- 1Las Palmeras, Instituto Milenio Biodiversidad de Ecosistemas Antárticos y Subantárticos (Mi-BASE), Ñuñoa, Santiago, Chile
- 2Facultad de Ciencias, Programa de Doctorado en Ciencias con mención en Biodiversidad y Biorecursos, Universidad Católica de la Santísima Concepción, Concepción, Chile
- 3Laboratorio de Genómica y Ecología Molecular Antártica y sub-Antártica (LAGEMAS), Instituto de Ciencias Marinas y Limnológicas (ICML), Universidad Austral de Chile, Valdivia, Chile
- 4Doctorado en Biología Marina, Instituto de Ciencias Marinas y Limnológicas (ICML), Universidad Austral de Chile, Valdivia, Chile
- 5Biogeosciences UMR 6282 CNRS, Université de Bourgogne, EPHE, Dijon, France
- 6Departamento de Ecología, Facultad de Ciencias, Universidad de Chile, Santiago, Chile
- 7Instituto Milenio en Socio-Ecología Costera (SECOS), Universidad Católica del Norte, Facultad de Ciencias del Mar, Departamento de Biología Marina, Coquimbo, Chile
- 8Universidad Austral de Chile, Centro Fondap de Investigación de Ecosistemas Marinos de Altas Latitudes (IDEAL), Valdivia, Chile
Rafting has been proposed as an effective mechanism for species without free-living pelagic larvae to achieve long-distance dispersal, theoretically preventing population differentiation over wide distributional ranges. Moreover, rafting has been advocated as a main dispersal mechanism for marine invertebrates with sub-Antarctic distributions, because of abundant buoyant kelps, driven by the Antarctic Circumpolar Current. Nonetheless, little attention has been given to the role of rafting to establish regular gene flow across the sub-Antarctic, and the geographic and temporal scales at which it occurs. Aiming to unravel these major questions about the extent of genetic connectivity across the Southern Ocean (SO), we studied the pulmonate limpet Siphonaria lateralis, a benthic species with encapsulated larvae, found on the rocky intertidal of sub-Antarctic islands and southern South America. Since S. lateralis is closely associated with D. antarctica, dispersal by rafting is plausible, as revealed by the absence of phylogeographic structure across the sub-Antarctic. We sampled 116 individuals from eight localities across the SO, and used 5,515 SNPs obtained through Genotyping-by-Sequencing, to determine contemporary genetic diversity, structure, and gene flow at two spatial scales; global, across the SO, and regional, within Kerguelen. Results identified substantial genetic structure, differentiating Patagonia, Falklands/Malvinas Islands, South Georgia and the Kerguelen archipelago, and low levels of contemporary gene flow. The most notable genetic differentiation was found between Patagonia/Falklands and South Georgia/Kerguelen. Structure was also significant between Patagonia and the Falkland/Malvinas Islands. Conversely, South Georgia and Kerguelen exhibited closer genetic affinity, and indications of recent but limited gene flow. Moreover, historical gene flow estimates between the four populations were low. At regional scale, noteworthy genetic structure persisted, and gene flow was insufficient to prevent genetic differentiation within Kerguelen. Consequently, rafting’s potential may be overestimated as a contemporary mechanism promoting gene flow across the SO, as these events may be sporadic, irregular, and unpredictable for marine invertebrates lacking a larval dispersal stage, since contemporary dispersal events don’t seem to facilitate high gene flow at both scales. Accordingly, other oceanographic factors or processes may hinder the establishment of species associated with macroalgae, and as consequence, contemporary genetic connectivity in the sub-Antarctic.
1 Introduction
Dispersal is a key trait for species from ecological and evolutionary perspectives, as movement plays a role in population dynamics, the extent of a species’ geographic range, the degree of gene flow, and the potential for local adaptation (Slatkin, 1985; Palumbi, 1994; Cowen and Sponaugle, 2009; Manel et al., 2023). In marine ecosystems, fish and invertebrates have complex life cycles where at least one stage may be mobile and promotes a species’ dispersal, either by active or passive movement, as dictated by life histories (Gaines et al., 2007). Because some of these earlier stages are often small sized, it’s difficult to directly measure dispersal (Weersing and Toonen, 2009). However, genetic data has been used to study the exchange of individuals and their genes between populations, by estimating a direct consequence of effective dispersal: population connectivity (henceforth ‘connectivity’), defined as the degree to which gene flow affects a species’ evolutionary processes (Lowe and Allendorf, 2010).
For most benthic invertebrate species with complex life cycles, connectivity is usually associated with pelagic larval stages, which, aided by interactions with oceanic currents and processes, can travel and settle up to tens to thousands of kilometers away from their spawning populations (Cowen and Sponaugle, 2009; White et al., 2019). While numerous larval traits influence dispersal and connectivity (Blanco et al., 2019), pelagic larval duration (PLD) is key, as the longer larvae spend in the water column, the higher the chances to traverse longer distances, increasing dispersal potential, gene flow and limiting genetic structure (i.e. FST) (Waples, 1987; Haye et al., 2014; Pinsky et al., 2017; Álvarez-Noriega et al., 2020; Hernawan et al., 2021). On the contrary, restricted or null dispersal, low gene flow and marked genetic structure is to be expected from species with short PLD (i.e. lecithotrophic larvae) or without pelagic larval stages (i.e. brooders or direct developers). While this paradigm is widely accepted, there are plenty of exceptions to both these assumptions, as some species with high dispersal potential display unexpectedly high levels of genetic structure (Selkoe et al., 2016; Larsson et al., 2017; Gaeta et al., 2020), and on the other end of the spectrum, species with low or null dispersal potential report low genetic structure spanning great geographic distances (Selkoe et al., 2016; Fleming et al., 2018; Gélin et al., 2018; Bertola et al., 2020). These ‘exceptions’ further illustrate that dispersal in the marine ecosystem is complex and multidimensional and is subjected to intrinsic and extrinsic mechanisms and processes that influence its extent and consequences. As such, while larvae are undoubtedly important and PLD can guarantee a minimum dispersal distance, these traits may be a poor predictor of gene flow (D’Aloia et al., 2015; Hilário et al., 2015; Esser et al., 2023), because other factors such as ecology, physiology, oceanography or habitat selectivity limit dispersal and/or settlement rates (Waters et al., 2013; Sjöqvist et al., 2015; Pascual et al., 2017; Gaeta et al., 2020). Moreover, regardless of development mode, other life stages can play an important role in a species dispersal, such as adult migrations (Frisk et al., 2014), or other mechanisms or processes of natural or anthropogenic origin that may facilitate dispersal (Carlton et al., 2017).
One of such mechanisms is rafting, defined as marine dispersal mediated by buoyant elements of organic or inorganic origin, often described for organisms that live in close association with macroalgae (Highsmith, 1985; Thiel and Gutow, 2005; Winston, 2012). Numerous studies provide global evidence supporting the hypothesis that direct developers and brooders can disperse through rafting (Haye et al., 2012, Haye et al, 2014; Trickey et al., 2016; Bertola et al., 2020), arguing that this mechanism may be as relevant for dispersal and connectivity as having a pelagic larvae dispersal phase (Carlton et al., 2017; Simkanin et al., 2019; Pfaller et al., 2019). The Southern Ocean (SO) is a vast mass of open ocean encircling Antarctica and tens of relatively small islands, and influencing a portion of the Australian and South American continents (Koubbi et al., 2014; Chapman et al., 2020). Within this area, the past two decades of phylogenetic and phylogeographic research have pointed out that rafting may be the leading hypothesis to explain the current distribution and high levels of genetic connectivity that some sub-Antarctic benthic species display (Nikula et al., 2010; Cumming et al., 2014; Moon et al., 2017; González-Wevar et al., 2018; Waters et al., 2018b; Fraser et al., 2020a; Güller et al., 2020; Macaya et al., 2020), as buoyant macroalgae may help achieve long-distance dispersal (LDD), a highly relevant evolutionary and ecological process (Jokiel, 1990; Gillespie et al., 2012; Waters et al., 2018a; Macaya et al., 2020). This hypothesis is not only supported by genetic data, as even considering the extension of the SO, as many coastal and benthic taxa are shared within the sub-Antarctic biogeographical region (Griffiths et al., 2009; De Broyer et al, 2014; Koubbi et al., 2014). Since most of these land masses are of heterogeneous origins and ages (continental or volcanic, ranging from ~95Ma to ~500Ka) and are geographically isolated (Quilty, 2007; Hodgson et al., 2014), the most plausible mechanism by which these shared taxa could have colonized their coasts is via transoceanic LDD, largely associated to kelp rafts of Durvillaea antarctica and Macrocystis pyrifera (Waters et al., 2018a; Fraser et al., 2020a). These macroalgae not only dominate near-shore, hard-substrate ecosystems, but are frequently observed floating in open waters, and around 20 million of viable rafts have been estimated to be adriftin the Southern Ocean (Smith, 2002; Stevens et al., 2002). Moreover, phylogeographic studies illustrate common haplotypes between sub-Antarctic islands and southern South America (Patagonia and Falklands/Malvinas Islands), suggesting that D. antarctica (Fraser et al., 2009, Fraser et al, 2010; Bussolini and Waters, 2015) and M. pyrifera (Macaya and Zuccarello, 2010; Assis et al., 2023) have great ability to disperse across the Southern Ocean. Coupled with the fact that live, viable benthic invertebrates have been registered on beached rafts (Fraser et al., 2010; Layton et al., 2022), it is to be expected that species associated with rafting macroalgae are able to withstand floating adrift, settle into new populations, and achieve contemporary gene flow unrelated to their developmental mode, hinted by the scarce studies with SNPs (Single Nucleotide Polymorphisms) in the Southern Ocean (Leiva et al., 2019; Zbawicka et al., 2019; Lau et al., 2023).
However, knowledge of this mechanism is still lacking, and less attention has been given to the frequency of these events and its consequences on contemporary patterns of connectivity (Gillespie et al., 2012; Waters et al., 2018a). Are rafting events rare enough that there’s only sporadic gene flow, to be able to sustain a species’ integrity? Or are they frequent enough to establish a regular, contemporary gene flow amongst isolated, distant coasts? To address this issue, we studied the pulmonate false limpet Siphonaria lateralis (Gastropoda: Heterobranchia) as a model to re-evaluate connectivity and rafting in the Southern Ocean. Despite its benthic protected development with encapsulated larvae in egg masses (Zabala et al., 2020), this species is widely distributed across the rocky intertidal of Patagonia, Falkland/Malvinas Islands, South Georgia, Kerguelen and Macquarie (Dayrat et al., 2014; Güller et al., 2015; González-Wevar et al., 2018), a paradoxical distribution for a species with low dispersal potential. Nevertheless, as discussed above, such extended distribution is not considered rare for species that live in close association with D. antarctica, and rafting has been proposed as the main mechanism explaining such a broad distribution of S. lateralis (Morton and Miller, 1973; Simpson, 1976; González-Wevar et al., 2018). Moreover, phylogeographic studies with mtDNA and nucDNA markers evidenced shallow genetic differentiation across the sub-Antarctic region (>8,000 Km) suggesting that dispersal through rafting may preserve the genetic integrity of the species over large geographic distances (González-Wevar et al., 2018). However, recent studies aiming to explore connectivity with multilocus genetic markers such as SNPs (Single Nucleotide Polymorphisms) have demonstrated that, even in light of absent or low phylogeographic structure, marine species exhibit significant genetic subdivision and restricted gene flow across populations (Pazmiño et al., 2017; Teske et al., 2018; Green et al., 2022). Moreover, while D. antarctica across the Southern Ocean consists of a single broader, sub-Antarctic clade, this macroalgae is sub-structured and there is substantial differentiation between islands according to SNP data (Fraser et al., 2018), that nevertheless seem to be sporadically connected, as supported by distributional modeling and beached kelp rafts, which provide evidence of LDD events across the Southern Ocean (Fraser et al., 2018; Fraser et al, 2022). Considering this information, we expect that associated species such as S. lateralis will display moderate levels of genetic structure and low levels of recent gene flow, enough to maintain contemporary connectivity. Furthermore, our objective is twofold. Firstly, to evaluate whether the long-debated mechanism of rafting maintains substantial contemporary levels of connectivity throughout the distribution range of S. lateralis. Secondly, to ascertain whether this mechanism is effective at a regional scale, facilitating connectivity among disjoint yet geographically proximate coastal populations, particularly within the Kerguelen Islands. Clarifying these two aspects will help towards drawing robust conclusions about the extent and implications of rafting on the Southern Ocean, and its effects on evolutionary history, biogeographic patterns, and eventual conservation strategies.
2 Materials and methods
2.1 Sampling, sequencing and SNP filtering
Between the years 2013 and 2017, 190 adult Siphonaria lateralis individuals were sampled from eight locations, spanning most of the species’ range (Figure 1). Specimens were stored in 96% ethanol for posterior genomic DNA extraction with Qiagen’s DNeasy Blood & Tissue™ Kit, following the manufacturer’s instructions, and DNA concentration was assessed with Qubit™ 3.0 fluorometer (Lifetechnologies). Samples were sent to the Biotechnology Center of the University of Wisconsin, USA. Sequencing and construction of genomic libraries was done by Genotyping by Sequencing (GBS) using Illumina NovaSeq 6000 platform, following a Reduced Representation Sequencing (RRS) approach, which is a reliable and affordable method to study population structure of non-model species without a reference genome, and produce a large number of Single Nucleotide Polymorphisms (SNPs) (Rovelli et al., 2019; Kunvar et al., 2021). Under this approach, library preparation used two restriction enzymes to reduce the complexity of the genome (NsiI-MspI), and barcode adaptors for each individual. Single end reads were visualized in FastQC for quality checks. To generate our SNP dataset, our data was prepared and analyzed following the UNEAK pipeline (Universal Network-Enabled Analysis Kit) implemented in TASSEL v.5, which is useful for de novo locus identification for non-model organisms (Lu et al., 2013). With the UNEAK pipeline, the dataset was demultiplexed, barcodes were removed and reads were trimmed from ~100 bp to 64 bp. Identical tags were aligned with an error tolerance rate of 0.03, to minimize considering real tags as sequencing errors, and we kept only biallelic loci. Following this, we assembled two SNPs datasets according to our research objectives, one global, encompassing S. lateralis individuals sampled across different Southern Ocean sub-Antarctic provinces, and a regional dataset, considering three populations from Kerguelen Islands. We used a 80% mnC (minimum call rate), 5% MAF (minimum allele frequency) to filter the SNPs from each dataset (global and regional), and we allowed a maximum of 40% missing data per individual in TASSEL v.5. Then, we excluded those SNPs that failed the Hardy-Weinberg equilibrium (HWE) test implemented in Arlequin v3.5 (Excoffier and Lischer, 2010) at p < 0.05, and we used a False Discovery Rate (FDR) correction to avoid false positives (incorrectly considering as a SNP as out of HWE), in at least 60% of each of the dataset populations. Since the interest of the study was to focus on neutral evolutionary processes, we identified potential outlier SNPs with BAYESCAN 2.1 (Foll and Gaggiotti, 2008). For this, 500,000 MCMC iterations were run with a 10% burnin’, a thinning interval of 10, and a prior odd of 1,000 (100 for the regional dataset), to minimize potential false positives, according to the large number of SNPs to test. Subsequently, we removed all SNPs after FDR q-value correction that had strong or very strong evidence to be under selection according to Jeffrey’s criterion, to obtain a global and a regional neutral SNPs dataset for downstream analyses.
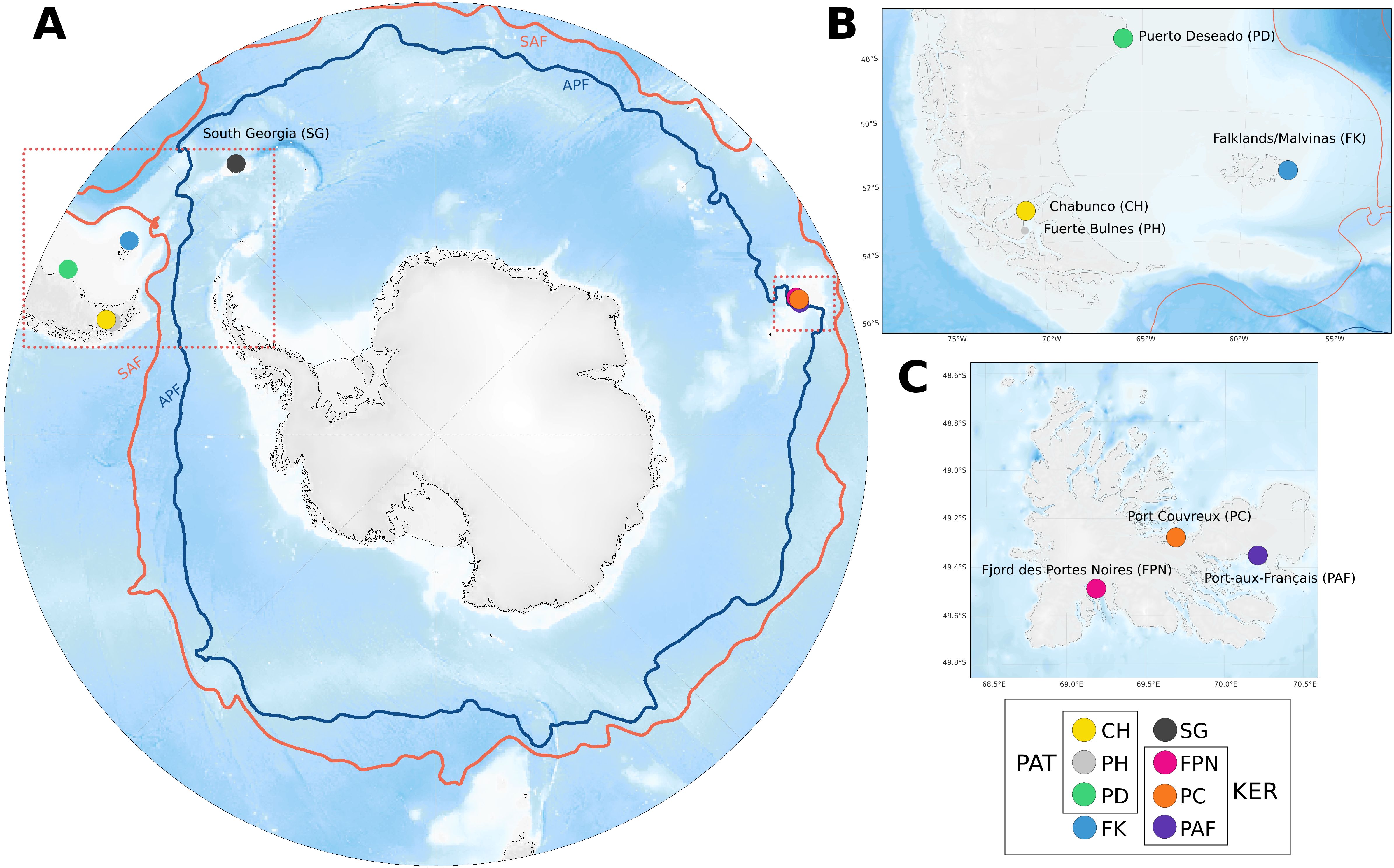
Figure 1. Map of the 8 sampled populations of Siphonaria lateralis (A), with inset maps of South America (B) and Kerguelen Islands (C) sampling sites. Label box provides regional grouping information (PAT = Patagonia, KER = Kerguelen).
2.2 Genetic diversity
Analyses were performed for the global and regional datasets to evaluate the differences in genetic diversity at both spatial scales. For each population, expected heterozygosity (He), observed heterozygosity (Ho), average number of alleles per locus (allelic richness, A), the proportion of polymorphic loci (%Po), and the inbreeding coefficient (FIS) were computed in GENETIX (Belkhir et al., 2004). Additionally, genetic diversity parameters were estimated for the four major geographic areas within the global dataset, and private alleles for each area, and within Kerguelen populations, were quantified in HP-RARE 1.0 (Kalinowski, 2005).Within Kerguelen populations, we estimated the effective population size (Ne) based on the linkage disequilibrium (LD) method using NeEstimator v2.1 (Do et al., 2014), and jackknife 95% confidence intervals (CIs) (Waples and Do, 2008).
2.3 Population structure
We used multiple approaches to assess population genetic structure at global and regional scale. First, pairwise population FST and their significance was estimated using a test of 10,000 permutations in Genodive v 3.0 (Meirmans, 2020). Corresponding FST p-values of multiple testing were adjusted through FDR (Narum, 2006). Secondly, we performed three individual-based population assignment tests to estimate genetic structure; 1) IBS analysis, which clusters together unrelated individuals based on similarities between genotypes at each locus, implemented in the R package SNPRelate (Zheng et al., 2012), 2) Principal Component Analysis (PCA), to determine the percentage of variation in allele frequencies explained by the major axes, implemented in adegenet (Jombart, 2008), and 3), STRUCTURE (Pritchard et al., 2000), a bayesian clustering method that assigns individuals to populations based on allele frequencies. Our aim with STRUCTURE was to discover the main genetic groups across the species’ distribution across the Southern Ocean, and secondly, to determine substructure within each main group. Due to the large number of SNPs in our datasets, we used StrAuto, a Python script that reduces analysis time by allowing for parallel computing of STRUCTURE runs (Chhatre and Emerson, 2017). Number of estimated ancestral populations (K) varied from one to six. Ten replicate runs were performed for each estimated K under the admixture model, with 600,000 MCMC, a 10% burnin’. The most probable K value was inferred based on the ΔK method (Evanno et al., 2005), utilizing Structure Harvester online (Earl and vonHoldt, 2012). Post processing of STRUCTURE results and cluster graphs were drawn in CLUMPAK (Kopelman et al., 2015). Finally, we implemented an Analysis of Molecular Variance (AMOVA) in Arlequin (Excoffier and Lischer, 2010) to evaluate three grouping scenarios/hypothesis of a priori partitioning, to determine which grouping hypothesis maximized the difference between groups.
2.4 Gene flow
Two methods were used to estimate migration rates between the four major geographical areas, and at regional scale; 1) a multilocus approach based on the number of rare alleles, assuming that populations are under migration–drift equilibrium, to estimate the historical effective number of migrants (Nm) (Barton and Slatkin, 1986) in GENEPOP v.4.5 (Rousset, 2008), and 2) BayesAss V3, a bayesian approach that uses individual information to estimate recent migration events, asymmetric patterns of gene flow and directionality. At the same time, this analysis allows to identify first, second or third generation migrants between populations (Wilson and Rannala, 2003).A total of 100,000,000 MCMC iterations were run with a 25% burnin’, sampling every 1,000 chains, to ensure chain independency, and convergence was confirmed in Tracer (Rambaut et al., 2018).
3 Results
3.1 Global and regional datasets
Raw data for each plate consisted of 260,135,231 and 263,506,046 reads, with 249,396,409 and 253,244,524 high-quality reads respectively. Using the UNEAK pipeline, 69,412 candidate SNP sites were detected across 140 individuals of Siphonaria lateralis from eight sampled populations. Out of the 140 individuals, only 116 had sufficient information to be included in the dataset after the bioinformatic filters, resulting in 6,277 SNPs. A total of 351 loci were removed due to being out of HWE in 60% or more of the populations after FDR correction, and another 411 loci were removed after Bayescan analysis identified these loci as outliers, with strong evidence of being under diversifying selection. The final global dataset was made up of 5,515 SNPs putatively neutral loci, for downstream diversity, structure, and gene flow analyses.
For the regional dataset, after implementing bioinformatic filters on 69,412 candidate SNPs from 53 individuals collected at Kerguelen Islands, 52 individuals showed sufficient data across a total of 1,646 SNPs. However, after HWE and FDR filters, we kept 1,298 SNPs, where a further 34 loci were removed for showing significant signals of being under selection. Accordingly, our putatively neutral working dataset consisted of 1,264 SNPs for diversity, structure and gene flow analyses on a smaller regional scale.
3.2 Population genetics across the Southern Ocean
3.2.1 Genetic diversity
All genetic diversity statistics were similar and low in each of the eight sampled populations of S. lateralis, except from Falklands/Malvinas Islands (for values and population codes hereinafter used, Table 1). Proportion of polymorphic loci ranged from 2.99% to 69.23%, where Falklands/Malvinas had the highest polymorphic loci, and contrarily, populations from South America (PH and PD) exhibited the lowest levels of genetic diversity. Observed heterozygosity (Ho) ranged from 0.0153 (FPN) to 0.2148 (FK), yet most values ranged from 0.0153 to 0.0288 (PAF), except from the FK population, which showed the highest Ho by more than an order of magnitude compared to all other populations. Expected heterozygosity (He) ranged from 0.0131 (PH) to 0.2406 (FK), and was slightly lower than Ho in half the populations (Table 1, values indicated in bold letters), and slightly higher than Ho in the other half. Allelic richness (A) ranged from 1.0299 (PH) to 1.6923 (FK), and was similar between all populations except for FK. Finally, FIS ranged from -0.25854 (PH) to 0.39936 (SG), with negative values found only in continental sites (CH, PH, PD) and PAF.
Grouping all populations by geographical area (Patagonia = PAT, Falklands/Malvinas = FK, South Georgia = SG, and Kerguelen = KER, Supplementary Table 1) illustrates similar diversity patterns, as FK remains the most diverse across all statistics. Polymorphism ranged from 14.84% (PAT) to 69.23% (FK), where at least 818 of the 5,515 loci were polymorphic. The He index ranged between 0.0193 (PAT) to 0.2350 (FK), and Ho ranged from 0.197 (SG) to 0.2148 (FK), where He was lower than Ho only in PAT. FIS ranged from -0.02749 (PAT) to 0.39936 (SG), with PAT being the only negative value and SG more than doubled the value of other areas. The number of alleles per area ranged from 6334 (PAT) to 9333 (FK), with 1% (SG) to 36% (FK) of them being private. The number of private alleles from FK were three times higher than those recorded in other areas.
3.2.2 Population structure
All pairwise FST comparisons were significant amongst the eight sampled populations, with high and extremely high values across the studied distribution of Siphonaria lateralis (Table 2). The lowest FST value between sampled populations was found between CH-PH (FST = 0.236), the closest populations. In a general pattern, populations within the same geographical areas (within Patagonia and within Kerguelen islands) exhibited the lowest levels of differentiation amongst themselves (FST < 0.3). The highest FST value was found between FPN-PC (FST = 0.962), some of the most distant populations (8,000 Km). In fact, the highest comparisons (FST > 0.9) were found comparing Kerguelen populations (FPN, PC, PAF) with Patagonia populations (CH, PH, PD), and South Georgia with Patagonia populations. Genetic differentiation was slightly lower between South Georgia and Falkland/Malvinas (FST = 0.752), which are separated by approximately 1,500-2,200 Km of open ocean. South Georgia and Kerguelen populations displayed lower levels of genetic differentiation (FST = 0.579 - 0.604), despite being geographically more distant (>6,500 Km). Falklands/Malvinas Islands, on the other hand, was moderately-highly differentiated with all sampled populations (FST = 0.655 - 0.752).
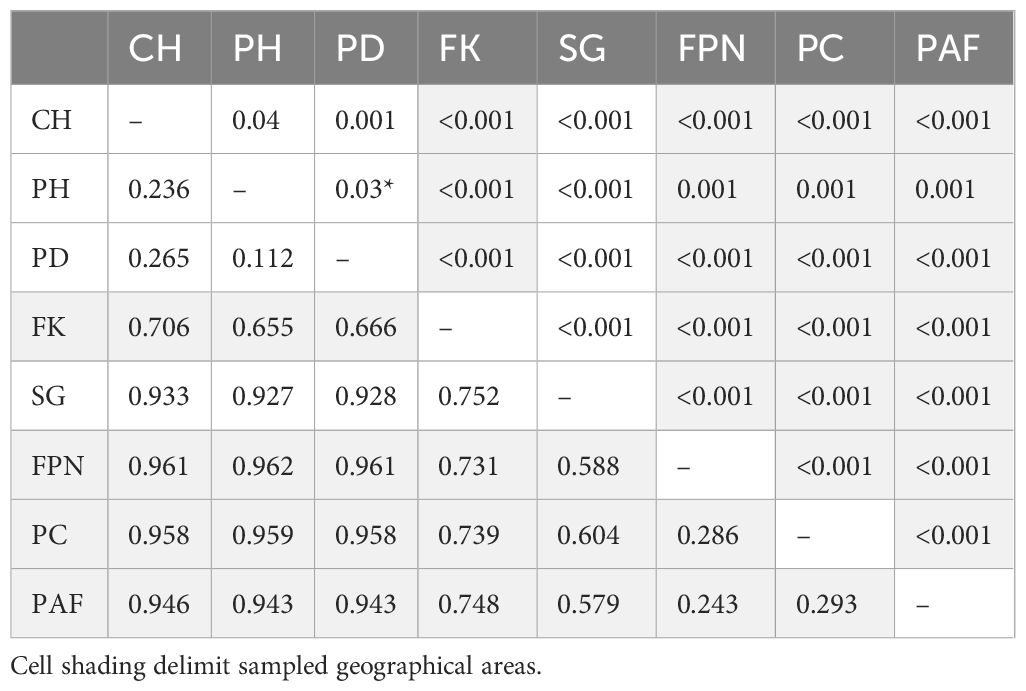
Table 2. Pairwise FST values (below diagonal) for all Southern Ocean populations, and their statistical significance (above diagonal).
Individual-based analyses of global genetic structure suggest the existence of at least three, if not four genetic groups. Based on the number of shared alleles between individuals, the IBS analysis (Supplementary Figure 1) recognized four groups, two clearly defined and separate, and two relatively closer, similar to the PCA (Figure 2). The first two Principal Components explain most of the genetic variance (>70%): the first axis clearly differentiates FK from all other populations, and the second axis segregates CH, PH, PD (PAT) from a FPN, PC, PAF and SG (KER + SG) (Figure 2A). When considering a third axis, while it explains little of the genetic variance (3.41%), the PCA was able to distinguish South Georgia as a slightly differentiated group compared to Kerguelen Islands (Figure 2B). STRUCTURE’s bayesian clustering and Evanno’s ΔK indicate that two genetic clusters make up the first hierarchical level of genetic structure (Figure 3B, Supplementary Figure 2), highlighting the stark divergence between southern South America (CH, PH, PD and FK) and sub-Antarctic Islands (SG, FPN, PC and PAF). While further exploration into K = 3 distinguishes Falklands/Malvinas from the rest of South America (Figure 3C), matching results from the first two principal components of the PCA and the IBS, the second hierarchical level of genetic structure is accounted for when observing K = 4, which reveals significant substructure within the 2 main genetic clusters, southern South America and sub-Antarctic islands, providing a visual representation of what pairwise FST and PC3 suggest (Table 2, Figure 2B): South Georgia is a genetically distinct group, that, however, has a significant presence of a genetic component from Kerguelen, which may correspond to third-generation migrants (Figure 3D).
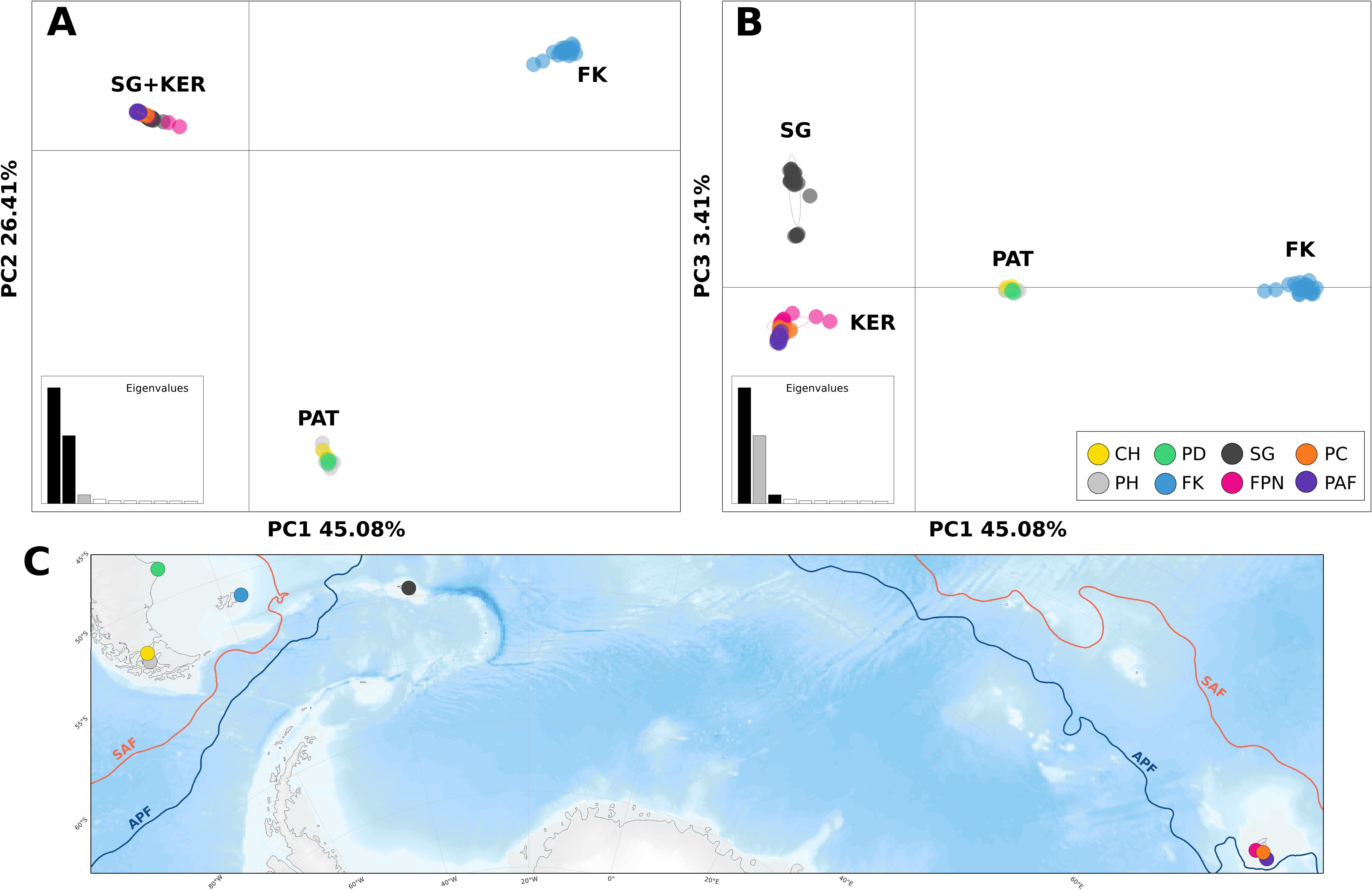
Figure 2. Global PCA scatterplot of the first 2 principal components (A), and second and third principal components (B), with percentage of explained variance by axis. Colors represent the sampling population of each individual, additional labels represent major geographical areas (C), to reference the map (PAT = Patagonia (CH, PH, PD), SG = South Georgia, FK = Falklands/Malvinas, and KER = Kerguelen (FPN, PC, PAF)).
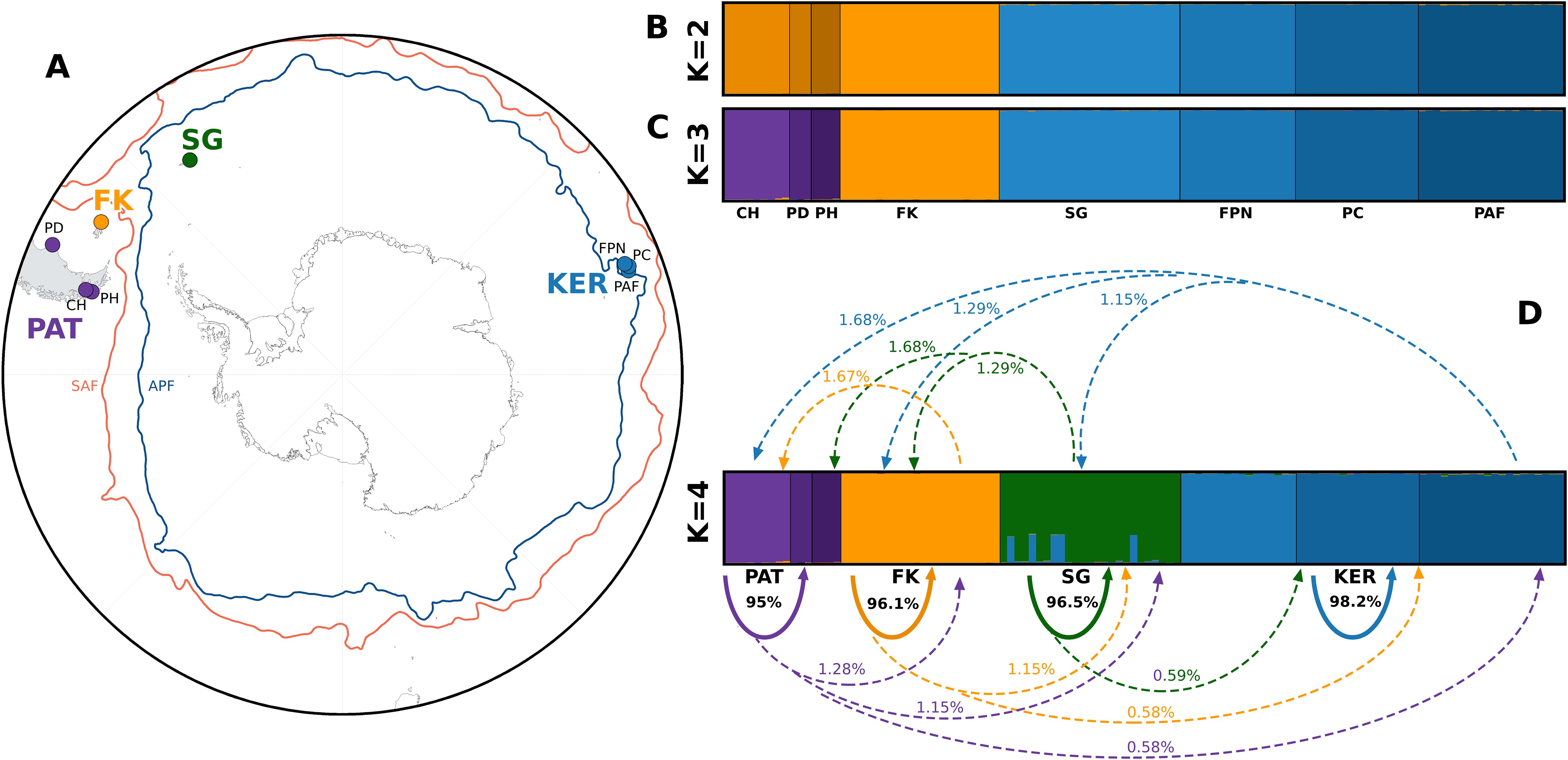
Figure 3. Southern Ocean map with the four major geographical areas (A), and Bayesian clustering inferred with STRUCTURE for K = 2 (B), K = 3 (C), and K = 4 its respective recent migration rates inferred by BA3 for K=4 (D). Colors represent geographical areas and are shaded to distinguish sampled populations. Solid arrows represent self-recruitment, dashed arrows represent migration towards other populations.
Additionally, to further clarify the global genetic structure, we performed an AMOVA analysis considering the three alternative grouping hypotheses provided by the genetic structuring analyses as follows: H1 = two groups South America - Sub-Antarctic Islands (SA-IS), H2 = three groups Patagonia - Falklands/Malvinas - sub-Antarctic Islands (PAT-FK-IS), and H3 = four groups Patagonia - Falklands/Malvinas - South Georgia - Kerguelen (PAT-FK-SG-KER) (Supplementary Table 3). H1 was discarded, given that variation among groups (Va = 57.56, FCT = 0.5755) was comparatively lower than for the other two hypotheses. While FCT was slightly higher for H2 than H3 (0.81269 versus 0.80529), we took into consideration the significant substructure within sub-Antarctic islands, illustrated by the percentage of variance among populations within groups in H2 (Vb = 4.69) and pairwise FST with K = 4 (KER-PAT FST = 0.572, p-value <0.001, Supplementary Table 2). Therefore, we computed how much variation was truly just because of grouping choice, controlling for variation amongst populations within groups (Va/Va+Vb), which supported the four groups hypothesis over other grouping hypotheses (H3 = 98.33% versus H2 = 94.84%), considering South Georgia as a fourth group on its own.
3.2.3 Gene flow estimates
Two approaches were used to estimate gene flow between the four genetic groups, across >8,500 Km in the Southern Ocean (Figure 3A). Historical gene flow inferred by GENEPOP was low (Nm<1), and ranged from 0.0135 (between Patagonia and Kerguelen) to 0.305 (between South Georgia and Kerguelen) (Table 3). However, migration between South Georgia and Kerguelen Islands, albeit still low, was an order of magnitude higher than between the other genetic groups. On the other hand, recent migration rates between groups, as indicated by the BA3 results (Figure 3D), illustrate two scenarios; pairwise population migration rates range from 0.58% to 1.68%, whereas the highest rates consist on self-recruitment, ranging from 95% to 98.2%, meaning that >95% of individuals were genetically assigned to their population of origin. However, pairwise population migration rates were not symmetrical; inferred gene flow from Patagonia, Falklands/Malvinas and South Georgia towards Kerguelen is an order of magnitude lower than inferred gene flow from Kerguelen to the other three areas (i.e. KER towards PAT = 1.68%, PAT towards KER = 0.58%). Finally, BA3 did not identify migrants of first, second, or third generations, with all individuals being assigned as ~100% belonging to their population of origin, unlike the five individuals visually identified as possible third-generation migrants in STRUCTURE (Figure 3D).
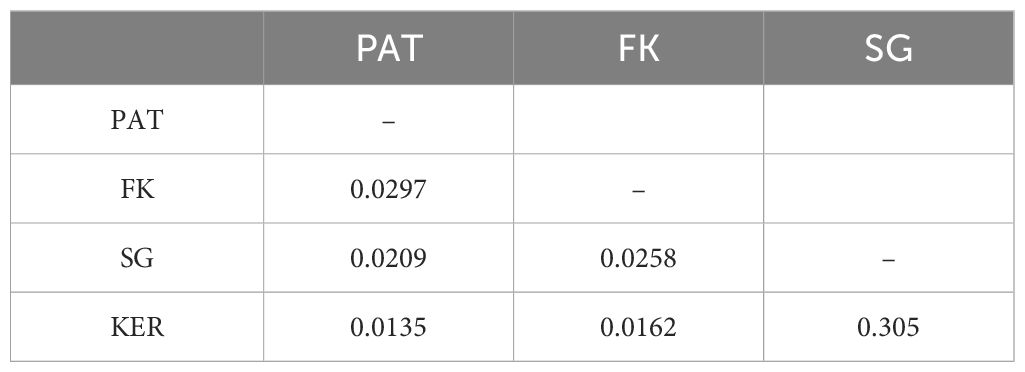
Table 3. Global pairwise estimates of the effective number of migrants (Nm) as determined with GENEPOP.
3.3 Regional population genetics
3.3.1 Kerguelen genetic diversity
For the regional scale, which encompasses the Kerguelen Archipelago, genetic diversity was similar across populations, yet slightly higher in PAF across all statistics (Table 4). Proportion of polymorphic loci ranged from 75.16% to 91.07%, expected heterozygosity (He) ranged from 0.271 to 0.345, and observed heterozygosity (Ho) ranged from 0.280 to 0.449, where He was lower than Ho for FPN and PAF. Allelic richness (A) within populations ranged from 1.75518 to 1.9107. FIS ranged from -0.278 to 0.055, negative only within PAF, suggesting a slight excess of heterozygotes and departure from HWE. Effective population size (Ne) was higher in PAF (224.4), and lowest in FPN (91.8). As for private alleles, FPN had the lowest value with 13 alleles, followed by PC with 64, and the highest value was observed in PAF with 155 alleles, representing between 1% and ~12% of the 1,264 loci in the regional dataset.
3.3.2 Kerguelen structure and gene flow
The pairwise FST analyzes all resulted in significance (p < 0.05), with values ranging from 0.124 to 0.154 (Supplementary Table 4). The presence of three genetic groups corresponding to the sampled locations observed is suggested by IBS (Supplementary Figure 3), and the PCA, where the first two principal components (PC1 and PC2) explain >40% of the genetic variance results (Figure 4A). Likewise, STRUCTURE analyses determined an optimal K = 3, with three genetic groups clearly defined, each corresponding to the different sampled locations across Kerguelen, with only a slight signal of mixed genetic component of other groups, and without visual evidence of first, second or third generation migrants (Figure 4C).
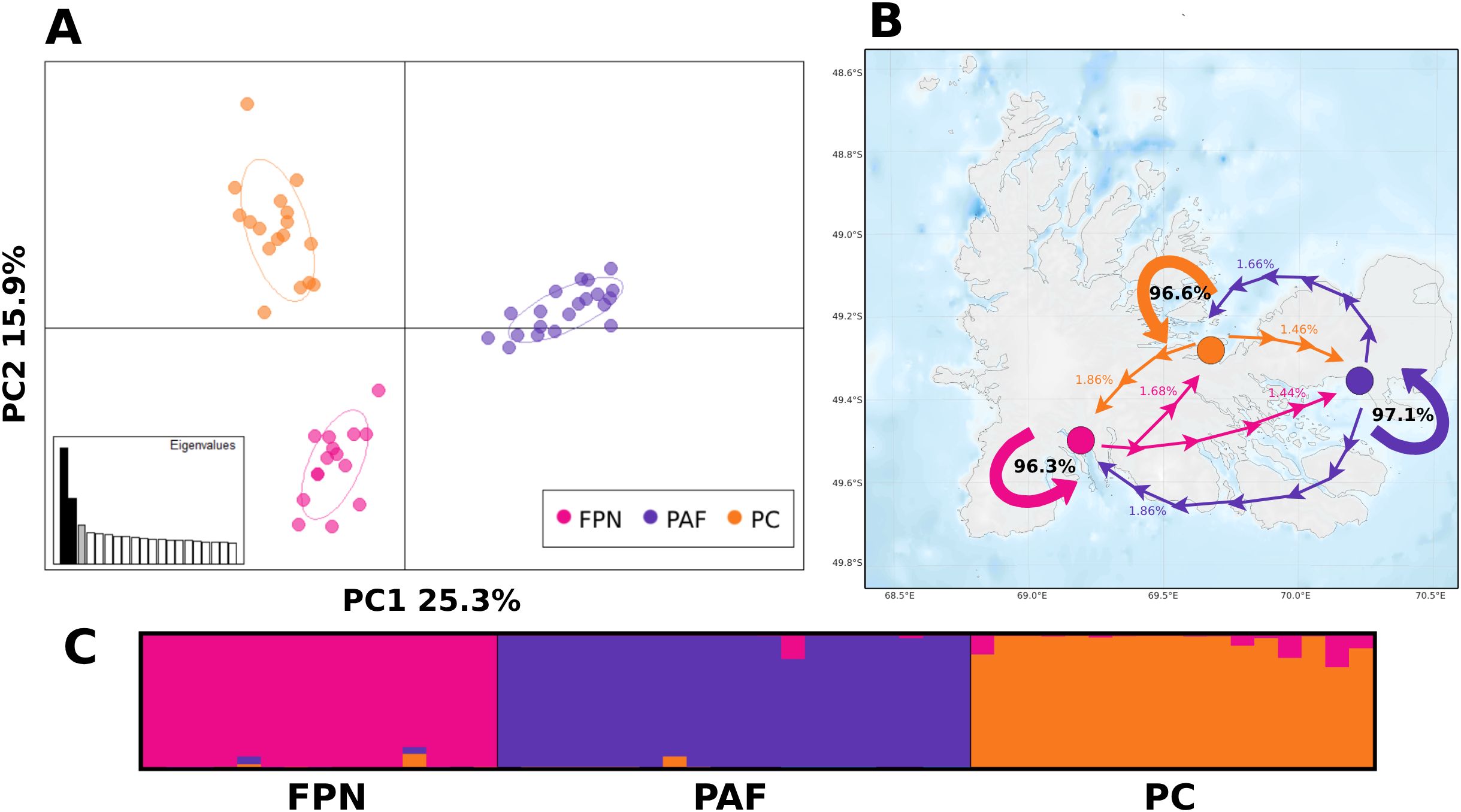
Figure 4. Genetic structure within Kerguelen Islands: PCA scatterplot showing the first 2 principal components and percentage of explained variance by axis (A), BA3 recent migration rates between sampled populations (B), and Bayesian clustering inferred with STRUCTURE (C). Colors represent the sampling population of each individual. Solid arrows represent self-recruitment, dashed arrows represent migration towards other populations.
Both historical and contemporary gene flow between these three groups is very low. According to GENEPOP, historical Nm between groups ranges from 0.06 to 0.077, the lowest genetic exchange being between PC and PAF (Supplementary Table 5). At contemporary scale, migration rates between the described populations ranged from 1.44% to 1.86%, were migration from FPN towards PAF (1.44%) was slightly lower than from PC to PAF (1.46%), providing evidence of low regional migration rates among the analyzed populations, which are mostly sustained by self-recruitment (>96%) (Figure 4B). Furthermore, BA3 did not provide any evidence for first, second, or third generation migrants.
4 Discussion
Through the analysis of genomic data (SNPs), we found that distant populations of Siphonaria lateralis distributed across the Southern Ocean are not sufficiently connected to prevent significant genetic differentiation. Buoyant kelp such as Durvillaea antarctica have probably played a crucial role in favoring transoceanic, long distance dispersal by rafting, and genetic homogeneity, of various coastal marine invertebrates, which largely match this macroalgae distribution (Fraser et al., 2010; Nikula et al., 2010; González-Wevar et al., 2018; Halanych and Mahon, 2018; Fraser et al., 2020a). This has allowed the colonization and re-colonization of sub-Antarctic coasts by different species (Leese et al., 2010; Nikula et al., 2010; Cumming et al., 2014; González-Wevar et al., 2018). Nonetheless, this dispersal mechanism would not necessarily prevent population differentiation. In light of our estimations of genetic structure and gene flow among populations at different geographical scales across the SO, based on more than 5,000 SNPs, we found that contemporary rafting does not promote sufficient ongoing gene flow in the pulmonate limpet S. lateralis, a species with benthic protected development, to homogenize distant populations. Furthermore, our results serve as evidence that connectivity is a process more complex than just accounting for dispersal potential, as Moon et al. (2017) argue, in which local dynamics, past and contemporary climate and physical barriers to dispersal, among other factors, may determine the extent of connectivity.
The eight sampled populations represent four major geographical areas; Patagonia, Falkland/Malvinas Islands, South Georgia, and Kerguelen. Genetic diversity was remarkably low across populations and geographical areas, in comparison to other genomic (SNPs) studies on gastropods across the world (i.e. Ho = 0.35-0.06) (Cortez et al., 2021; Maes et al., 2022; Morrissey et al., 2022; Quintero-Galvis et al., 2023). Moreover, values were low, regardless of whether populations were under-sampled or well-sampled. Falkland/Malvinas Islands was the only exception to this pattern, displaying genetic diversity values more than an order of magnitude higher than the rest of the populations, but falling in line with diversity from other gastropods. At global scale, patterns of low genetic diversity most likely reflect a founder takes all effect (Waters et al., 2013), where LDD rafting allow few founder alleles to colonize new populations, and genetic diversity remains low after demographic growth due to density dependent processes, with new alleles having little success in arriving and establishing in high-density populations. During Quaternary glaciations, the majority of the Pacific coast of southern South America was covered by the Patagonian Ice Sheet (Rabassa et al., 2005, Rabassa et al, 2011), extinguishing most of the benthic taxa (Clarke and Crame, 2010; Post et al., 2014), yet some taxa survived in pockets of glacial refugia (Montecinos et al., 2012; Trovant et al., 2015). On the contrary, the Atlantic coastline receded and exposed the continental shelf (Glasser and Jansson, 2008), causing local extinctions and/or range contractions, with refugium at lower latitudes for cold-temperate taxa. Moreover, SO islands had different glaciation scenarios; Falklands/Malvinas Islands had little to no ice during the Last Glacial Maximum (LGM), while South Georgia and Kerguelen Islands had terrestrial and marine glaciers (Fraser et al., 2009; Hodgson et al., 2014). While these scenarios offer different paths for recolonization events, phylogeographic patterns of S. lateralis support the hypothesis of two independent glacial refugia for the species (González-Wevar et al., 2018). One in Falklands/Malvinas, refugium illustrated by other benthic taxa (Leese et al., 2010; González-Wevar et al., 2012), which has thrived in isolation to this day, as illustrated by the rich genetic diversity found in our results (heterozygosity, polymorphism and number of private alleles). And a second refugium, in southern South America, with a reduced genetic diversity that recolonized both Patagonian coasts and distant populations across the SO such as South Georgia and Kerguelen Islands, which are less diverse in comparison to Falklands/Malvinas Islands, that have evolved separately with little to no allele surfing from other populations.
According to what we expected, we found significant genetic structure in S. lateralis across the SO. Results indicated that a substantial differentiation coincided with areas North and South of the Antarctic Convergence, an oceanographic feature that according to recent genomic studies, both represents a genetic barrier for gene flow on some species (Moore et al., 2018) or has no influence whatsoever (Galaska et al., 2017). However, we found strong evidence of four genetic clusters, matching the four major geographical areas sampled; Patagonia, Falklands/Malvinas Islands, South Georgia, and Kerguelen. The highest differentiation occurred between the two most distant populations, Patagonia and Kerguelen, however, Patagonia and South Georgia were also markedly differentiated, which is interesting to note, because it may hint at a deeper or older differentiation between these sub-Antarctic islands and the continent. On the other hand, the marked differentiation of Falklands/Malvinas Islands from the rest of the SO is most likely due to a historical differentiation (Quaternary glaciations) from the rest of southern South America, also evidenced in other studies that evaluate the phylogeographic structure of marine invertebrates without free-living larvae, which display significant phylogeographic structure between Falklands/Malvinas Islands and Patagonia (Leese et al., 2008; González-Wevar et al., 2018; González-Wevar et al., 2021). However, there are some exceptions, as some benthic brooders lack genetic structure between both populations (Cumming et al., 2014; Segovia et al., 2022), and few benthic broadcasters display significant differentiation (González-Wevar et al., 2021). Therefore, in addition to historical patterns, other factors must be sustaining and/or promoting this differentiation, as either environmental discontinuities arising from oceanographic characteristics, or species traits that could limit dispersal and act as gene flow barriers (Gillespie and Roderick, 2014; Moon et al., 2017), as seen in studies compending the notothenioid fish Harpafiger, which has reduced phylogeographic structure between Falklands/Malvinas Islands and Patagonia, yet its population discrimination analyses using SNPs clearly differentiate both populations (Segovia et al., 2022).
On the other hand, similar to what was found with traditional markers, multilocus markers exhibit significant, but lesser genetic structuring between South Georgia and Kerguelen. Our data provide scarce evidence of historical long-distance dispersal (LDD) on S. lateralis across sub-Antarctic islands approximately 7,000 Km apart, potentially via rafting, as previously hypothesized by González-Wevar et al. (2018). Despite noteworthy estimated historical migration rates between South Georgia and Kerguelen, in comparison to other pairwise estimates, these values are low (Nm < 1) and insufficient to prevent polymorphism loss and population differentiation (Wright, 1931; Vucetich and Waite, 2000). Moreover, self-recruitment dominates S. lateralis population dynamics, where 95% of individuals remain in their population of origin, and <2% migrate to other populations, insufficient gene flow to prevent genetic structuring across the SO. Interestingly, estimated contemporary migration rates, albeit marginal, evidence double the gene flow from Kerguelen to South Georgia, spanning ~16,000 Km, than from South Georgia towards Kerguelen, the shortest route, by ~10,000 Km less. This is plausible because the Antarctic Circumpolar Current flows eastward, connecting all SO land masses and remote islands by oceanic circulation and drifting macroalgae (Leese et al., 2010; Hunt et al., 2016), and simulated trajectories by Fraser et al. (2018) illustrate multiple pathways within ecologically viable timeframes for D. antarctica and the lifespan of associated hitchhiking invertebrates to reach Antarctica and sub-Antarctic islands (Fraser et al., 2010; López et al., 2017). However, as Moon et al. (2017) argue, and even in light of documented rafting events, these events may be insufficient to sustain levels of contemporary gene flow capable of preventing population structuring, as illustrated by D. antarctica, which displays significant genetic structure across the SO (Fraser et al., 2018; Fraser et al, 2022). Moreover, the current location and movement of the Antarctic Polar Front (APF) may limit dispersal between South Georgia and Kerguelen through different abiotic factors, such as temperature, which may affect survivorship or development and is a significant driver of genetic structure (Boulanger et al., 2022; Mendes et al., 2022), as rafting also depends on species traits and physiological tolerances (Simkanin et al., 2019). Therefore, while LDD through rafting remains a relevant dispersal mechanism at evolutionary timescales, our SNP data offer little support to the hypothesis that it promotes high levels of gene flow to sustain significant levels of contemporary connectivity, and population genetic structure may arise, as illustrated by our results.
Finally, at a regional scale within Kerguelen, genetic diversity was higher in PAF. Genetic structuring analyses within Kerguelen Islands reveal three similarly differentiated groups. Additionally, contemporary gene flow analyses failed to detect recent dispersal events by first, second or third generation migrants between the studied locations, with historical genetic flow being marginal at best. Few studies have evaluated contemporary genetic structure and gene flow within Kerguelen Islands, and yet both brooders and broadcasters exhibit marked genetic structure between North, South and East (Ledoux et al., 2012; Fraïsse et al., 2021). This is an even more interesting finding than the genetic structure of S. lateralis across the sub-Antarctic region, as rafting events across hundreds to thousands of kilometers have proven to be historically relevant, yet may be unpredictable and irregular at present day (Moon et al., 2017). But if macroalgae such as D. antarctica and M. pyrifera are as abundant as they are within Kerguelen Islands (Féral et al., 2021), surely, rafting at regional scale could be more frequent and/or less stochastic that at larger scales, as gene flow would offset genetic structuring. Studies within and around New Zealand provide evidence of sporadic rafting of direct developers across >500 Km (Fraser et al., 2020b; Donald et al., 2020). However, local upwelling processes, environmental discontinuities or habitat specificity are some of the potential drivers of genetic divergence regardless of developmental mode, leading to significant genetic fragmentation over short geographical distances (Johansson et al., 2008; Ayre et al., 2009; Gonzalez et al., 2016; Donald et al., 2020). Within Kerguelen, the intertidal zone has abundant and sub-divided kelp forests forming defined patches of habitat, all of which are differently exposed to the diverse influences of both open sea and land water (rivers and melting glaciers) (Koubbi et al., 2016). On the other hand, the Kerguelen coast is heterogeneous, as it has both jagged and linear coastlines, and exposed or sheltered bays, which in turn determine the spatial distribution of benthic species (Poulin and Féral, 1995; Améziane et al., 2011). Therefore, this oceanographic and environmental patchiness, even under the assumption that S. lateralis disperses by rafting within Kerguelen, could lead to the levels of genetic structure found in our study. On the other hand, currents around the Kerguelen Islands either transport waters coastward, eastward or northward of the Kerguelen Plateau (Park et al., 2008; Zhou et al., 2014), providing little means for kelp rafts to move within Kerguelen, which could explain the low levels of gene flow and high estimates of self-recruitment among sampled populations of S. lateralis. Furthermore, Fraïsse et al. (2021) tested for the role of habitat heterogeneity in explaining the North-South differentiation of Mytilus in Kerguelen, and found that the presence of Macrocystis kelps, substrate type and coastal slope had a low but significant role in genetic differentiation.
Therefore, considering the observed patterns at global and regional scale, multilocus markers such as SNPs provide further tools to comprehend the extent and reach of dispersal and connectivity paradigms in the SO. Rafting has been an important Quaternary dispersal mechanism for benthic fauna, which provided the means to colonize/recolonize the sub-Antarctic region, yet its present relevance in promoting high levels of gene flow is disputed by our data, which, albeit limited to a single species, demonstrates that rafting sustains low levels of gene flow, evidenced in significant contemporary genetic structure across SO populations. Nevertheless, further research is needed to clarify contemporary dispersal patterns across the SO, which need to consider studying SNPs or whole genome sequencing in other species with different dispersal potential as study models, as well as environmental/oceanographical characteristics as potential barriers to present-day dispersal and genetic connectivity in the SO, where seascape genomic studies could help clarify these patterns.
Data availability statement
The datasets presented in this study can be found in online repositories. The names of the repository/repositories and accession number(s) can be found below: https://figshare.com/, https://doi.org/10.6084/m9.figshare.25939687.v2.
Ethics statement
The animal study was approved by Comité de Ética, Facultad de Ciencias, Universidad Austral de Chile. The study was conducted in accordance with the local legislation and institutional requirements. The study was conducted in accordance with the local legislation and institutional requirements.
Author contributions
CM-M: Data curation, Formal analysis, Methodology, Software, Validation, Visualization, Writing – original draft. ML: Data curation, Formal analysis, Methodology, Software, Validation, Visualization, Writing – original draft. TS: Funding acquisition, Project administration, Resources, Writing – review & editing. EP: Conceptualization, Formal analysis, Funding acquisition, Investigation, Methodology, Project administration, Resources, Supervision, Validation, Writing – review & editing. NS: Conceptualization, Data curation, Formal analysis, Methodology, Project administration, Resources, Software, Supervision, Validation, Writing – review & editing. CG-W: Conceptualization, Formal analysis, Funding acquisition, Investigation, Methodology, Project administration, Resources, Supervision, Validation, Writing – review & editing.
Funding
The author(s) declare financial support was received for the research, authorship, and/or publication of this article. This study was funded by the Millennium Science Initiative program (ANID) – ICN2021_002 to EP and CG-W. We also thank the ANID Scholarship Program, 2020 - 22200749, to CM-M, and Fondecyt 1210787, to CG-W.
Acknowledgments
The authors thank Valentina Bernal-Durán and Claudia Maturana, for assistance and feedback on analyses and troubleshooting errors. We would also like to thank the Chilean Antarctic Institute (INACH) and the French Polar Institute project 1044 PROTEKER for field sampling.
Conflict of interest
The authors declare that the research was conducted in the absence of any commercial or financial relationships that could be construed as a potential conflict of interest.
Publisher’s note
All claims expressed in this article are solely those of the authors and do not necessarily represent those of their affiliated organizations, or those of the publisher, the editors and the reviewers. Any product that may be evaluated in this article, or claim that may be made by its manufacturer, is not guaranteed or endorsed by the publisher.
Supplementary material
The Supplementary Material for this article can be found online at: https://www.frontiersin.org/articles/10.3389/fevo.2024.1441397/full#supplementary-material
References
Álvarez-Noriega M., Burgess S. C., Byers J. E., Pringle J. M., Wares J. P., Marshall D. J. (2020). Global biogeography of marine dispersal potential. Nat. Ecol. Evol. 4, 1196–1203. doi: 10.1038/s41559-020-1238-y
Améziane N., Eléaume M., Hemery L., Monniot F., Hemery A., Hautecoeur M., et al. (2011). Biodiversity of the benthos off Kerguelen Islands: overview and perspectives. Cybium 35 (SP), 157–167. doi: 10.26028/cybium/2011-35SP-016
Assis J., Alberto F., Macaya E. C., Castilho Coelho N., Faugeron S., Pearson G. A., et al. (2023). Past climate-driven range shifts structuring intraspecific biodiversity levels of the giant kelp (Macrocystis pyrifera) at global scales. Sci. Rep. 13, 12046. doi: 10.1038/s41598-023-38944-7
Ayre D. J., Minchinton T. E., Perrin C. (2009). Does life history predict past and current connectivity for rocky intertidal invertebrates across a marine biogeographic barrier? Mol. Ecol. 18, 1887–1903. doi: 10.1111/j.1365-294X.2009.04127.x
Barton N. H., Slatkin M. (1986). A quasi-equilibrium theory of the distribution of rare alleles in a subdivided population. Heredity 56, 409–415. doi: 10.1038/hdy.1986.63
Belkhir K., Borsa P., Chikhi L., Raufaste N., Bonhomme F. (2004). GENETIX 4.05, logiciel sous Windows TM pour la génétique des populations. Laboratoire Génome Populations Interactions CNRS UMR, 5000, Université de Montpellier II, Montpellier (France).
Bertola L. D., Boehm J. T., Putman N. F., Xue A. T., Robinson J. D., Harris S., et al. (2020). Asymmetrical gene flow in five co-distributed syngnathids explained by ocean currents and rafting propensity. Proc. R. Soc. B: Biol. Sci. 287, 20200657. doi: 10.1098/rspb.2020.0657
Blanco M., Ospina-Álvarez A., Navarrete S. A., Fernández M. (2019). Influence of larval traits on dispersal and connectivity patterns of two exploited marine invertebrates in central Chile. Mar. Ecol. Prog. Ser. 612, 43–64. doi: 10.3354/meps12870
Boulanger E., Benestan L., Guerin P.-E., Dalongeville A., Mouillot D., Manel S. (2022). Climate differently influences the genomic patterns of two sympatric marine fish species. J. Anim. Ecol. 91, 1180–1195. doi: 10.1111/1365-2656.13623
Bussolini L. T., Waters J. M. (2015). Genetic analyses of rafted macroalgae reveal regional oceanographic connectivity patterns. J. Biogeography 42, 1319–1326. doi: 10.1111/jbi.12491
Carlton J. T., Chapman J. W., Geller J. B., Miller J. A., Carlton D. A., McCuller M. I., et al. (2017). Tsunami-driven rafting: transoceanic species dispersal and implications for marine biogeography. Science 357, 1402–1406. doi: 10.1126/science.aao1498
Chapman C. C., Lea M.-A., Meyer A., Sallée J.-B., Hindell M. (2020). Defining Southern Ocean fronts and their influence on biological and physical processes in a changing climate. Nat. Climate Change 10, 209–219. doi: 10.1038/s41558-020-0705-4
Chhatre V. E., Emerson K. J. (2017). StrAuto: automation and parallelization of STRUCTURE analysis. BMC Bioinf. 18, 192. doi: 10.1186/s12859-017-1593-0
Clarke A., Crame J. A. (2010). Evolutionary dynamics at high latitudes: speciation and extinction in polar marine faunas. Philos. Trans. R. Soc. B: Biol. Sci. 365, 3655–3666. doi: 10.1098/rstb.2010.0270
Cortez T., Amaral R. V., Sobral-Souza T., Andrade S. C. S. (2021). Genome-wide assessment elucidates connectivity and the evolutionary history of the highly dispersive marine invertebrate Littoraria flava (Littorinidae: Gastropoda). Biol. J. Linn. Soc. 133, 999–1015. doi: 10.1093/biolinnean/blab055
Cowen R. K., Sponaugle S. (2009). Larval dispersal and marine population connectivity. Annu. Rev. Mar. Sci. 1, 443–466. doi: 10.1146/annurev.marine.010908.163757
Cumming R. A., Nikula R., Spencer H. G., Waters J. M. (2014). Transoceanic genetic similarities of kelp-associated sea slug populations: long-distance dispersal via rafting? J. Biogeography 41, 2357–2370. doi: 10.1111/jbi.12376
D’Aloia C. C., Bogdanowicz S. M., Francis R. K., Majoris J. E., Harrison R. G., Buston P. M. (2015). Patterns, causes, and consequences of marine larval dispersal. Proc. Natl. Acad. Sci. 112, 13940–13945. doi: 10.1073/pnas.1513754112
Dayrat B., Goulding T. C., White T. R. (2014). Diversity of Indo-West Pacific Siphonaria (Mollusca: Gastropoda: Euthyneura). Zootaxa 3779, 246. doi: 10.11646/zootaxa.3779.2.7
De Broyer C., Koubbi P., Scientific Commitee on Antarctic Research (2014). “Chapter 1.1. The biogeography of the Southern Ocean,” in Biogeographic Atlas of the Southern Ocean. Eds. De Broyer C., Koubbi P., Griffiths H. J., Raymond B., d’Udekem d’Acoz C., Van de Putte A. P., Danis B. (ublished by The Scientific Committee on Antarctic Research, Scott Polar reseach Institute, Cambridge), 1–9.
Do C., Waples R. S., Peel D., Macbeth G. M., Tillett B. J., Ovenden J. R. (2014). NeEstimator v2: re-implementation of software for the estimation of contemporary effective population size (Ne) from genetic data. Mol. Ecol. Resour. 14, 209–214. doi: 10.1111/1755-0998.12157
Donald K. M., McCulloch G. A., Dutoit L., Spencer H. G. (2020). Population structure of the New Zealand whelk, Cominella glandiformis (Gastropoda: Buccinidae), suggests sporadic dispersal of a direct developer. Biol. J. Linn. Soc. 130, 49–60. doi: 10.1093/biolinnean/blaa033
Earl D. A., vonHoldt B. M. (2012). STRUCTURE HARVESTER: A website and program for visualizing STRUCTURE output and implementing the evanno method. Conserv. Genet. Resour. 4, 359–615. doi: 10.1007/s12686-011-9548-7
Esser E. A., Pringle J. M., Byers J. E. (2023). Neither larval duration nor dispersal distance predict spatial genetic diversity in planktonic dispersing species. Mar. Ecol. Prog. Ser. 721, 161–167. doi: 10.3354/meps14419
Evanno G., Regnaut S., Goudet J. (2005). Detecting the number of clusters of individuals using the software structure: A simulation study. Mol. Ecol. 14, 2611–2620. doi: 10.1111/j.1365-294X.2005.02553.x
Excoffier L., Lischer H. E. L. (2010). Arlequin suite ver 3.5: A new series of programs to perform population genetics analyses under linux and windows. Mol. Ecol. Resour. 10, 564–567. doi: 10.1111/j.1755-0998.2010.02847.x
Féral J.-P., Verlaque M., Rosenfeld S., Poulin E., Chenuil A., Saucède T. (2021). The marine vegetation of the Kerguelen Islands: history of scientific campaigns, inventory of the flora and first analysis of its biogeographical affinities. Cryptogamie Algologie 42, 173–216. doi: 10.5252/cryptogamie-algologie2021v42a12
Fleming A. M., Dohner M. M., Phillips N. E., Ritchie P. A. (2018). Genetic Connectivity among Populations of Two Congeneric Direct-Developing Whelks Varies across Spatial Scales. New Z. J. Mar. Freshw. Res. 52, 100–117. doi: 10.1080/00288330.2017.1340899
Foll M., Gaggiotti O. (2008). A genome-scan method to identify selected loci appropriate for both dominant and codominant markers: A Bayesian perspective. Genetics 180, 977–993. doi: 10.1534/genetics.108.092221
Fraïsse C., Haguenauer A., Gérard K., Anh-Thu Weber A., Bierne N., Chenuil A. (2021). Fine-grained habitat-associated genetic connectivity in an admixed population of mussels in the small isolated Kerguelen Islands. Peer Community J. 1, e10. doi: 10.24072/pcjournal.18
Fraser C. I., Dutoit L., Morrison A. K., Pardo L. M., Smith S. D. A., Pearman W. S., et al. (2022). Southern hemisphere coasts are biologically connected by frequent, long-distance rafting events. Curr. Biol. 32, 3154–3160.e3. doi: 10.1016/j.cub.2022.05.035
Fraser C. I., Morrison A. K., A.M.c.M. Hogg E. C., van Sebille E., Ryan P. G., Padovan A., et al. (2018). Antarctica’s ecological isolation will be broken by storm-driven dispersal and warming. Nat. Climate Change 8, 704–785. doi: 10.1038/s41558-018-0209-7
Fraser C. I., Morrison A., Olmedo-Rojas P. (2020a). “Biogeographic processes influencing Antarctic and sub-Antarctic seaweeds,” in Antarctic seaweeds: diversity, adaptation and ecosystem services. Ed. Gómez and P. Huovinen I. (Springer International Publishing, Cham), 43–57. doi: 10.1007/978-3-030-39448-6_3
Fraser C. I., Nikula R., Spencer H. G., Waters J. M. (2009). Kelp genes reveal effects of subAntarctic sea ice during the Last Glacial Maximum. Proc. Natl. Acad. Sci. United States America 106, 3249–3253. doi: 10.1073/pnas.0810635106
Fraser C. I., Nikula R., Waters J. M. (2010). Oceanic rafting by a coastal community. Proc. R. Soc. B: Biol. Sci. 278, 649–655. doi: 10.1098/rspb.2010.1117
Fraser C. I., Velásquez M., Nelson W. A., Macaya E. C., Hay C. H. (2020b). The biogeographic importance of buoyancy in macroalgae: A case study of the southern bull-Kelp genus Durvillaea (Phaeophyceae), including descriptions of two new species. J. Phycology 56, 23–365. doi: 10.1111/jpy.12939
Frisk M. G., Jordaan A., Miller T. J. (2014). Moving beyond the current paradigm in marine population connectivity: are adults the missing link. Fish Fisheries 15, 242–545. doi: 10.1111/faf.12014
Gaeta J., Acevedo I., López-Márquez V., Freitas R., Cruz R., Maggioni R., et al. (2020). Genetic differentiation among atlantic island populations of the brown spiny lobster Panulirus echinatus (Decapoda: Palinuridae). Aquat. Conservation: Mar. Freshw. Ecosyst. 30, 868–881. doi: 10.1002/aqc.3297
Gaines S. D., Gaylord B., Gerber L. R., Hastings A., Kinlan B. P. (2007). Connecting places: the ecological consequences of dispersal in the sea. Oceanography 20, 90–99. doi: 10.5670/oceanog
Galaska M. P., Sands C. J., Santos S. R., Mahon A. R., Halanych K. M. (2017). Crossing the divide: admixture across the Antartic Polar Front revealed by the brittle star Astrotoma agassizii. Biol. Bull. 232, 198–211. doi: 10.1086/693460
Gélin P., Pirog A., Fauvelot C., Magalon H. (2018). High genetic differentiation and low connectivity in the coral Pocillopora damicornis type β at different spatial scales in the Southwestern Indian Ocean and Tropical Southwestern Pacifi. Mar. Biol. 165, 167. doi: 10.1007/s00227-018-3428-6
Gillespie R. G., Baldwin B. G., Waters J. M., Fraser C. I., Nikula R., Roderick G. K. (2012). Long-distance dispersal: A framework for hypothesis testing. Trends Ecol. Evol. 27, 47–56. doi: 10.1016/j.tree.2011.08.009
Gillespie R. G., Roderick G. K. (2014). Evolution: geology and climate drive diversification. Nature 509, 297–298. doi: 10.1038/509297a
Glasser N., Jansson K. (2008). The glacial map of southern South America. J. Maps 4, 175–196. doi: 10.4113/jom.2008.1020
Gonzalez E. B., Knutsen H., Jorde P. E. (2016). Habitat discontinuities separate genetically divergent populations of a rocky shore marine fish. PloS One 11, e0163052. doi: 10.1371/journal.pone.0163052
González-Wevar C. A., Hüne M., Cañete J. I., Mansilla A., Nakano T., Poulin E. (2012). Towards a model of postglacial biogeography in shallow marine species along the Patagonian Province: lessons from the limpet Nacella magellanica (Gmelin, 1791). BMC Evolutionary Biol. 12, 139. doi: 10.1186/1471-2148-12-139
González-Wevar C. A., Segovia N. I., Rosenfeld S., Noll D., Maturana C. S., Hüne M., et al. (2021). Contrasting biogeographical patterns in Margarella (Gastropoda: Calliostomatidae: Margarellinae) across the Antarctic polar front. Mol. Phylogenet. Evol. 156, 107039. doi: 10.1016/j.ympev.2020.107039
González-Wevar C. A., Segovia N. I., Rosenfeld S., Ojeda J., Hüne M., Naretto J., et al. (2018). Unexpected absence of island endemics: long-distance dispersal in higher latitude sub-Antarctic Siphonaria (Gastropoda: Euthyneura) species. J. Biogeography 45, 874–884. doi: 10.1111/jbi.13174
Green M. E., Appleyard S. A., White W. T., Tracey S. R., Heupel M. R., Ovenden J. R. (2022). Updated connectivity assessment for the scalloped hammerhead (Sphyrna lewini) in Pacific and Indian oceans using a multi-marker genetic approach. Fisheries Res. 251, 106305. doi: 10.1016/j.fishres.2022.106305
Griffiths H. J., Barnes D. K.A., Linse K. (2009). Towards a generalized biogeography of the Southern Ocean benthos. J. Biogeography 36, 162–775. doi: 10.1111/j.1365-2699.2008.01979.x
Güller M., Puccinelli E., Zelaya D. G. (2020). The Antarctic Circumpolar Current as a dispersive agent in the Southern Ocean: evidence from bivalves. Mar. Biol. 167, 143. doi: 10.1007/s00227-020-03746-2
Güller M., Zelaya D. G., Ituarte C. (2015). How many Siphonaria species (Gastropoda: Euthyneura) live in southern South America. J. Molluscan Stud. 82 (1), eyv036. doi: 10.1093/mollus/eyv036
Halanych K. M., Mahon A. R. (2018). Challenging dogma concerning biogeographic patterns of Antarctica and the Southern Ocean. Annu. Rev. Ecology Evolution Systematics 49, 355–378. doi: 10.1146/annurev-ecolsys-121415-032139
Haye P. A., Segovia N. I., Muñoz-Herrera N. C., Gálvez F. E., Martínez A., Meynard A., et al. (2014). Phylogeographic structure in benthic marine invertebrates of the Southeast Pacific coast of Chile with differing dispersal potential. PloS One 9, 88613. doi: 10.1371/journal.pone.0088613
Haye P. A., Varela A. I., Thiel M. (2012). Genetic signatures of rafting dispersal in algal-dwelling brooders Limnoria spp. (Isopoda) along the SE Pacific (Chile). Mar. Ecol. Prog. Ser. 455, 111–122. doi: 10.3354/meps09673
Hernawan U. E., Lavery P. S., Kendrick G. A., van Dijk K. J., Ulumuddin Y. I., Triandiza T., et al. (2021). Predictors of marine genetic structure in the Indo-Australian Archipelago. Regional Stud. Mar. Sci. 47, 101919. doi: 10.1016/j.rsma.2021.101919
Highsmith R. (1985). Floating and algal rafting as potential dispersal mechanisms in brooding invertebrates. Mar. Ecol. Prog. Ser. 25, 169–179. doi: 10.3354/meps025169
Hilário A., Metaxas A., Gaudron S. M., Howell K. L., Mercier A., Mestre N. C., et al. (2015). Estimating dispersal distance in the deep sea: challenges and applications to marine reserves. Front. Mar. Sci. 2. doi: 10.3389/fmars.2015.00006
Hodgson D. A., Graham A. G. C., Roberts S. J., Bentley M. J., Cofaigh C.Ó., Verleyen E., et al. (2014). Terrestrial and submarine evidence for the extent and timing of the Last Glacial Maximum and the onset of deglaciation on the maritime-Antarctic and sub-Antarctic islands. Quaternary Sci. Reviews Reconstruction Antarctic Ice Sheet Deglaciation (RAISED) 100, 137–158. doi: 10.1016/j.quascirev.2013.12.001
Hunt G. L., Drinkwater K. F., Arrigo K., Berge J., Daly K. L., Danielson S., et al. (2016). Advection in polar and sub-polar environments: impacts on high latitude marine ecosystems. Prog. Oceanography 149, 40–81. doi: 10.1016/j.pocean.2016.10.004
Johansson M. L., Banks M. A., Glunt K. D., Hassel-Finnegan H. M., Buonaccorsi V. P. (2008). Influence of habitat discontinuity, geographical distance, and oceanography on fine-scale population genetic structure of copper rockfish (Sebastes caurinus). Mol. Ecol. 17, 3051–3061. doi: 10.1111/j.1365-294X.2008.03814.x
Jokiel P. L. (1990). Long-distance dispersal by rafting: reemergence of an old hypothesis. Endeavour 14, 66–73. doi: 10.1016/0160-9327(90)90074-2
Jombart T. (2008). Adegenet: A R package for the multivariate analysis of genetic markers. Bioinformatics. Bioinformatics 24, 1403–1405. doi: 10.1093/bioinformatics/btn129
Kalinowski S. T. (2005). HP-RARE 1.0: A computer program for performing rarefaction on measures of allelic richness. Mol. Ecol. Notes 5, 187–189. doi: 10.1111/j.1471-8286.2004.00845.x
Kopelman N. M., Mayzel J., Jakobsson M., Rosenberg N. A., Mayrose I. (2015). CLUMPAK: A program for identifying clustering modes and packaging population structure inferences across K. Mol. Ecol. Resour. 15, 1179–1191. doi: 10.1111/1755-0998.12387
Koubbi P., De Broyer C., Griffiths H. J., Raymond B., d’Udekem d’Acoz C., Van de Putte A. P., et al. (2014). “Chapter 12. Conclusions: present and future of Southern Ocean biogeography,” in Biogeographic Atlas of the Southern Ocean. Eds. De Broyer C., Koubbi P., Griffiths H. J., Raymond B., d’Udekem d’Acoz C., Van de Putte A. P., Danis B., et al (Published by The Scientific Committee on Antarctic Research, Scott Polar reseach Institute, Cambridge), 1–9.
Koubbi P., Guinet C., Alloncle N., Améziane N., Azam C. S., Baudena A., et al. (2016). Ecoregionalisation of the Kerguelen and Crozet islands oceanic zone. Part I: introduction and Kerguelen oceanic zone. CCAMLR Report WG-EMM- 16/43. doi: 10.13140/RG.2.2.17278.18246
Kunvar S., Czarnomska S., Pertoldi C., Tokarska M.a (2021). In search of species-Specific SNPs in a non-Model animal (European bison (Bison bonasus))-Comparison of de novo and reference-Based integrated pipeline of STACKS using genotyping-by-Sequencing (GBS) data. Animals: Open Access J. MDPI 11, 22265. doi: 10.3390/ani11082226
Larsson J., Lind E. E., Corell H., Grahn M., Smolarz K., Lönn M. (2017). Regional genetic differentiation in the blue mussel from the Baltic sea area. Estuarine Coast. Shelf Science Understanding Baltic Sea 195, 98–109. doi: 10.1016/j.ecss.2016.06.016
Lau S. C. Y., Strugnell J. M., Sands C. J., Silva C. N. S., Wilson N. G. (2023). Genomic insights of evolutionary divergence and life history innovations in Antarctic brittle stars. Mol. Ecol. 32, 3382–3402. doi: 10.1111/mec.16951
Layton C., Vermont H., Beggs H., Brassington G. B., Burke A. D., Hepburn L., et al. (2022). Giant kelp rafts wash ashore 450 Km from the nearest populations and against the dominant ocean current. Ecology 103, e3795. doi: 10.1002/ecy.3795
Ledoux J.-B., Tarnowska K., Gérard K., Lhuillier E., Jacquemin B., Weydmann A., et al. (2012). Fine-scale spatial genetic structure in the brooding sea urchin Abatus cordatus suggests vulnerability of the Southern Ocean marine invertebrates facing global change. Polar Biol. 35, 611–623. doi: 10.1007/s00300-011-1106-y
Leese F., Agrawal S., Held C. (2010). Long-Distance Island Hopping without Dispersal Stages: Transportation across Major Zoogeographic Barriers in a Southern Ocean Isopod. Naturwissenschaften 97, 583–594. doi: 10.1007/s00114-010-0674-y
Leese F., Kop A., Wägele J.-W., Held C. (2008). Cryptic speciation in a benthic isopod from Patagonian and Falkland Island waters and the impact of glaciations on its population structure. Front. Zoology 5, 19. doi: 10.1186/1742-9994-5-19
Leiva C., Taboada S., Kenny N. J., Combosch D., Giribet G., Jombart T., et al. (2019). Population substructure and signals of divergent adaptive selection despite admixture in the sponge Dendrilla antarctica from shallow waters surrounding the Antarctic Peninsula. Mol. Ecol. 28, 3151–3170. doi: 10.1111/mec.15135
López B. A., Macaya E. C., Tala F., Tellier F., Thiel M. (2017). The variable routes of rafting: stranding dynamics of floating bull kelp Durvillaea antarctica (Fucales, Phaeophyceae) on beaches in the SE Pacific. J. Phycology 53, 70–84. doi: 10.1111/jpy.12479
Lowe W. H., Allendorf F. W. (2010). What can genetics tell us about population connectivity? Mol. Ecol. 19, 3038–3051. doi: 10.1111/j.1365-294X.2010.04688.x
Lu F., Lipka A. E., Glaubitz J., Elshire R., Cherney J. H., Casler M. D., et al. (2013). Switchgrass genomic diversity, ploidy, and evolution: novel insights from a network-based SNP discovery protocol. PloS Genet. 9, e10032155. doi: 10.1371/journal.pgen.1003215
Macaya E. C., Tala F., Hinojosa I. A., Rothäusler E. (2020). “Detached seaweeds as important dispersal agents across the Southern Ocean,” in Antarctic seaweeds: diversity, adaptation and ecosystem services. Ed. Gómez and P. Huovinen I. (New York: Springer International Publishing), 59–81. doi: 10.1007/978-3-030-39448-6_4
Macaya E. C., Zuccarello G. C. (2010). Genetic structure of the giant kelp Macrocystis pyrifera along the Southeastern Pacific. Mar. Ecol. Prog. Ser. 420, 103–112. doi: 10.3354/meps08893
Maes T., De Corte Z., Vangestel C., Virgilio M., Smitz N., Djuikwo-Teukeng F. F., et al. (2022). Large-scale and small-scale population genetic structure of the medically important gastropod species Bulinus truncatus (Gastropoda, Heterobranchia). Parasites Vectors 15, 328. doi: 10.1186/s13071-022-05445-x
Manel S., Boulanger E., Benestan L., Mouillot D., Dalongeville A. (2023). Revisiting long-distance dispersal in a coastal marine fish. Ecography 2023, e06867. doi: 10.1111/ecog.06867
Meirmans P. G. (2020). Genodive version 3.0: easy-to-use software for the analysis of genetic data of diploids and polyploids. Mol. Ecol. Resour. 20, 1126–1131. doi: 10.1111/1755-0998.13145
Mendes C. B., Cortez T., Santos C. S. G., Sobral-Souza T., Santos A. D., Sasaki D. K., et al. (2022). Seascape genetics in a polychaete worm: disentangling the roles of a biogeographic barrier and environmental factors. J. Biogeography 49, 2296–2308. doi: 10.1111/jbi.14504
Montecinos A., Broitman B. R., Faugeron S., Haye P. A., Tellier F., Guillemin. M. L. (2012). Species Replacement along a Linear Coastal Habitat: Phylogeography and Speciation in the Red Alga Mazzaella laminarioides along the South East Pacific. BMC Evolutionary Biol. 12, 975. doi: 10.1186/1471-2148-12-97
Moon K. L., Chown S. L., Fraser C. I. (2017). Reconsidering connectivity in the sub-Antarctic. Biol. Rev. 92, 2164–2181. doi: 10.1111/brv.12327
Moore J. M., Carvajal J. I., Rouse G. W., Wilson N. G. (2018). The Antarctic Circumpolar Current isolates and connects: structured circumpolarity in the sea star Glabraster antarctica. Ecol. Evol. 8, 10621–10633. doi: 10.1002/ece3.4551
Morrissey D., Goodall J., Castilho R., Cameron T. C., Taylor M. L. (2022). Population genomics reveals a single semi-continuous population of a commercially exploited marine gastropod. Fisheries Res. 254, 106418. doi: 10.1016/j.fishres.2022.106418
Narum S. R. (2006). Beyond bonferroni: less conservative analyses for conservation genetics. Conserv. Genet. 7, 783–787. doi: 10.1007/s10592-005-9056-y
Nikula R., Fraser C., Spencer H., Waters J. (2010). Circumpolar dispersal by rafting in two subAntarctic kelp-dwelling crustaceans. Mar. Ecol. Prog. Ser. 405, 221–230. doi: 10.3354/meps08523
Palumbi S. R. (1994). Genetic divergence, reproductive isolation, and marine speciation. Annu. Rev. Ecol. Systematics 25, 547–572. doi: 10.1146/annurev.es.25.110194.002555
Park Y.-H., Gasco N., Duhamel G. (2008). Slope currents around the Kerguelen Islands from demersal longline fishing records. Geophysical Res. Lett. 35, L09604. doi: 10.1029/2008GL033660
Pascual M., Rives B., Schunter C., Macpherson E. (2017). Impact of life history traits on gene flow: A multispecies systematic review across oceanographic barriers in the Mediterranean Sea. PloS One 12, 176419. doi: 10.1371/journal.pone.0176419
Pazmiño D. A., Maes G. E., Simpfendorfer C. A., Salinas-de-León P., van Herwerden L. (2017). Genome-wide SNPs reveal low effective population size within confined management units of the highly vagile galapagos shark (Carcharhinus galapagensis). Conserv. Genet. 18, 1151–1163. doi: 10.1007/s10592-017-0967-1
Pfaller J. B., Payton A. C., Bjorndal K. A., Bolten A. B., McDaniel S. F. (2019). Hitchhiking the high seas: global genomics of rafting crabs. Ecol. Evol. 9, 957–974. doi: 10.1002/ece3.4694
Pinsky M. L., Saenz-Agudelo P., Salles O. C., Almany G. R., Bode M., Berumen M. L., et al. (2017). Marine dispersal scales are congruent over evolutionary and ecological time. Curr. Biol. 27, 149–154. doi: 10.1016/j.cub.2016.10.053
Post A. L., Meijers A. J. S., Fraser A. D., Meiners K. M., Ayers J., Bindoff N. L., et al. (2014). “Chapter 4. Environmental setting,” in Biogeographic Atlas of the Southern Ocean. Eds. De Broyer C., Koubbi P., Griffiths H. J., Raymond B., d’Udekem d’Acoz C., Van de Putte A. P., Danis B., et al (Published by The Scientific Committee on Antarctic Research, Scott Polar research Institute, Cambridge), 46–64.
Poulin E., Féral J.-P. (1995). Pattern of spatial distribution of a brood-protecting schizasterid echinoid, Abatus cordatus, endemic to the Kerguelen Islands. Mar. Ecol. Prog. Ser. 118, 179–186. doi: 10.3354/meps118179
Pritchard J. K., Stephens M., Donnelly P. (2000). Inference of population structure using multilocus genotype data. Genetics 155, 945–959. doi: 10.1093/genetics/155.2.945
Quilty P. G. (2007). Origin and evolution of the sub-Antarctic islands: the foundation. Papers and Proceedings of the Royal Society of Tasmania, 14(1), 35–58. doi: 10.26749/rstpp.141.1.35
Quintero-Galvis J. F., Ocampo-Zuleta K., Castro L. R., Narváez-Barandica J. C. (2023). Limited population genetic structure in the littoral gastropod Nerita tessellata (Neritimorpha, Neritidae) suggests high dispersal across the Caribbean Sea. J. Exp. Mar. Biol. Ecol. 568, 151942. doi: 10.1016/j.jembe.2023.151942
Rabassa J., Coronato A. M., Martínez O. (2011). Late Cenozoic glaciations in Patagonia and Tierra del Fuego: an updated review. Biol. J. Linn. Soc. 103, 316–335. doi: 10.1111/j.1095-8312.2011.01681.x
Rabassa J., Coronato A. M., Salemme M. (2005). Chronology of the Late Cenozoic Patagonian glaciations and their correlation with biostratigraphic units of the Pampean region (Argentina). J. South Am. Earth Sciences Quaternary Paleontology biostratigraphy South. South Afr. 20, 81–103. doi: 10.1016/j.jsames.2005.07.004
Rambaut A., Drummond A. J., Xie D., Baele G., Suchard M. A. (2018). Posterior summarization in bayesian phylogenetics using tracer 1.7. Systematic Biol. 67, 901–904. doi: 10.1093/sysbio/syy032
Rousset F. (2008). Genepop’007: A complete re-implementation of the genepop software for windows and linux. Mol. Ecol. Resour. 8, 103–106. doi: 10.1111/j.1471-8286.2007.01931.x
Rovelli V., Ruiz-González A., Vignoli L., Macale D., Buono V., Davoli F., et al. (2019). Genotyping-by-sequencing (GBS) of large amphibian genomes: A comparative study of two non-model species endemic to Italy. Anim. Biol. 69, 307–326. doi: 10.1163/15707563-00001094
Segovia N. I., González-Wevar C. A., Naretto J., Rosenfeld S., Brickle P., Hüne M., et al. (2022). The right tool for the right question: contrasting biogeographic patterns in the notothenioid fish Harpagifer spp. along the Magellan Province. Proc. R. Soc. B: Biol. Sci. 289, 20212738. doi: 10.1098/rspb.2021.2738
Selkoe K. A., D’Aloia C., Crandall E., Iacchei M., Liggins L., Puritz J. B., et al. (2016). A decade of seascape genetics: contributions to basic and applied marine connectivity. Mar. Ecol. Prog. Ser. 554, 1–19. doi: 10.3354/meps11792
Simkanin C., Carlton J. T., Steves B., Fofonoff P., Nelson J. C., Clarke Murray C., et al. (2019). Exploring potential establishment of marine rafting species after transoceanic long-distance dispersal. Global Ecol. Biogeography 28, 588–600. doi: 10.1111/geb.12878
Simpson R. D. (1976). Physical and biotic factors limiting the distribution and abundance of littoral molluscs on Macquarje island (sub-Antarctic). J. Exp. Mar. Biol. Ecol. 21, 11–49. doi: 10.1016/0022-0981(76)90067-8
Sjöqvist C., Godhe A., Jonsson P. R., Sundqvist L., Kremp A. (2015). Local adaptation and oceanographic connectivity patterns explain genetic differentiation of a marine diatom across the North Sea-Baltic Sea salinity gradient. Mol. Ecol. 24, 2871–2885. doi: 10.1111/mec.13208
Slatkin M. (1985). Gene flow in natural populations. Annual review of ecology and systematics. Annu. Rev. Ecol. Evol. Syst. 16, 393–430. doi: 10.1146/annurev.es.16.110185.002141
Smith S. D. A. (2002). Kelp rafts in the Southern Ocean. Global Ecol. Biogeography 11, 67–69. doi: 10.1046/j.1466-822X.2001.00259.x
Stevens C. L., Hurd C. L., Smith M. J. (2002). Field measurement of the dynamics of the bull kelp Durvillaea antarctica (Chamisso) Heriot. J. Exp. Mar. Biol. Ecol. 2, 147–171. doi: 10.1016/S0022-0981(02)00007-2
Teske P. R., Golla T. R., Sandoval-Castillo J., Emami-Khoyi A., van der Lingen C. D., Heyden S.v. d., et al. (2018). Mitochondrial DNA is unsuitable to test for isolation by distance. Sci. Rep. 8, 84485. doi: 10.1038/s41598-018-25138-9
Thiel M., Gutow L. (2005). The ecology of rafting in the marine environment. I. The floating subtrata. Oceanography Mar. Biology: Annu. Rev. 42, 181–264. doi: 10.1201/9780203507810.ch6
Trickey J. S., Thiel M., Waters J. M. (2016). Transoceanic dispersal and cryptic diversity in a cosmopolitan rafting nudibranch. Invertebrate Systematics 30, 290–301. doi: 10.1071/IS15052
Trovant B., Orensanz J. M., Ruzzante D. E., Stotz W., Basso N. G. (2015). Scorched mussels (Bivalvia: Mytilidae: Brachidontinae) from the temperate coasts of South America: phylogenetic relationships, trans-Pacific connections and the footprints of Quaternary glaciations. Mol. Phylogenet. Evol. 82, 60–74. doi: 10.1016/j.ympev.2014.10.002
Vucetich J. A., Waite T. A. (2000). Is one migrant per generation sufficient for the genetic management of fluctuating populations? Anim. Conserv. 3, 261–266. doi: 10.1111/j.1469-1795.2000.tb00111.x
Waples R. S. (1987). A multispecies approach to the analysis of gene flow in marine shore fishes. Evolution 41, 385–400. doi: 10.1111/j.1558-5646.1987.tb05805.x
Waples R. S., Do C. (2008). Ldne: A program for estimating effective population size from data on linkage disequilibrium. Mol. Ecol. Resour. 8, 753–756. doi: 10.1111/j.1755-0998.2007.02061.x
Waters J. M., Fraser C. I., Hewitt G. M. (2013). Founder takes all: density-dependent processes structure biodiversity. Trends Ecol. Evol. 28, 78–85. doi: 10.1016/j.tree.2012.08.024
Waters J. M., King T. M., Fraser C. I., Craw D. (2018a). An integrated ecological, genetic and geological assessment of long-distance dispersal by invertebrates on kelp rafts. Front. Biogeography 10, (3–4). doi: 10.21425/F5FBG40888
Waters J. M., King T. M., Fraser C. I., Garden C. (2018b). Rafting dispersal in a brooding southern sea star (Asteroidea : Anasterias). Invertebrate Systematics 32, 253–258. doi: 10.1071/IS17037
Weersing K., Toonen R. J. (2009). Population genetics, larval dispersal, and connectivity in marine systems. Mar. Ecol. Prog. Ser. 393, 1–12. doi: 10.3354/meps08287
White J. W., Carr M., Caselle J., Washburn L., Woodson C. B., Palumbi S., et al. (2019). Connectivity, dispersal, and recruitment: connecting benthic communities and the coastal ocean. Oceanography 32, 50–59. doi: 10.5670/oceanog.2019.310
Wilson G. A., Rannala B. (2003). Bayesian inference of recent migration rates using multilocus genotypes. Genetics 163, 1177–1915. doi: 10.1093/genetics/163.3.1177
Winston J. E. (2012). Dispersal in marine organisms without a pelagic larval phase. Integr. Comp. Biol. 52, 447–457. doi: 10.1093/icb/ics040
Wright S. (1931). Evolution in mendelian populations. Genetics 16, 97–159. doi: 10.1093/genetics/16.2.97
Zabala S., Averbuj A., Bigatti G., Penchaszadeh P. E. (2020). Embryonic development of the false limpet Siphonaria lateralis from Atlantic Patagonia. Invertebrate Biol. 139, e12276. doi: 10.1111/ivb.12276
Zbawicka M., Gardner J. P. A., Wenne R. (2019). Cryptic diversity in smooth-shelled mussels on Southern Ocean islands: connectivity, hybridisation and a marine invasion. Front. Zoology 16, 32. doi: 10.1186/s12983-019-0332-y
Zheng X., Levine D., Shen J., Gogarten S. M., Laurie C., Weir B. S. (2012). A high-performance computing toolset for relatedness and principal component analysis of SNP data. Bioinf. (Oxford England) 28, 3326–3328. doi: 10.1093/bioinformatics/bts606
Keywords: long distance dispersal, sub-Antarctic, rafting, Antarctic Circumpolar Current, benthic protected development, Genotyping-by-Sequencing, gene flow
Citation: Millán-Medina C, Lizama M, Saucède T, Poulin E, Segovia NI and González-Wevar C (2024) Does rafting promote contemporary gene flow? Global and regional patterns of population genetic diversity and structure on the false limpet Siphonaria lateralis in the Southern Ocean. Front. Ecol. Evol. 12:1441397. doi: 10.3389/fevo.2024.1441397
Received: 31 May 2024; Accepted: 21 August 2024;
Published: 09 September 2024.
Edited by:
Peter Convey, British Antarctic Survey (BAS), United KingdomReviewed by:
Aurélien De Jode, University of Gothenburg, SwedenLuis Amador, University of New Mexico, United States
Copyright © 2024 Millán-Medina, Lizama, Saucède, Poulin, Segovia and González-Wevar. This is an open-access article distributed under the terms of the Creative Commons Attribution License (CC BY). The use, distribution or reproduction in other forums is permitted, provided the original author(s) and the copyright owner(s) are credited and that the original publication in this journal is cited, in accordance with accepted academic practice. No use, distribution or reproduction is permitted which does not comply with these terms.
*Correspondence: Marcelo Lizama, bWFyY2Vsby5saXphbWFAdWcudWNoaWxlLmNs
†These authors have contributed equally to this work