate-induced forest die-off. Proc. Natl. Acad. Sci. 109, 233–237. doi: 10.1073/pnas.1107891109
Ansari W. A., Atri N., Pandey M., Singh A. K., Singh B., Pandey S. (2019). Influence of drought stress on morphological, physiological and biochemical attributes of plants: A review. Biosci. Biotechnol. Res. Asia 16, 697–709. doi: 10.13005/bbra/2785
Aref I. M., El Atta H. A., Alshahrani T., Alazba A., Ahmad A. I. (2013). Evaluation of the physiological and growth response of Juniperus procera Hochst. Ex Endlicher to some types of microcatchments. Int. J. Plant Anim. Environ. Sci. 3, 234–241.
Azhar N., Hussain B., Ashraf M. Y., Abbasi K. Y. (2011). Water stress mediated changes in growth, physiology and secondary metabolites of desi ajwain (Trachyspermum ammi L.). Pakistanian J. Bot. 43, 15–19.
Azzeme A. M., Abdullah S. N. A., Aziz M. A., Wahab P. E. M. (2016). Oil palm leaves and roots differ in physiological response, antioxidant enzyme activities and expression of stress-responsive genes upon exposure to drought stress. Acta physiologiae plantarum 38, 1–12. doi: 10.1007/s11738-016-2073-2
Bandurska H. (2022). Drought stress responses: Coping strategy and resistance. Plants 11, 922. doi: 10.3390/plants11070922
Barigah T. S., Chareier O., Douris M., Bonhomme M., Herbette S., Ameglio T., et al. (2013). Water stress-induced xylem hydraulic failure is a causal factor of tree mortality in beech and poplar. Ann. Bot. 112, 1431–1437. doi: 10.1093/aob/mct204
Bhargava S., Sawant K. (2013). Drought stress adaptation: metabolic adjustment and regulation of gene expression. Plant Breed. 132, 21–32. doi: 10.1111/pbr.12004
Bodner G., Nakhforoosh A., Kaul H. P. (2015). Management of crop water under drought: a review. Agron. Sustain. Dev. 35, 401–442. doi: 10.1007/s13593-015-0283-4
Bota J., Medrano H., Flexas J. (2004). Is photosynthesis limited by decreased Rubisco activity and RuBP content under progressive water stress? New Phytol. 162, 671–681. doi: 10.1111/j.1469-8137.2004.01056.x
Brito C., Dinis L. T., Moutinho-Pereira J., Correia C. M. (2019). Drought stress effects and olive tree acclimation under a changing climate. Plants 8, 232. doi: 10.3390/plants8070232
Brodribb T. J., Bienaime D., Marmottant P. (2016). Revealing catastrophic failure of leaf network under stress. PNAS 113, 4865–4869. doi: 10.1073/pnas.1522569113
Brunner I., Herzog C., Dawes M. A., Arend M., Sperisen C. (2015). How tree roots respond to drought. Front. Plant Sci. 6, 547. doi: 10.3389/fpls.2015.00547
Camarero J. J. (2021). The drought−dieback−death conundrum in trees and forests. Plant Ecol. Diversity 14, 1–12. doi: 10.1080/17550874.2021.1961172
Cano F. J., Sanchez-Gomez D., Rodriguez-Calcerrada J., Warren C. R., Gil L., Aranda I. (2013). Effects of drought on mesophyll conductance and photosynthetic limitations at different tree canopy layers. Plant Cell Environ. 36, 1961–1980. doi: 10.1111/pce.12103
Carraro E., Di Iorio A. (2022). Eligible strategies of drought response to improve drought resistance in woody crops: a mini-review. Plant Biotechnol. Rep. 16, 265–282. doi: 10.1007/s11816-021-00733-x
Chaves M. M., Maroco J. P., Pereira J. S. (2003). Understanding plant responses to drought: from genes to the whole plant. Funct. Plant Biol. 30, 239–264. doi: 10.1071/fp02076
Chen D., Wang S., Cao B., Cao D., Leng G., Li H., et al. (2016). Genotypic variation in growth and physiological response to drought stress and re-watering reveals the critical role of recovery in drought adaptation in maize seedlings. Front. Plant Sci. 6, 1241. doi: 10.3389/fpls.2015.01241
Choat B., Brodribb T. J., Broderson C. R., Duursma R. A., Lopez R., Medlyn B. (2018). Triggers of tree mortality under drought. Nature 558, 1–9. doi: 10.1038/s41586-018-0240-x
Cruz de Carvalho M. H. (2008). Drought stress and reactive oxygen species: Production, scavenging and signaling. Plant Signal Behav. 3, 156–165. doi: 10.4161/psb.3.3.5536
Dang Q.-L., Margolis H. A., Coyea M. R., M S. Y., Collaz G. J. (1997). Regulation of branch-level gas exchange of boreal trees: roles of shoot water potential and vapour pressure difference. Tree Physiol. 17, 521–535. doi: 10.1093/treephys/17.8-9.521
Das S., Rawat P., Shankhdhar D., Shankhdhar S. C. (2020). “Drought stress: an impact of climate change, its consequences and amelioration through silicon (Si),” in Sustainable Agriculture in the Era of Climate Change (Springer, Cham), 169–185.
Delatorre J., Pinto M., Cardemil L. (2008). Effects of water stress and high temperature on photosynthetic rates of two species of Prosopis. J. Photochem. Photobiol. B: Biol. 92, 67–76. doi: 10.1016/j.jphotobiol.2008.04.004
Dias M. C., Brueggemann W. (2010). Limitation of photosynthesis in Phaseolus vulgaris under drought stress: gas exchange, chlorophyll fluorescence and Calvin cycle enzymes. Photosynthetica 48, 96–102. doi: 10.1007/s11099-010-0013-8
Dietz K. J., Zörb C., Geilfus C. M. (2021). Drought and crop yield. Plant Biol. 23, 881–893. doi: 10.1111/plb.13304
Din J., Khan S. U., Ali I., Gurmani A. R. (2011). Physiological and agronomic response of canola varieties to drought stress. J. Anim. Plant Scince. 21, 78–82.
Fahad S., Bajwa A. A., Nazir U., Anjum S. A., Farooq A., Zohaib A., et al. (2017). Crop production under drought and heat stress: plant responses and management options. Front. Plant Sci. 8, 1–16. doi: 10.3389/fpls.2017.01147
Fang Y., Xiong L. (2015). General mechanisms of drought response and their application in drought resistance improvement in plants. Cell. Mol. Life Sci. 72, 673–689. doi: 10.1007/s00018-014-1767-0
Farooq M., Wahid A., Kobayashi N., Fujita D., Basra S. M. A. (2009). Plant drought stress: effects, mechanisms and management. Agron. Sustain. Dev. 29, 185–212. doi: 10.1051/agro:2008021
Fidler J., Graska J., Gietler M., Nykiel M., Prabucka B., Rybarczyk-Płońska A., et al. (2022). PYR/PYL/RCAR receptors play a vital role in the abscisic-acid-dependent responses of plants to external or internal stimuli. Cells 11, 1352. doi: 10.3390/cells11081352
Foyer C. H., Descourvieres P., Kunert K. J. (1994). Photo oxidative stress in plants. Plant Physiol. 92, 696–717. doi: 10.1111/j.1399-3054.1994.tb03042.x
Foyer C. H., Noctor G. (2005). Redox homeostasis and antioxidant signalling: A metabolic interface between stress perception and physiological responses. Plant Cell. 17, 1866–1875. doi: 10.1105/tpc.105.033589
Galeano E., Vasconcelos T. S., Novais de Oliveira P., Carrer H. (2019). Physiological and molecular responses to drought stress in teak (Tectona grandis L.f.). PLoS One 14, 1–26. doi: 10.1371/journal.pone.0221571
Gomes M. M. A., Lagoa A. M. M. A., Medina C. L., MaChado E. C., MaChado M. A. (2004). Interaction between leaf water potential, stomatal conductance and abscisic acid content of orange trees submitted to drought stress. Braz. J. Plant Physiol. 16, 155–161. doi: 10.1590/s1677-04202004000300005
Grzesiak M. T., Grzesiak S., Skoczowski A. (2006). Changes in leaf water potential and gas exchange during and after drought in triticale and maize genotypes differing in drought tolerance. Photosynthetica 44, 561–568. doi: 10.1007/s11099-006-0072-z
Haider M. Z., Saeed F., Ali A., Ali Q., Habib N., Javed M. T., et al. (2020). “Involvement of microbes in different abiotic stress environments of cropping lands,” in Sustainable Agriculture in the Era of Climate Change (Springer, Cham), 441–479.
Hassan I. A. (2006). Effects of water stress and high temperature on gas exchange and chlorophyll fluorescence in Triticum aestivum L. Photosynthetica 4, 312–315. doi: 10.1007/s11099-006-0024-7
He W., Liu H., Qi Y., Liu F., Zhu X. (2020). Patterns in nonstructural carbohydrate contents at the tree organ level in response to drought duration. Global Change Biol. 26, 3627–3638. doi: 10.1111/gcb.15078
Huang C. Y., Anderegg W. R. L. (2011). Large drought-induced aboveground live biomass losses in southern Rocky mountain aspen forests. Global Change Biol. 18, 1016–1027. doi: 10.1111/j.1365-2486.2011.02592.x
Hunt J. E. R., Lavigne M. B., Franklin S. E. (1999). Factors controlling the decline of net primary production with stand age for balsam fir in Newfoundland assessed using an ecosystem simulation model. Ecol. Model. 122, 151–164. doi: 10.1016/s0304-3800(99)00135-0
Ilyas M., Nisar M., Khan N., Hazrat A., Khan A. H., Hayat K., et al. (2021). Drought tolerance strategies in plants: a mechanistic approach. J. Plant Growth Regul. 40, 926–944. doi: 10.1007/s00344-020-10174-5
Iqbal S., Wang X., Mubeen I., Kamran M., Kanwal I., Díaz G. A., et al. (2022). Phytohormones trigger drought tolerance in crop plants: outlook and future perspectives. Front. Plant Sci. 12, 1–14. doi: 10.3389/fpls.2021.799318
Jaldhani V., Rao D. S., Beulah P., Nagaraju P., Suneetha K., Veronica N., et al. (2022). “Drought and heat stress combination in a changing climate,” in Climate Change and Crop Stress (Academic press, An Imprint of Elsevier Science (USA)), 33–70.
Kamanga R. M., Mbega E., Ndakidemi P. (2018). Drought tolerance mechanisms in plants: physiological responses associated with water deficit stress in Solanum lycopersicum. Adv. Crop Sci. Technol. 6, 1–9. doi: 10.4172/2329-8863.1000362
Kaur G., Asthir B. (2017). Molecular responses to drought stress in plants. Biol. Plantarum 61, 201–209. doi: 10.1007/s10535-016-0700-9
Kitao M., Lei T. T., Koike T., Tobita H., Maruyama Y. (2003). Higher electron transport rate observed at low intercellular CO2 concentration in long-term drought-acclimated leaves of Japanese mountain birch (Betula ermanii). Physiologia Plantarum 118, 406–413. doi: 10.1034/j.1399-3054.2003.00120.x
Kolb T. E., Stone J. E. (2000). Differences in leaf gas exchange and water relations among species and tree sizes in an Arizona pine–oak forest. Tree Physiol. 20, 1–12. doi: 10.1093/treephys/20.1.1
Kramer P. J., Boyer J. S. (1995). Water Relations of Plants and Soils (Academic press, Alsevier Science (USA).
Lamaoui M., Jemo M., Datla R., Bekkaoui F. (2018). Heat and drought stresses in crops and approaches for their mitigation. Front. Chem. 6, 1–14. doi: 10.3389/fchem.2018.00026
Leuschner C. (2020). Drought response of European beech (Fagus sylvatica L.)—A review. Perspect. Plant Ecology Evol. Systematics 47, 125576. doi: 10.1016/j.ppees.2020.125576
Li S., Li M., Zhang W., Li Y., Ai X., Liu B., et al. (2019). Effects of CO2 enrichment on photosynthetic characteristics and reactive oxygen species metabolism in leaves of cucumber seedlings under salt stress. Acta Ecologia Sin. 39, 1–9. doi: 10.1002/ece3.333
Lindermayr C., Durner J. (2015). Interplay of reactive oxygen species and nitric oxide: Nitric oxide coordinates reactive oxygen species homeostasis. Plant Physiol. 167, 1209–1210. doi: 10.1104/pp.15.00293
Manes F., Vitale M., Donato E., Giannini M., Puppi G. (2006). Different ability of three Mediterranean oak species to tolerate progressive water stress. Photosynthertica 44, 387–393. doi: 10.1007/s11099-006-0040-7
Maqbool M. A., Aslam M., Ali H. (2017). Breeding for improved drought tolerance in Chickpea (Cicer arietinum L.). Plant Breed 136, 300–318. doi: 10.1111/pbr.12477
Mathur P., Roy S. (2021). Insights into the plant responses to drought and decoding the potential of root associated microbiome for inducing drought tolerance. Physiologia Plantarum 172, 1016–1029. doi: 10.1111/ppl.13338
McDowell N. G. (2011). Mechanisms linking drought, hydraulics, carbon metabolism, and vegetation mortality. Plant Physiol. 155, 1051–1059. doi: 10.1104/pp.110.170704
McDowell N., Pockman W. T., Allen C. D., Breshears D. D., Cobb N., Kolb T., et al. (2008). Mechanisms of plant survival and mortality during drought: why do some plants survive while others succumb to drought? New Phytol. 178, 719–739. doi: 10.1111/j.1469-8137.2008.02436.x
Moradi P. (2016). Key plant products and common mechanisms utilized by plants in water deficit stress responses. Botanical Sci. 94, 657–671. doi: 10.17129/botsci.709
Moran J. F., Becana M., Iturbe-Ormaetxe I., Frechilla S., Klucas R. V., Aparicio-Trejo P. (1994). Drought induces oxidative stress in pea plants. Planta 194, 346–352. doi: 10.1007/bf00197534
Nerd A., Nobel P. S. (1991). Effects of drought on water relations and nonstructural carbohydrates in cladodes of Opuntia ficus-indica, Physiol. Plant 81, 495–500. doi: 10.1034/j.1399-3054.1991.810408.x
Nezhadahmadi A., Prodhan Z. H., Faruq G. (2013). Drought tolerance in wheat. Sci. World J. 2013, 1–12. doi: 10.1155/2013/610721
Oguz M. C., Aycan M., Oguz E., Poyraz I., Yildiz M. (2022). Drought stress tolerance in plants: interplay of molecular, biochemical and physiological responses in important development stages. Physiologia 2, 180–197. doi: 10.3390/physiologia2040015
Ohashi Y., Nakayama N., Saneoka H., Fujita K. (2006). Effects of drought stress on photosynthetic gas exchange, chlorophyll fluorescence and stem diameter of soybean plants. Biol. Plantarum 50, 138–141. doi: 10.1007/s10535-005-0089-3
Osmolovskaya N., Shumilina J., Kim A., Didio A., Grishina T., Bilova T., et al. (2018). Methodology of drought stress research: Experimental setup and physiological characterization. Int. J. Mol. Sci. 19, 1–25. doi: 10.3390/ijms19124089
Parry M. A. J., Andralojc P. J., Khan S., Lea P. J., Keys A. J. (2002). Rubisco activity: Effects of drought stress. Ann. Bot. 89, 833–839. doi: 10.1093/aob/mcf103
Patanè C., Cosentino S. L., Romano D., Toscano S. (2022). Relative water content, proline, and antioxidant enzymes in leaves of long shelf-life tomatoes under drought stress and rewatering. Plants 11, 30–45. doi: 10.3390/plants11223045
Peguero-Pina J. J., Vilagrosa A., Alonso-Forn D., Ferrio J. P., Sancho-Knapik D., Gil-Pelegrín E. (2020). Living in drylands: Functional adaptations of trees and shrubs to cope with high temperatures and water scarcity. Forests 11, 1–23. doi: 10.3390/f11101028
Peuke A. D., Rennenberg H. (2004). Carbon, nitrogen, phosphorus, and sulphur concentration and partitioning in beech ecotypes (Fagus sylvatica L.): phosphorus most affected by drought. Trees 18, 639–648. doi: 10.1007/s00468-004-0335-x
Ping M. A., Bai T. H. (2015). Effects of progressive drought on photosynthesis and partitioning of absorbed light in apple trees. J. Integr. Agric. 14, 681–690. doi: 10.1016/S2095-3119(14)60871-6
Polle A., Chen S. L., Eckert C., Harfouche A. (2019). Engineering drought resistance in forest trees. Front. Plant Sci. 9, 1–18. doi: 10.3389/fpls.2018.01875
Poudel M., Mendes R., Costa L. A., Bueno C. G., Meng Y., Folimonova S. Y., et al. (2021). The role of plant-associated bacteria, fungi, and viruses in drought stress mitigation. Front. Microbiol. 12, 1–21. doi: 10.3389/fmicb.2021.743512
Poudel S., Vennam R. R., Shrestha A., Reddy K. R., Wijewardane N. K., Reddy K. N., et al. (2023). Resilience of soybean cultivars to drought stress during flowering and early-seed setting stages. Sci. Rep. Switzerland AG: Springer Nature. 13, 1277. doi: 10.1038/s41598-023-28354-0
Rai A. C., Rai K. R. (2020). “Drought stress and its mitigation and management strategies in crop plants,” in Sustainable Agriculture in the Era of Climate Change, 143–168.
Schiop S. T., Al Hassan M., Sestras A. F., Boscaiu M., Sestras R. E., Vicente O. (2017). Biochemical responses to drought, at the seedling stage, of several Romanian Carpathian populations of Norway spruce (Picea abies L. Karst). Trees 31, 1479–1490. doi: 10.1007/s00468-017-1563-1
Schmitt K. F. M., do Amaral Junior A. T., Kamphorst S. H., Pinto V. B., de Lima V. J., de Oliveira U. A., et al. (2024). Decoding the effects of drought stress on popcorn (Zea mays var. everta) flowering combining proteomics and physiological analysis. Plant Physiol. Biochem. 208, 108444. doi: 10.1016/j.plaphy.2024.108444
Schulte M., Herschbach C., Rennenberg H. (1998). Interactive effects of elevated atmospheric CO 2, mycorrhization and drought on long-distance transport of reduced sulphur in young pedunculate oak trees (Quercus robur L.). Plant Cell Environ. 21, 917–926. doi: 10.1046/j.1365-3040.1998.00337.x
Seleiman M. F., Al-Suhaibani N., Ali N., Akmal M., Alotaibi M., Refay Y., et al. (2021). Drought stress impacts on plants and different approaches to alleviate its adverse effects. Plants 10, 1–25. doi: 10.3390/plants10020259
Senf C., Buras A., Zang C. S., Rammig A., Seidl R. (2020). Excess forest mortality is consistently linked to drought across Europe. Nat. Commun. 11, 1–8. doi: 10.1038/s41467-020-19924-1
Song Y., Li J., Liu M., Meng Z., Liu K., Sui N. (2019b). Nitrogen increases drought tolerance in maize seedlings. Funct. Plant Biol. 46, 350–359. doi: 10.1071/fp18186
Song J., Wang Y., Pan Y., Pang J., Zhang X., Fan J., et al. (2019a). The influence of nitrogen availability on anatomical and physiological responses of Populus alba x P. glandulosa to drought stress. BMC Plant Biol. 19, 1–12. doi: 10.1186/s12870-019-1667-4
Subrahmanyam D., Subash N., Haris A., Sikka A. K. (2006). Influence of water stress on leaf photosynthetic characteristics in wheat cultivars differing in their susceptibility to drought. Photosynthetica 44, 125–129. doi: 10.1007/s11099-005-0167-y
Tenorio Berrío R., Nelissen H., Inzé D., Dubois M. (2022). Increasing yield on dry fields: molecular pathways with growing potential. Plant J. 109, 323–341. doi: 10.1111/tpj.15550
Tuberosa R. (2012). Phenotyping for drought tolerance of crops in the genomics era. Front. Physiol. 3, 347. doi: 10.3389/fphys.2012.00347
Verslues P. E., Sharp R. E. (1999). Proline accumulation in maize (Zea mays L.) primary roots at low water potentials. II. Metabolic source of increased proline deposition in the elongation zone. Plant Physiol. 119, 1349–1360. doi: 10.1104/pp.119.4.1349
Wach D., Skowron P. (2022). An overview of plant responses to the drought stress at morphological, physiological and biochemical levels. Polish J. Agron. 50, 25–34. doi: 10.26114/pja.iung.435.2022.04
Wang K., Kellomaeke S., Laitinen K. (1995). Effects of needle age, long-term temperature and CO2 treatments on the photosynthesis of Scots pine. Tree Physiol. 15, 211–218. doi: 10.1093/treephys/15.4.211
Wang Z., Yang Y., Yadav V., Zhao W., He Y., Zhang X., et al. (2022). Drought-induced proline is mainly synthesized in leaves and transported to roots in watermelon under water deficit. Hortic. Plant J. 8, 615–626. doi: 10.1016/j.hpj.2022.06.009
Wani K. I., Naeem M., Castroverde C. D. M., Kalaji H. M., Albaqami M., Aftab T. (2021). Molecular mechanisms of nitric oxide (NO) signaling and reactive oxygen species (ROS) homeostasis during abiotic stresses in plants. Int. J. Mol. Sci. 22, 9656. doi: 10.3390/ijms22179656
Yang X., Lu M., Wang Y., Wang Y., Liu Z., Chen S. (2021). Response mechanism of plants to drought stress. Horticulturae 7, 1–36. doi: 10.3390/horticulturae7030050
Yang X., Wang B., Chen L., Li P., Cao C. (2019). The different influences of drought stress at the flowering stage on rice physiological traits, grain yield, and quality. Sci. Rep. 9, 3742. doi: 10.1038/s41598-019-40161-0
Yuhas A. N., Scuderi L. A. (2009). MODIS-derived NDVI characterization of drought-induced evergreen die-off in western north America. Geographical Res. 47, 34–45. doi: 10.1111/j.1745-5871.2008.00557.x
Zargar M., Mahajan R., Malik F. A., Sofi N. R., Shikari A. B., Salgotra R. K. (2017). Impact of drought on photosynthesis: Molecular perspective. Plant Gene 11, 154–159. doi: 10.1016/j.plgene.2017.04.003
Keywords: drought stress, drought-adaptive strategies, drought-responses mechanisms, tree mortality, crop adaptation
Citation: Nour MM, Aljabi HR, AL-Huqail AA, Horneburg B, Mohammed AE and Alotaibi MO (2024) Drought responses and adaptation in plants differing in life-form. Front. Ecol. Evol. 12:1452427. doi: 10.3389/fevo.2024.1452427
Received: 20 June 2024; Accepted: 30 September 2024;
Published: 21 November 2024.
Edited by:
Giovanna Battipaglia, University of Campania Luigi Vanvitelli, ItalyReviewed by:
Soham Sengupta, St. Jude Children’s Research Hospital, United StatesDevasantosh Mohanty, University of Missouri, United States
Copyright © 2024 Nour, Aljabi, AL-Huqail, Horneburg, Mohammed and Alotaibi. This is an open-access article distributed under the terms of the Creative Commons Attribution License (CC BY). The use, distribution or reproduction in other forums is permitted, provided the original author(s) and the copyright owner(s) are credited and that the original publication in this journal is cited, in accordance with accepted academic practice. No use, distribution or reproduction is permitted which does not comply with these terms.
*Correspondence: Modhi O. Alotaibi, bW91b3RhZWJlQHBudS5lZHUuc2E=
†These authors have contributed equally to this work
‡ORCID: Arwa Alhugail, orcid.org/0000-0002-1226-499X
Afrah E. Mohammed, orcid.org/0000-0003-3294-8560
Modhi O. Alotaibi, orcid.org/0000-0002-6604-1652