- 1Department of Bioscience and Informatics, Faculty of Science and Technology, Keio University, Yokohama, Japan
- 2School of Frontier Engineering, Kitasato University, Sagamihara, Japan
Organismal transparency is an ecologically important trait that can provide camouflage advantages to diverse organisms. Transparent organisms are quite common—especially in oceans. Organismal transparency requires low absorption and scattering of light in the body across multi-scale levels. However, it is still not fully understood how such organisms achieve these requirements. Understanding this process requires multiple approaches from various fields and methods. Here, we offer recent insights on this topic from the viewpoints of evolution, developmental biology, and evaluation methodologies of organismal transparency. We also propose “organismal transparency biology” as a new interdisciplinary field of study. Furthermore, we suggest that tunicates are an ideal model animal for studying in vivo organismal transparency.
1 Introduction
Organismal transparency is an important camouflage strategy that aids in defense against visual predators. Organismal transparency is commonly seen in aquatic animals such as jellyfish, siphonophores, some crustaceans, pteropods, some squids, fish larvae and tunicates (McFall-Ngai, 1990; Johnsen, 2001, 2014; Kakiuchida et al., 2017; Bagge, 2019; Shito et al., 2020). Transparency requires that the species minimize both light absorption and scattering (Kerker, 1969). This involves equalizing the refractive index inside and outside the organism, altering its physical surface properties, and avoiding the creation of opaque organic molecules like pigments. Here, we introduce how light scattering, reflection, and absorption relate to organismal transparency and then describe the factors that contribute to improving transparency—namely optical cleaning agents (OCA).
1.1 Light absorption
As incident light passes through the interior of the organism, its intensity decreases due to light absorption. Most organic molecules in organisms do not absorb visible light except for pigments (Inyushin et al., 2019). Natural pigments and other molecules found in marine organisms have specific absorption wavelengths (Pettersen et al., 2014). Pigments absorb the light, and this feature protects animals from harmful UV radiation, transport oxygen by blood cells, and facilitating optical sensation. There are many different strategies to reduce light absorption of pigments. For example, icefish eliminate their own hemoglobin and have transparent blood cells (Ruud, 1954). Transparent glass frogs conceal their body by relocating approximately 89% of the red blood cells to the liver during sleep. Relocation of red blood cells to liver minimizes light absorption and scattering in other tissues, thus enhancing transparency at the whole-body level. Thus, transparency in this species increases by two- to three-fold due to the inability to see red blood cells (Taboada et al., 2022).
Transparency is not only achieved via the absence of pigmentation as in some albino mutants. A relatively transparent mutant lacking pigment (Wakamatsu et al., 2001) was created in medaka leading to transparency. Even in such cases, achieving perfect transparency is limited due to optical scattering. Transparency is also immediately opaque after the death of an transparent oocyte (Shito et al., 2023). This means that not having pigmentation is a minimum requirement for organismal transparency which is not an active strategy for organismal transparency (Bhandiwad and Johnsen, 2011).
The light absorption of living organisms also changes with metabolism (Table 1). For example, in anemone shrimp, the hemolymph penetrates the muscle fibers more when metabolic activity is high due to exercise, thus disrupting the uniformity of the refractive index of the tissue and making the muscle appear opaque (Bagge et al., 2017).
1.2 Light reflection
Light reflection arises at the interface between two optical media with different refractive indices. The main focus in this perspective is marine organismal transparency, which requires the organisms’ refractive index to be near seawater’s refractive index of 1.33. To achieve optimal transparency, the organism’s media should have uniform refractive indices across multiple scales (tissue, cell/Extracelllar Matrix (ECM), and organelles) (Figure 1). For example, the gelatinous body of transparent marine animals such as jellyfish and leptocephalus contains a lot of water, which allows their refractive index to remain transparent in seawater because it is not very different from seawater’s reflective index 1.33 (Pfeiler et al., 2002; Warrant and Locket, 2004). In the highly hydrated extracellular gelatinous body matrix of leptocephalus, the body water content is approximately 92% (Pfeiler and Govoni, 1993; Bishop et al., 2000). However, during metamorphosis to the adult eel, approximately 80% of the total body water content is lost (Pfeiler, 1984).
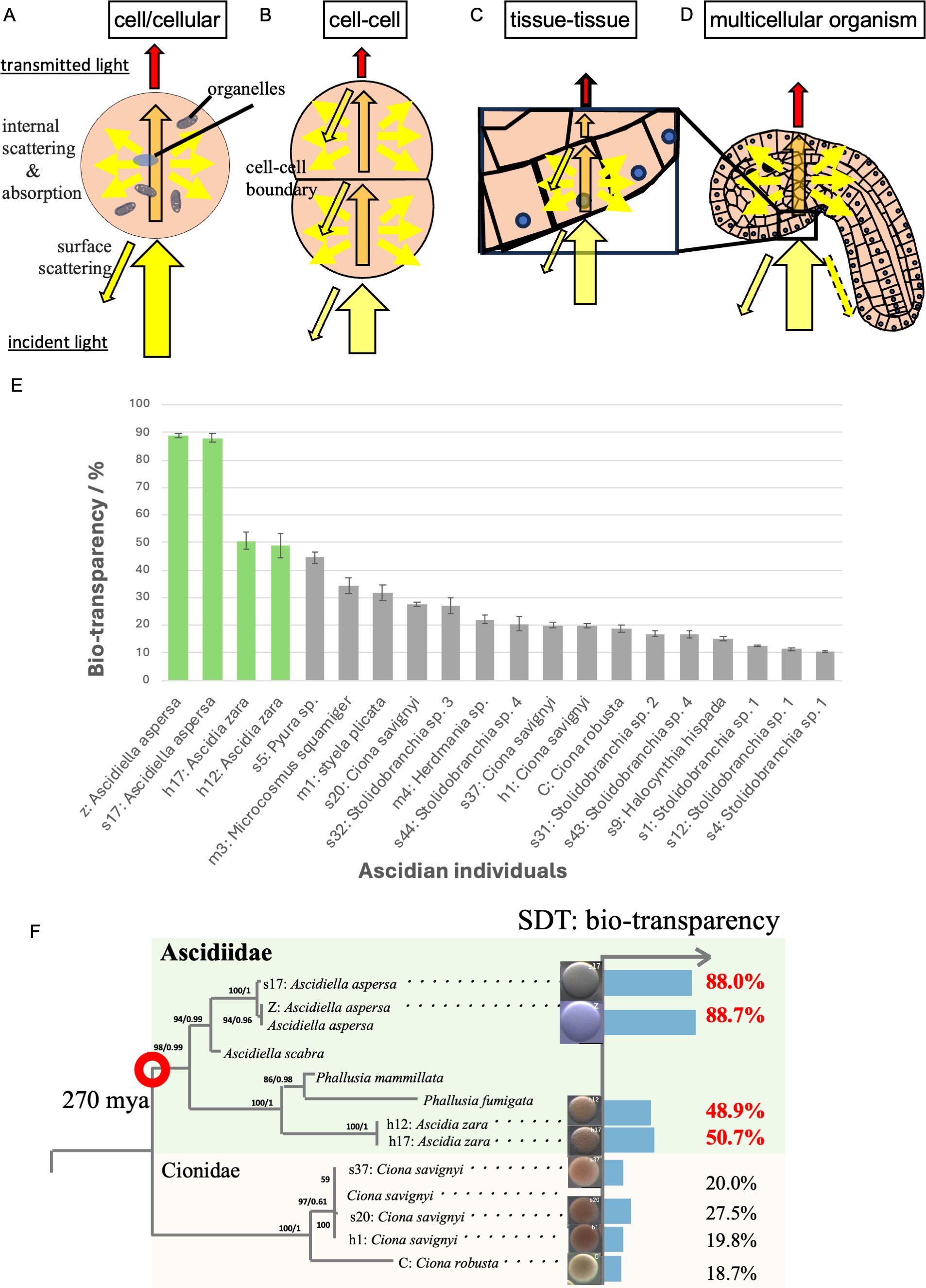
Figure 1. Possible multi-scale factors that inhibit organismal transparency in ascidian eggs. (A) In a single cell (oocyte in this case), light scattering within the cell is the surface scattering of the cell surface for incident light (black frame with yellow arrow), and the internal scattering in the presence of various organelles inside the cell. Due to the absorption and the internal scattering, the transmitted light (red arrow) is attenuated (orange arrow) when passing through the inside of the organism. (B) At the cell-cell boundary, surface scattering occurs due to the cell membrane and the ECM between the cells. (C) Similar to cells, surface scattering occurs between tissues with different refractive indexes. (D) There are ways to attenuate the surface scattering in some organisms by changing the surface ultra-structure (ex: nipple array). (E) Ascidian eggs have a variety of organismal transparency ranging from 10% to 90%, which is comparable to window glass. Green bars indicate species belonging to the Ascidiidae family. (F) Ascidiidae, generally characterized by high egg transparency, is believed to have diverged from Cionidae in ascidians approximately 270 million years ago (marked with red circle). This figure was modified from Shito et al. (2020).
1.3 Light scattering
Light scattering is divided into surface scattering (Bagge et al., 2016) on the cell surface for incident light, and internal scattering (Marina et al., 2012; Bagge et al., 2017) that occurs in the presence of various organelles inside the cell. In multicellular organisms, surface scattering occurs at the interface between cells, the cell membrane, and the ECM (Figure 1).
In surface scattering, certain organisms have reduced or eliminated this reflection through the array of sub-microscopic projections (Miller and Autrum, 1979; Wilson and Hutley, 1982; Kakiuchida et al., 2017). This structure does not scatter light but rather mimics a material with a graded reflective index (Johnsen, 2003). In the hyperiid Cystisoma, the leg surfaces are covered with tiny nanostructures, thus making the surface resemble long-pile carpet, thus weakening and softening surface light scattering at the interface (Bagge et al., 2016).
Many reports have suggested that transparent organisms employ various strategies to mitigate light absorption and scattering—these are crucial optical factors that facilitate their transparency (Bagge, 2019). Many animals are opaque because the density and refractive index of biological media vary at different spatial scales, i.e., individual, tissue/organ, cell, and organelle levels (Johnsen and Widder, 1999; Johnsen, 2014; Tarique et al., 2023). At the cellular level, the cornea and lens in the eye develop via degradation of organelles by autophagy (Brennan et al., 2023). In the lens cells, organelles such as mitochondria and the endoplasmic reticulum are degraded by a lipid-degrading enzyme from the PLAAT family. Inhibiting this process leads to lens transparency (Morishita et al., 2021).
It is necessary to devise ways to suppress light scattering at each boundary region between cells, tissues, and organs as described in Section 2.2. At this time, surface scattering on the cell surface is treated as internal scattering at the higher tissue level. In other words, what is treated as surface scattering at a lower level is regarded as internal scattering at higher level (Figure 1A).
1.4 Optical clearing agents
The presence of cellular organelles within the cells (Figure 1A) is thus a factor contributing to tissue opacification. Crystallins have a structure in which specific amino acid residues are arranged regularly, which suppresses light scattering and enhances transparency. The α-crystallin exhibits chaperone-like functions and solubilizes denatured βγ-crystallin (Rao et al., 1995). α-Crystallin prevents the aggregation of denatured βγ-crystallins by solubilizing them. Aggregated proteins scatter light within the lens, thus reducing transparency. α-Crystallin helps maintain a uniform refractive index within the lens by solubilizing denatured βγ-crystallins and preventing their aggregation, which can reduce light scattering and preserve lens transparency (Horwitz, 1992; Rao et al., 1995). In other words, crystallin molecules in the eye serve as natural endogenous optical clearing agents (OCA). Here, we defined OCA as materials—such as chemical substances, proteins, ECMs, or enzymes—that reduce light scattering and improve optical transparency in biological structures.
Endogenous OCAs have been reported in other transparent animal tissues as well, e.g., fish glycosaminoglycans and antifreeze proteins (see review (Inyushin et al., 2019).
1.5 The inhibiting factors challenging organismal transparency
Many animals are opaque due to the necessity of pigments for UV protection, thus illustrating a delicate balance in their survival (Hansson, 2000). However, as described previously, transparent organisms have a remarkable ability to overcome multiple inhibiting factors that challenge transparency. These factors include structural, physiological, and environmental aspects and cause optically reduces organismal transparency by light absorption and/or light scattering (Table 1).
Despite understanding of the inhibiting factors against organismal transparency, further research is needed to address the following questions: How has transparency evolved in specific lineages? How have organisms acquired transparency during development? What are the specific molecular mechanisms that enable transparency?
These answers require research in various fields and various scientific methods. Here, we discuss new perspectives: evolutionary genomics, developmental biology, and quantification and evaluation methodologies of organismal transparency. These areas have not received sufficient attention and should be studied further in the future.
2 Areas that should be enhanced to understand organismal transparency
2.1 Evolutionary genomics
Formal phylogenetic analysis with a quantitative measurement of transparency has only been performed in a few taxa such as lepidopterans (Gomez et al., 2021) and ascidians (Shito et al., 2020), but the results show that animal transparency is polyphyletic. In lepidopterans, transparent or translucent wings were found across 31 families suggesting that transparency evolved multiple times independently and is due to a massive diversity of structural strategies (Gomez et al., 2021).
Variability in the transparency of ascidian eggs ranges from 10% to 90% across different species (Figure 1E). Interestingly, species within the Ascidiidae family have highly transparent eggs. One hypothesis is that they evolved to enhance their transparency from an opaque ancestor 270 mya (Figure 1F). This suggests the presence of shared genetic changes within this family as shared derived traits (SDTs). Focusing on such animal groups with transparency as a SDT—rather than solely as individual convergent evolution cases—could be an important taxa for elucidating the genetic mechanism of acquiring transparency. Comparative genomics of the Ascidiidae family and other relatives might elucidate the shared genetic mutation among Ascidiidae for organismal transparency that might identify the natural OCAs in transparent tissues of ascidians.
2.2 Developmental biology
The absence of pigments is a prerequisite for organismal transparency as described in 1.1. There is currently no research on how transparency is acquired during the developmental process. We measured bio-transparency (Shito et al., 2020) of developing oocytes according to the oogenesis period of Ciona robusta (also called Ciona intestinalis type A) (Sakai et al., 2023). The results showed that Ascidiella aspersa maintains transparency throughout oogenesis stages I to III, while oocytes of Ciona robusta are initially transparent but rapidly become opaque during stage II (Figure 2A) (Satake et al., 2024). These results indicate that oocytes in ascidians are originally transparent, and A. aspersa maintains its transparency throughout oogenesis. Additionally, the transmittance spectrum of A. aspersa oocyte is maintained during subsequent embryonic development (Figure 2B). Therefore, oocyte transparency has a significant impact on the transparency acquisition of embryos as seen by the consistent transparency from the oogenesis stages (Figure 2A).
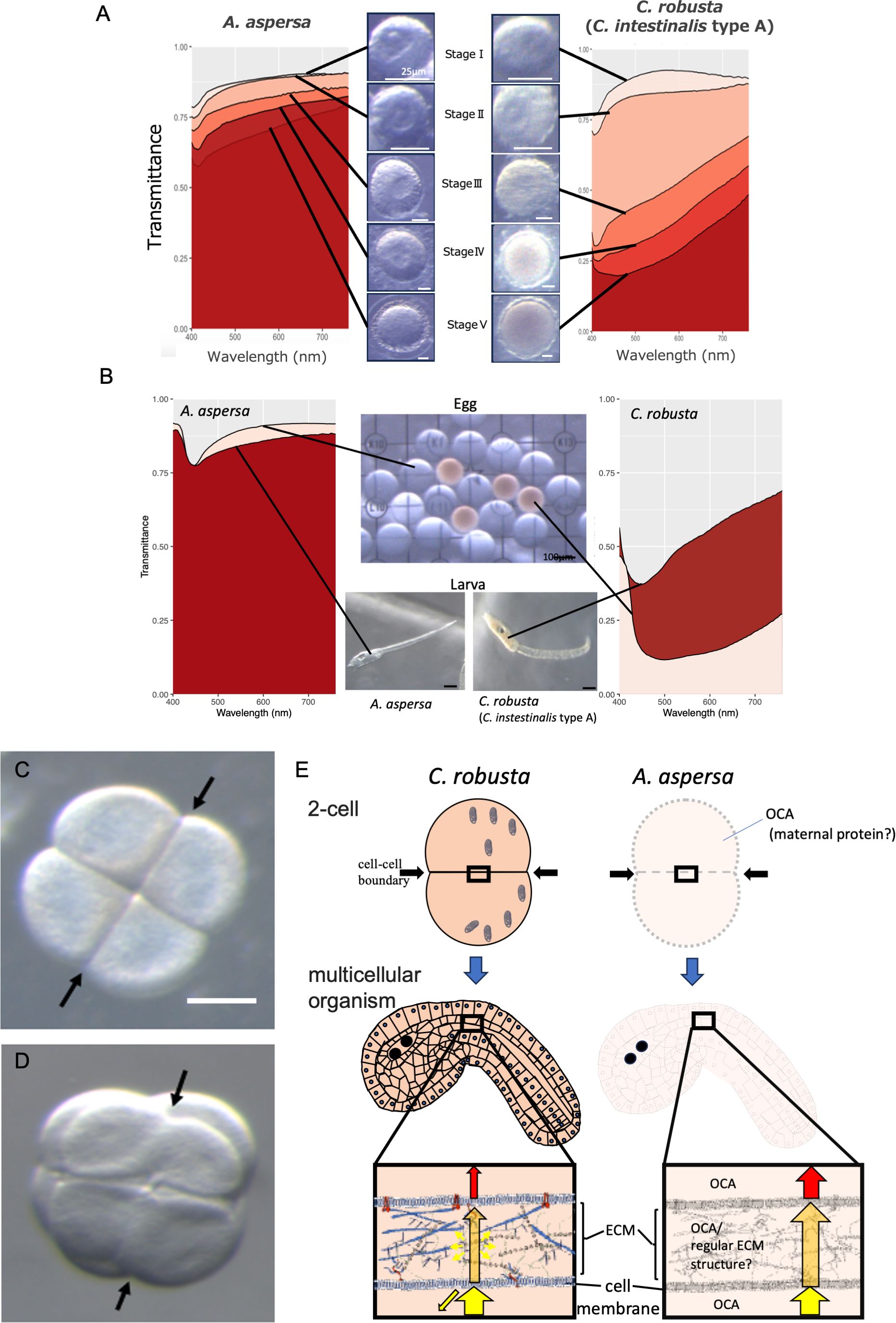
Figure 2. Comparison of egg transparency between A. aspersa from the Ascidiidae family and opaque species of ascidians, C. robusta. (A) Quantitative comparison of organismal transparency spectra from Stage I to Stage V during the oocyte maturation process in different ascidian species: A. aspersa and C. robusta. Spectre data were obtained via a hyperspectral camera (custom model NH-KO, EBA JAPAN, Tokyo, Japan). (B) Quantitative comparison of organismal transparency spectra between eggs and larvae in different ascidian species: A. aspersa and C. robusta. (C) Microscopic images of four-cell stage embryos after compaction in C. robusta embryo. (D) Microscopic images of four-cell stage embryos after compaction in A. aspersa embryo. Distinct boundaries are discernible in C. robusta at the newly formed cleavage planes in the 4-cell stage, but not in A. aspersa embryo (arrows). (E) Schematic representation of opaque C. robusta and transparent A. aspersa embryos. In the cleavage period (two-cell stage), there is no pigment accumulation in the cytoplasm after oogenesis. This suggests the possibility of the presence of some OCAs preventing the internal scattering of transmitted light in A. aspersa. A. aspersa minimizes light scattering despite the presence of diverse ECM at cell-cell boundary regions even in the multicellular stage. A. aspersa likely possesses special factors, e.g., regular ECM structures as well as the presence of OCA with both hydrophilic and hydrophobic properties in the ECM.
Hence, we propose that exploring the mechanism of maintaining oocyte transparency to later developmental stages can help answer more general questions about why the organism body is transparent. This phenomenon may result from the absence or suppression of specific genes involved in pigment synthesis within yolk granules as well as the presence of OCAs that maintain the properties of cellular components responsible for low light scattering. These findings suggest the need to understand the temporal expression of such components via a developmental biology approach.
We also found interesting phenomena about the transparency of A. aspersa during the cleavage period. There are changes in the transparency of the cell-cell boundary region. Transparency requires a constant refractive index at the internal and external boundaries. Immediately after cell division, the cell boundaries are observable in both A. aspersa and C. robusta. However, only the cell-cell boundary in A. aspersa becomes transparent after subsequent compaction (Supplementary Video 1; Figures 2C, D). The exact mechanism responsible for this optical property is not known, but the finding suggests that the region of cell-cell boundary (the cell membrane or ECM) in A. aspersa uses different properties with that of C. robusta. The ECM is generally hydrophilic, and a difference in the refractive index may occur at the boundary with lipophilic cell membranes. Therefore, OCA with both hydrophilic and lipophilic properties might exist in the transparent ECM of A. aspersa. Another possibility is that the structural proteins present in the ECM, such as collagen and keratin, are often transparent if these proteins have a regular structure (Poole and Mostaço-Guidolin, 2021), which results in less light scattering. Studies into whether the ultrastructure of ECM would be regular or not is one of the key factors in the organismal transparency of A. aspersa (Figure 2E).
Transparency is physiologically affected (Table 1). We found that actinomycin D transcription inhibitor did not change transparency, but cycloheximide translation inhibitor significantly decreased egg transparency (Shito et al., 2023). Considering the differences between the two inhibitors, we propose that the transparency does not require the expression of new genes but rather relies on the function, metabolism, or enzymatic reactions of already transcribed proteins (maternal proteins). This is consistent with the importance of lipid metabolism in maintaining the optical transparency of the vertebrate lens (Borchman and Yappert, 2010).
2.3 Quantification and evaluation methods of organismal transparency
Advancements in measurement systems are crucial to elucidating the mechanisms of organismal transparency. Recently, Taboada et al. discovered that glass frogs enhance transparency by redistributing 89% of their red blood cells to the liver during sleep as tracked through photoacoustic imaging of glass frog erythrocyte dynamics (Taboada et al., 2022). Concurrently, the reflective indices of a salp were measured by an Abbe refractometer, and the values were slightly greater than seawater (Kakiuchida et al., 2017).
Several other methods have been used to quantify the organismal transparency. A spectrometer can calculate light transmission in one or several spectra (Johnsen and Widder, 1999). Hyperspectral imaging can measure transmittance at various wavelengths from the ultraviolet to the infrared with high resolution (Velasco-Hogan et al., 2019; Shito et al., 2020, 2023). The transmittance differs depending on each spectrum, and different factors such as environmental changes like pH, temperature and salinity, or bioactive substances should influence each spectrum. For instance, higher acidity (lower pH) and temperature variations can alter the protein structures within the organism potentially leading to changes in light scattering and transparency (Surewicz and Olesen, 1995).
There is a need for an evaluation system that can understand the correspondence between these different factors and transmittance for each spectrum. Combining a hyperspectral camera with analytical methods like principal component analysis (PCA) can evaluate the organismal transparency in a multi-dimensional way (Shito et al., 2023). By compressing multidimensional spectral data, information on organismal transparency can be mapped onto two- or three-dimensional space, thus enabling evaluation with higher resolution than before. With this evaluation system, one can clearly see that there are multiple states based on the differences in spectra even though the transparency has the same value.
Raman microscopy is also a powerful tool that potentially can link intrinsic molecular states and transparency over the entire wavelength range (Nakamura et al., 2013). Raman microscopy uses Raman scattering to analyze the molecular vibrations and rotations of materials within a sample. The spectral features of corneal collagen change due to heating and aging are detected using Raman microscopy (Goheen et al., 1978).
Analysis based on these spectral data allows for precise and detailed analysis of transparency by wavelength. For example, by detecting specific wavelengths that are affected by various environmental factors or inhibitors on transparency, we can further understand the mechanism of organismal transparency. Furthermore, such analysis may enable us to identify OCAs present in transparent organisms.
2.4 Implications for biological and medical studies
The fields of biology and medical research have shown that transparency techniques are critical for high-resolution optical imaging of tissues. Deep imaging is hindered by the opacity of tissues, and thus techniques are being developed to make tissue specimens almost transparent by matching the refractive indices of fixed specimens and media to the refractive index of lipid layers or by removing lipids (Hama et al., 2015; Zheng and Rinaman, 2016; Murakami et al., 2018; Umezawa et al., 2019; Tian et al., 2021). This allows for applications such as constructing a 3D atlas of the brain by making tissues and organs transparent (Murakami et al., 2018) or detecting micrometastases of cancer cells (Kingston et al., 2019). However, these specimens are derived from deceased and chemically fixed tissues. Alternatively, we can learn from transparent organisms how they make in vivo tissues transparent. Research that learns the molecular mechanisms of making or maintaining transparency from living transparent organisms, rather than focusing on fixed specimens, is a completely different approach. Therefore, the study of organismal transparency can lead to the development of new technologies for in vivo imaging of living tissues.
3 Ideal model for unravelling the mechanism of organismal transparency
Tunicates are marine invertebrate chordates and are most closely related to vertebrates. The salps, larvaceans, and some ascidian species are known to be highly transparent. They are classified into three classes: Ascidiacea, Thaliacea and Appendicularia. Some species of Ascidiacea are used as model organisms for the study of developmental biology. They have the following advantages as a model for unravelling the mechanism of organismal transparency.
3.1 Ease of comparative analysis of transparency
As mentioned earlier, the transparency of the egg is a significant factor that determines the subsequent transparency of the embryo. Therefore, ascidians, which facilitate the measurement and comparison of various egg transparencies (Figure 1F), provide an unparalleled simplest experimental system for organismal transparency.
Compared to tissues, organs, or individuals that have different and complex shapes across species, ascidian eggs are simply spherical thus normalizing the effects of differences in thickness and surface structure on transparency (Shito et al., 2020). This in turn facilitates comparative analysis of organism transparency between different species.
Furthermore, both eggs and ascidian cleavage patterns are conserved across species (Dumollard et al., 2017), and thus differences in transparency between internal scattering in the cytoplasm and external scattering at cell boundaries can be easily distinguished at homologous locations at the cellular level for comparative analysis between species (Figure 2E).
3.2 The Ascidiidae family is an ideal experimental resource
The Ascidiidae family showed high eggs transparency (Ascidiella aspersa: 88.7% and Ascidia zara: 50.7% (Shito et al., 2020)) including Phallusia mammillata, Phallusia nigra, and Ascidia ahodori (Sardet et al., 2011; Yasuo and McDougall, 2018). The Ascidiidae family is thought to have branched off from ascidians, which have a wide variety of transparency (Figure 1F; ranging from 10% to 90%) (Shito et al., 2020). The Ascidiidae family provides an ideal resource for exploring common genetic alterations within the family during the evolution of transparency (e.g., genetic mutations that suppress pigmentation, the mutations in structural proteins such as α-crystallin and water-rich proteoglycans, and mutations in genes involved in the synthesis of ECM at cell-cell boundaries that may suppress light scattering). Thus, a cohort of the Ascidiidae family is an ideal experimental resource to identify the genetic factors underlying the previously enigmatic evolution of transparency in aquatic organisms.
3.3 Convenience of molecular verification experiments
Family Ascidiidae has five genera (Ascidia, Ascidiella, Fimbrora, Phallusia, Psammascidia) and is distributed worldwide in seas with various temperatures. The ANISEED database has genome and gene annotations of 11 ascidians containing two Ascidiid species (Phallusia mammillata and Phallusia fumigata) (Dardaillon et al., 2020). Phallusia mammillata is known for its transparent eggs and is used in live imaging studies (Yasuo and McDougall, 2018). Genome sequence of Ascidia mentula was recently published (Bishop et al., 2023). Ascidiella aspersa is distributed worldwide and has well-defined developmental stages that facilitates gene transfer experiments (Funakoshi et al., 2021). Genome sequencing of A. apsersa from different habitats including in Japan and Europe are underway. Ciona species are already used as model organisms for developmental biology. The abundance of genome data including those from Ascidiidae as well as opaque species (De Thier et al., 2024) allow for genome-wide comparative analysis of gene models and further validation experiments through functional analysis of candidate genes. In this way, ascidians provide a useful environment for elucidating the molecular mechanisms of organismal transparency.
4 Conclusion
Organismal transparency needs to be analyzed by at least separating internal and external scattering. Furthermore, by incorporating the perspectives of development and evolution, we have derived the view that maternal proteins during oogenesis in internal scattering. There is also an unknown mechanism that makes cell boundaries transparent during cleavage in external scattering. Both are important for the organismal transparency of early Ascidiidae embryos.
Future work should study maternally conserved proteins through proteomics and should study the ultrastructure of cell boundaries by electron microscopy or Raman microscopy. This in turn will lead to the discovery of new OCAs that are evolutionarily conserved among Ascidiidae.
Research into the mechanism of organismal transparency is becoming increasingly important and gives rise to the interdisciplinary field of “organismal transparency biology.” This is a challenge, and the scientific community should tackle this challenge together.
Data availability statement
The original contributions presented in the study are included in the article/Supplementary Material. Further inquiries can be directed to the corresponding author.
Ethics statement
The manuscript presents research on animals that do not require ethical approval for their study.
Author contributions
KH: Conceptualization, Funding acquisition, Methodology, Project administration, Resources, Supervision, Visualization, Writing – original draft, Writing – review & editing. SM: Data curation, Formal analysis, Investigation, Visualization, Writing – review & editing. KO: Supervision, Writing – review & editing. TS: Data curation, Formal analysis, Funding acquisition, Investigation, Methodology, Resources, Software, Validation, Visualization, Writing – review & editing.
Funding
The author(s) declare financial support was received for the research, authorship, and/or publication of this article. This study was supported in part by JSPS KAKENHI (21H00440, 23H04717, 24K02038), Keio University Research and Education Centre for Natural Sciences Budget, and KLL Keio Leading Program to KH. The Research Institute of Marine Invertebrates (IKU2021-02) supported TS. The Keio University Doctorate Student Grant-in-Aid Program from Ushioda Memorial Fund supported TS. JSPS KAKENHI Grant Number JP 22J22628 supported TS.
Acknowledgments
Dr. Minoru Ikeda and Captain Toyokazu Hiratsuka (Onagawa Field Center of Tohoku University), Dr. Makoto Kanamori, Dr. Masafumi Natsuike, and Takuya Mizukami (Hokkaido Research Organization, Hakodate Fisheries Research Institute), Dr. Gaku Kumano (Graduate School of Life Sciences, Tohoku University), Mr. Akio Takiya, Dr. Tatsunari Mori, Dr. Takaaki Kayaba, and Dr. Motohito Yamaguchi (Hokkaido Research Organization, Central Fisheries Research Institute) for their help in collecting the samples. We thank Yuki S. Kogure for giving us critical comments for preparing this manuscript. We thank Haruka Miyama Funakoshi and Shimon Kawai for handling A. aspersa. EBA JAPAN (Tokyo, Japan) provided a custom model hyperspectral camera (NH-KO) for our study. The Ciona individuals were provided by Dr. Yutaka Satou (Kyoto University), Dr. Manabu Yoshida (The University of Tokyo) and Dr. Yasunori Sasakura (University of Tsukuba) with support from the NBRP of AMED, Japan.
Conflict of interest
The authors declare that the research was conducted in the absence of any commercial or financial relationships that could be construed as a potential conflict of interest.
The author(s) declared that they were an editorial board member of Frontiers, at the time of submission. This had no impact on the peer review process and the final decision.
Publisher’s note
All claims expressed in this article are solely those of the authors and do not necessarily represent those of their affiliated organizations, or those of the publisher, the editors and the reviewers. Any product that may be evaluated in this article, or claim that may be made by its manufacturer, is not guaranteed or endorsed by the publisher.
Supplementary material
The Supplementary Material for this article can be found online at: https://www.frontiersin.org/articles/10.3389/fevo.2024.1428976/full#supplementary-material
Supplementary Video 1 | Timelapse movie of the early cleavage of A. aspersa from two-cell stage to 32-cell stage. The visibility of cell boundaries decreases at compaction after each cleavage event.
References
Bagge L. E. (2019). Not as clear as it may appear: challenges associated with transparent camouflage in the ocean. Integr. Comp. Biol. 59, 1653–1663. doi: 10.1093/icb/icz066
Bagge L. E., Kinsey S. T., Gladman J., Johnsen S. (2017). Transparent anemone shrimp (Ancylomenes pedersoni) become opaque after exercise and physiological stress in correlation with increased hemolymph perfusion. J. Exp. Biol. 220, 4225–4233. doi: 10.1242/jeb.162362
Bagge L. E., Osborn K. J., Johnsen S. (2016). Nanostructures and monolayers of spheres reduce surface reflections in Hyperiid amphipods. Curr. Biol. 26, 3071–3076. doi: 10.1016/j.cub.2016.09.033
Bhandiwad A., Johnsen S. (2011). The effects of salinity and temperature on the transparency of the grass shrimp Palaemonetes pugio. J. Exp. Biol. 214, 709–716. doi: 10.1242/jeb.049296
Bishop J., Wood C., Marine Biological Association Genome Acquisition Lab, Darwin Tree of Life Barcoding collective, Wellcome Sanger Institute Tree of Life Management, Samples and Laboratory team, Wellcome Sanger Institute Scientific Operations: Sequencing Operations, et al. (2023). The genome sequence of a solitary sea squirt, Ascidia mentula (Müller 1776). Wellcome Open Res. 8, 583. doi: 10.12688/wellcomeopenres
Bishop R. E., Torres J. J., Crabtree R. E. (2000). Chemical composition and growth indices in leptocephalus larvae. Mar. Biol. 137, 205–214. doi: 10.1007/s002270000362
Borchman D., Yappert M. C. (2010). Lipids and the ocular lens. J. Lipid Res. 51, 2473–2488. doi: 10.1194/jlr.R004119
Brennan L., Costello M. J., Hejtmancik J. F., Menko A. S., Riazuddin S. A., Shiels A., et al. (2023). Autophagy requirements for eye lens differentiation and transparency. Cells 12. doi: 10.3390/cells12030475
Dardaillon J., Dauga D., Simion P., Faure E., Onuma T. A., DeBiasse M. B., et al. (2020). ANISEED 2019: 4D exploration of genetic data for an extended range of tunicates. Nucleic Acids Res. 48, D668–D675. doi: 10.1093/nar/gkz955
De Thier O., Tawfeeq M. M., Faure R., Lebel M., Dru P., Blanchoud S., et al. (2024). First chromosome-level genome assembly of the colonial tunicate Botryllus schlosseri. bioRxiv 2024, 5.29.594498. doi: 10.1101/2024.05.29.594498
Dumollard R., Minc N., Salez G., Aicha S. B., Bekkouche F., Hebras C., et al. (2017). The invariant cleavage pattern displayed by ascidian embryos depends on spindle positioning along the cell’s longest axis in the apical plane and relies on asynchronous cell divisions. Elife 6. doi: 10.7554/elife.19290
Funakoshi H. M., Shito T. T., Oka K., Hotta K. (2021). Developmental table and three-dimensional embryological image resource of the ascidian Ascidiella aspersa. Front. Cell Dev. Biol. 9. doi: 10.3389/fcell.2021.789046
Goheen S. C., Lis L. J., Kauffman J. W. (1978). Raman spectroscopy of intact feline corneal collagen. Biochim. Biophys. Acta 536, 197–204. doi: 10.1016/0005-2795(78)90065-X
Gomez D., Pinna C., Pairraire J., Arias M., Barbut J., Pomerantz A., et al. (2021). Wing transparency in butterflies and moths: structural diversity, optical properties, and ecological relevance. Ecol. Monogr. 91. doi: 10.1002/ecm.1475
Hama H., Hioki H., Namiki K., Hoshida T., Kurokawa H., Ishidate F., et al. (2015). ScaleS: an optical clearing palette for biological imaging. Nat. Neurosci. 18, 1518–1529. doi: 10.1038/nn.4107
Hansson L. A. (2000). Induced pigmentation in zooplankton: a trade-off between threats from predation and ultraviolet radiation. Proc. Biol. Sci. 267, 2327–2331. doi: 10.1098/rspb.2000.1287
Horwitz J. (1992). Alpha-crystallin can function as a molecular chaperone. Proc. Natl. Acad. Sci. U. S. A. 89, 10449–10453. doi: 10.1073/pnas.89.21.10449
Inyushin M., Meshalkina D., Zueva L., Zayas-Santiago A. (2019). Tissue transparency in vivo. Molecules 24. doi: 10.3390/molecules24132388
Johnsen S. (2001). Hidden in plain sight: the ecology and physiology of organismal transparency. Biol. Bull. 201, 301–318. doi: 10.2307/1543609
Johnsen S. (2003). Lifting the cloak of invisibility: the effects of changing optical conditions on pelagic crypsis1. Integr. Comp. Biol. 43, 580–590. doi: 10.1093/icb/43.4.580
Johnsen S. (2014). Hide and seek in the open sea: pelagic camouflage and visual countermeasures. Ann. Rev. Mar. Sci. 6, 369–392. doi: 10.1146/annurev-marine-010213-135018
Johnsen S., Widder E. A. (1999). The physical basis of transparency in biological tissue: ultrastructure and the minimization of light scattering. J. Theor. Biol. 199, 181–198. doi: 10.1006/jtbi.1999.0948
Kakiuchida H., Sakai D., Nishikawa J., Hirose E. (2017). Measurement of refractive indices of tunicates’ tunics: light reflection of the transparent integuments in an ascidian Rhopalaea sp. and a salp Thetys vagina. Zoological Lett. 3, 7. doi: 10.1186/s40851-017-0067-6
Kerker M. (1969). The scattering of light and other electromagnetic radiation (Academic press). Available online at: https://www.abebooks.com/scattering-light-electromagnetic-radiation-Kerker-Milton/31404218033/bd (Accessed March 21, 2024).
Kingston B. R., Syed A. M., Ngai J., Sindhwani S., Chan W. C. W. (2019). Assessing micrometastases as a target for nanoparticles using 3D microscopy and machine learning. Proc. Natl. Acad. Sci. U. S. A. 116, 14937–14946.
Marina O. C., Sanders C. K., Mourant J. R. (2012). Correlating light scattering with internal cellular structures. Biomed. Opt. Express 3, 296–312. doi: 10.1364/BOE.3.000296
McFall-Ngai M. J. (1990). Crypsis in the pelagic environment. Am. Zool. 30, 175–188. doi: 10.1093/icb/30.1.175
Miller W. H., Autrum H. (1979). Handbook of sensory physiology, Vol. VII A 6. 69–143.New York: Springer.
Morishita H., Eguchi T., Tsukamoto S., Sakamaki Y., Takahashi S., Saito C., et al. (2021). Organelle degradation in the lens by PLAAT phospholipases. Nature 592, 634–638. doi: 10.1038/s41586-021-03439-w
Murakami T. C., Mano T., Saikawa S., Horiguchi S. A., Shigeta D., Baba K., et al. (2018). A three-dimensional single-cell-resolution whole-brain atlas using CUBIC-X expansion microscopy and tissue clearing. Nat. Neurosci. 21, 625–637. doi: 10.1038/s41593-018-0109-1
Nakamura M. J., Hotta K., Oka K. (2013). Raman spectroscopic imaging of the whole Ciona intestinalis embryo during development. PloS One 8, e71739. doi: 10.1371/journal.pone.0071739
Pettersen R., Johnsen G., Bruheim P., Andreassen T. (2014). Development of hyperspectral imaging as a bio-optical taxonomic tool for pigmented marine organisms. Org. Divers. Evol. 14, 237–246. doi: 10.1007/s13127-013-0163-1
Pfeiler E. (1984). Glycosaminoglycan breakdown during metamorphosis of larval bonefish albula. Mar. Biol. Lett. 5, 241–250.
Pfeiler E., Govoni J. J. (1993). Metabolic rates in early life history stages of elopomorph fishes. Biol. Bull. 185, 277–283. doi: 10.2307/1542007
Pfeiler E., Toyoda H., Williams M. D., Nieman R. A. (2002). Identification, structural analysis and function of hyaluronan in developing fish larvae (leptocephali). Comp. Biochem. Physiol. B Biochem. Mol. Biol. 132, 443–451. doi: 10.1016/S1096-4959(02)00060-X
Poole J. J. A., Mostaço-Guidolin L. B. (2021). Optical microscopy and the extracellular matrix structure: A review. Cells 10. doi: 10.3390/cells10071760
Rao P. V., Huang Q. L., Horwitz J., Zigler J. S. Jr (1995). Evidence that alpha-crystallin prevents non-specific protein aggregation in the intact eye lens. Biochim. Biophys. Acta 1245, 439–447. doi: 10.1016/0304-4165(95)00125-5
Ruud J. T. (1954). Vertebrates without erythrocytes and blood pigment. Nature 173, 848–850. doi: 10.1038/173848a0
Sakai T., Yamamoto T., Watanabe T., Hozumi A., Shiraishi A., Osugi T., et al. (2023). Characterization of a novel species-specific 51-amino acid peptide, PEP51, as a caspase-3/7 activator in ovarian follicles of the ascidian, Ciona intestinalis Type A. Front. Endocrinol. 14, 1260600. doi: 10.3389/fendo.2023.1260600
Sardet C., McDougall A., Yasuo H., Chenevert J., Pruliere G., Dumollard R., et al. (2011). Embryological methods in ascidians: the Villefranche-sur-Mer protocols. Methods Mol. Biol. 770, 365–400. doi: 10.1007/978-1-61779-210-6_14
Satake H., Kawada T., Osugi T., Sakai T., Shiraishi A., Yamamoto T., et al. (2024). Ovarian follicle development in ascidians. Zoolog. Sci. 41, 60–67. doi: 10.2108/zs230054
Shito T. T., Hasegawa N., Oka K., Hotta K. (2020). Phylogenetic comparison of egg transparency in ascidians by hyperspectral imaging. Sci. Rep. 10, 20829. doi: 10.1038/s41598-020-77585-y
Shito T. T., Oka K., Hotta K. (2023). Multimodal factor evaluation system for organismal transparency by hyperspectral imaging. PloS One 18, e0292524. doi: 10.1371/journal.pone.0292524
Surewicz W. K., Olesen P. R. (1995). On the thermal stability of alpha-crystallin: a new insight from infrared spectroscopy. Biochemistry 34, 9655–9660. doi: 10.1021/bi00030a001
Taboada C., Delia J., Chen M., Ma C., Peng X., Zhu X., et al. (2022). Glassfrogs conceal blood in their liver to maintain transparency. Science 378, 1315–1320. doi: 10.1126/science.abl6620
Tarique I., Lu T., Tariq M. (2023). Cellular activity of autophagy and multivesicular bodies in lens fiber cells during early lens development in rbm24a mutant of zebrafish: Ultrastructure analysis. Micron 169, 103446. doi: 10.1016/j.micron.2023.103446
Tian T., Yang Z., Li X. (2021). Tissue clearing technique: Recent progress and biomedical applications. J. Anat. 238, 489–507. doi: 10.1111/joa.v238.2
Umezawa M., Haruguchi S., Fukushima R., Sekiyama S., Kamimura M., Soga K. (2019). Rapid increase in transparency of biological organs by matching refractive index of medium to cell membrane using phosphoric acid. RSC Adv. 9, 15269–15276. doi: 10.1039/C9RA01445D
Velasco-Hogan A., Deheyn D. D., Koch M., Nothdurft B., Arzt E., Meyers M. A. (2019). On the nature of the transparent teeth of the deep-sea dragonfish, Aristostomias scintillans. Matter 1, 235–249. doi: 10.1016/j.matt.2019.05.010
Wakamatsu Y., Pristyazhnyuk S., Kinoshita M., Tanaka M., Ozato K. (2001). The see-through medaka: a fish model that is transparent throughout life. Proc. Natl. Acad. Sci. U. S. A. 98, 10046–10050. doi: 10.1073/pnas.181204298
Warrant E. J., Locket N. A. (2004). Vision in the deep sea. Biol. Rev. Camb. Philos. Soc 79, 671–712. doi: 10.1017/S1464793103006420
Wilson S. J., Hutley M. C. (1982). The optical properties of “Moth eye” Antireflection surfaces. Optica Acta: Int. J. Optics 29, 993–1009. doi: 10.1080/713820946
Yasuo H., McDougall A. (2018). Practical guide for ascidian microinjection: Phallusia mammillata. Adv. Exp. Med. Biol. 1029, 15–24. doi: 10.1007/978-981-10-7545-2_3
Keywords: bio-transparency, eco-evo-devo, hyperspectral imaging, tunicate, Ascidiidae, Ascidiella aspersa
Citation: Hotta K, Miyasaka SO, Oka K and Shito TT (2024) Staring into a crystal ball: understanding evolution and development of in vivo aquatic organismal transparency. Front. Ecol. Evol. 12:1428976. doi: 10.3389/fevo.2024.1428976
Received: 07 May 2024; Accepted: 22 October 2024;
Published: 15 November 2024.
Edited by:
Maria Ina Arnone, Stazione Zoologica Anton Dohrn, ItalyReviewed by:
Filomena Ristoratore, Zoological Station Anton Dohrn, ItalyYiyang Yuan, Shandong Academy of Agricultural Sciences, China
Copyright © 2024 Hotta, Miyasaka, Oka and Shito. This is an open-access article distributed under the terms of the Creative Commons Attribution License (CC BY). The use, distribution or reproduction in other forums is permitted, provided the original author(s) and the copyright owner(s) are credited and that the original publication in this journal is cited, in accordance with accepted academic practice. No use, distribution or reproduction is permitted which does not comply with these terms.
*Correspondence: Kohji Hotta, a2hvdHRhQGtlaW8uanA=