- 1Alaska Department of Fish and Game, Kenai Moose Research Center, Soldotna, AK, United States
- 2Alaska Department of Fish and Game, Soldotna, AK, United States
- 3Alaska Department of Fish and Game, Homer, AK, United States
- 4Alaska Department of Fish and Game, Anchorage, AK, United States
- 5U.S. Fish and Wildlife Service, Kenai National Wildlife Refuge, Soldotna, AK, United States
Wildlife contend with seasonal fluctuations in resource availability and have adapted survival and reproductive strategies to overcome resource limitations. Many northern ungulates are adapted to a dynamic nutritional landscape and rely on somatic reserves accumulated during the short growing season. Moose (Alces alces) populations in the boreal forest respond to variation in their nutritional landscapes that quickly change after wildland fires. We tested associations between somatic energy reserves of female moose and a suite of factors relevant to energy demands and nutrient availability after landscape scale wildland fires on the Kenai Peninsula, Alaska. From 2015–2022, we immobilized 97 individual, adult moose (n=163 early winter; n=98 late winter) and collected over 223,000 GPS locations. We evaluated if somatic energy reserves of cow moose were influenced by endogenous or exogenous energy demands, or access to moose forage to accumulate energy reserves. Cows that gave birth and lost their neonate(s) early in the summer had more early winter body fat (14.39% ± 0.24SE) compared with cows that gave birth and the neonate survived to 4-months-old (10.59% ± 0.34SE). Body fat measured in early winter was positively correlated with home ranges of cows during summer with a higher percent cover of aspen forage. Late winter body fat of cow moose was negatively correlated with home ranges with higher percent cover of aspen forage, but positively correlated with home ranges with higher percent cover of willows and shoulder season forages. Our results highlight that a suite of plant species and seral states is needed across the landscape for moose to accumulate and moderate the loss of somatic energy reserves over the year. Furthermore, our results emphasize the importance of shoulder season forages for moose when snow depth is low. Managing the nutritional landscape of the boreal forest through interagency wildland fire management could create a mosaic of seral states that enhances moose forage, while reducing wildland fire hazards along the wildland urban interface and providing ecosystem services.
Introduction
Wildlife contend with trade-offs across the landscape to meet daily and seasonal requirements for energy and protein (Robbins, 2001; Barboza et al., 2009) to maximize fitness. In mammals, adult females have high energy and protein requirements for reproduction, which can be split between in utero requirements during gestation and post-parturition requirements during lactation (Robbins, 2001; Barboza et al., 2009). Income and capital breeding describe two dichotomous categories that typify life history strategies to fulfill reproductive requirements in wildlife; however, it is understood that there is a continuum of life history strategies for reproduction between these two categories (Jönsson, 1997; Stephens et al., 2009). For ungulates in northern ecosystems, life history strategies generally fall on the capital breeder side of the continuum, requiring these animals to accumulate somatic energy and protein reserves during the short growing season and use these somatic reserves for survival and reproductive expenses during unpredictable winter conditions and into the following spring (Worden and Pekins, 1995; Cook et al., 2013, 2021a, Monteith et al., 2014). Additionally, some ungulate species give birth before adequate summer forage is available, requiring use of somatic reserves to meet early lactational demands (Barboza and Parker, 2008; Taillon et al., 2013). The rate at which ungulates accumulate or deplete these somatic reserves is influenced by daily and seasonal energy and protein demands, and the availability and quality of forage on the landscape (Mautz, 1978; Parker et al., 2009).
Exogenous and endogenous demands for energy and protein vary by reproductive status and additional demands of thermoregulation, immune responses, and locomotion. In northern ungulates, gestation increases demands for somatic reserves but demands of both energy and protein during lactation far exceed those during gestation (Pekins et al., 1998; Robbins, 2001; Barboza and Parker, 2008; Barboza et al., 2009; Taillon et al., 2013). Ambient temperature conditions can also increase energy demands for thermoregulation, with increased metabolic rates as animals respond to either cold or hot temperatures (Mitchell et al., 2018). During summer, biting insects also incur energetic costs to ungulate hosts, and can transmit parasites and diseases to hosts, resulting in increased somatic energy costs for immune responses (Kutz et al., 2009; Grunenwald et al., 2016; Benedict and Barboza, 2022; Benedict et al., 2023). Furthermore, during winter, ungulates may incur additional energy demands for traveling and foraging as snow depth increases (Parker et al., 1984; Fancy and White, 1985; Miquelle et al., 1992). As a conservation strategy, ungulates with lower somatic reserves may alter daily movement rates to decrease energy expended on locomotion (Long et al., 2014). For ungulates to meet these daily energy and protein demands, they need access to high quality forage, or must mobilize somatic reserves.
The ability of ungulates to accumulate somatic energy reserves during the growing season relies on their ability to consume enough forage to exceed daily demands for energy and protein. Lactating ungulates increase intake rates, through increased time foraging, to meet the demands of lactation (Hamel and Côté, 2008, 2009, Barboza et al., 2024). However, lactating females may also select habitats with varying nutritional qualities depending on predation risk avoidance strategies (Bergerud et al., 1984, Hamel and Côté, 2007, Blum et al., 2023). Some ungulates migrate to maximize access to high quality forage by following the emergence of spring vegetation and senescence of autumn vegetation prior to snow accumulation (Merkle et al., 2016; Aikens et al., 2017; Middleton et al., 2018). Ungulates must also contend with other aspects of the landscape that can limit daily energy and protein intake. For example, warm ambient temperatures during summer can result in lost foraging time through behavioral thermoregulatory responses and insect avoidance (Mörschel and Klein, 1997; Ditmer et al., 2018; Benedict and Barboza, 2022; Trondrud et al., 2023). The presence of predators across the landscape can also alter ungulate habitat selection to areas with lower quality forage (Creel et al., 2005; Dwinnell et al., 2019; Cain et al., 2024). Even if northern ungulates can alter their behavior to overcome these energetic challenges on the landscape to access sufficient forage, they still must contend with variability in forage availability and quality.
The quality and quantity of forage available to northern ungulates during the short growing season is dynamic and varies annually. Forage quality varies during the growing season as vegetation grows and senesces, with fiber and plant secondary metabolites increasing and digestible energy and protein declining (McArt et al., 2009; Thompson and Barboza, 2014; Johnson et al., 2021; Denryter et al., 2022). Furthermore, the availability of energy or protein from the same plant species can vary across the landscape and there can be inter-annual variation (McArt et al., 2009; Proffitt et al., 2016; Merems et al., 2020; Denryter et al., 2022). Seasonal and annual climatic variability can positively or negatively influence plant productivity and growing season length, altering the availability and quality of forage on the landscape for ungulates (Aanes et al., 2002; Hurley et al., 2014; Johnson et al., 2022; Monzingo et al., 2023). Disturbances to vegetative communities, including forest management and wildland fires, can alter the nutritional landscape for ungulates by increasing biomass and/or nutritional quality of preferred forage (Lord and Kielland, 2015; Proffitt et al., 2019; Ulappa et al., 2020; Snobl et al., 2022). Increased forage quality and quantity has been shown to increase somatic reserves in northern ungulates (Hurley et al., 2014; Proffitt et al., 2016; Merems et al., 2020), emphasizing the importance of the nutritional landscape to ungulate populations.
Moose (Alces alces), a northern capital breeder, accumulate somatic energy reserves during the relatively short growing season, and the level of reserves influences both adult cow survival and reproduction (Testa and Adams, 1998; Keech et al., 2000; Ruprecht et al., 2016; Cook et al., 2021b; Jesmer et al., 2021; Oates et al., 2021). Additionally, somatic reserves of gestating moose influence subsequent calf birth-mass and calf survival during the following spring and summer (Testa and Adams, 1998; Keech et al., 2000). Accordingly, moose population fitness may respond positively to landscape disturbances, such as wildland fires, that can increase moose forage quality and quantity (Lord and Kielland, 2015; Brown et al., 2018; Fredriksson et al., 2023; Mumma et al., 2024). The objective of our study was to examine some potential influences on the accumulation or depletion of somatic energy reserves in adult, female moose during early and late winter in a fire-mediated landscape on the Kenai Peninsula, Alaska. We predicted that individual female moose that have lower energetic costs during summer (e.g., lactation, activity, parasite loads), and whose summer home range had higher nutritional value (e.g., forage cover, length of growing season) would accumulate more somatic energy reserves going into winter. Furthermore, we predicted that individual female moose that had lower energetic costs (e.g., gestation, activity, locomotion in snow) and whose home range had higher nutritional value (e.g., moose forage cover) during the winter would have greater somatic energy reserves remaining in late winter.
Materials and methods
Study area
We studied adult female moose within Alaska Department of Fish and Game, Game Management Units 15A and 15B on the Kenai Peninsula, Alaska, USA (60°36′ N, 150°40′ W) from 2014–2022 (Figure 1). The study area (6,300 km2) encompasses a large portion of the Kenai Peninsula lowlands (<200m elevation), is flanked on the east by the Kenai Mountains (2,000m highest elevation), and sits within the Kenai River, Kasilof River, Swanson River, and Chickaloon River watersheds (Figure 1). The Kenai Peninsula lowlands were comprised of a mixed seral stage boreal forest (black spruce [Picea mariana], white spruce [Picea glauca], Alaska birch [Betula neoalaskana], quaking aspen [Populus tremuloides], Scouler’s willow [Salix scouleriana]), interspersed with numerous wetlands and lakes. As elevation increases into the Kenai Mountains, white spruce dominated stands of the boreal forest transition into mountain hemlock (Tsuga mertensiana) and alder (Alnus viridis) before seceding to tree line. Landscape scale wildland fires (Figure 1) occurred within the study area in 2014 (Funny River Fire; ~795 km2), 2015 (Card Street Fire; ~35 km2), and 2019 (Swan Lake Fire; ~675 km2), creating early seral stage mixed boreal forest. The remainder of the study area was comprised of mid to late seral stage boreal forest as a result of landscape scale wildland fires in 1947 and 1969, with some areas undisturbed for >120 years (Miner, 2000).
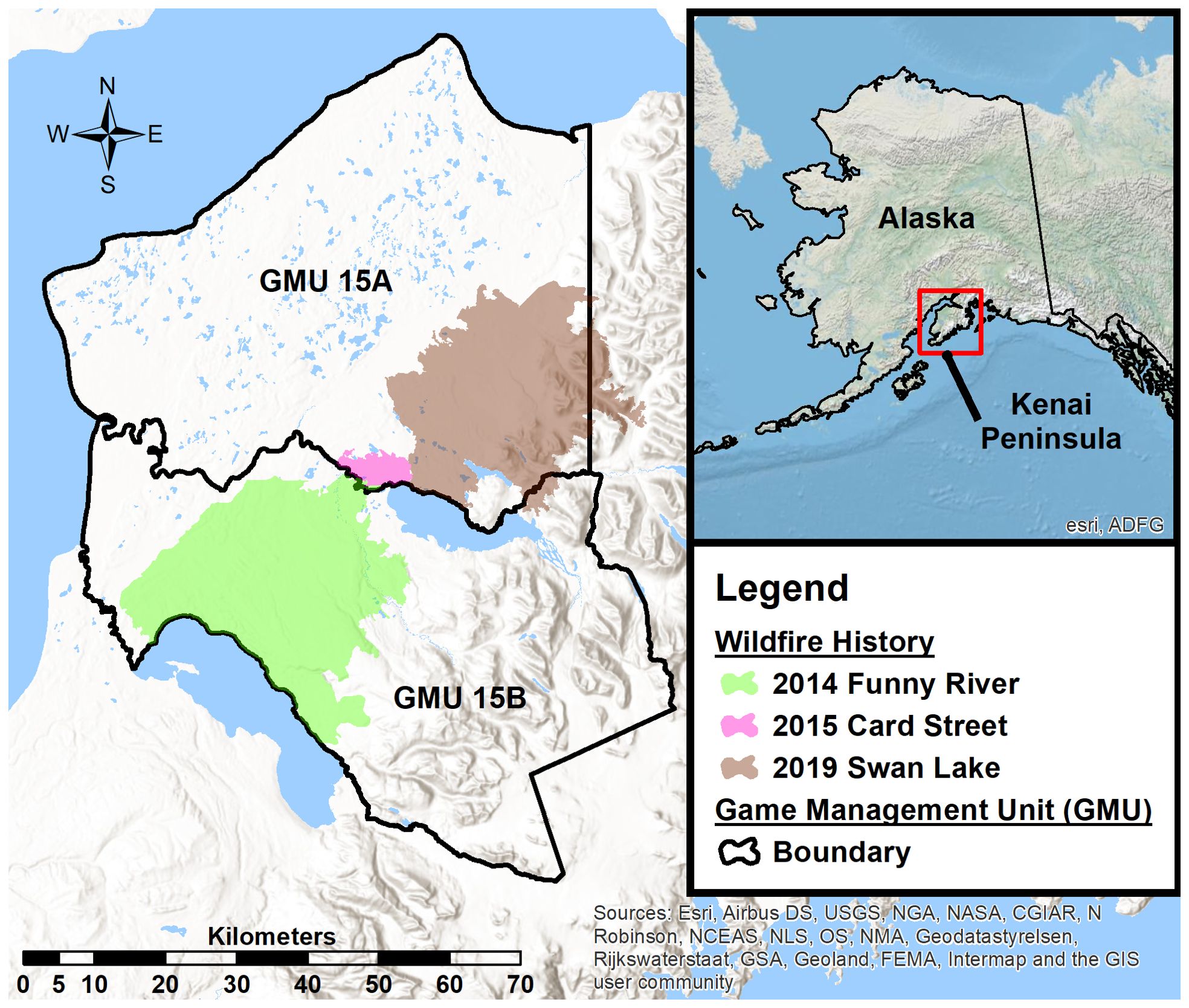
Figure 1. Study area located on the Kenai Peninsula, Alaska, USA. The Alaska Department of Fish and Game defines two Game Management Units (GMU) within the study area. Recent fire history depicts the 2 large landscape scale wildfires that occurred in 2014 and 2019.
Animal handling
All procedures for care, handling, and experimentation followed guidelines of the American Society of Mammalogists (Sikes and The Animal Care and Use Committee of the American Society of Mammalogists, 2016) and were approved by the Alaska Department of Fish and Game Division of Wildlife Conservation Animal Care and Use Committee (protocols 2014-17, 2015-29, 2016-40 and 0046 [2017–2022]).
We chemically immobilized female moose (≥ 3 years old) using aerial darting methods with a combination of opioid and alpha2 adrenoceptor agonists administered in a 2 or 3-cc dart delivered from a projector (Palmer Cap‐Chur™, Douglasville, GA, USA; Pneudart Inc., Williamsport, PA, USA) out of a helicopter (Robinson-44, Robinson Helicopter Company, Torrance, CA, USA; Hughes-500, McDonnell Douglas Helicopter Systems, Mesa, AZ, USA). We used either carfentanil citrate (2014–2016; 0.01 mg • kg-1 estimated body mass; 3mg • mL-1; ZooPharm, Windsor, CO, USA) or thiafentanil oxolate (2017–2022; 0.03 mg • kg-1 estimated body mass; 10mg • mL-1; ZooPharm LLC, Laramie, WY, USA) mixed with xylazine HCl (0.20–0.26 mg • kg-1 estimated body mass; 100mg • mL-1; Lloyd Laboratories, Shenandoah, IA, USA) to chemically immobilize adult female moose. We antagonized opioids with naltrexone HCl (100 mg • mg-1 carfentanil; 10 mg • mg-1 thiafentanil; intramuscular; 50 mg • mL-1; ZooPharm). We antagonized xylazine HCl with either 400 mg tolazoline HCl (2014–2015; 0.88 mg • kg-1 estimated body mass; ¼ dose intravenous, ¾ intramuscular; 200 mg • mL-1; ZooPharm) or atipamezole HCl (2016 – 2022; 0.02 – 0.04 mg • kg-1 estimated body mass; ¼ dose intravenous, ¾ intramuscular; 25 mg • mL-1; Zoetis, Parsippany, NJ, USA). If moose showed signs of respiratory depression (i.e., respiration rates <6 breaths • min-1 or capillary refill in the gums not instantaneous), we administered either 100–200mg doxapram HCl (2014–2015; intravenous; 20mg • mL-1; Henry Schein, Dublin, OH, USA), or the antagonist dose of atipamezole HCl (2016–2022).
From November 2014 to March 2022, we conducted repeated captures of female moose during early winter (November–December) and late winter (February–March). We determined the age of each moose by either extracting an incisor canine and analyzing for cementum annuli (Matson’s Lab, Miltown, MT, USA; Sergeant and Pimlott 1959; Gasaway et al., 1978), catching known aged individuals (e.g., first captured as 10-month-old calf), or by estimating age based on tooth wear. We estimated percent body fat of moose from measurements of maximum rump fat thickness (MAXFAT; cm) by ultrasonography (Bantam II, Ibex® Pro, E.I. Medical Imaging, Loveland, CO, USA; Stephenson et al., 1998), which we then converted to percent ingesta-free body fat for analysis (IFBFAT; Stephenson et al., 1998). One moose did not have measurable rump fat during late winter captures and we estimated body fat from body condition score assigned to that individual following palpation (Levine et al., 2022). Because the number of days between repeated captures of individuals varied up to 27 days, we calculated the rate of change in percent body fat for each individual during the summer or winter, respectively. During summer, we calculated the daily rate of change in percent body fat by subtracting the percent body fat in early winter from percent body fat in the preceding late winter, and then divided by the number of days between each body fat estimate. We used the same calculation to determine the daily rate of change in body fat during winter using the difference in early and late winter percent body fat. We then converted all daily rate of change in percent body fat values to monthly rate of change in percent body fat by multiplying by 30.
We collected blood samples using 10ml glass serum tubes (BD Vacutainer® PN#366430; Becton, Dickinson and Company, Franklin Lakes, NJ, USA) and 6ml plastic trace element whole blood tubes (K2 EDTA; BD Vacutainer® PN#368381; Becton, Dickinson and Company) by jugular venipuncture. To determine pregnancy status, we analyzed serum for pregnancy-specific protein B (BioTracking Inc., Moscow, ID, USA; Herd Health Diagnostics, Pullman, WA, USA; Sasser et al., 1986; Huang et al., 2000). We also analyzed frozen whole blood samples collected in early winter for parasite load (filaria nematodes) with a modified Knotts technique (Texas A&M Veterinary Diagnostics Laboratory, College Station, TX, USA; Laaksonen et al., 2009). We fitted each moose with a global positioning system (GPS) collar (2014–2016: model TGW‐4700‐3; 2017–2022: model TGW-4677-4; Telonics Inc., Mesa AZ, USA) that recorded GPS locations at 0.5-hour (2014–2016) and 4-hour (2017–2022) intervals. Each GPS collar also collected 5-minute activity (3-axis accelerometer; number of active seconds on any axis) and collar temperature (°C). To create a variable that considers the energetic costs associated with movement, we established a relationship between daily movement rates estimated with 0.5-hour GPS fixes and total daily activity counts from 5-minute accelerometer values (Supplementary Data Sheet 1). We then applied this relationship to activity values collected for all study animals, which provided a proxy for movement rates for GPS collars that collected courser scale GPS locations (i.e., 4-hour interval).
Given the spatial variation and elevation gradient within our study area (e.g., rolling coastal hills at sea level, mountainous terrain; Figure 1), we expected moose to be exposed to different growing season lengths and snow depths throughout the year. We established a relationship between the temperature recorded on the GPS collar and ambient air temperature (Supplementary Data Sheet 2), and then estimated ambient air temperature for each recorded temperature from the GPS collars. Growing degree days can be used as a predictor of plant growth in arctic environments (Chapin, 1983, Kelsey et al., 2021). We generated a variable for growing degree days >5°C for each moose and for each growing season by calculating the number of degrees that the mean estimated daily ambient air temperature was >5°C, summed across all days for the period between the spring and autumn equinox (Bowyer et al., 1998; Stewart et al., 2005).
The relative increase in net cost of locomotion can be estimated using snow depth and brisket height for moose (Miquelle et al., 1992; Supplementary Data Sheet 3). We used the daily centroid GPS locations for each study animal to extract daily snow water equivalent (kg • m-2) values from Daymet (Thornton et al., 2024). To establish a relationship between snow water equivalent and snow depth, we used a paired dataset with Daymet and Natural Resources Conservation Service Snowpack Telemetry Network data at the Kenai Moose Research Center (Natural Resources Conservation Service, 2024; SNOTEL; Kenai Moose Pens 366; Supplementary Data Sheet 3). We then estimated the daily relative increase in net cost of locomotion for each study animal during winter (Supplementary Data Sheet 3).
Given the remoteness of our study area, it was not feasible to conduct large-scale vegetation biomass and subsequent nutritional analysis of moose forage. However, vegetation cover can be used as a proxy for vegetation biomass (Monzingo et al., 2022) and we used 3 vegetation classification efforts within the study area to generate a moose forage index based on percent cover of forage species preferred by moose (Supplementary Data Sheet 4). First, we used field-based vegetation cover data collected at 605 sites within 28 dominance types in 2017, which was the basis for the 2019 Kenai Peninsula Existing Vegetation Map Project (Bellante et al., 2020). We determined mean percent cover of each forage species for moose in each dominance type for summer and winter (Supplementary Data Sheet 4). The 2014 Funny River Fire, 2015 Card Street Fire, and 2019 Swan Lake Fire, created early seral habitats within our study area. In 2020, the Alaska Department of Fish and Game completed a vegetation survey within the Funny River Fire footprint (Beattie et al., 2024), and percent cover of moose forage during summer and winter from this effort was then used to redefine the dominance types within the Funny River Fire and Card Street Fire footprints (Supplementary Data Sheet 4). During 2020–2021, using the same vegetation survey protocol (Beattie et al., 2024), we conducted vegetation surveys within the 2019 Swan Lake Fire footprint (D.P. Thompson, unpublished data), and redefining the percent cover of moose forage during summer and winter in map group within the Swan Lake Fire footprint (Supplementary Data Sheet 4). We then calculated cumulative percent cover for each map group and dominance type (2019 Kenai Peninsula Existing Vegetation Map Project; Bellante et al., 2020), redefined dominance type (Funny River and Card Street Fire footprints; Beattie et al., 2024), or redefined map group (Swan Lake Fire footprint, Supplementary Data Sheet 4).
Using GPS locations for each moose, we generated home ranges for each moose using the BBMM Brownian bridge movement model in R (Nielson et al., 2013; R Core Team, 2023). We aggregated GPS data from 2014–2016 to 4-hour intervals to create home ranges with a similar dataset as GPS locations collected from 2017–2022. We created 95% isopleths home ranges for each moose for each year (moose-year), during two periods: lactation (15 May – 15 September) and gestation (16 September – 15 March). We limited the gestation period to March 15 because a large portion of deployed GPS collars were removed during captures in March. For each moose-year and period, we then calculated home range size, and the proportion of the home range within each dominance type or map group. For each moose-year and period, we then multiplied the proportion of their home range that was within a given dominance type or map group by the cumulative percent cover of moose forage for the same dominance type or map group (Supplementary Data Sheet 4). We then summed the values (proportion home range multiplied by cumulative percent cover moose forage) for all dominance type or map group for each moose-year and period into a new variable, moose forage index.
We used vaginal implant transmitters (VITs) and aerial radiotracking to determine parturition dates and twinning rates for radio collared moose. During 2014–2018 captures, we deployed vaginal implant transmitters (Model M3970; Advanced Telemetry Systems, Isanti, MN, USA) to aid in parturition detection and for thermoregulation studies (Thompson et al., 2018; McDonough et al., 2022). Deployment of VITs in moose followed procedures developed at the Kenai Moose Research Center (McDonough et al., 2022) and outlined in Patterson et al. (2013). From 12 May through 7 June each year, female moose were aerially radio tracked and observed every 24–48 hours using fixed-wing aircraft to determine parturition date and twinning status (McDonough et al., 2022). After 7 June, non-parturient moose were flown weekly through 30 June to determine parturition status. Monthly aerial radiotracking was conducted after parturition to determine calf-at-heel status for each moose. We estimated calf mortality dates, using the midpoint between the last known observation date and the first missing observation date and we assumed that a calf not observed with the radio collared cow was dead.
Data analysis
We analyzed data using program in R (R Core Team, 2023). We used linear mixed effect regression models “lmer” in the lme4 package (Bates et al., 2015) to evaluate what influenced body condition of adult female moose during lactation (15 May – 15 September) and gestation (16 Sept – 15 March). We scaled continuous predictor variables to allow for direct comparisons between predictor variables and we included individual as a random effect. For early winter body condition, we evaluated the response variable percent body fat of adult cow moose in November (n = 163) against several variables that could have influenced energy accumulation and depletion during lactation: the categorical variable summer lactation status (no birth, birth but neonate did not survive to 4-months-old, birth and neonate survived to 4-months-old), and the continuous variables summer growing degree days, mean daily activity count, parasite load, summer moose forage index, age and year. We evaluated the response variable monthly rate of change in percent body fat during the growing season from late winter to early winter (i.e., March to November; n = 55) against the same 5 predictor variables during lactation and late winter body fat preceding the growing season. To examine late winter body condition, we evaluated the response variable percent body fat of adult cow moose in March (n = 98) against variables that could have influenced energy accumulation and depletion during gestation: the categorical variable gestation status (non-pregnant, pregnant with singleton, pregnant with twins), and the continuous variables mean daily activity count, mean daily relative increase in net cost of locomotion in snow, interaction between daily activity count and daily relative increase in net cost of locomotion in snow, winter moose forage index, age and year. Similarly, we also evaluated the monthly rate of change in percent body fat over winter (November to March; n = 61) for the same variables during gestation and early winter body fat preceding the winter. We evaluated model fit using Akaike’s information criterion adjusted for small sample sizes (AICc; Burnham and Anderson, 2002). Using the package MuMIn in R (Bartoń, 2023), we selected the simplest model within 2 AICc of the top model (Burnham and Anderson, 2002), and estimated model variance (R2) for fixed effects (marginal variance) and for fixed and random effects (conditional variance). If the selected model contained the variable moose forage index, we then ran the selected model and split out the moose forage index variable to evaluate if the percent cover of the 5 predominate moose forages was influencing the model, and then ran AICc model selection. We split the moose forage index into 5 variables for summer percent cover: forb cover, willow cover, aspen cover, Alaska birch cover, and shrub cover (Supplementary Data Sheet 4). Similarly, 5 moose forage variables were evaluated for winter percent cover: willow cover, aspen cover, Alaska birch cover, shrub cover, and shoulder season forage cover (Supplementary Data Sheet 4).
Results
During our 8-year study, we collected over 223,000 GPS locations from 97 individual adult female moose, which we immobilized a total of 261 times (n = 163 early winter; n = 98 late winter). The average age of moose captured was 8.7 years old ± 0.2 SE (range 3–18 years old). During winter, 94% of female moose were pregnant (n = 92). During spring, 88% of female moose (n = 144) were parturient but, only 31% of parturient females successfully retained a calf-at-heel through lactation (n = 44). Mean parturition date was May 19th (± 5.5 days SE). During the growing season, daily activity counts (mean = 4684.9; range 1721.0–9291.0) were similar among years (Supplementary Data Sheet 5, Supplementary Figure 5.1A) but sum of daily growing degree days (mean = 1559.8; range 1167.0–2030.0) was higher in 2016 and 2019 compared with other years (Supplementary Data Sheet 5, Supplementary Figure 5.1B). We detected filaria nematode densities in blood in 38% of female moose sampled during early winter (n = 62; mean = 424.2 filaria • ml-1 blood; range 2.0–3622.0 filaria • ml-1) and early winter nematode densities were similar among years (Supplementary Data Sheet 5, Supplementary Figure 5.1C). During winter, daily activity counts (mean = 4123.2; range 1374.0–6430.0) were similar among years (Supplementary Data Sheet 5, Supplementary Figure 5.2A) but the daily relative increase in locomotion from moving in snow (mean = 93.5%; range 4.5–345.7%) was lowest in 2015 and highest in 2020 and 2021 (Supplementary Data Sheet 5, Supplementary Figure 5.2B). Home ranges for adult moose (95% isopleth) were 16.2 km2 ± 1.19 SE during lactation, and 21.6 km2 ± 4.6 SE during gestation.
The percent cover of the 5 predominate moose forages in home ranges of moose differed by wildland fire history. The percent cover of forbs in summer home ranges of moose decreased with time since wildland fire (Figure 2A). A higher percent cover of aspen forage in summer home ranges of moose was found in the Funny River Fire footprint (6 years post burn), while the percent cover of Alaska birch forage was higher in summer home ranges of moose containing older seral stands (Figure 2A). The percent cover of forage for willows and shrubs were similar in summer home ranges of moose in the Funny River Fire footprint and older seral stands, but lower in the recently burned footprint of the Swan Lake Fire (Figure 2A). Similar relationships between percent cover of forage in home ranges of moose during winter were observed for aspen, willows, Alaska birch, and shrubs (Figure 2B). The percent cover of shoulder season forage during winter was considerably higher in home ranges of moose within the Funny River Fire footprint compared with older seral stands and recently burned areas within the Swan Lake Fire footprint (Figure 2B).
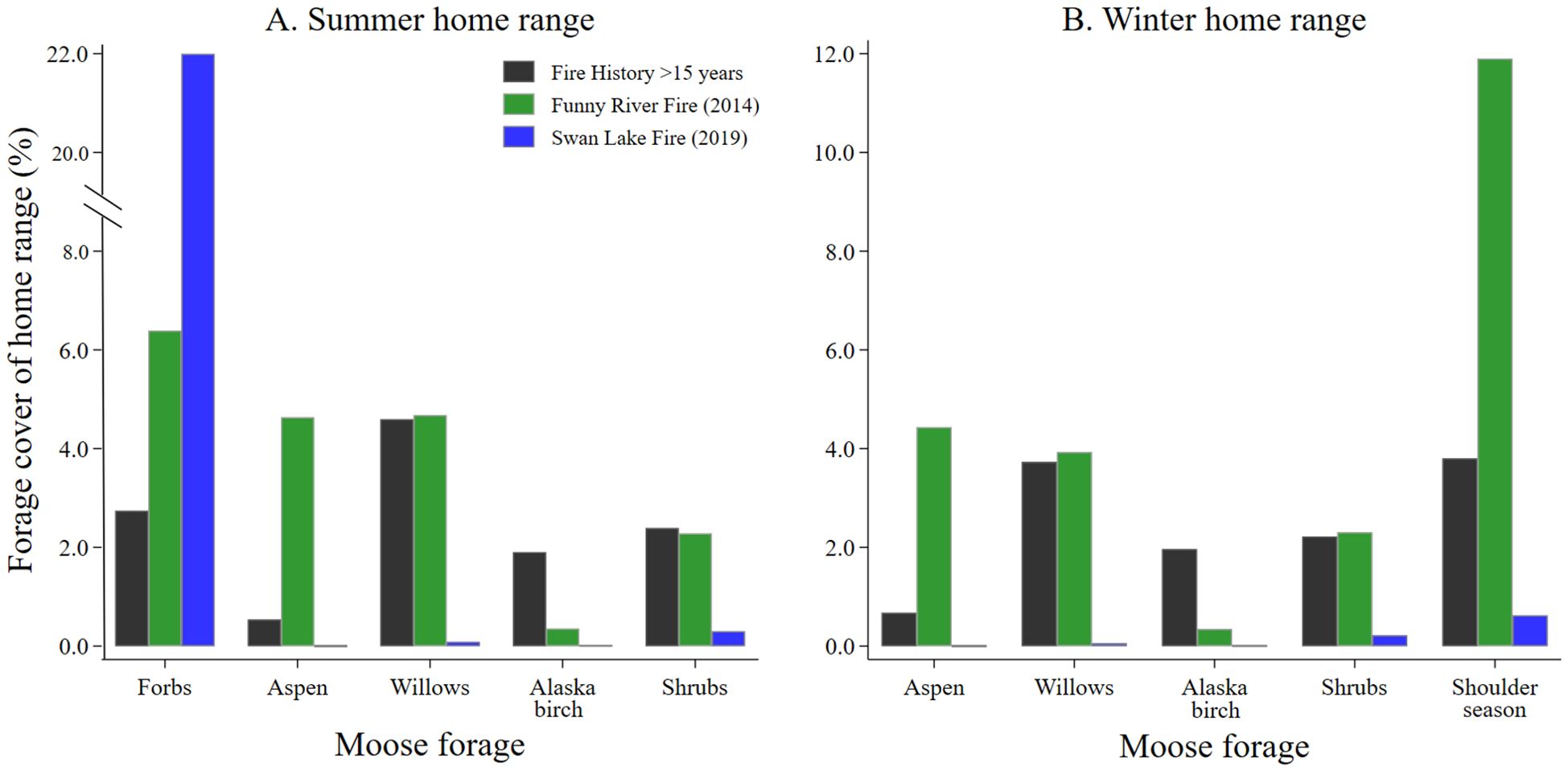
Figure 2. The percent cover of moose forage species in moose (A) summer and (B) winter home ranges by recent fire history collected from adult female moose on the Kenai Peninsula, Alaska, USA from 2015 – 2022.
The best model predicting body fat in early winter of adult female moose included lactation status and moose forage index (Supplementary Data Sheet 6; Supplementary Table 6.1, 6.2). Because the selected model included moose forage index, we then ran the selected model and split moose forage index into 5 summer forage variables and reanalyzed the model. Lactation status and aspen forage index were the only variables in the selected model (Table 1; Supplementary Data Sheet 6, Supplementary Table 6.3). Female moose that gave birth and lost their neonate during the summer, had more body far in early winter (14.39% ± 0.24 SE) compared with female moose that, either did not give birth (11.53% ± 0.52 SE), or that gave birth and the neonate survived to 4-months-old (10.59% ± 0.34 SE). Post hoc pairwise comparison with Bonferroni method of body fat in early winter of moose who incurred a full lactational demand showed no difference between retaining a singleton (n = 37) or twin (n = 7) neonates through 4-months-old (z = -0.83; P = 1.00). Body fat in early winter was positively correlated to summer home ranges of moose with higher percent cover of aspen forage (Table 1; Figure 3A). The selected model for the monthly rate of change in body fat over the summer included the variable lactation status and prior body condition (Table 1; Supplementary Data Sheet 6, Supplementary Table 6.4). Female moose whose neonate survived through summer had a lower monthly rate of change in body fat (0.24% • month -1 ± 0.08 SE) compared with moose that did not give birth (0.37% • month -1 ± 0.10 SE) and moose that gave birth but lost their neonates before the end of summer (0.65% • month -1 ± 0.05 SE). Furthermore, moose with lower body fat in late winter accumulated body fat faster during the subsequent growing season (Figure 4A).
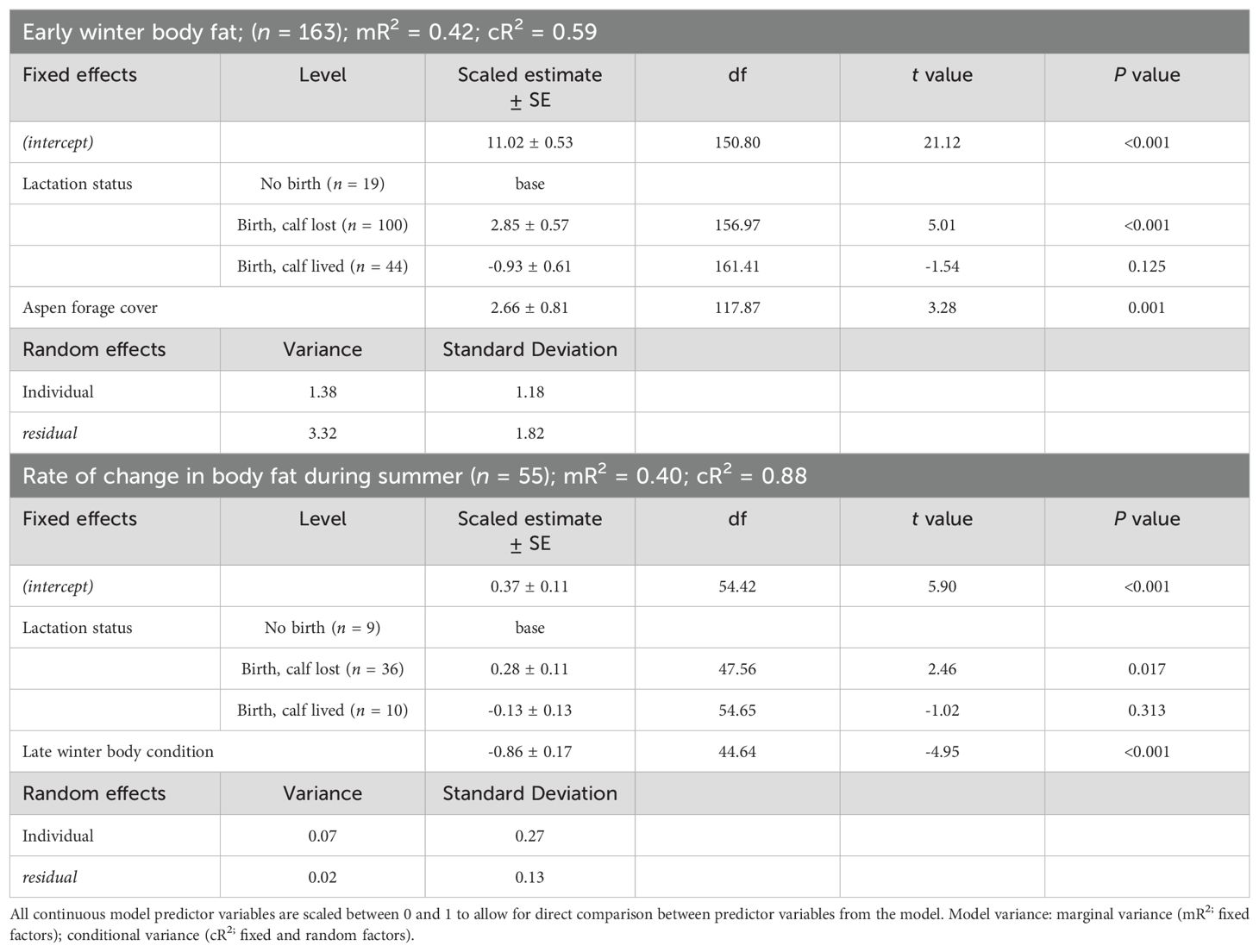
Table 1. Parameter estimates from linear mixed-effect model regression models that influence early winter body fat and the rate of change in body fat during the summer of adult, female moose on the Kenai Peninsula, Alaska, USA from 2015 – 2021.
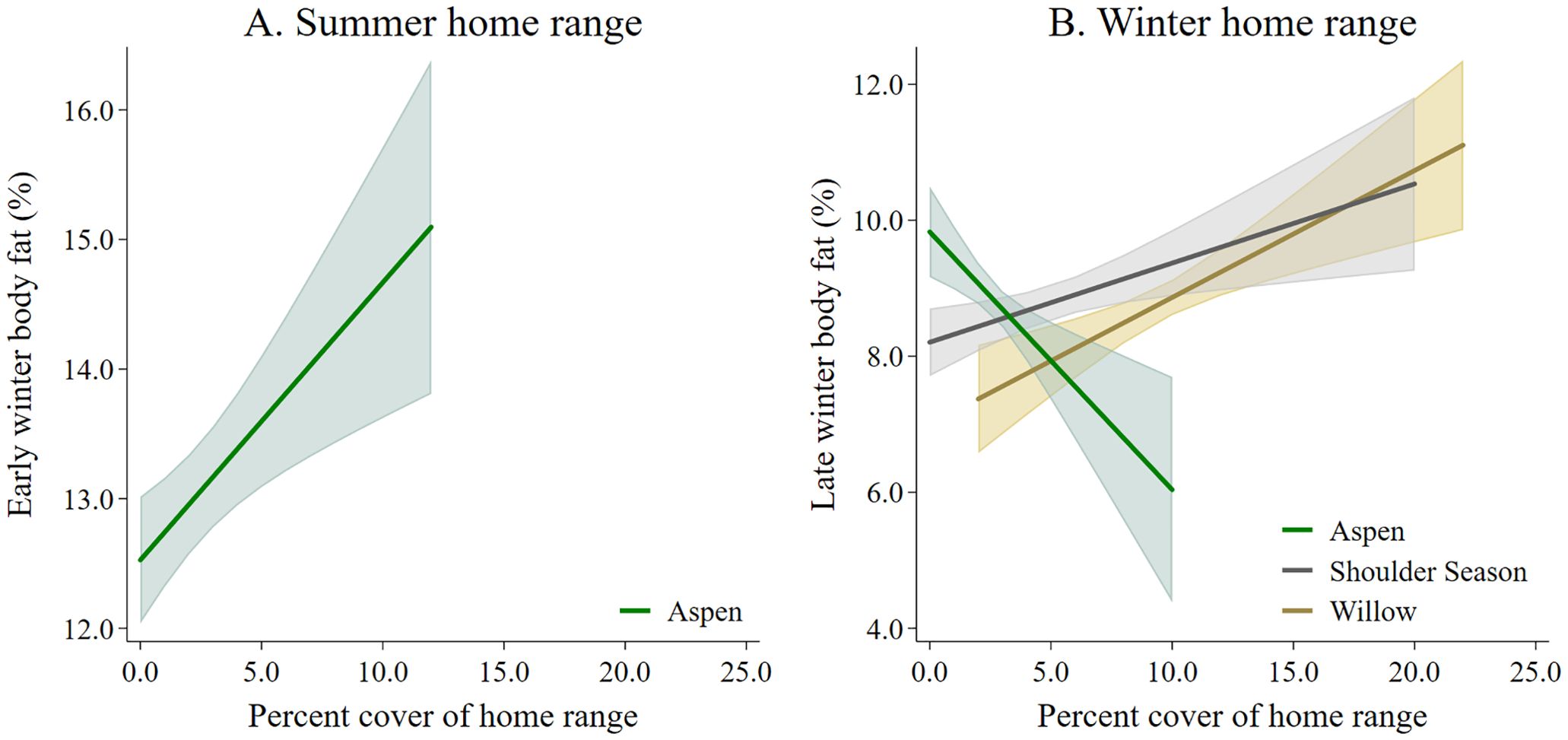
Figure 3. The influence of percent cover of moose forage species in moose home ranges on (A) early winter and (B) late winter body fat collected from adult female moose on the Kenai Peninsula, Alaska, USA from 2015 – 2022.
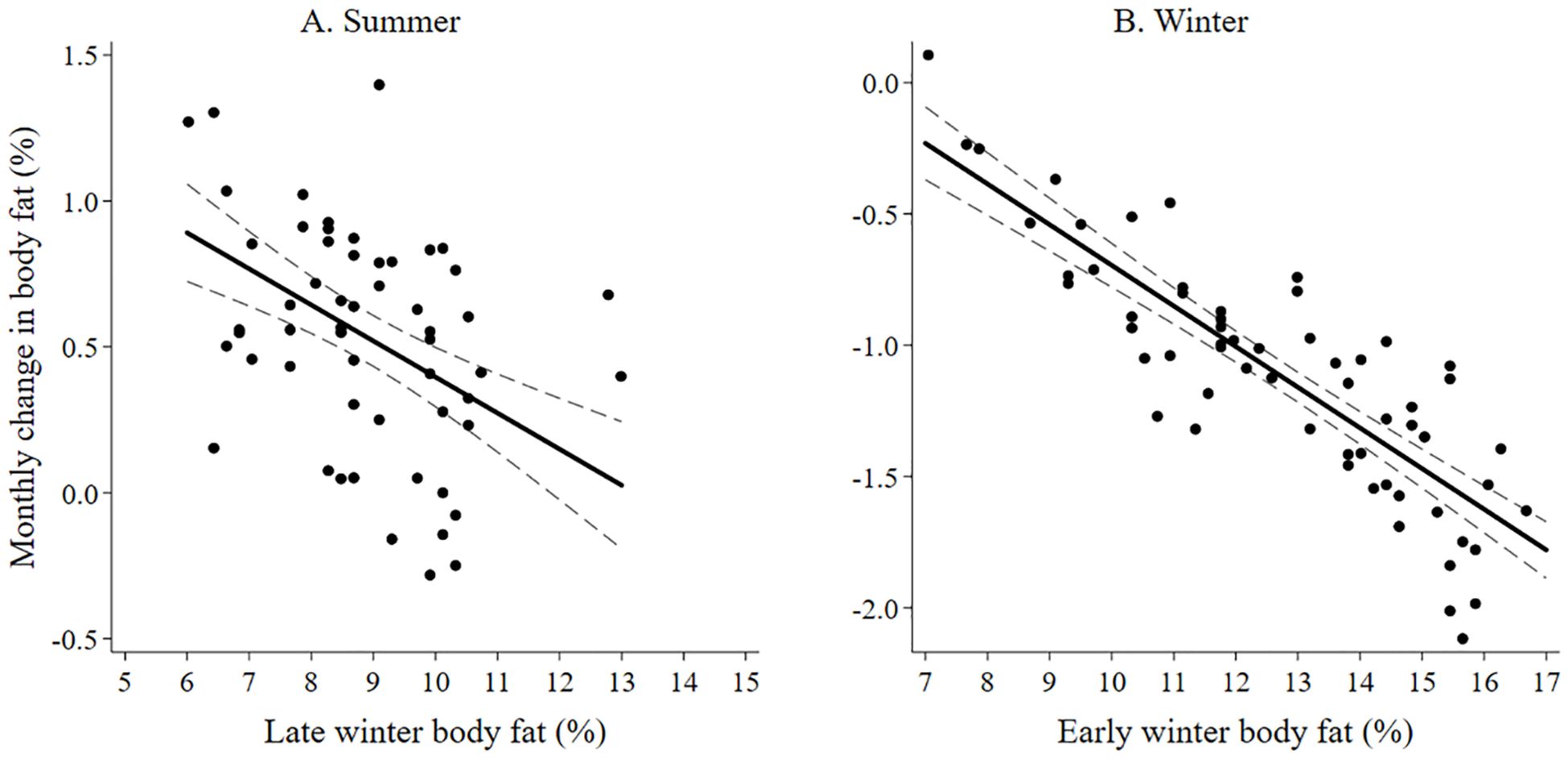
Figure 4. The monthly change in body fat of adult female moose on the Kenai, Peninsula, Alaska, USA from 2015 – 2022, is influenced (A) during summer by body fat in March (late winter) and (B) during winter by body fat in November (early winter).
The best model that influenced body fat in late winter of female moose included the variables moose forage index and year (Supplementary Data Sheet 6, Supplementary Tables 6.5, 6.6). Similar to the body fat in early winter model, we reanalyzed the selected model splitting out winter moose forage index into 5 variables. The model best predicting body fat in late winter body of female moose included the variables year, aspen forage index, willow forage index, and shoulder season forage index (Table 2; Supplementary Data Sheet 6, Supplementary Table 6.7). Average body fat in late winter was 8.3% ± 0.1 SE in 2016 and increased annually by 0.17% to 9.3% in 2022. Body fat in late winter was negatively correlated with home ranges of moose that had higher percent cover of aspen forage (Table 2, Figure 3B) but positively correlated with home ranges with higher percent cover of willows and shoulder season forages (Table 2, Figure 3B). The monthly rate of change in body fat over winter was influenced by body condition going into winter and activity. (Table 2; Supplementary Data Sheet 6, Supplementary Table 6.8). Moose with higher levels of body fat in early winter lost body fat at a higher rate during gestation than moose with less body fat reserves (Figure 4B). Increased activity also resulted in a higher rate of fat loss during gestation, accounting for 0.34% difference in the monthly rate of loss of body fat over the range of activity recorded.
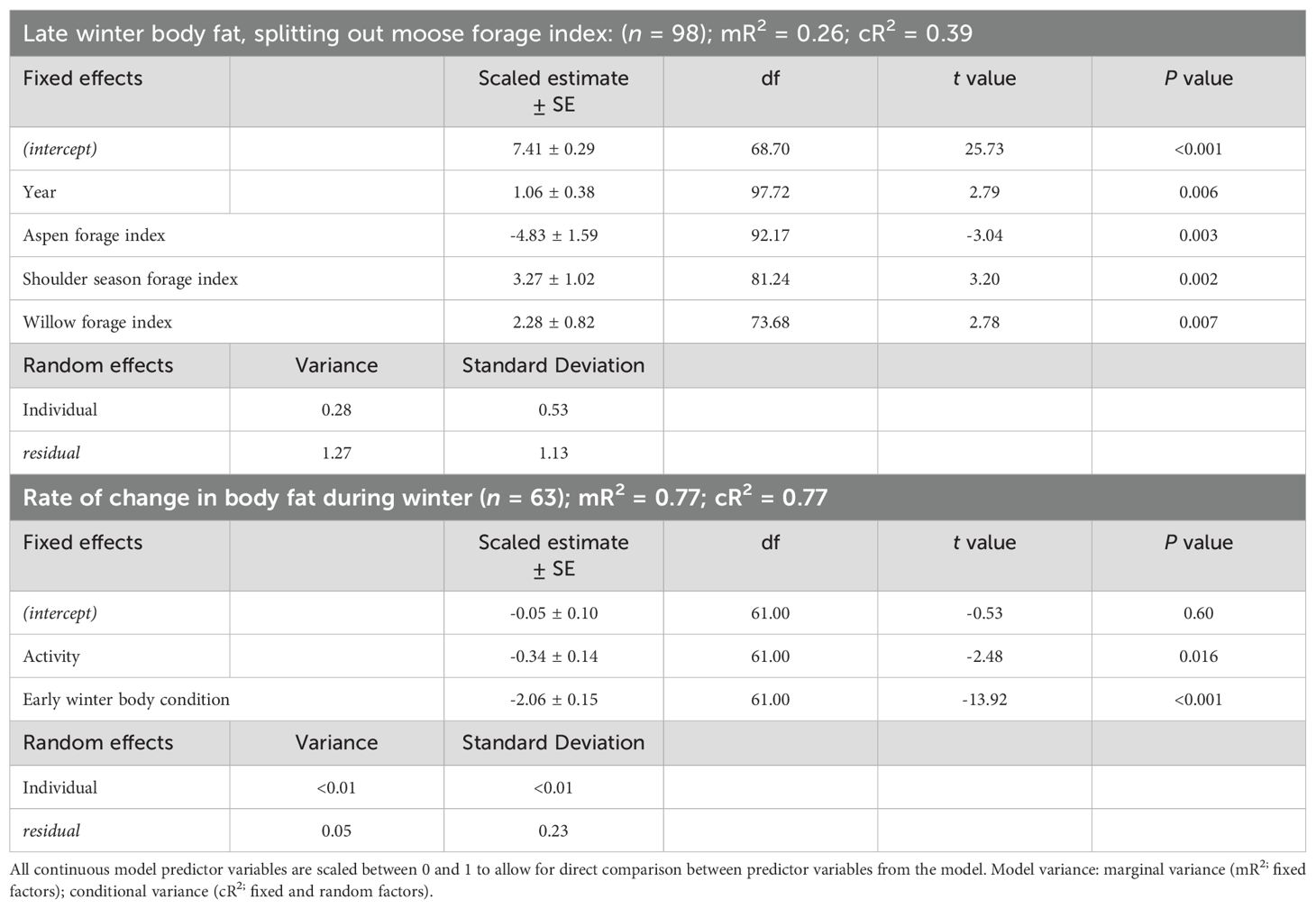
Table 2. Parameter estimates from linear mixed-effect model regression models that influence late winter body fat and the rate of change in body fat of adult, female moose on the Kenai Peninsula, Alaska, USA from 2015 – 2022.
Discussion
Northern ungulates, such as moose, have responded to seasonal availability of nutrients and energetic costs by evolving mechanisms and reproductive strategies that are buffered by somatic reserves. Although we analyzed several factors that could contribute to the accumulation or depletion of somatic energy reserves in female moose during the growing season, our results found that only the endogenous demands for lactation and aspects of the nutritional landscape influenced somatic energy reserves during early winter. Similar to other northern ungulates (Cook et al., 2013, 2021a, Monteith et al., 2014; Proffitt et al., 2016), the energetic costs of lactation were apparent in our study, with female moose having ~3.8% lower percent body fat in early winter compared with moose that incurred only partial lactation energy demands. Furthermore, moose whose home ranges had higher percentage of moose forage cover during the growing season, a result of recent wildland fires, had higher somatic energy reserves in early winter, which was consistent with summer nutritional landscapes influencing somatic reserves in other northern ungulates (Monteith et al., 2013, 2014, Proffitt et al., 2016; Merems et al., 2020). During winter, we found that aspects of the nutritional landscape and year influenced late winter somatic energy reserves while reproductive status did not. Contrary to our prediction, we did not find that gestating moose had similar somatic energy reserves than non-pregnant moose. This result could be an artifact of high pregnancy rates in our study, and our measurements of somatic energy reserves occurred near the beginning of the third trimester prior to the most costly period of gestation (Pekins et al., 1998; Robbins, 2001). Percent cover of winter browse in home ranges of moose also influenced somatic energy reserves of moose during late winter; however, we documented trade-offs in somatic reserves for moose whose home ranges included forage cover of aspen, willows, and shoulder season forages (e.g., lowbush cranberry [Vaccinium vitis-idaea], bunchberry dogwood [Cornus canadensis]); Supplementary Data Sheet 4). Cumulatively, our results suggest that both endogenous demands for lactation and local availability of quality forage influence moose somatic reserves during early and late winter.
Body condition measurements of Alaskan moose (A. a. gigas) have been documented across their range. During early winter, our body fat measurements were lower (10.6 – 14.4% body fat) than earlier measurements on the Kenai Peninsula (2006: 13 – 17% body fat; (Kraft, 2011); however, our captures occurred 3 to 4 weeks later in early winter and could have accounted for some of this difference. Although moose on the Kenai Peninsula in this study had considerably lower accumulation rates of body fat during the growing season (0.24 – 0.65% body fat • month -1) than moose on the Gustavus Forelands of Southeast Alaska (0.88 – 1.25% body fat • month -1), both studies exhibited a lower monthly rate of body fat accumulation for female moose that retained its calf through the summer (White et al., 2014). Our measurements of body condition during late winter on the Kenai Peninsula (8.3 – 9.3% body fat) were higher than those collected in the Gustavus Forelands (7.9%; White et al., 2014), the Tanana and Yukon Flats of Interior Alaska (6.4 – 8.9% body fat; Keech et al., 2000; Bertram and Vivion, 2002; Boertje et al., 2007), and earlier measurements on the Kenai Peninsula (2007: 7.6% body fat; Kraft, 2011). Additionally, average body condition during late winter of our moose increased annually; this may be a result of increased winter browse availability within recently burned areas (Schwartz and Franzmann, 1989; Weixelman et al., 1998). Similar to other ungulates, moose in our study with better body condition going into winter lost body fat at a higher rate (Monteith et al., 2013; Cook et al., 2013, 2021a).
Ungulates using capital breeding strategies have high energy and protein demands to produce milk for neonates (Robbins, 2001; Barboza et al., 2009). To raise a single moose calf through weaning, a cow moose would need ~33.4 kg fat for milk production (Reese and Robbins, 1994; Shochat and Robbins, 1997; Thompson et al., 2023), which is the equivalent to accumulating 7.4% body fat during the same time period for a 450 kg moose. Both lactating and non-lactating moose have high digestible energy intakes during the growing season (Shively et al., 2019). Lactating moose in our study, however, could not overcome the energetic costs of milk production compared with those individuals with no or reduced lactation cost, and subsequently entered winter with lower somatic energy reserves. Additionally, some northern ungulates follow a risk averse habitat selection during the growing season by selecting habitats that provide more security for their offspring at the expense of nutritional quality of the available forage (Bergerud et al., 1984, Hamel and Côté, 2007, Blum et al., 2023). Moose have been documented to employ both risk-averse and risk-prone strategies for birth site selection and summer habitat use during lactation (Bowyer et al., 1999; Poole et al., 2007; Severud et al., 2019; Francis et al., 2021). Pregnant moose with higher somatic reserves give birth to larger calves that have higher survival rates (Testa and Adams, 1998; Keech et al., 2000) but incur the costs of lactation during summer, while simultaneously attempting to accumulate somatic reserves to survive the coming winter. Relatively low somatic reserves among lactating female moose at the end of the growing season (White et al., 2014; Ruprecht et al., 2016; Cook et al., 2021b) may result in cow moose exhibiting a risk-sensitive reproductive allocation by lowering their chance of becoming pregnant in autumn or terminating a pregnancy in utero to maintain sufficient somatic energy reserves for winter survival (Testa and Adams, 1998; Keech et al., 2000; Boertje et al., 2019; Jesmer et al., 2021; Oates et al., 2021). This risk-sensitive strategy among moose may also hinder our ability to detect the effects of other energetic expenses that could influence somatic reserves.
We investigated the influence of parasite loads, activity counts, and environmental conditionals on somatic reserves in adult female moose. Although we detected filarial nematodes (Setaria yehi, Rumenfilaria andersoni) in 38% of female moose sampled during early winter, nematode densities did not demonstrably influence early winter somatic reserves. Body condition may also buffer the effects of parasites with tradeoffs during the summer for foraging (Benedict et al., 2024). Setaria spp. can cause peritonitis in Scandinavian reindeer calves (Rangifer tarandus; Laaksonen et al., 2007) and moose calves in Alaska (D.P. Thompson, Alaska Department of Fish and Game, unpublished data) but adult animals may act as asymptotic carriers (Laaksonen et al., 2007). Other parasite species (e.g., Dermacentor albipictus; Parelaphostrongylus tenuis; Elaeophora schneideri) can also have detrimental effects on moose populations (Wünschmann et al., 2015; Grunenwald et al., 2018; Ellingwood et al., 2020) but these parasites have not been documented in Alaska (Kutz et al., 2012).
We did find a relationship between sum of daily activity counts and the rate of change in somatic reserves in female moose during winter. Late winter somatic reserves in elk (Cervus elaphus) exhibited a positive relationship with energy expended on movement for animals with < 8% body fat (Long et al., 2014). We did not find a relationship with somatic energy reserves for moose in late winter with the interaction between daily activity counts and energy expended for locomotion in snow. Moose in Scandinavia showed a decrease in movement rates until snow depths reached 40 cm at which time movement rates remained stable as snow continued to increase (Melin et al., 2023). Additionally, we used equations from Miquelle et al. (1992) that assume a static snow density and that moose completely sink to the ground in each step when estimating the relative increase in energy for locomotion in snow. Snow density increases over winter (Fancy and White, 1985), with the relative cost of locomotion also increasing (Parker et al., 1984). However, there is a tradeoff with snow density and sinking depth (Sullender et al., 2023), which we could not quantify with the remotely sensed data available to estimate snow depth (Thornton et al., 2024). Furthermore, snow density, snow depth, and surface hardness can increase the energetic costs for caribou to access ground vegetation (Fancy and White, 1985), which would also apply to moose cratering to access shoulder season vegetation (LeResche and Davis, 1973) or in recently burned habitats (Weixelman et al., 1998). Advances in animal borne biologging that include heart rate sensors (Signer et al., 2010; Trondrud et al., 2021; Kirchner, 2024) could be used to estimate the energetic costs of locomotion in snow, which, paired with GPS and accelerometer data could be used to determine the influence of snow conditions on the rate of depletion of somatic reserves of northern ungulates during winter.
During summer, we did not detect any influence of growing degree days on somatic energy reserves among female moose in early winter, even during years with relatively warm summer temperatures (e.g., 2016, 2019). Moose in this study at lower elevations should have experienced more growing degree days but could have also been exposed to warmer daily temperatures. Warm summer temperatures can decrease forage quality, forage intake, and alter behavior of moose (Bowyer et al., 1998; Street et al., 2015; Montgomery et al., 2019; Shively et al., 2019), which could have negated the effect of increased growing degree days on accumulation of somatic energy reserves for moose in this study. Warm summers can negatively impact the accumulation rate of somatic reserves in mule deer (Odocoileus hemionus) and reindeer (Monteith et al., 2013; Trondrud et al., 2023). Conversely, warm summers did not influence somatic reserve accumulation in elk (Cervus elaphus; Stewart et al., 2005). Although snow depth and summer growing degree days were not significant factors influencing accumulation of somatic reserves among moose in our study, these factors could influence the quality and quantity of summer forage through small changes to the nutritional landscape and could influence accumulation of somatic reserves at scales not detectable with our dataset through multiplier effects (White, 1983).
The nutritional landscape plays a critical role in the accumulation of somatic energy reserves among northern ungulates. During summer, we found that moose with home ranges exhibiting a higher percent cover of aspen within foraging height had more somatic reserves during early winter. Aspen stands with the highest percent cover were found in the early seral habitats associated with the Funny River Fire footprint (Supplementary Data Sheet 4). Aspen stands also had a higher diversity of other summer moose forage species (Supplementary Data Sheet 4, Supplementary Figure 4.1) including forbs (e.g., fireweed [Chamerion angustifolium; Chamerion latifolium]) and shrubs (e.g., prickly rose [Rosa acicularis]) compared with other habitat types (Supplementary Data Sheet 4). Consumption of a mixed diet by moose may maximize energy and protein consumption, while alleviating the negative effects of plant secondary metabolites (Iason and Villalba, 2006; Estell, 2010; Felton et al., 2021). Although we used percent cover as a proxy for forage available to moose during the summer (Monzingo et al., 2022), percent cover may not accurately reflect the actual biomass available on the landscape, which could be quantified through traditional methods (Oldemeyer and Regelin, 1987; Proffitt et al., 2016; Merems et al., 2020; Snobl et al., 2022). Likewise, we classified winter moose browse based on summer canopy cover, which may not have accurately reflected the actual density of available browse. We found a negative relationship in late winter somatic energy reserves among moose that had a higher percent of aspen cover in their winter home range. Conversely, we found a positive relationship with moose somatic reserves among individuals with a higher percent cover of willows and shoulder season forage in their winter home range. Previous studies on the Kenai Peninsula found greater willow densities per hectare within 10 years after wildland fire compared with aspen (Oldemeyer and Regelin, 1987) and reported that moose winter browse was considerably higher on willows compared with both aspen and Alaska birch (Weixelman et al., 1998; Miner, 2000). Additionally, during winter, biomass per diameter is 1.7 times greater per mm thickness for Barclay’s willow (Salix barclayi) compared with aspen (Welch et al., 2015). Such differences in stem densities, biomass per stem diameter, and moose preference, may account for the contrary responses in somatic energy reserves for moose with home ranges containing high percent cover of aspen or willows. Our results also emphasize the importance of shoulder season forage species that have been shown to influence late winter somatic reserves in other ungulates (Hurley et al., 2014; Long et al., 2016). These shoulder season forage species for moose (e.g., lowbush cranberry, bunchberry dogwood) have been documented to be important forage species for moose when snow depths are minimal (LeResche and Davis, 1973; Oldemeyer and Regelin, 1987; Weixelman et al., 1998). Our results reinforce the importance of a mosaic of vegetation communities and seral states across the landscape for moose populations (Joly et al., 2016; Brown et al., 2018; Mumma et al., 2024). Other aspects that could influence moose somatic reserves, such as the thermal landscape, also warrant further investigation (Long et al., 2014, 2016, Thompson et al., 2021; Verzuh et al., 2021).
Managing the nutritional landscape, either through habitat enhancement, silviculture, or fuel reduction to mitigate wildland fires, can increase the availability and quality of forage for northern ungulates (Long et al., 2008; Bergman et al., 2014; Cook et al., 2016; Ulappa et al., 2020), particularly for moose (Oldemeyer and Regelin, 1987; Collins and Schwartz, 1998; Paragi and Haggstrom, 2007; Fredriksson et al., 2023). Incorporating nutrition as a component of adaptable management plans is becoming more prevalent (Vales et al., 2017; Rowland et al., 2018). However, one limitation to managing the nutritional landscape is the uncertainty and severity of wildland fires (Rowland et al., 2018). Indeed, the frequency of wildland fires is increasing in temperate, boreal, and tundra ecosystems (Kasischke and Turetsky, 2006; Dennison et al., 2014; Masrur et al., 2022), with both positive and/or negative effects on northern ungulate populations (DeMars et al., 2019; Proffitt et al., 2019; Konkolics et al., 2021; Snobl et al., 2022; Mumma et al., 2024). In boreal forests, wildland fires are the largest annual ecological disturbance (Chapin et al., 2008; Kasischke et al., 2010) that provide early seral stage habitats ideal for moose (Lord and Kielland, 2015; Joly et al., 2016; Brown et al., 2018; DeMars et al., 2019). In recently burned areas of the boreal forest, the emerging nutritional landscape for moose is influenced by the plant communities prior to wildland fire and the burn severity (Shenoy et al., 2011; Wan et al., 2014; Johnstone et al., 2020). Vegetation in burned areas of the boreal forest quickly regenerates with moose forage quantity and quality typically peaking at 15–20 years after wildland fire (Schwartz and Franzmann, 1989) including a concomitant response in moose densities (Loranger et al., 1991). In Alaska, where state wildlife managers are mandated in certain areas to manage for high moose densities (Hundertmark and Schwartz, 1996; Young et al., 2006), increasing the nutritional carrying capacity of the landscape through interagency wildland fire management could help meet these management objectives. Developing a strategic approach to achieve this objective will require coordination between protecting and jurisdictional agencies through the Alaska Interagency Wildland Fire Management Plan. Interagency efforts to manage wildland fires on the landscape can create a mosaic of seral states beneficial to both sexes of moose (Bowyer, 2022), including shoulder season forages, while decreasing wildland fire fuel loads along the urban wildland interface and providing ecosystem services (Kasischke et al., 2010).
Data availability statement
Data for this project is available upon reasonable request from the Alaska Department of Fish and Game and subject to a data sharing agreement. Requests to access the datasets should be directed to dan.thompson2@alaska.gov.
Ethics statement
The animal study was approved by Alaska Department of Fish and Game Division of Wildlife Conservation Animal Care and Use Committee. The study was conducted in accordance with the local legislation and institutional requirements.
Author contributions
DT: Conceptualization, Data curation, Formal analysis, Funding acquisition, Investigation, Methodology, Project administration, Resources, Writing – original draft, Writing – review & editing. NF: Formal analysis, Software, Writing – review & editing. JC: Conceptualization, Investigation, Methodology, Writing – review & editing. TM: Conceptualization, Investigation, Methodology, Writing – review & editing. OB: Data curation, Writing – review & editing. MS: Formal analysis, Software, Writing – review & editing. DW: Funding acquisition, Investigation, Writing – review & editing. SR: Conceptualization, Funding acquisition, Writing – review & editing.
Funding
The author(s) declare financial support was received for the research, authorship, and/or publication of this article. This work was supported by the Alaska Department of Fish and Game Federal Wildlife Restoration Grant AKW-15, AKW-16 (Project No. 215647484), and AKW-33 (Project No. 1.72), and the U.S. Fish and Wildlife Service, Kenai National Wildlife Refuge.
Acknowledgments
We thank J. de Creeft (Western Data), T. Cambier (Chena River Aviation), M. Keech (Swift Fork Air), J. Fieldman (Soloy Helicopters), C. Ramsey (Regional Helicopters), and N. Olson (Kenai National Wildlife Refuge) for assistance with wild moose captures, parturition and calf survival surveys, and GPS collar retrieval.
Conflict of interest
The authors declare that the research was conducted in the absence of any commercial or financial relationships that could be construed as a potential conflict of interest.
Publisher’s note
All claims expressed in this article are solely those of the authors and do not necessarily represent those of their affiliated organizations, or those of the publisher, the editors and the reviewers. Any product that may be evaluated in this article, or claim that may be made by its manufacturer, is not guaranteed or endorsed by the publisher.
Supplementary material
The Supplementary Material for this article can be found online at: https://www.frontiersin.org/articles/10.3389/fevo.2024.1433485/full#supplementary-material
Supplementary Data Sheet 1 | Correlation of 5-minute activity values and 30-minute Euclidean distance collected from GPS collars deployed on moose on the Kenai Peninsula, Alaska, USA.
Supplementary Data Sheet 2 | Correlation of temperature collected from GPS collars deployed on moose and ambient air temperature on the Kenai Peninsula, Alaska, USA.
Supplementary Data Sheet 3 | Remote sensing data to estimate increases in energy cost for moose moving through snow on the Kenai Peninsula, Alaska, USA.
Supplementary Data Sheet 4 | Indexing available moose forage from field-based vegetation plots on the Kenai Peninsula, Alaska, USA.
Supplementary Data Sheet 5 | Box plots of data for variables use in linear mixed-effect model regression for percent body fat in adult female moose on the Kenai Peninsula, Alaska, USA (2015 – 2022).
Supplementary Data Sheet 6 | Seasonal model selection for influences on moose somatic reserves on the Kenai Peninsula, Alaska, USA.
References
Aanes R., Sæther B., Smith F. M., Cooper E. J., Wookey P. A., Øritsland N. A. (2002). The Arctic Oscillation predicts effects of climate change in two trophic levels in a high-arctic ecosystem. Ecol. Lett. 5, 445–453. doi: 10.1046/j.1461-0248.2002.00340.x
Aikens E. O., Kauffman M. J., Merkle J. A., Dwinnell S. P. H., Fralick G. L., Monteith K. L. (2017). The greenscape shapes surfing of resource waves in a large migratory herbivore. Ecol. Lett. 20, 741–750. doi: 10.1111/ele.12772
Barboza P. S., Parker K. L. (2008). Allocating protein to reproduction in Arctic reindeer and caribou. Physiol. Biochem. Zool. 81, 835–855. doi: 10.1086/590414
Barboza P. S., Parker K. L., Hume I. D. (2009). Integrative Wildlife Nutrition (Berlin, Heidelberg: Springer).
Barboza P. S., Shively R. D., Thompson D. P. (2024). Robust responses of female caribou to changes in food supply. Ecol. Evolutionary Physiol. 97, 29–52. doi: 10.1086/729668
Bates D., Mächler M., Bolker B., Walker S. (2015). Fitting linear mixed-effects models using lme4. J. Stat. Software 67, 1–48. doi: 10.18637/jss.v067.i01
Beattie D., Hill M. J., Thompson D. P., Rodman S. (2024). Evaluation of vegetation within the Funny River Fire footprint, GMU 15B (Juneau: Alaska Department of Fish and Game, Wildlife Technical Bulletin). In review.
Bellante G., Boucher T., Charnon B., Goetz W., Homan K., Pan C., et al. (2020). Kenai Peninsula Existing Vegetation Map Project (Salt Lake City, UT, USA: U.S. Department of Agriculture, Forest Service, Geospatial Technology and Applications Center), 39.
Benedict B. M., Barboza P. S. (2022). Adverse effects of Diptera flies on northern ungulates: Rangifer, Alces, and Bison. Mammal Rev. 52, 425–437. doi: 10.1111/mam.12287
Benedict B. M., Barboza P. S., Crouse J. A., Groch K. R., Kulpa M. R., Thompson D. P., et al. (2023). Sores of boreal moose reveal a previously unknown genetic lineage of parasitic nematode within the genus Onchocerca. PloS One 18, e0278886. doi: 10.1371/journal.pone.0278886
Benedict B. M., Thompson D. P., Crouse J. A., Hamer G. L., Barboza P. S. (2024). Wounded but unstressed: Moose tolerate injurious flies in the boreal forest. J. Mammal. in press. doi: 10.1093/jmammal/gyae081
Bergerud A. T., Butler H. E., Miller D. R. (1984). Antipredator tactics of calving caribou: Dispersion in mountains. Can. J. Zool. 62, 1566–1575. doi: 10.1139/z84-229
Bergman E. J., Doherty P. F., Bishop C. J., Wolfe L. L., Banulis B. A. (2014). Herbivore body condition response in altered environments: Mule deer and habitat management. PloS One 9, e106374. doi: 10.1371/journal.pone.0106374
Bertram M. R., Vivion M. T. (2002). Moose mortality in eastern interior Alaska. J. Wildlife Manage. 66, 747–756. doi: 10.2307/3803140
Blum M. E., Stewart K. M., Shoemaker K. T., Cox M., Wakeling B. F., Dilts T. E., et al. (2023). Changes in selection of resources with reproductive state in a montane ungulate. Movement Ecol. 11, 20. doi: 10.1186/s40462-023-00378-1
Boertje R. D., Frye G. G., Young D. D. (2019). Lifetime, known-age moose reproduction in a nutritionally stressed population. J. Wildlife Manage. 83, 610–626. doi: 10.1002/jwmg.21613
Boertje R. D., Kellie K. A., Seaton C. T., Keech M. A., Young D. D., Dale B. W., et al. (2007). Ranking Alaska moose nutrition: Signals to begin liberal antlerless harvests. J. Wildlife Manage. 71, 1494–1506. doi: 10.2193/2006-159
Bowyer R. T. (2022). Sexual segregation in ungulates: ecology, behavior, and conservation (Baltimore, Maryland: John Hopkins University Press).
Bowyer R. T., Van Ballenberghe V., Kie J. G. (1998). Timing and synchrony of parturition in Alaskan moose: Long-term versus proximal effects of climate. J. Mammal. 79, 1332–1344. doi: 10.2307/1383025
Bowyer R. T., Van Ballenberghe V., Kie J. G., Maier J. A. K. (1999). Birth-site selection by Alaskan moose: Maternal strategies for coping with a risky environment. J. Mammal. 80, 1070–1083. doi: 10.2307/1383161
Brown C. L., Kielland K., Euskirchen E. S., Brinkman T. J., Ruess R. W., Kellie K. A. (2018). Fire-mediated patterns of habitat use by male moose (Alces alces) in Alaska. Can. J. Zool. 96, 183–192. doi: 10.1139/cjz-2017-0069
Burnham K. P., Anderson D. R. (2002). Model selection and multimodel inference: a practical information-theoretic approach. 2nd ed (New York: Springer).
Cain J. W. III, Kay J. H., Liley S. G., Gedir J. V. (2024). Mule deer (Odocoileus hemionus) resource selection: trade-offs between forage and predation risk. Front. Ecol. Evol. 12, 1121439. doi: 10.3389/fevo.2024.1121439
Chapin III, F. S. (1983). Direct and indirect effects of temperature on arctic plants. Polar Biol. 2, 47–52. doi: 10.1007/BF00258285
Chapin F. S., Trainor S. F., Huntington O., Lovecraft A. L., Zavaleta E., Natcher D. C., et al. (2008). Increasing wildfire in Alaska’s boreal forest: Pathways to potential solutions of a wicked problem. BioScience 58, 531–540. doi: 10.1641/B580609
Collins W. B., Schwartz C. C. (1998). Logging in Alaska’s boreal forest: Creation of grasslands or enhancement of moose habitat. Alces 34, 355–374. Available at: https://alcesjournal.org/index.php/alces/article/view/761.
Cook J. G., Cook R. C., Davis R. W., Irwin L. L. (2016). Nutritional ecology of elk during summer and autumn in the Pacific Northwest. Wildlife Monogr. 195, 1–81. doi: 10.1002/wmon.1020
Cook R. C., Cook J. G., Vales D. J., Johnson B. K., Mccorquodale S. M., Shipley L. A., et al. (2013). Regional and seasonal patterns of nutritional condition and reproduction in elk. Wildlife Monogr. 184, 1–45. doi: 10.1002/wmon.1008
Cook J. G., Kelly A. P., Cook R. C., Culling B., Culling D., McLaren A., et al. (2021a). Seasonal patterns in nutritional condition of caribou (Rangifer tarandus) in the southern Northwest Territories and northeastern British Columbia, Canada. Can. J. Zool. 99, 845–858. doi: 10.1139/cjz-2021-0057
Cook R. C., Oyster J., Mansfield K., Harris R. B. (2021b). Evidence of summer nutritional limitations in a Northeastern Washington moose population. Alces 57, 23–46. Available at: https://alcesjournal.org/index.php/alces/article/view/289.
Creel S., Winnie J., Maxwell B., Hamlin K., Creel M. (2005). Elk alter habitat selection as an antipredator response to wolves. Ecology 86, 3387–3397. doi: 10.1890/05-0032
DeMars C. A., Serrouya R., Mumma M. A., Gillingham M. P., McNay R. S., Boutin S. (2019). Moose, caribou, and fire: Have we got it right yet? Can. J. Zool. 97, 866–879. doi: 10.1139/cjz-2018-0319
Dennison P. E., Brewer S. C., Arnold J. D., Moritz M. A. (2014). Large wildfire trends in the western United States 1984-2011. Geophysical Res. Lett. 41, 2928–2933. doi: 10.1002/2014GL059576
Denryter K., Cook R. C., Cook J. G., Parker K. L. (2022). Animal-defined resources reveal nutritional inadequacies for woodland caribou during summer–autumn. J. Wildlife Manage. 86, e22161. doi: 10.1002/jwmg.22161
Ditmer M. A., Moen R. A., Windels S. K., Forester J. D., Ness T. E., Harris T. R. (2018). Moose at their bioclimatic edge alter their behavior based on weather, landscape, and predators. Curr. Zool. 64, 419–432. doi: 10.1093/cz/zox047
Dwinnell S. P. H., Sawyer H., Randall J. E., Beck J. L., Forbey J. S., Fralick G. L., et al. (2019). Where to forage when afraid: Does perceived risk impair use of the foodscape? Ecol. Appl. 29, e01972. doi: 10.1002/eap.1972
Ellingwood D. D., Pekins P. J., Jones H., Musante A. R. (2020). Evaluating moose Alces alces population response to infestation level of winter ticks Dermacentor albipictus. Wildlife Biol. 2020 (2), wlb.00619. doi: 10.2981/wlb.00619
Estell R. E. (2010). Coping with shrub secondary metabolites by ruminants. Small Ruminant Res. 94, 1–9. doi: 10.1016/j.smallrumres.2010.09.012
Fancy S. G., White R. G. (1985). Energy expenditures by caribou while cratering in snow. J. Wildlife Manage. 49, 987–993. doi: 10.2307/3801384
Felton A. M., Wam H. K., Felton A., Simpson S. J., Stolter C., Hedwall P., et al. (2021). Macronutrient balancing in free-ranging populations of moose. Ecol. Evol. 11, 11223–11240. doi: 10.1002/ece3.7909
Francis A. L., Procter C., Kuzyk G., Fisher J. T. (2021). Female moose prioritize forage over Mortality risk in harvested landscapes. J. Wildlife Manage. 85, 156–168. doi: 10.1002/jwmg.21963
Fredriksson E., Wallgren M., Löfroth T. (2023). Wildfire and prescribed burning impact moose forage availability and browsing levels in the northern boreal forest. Scandinavian J. For. Res. 38, 58–69. doi: 10.1080/02827581.2023.2184489
Gasaway W. C., Harkness D. B., Rausch R. A. (1978). Accuracy of moose age determinations from Incisor cementum layers. J. Wildl. Manage. 42, 558–563. doi: 10.2307/3800818
Grunenwald C. M., Butler E., Wünschmann A., Armien A. G., Carstensen M., Hildebrand E., et al. (2018). Emergence of the arterial worm Elaeophora schneideri in moose (Alces alces) and tabanid fly vectors in northeastern Minnesota, USA. Parasites Vectors 11, 507. doi: 10.1186/s13071-018-3077-0
Grunenwald C. M., Carstensen M., Hildebrand E., Elam J., Laaksonen S., Oksanen A., et al. (2016). Epidemiology of the lymphatic-dwelling filarioid nematode Rumenfilaria andersoni in free-ranging moose (Alces alces) and other cervids of North America. Parasites Vectors 9, 450. doi: 10.1186/s13071-016-1740-x
Hamel S., Côté S. D. (2007). Habitat use patterns in relation to escape terrain: are alpine ungulate females trading off better foraging sites for safety? Can. J. Zool. 85, 933–943. doi: 10.1139/Z07-080
Hamel S., Côté S. D. (2008). Trade-offs in activity budget in an alpine ungulate: contrasting lactating and nonlactating females. Anim. Behav. 75, 217–227. doi: 10.1016/j.anbehav.2007.04.028
Hamel S., Côté S. D. (2009). Foraging decisions in a capital breeder: Trade-offs between mass gain and lactation. Oecologia 161, 421–432. doi: 10.1007/s00442-009-1377-y
Huang F., Cockrell D. C., Stephenson T. R., Noyes J. H., Sasser R. G. (2000). A serum pregnancy test with a specific radioimmunoassay for moose and elk pregnancy-specific protein B. J. Wildlife Manage. 64, 492–499. doi: 10.2307/3803246
Hundertmark K. J., Schwartz C. C. (1996). Considerations for intensive management of moose in Alaska. Alces 32, 15–24. Available at: https://alcesjournal.org/index.php/alces/article/view/833.
Hurley M. A., Hebblewhite M., Gaillard J.-M., Dray S., Taylor K. A., Smith W. K., et al. (2014). Functional analysis of Normalized Difference Vegetation Index curves reveals overwinter mule deer survival is driven by both spring and autumn phenology. Philos. Trans. R. Soc. B: Biol. Sci. 369, 20130196. doi: 10.1098/rstb.2013.0196
Iason G. R., Villalba J. J. (2006). Behavioral strategies of mammal herbivores against plant secondary metabolites: The avoidance–tolerance continuum. J. Chem. Ecol. 32, 1115–1132. doi: 10.1007/s10886-006-9075-2
Jesmer B. R., Kauffman M. J., Courtemanch A. B., Kilpatrick S., Thomas T., Yost J., et al. (2021). Life-history theory provides a framework for detecting resource limitation: a test of the nutritional buffer hypothesis. Ecol. Appl. 31, e02299. doi: 10.1002/eap.2299
Johnson H. E., Golden T. S., Adams L. G., Gustine D. D., Lenart E. A., Barboza P. S. (2021). Dynamic selection for forage quality and quantity in response to phenology and insects in an Arctic ungulate. Ecol. Evol. 11, 11664–11688. doi: 10.1002/ece3.7852
Johnson H. E., Lenart E. A., Gustine D. D., Adams L. G., Barboza P. S. (2022). Survival and reproduction in arctic caribou are associated with summer forage and insect harassment. Front. Ecol. Evol. 10, 899585. doi: 10.3389/fevo.2022.899585
Johnstone J. F., Celis G., Iii F. S. C., Hollingsworth T. N., Jean M., Mack M. C. (2020). Factors shaping alternate successional trajectories in burned black spruce forests of Alaska. Ecosphere 11, e03129. doi: 10.1002/ecs2.3129
Joly K., Sorum M. S., Craig T., Julianus E. L. (2016). The effects of sex, terrain, wildfire, winter severity, and maternal status on habitat selection by moose in north-Central Alaska. Alces 52, 101–115. Available at: https://alcesjournal.org/index.php/alces/article/view/165.
Jönsson K. I. (1997). Capital and income breeding as alternative tactics of resource use in reproduction. Oikos 78, 57. doi: 10.2307/3545800
Kasischke E. S., Turetsky M. R. (2006). Recent changes in the fire regime across the North American boreal region—Spatial and temporal patterns of burning across Canada and Alaska. Geophysical Res. Lett. 33, Lo9703. doi: 10.1029/2006GL025677
Kasischke E. S., Verbyla D. L., Rupp T. S., McGuire A. D., Murphy K. A., Jandt R., et al. (2010). Alaska’s changing fire regime — implications for the vulnerability of its boreal forests. Can. J. For. Res. 40, 1313–1324. doi: 10.1139/X10-098
Keech M. A., Bowyer R. T., Hoef J. M. V., Boertje R. D., Dale B. W., Stephenson T. R. (2000). Life-history consequences of maternal condition in Alaskan moose. J. Wildlife Manage. 64, 450–462. doi: 10.2307/3803243
Kelsey K. C., Pedersen S. H., Leffler A. J., Sexton J. O., Feng M., Welker J. M. (2021). Winter snow and spring temperature have differential effects on vegetation phenology and productivity across Arctic plant communities. Global Change Biol. 27, 1572–1586. doi: 10.1111/gcb.15505
Kirchner T. (2024). Do you mind? Using biologging tools to study anthropogenic disturbance effects on wildlife behavior and energetics – a case study on moose. Evenstad, Norway: Inland Norway University of Applied Sciences.
Konkolics S., Dickie M., Serrouya R., Hervieux D., Boutin S. (2021). A burning question: what are the implications of forest fires for woodland Caribou? J. Wildlife Manage. 85, 1685–1698. doi: 10.1002/jwmg.22111
Kraft B. R. (2011). Energetics and space use of female moose during winter. Fairbanks, Alaska, USA: University of Alaska, Fairbanks.
Kutz S. J., Ducrocq J., Hoar B. M., Colwell D. D., Beckmen K. B., Polley L., et al. (2012). “Parasites in ungulates of Arctic North America and Greenland: A view of contemporary diversity, ecology, and impact in a world under change,” in Advances in Parasitology. Eds. Rollinson D., Hay S. I. (Academic Press, Amsterdam), 99–252.
Kutz S. J., Jenkins E. J., Veitch A. M., Ducrocq J., Polley L., Elkin B., et al. (2009). The Arctic as a model for anticipating, preventing, and mitigating climate change impacts on host–parasite interactions. Veterinary Parasitol. 163, 217–228. doi: 10.1016/j.vetpar.2009.06.008
Laaksonen S., Kuusela J., Nikander S., Nylund M., Oksanen A. (2007). Outbreak of parasitic peritonitis in reindeer in Finland. Veterinary Rec. 160, 835–841. doi: 10.1136/vr.160.24.835
Laaksonen S., Solismaa M., Orro T., Kuusela J., Saari S., Kortet R., et al. (2009). Setaria tundra microfilariae in reindeer and other cervids in Finland. Parasitol. Res. 104, 257–265. doi: 10.1007/s00436-008-1184-0
LeResche R. E., Davis J. L. (1973). Importance of nonbrowse foods to moose on the Kenai Peninsula, Alaska. J. Wildlife Manage. 37, 279–287. doi: 10.2307/3800118
Levine R. L., Smiley R. A., Jesmer B. R., Oates B. A., Stephenson T. R., Kauffman M. J., et al. (2022). Extending body condition scoring beyond measurable rump fat to estimate full range of nutritional condition for moose. Alces 58, 91–99. Available at: https://alcesjournal.org/index.php/alces/article/view/1883
Long R. A., Bowyer R. T., Porter W. P., Mathewson P., Monteith K. L., Findholt S. L., et al. (2016). Linking habitat selection to fitness−related traits in herbivores: The role of the energy landscape. Oecologia 181, 709–720. doi: 10.1007/s00442-016-3604-7
Long R. A., Bowyer R. T., Porter W. P., Mathewson P., Monteith K. L., Kie J. G. (2014). Behavior and nutritional condition buffer a large-bodied endotherm against direct and indirect effects of climate. Ecol. Monogr. 84, 513–532. doi: 10.1890/13-1273.1
Long R. A., Rachlow J. L., Kie J. G., Vavra M. (2008). Fuels reduction in a Western coniferous forest: Effects on quantity and quality of forage for elk. Rangeland Ecol. Manage. 61, 302–313. doi: 10.2111/07-046.1
Loranger A. J., Bailey T. N., Larned W. W. (1991). Effects of forest succession after fire in moose wintering habitats on the Kenai Peninsula, Alaska. Alces 27, 100–109. Available at: https://alcesjournal.org/index.php/alces/article/view/1105
Lord R., Kielland K. (2015). Effects of variable fire severity on forage production and foraging behavior of moose in winter. Alces 51, 23–34. Available at: https://alcesjournal.org/index.php/alces/article/view/147
Masrur A., Taylor A., Harris L., Barnes J., Petrov A. (2022). Topography, climate and fire history regulate wildfire activity in the Alaskan tundra. J. Geophysical Res.: Biogeosci. 127, e2021JG006608. doi: 10.1029/2021JG006608
Mautz W. W. (1978). Sledding on a bushy hillside: the fat cycle in deer. Wildlife Soc. Bull. 6, 88–90. Available at: https://www.jstor.org/stable/3781295.
McArt S. H., Spalinger D. E., Collins W. B., Schoen E. R., Stevenson T., Bucho M. (2009). Summer dietary nitrogen availability as a potential bottom-up constraint on moose in south-central Alaska. Ecology 90, 1400–1411. doi: 10.1890/08-1435.1
McDonough T. J., Thompson D. P., Crouse J. A., Dale B. W., Badajos O. H. (2022). Evaluation of impacts of vaginal implant transmitter use in moose. Wildlife Soc. Bull. 46, e1378. doi: 10.1002/wsb.1378
Melin M., Matala J., Mehtätalo L., Pusenius J., Packalen T. (2023). The effect of snow depth on movement rates of GPS-collared moose. Eur. J. Wildlife Res. 69, 21. doi: 10.1007/s10344-023-01650-w
Merems J. L., Shipley L. A., Levi T., Ruprecht J., Clark D. A., Wisdom M. J., et al. (2020). Nutritional-landscape models link habitat use to condition of mule deer (Odocoileus hemionus). Front. Ecol. Evol. 8, 98. doi: 10.3389/fevo.2020.00098
Merkle J. A., Monteith K. L., Aikens E. O., Hayes M. M., Hersey K. R., Middleton A. D., et al. (2016). Large herbivores surf waves of green-up during spring. Proc. R. Soc. B: Biol. Sci. 283, 20160456. doi: 10.1098/rspb.2016.0456
Middleton A. D., Merkle J. A., McWhirter D. E., Cook J. G., Cook R. C., White P. J., et al. (2018). Green-wave surfing increases fat gain in a migratory ungulate. Oikos 127, 1060–1068. doi: 10.1111/oik.05227
Miner B. (2000). Forest regeneration and use of browse by moose in large-scale wildfires and managed habitat areas, Kenai National Wildlife Refuge, Alaska. Anchorage, Alaska, USA: Alaska Pacific University.
Miquelle D. G., Peek J. M., Ballenberghe V. V. (1992). Sexual segregation in Alaskan moose. Wildlife Monogr. 122, 1–57. Available at: https://www.jstor.org/stable/3830827.
Mitchell D., Snelling E. P., Hetem R. S., Maloney S. K., Strauss W. M., Fuller A. (2018). Revisiting concepts of thermal physiology: Predicting responses of mammals to climate change. J. Anim. Ecol. 87, 956–973. doi: 10.1111/1365-2656.12818
Monteith K. L., Bleich V. C., Stephenson T. R., Pierce B. M., Conner M. M., Kie J. G., et al. (2014). Life-history characteristics of mule deer: Effects of nutrition in a variable environment. Wildlife Monogr. 186, 1–62. doi: 10.1002/wmon.1011
Monteith K. L., Stephenson T. R., Bleich V. C., Conner M. M., Pierce B. M., Bowyer R. T. (2013). Risk-sensitive allocation in seasonal dynamics of fat and protein reserves in a long-lived mammal. J. Anim. Ecol. 82, 377–388. doi: 10.1111/1365-2656.12016
Montgomery R. A., Redilla K. M., Moll R. J., Van Moorter B., Rolandsen C. M., Millspaugh J. J., et al. (2019). Movement modeling reveals the complex nature of the response of moose to ambient temperatures during summer. J. Mammal. 100, 169–177. doi: 10.1093/jmammal/gyy185
Monzingo D. S., Cook J. G., Cook R. C., Horne J. S., Shipley L. A. (2023). Influences of succession and biogeoclimate on forage resources for elk in Northern Idaho. Northwest Sci. 96, 94–116. doi: 10.3955/046.096.0107
Monzingo D. S., Shipley L. A., Cook R. C., Cook J. G. (2022). Factors influencing predictions of understory vegetation biomass from visual cover estimates. Wildlife Soc. Bull. 46, e1300. doi: 10.1002/wsb.1300
Mörschel F. M., Klein D. R. (1997). Effects of weather and parasitic insects on behavior and group dynamics of caribou of the Delta Herd, Alaska. Can. J. Zool. 75, 1659–1670. doi: 10.1139/z97-793
Mumma M. A., Bevington A. R., Marshall S., Gillingham M. P. (2024). Delineating wildfire burns and regrowth using satellite imagery to assess moose (Alces alces) spatial responses to burns. Ecosphere 15, e4793. doi: 10.1002/ecs2.4793
Natural Resources Conservation Service (2024). Snow telemetry SNOTEL and snow course data and products. Available at: https://www.nrcs.usda.gov/wps/portal/wcc/ home/.
Nielson M. R., Sawyer H., McDonald T. L. (2013). _BBMM: Brownian bridge movement model_. Available at: https://CRAN.R-project.org/package=BBMM.
Oates B. A., Monteith K. L., Goheen J. R., Merkle J. A., Fralick G. L., Kauffman M. J. (2021). Detecting resource limitation in a large herbivore population is enhanced with measures of nutritional condition. Front. Ecol. Evol. 8, 522174. doi: 10.3389/fevo.2020.522174
Oldemeyer J. L., Regelin W. L. (1987). Forest succession, habitat management, and moose on the Kenai National Wildlife Refuge. Swedish Wildlife Res. Supplement 1, 163–179.
Paragi T. F., Haggstrom D. A. (2007). Short-term responses of aspen to fire and mechanical treatments in interior Alaska. Northern J. Appl. Forestry 24, 153–157. doi: 10.1093/njaf/24.2.153
Parker K. L., Barboza P. S., Gillingham M. P. (2009). Nutrition integrates environmental responses of ungulates. Funct. Ecol. 23, 57–69. doi: 10.1111/j.1365-2435.2009.01528.x
Parker K. L., Robbins C. T., Hanley T. A. (1984). Energy expenditures for locomotion by mule deer and elk. J. Wildlife Manage. 48, 474–488. doi: 10.2307/3801180
Patterson B. R., Benson J. F., Middel K. R., Mills K. J., Silver A., Obbard M. E. (2013). Moose calf mortality in central Ontario, Canada. J. Wildlife Manage. 77, 832–841. doi: 10.1002/jwmg.516
Pekins P. J., Smith K. S., Mautz W. W. (1998). The energy cost of gestation in white-tailed deer. Can. J. Zool. 76, 1091–1097. doi: 10.1139/z98-032
Poole K. G., Serrouya R., Stuart-Smith K. (2007). Moose calving strategies in interior montane ecosystems. J. Mammal. 88, 139–150. doi: 10.1644/06-MAMM-A-127R1.1
Proffitt K. M., DeVoe J., Barker K., Durham R., Hayes T., Hebblewhite M., et al. (2019). A century of changing fire management alters ungulate forage in a wildfire-dominated landscape. Forestry: Int. J. For. Res. 92, 523–537. doi: 10.1093/forestry/cpz017
Proffitt K. M., Hebblewhite M., Peters W., Hupp N., Shamhart J. (2016). Linking landscape-scale differences in forage to ungulate nutritional ecology. Ecol. Appl. 26, 2156–2174. doi: 10.1002/eap.1370
R Core Team (2023). R: A language and environment for statistical computing (Vienna, Austria: R Foundation for Statistical Computing).
Reese E. O., Robbins C. T. (1994). Characteristics of moose lactation and neonatal growth. Can. J. Zool. 72, 953–957. doi: 10.1139/z94-130
Rowland M. M., Wisdom M. J., Nielson R. M., Cook J. G., Cook R. C., Johnson B. K., et al. (2018). Modeling elk nutrition and habitat use in western Oregon and Washington. Wildlife Monogr. 199, 1–69. doi: 10.1002/wmon.1033
Ruprecht J. S., Hersey K. R., Hafen K., Monteith K. L., DeCesare N. J., Kauffman M. J., et al. (2016). Reproduction in moose at their southern range limit. J. Mammal. 97, 1355–1365. doi: 10.1093/jmammal/gyw099
Sasser R. G., Ruder C. A., Ivani K. A., Butler J. E., Hamilton W. C. (1986). Detection of pregnancy by radioimmunoassay of a novel pregnancy-specific protein in serum of cows and a profile of serum concentrations during gestation. Biol. Reprod. 35, 936–942. doi: 10.1095/biolreprod35.4.936
Schwartz C. C., Franzmann A. W. (1989). Bears, wolves, moose and forest succession, some management considerations on the Kenai Peninsula, Alaska. Alces 25, 1–10. Available at: https://alcesjournal.org/index.php/alces/article/view/1179.
Sergeant D. E., Pimlott D. H. (1959). Age determination in moose from sectioned incisor teeth. J Wildl Manage. 23, 315–321. doi: 10.2307/3796891
Severud W. J., DelGiudice G. D., Obermoller T. R. (2019). Association of moose parturition and post-parturition habitat with calf survival. J. Wildlife Manage. 83, 175–183. doi: 10.1002/jwmg.21570
Shenoy A., Johnstone J. F., Kasischke E. S., Kielland K. (2011). Persistent effects of fire severity on early successional forests in interior Alaska. For. Ecol. Manage. 261, 381–390. doi: 10.1016/j.foreco.2010.10.021
Shively R. D., Crouse J. A., Thompson D. P., Barboza P. S. (2019). Is summer food intake a limiting factor for boreal browsers? Diet, temperature, and reproduction as drivers of consumption in female moose. PloS One 14, e0223617. doi: 10.1371/journal.pone.0223617
Shochat E., Robbins C. T. (1997). Nutrition and behavioral management of bottle-raised moose calves. Zoo Biol. 16, 495–503. doi: 10.1002/(ISSN)1098-2361
Signer C., Ruf T., Schober F., Fluch G., Paumann T., Arnold W. (2010). A versatile telemetry system for continuous measurement of heart rate, body temperature and locomotor activity in free-ranging ruminants. Methods Ecol. Evol. 1, 75–85. doi: 10.1111/j.2041-210X.2009.00010.x
Sikes R. S., The Animal Care and Use Committee of the American Society of Mammalogists (2016). 2016 Guidelines of the American Society of Mammalogists for the use of wild mammals in research and education. J. Mammal. 97, 663–688. doi: 10.1093/jmammal/gyw078
Snobl L. A., Proffitt K. M., Millspaugh J. J. (2022). Wildfire extends the shelf life of elk nutritional resources regardless of fire severity. Ecosphere 13, e4178. doi: 10.1002/ecs2.4178
Stephens P. A., Boyd I. L., McNamara J. M., Houston A. I. (2009). Capital breeding and income breeding: their meaning, measurement, and worth. Ecology 90, 2057–2067. doi: 10.1890/08-1369.1
Stephenson T. R., Hundertmark K. J., Schwartz C. C., Ballenberghe V. V. (1998). Predicting body fat and body mass in moose with ultrasonography. Can. J. Zool. 76, 717–722. doi: 10.1139/z97-248
Stewart K. M., Terry Bowyer R., Dick B. L., Johnson B. K., Kie J. G. (2005). Density-dependent effects on physical condition and reproduction in North American elk: An experimental test. Oecologia 143, 85–93. doi: 10.1007/s00442-004-1785-y
Street G. M., Rodgers A. R., Fryxell J. M. (2015). Mid-day temperature variation influences seasonal habitat selection by moose. J. Wildlife Manage. 79, 505–512. doi: 10.1002/jwmg.859
Sullender B. K., Cunningham C. X., Lundquist J. D., Prugh L. R. (2023). Defining the danger zone: critical snow properties for predator–prey interactions. Oikos 2023, e09925. doi: 10.1111/oik.09925
Taillon J., Barboza P. S., Côté S. D. (2013). Nitrogen allocation to offspring and milk production in a capital breeder. Ecology 94, 1815–1827. doi: 10.1890/12-1424.1
Testa J. W., Adams G. P. (1998). Body condition and adjustments to reproductive effort in female moose (Alces alces). J. Mammal. 79, 1345–1354. doi: 10.2307/1383026
Thompson D. P., Barboza P. S. (2014). Nutritional implications of increased shrub cover for caribou (Rangifer tarandus) in the Arctic. Can. J. Zool. 92, 339–351. doi: 10.1139/cjz-2013-0265
Thompson D. P., Crouse J. A., Barboza P. S., Spathelf M. O., Herberg A. M., Parker S. D., et al. (2021). Behaviour influences thermoregulation of boreal moose during the warm season. Conserv. Physiol. 9, coaa130. doi: 10.1093/conphys/coaa130
Thompson D. P., Crouse J. A., Crouse S., Newberry S. M., Benedict B. M. (2023). Growth and vital signs of hand-raised moose calves in Alaska. Alces 59, 53–67. Available at: https://alcesjournal.org/index.php/alces/article/view/1937.
Thompson D. P., Crouse J. A., McDonough T. J., Badajos O. H., Adsem J., Barboza P. S. (2018). Vaginal implant transmitters for continuous body temperature measurement in moose. Wildlife Soc. Bull. 42, 321–327. doi: 10.1002/wsb.857
Thornton M. M., Shrestha R., Wei Y., Thornton P. E., Kao S.-C., Wilson B. E. (2024). Daymet: Daily Surface Weather Data on a 1-km Grid for North America, Version 4 R1 (Oak Ridge, Tennessee, USA). doi: 10.3334/ORNLDAAC/2129
Trondrud L. M., Pigeon G., Albon S., Arnold W., Evans A. L., Irvine R. J., et al. (2021). Determinants of heart rate in Svalbard reindeer reveal mechanisms of seasonal energy management. Philos. Trans. R. Soc. B: Biol. Sci. 376, 20200215. doi: 10.1098/rstb.2020.0215
Trondrud L. M., Pigeon G., Król E., Albon S., Ropstad E., Kumpula J., et al. (2023). A summer heat wave reduced activity, heart rate, and autumn body mass in a cold-adapted ungulate. Physiol. Biochem. Zool. 96, 282–293. doi: 10.1086/725363
Ulappa A. C., Shipley L. A., Cook R. C., Cook J. G., Swanson M. E. (2020). Silvicultural herbicides and forest succession influence understory vegetation and nutritional ecology of black-tailed deer in managed forests. For. Ecol. Manage. 470–471, 118216. doi: 10.1016/j.foreco.2020.118216
Vales D. J., Middleton M. P., McDaniel M. (2017). A nutrition-based approach for elk habitat management on intensively managed forestlands. J. Forestry 115, 406–415. doi: 10.5849/jof.16-032
Verzuh T. L., Hall L. E., Cufaude T., Knox L., Class C., Monteith K. L. (2021). Behavioural flexibility in a heat-sensitive endotherm: the role of bed sites as thermal refuges. Anim. Behav. 178, 77–86. doi: 10.1016/j.anbehav.2021.05.020
Wan H. Y., Rhodes A. C., St. Clair S. B. (2014). Fire severity alters plant regeneration patterns and defense against herbivores in mixed aspen forests. Oikos 123, 1479–1488. doi: 10.1111/oik.01521
Weixelman D. A., Bowyer R. T., Van Ballenberghe V. (1998). Diet selection by Alaskan moose during winter: effects of fire and forest succession. Alces 34, 213–238. Available at: ttps://alcesjournal.org/index.php/alces/article/view/739.
Welch J. H., Barboza P. S., Farley S. D., Spalinger D. E. (2015). Nutritional value of habitat for moose on urban and military lands. J. Fish Wildlife Manage. 6, 158–175. doi: 10.3996/062014-JFWM-045
White R. G. (1983). Foraging patterns and their multiplier effects on productivity of northern ungulates. Oikos 40, 377. doi: 10.2307/3544310
White K. S., Barten N. L., Crouse S., Crouse J. (2014). Benefits of migration in relation to nutritional condition and predation risk in a partially migratory moose population. Ecology 95, 225–237. doi: 10.1890/13-0054.1
Worden K. A., Pekins P. J. (1995). Seasonal change in feed intake, body composition, and metabolic rate of white-tailed deer. Can. J. Zool. 73, 452–457. doi: 10.1139/z95-052
Wünschmann A., Armien A. G., Butler E., Schrage M., Stromberg B., Bender J. B., et al. (2015). Necropsy findings in 62 opportunistically collected free-ranging moose (Alces alces) from Minnesota, USA, (2003-2013). J. Wildlife Dis. 51, 157–165. doi: 10.7589/2014-02-037
Young J. D.D., Boertje R. D., Seaton C. T., Kellie K. A. (2006). Intensive management of moose at high density: Impediments, achievements, and recommendations. Alces 42, 41–48. Available at: https://alcesjournal.org/index.php/alces/article/view/379.
Keywords: Alaska, Alces alces, body condition, moose, nutritional landscape, reproduction, somatic reserves, wildland fire
Citation: Thompson DP, Fowler NL, Crouse JA, McDonough TJ, Badajos OH, Spathelf MO, Watts DE and Rodman SU (2024) Seasonal somatic reserves of a northern ungulate influenced by reproduction and a fire-mediated landscape. Front. Ecol. Evol. 12:1433485. doi: 10.3389/fevo.2024.1433485
Received: 15 May 2024; Accepted: 26 August 2024;
Published: 12 September 2024.
Edited by:
Daniel Becker, University of Oklahoma, United StatesReviewed by:
R. Terry Bowyer, University of Alaska Fairbanks, United StatesBrock McMillan, Brigham Young University, United States
Copyright © 2024 Thompson, Fowler, Crouse, McDonough, Badajos, Spathelf, Watts and Rodman. This is an open-access article distributed under the terms of the Creative Commons Attribution License (CC BY). The use, distribution or reproduction in other forums is permitted, provided the original author(s) and the copyright owner(s) are credited and that the original publication in this journal is cited, in accordance with accepted academic practice. No use, distribution or reproduction is permitted which does not comply with these terms.
*Correspondence: Daniel P. Thompson, dan.thompson2@alaska.gov