- 1Department of Biology, University of New Brunswick, Fredericton, NB, Canada
- 2Atlantic Forestry Centre, Corner Brook Office – Canadian Forest Service, Natural Resources Canada, Corner Brook, NL, Canada
Fire is the largest natural disturbance factor in the boreal forest and plays a critical role in the composition, structure, and succession of stands and landscapes. The island of Newfoundland, located in eastern Canada, is subjected to a greater maritime influence, which may result in longer fire return intervals. The limited data on the fire regime does not account for interactions between fire, vegetation, and climate throughout the Holocene. We used sediment cores from Arnold’s Pond, Terra Nova National Park, which covered the last ~11,800 cal. yr BP, to investigate these interactions. We recognize 4 pollen zones and macroscopic charcoal analysis detected 45 local fire events. The 250-year mean fire return interval associated with the current vegetation is longer than a previous estimate for the park, but significantly shorter than other estimates for the island. Our mean fire return interval is within the range of fire estimates from Québec with similar vegetation. Our results suggest that the fire regime was primarily influenced by vegetation and climate. The transition to an open forest from a shrub tundra resulted in increased fire activity and fire frequency, which were likely driven by additional fuel on the landscape, but could have also been influenced by unknown climatic factors. We identified several examples of changes in the fire frequencies and/or charcoal accumulation that coincided with regional climate shifts, but we also identified a non-synchronous change. The non-synchronous shift to drier conditions resulted in a ~500-year time lag between peak Pinus strobus abundance and maximum fire frequency. Synchronous shifts in fire activity and/or fire frequency coincided with the 8200 event, Medieval Climate Anomaly and Little Ice Age. We also noted a decrease in fire frequency between 2600–1500 cal. yr BP that coincided with similar changes in the fire frequency from ~3000–1000 cal. yr BP in Québec. Our study highlights the complex interactions influencing the fire regime in our study area during the Holocene.
Introduction
The circumpolar boreal zone covers vast areas of North America and Eurasia (Brandt, 2009). The forests of this zone play an important role in both carbon sequestration and the release of carbon through combustion, which has implications for greenhouse gas production (Amiro et al., 2009). Within the boreal forest, natural disturbance factors, such as fire, insects and pathogens, affect the composition, structure, and succession of stands and landscapes (Sirois and Payette, 1991; Johnstone and Chapin, 2006; Kautz et al., 2017; Hart et al., 2019; Foster et al., 2022). Depending on the intensity and frequency of fire events, there can be drastic impacts on the heterogeneity of a stand, cycling of nutrients in the soil and on the aerial or soil seed banks of fire-adapted species (Greene et al., 1999; Arseneault, 2001; Bergeron and Fenton, 2012; Hart et al., 2019). Frequent and severe fire events can shift the landscape to favor shade-intolerant broadleaf species and create conditions that are no longer competitive for fire-facilitated taxa, such as Pinus spp. and Picea mariana (Mill.) Britton, Sterns & Poggenb (Greene et al., 1999; Arseneault, 2001; Johnstone and Chapin, 2006; Fourrier et al., 2013; Remy et al., 2017b; Whitman et al., 2019; Augustin et al., 2022).
Paleoecological approaches are often used to reconstruct vegetation dynamics, disturbance events and climate change during the Holocene (MacPherson, 1995; Ali et al., 2009; Finkenbinder et al., 2022). In the boreal forest of eastern Canada, the time between fires is typically longer than in western and central Canada (Erni et al., 2020; Arsenault, 2015; Zhang and Chen, 2007). Depending on latitude, climate, vegetation, anthropogenic influences and ignition factors, the time between fire events can vary greatly in eastern Canada. In northern Québec near James Bay, the northern Forest-Tundra fire rotation period is estimated to be 1460 yr, but in central Québec the fire cycle is 273 yr (Payette et al., 1989; Bergeron et al., 2001). In the Côte-Nord region of eastern Québec, the mean fire return interval (mFRI) is 181 to >500 yr depending on the timeframe and techniques used to calculate the values (Bouchard et al., 2008; Cyr et al., 2012; Couillard et al., 2021; Tcheumeleu et al., 2023). mFRI (mean number of years between successive fire events) was calculated in the above studies using forest inventory plots, fire maps, fire scars, tree rings and soil charcoal. In southern Labrador the estimated fire rotation period is >500 yr (Foster, 1983).
Holocene fire histories from Québec are a good point of comparison as regions of Newfoundland have similar vegetation and climate. Studies from the black spruce-moss and black spruce-lichen subdomains of Québec indicate that the current range of regional mFRIs is 180–410 yr. (Carcaillet et al., 2010; Hély et al., 2010; Ali et al., 2012; Oris et al., 2014b; El-Guellab et al., 2015; Remy et al., 2017b; Hennebelle et al., 2018; Couillard et al., 2021; Tcheumeleu et al., 2023) Studies in this paragraph calculated mFRI using charcoal from lake sediment with the exception of Couillard et al., 2021, which used soil charcoal.
Fire return intervals for Newfoundland have not been extensively studied. One estimate suggests the fire cycle for the island is ~770 yr, based on contemporary data that do not account for how the fire frequency varies over space and time (Arsenault, 2015; Arsenault et al., 2016). A mean stand age estimate for Terra Nova National Park of ~100 yr implied a fire return interval of that same duration (Power, 1996). With a greater maritime influence on the island of Newfoundland, a longer fire return interval may be expected on the island than on the mainland of eastern boreal Canada.
With limited data on how the fire regime has varied over time in the eastern-most boreal forest of Newfoundland, we identified three objectives: (1) Is the fire frequency in Terra Nova National Park significantly longer than other parts of the Boreal Forest in eastern Canada? (2) To what extent has vegetation change driven changes in the fire regime? (3) To what extent has climate driven changes in the fire regime? Results from this study will not only provide a high-resolution, long-term record of local fire events, but also provide data that can be used to aid park ecosystem managers in developing a more detailed fire management plan that better coincides with the natural fire regime. If we have a better understanding of the fire intervals, park ecosystem mangers in Terra Nova National Park can devise a fire regime in the study area that uses prescribed burns combined with letting natural/accidentally set fires burn. This data will aid them in fulfilling their mandate to maintain the park in a “natural” state, which includes an appropriate fire regime [Canada National Parks Act (S.C. 2000, c. 32)] (Parks Canada Agency, 2000).
Site description
Arnold’s Pond (Figure 1) is a small headwater pond (N48.63063, W−53.97486, 74 m a.s.l., 4 ha, max water depth 5 m) on the northern tip of Terra Nova National Park, Newfoundland and Labrador and is in the Central Newfoundland, Forest ecoregion (Damman, 1983; Meades and Moore, 1994). The bedrock surrounding the pond is Neoproterozoic volcanic with minor siliciclastic sedimentary rocks (Sparks and Dunning, 2014). The soils are orthic humo-ferric podzols formed on medium-textured glacial till (Heringa and Woodrow, 1991). Vegetation around the pond is primarily Picea mariana-moss forest with a crown density greater than 75% and open Kalmia-Picea mariana with crown density between 25–75% (Simpson, 2007). To a lesser extent, patches of Kalmia angustifolia L. barrens and open scrub Picea are present around the site (Simpson, 2007). Picea mariana is the dominant tree with other tree species such as Abies balsamea L. Mill., Betula papyrifera Marshall, Populus tremuloides Michx., Larix laricina (Du Roi.) K. Koch, Pinus strobus L., and Acer rubrum L., also present in the park (Power, 1996; Simpson, 2007; Parks Canada, 2019). Kalmia angustifolia can be found in most cover types and is the dominant ericaceous shrub (Power, 1996).
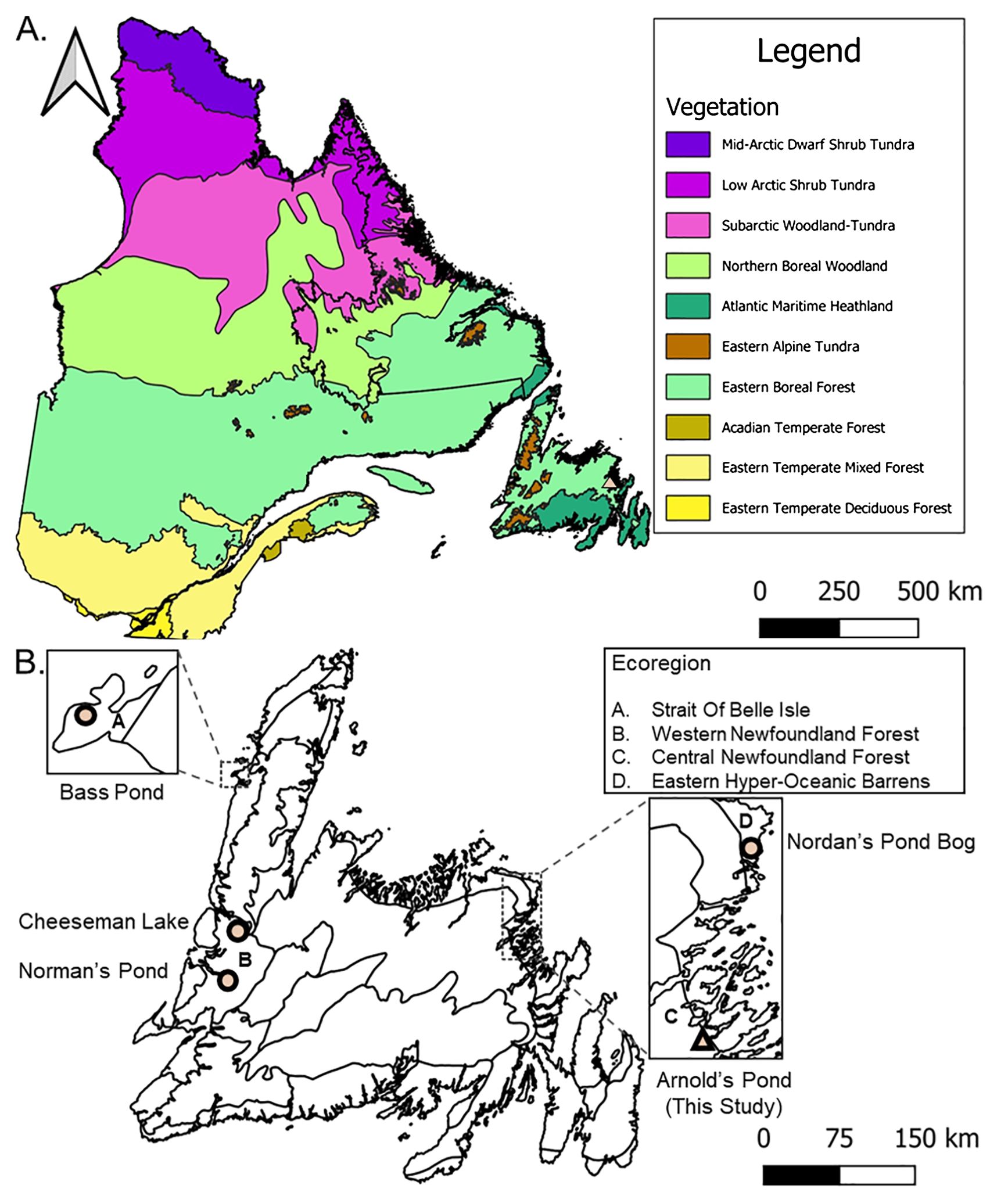
Figure 1. (A) Québec-Newfoundland and Labrador regional context with level two vegetation types (Baldwin et al., 2019). The colored triangle indicates our study area of Arnold’s Pond in Terra Nova National Park, Newfoundland and Labrador (B) Newfoundland ecoregions mentioned in text after Damman (1983) and Meades and Moore (1994).The colored triangle indicates our study area. Colored circles are other Newfoundland study sites: Bass Pond (Rosenburg et al., 2005), Cheeseman Lake (Finkenbinder et al., 2016), Norman’s Pond (Finkenbinder et al., 2022) and Nordan’s Pond Bog (Daley et al., 2009). Data sources: Department of Environment and Climate Change (2024), Statistics Canada (2021) and Baldwin et al., (2019).
Terra Nova National Park is subject to prevailing westerly winds and the influence of the Labrador Current, which result in a continental climate with significant maritime influences (Power, 1995; Parks Canada, 2019). The park has brief summers along with moderate winters. June–August average (total average was 4.8) temperature between 1981 and 2010 was 14.9°C with total annual average precipitation of 1218 mm (Environment Canada and Climate Change, 2023).
Field and laboratory methods
Sediment collection and chronology
A 5.2 m long sediment core was collected from Arnold’s Pond in 2017 using a modified Livingstone piston corer (Wright, 1967) operated from a raft anchored by ropes to the shore. The uppermost 65 cm was collected using an acrylic tube fitted with a piston and attached to rods with the top 27 cm consisting of flocculent sediment and the rest was gyttja (Wright, 1991).
We picked 13 terrestrial plant macrofossil samples for accelerator mass spectrometry 14C dating by the Radiochronology Laboratory at the University of Laval in Québec, Canada (Table 1). We calibrated the radiocarbon dates to calendar years before present (cal. yr BP) using the IntCal20 calibration curve in OxCal v4.4, with the mud/water interface set to −67 cal. yr BP to reflect the age of the uppermost sediment at the time of sampling (Bronk Ramsey, 2009; Reimer et al., 2020). To reconstruct sedimentation rates, we created an age–depth model (Figure 2) in v2.5 of the R package “BACON” using Bayesian statistics (Blaauw and Christen, 2011).
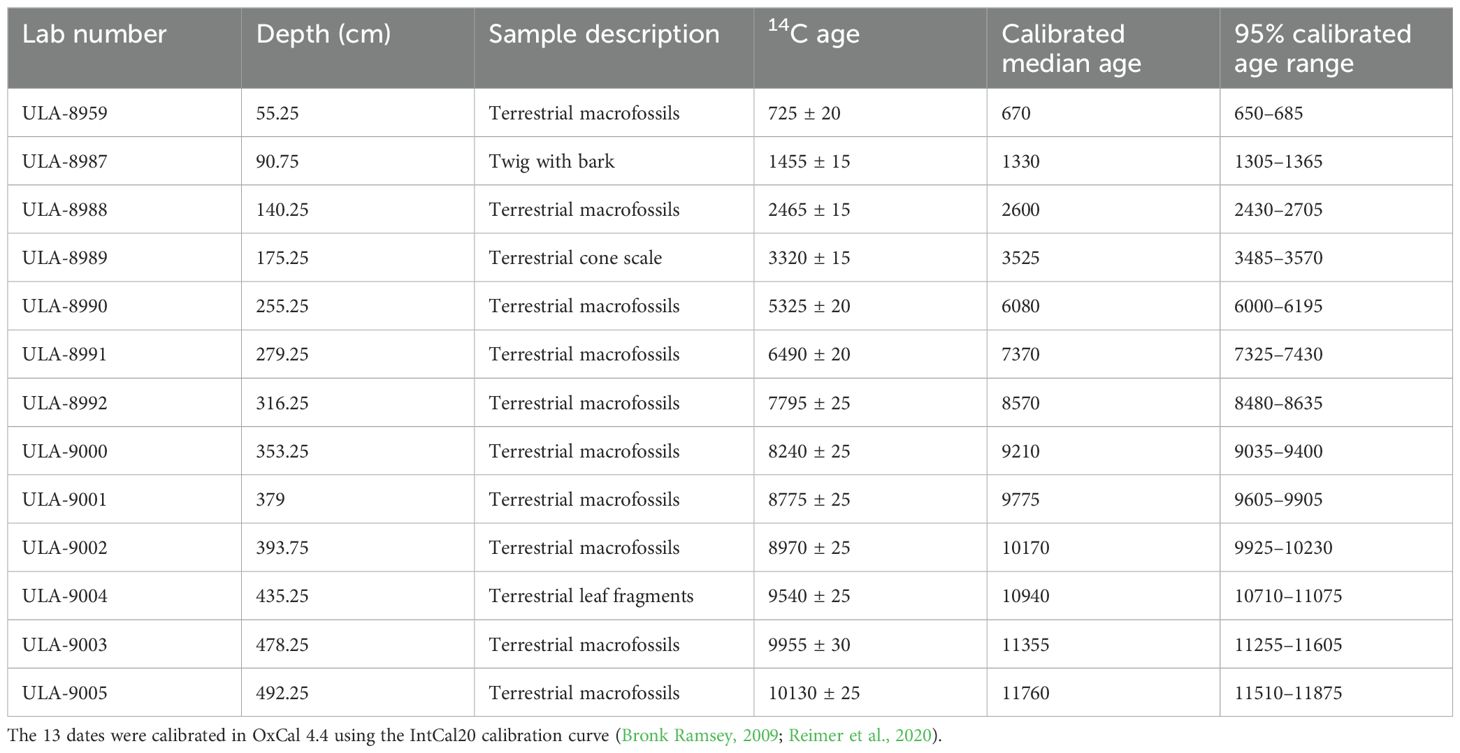
Table 1. Radiocarbon dates from Arnold’s Pond, Terra Nova National Park, Newfoundland and Labrador, Canada.
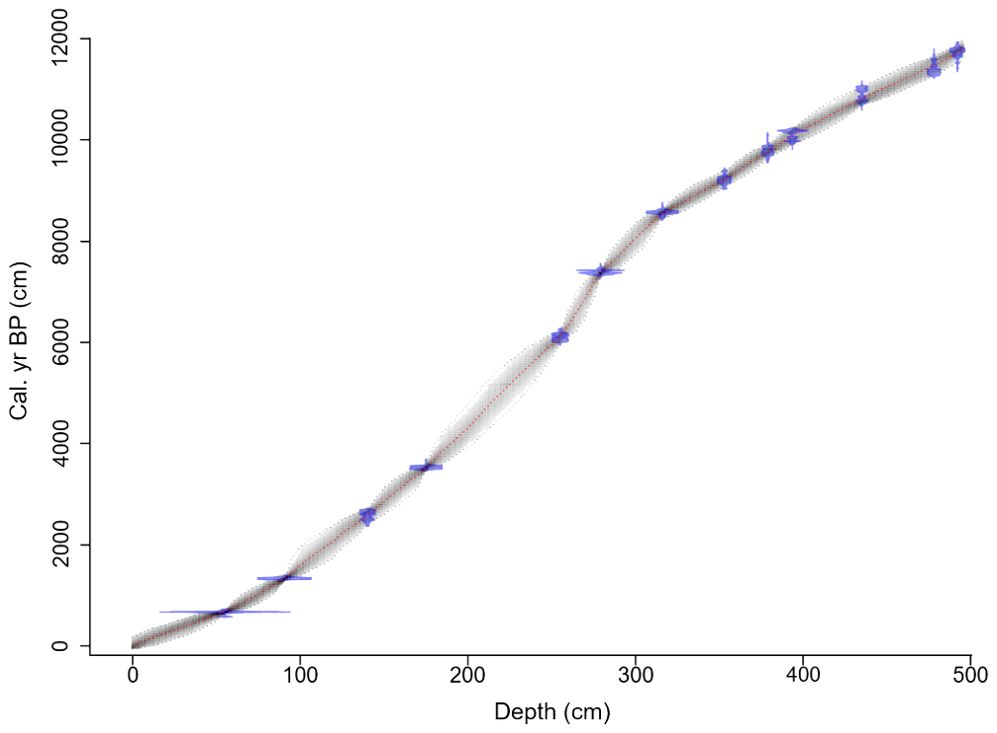
Figure 2. Bayesian age–depth model for Arnold’s Pond. We radiocarbon dated 13 samples, most of which consisted of multiple terrestrial plant macrofossils. We created the model in v2.5 the R package “BACON” using IntCal20 (Bronk Ramsey, 2009; Reimer et al., 2020). The blue bands represent the highest probable density of each date, and the grey outline represents the 95% confidence intervals of the calibration range. The red line shows optimal model based on the mean age for each depth.
Sediment subsampling
We contiguously subsampled 2.0–6.0 cm3 of sediment at 0.5 cm increments for charcoal and sand analysis. We prepared the samples for charcoal counting using the methods described by Long et al. (1998), Enache and Cumming (2007), and Walsh et al. (2010). We placed samples into 100 ml beakers, added 40 ml of 10% KOH and let them stand overnight. The next morning, we added 40 ml of commercial bleach to each sample at 7-minute intervals and lightly stirred with a wooden stir stick. Each sample sat for an hour before we gently sieved it through a 250 μm sieve stacked on a 150 μm sieve. We transferred the residues to a gridded Petri dish for counting. An effort was made to count a minimum of 10 charcoal pieces per sample as recommended by Higuera et al. (2010). We counted charcoal pieces between 150 and 250 μm to reconstruct local fire events. Previous studies have shown that macrocharcoal pieces (>150 μm) are well suited for reconstructing local fires within 0–3 km of the site, although regional fires >32 km away also contribute some macrocharcoal (Clark et al., 1998; Pisaric, 2002; Lynch et al., 2004; Higuera et al., 2007; Walsh et al., 2010; Oris et al., 2014a; Hennebelle et al., 2020). We counted sand grains in the >250 μm fraction as an indicator of landscape stability. We enumerated sand grains and charcoal using a stereomicroscope set to 17× magnification.
We indexed sediment organic content by loss-on-ignition analysis (LOI) following Dean (1974) and Heiri et al. (2001). We contiguously subsampled 1.0 cm3 of sediment at 1 cm increments.
We conducted the pollen analysis using 0.5–1.0 cm3 of sediment taken at 8 cm intervals and processed using standard methods (Faegri et al., 1989). We added Lycopodium clavatum (L.) spores as a spike to calculate pollen concentration and pollen accumulation rates (Davis and Deevey, 1964). We identified a minimum of 300 terrestrial pollen grains and spores using a compound microscope at 400× magnification. We identified pollen grains using a reference collection at the University of New Brunswick and the key of McAndrews et al. (1973). Picea mariana, P. glauca (Moench) Voss and P. rubens Sarg. grains were identified following Lindbladh et al. (2002). Alnus viridis (Chaix) DC and Alnus incana (L.) Moench separation was aided with reference to Richard (1970). Separation of the aboreal fraction of Betula spp. follows Ives (1977); grains measuring 20 µm or less are presumed to be from dwarf birches. Pollen abundance was expressed as a percentage of all terrestrial herb, shrub, and arboreal pollen. Picea and Pinus species abundances were determined by ratio, at 16 cm intervals. This approach was utilized as we wanted to use species level abundance for zonation, but the undifferentiated portion would have been the dominant component in every sample of Picea and Pinus. To account for this, we assigned grains that we could not identify due to their orientation as undifferentiated and added these counts to identified grains to form a new component Picea or Pinus total. After primary counting was complete, we went back and identified at least 25 grains of Picea with the exception of one sample and at least 25 grains of Pinus where overall abundance was great enough. We then determined the ratio of each taxon per sample and multiplied the resulting number by the total number of Picea and Pinus grains present in the sample. This resulted in a new grain count for each taxon and these new values were used in zonation.
Statistical treatment of data
We determined the number of pollen zones using a stratigraphically constrained cluster analysis (CONISS) in version 1.0-5 of the R package “rioja” (Juggins, 2022). Terrestrial taxa with an abundance of at least 5% in one sample were included for zonation. Picea totals were added post zonation for additional clarity to show the overall trends in Picea at a higher resolution.
Prior to subjecting the charcoal data to peak detection in CharAnalysis (Higuera et al., 2009), an ensemble member approach was applied to the charcoal data in order to reduce user bias when selecting the optimal pretreatment parameters for smoothing window size and filter type (Blarquez et al., 2013). We then subjected charcoal data to peak detection in CharAnalysis, where charcoal concentration (pieces cm−3) and sediment accumulation (cm yr−1) were resampled to equal intervals based on median sampling from the raw charcoal record to produce an interpolated series (CHARint). A window of 750 yr utilizing a moving median smoothing filter was applied to the (CHARint) series to define and model the low frequency trends (CHARback). High frequency trends (CHARpeak) were defined via residuals (CHARpeak = CHARint − CHARback) and assumed to be controlled by two additive components of CHARnoise and CHARfire, where CHARnoise represents the variability caused by sediment mixing and within pond redistribution of charcoal. CHARfire consists of values that exceed CHARnoise and represent local fires. The mean and variation around the distribution of CHARnoise was determined by using a Gaussian mixture model and the 95th percentile of a locally defined threshold was used for CHARfire. Peaks in the charcoal data that exceed the threshold were screened using a Poisson distribution test to ensure that the detected peaks varied significantly from the minimum charcoal count within the proceeding 75 yr. Samples with less than a 5% chance of originating from the same distribution represent peaks in the charcoal record that originated from a local fire. Fire frequency and fire return intervals were smoothing using a 1000 yr window. A signal-to-noise index was used to ensure that the charcoal record is suitable for peak detection. This index describes the separation between CHARfire and CHARnoise. It has been suggested that a record with a signal-to-noise index > 3 is suitable (Kelly et al., 2011).
Results
Chronology
The chronology for Arnold’s Pond uses 13 calibrated radiocarbon dates from terrestrial plant macrofossils (Table 1). All 13 samples were in chronological order. On average, each 1 cm of sediment represented 27 yrs from 0–300 cm and 19 yrs from 301–495 cm. Using the model, we extrapolated from 492.25 cm to the end of the organic section of the core at 495 cm and obtained a basal age estimate of ~11,800 cal. yr BP (Figure 2). Using the model, we interpolated the ages between 55.25 cm and the mud/water interface.
Sediment stratigraphy
The basal unit of the core (520–495 cm) consisted of grey clay with gravel (Figure 3A) and was characterized by very low LOI (~1%, Figure 3B) with a high sand content. Two small bands of brownish silt at 495–492 cm marked a rise in the LOI profile (~8%) and sharp decline in sand grain concentration (Figure 3C). From 487–475 cm there was a grey silt layer where the LOI dropped slightly, coincident with a large increase in sand grain counts. Following this grey silty layer, organic content rose from 475–425 cm (~15%) as the sediment transitioned from a light brown to dark brown organic silt with periodic black layers, as sand grain counts decreased. From 425–27 cm the core was a uniform dark brown/black gyttja with an average LOI of ~34% with abundant charcoal (Figures 3D, E). From 27–0 cm the core was brown and flocculent with an average LOI of ~32% (Lake, 2022).
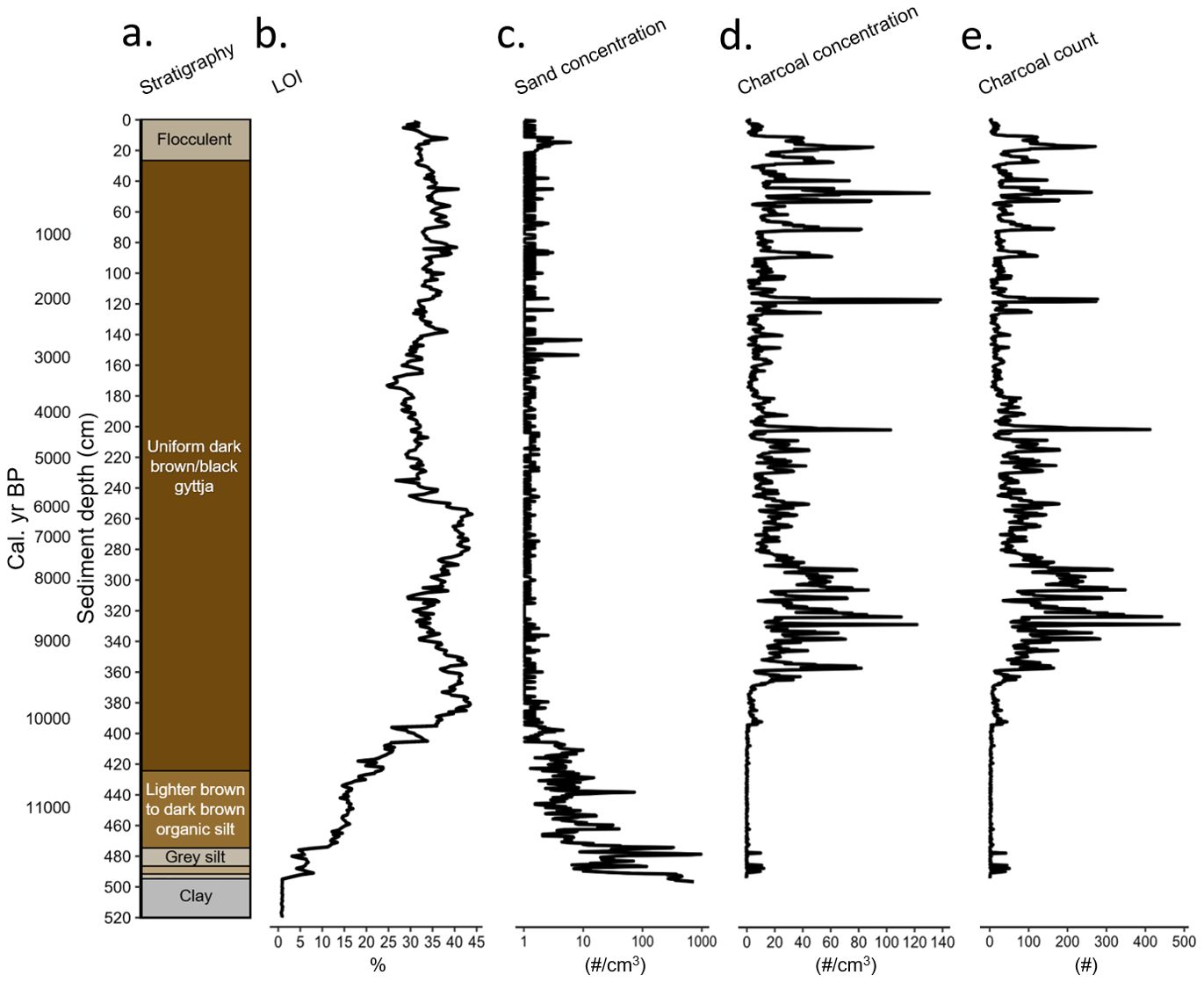
Figure 3. Arnold’s Pond: (A) Sediment stratigraphy, (B) Loss-on-ignition (LOI%), (C) Log10 transformed sand concentration (#/cm3), (D) Charcoal particle concentration (#/cm3), (E) Charcoal particle count (#).
Pollen zones
We initially identified five pollen zones, however, because the first zone comprises only a single sample at the very start of the record, we rejected this zone and recognize only four zones. Each zone was named after the dominant terrestrial taxa or taxon (Figure 4). Zone 4 was subdivided further into subzones 4a and 4b.
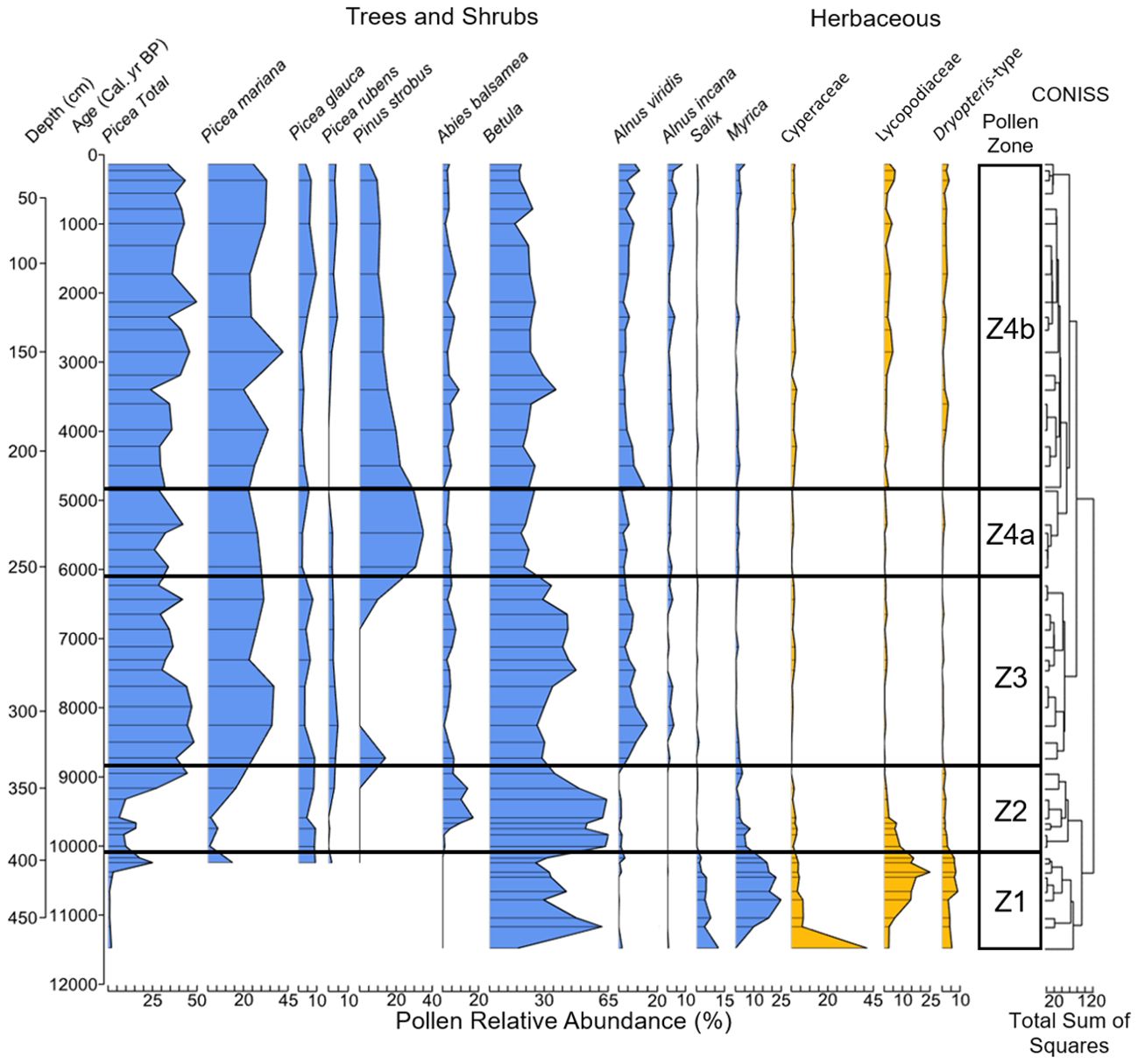
Figure 4. Pollen relative abundance (%) of the most common non-aquatic taxa (≥5% in ≥1 samples). We determined the number of pollen zones using a stratigraphically constrained cluster analysis in version 1.0-5 of the R package “rioja” (Juggins, 2022).
Zone 1 shrub-herb (478–394 cm, ~11,500–10,100 cal. yr BP)
Zone 1 was dominated by shrub and herbaceous taxa. Shrub-Betula was the most abundant shrub, as Salix, Myrica and Cyperaceae reached their maximum relative abundances and total pollen accumulations (Figures 4, 5, 6A). Lycopodiaceae and Dryopteris-type abundances increased towards the end of the zone, as Picea arrived on the landscape. The arrival of Picea coincides with a brief spike in the percentage of Betula grains originating from trees (Figure 6A).
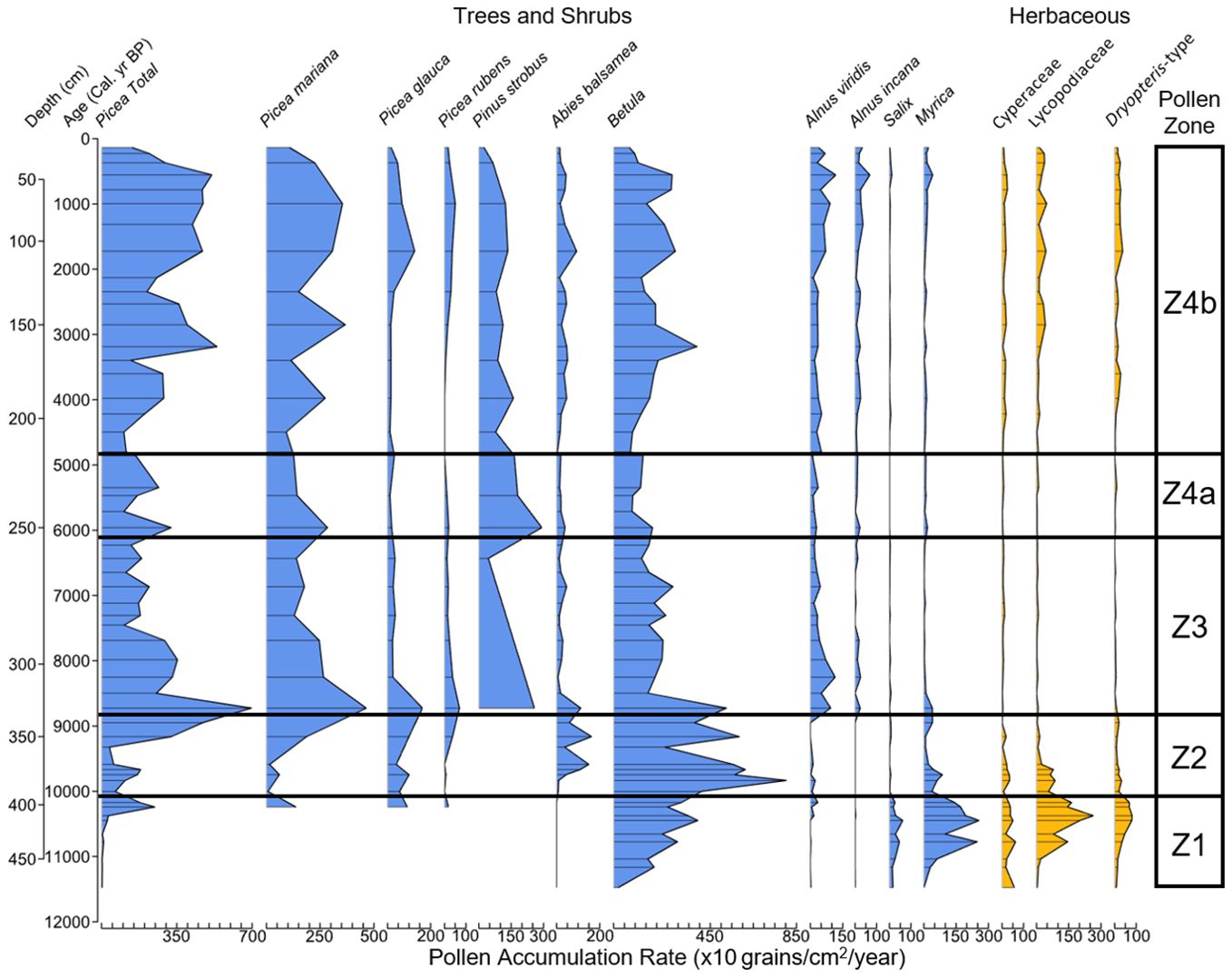
Figure 5. Pollen accumulation rate (x10 grains/cm2/year) of the most common non-aquatic taxa present at Arnold’s Pond, classified by pollen zone.
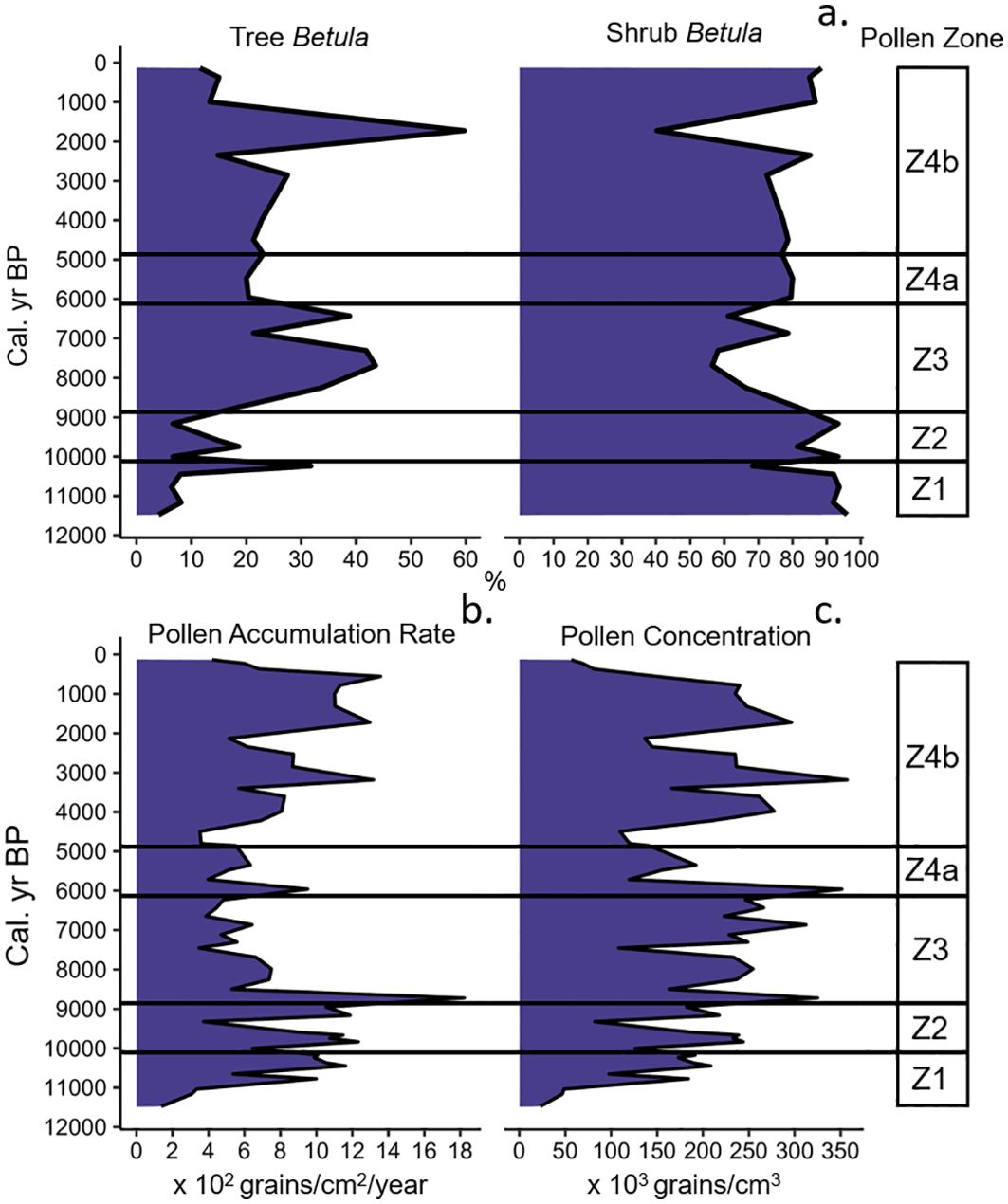
Figure 6. (A) Percentage of Betula spp. grains originating from trees and shrubs, (B) Pollen accumulation rate (x103 grains/cm2/year), (C) Pollen concentration (x103 grains/cm3) for Arnold’s Pond, classified by pollen zone.
Zone 2 Betula-Picea-Abies (394–333 cm, ~10,100–8850 cal. yr BP)
Betula continued to be the dominant taxon throughout this zone. Abies arrived ~9700 cal. yr BP and reached its maximum values (~17%) ~9600 cal. yr BP and then declined to modern values. Picea abundance increased at ~9200 cal. yr BP and continued to rise as Betula abundance declined into the zone transition.
Zone 3 Picea-Betula-Alnus (333–255 cm, ~8850–6100 cal. yr BP)
Picea abundance initially increased and then remained constant until ~7700 cal. yr BP. P. rubens reached its maximum abundance during this time of ~5% ~8300 cal. yr BP. Betula abundance remained constant until ~8000 cal. yr BP. During this time, the percentage of tree-Betula pollen increased and reached a maximum of ~43% of total Betula by ~7700 cal. yr BP and remained elevated until ~7300 cal. yr BP. The average percentage of tree-Betula grains was ~36%, which was the greatest of the record. A.viridis increased during this zone and peaked ~8250 cal. yr BP, then leveled out to modern values. A. incana abundance also increased, however, A. viridis remained the dominant alder type in this zone and the rest of the record. A brief peak in P. strobus ~8750 cal. yr BP marks the arrival of this species and coincides with a spike in both pollen concentration and accumulation rate (Figures 6B, C). Betula returned to being the dominant taxon on the landscape for much of zone following a sharp decline in Picea ~7450 cal. yr BP.
Zone 4a Pinus strobus (255–215 cm, ~6100–4850 cal. yr BP)
The distinct peak of P. strobus characterizes this zone. Other tree taxa (Picea, Betula, Abies) remain somewhat constant throughout this zone as total P. strobus abundance peaked ~5500 cal. yr BP at ~35% and then slowly declined.
Zone 4b Picea-Pinus strobus-Betula (215–0 cm, ~4850–14 cal. yr BP)
With the decline of P. strobus, Picea became the most dominant tree taxon (~37%). Both P. mariana and P. glauca reached their maximum abundances during this zone of ~42% at ~2900 cal. yr BP and 10% at ~1700 cal. yr BP respectively. Lycopodiaceae and Dryopteris-type abundances continued to increase throughout the zone along with both types of Alnus. The final sample of the zone showed A. incana reaching its maximum abundance, with Myrica attaining ~5%, which was not seen since zone 2.
Charcoal and local fire events
We omitted pollen zone 1 from CharAnalysis due to low charcoal counts. Of the 169 samples that comprise zone 1, only 3 met or exceeded counts of 10 charcoal pieces per sample, despite increasing the sediment volume to 4 cc. The average charcoal concentration for zone 1 was 0.7 pieces cm−3. With such sparse data, a fire frequency reconstruction for this zone would not be reliable (Magne et al., 2020).
Interpolated charcoal concentration across the entire record ranges from 0.0–121.4 pieces cm−3 with an average of 20.6 pieces cm−3. Charcoal accumulation ranges from 0.0–8.2 pieces cm−2 yr−1 with an average of 0.8 pieces cm−2 yr−1. CharAnalysis identified 45 fire events across pollen zones 2–4b with an average mFRI of ~215 yr. The global signal-to-noise index is 4.7, which is well above the generally accepted minimum value of 3. We interpret large peak-magnitude fire events to reflect the proximity and/or severity of a fire event (Walsh et al., 2010).
Zone 2 Betula-Picea-Abies (394–333 cm, ~10,100–8850 cal. yr BP)
CHAR was low between ~10,100–9600 cal. yr BP (Figure 7A). There was a rapid uptick in CHAR beginning ~9600 cal. yr BP, and it remained elevated into the zone transition. Six fire events (Figure 7A) were detected, with a mean fire frequency of 4.5 fires 1000 yr−1 (Figure 7B). One large peak-magnitude event occurred at ~9350 cal. yr BP (Figure 7C). The signal-to-noise index was < 3 for the first ~100 yr of the zone, but no fire events were detected during this period (Figure 7D).
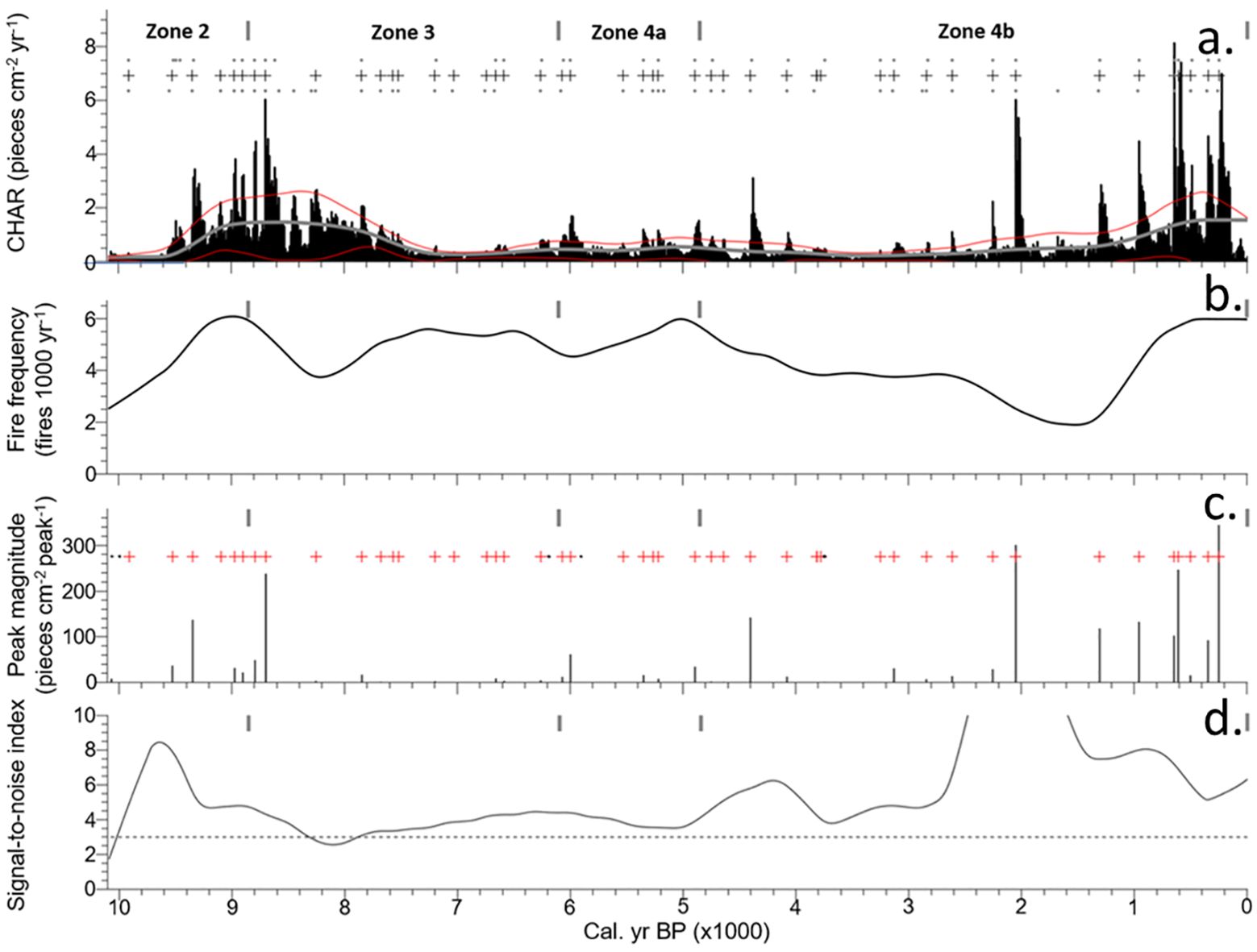
Figure 7. Charcoal records for Arnold’s Pond across four pollen zones: (A) Interpolated charcoal influx with background levels (greyline), threshold limit (red line), and local fire events (grey cross), (B) Time series of smoothed fire frequency, (C) Sum of all samples that exceeded the peak threshold with local fire events (red cross), and peaks that fail to pass the Poisson minimum-count screen (black dots), (D) Signal-to-noise index.
Zone 3 Picea-Betula-Alnus (333–255 cm, ~8850–6100 cal. yr BP)
Initially, CHAR was high, with one large peak-magnitude event within the first 150 yr of the zone. This elevated level of CHAR was followed by a steady decline until ~7200 cal. yr BP, when values bottomed out and remained low until the end of the zone. From ~8300–7900 cal. yr BP the signal-to-noise index was < 3 despite high charcoal concentrations. Within this low signal-to-noise window, 1 of the 13 fire events was detected. The mean fire frequency for the zone was 5.0 fires 1000 yr−1.
Zone 4a Pinus strobus (255–215 cm, ~6100–4850 cal. yr BP)
CHAR was lower than previous zones, but relatively constant. One large peak-magnitude fire event was detected at ~6000 cal. yr BP. Seven fire events were detected with a mean fire frequency of 5.2 fires 1000 yr−1. This zone has the lowest mFRI of the record at 196 yr (Figure 8).
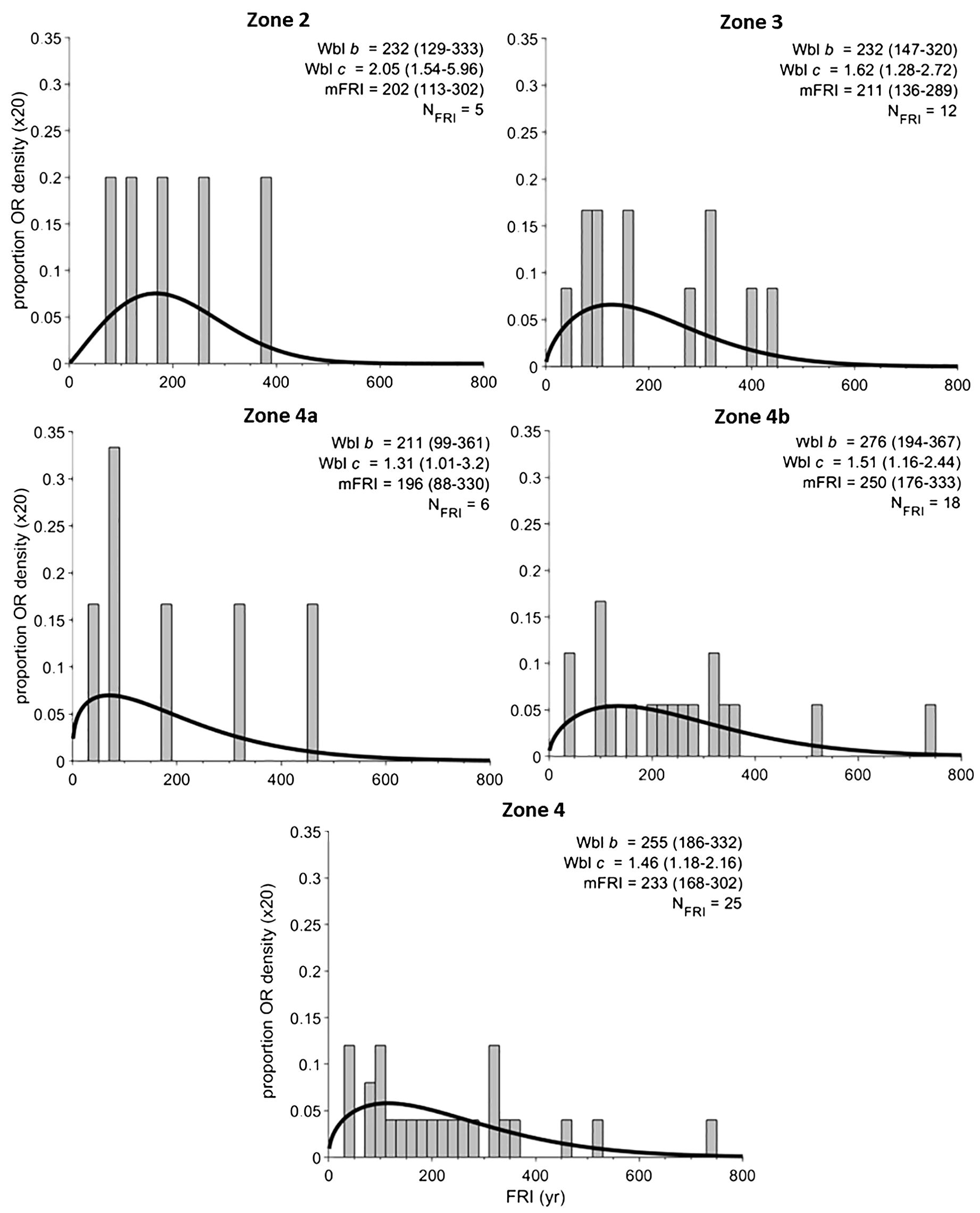
Figure 8. Fire return intervals for each pollen zone. The proportion or scaled density (y-axis) of fire return intervals within each zone displayed in a histogram with 20-yr bins. Zones that fit the Weibull model and pass the goodness-of-fit test are described by the variables WBL c and b (unitless) with 95% confidence estimates. Also listed are mean fire return interval (mFRI) along with the 95% confidence estimates. The number of fire return intervals for each zone is listed as NFRI.
Zone 4b Picea-Pinus strobus-Betula (215–0 cm, ~4850–14 cal. yr BP)
CHAR at the start of this zone was low, but gradually increased with the second-largest peak-magnitude fire event of the record at ~2050 cal. yr BP. CHAR quickly increased following a fire event at ~1300 cal. yr BP and leveled out at ~600 cal. yr BP and remained elevated for the rest of the record. The final ~1300 yr of the record featured 6 large peak-magnitude fire events with the largest peak-magnitude event of the record at ~200 cal. yr BP. Nineteen fire events were detected in this zone with a mean fire frequency of 3.9 fires 1000 yr−1. Background levels of CHAR gradually increased thorough the zone.
Trends in sand
Sand grain concentration from ~498–473 cm was high (Figure 3C), but variable with an average concentration of ~156 grains/cc and a maximum of ~941 grains/cc. Sand concentration declined from ~472–409 cm with an average of ~7 grains/cc. One spike of ~71 grains/cc occurred at ~439 cm. Sand grain concentration for the remainder of the record was low, with an average of ~0.4 grains/cc. Three further spikes in sand concentration occur at 153.75, 143.75 and 14.75 cm, when sand concentrations reached 8, 7 and 6 grains/cc respectively.
Discussion
Fire regime of the current Boreal Forest at Arnold’s Pond
The current mix of Picea-Pinus strobus-Betula found at Arnold’s Pond has been on the landscape since ~4850 cal. yr BP, as represented by pollen zone 4b. The mFRI for this zone is 250 yr (Figure 8), with confidence estimates of 176–333 yr. Our results indicate that the average fire return interval associated with the current vegetation type is significantly longer than the park’s mean stand age of ~100 yr (Power, 1996), which has been used as a baseline for ecosystem management by Parks Canada (Simpson, 2007). This suggests that the park is recovering from a series of disturbance events other than just fire, such as windthrow, insect defoliators, disease agents and anthropogenic influences, i.e., logging. The potential impact of indigenous fire usage on the landscape is poorly understood, but could have affected the fire regime. The island of Newfoundland has been occupied by various cultural complexes of indigenous peoples since ~5100 BP (Holly et al., 2022). As of 2019, Terra Nova National Park has documented 32 archeological sites covering over 5000 years of cultural complexes (Higdon and Homosits, 2020).
The 250 yr mFRI found at Arnold’s Pond fits within the range of sedimentary charcoal-based fire history reconstructions within the western Québec black spruce-moss and western black spruce-lichen bioclimatic subdomains. Hennebelle et al. (2018) used paleoecological data from 16 sites to reconstruct trends in vegetation and fire during the Holocene across the subdomains of western Québec. They found that the regional mFRI during the Holocene was 240.1 yr. Previous studies of the same sites indicate that the current mFRI is between 180–333 yr (Carcaillet et al., 2010; Hély et al., 2010; Ali et al., 2012; Oris et al., 2014b; El-Guellab et al., 2015; Remy et al., 2017a, b). Current vegetation in these subdomains is similar to that of Arnold’s Pond, with P. mariana being the most dominant species with a mix of A. balsamea and other species depending on the area (Remy et al., 2017a, b; Hennebelle et al., 2018). These western Québec subdomains have an east–west precipitation gradient from 1005–721 mm and a north-south June–July average temperature gradient from 11.7–14.6°C (Natural Resources and Forests, 2023).
The eastern black spruce-moss and eastern paper birch-fir bioclimatic subdomains in the Côte-Nord region of Québec also show similar fire estimates to ours. Cyr et al. (2012) and Couillard et al. (2021) reported mean fire intervals ranging between 226–410. Tcheumeleu et al. (2023) and Bouchard et al. (2008) indicated MFIs of 181 and 270 to >500 yr. Precipitation in the subdomain ranges from 1006–1108 mm and temperature from 12.9–14.6°C (Natural Resources and Forests, 2023). The dominant species in these studies are similar to the previous subdomain, with current stands dominated by P. mariana and/or A. balsamea, with other species playing a minor role (Bouchard et al., 2008; Cyr et al., 2012; Tcheumeleu et al., 2023).
Our mFRI estimate is significantly shorter than the estimation of ~770 yr for the entire island of Newfoundland as suggested by Arsenault (2015) and Arsenault et al. (2016). However, the authors did indicate an increased prevalence of historical wildfire in this ecoregion of Central Newfoundland due to the more continental climate. This, coupled with a fire estimate similar to that of the eastern, mainland boreal forest, indicates that these forests are likely influenced by similar climatic forcings.
Influence of vegetation on the fire regime
The mFRI was not calculated between ~11,500–10,100 cal. yr BP because only 3 of the 169 samples met the required minimum charcoal count of 10 pieces (Higuera et al., 2010), despite generally using larger samples of 4 cc of sediment (Figure 3E). The average concentration across those samples was 0.6 pieces cm−3, indicating that fire was not a major influence on the landscape during zone 1 The amount of fire activity can be inferred by comparing the charcoal concentration in this zone to a study from Alaska. The dominance of shrub-Betula, Salix, Myrica, and herbs from ~11,500–10,100 cal. yr BP, suggests that the landscape was a shrub tundra. The mean CHAR values for three sites in Alaska classified as tussock-sedge, dwarf-shrub and low dwarf-shrub tundra ranged from 0.002–0.008 pieces cm−2 yr−1 (Chipman et al., 2015). The average charcoal concentration ranged from 0.14–0.59 pieces cm−3, with a mFRI ranging from 4730–6045 yr (Chipman et al., 2015). The average charcoal concentration in zone 1 ranged from 0.0–8.25 pieces cm−3 with ~80% of samples having a concentration less than 1.0 pieces cm−3. The average of 0.6 pieces cm−3 is similar to the Alaskan sites. From ~11,500–10,100 cal. yr BP, LOI (Figure 3B) was initially low while sand grain concentration was high (Figure 3C). This large influx of sand suggests that the landscape surrounding the pond was highly unstable and prone to erosion. This lack of stability helps to explain in part why charcoal (Figures 3D, E) was low during this ~1200 yr period, as shrub and herbaceous taxa are often patchily distributed on unstable landscapes, which lowers the probability that a fire can be sustained and spread (Higuera et al., 2009, 2011).
A significant transition from a shrub tundra to an open forest occurred between ~10,100–8850 cal. yr BP (zone 2), which further increased fuel availability (Figures 4, 7). During the initial Betula-dominated phase of this interval, only three fire events occurred. However, when Picea increased in the latter third of zone 2, the fire frequency increased.
The fire-facilitated Picea was also likely in low density stands for much of zone 2, as the average pollen influx of ~1,750 grains/cm2/year was lower than the average Picea influx of pollen zone 4b where a closed canopy was likely present (~3,100 grains/cm2/year) (Figure 6B). The rise of Picea abundance and accumulation from ~9200 cal. yr BP marked a sharp rise in CHAR (Figures 4, 7A, 9A), as LOI (Figures 3B, 9B) declined and the fire frequency continued to increase, reaching the highest value of the record at ~9000 cal. yr BP, with 6.1 fires 1000 yr−1 (Figures 7B, 9C). The rise of Picea likely influenced the increase in CHAR due to the addition of fuel for fire events. This interpretation is supported by studies from Alaska and the Yukon, which found that the transition from a shrub to Picea-dominated landscape coincided with a substantial increase in fire activity (Hu et al., 2006; Higuera et al., 2009; Kelly et al., 2013; Prince et al., 2018).
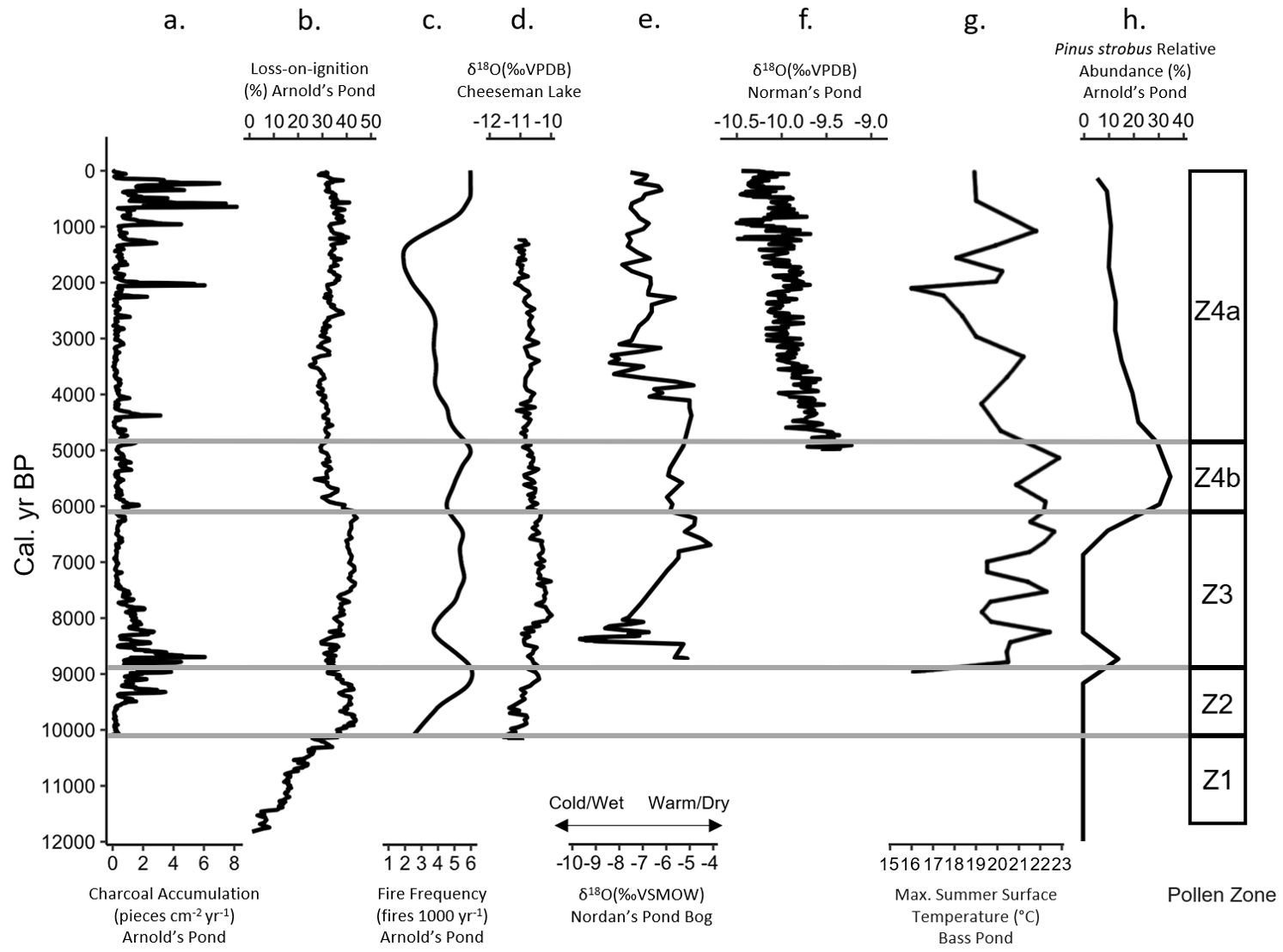
Figure 9. Cumulative figure comparing Arnold’s Pond data to other paleoclimate proxies present on the island of Newfoundland, classified by pollen zone. (A) Arnold’s Pond charcoal accumulation (pieces cm−2 yr−1), (B) Arnold’s Pond Loss-on-ignition (%), (C) Arnold’s Pond fire frequency (fires 1000 yr−1), (D) Cheeseman Lake carbonate δ18O(‰VPDB) (Finkenbinder et al., 2016), (E) Nordan’s Pond Bog δ18O(‰VSMOW) (Daley et al., 2009), (F) Norman’s Pond δ18O (‰VPDB) (Finkenbinder et al., 2022), (G) Bass Pond chironomid inferred summer surface temperature (°C) (Rosenburg et al., 2005), (H) Arnold’s Pond Pinus strobus relative abundance (%).
It is not clear if vegetation was the sole factor influencing fire activity during zone 2. The lack of independent paleoclimate reconstructions on the island of Newfoundland prior to ~10,100 cal. yr BP leaves us without a climatic baseline and introduces uncertainty around the influence of climate on fire activity. One reconstruction ~620 km northeast of our study area finds a positive summer warming trend from ~10,750 cal. yr BP until ~9500 cal. yr BP, which was followed by cooler and more variable temperatures for the remainder of the zone (de Vernal and Hillaire-Marcel, 2006). For most of the Holocene, however, other factors, such as changing climate, predominantly influenced the time between fire events.
Influence of climate on the fire regime
Newfoundland has several paleoclimate proxy studies available that provide a framework for insights into past Holocene climatic conditions. Cheeseman Lake (Finkenbinder et al., 2016) located in the Western Newfoundland Forest ecoregion, Nordan’s Pond Bog (Hughes et al., 2006) located in Eastern Hyper-Oceanic Barrens and Norman’s Pond (Finkenbinder et al., 2022) located in the Western Newfoundland Forest ecoregion δ18O records provide reconstructions of changes in temperature and precipitation over time (Figures 1, 9D–F) (Damman, 1983; Meades and Moore, 1994). The Cheeseman Lake δ18O record is primarily controlled by summer water temperature with seasonality of precipitation and surface boundary conditions playing secondary roles (Finkenbinder et al., 2016). The Norman’s Pond record is primarily influenced by annual changes in temperature and precipitation with seasonal changes in precipitation also playing a primary role (Finkenbinder et al., 2022). The Nordan’s Pond Bog δ18O record is strongly influenced by changes in precipitation, thus it primarily reflects changes in potential energy or water balance (Finkenbinder et al., 2016). Bass Pond (Rosenburg et al., 2005) located in the Strait Of Belle Isle ecoregion provides a fossil midge-inferred maximum summer surface-water-temperature record (Figures 1, 9G) (Damman, 1983; Meades and Moore, 1994). These proxies form the basis for the comparisons drawn below. Unfortunately, none of these sites has a complete Holocene record as they are all at most 10,100 yr old or younger.
At approximately 8850 cal. yr BP, fire frequency decreased with notable dips in CHAR coinciding with regional climate variability and the 8200 event (Figures 7A, B, 9A, C). It is likely that Picea sustained the high levels of CHAR at the zone transition, but a shift towards a more variable climate ~8500 cal. yr BP impacted fire activity. Two dips in CHAR occurred ~8500 and 8350 cal. yr BP and these coincided with a reduction in LOI values between 8450–8400 cal. yr BP (Figures 3B, 7A, 9A, B). The Cheeseman Lake and Nordan’s Pond Bog δ18O records both shifted towards more negative values beginning ~8500 and 8400 cal. yr BP respectively (Figures 9D, E) (Daley et al., 2009; Finkenbinder et al., 2016). A testate-amoeba water-table depth reconstruction for Nordan’s Pond Bog also showed a shift towards wetter conditions ~8300 cal. yr BP (Hughes et al., 2006; Amesbury et al., 2013). Finkenbinder et al. (2016), Daley et al. (2009) and Hughes et al. (2006) suggested that the abrupt change in climate is associated with the 8200 cal. yr BP cold event (Barber et al., 1999). Fire frequency continued to decline during this cooler/wetter climate and bottomed out ~8200 cal. yr BP, before increasing as the climate began to warm/dry (Figures 7B, 9C). It is likely that the shift towards cooler and wetter conditions resulted in the observed decrease in fire frequency and CHAR between ~8800 and 8200 cal. yr BP.
The warmest temperature of the Holocene, as indicated by the Bass Pond record (Rosenburg et al., 2005) coincided with peak P. strobus values ~5500 cal. yr BP at Arnold’s Pond (Figures 9G, H). Peak Pinus pollen abundances in the mid-Holocene have generally been regarded in Newfoundland as an indicator of the Holocene Thermal Maximum (MacPherson, 1995). LOI reached its maximum value ~600 yr before peak P. strobus (Figures 3B, 9B, H), which suggests that the Holocene Thermal Maximum may have occurred earlier than suggested by the rise in P. strobus and fits within the 4000–7000 cal. yr BP range of the Holocene Thermal Maximum from a chironomid-inferred mean August temperature reconstruction from eastern Québec (Tcheumeleu et al., 2023). The period of elevated P. strobus abundance coincides with the maximum temperatures and dryness suggested by the Norman’s Pond δ18O record, but is offset relative to the Cheeseman Lake record ~300 km to the west (Figures 9D, H). Fire frequency increased with P. strobus abundance, but there was a time lag of ~500 yr between peak P. strobus and the peak fire frequency of 6.0 fires 1000 yr−1 (Figures 7B, 9C, H). This delayed response in the fire frequency occurred during a shift towards generally drier conditions with some reversions to wet conditions ~5500–4500 cal. yr BP as indicated by the Nordan’s Pond Bog water-table depth reconstruction (Hughes et al., 2006; Amesbury et al., 2013). Our trend of increased fire frequency between ~5800–4600 cal. yr BP (Figure 7B, 9C) corresponds well with sites in northern Québec and Canada. El-Guellab et al. (2015) noted that the average regional fire frequency across 4 lakes in northern Québec was particularly high between 5500–3500 cal. yr BP. A study along an ~2000 km west-to-east transect covering 42 lakes in the boreal forest of eastern Canada noted a pronounced subcontinental biomass burning peak at 4800 cal. yr BP, which occurred ~200 yr after the peak fire frequency of our subzone 4a (Girardin et al., 2019). Our data, in combination with climate reconstructions, suggest that the warmer and drier conditions coinciding with P. strobus zone 4a were likely responsible for the observed increase in fire frequency between ~5800–4600 cal. yr BP and the shortest mFRI of the record of 196 yr (Figure 8).
Following the warmer and drier conditions associated with peak P. strobus, independent regional data from Newfoundland indicate a shift toward cooler and/or wetter conditions (Figures 9E–G), including the Nordan’s Pond Bog water-table depth reconstruction (Hughes et al., 2006; Amesbury et al., 2013). Finkenbinder et al. (2022) hypothesized that the trend of decreasing δ18O values from mid-Holocene onwards, resulted from a combination of cooling and a shift in the distribution of precipitation from the warm to the cold season. Cheeseman Lake is the exception to this trend, as that record displays more variable conditions (Figure 9D). Our data showed that fire frequency declined from 3.8–1.9 fires 1000 yr−1 from ~2600–1500 cal. yr BP (Figures 7B, 9C) and this coincides with regional trends of declining fire frequencies from ~3000–1000 cal. yr BP. This trend has been observed in several studies from Québec and has been partly attributed to a shift towards wetter and cooler conditions during the Neoglacial (Ali et al., 2009, 2012; Oris et al., 2014b; El-Guellab et al., 2015; Remy et al., 2017a, b). An abrupt decrease in solar activity during this time could have also played a role (Van Geel et al., 2000).
From ~1400 cal. yr BP to present, climate was more variable with several abrupt shifts in the δ18O records that impacted the fire activity (Figures 9E, F). Our data indicates that beginning ~1400 cal. yr BP, CHAR and fire frequency increased through to ~500 cal. yr BP when the fire frequency and CHAR leveled out but remained elevated to the end of the record (Figures 7A, B, 9A, C). This period of climate variability coincided with many large peak-magnitude fire events. We noted six large peak-magnitude fire events within the last 1400 years of the record, with the timing of the initial two fire events corresponding well with a period of sub-continental biomass burning between 1250–1050 cal. yr BP (Figure 7C) (Girardin et al., 2019). The variable climate resulting from the Medieval Climate Anomaly and Little Ice Age from 1000–100 cal. yr BP likely contributed to the observed trends in the fire data (Masson-Delmotte et al., 2013). Anthropogenic influences could have been responsible for the increased CHAR production during this time, as there is evidence of indigenous sites in Terra Nova National Park dating to this time period (Curtis, 2015). The combination of a variable climate, along with possible indigenous influences likely resulted in the observed trends in the fire data.
Conclusion
We determined that the mFRI associated with the current vegetation type at Arnold’s Pond is 250 yr. This is longer than that of much of the western and central boreal forest regions of Canada, but is within the 180–330 yr regional mFRI estimates for the black spruce-moss, black spruce lichen and paper birch-fir subdomains of Québec (Carcaillet et al., 2010; Hély et al., 2010; Ali et al., 2012; El-Guellab et al., 2015; Remy et al., 2017a; Hennebelle et al., 2018). Our mFRI is also close to the fire estimates from the Côte-Nord region of Québec where previous studies found the mean fire interval between 226–410 yr and a mFRI between 181 to >500 yr (Bouchard et al., 2008; Cyr et al., 2012; Couillard et al., 2021; Tcheumeleu et al., 2023). However, our estimate is much shorter than the ~770 yr suggested by Arsenault (2015) and Arsenault et al. (2016) for the entire island of Newfoundland, which reflects the higher occurrence of fire in the Central Newfoundland ecoregion characterized by a more continental climate (Arsenault et al., 2016). With our estimate being very similar to that of the eastern, mainland boreal forest as described above, this indicates that these forests are likely influenced by similar climatic forcings.
We found one example where it appears likely that the fire frequency or fire activity shifted as the vegetation changed, but we could not definitively prove if vegetation was the sole factor due to a lack of regional climate data. This occurred during the transition from a shrub tundra landscape to an open forest, which provided additional fuel as Picea became more abundant on the landscape.
We identified an example of a non-synchronous change in the fire frequency and/or CHAR coinciding with a change in climate. This was observed when P. strobus abundance rose but did not initially alter the fire frequency. Instead, there was a ~500‐yr time lag between peak P. strobus and the maximum fire frequency, which was likely due to conditions becoming drier and warmer.
We identified several examples where changes in the fire frequency and/or CHAR coincided with known regional climate events. CHAR and fire frequency declined between ~8800–8200 cal. yr BP and coincided with a shift towards cooler and wetter conditions associated with the 8200 cal. yr BP cold event (Hughes et al., 2006; Daley et al., 2009; Finkenbinder et al., 2016). From ~2600–1500 cal. yr BP a decrease in fire frequency coincided with regional trends of declining fire frequencies from ~3000–1000 cal. yr BP. This can be attributed to a shift towards wetter, cooler conditions during the Neoglacial and possibly an abrupt decrease in solar activity (Van Geel et al., 2000; Ali et al., 2009, 2012; Oris et al., 2014b; El-Guellab et al., 2015; Remy et al., 2017a, b). From ~1400 cal. yr BP to present, fire frequency increased, despite the absence of a major shift in vegetation. This was likely caused by increased climate variability or possibly a change in indigenous people’s use of the land.
Data availability statement
The raw data supporting the conclusions of this article will be made available by the authors, without undue reservation.
Author contributions
NL: Formal analysis, Visualization, Writing – original draft, Writing – review & editing, Investigation, Software, Data curation, Validation. AA: Supervision, Writing – review & editing, Conceptualization, Resources, Project administration, Funding acquisition. LC: Conceptualization, Funding acquisition, Investigation, Project administration, Resources, Supervision, Writing – review & editing.
Funding
The author(s) declare financial support was received for the research, authorship, and/or publication of this article. Funding for this project was supported by a Discovery Grants Program of the Natural Sciences and Engineering Research Council of Canada to LC (RGPIN-2021-02753). Funding for this project was also funded in part by a contribution agreement from Newfoundland and Labrador’s Centre for Forest Science and Innovation and from the Canadian Forest Service. Parks Canada contributed in kind by providing accommodations during field-work.
Acknowledgments
We would like to thank K. Costanza, D. Łuców and J. M. St-Jacques for constructive comments used to improve the manuscript. We would also like to thank R. Skinner and K. Gaudet for their aid in the field and lab. The contents of this manuscript build upon the thesis of NL.
Conflict of interest
The authors declare that the research was conducted in the absence of any commercial or financial relationships that could be construed as a potential conflict of interest.
Publisher’s note
All claims expressed in this article are solely those of the authors and do not necessarily represent those of their affiliated organizations, or those of the publisher, the editors and the reviewers. Any product that may be evaluated in this article, or claim that may be made by its manufacturer, is not guaranteed or endorsed by the publisher.
References
Ali A. A., Blarquez O., Girardin M. P., Hély C., Tinquaut F., El Guellab A., et al. (2012). Control of the multimillennial wildfire size in boreal North America by spring climatic conditions. Proc. Natl. Acad. Sci. 109, 20966–20970. doi: 10.1073/pnas.1203467109
Ali A. A., Carcaillet C., Bergeron Y. (2009). Long-term fire frequency variability in the eastern Canadian boreal forest: The influences of climate vs. local factors. Glob. Change Biol. 15, 1230–1241. doi: 10.1111/j.1365-2486.2009.01842.x
Amesbury M. J., Mallon G., Charman D. J., Hughes P. D. M., Booth R. K., Daley T. J., et al. (2013). Statistical testing of a new testate amoeba-based transfer function for water-table depth reconstruction on ombrotrophic peatlands in north-eastern Canada and Maine, United States. J. Quat. Sci. 28, 27–39. doi: 10.1002/jqs.2584
Amiro B. D., Cantin A., Flannigan M. D., De Groot W. J. (2009). Future emissions from Canadian boreal forest fires. Can. J. For. Res. 39, 383–395. doi: 10.1139/X08-154
Arsenault A. (2015). “The role of large fires in the Canadian boreal ecosystem,” in The Ecological Importance of Mixed-Severity Fires: Nature’s Phoenix. Eds. DellaSala D. A., Hanson C. T. (Elsevier, Waltham, MA), 247–264.
Arsenault A., LeBlanc R., Earle E., Brooks D., Clarke B., Lavigne D., et al. (2016). Unravelling the past to manage Newfoundland’s forests for the future. For. Chron. 92, 487–502. doi: 10.5558/tfc2016-085
Arseneault D. (2001). Impact of fire behavior on postfire forest development in a homogeneous boreal landscape. Can. J. For. Res. 31, 1367–1374. doi: 10.1139/cjfr-31-8-1367
Augustin F., Girardin M. P., Terrier A., Grondin P., Lambert M.-C., Leduc A., et al. (2022). Projected changes in fire activity and severity feedback in the spruce–feather moss forest of western Quebec, Canada. Trees. For. People 8, 100229. doi: 10.1016/j.tfp.2022.100229
Baldwin K., Allen L., Basquill S., Chapman K., Downing D., Flynn N., et al. (2019). Vegetation Zones of Canada: a Biogeoclimatic Perspective. Available online at: https://open.Canada.ca/data/en/dataset/22b0166b-9db3-46b7-9baf-6584a3acc7b1 (Accessed June 27, 2024).
Barber D. C., Dyke A., Hillaire-Marcel C., Jennings A. E., Andrews J. T., Kerwin M. W., et al. (1999). Forcing of the cold event of 8,200 years ago by catastrophic drainage of Laurentide lakes. Nature 400, 344–348. doi: 10.1038/22504
Bergeron Y., Fenton N. J. (2012). Boreal forests of eastern Canada revisited: old growth, nonfire disturbances, forest succession, and biodiversity. Botany 90, 509–523. doi: 10.1139/b2012-034
Bergeron Y., Gauthier S., Kafka V., Lefort P., Lesieur D. (2001). Natural fire frequency for the eastern Canadian boreal forest: consequences for sustainable forestry. Can. J. For. Res. 31, 384–391. doi: 10.1139/cjfr-31-3-384
Blaauw M., Christen J. A. (2011). Flexible paleoclimate age-depth models using an autoregressive gamma process. Bayesian. Anal. 6, 457–474. doi: 10.1214/11-BA618
Blarquez O., Girardin M. P., Leys B., Ali A. A., Aleman J. C., Bergeron Y., et al. (2013). Paleofire reconstruction based on an ensemble-member strategy applied to sedimentary charcoal. Geophys. Res. Lett. 40, 2667–2672. doi: 10.1002/grl.50504
Bouchard M., Pothier D., Gauthier S. (2008). Fire return intervals and tree species succession in the North Shore region of eastern Quebec. Can. J. For. Res. 38, 1621–1633. doi: 10.1139/X07-201
Brandt J. P. (2009). The extent of the North American boreal zone. Environ. Rev. 17, 101–161. doi: 10.1139/A09-004
Bronk Ramsey C. (2009). Bayesian analysis of radiocarbon dates. Radiocarbon 51, 337–360. doi: 10.1017/S0033822200033865
Carcaillet C., Richard P. J. H., Bergeron Y., Fŕchette B., Ali A. A. (2010). Resilience of the boreal forest in response to Holocene fire-frequency changes assessed by pollen diversity and population dynamics. Int. J. Wildl. Fire. 19, 1026–1039. doi: 10.1071/WF09097
Chipman M. L., Hudspith V., Higuera P. E., Duffy P. A., Kelly R., Oswald W. W., et al. (2015). Spatiotemporal patterns of tundra fires: late-Quaternary charcoal records from Alaska. Biogeosciences 12, 4017–4027. doi: 10.5194/bg-12-4017-2015
Clark J. S., Lynch J., Stocks B. J., Goldammer J. G. (1998). Relationships between charcoal particles in air and sediments in west-central Siberia. Holocene. 8, 19–29. doi: 10.1191/095968398672501165
Couillard P. L., Payette S., Lavoie M., Frégeau M. (2021). Precarious resilience of the boreal forest of eastern North America during the Holocene. For. Ecol. Manage. 485, 118954. doi: 10.1016/j.foreco.2021.118954
Curtis J. (2015). “Parks Canada archaeology in Terra Nova National Park,” in Provincial Archaeology Office 2014 Archaeology Review, vol. 13 . Eds. Hull S., Mercer D. (Department of Business, Tourism, Culture and Rural Development, St. John’s, NL), 44–47. Available at: https://www.gov.nl.ca/tcar/archaeology/arch-resources/archaeology-reviews-and-reports/.
Cyr D., Gauthier S., Bergeron Y. (2012). The influence of landscape-level heterogeneity in fire frequency on canopy composition in the boreal forest of eastern Canada. J. Veg. Sci. 23, 140–150. doi: 10.1111/j.1654-1103.2011.01338.x
Daley T. J., Street-Perrott F. A., Loader N. J., Barber K. E., Hughes P. D. M., Fisher E. H., et al. (2009). Terrestrial climate signal of the “8200 yr B.P. cold event” in the Labrador Sea region. Geology 37, 831–834. doi: 10.1130/G30043A.1
Damman A. W. H. (1983). “An ecological subdivision of the island of Newfoundland,” in Biogeography and ecology of the island of Newfoundland. Ed. South G. R. (Dr. W. Junk Publisher, The Hague, Netherlands), 163–206.
Davis M. B., Deevey E. S. (1964). Pollen accumulation rates: Estimates from late-glacial sediment of Rogers Lake. Science 145, 1293–1295. doi: 10.1126/science.145.3638.1293
Dean W. E. (1974). Determination of carbonate and organic matter in calcareous sediments and sedimentary rocks by loss on ignition: comparison with other methods. SEPM. J. Sediment. Res. 44, 242–248. doi: 10.1306/74D729D2-2B21-11D7-8648000102C1865D
Department of Environment and Climate Change (2024). Natural regions of Newfoundland. Available online at: https://www.gov.nl.ca/ecc/natural-areas/gis/gis-data (Accessed June 21, 2024).
de Vernal A., Hillaire-Marcel C. (2006). Provincialism in trends and high frequency changes in the northwest North Atlantic during the Holocene. Glob. Planet. Change 54, 263–290. doi: 10.1016/j.gloplacha.2006.06.023
El-Guellab A., Asselin H., Gauthier S., Bergeron Y., Ali A. A. (2015). Holocene variations of wildfire occurrence as a guide for sustainable management of the northeastern Canadian boreal forest. For. Ecosyst. 2, 15. doi: 10.1186/s40663-015-0039-2
Enache M. D., Cumming B. F. (2007). Charcoal morphotypes in lake sediments from British Columbia (Canada): an assessment of their utility for the reconstruction of past fire and precipitation. J. Paleolimnol. 38, 347–363. doi: 10.1007/s10933-006-9084-8
Environment Canada and Climate Change (2023). Canadian climate normals 1981-2010. Available online at: https://climate.weather.gc.ca/climate_normals/index_e.html (Accessed April 14, 2023).
Erni S., Wang X., Taylor S., Boulanger Y., Swystun T., Flannigan M., et al. (2020). Developing a two-level fire regime zonation system for Canada. Can. J. For. Res. 50, 259–273. doi: 10.1139/cjfr-2019-0191
Faegri K., Kaland P. E., Kzywinski K. (1989). Textbook of pollen Analysis 4th Edn (Hoboken, NJ: John Wiley), 328.
Finkenbinder M. S., Abbott M. B., Steinman B. A. (2016). Holocene climate change in Newfoundland reconstructed using oxygen isotope analysis of lake sediment cores. Glob. Planet. Change 143, 251–261. doi: 10.1016/j.gloplacha.2016.06.014
Finkenbinder M. S., Steinman B. A., Bird B. W., Heilman E. C., Aspey A. R., Mark S. Z., et al. (2022). A 5000-year lacustrine sediment oxygen isotope record of late Holocene climate change in Newfoundland, Canada. Quat. Sci. Rev. 278, 107376. doi: 10.1016/j.quascirev.2022.107376
Foster D. R. (1983). The history and pattern of fire in the boreal forest of southeastern Labrador. Can. J. Bot. 61, 2459–2471. doi: 10.1139/b83-269
Foster A. C., Wang J. A., Frost G. V., Davidson S. J., Hoy E., Turner K. W., et al. (2022). Disturbances in North American boreal forest and Arctic tundra: impacts, interactions, and responses. Environ. Res. Lett. 17, 113001. doi: 10.1088/1748-9326/ac98d7
Fourrier A., Pothier D., Bouchard M. (2013). A comparative study of long-term stand growth in eastern Canadian boreal forest: Fire versus clear-cut. For. Ecol. Manage. 310, 10–18. doi: 10.1016/j.foreco.2013.08.011
Girardin M. P., Portier J., Remy C. C., Ali A. A., Paillard J., Blarquez O., et al. (2019). Coherent signature of warming-induced extreme sub-continental boreal wildfire activity 4800 and 1100 years BP. Environ. Res. Lett. 14, 124042. doi: 10.1088/1748-9326/ab59c9
Greene D. F., Zasada J. C., Sirois L., Kneeshaw D., Morin H., Charron I., et al. (1999). A review of the regeneration dynamics of North American boreal forest tree species. Can. J. For. Res. 29, 824–839. doi: 10.1139/x98-112
Hart S. J., Henkelman J., McLoughlin P. D., Nielsen S. E., Truchon-Savard A., Johnstone J. F. (2019). Examining forest resilience to changing fire frequency in a fire-prone region of boreal forest. Glob. Change Biol. 25, 869–884. doi: 10.1111/gcb.14550
Heiri O., Lotter A. F., Lemcke G. (2001). Loss on ignition as a method for estimating organic and carbonate content in sediments: reproducibility and comparability of results. J. Paleolimnol. 25, 101–110. doi: 10.1023/A:1008119611481
Hély C., Girardin M. P., Ali A. A., Carcaillet C., Brewer S., Bergeron Y. (2010). Eastern boreal North American wildfire risk of the past 7000 years: A model-data comparison. Geophys. Res. Lett. 37, 1–6. doi: 10.1029/2010GL043706
Hennebelle A., Aleman J. C., Ali A. A., Bergeron Y., Carcaillet C., Grondin P., et al. (2020). The reconstruction of burned area and fire severity using charcoal from boreal lake sediments. Holocene 30, 1400–1409. doi: 10.1177/0959683620932979
Hennebelle A., Grondin P., Aleman J. C., Ali A. A., Bergeron Y., Borcard D., et al. (2018). Using paleoecology to improve reference conditions for ecosystem-based management in western spruce-moss subdomain of Québec. For. Ecol. Manage. 430, 157–165. doi: 10.1016/j.foreco.2018.08.007
Heringa P. K., Woodrow E. F. (1991). Soils of the Bonavista Peninsula, Newfoundland. Report No. 7 Newfoundland Soil Survey (Ottawa, ON: Agriculture Canada Research Branch).
Higdon J., Homosits M. (2020). ““Parks Canada 2019 archaeological field activities,” in Provincial Archaeology Office Annual Review 2019, vol. 18 . Eds. Hull S., Mercer D. (Department of Tourism, Culture, Industry and Innovation, St. John’s, NL), 90–120. Available at: https://www.gov.nl.ca/tcar/archaeology/arch-resources/archaeology-reviews-and-reports/.
Higuera P. E., Brubaker L. B., Anderson P. M., Hu F. S., Brown T. A. (2009). Vegetation mediated the impacts of postglacial climate change on fire regimes in the vegetation mediated the impacts of postglacial climate change on fire regimes in the south-central brooks range, Alaska. Ecol. Monogr. 79, 201–219. doi: 10.1890/07-2019.1
Higuera P. E., Chipman M. L., Barnes J. L., Urban M. A., Hu F. S. (2011). Variability of tundra fire regimes in Arctic Alaska: Millennial-scale patterns and ecological implications. Ecol. Appl. 21, 3211–3226. doi: 10.1890/11-0387.1
Higuera P. E., Gavin D. G., Bartlein P. J., Hallett D. J. (2010). Peak detection in sediment - charcoal records: impacts of alternative data analysis methods on fire-history interpretations. Int. J. Wildl. Fire. 19, 996. doi: 10.1071/WF09134
Higuera P. E., Peters M. E., Brubaker L. B., Gavin D. G. (2007). Understanding the origin and analysis of sediment-charcoal records with a simulation model. Quat. Sci. Rev. 26, 1790–1809. doi: 10.1016/j.quascirev.2007.03.010
Holly D. H., Wolff C. B., Hull S. H. (2022). “The struggle was real: on the end of the archaic on the island of newfoundland and labrador,” in The Far Northeast: 3000 BP to contact, Mercury Series Archaeology Paper. Eds. Holyoke K. R., Hrynick G. M. (University of Ottawa Press, Ottawa, Ontario), 23–47.
Hu F. S., Brubaker L. B., Gavin D. G., Higuera P. E., Lynch J. A., Rupp T. S., et al. (2006). How climate and vegetation influence the fire regime of the alaskan boreal biome: the holocene perspective. Mitig. Adapt. Strateg. Glob. Change 11, 829–846. doi: 10.1007/s11027-005-9015-4
Hughes P. D. M., Blundell A., Charman D. J., Bartlett S., Daniell J. R. G., Wojatschke A., et al. (2006). An 8500 cal. year multi-proxy climate record from a bog in eastern Newfoundland: contributions of meltwater discharge and solar forcing. Quat. Sci. Rev. 25, 1208–1227. doi: 10.1016/j.quascirev.2005.11.001
Ives J. W. (1977). Pollen separation of three north american birches. Arct. Alp. Res. 9, 73. doi: 10.2307/1550410
Johnstone J. F., Chapin F. S. (2006). Fire interval effects on successional trajectory in boreal forests of northwest Canada. Ecosystems 9, 268–277. doi: 10.1007/s10021-005-0061-2
Juggins S. (2022). rioja: Analysis of Quaternary Science Data. R package version (1.0-5). Available online at: https://cran.r-project.org/package=rioja (Accessed April 24, 2023).
Kautz M., Meddens A. J. H., Hall R. J., Arneth A. (2017). Biotic disturbances in Northern Hemisphere forests - a synthesis of recent data, uncertainties and implications for forest monitoring and modelling. Glob. Ecol. Biogeogr. 26, 533–552. doi: 10.1111/geb.12558
Kelly R., Chipman M. L., Higuera P. E., Stefanova I., Brubaker L. B., Hu F. S. (2013). Recent burning of boreal forests exceeds fire regime limits of the past 10,000 years. Proc. Natl. Acad. Sci. U. S. A. 110, 13055–13060. doi: 10.1073/pnas.1305069110
Kelly R. F., Higuera P. E., Barrett C. M., Hu F. S. (2011). A signal-to-noise index to quantify the potential for peak detection in sediment-charcoal records. Quat. Res. 75, 11–17. doi: 10.1016/j.yqres.2010.07.011
Lake N. F. (2022). Holocene fire history of Terra Nova National Park (MSc. thesis, Fredericton, NB: University of New Brunswick).
Lindbladh M., O’Connor R., Jacobson G. L. (2002). Morphometric analysis of pollen grains for paleoecological studies: classification of Picea from eastern North America. Am. J. Bot. 89, 1459–1467. doi: 10.3732/ajb.89.9.1459
Long C. J., Whitlock C., Bartlein P. J., Millspaugh S. H. (1998). A 9000-year fire history from the Oregon Coast Range, based on a high-resolution charcoal study. Can. J. For. Res. 28, 774–787. doi: 10.1139/x98-051
Lynch J. A., Clark J. S., Stocks B. J. (2004). Charcoal production, dispersal, and deposition from the Fort Providence experimental fire: Interpreting fire regimes from charcoal records in boreal forests. Can. J. For. Res. 34, 1642–1656. doi: 10.1139/X04-071
MacPherson J. B. (1995). A 6 ka BP reconstruction for the island of newfoundland from a synthesis of holocene lake-sediment pollen records. Géographie. Phys. Quat. 49, 163–182. doi: 10.7202/033035ar
Magne G., Brossier B., Gandouin E., Paradis L., Drobyshev I., Kryshen A., et al. (2020). Lacustrine charcoal peaks provide an accurate record of surface wildfires in a North European boreal forest. Holocene. 30, 380–388. doi: 10.1177/0959683619887420
Masson-Delmotte V., Schulz M., Abe-Ouchi A., Beer J., Ganopolski A., González Rouco J. F., et al. (2013). “Information from paleoclimate archives,” in Climate change 2013: The physical science basis. contribution of working group I to the fifth assessment report of the Intergovernmental Panel on Climate Change. Eds. Stocker T. F., Qin D., Plattner G. K., Tignor M., Allen S. K., Boschung J., Nauels A., Xia Y., Bex V., Midgley P. M. (Cambridge University Press, Cambridge), 383–464.
McAndrews J. H., Berti A. A., Norris G. (1973). Key to the quaternary pollen and spores of the Great Lakes region (Toronto, Ontario: Royal Ontario Museum).
Meades W. J., Moore L. (1994). Forest site classification manual: A guide to the Damman forest types of Newfoundland 2nd Edn. FRDA Report 003 (Corner Brook, Newfoundland and Labrador: Western Newfoundland Model Forest Inc).
Natural Resources and Forests (2023). Classification écologique du territoire Québécois//Ecological classification of Québec territory. Available online at: https://www.donneesquebec.ca/recherche/dataset/systeme-hierarchique-de-classification-ecologique-du-territoire (Accessed April 14, 2023).
Oris F., Ali A. A., Asselin H., Paradis L., Bergeron Y., Finsinger W. (2014a). Charcoal dispersion and deposition in boreal lakes from 3 years of monitoring: Differences between local and regional fires. Geophys. Res. Lett. 41, 6743–6752. doi: 10.1002/2014GL060984
Oris F., Asselin H., Finsinger W., Hély C., Blarquez O., Ferland M.-E., et al. (2014b). Long-term fire history in northern Quebec: implications for the northern limit of commercial forests. J. Appl. Ecol. 51, 675–683. doi: 10.1111/1365-2664.12240
Parks Canada (2019). Terra Nova National Park of Canada: Management Plan 2019 (Glovertown, NL: Parks Canada).
Parks Canada Agency (2000). Unimpaired for Future Generations”? Protecting Ecological Integrity Within Canada’s National Parks (Ottawa, ON: Canada’s National parks). Available at: https://publications.gc.ca/collections/Collection/R62-323-2000-1E.pdf.
Payette S., Morneau C., Sirois L., Desponts M. (1989). Recent fire history of the northern quebec biomes. Ecology 70, 656–673. doi: 10.2307/1940217
Pisaric M. F. J. (2002). Long-distance transport of terrestrial plant material by convection resulting from forest fires. J. Paleolimnol. 28, 349–354. doi: 10.1023/A:1021630017078
Power R. G. (1995). Seedbed micro-sites and their role in post-fire succession of the lichen-black spruce woodland in Terra Nova National Park, Newfoundland (MSc. thesis, St. Johns, NL: Memorial University of Newfoundland).
Power R. G. (1996). Forest fire history and vegetation analysis of Terra Nova National Park. Parks Canada technical reports in ecosystem Science (Halifax, NS: Parks Canada, Atlantic Region).
Prince T. J., Pisaric M. F. J., Turner K. W. (2018). Postglacial reconstruction of fire history using Sedimentary charcoal and pollen from a small lake in southwest Yukon Territory, Canada. Front. Ecol. Evol. 6. doi: 10.3389/fevo.2018.00209
Reimer P. J., Austin W. E. N., Bard E., Bayliss A., Blackwell P. G., Bronk Ramsey C., et al. (2020). The IntCal20 Northern Hemisphere Radiocarbon Age Calibration Curve (0-55 cal kBP). Radiocarbon 62, 725–757. doi: 10.1017/RDC.2020.41
Remy C. C., Hély C., Blarquez O., Magnan G., Bergeron Y., Lavoie M., et al. (2017b). Different regional climatic drivers of Holocene large wildfires in boreal forests of northeastern America. Environ. Res. Lett. 12, 035005. doi: 10.1088/1748-9326/aa5aff
Remy C. C., Lavoie M., Girardin M. P., Hély C., Bergeron Y., Grondin P., et al. (2017a). Wildfire size alters long-term vegetation trajectories in boreal forests of eastern North America. J. Biogeogr. 44, 1268–1279. doi: 10.1111/jbi.12921
Richard P. J. H. (1970). Atlas pollinique des arbres et de quelques arbustes indigènes du Québec. Le. Naturaliste. Canadienne. 97, 1–34, 97-161, 241-306.
Rosenburg S. M., Walker I. R., Macpherson J. B. (2005). Environmental Changes at Port au Choix as Reconstructed from Fossil Midges. Newfoundl. Labrador. Stud. 20, 57–73.
Simpson M. (2007). Fire Management Plan for Terra Nova National Park of Canada: 2007-2017 (Glovertown, NL: Parks Canada).
Sirois L., Payette S. (1991). Reduced postfire tree regeneration along A boreal forest-forest-tundra transect in northern quebec. Ecology 72, 619–627. doi: 10.2307/2937202
Sparks G. W., Dunning G. R. (2014). Late Neoproterozoic Epithermal Alteration and Mineralization in the Western Avalon Zone: A Summary of Mineralogical Investigations and New U/Pb Geochronological Results (St. John’s, NL: Newfoundland and Labrador Department of Natural Resources Geological Survey Division). Available at: https://www.gov.nl.ca/iet/files/mines-geoscience-publications-currentresearch-2014-sparkes-2014.pdf.
Statistics Canada (2021). 2021 census boundary files. Available online at: https://www12.statcan.gc.ca/census-recensement/alternative_alternatif.cfm?l=eng&dispext=zip&teng=lpr_000b21a_e.zip&k=%20%20%20130596&loc=//www12.statcan.gc.ca/census-recensement/2021/geo/sip-pis/boundary-limites/files-fichiers/lpr_000b21a_e.zip (Accessed June 21, 2024).
Tcheumeleu A. F., Millet L., Rius D., Ali A. A., Bergeron Y., Grondin P., et al. (2023). An 8500-year history of climate-fire-vegetation interactions in the eastern maritime black spruce–moss bioclimatic domain, Québec, Canada. Écoscience 00, 1–17. doi: 10.1080/11956860.2023.2292354
Van Geel B., Heusser C. J., Renssen H., Schuurmans C. J. E. (2000). Climatic change in Chile at around 2700 BP and global evidence for solar forcing: A hypothesis. Holocene 10, 659–664. doi: 10.1191/09596830094908
Walsh M. K., Whitlock C., Bartlein P. J. (2010). 1200 years of fire and vegetation history in the Willamette Valley, Oregon and Washington, reconstructed using high-resolution macroscopic charcoal and pollen analysis. Palaeogeogr. Palaeoclimatol. Palaeoecol. 297, 273–289. doi: 10.1016/j.palaeo.2010.08.007
Whitman E., Parisien M. A., Thompson D. K., Flannigan M. D. (2019). Short-interval wildfire and drought overwhelm boreal forest resilience. Sci. Rep. 9, 1–12. doi: 10.1038/s41598-019-55036-7
Wright H. E. (1967). A square-rod piston sampler for lake sediments. J. Sediment. Res. 37, 975–976. doi: 10.1306/74d71807-2b21-11d7-8648000102c1865d
Keywords: fire return interval, Holocene, pollen analysis, charcoal analysis, Terra Nova National Park, fire frequency, climate, Newfoundland
Citation: Lake NF, Arsenault A and Cwynar LC (2024) A Holocene fire history from Terra Nova National Park, Newfoundland, Canada: vegetation and climate change both influenced the fire regime. Front. Ecol. Evol. 12:1419121. doi: 10.3389/fevo.2024.1419121
Received: 17 April 2024; Accepted: 08 August 2024;
Published: 19 September 2024.
Edited by:
Gael Le Roux, UMR5245 Laboratoire Ecologie Fonctionnelle et Environnement (ECOLAB), FranceReviewed by:
Debra Willard, United States Geological Survey (USGS), United StatesAdam A. Ali, Université de Montpellier, France
Copyright © 2024 Lake, Arsenault and Cwynar. This is an open-access article distributed under the terms of the Creative Commons Attribution License (CC BY). The use, distribution or reproduction in other forums is permitted, provided the original author(s) and the copyright owner(s) are credited and that the original publication in this journal is cited, in accordance with accepted academic practice. No use, distribution or reproduction is permitted which does not comply with these terms.
*Correspondence: Nickolas F. Lake, Tmlja29sYXMubGFrZUB1bmIuY2E=