- 1Haub School of Environment and Natural Resources, Wyoming Cooperative Fish and Wildlife Research Unit, Department of Zoology and Physiology, University of Wyoming, Laramie, WY, United States
- 2California Department of Fish and Wildlife, Sierra Nevada Bighorn Sheep Recovery Program, Bishop, CA, United States
Group living has well-known costs and benefits. Large groups may experience greater competition for resources, while simultaneously benefit from reduced risk through predator dilution. When there is a tradeoff between forage acquisition and predation risk, the ability to congregate into large groups may unlock access to previously unavailable habitat with high risk of predation, thereby increasing forage available to the population. We evaluated whether forage availability increased with population size and how it was mediated through changes in group size. There was a tradeoff between forage availability and predation risk. Larger groups used areas with more forage biomass and greater predation risk than smaller groups. Group size also increased with population abundance, meaning bighorn sheep used gentler terrain and areas with more forage biomass at greater population abundance. Group size functionally increased carrying capacity by yielding access to more resources for growing populations of gregarious ungulates.
1 Introduction
To enhance fitness, living organisms must acquire resources, while simultaneously avoiding mortality risk to themselves and their offspring (Moran et al., 2021). Given the diversity of life forms, there is no one single strategy to maximize fitness; rather, a suite of environmental factors–which are constantly changing–determine the viability of life-history strategies at any one time and place (Acasuso-Rivero et al., 2019). Although certain aspects of animal behavior are thought to be canalized traits to some degree (e.g., boldness), other aspects of behavior are plastic (Bell et al., 2009; Ducatez et al., 2020). Plasticity in behavior often is the first mechanism animals employ to balance tradeoffs in resource acquisition and mortality risk as environmental conditions change (Ducatez et al., 2020; Moran et al., 2021).
One behavior that can increase fitness is group living (Hamilton, 1971). According to selfish herd theory, risk of predation decreases with increasing group (i.e., herd) size (Hamilton, 1971). Collective detection of predators increases with group size, allowing each animal to devote more time to resource acquisition (e.g., foraging) and less time to vigilance behavior (Pulliam, 1973). Once a threat has been detected, many group-living animals provide conspicuous cues alerting their conspecifics to the presence of danger (Pulliam, 1973; Smith, 1991). The alarm calls in capuchin monkeys (Cebus apella) which alerts other troop members to the specific type (i.e., feline, snake, or raptor) of danger detected, is one example (Wheeler, 2008). The positive benefits derived from group living continue after detection of danger in the form of diluted risk of predation and smaller domain of danger (Hamilton, 1971; van Deventer and Shrader, 2021). Only animals on the outside perimeter of a tightly formed group are accessible to predators, whereas animals scattered across the landscape lack the safety provided at the center of a group (van Deventer and Shrader, 2021). Lastly, residing in a group of conspecifics provides animals with cues about the distribution of food resources allowing them to spend less time searching and more time handling food items (Caraco and Giraldeau, 1991).
Although group living comes with several positive attributes, there are also downsides to living in a group (Manlove et al., 2014; Markham et al., 2015). Indeed, competition for resources among conspecifics is a common phenomenon that produces negative density-dependent responses in almost all facets of biology (Clutton-Brock et al., 1987; Kvalnes et al., 2022). Classic examples of density-dependence, often focus on the 3 central tenets of biology–survival, growth, and reproduction (Clutton-Brock et al., 1987). When density-dependent feedback, mediated through resource competition, affects survival and reproduction, it establishes an upper limit on population growth, a concept called nutritional carrying capacity (McCullough, 1979).
Although both positive (selfish herd theory) and negative (density-dependent competition for resources) attributes of group living are common themes in biology, the combined influence of both on nutritional carrying capacity is an underexplored topic. Habitat selection can be a density-dependent process (van Beest et al., 2014). According to the ideal free distribution theory, animals should be forced to use lower quality habitat as conspecific density increases (Smith et al., 2023). Habitat quality, however, is a multifaceted metric (Morrison et al., 2006); rarely can ideal elements of predation and parasite risk, or food quality and abundance, be achieved without balancing tradeoffs among them (Becker et al., 2021; Smith et al., 2023). Therefore, there is an opportunity for animals to balance the tradeoffs between different components of habitat quality with changes in behavior (Becker et al., 2021). As population size increases, it is easier for animals to aggregate into large groups (Mooring et al., 2004; McLellan et al., 2010). In turn, large groups, which have lowered per capita risk of predation, potentially leads to animals venturing into previously unoccupied areas that provide high reward, in the form of plentiful resources, but come at an elevated risk of mortality (Schroeder et al., 2010). By altering group size, per capita risk may remain unchanged while the group accesses more forage resources; thus, expanding the resources available to the population and functionally increasing nutritional carrying capacity. Although theory suggests that sociality, in the form of group size, should hold consequences for the realized measure of nutritional carrying capacity, little consideration has been given to the implications of this phenomenon.
Bighorn sheep (Ovis canadensis) provide an ideal organism to evaluate hypotheses pertaining to density-dependent changes in nutritional carrying capacity. Bighorn sheep inhabit rugged terrain throughout western North America and seek refuge in steep terrain (i.e., escape terrain) when frightened, even in the absence of coursing predators (Mckinney et al., 2006; Schroeder et al., 2010). Slope gradient, or terrain steepness is, thus, an easily defined metric of safety that may force a tradeoff with forage availability, because bighorn sheep live in resource depauperate environments with little to no forage. Bighorn sheep live in variable group sizes (Schroeder et al., 2010), and may exhibit density-dependent changes in group size that would in turn, mediate risk of predation and increase their willingness to venture into risky terrain with more forage.
We evaluated the hypothesis that for group-living ungulates, changes in sociality, or group size, as a population grows will alter habitat use providing animals with access to more forage, functionally increasing the carrying capacity of an area. We developed 4 testable predictions stemming from our hypothesis: 1) herbaceous biomass and slope angle exhibit a negative relationship creating a tradeoff between safety and forage acquisition; 2) group size is related positively to population abundance; 3) bighorn sheep use gentler terrain with greater forage availability when in larger groups; 4) bighorn sheep lessen their avoidance of high-risk areas with more forage resources as population size grows.
2 Materials and methods
2.1 Study area
We studied Sierra Nevada bighorn sheep (O. canadensis sierrae), a federally endangered subspecies, in the Sierra Nevada mountain range (37°24’N, 118°41’W), located in California, USA. Within the Sierra Nevada, bighorn sheep occurred primarily along the Sierra Crest and Great Western Divide. The Great Western Divide was a secondary mountain range within the Sierra Nevada with peaks >4,000 m in elevation and was separated from the Sierra Crest by the Kern River. The eastern side of the Sierra Nevada was characterized by an abrupt change in elevation quickly increasing from ~1,000 m above sea level in the Owens Valley to peaks >4,000 m and passes of >3,000 m along the Sierra Crest (Forshee et al., 2022). The western slopes of the Sierra Crest and Great Western Divide had gentler slopes (2-5%) with more abundant and dense vegetation and forest cover (Monteith et al., 2014). Bighorn sheep inhabited a mixture of alpine areas, xeric slopes, and canyon slopes along rivers and creeks. Much of the topography was created during the Pleistocene glacial period, resulting in the numerous waterfalls, cirques, and U-shaped valleys in the area (Wehausen, 1980). The crest of the Sierra Nevada mountains created a rain shadow and most precipitation east of the crest was received as snow between November and April (Johnson et al., 2010). Much of the eastern escarpment was covered in talus fields and steep exposed granite slabs which were largely devoid of vegetation.
Flora of the region largely followed elevation gradients. Low elevation vegetation communities were characterized by shrub dominated communities, with species such as big sagebrush (Artemisia tridentata) and bitterbrush (Purshia tridentata) being common (Schroeder et al., 2010). Mid-elevations with sufficient soil depths were dominated by subalpine forest (Wehausen, 1980). High-elevation areas were dominated by alpine vegetation including various sedges (Carex spp.) and willows (Salix spp.; Schroeder et al., 2010). Water birch (Betula occidentalis) and white fir (Abies concolor) were common along the mountain streams found in canyon bottoms. Fauna that regularly interacted with bighorn sheep in the Sierra Nevada included mule deer (Odocoileus hemionus), mountain lions (Puma concolor), coyotes (Canis latrans), bobcats (Lynx rufus), black bears (Ursus americanus), and golden eagles (Aquila chrysaetos).
2.2 Data collection
We used data on group size, population size, locations of bighorn sheep from global positioning system (GPS) collars, locations from field necropsies of bighorn sheep killed by mountain lions, annual herbaceous biomass, and slope angle for our analyses. Personnel with the California Department of Fish and Wildlife have recorded group size and composition of Sierra Nevada bighorn sheep as part of ongoing recovery and monitoring efforts for >20 years. Although many observations of group size and composition were associated with population surveys, any visual observation of bighorn sheep, incidental or otherwise, was recorded. We used minimum counts of female bighorn sheep as an index of population size. We obtained minimum counts from ground-based surveys conducted by systematically hiking through herd units (defined by the United States Fish and Wildlife Service for the purpose of subspecies recovery as geographically distinct populations with minimal immigration and emigration; U.S. Fish and Wildlife Service, 2007; Conner et al., 2018) and scanning for bighorn sheep with binoculars and spotting scopes (Johnson et al., 2010). We used radio telemetry to aid in survey efforts, because of logistical constraints associated with monitoring endangered ungulates (Clement et al., 2022). Survey efforts were focused on the female segment of the population, because adult females were responsible for population dynamics and recovery goals tied to legal protection were, therefore, based on minimum counts of female bighorn sheep (U.S. Fish and Wildlife Service, 2007; Johnson et al., 2010).
We used location data from GPS collars deployed as part of ongoing recovery and monitoring efforts. Collars were of various makes (Advanced Telemetry Solutions, Isanti MN, USA; Lotek, Newmarket, ON, Canada; North Star Science and Technology, Oakton, VA, USA; SirTrack, Hawkes Bay, New Zealand; Telemetry Solutions, Concord, CA, USA; Televilt, Lindesberg, Sweden; Vectronics Aerospace, Berlin, Germany) and models. Our GPS collar data was collected for many objectives since 2004 and as a result we had numerous fix schedules to work with. We removed erroneous GPS collar locations when the absolute value of the turning angle created by 3 consecutive locations was >3 radians, the distance between sequential points was >3 standard deviations from the mean distance between sequential points, and the value of the distance between sequential points divided by the following step length was <0.9 (Villepique et al., 2008; Glass et al., 2022). We also removed GPS locations with a dilution of precision ≥10 (Glass et al., 2022). We only used GPS collar locations collected between sunrise and sunset, as astronomically defined, because we were interested in tradeoffs in habitat selection while bighorn sheep were foraging, rather than general habitat selection. Bighorn sheep research was approved under University of Montana Institutional Animal Care and Use Committee (024-07MHWB-071807 and 46-11), California Department of Fish and Wildlife Animal Welfare Policy (Sierra Nevada Bighorn Sheep Capture Plan 2006-10-2018-10), and United States Fish and Wildlife Service Recovery Permit TE050122-6.
We created a raster layer of slope angle with a spatial resolution of 10 m using a United States Geological Service digital elevation model (Horn, 1981). Bighorn sheep possess evolutionary adaptations that make steep terrain (often referred to as escape terrain) safer than gentler terrain for them (Geist, 1971). The use of gentler terrain has previously been shown to have increased predation risk for bighorn sheep (Jones et al., 2022). We ensured that the well-known relationship between terrain steepness (i.e., slope angle) and predation risk was true in our specific system, using locations from bighorn sheep killed by mountain lions. We used the equation:
derived from Bayesian logistic regression. We used the slope at locations of bighorn sheep killed by mountain lions as the successes and the average value of all locations obtained for each bighorn sheep wearing a GPS collar as the failures in our model.
To represent available forage, we summed above-ground herbaceous biomass with new stem growth and leaves of shrubs, because bighorn sheep consume diets ranging from >90% graminoids to >90% browse (Smith, 1992; Kissell, 1996). As our estimate of above-ground herbaceous biomass for each year, we used raster layers with a spatial resolution of 30 m available from the Rangeland Analysis Platform (Robinson et al., 2019; Jones et al., 2021). We estimated annual biomass of shrub leaf and new stem growth rasters with a 30 m spatial resolution using predictive equations based on crown volume measurements (Cleary et al., 2008). We calculated crown volume of shrubs by multiplying crown area rasters (i.e., annual shrub cover downloaded from the Rangeland Analysis Platform; Robinson et al., 2019; Jones et al., 2021) by a shrub height raster from the National Land Cover Database (Xian et al., 2015; Rigge et al., 2020).
2.3 Statistical analyses
To test our prediction that forage biomass decreased with increasing slope angle, which would create a tradeoff between safety and resource acquisition for bighorn sheep, we regressed herbaceous biomass against slope. We extracted paired slope and forage biomass values from our slope and biomass raster layers at 100,000 random points. We determined the relationship between slope and herbaceous biomass using a Bayesian Tweedie generalized linear model, implemented within the R package cplm (Zhang, 2013), with a log link function with the form:
We used the non-informative priors: βj~N(0, 10,000), ϕ~U(0, 100), p~U(1.01, 1.99), and σb2~Inv-Ga(0.001, 0.001) (Zhang, 2013). We estimated parameters using 3 chains of 110,000 draws each with an initial burn-in period of 10,000 draws. We retained every tenth draw for a sample of 10,000 draws per chain.
We regressed group size against the minimum count of female bighorn sheep using a generalized linear mixed model with a negative binomial distribution of errors in a Bayesian framework, to test our prediction that group size increased with increasing bighorn sheep abundance. We paired group size observations with annual population indices across 14 herd units for our analysis. We included a crossed random intercept term with unstructured variance-covariance matrices for year and herd unit in our model to account for possible serial autocorrelation and non-independence of observations. Our model had the following form:
We used non-informative priors βj~N(0, 1,000,000) and σbj2~N(0, 1,000,000) for our model, so our data would largely dictate model parameter estimation. We estimated parameters using 3 chains of 105,000 draws each with an initial burn-in period of 5,000 draws. We retained every tenth draw for a sample of 10,000 draws per chain.
To test our prediction that bighorn sheep increase their use of gentler terrain and areas with more forage with increasing group size, we paired bighorn sheep locations of known group size with remotely sensed data on forage biomass and slope. We used locations obtained from GPS collars within 48-hours from when a marked bighorn sheep was recorded as part of a group observation to extract the corresponding slope and herbaceous biomass values from our raster layers. We implemented a Bayesian linear mixed-effects model with the following form:
to assess how group size affected the slope of used locations. To account for non-independence in repeated observations of the same bighorn sheep, we included a random intercept term with an unstructured variance-covariance matrix in our model. We specified the non-informative priors βj~N(0, 1,000,000) and σb2~N(0, 1,000,000) for our model. To describe the relationship between group size and herbaceous biomass of locations used by bighorn sheep we used a Bayesian Tweedie generalized linear mixed-effects model with a log link function with the form:
Tweedie generalized linear models are well suited for zero-inflated continuous data, such as biomass data encountered in many ecological settings (Lecomte et al., 2013). We included a random intercept term with an unstructured variance-covariance matrix in our model to account for non-independence in repeated observations of the same bighorn sheep. We implemented our model using an MCMC procedure using the random walk Metropolis-Hastings algorithm in the R package cplm (Zhang, 2013). We used the non-informative priors: βj~N(0, 10,000), ϕ~U(0, 100), p~U(1.01, 1.99), and σb2~Inv-Ga(0.001, 0.001) (Zhang, 2013). For both models, we estimated parameters using 3 chains of 105,000 draws each with an initial burn-in period of 5,000 draws. We retained every tenth draw for a sample of 10,000 draws per chain.
Nutritional carrying capacity is the number of animals that the nutritional resources in an area can support (Robbins, 1973; DeYoung et al., 2000; Monteith et al., 2014; Smythe et al., 2019). Though we did not estimate nutritional carrying capacity directly, we approximated the proportional change in nutritional carrying capacity for Sierra Nevada bighorn sheep across the range of group sizes we observed. To estimate nutritional carrying capacity we used the range supply method constrained by habitat (e.g., terrain steepness; Beck et al., 2006). We summed the total amount of estimated biomass for all areas within the 14 herd units for all areas with a terrain steepness greater than or equal to the average terrain steepness used by collared bighorn sheep at the desired group size. We were only interested in the magnitude of change in nutritional carrying capacity, thus, there was no need to convert total forage biomass values into a different unit of measurement (e.g., Animal Unit Month, total bighorn sheep, etc.).
To test our prediction that bighorn sheep will increase use of gentle terrain and areas with high forage biomass with increasing conspecific density, we used a combination of resource selection analysis and Bayesian linear regression. We analyzed winter (October–April) and summer (May–September) data separately to account for potential differences in seasonal habitat use. We used a binomial generalized linear model for each animal and year combination with fixed effects terms for herbaceous biomass and slope, based on data from our slope and biomass raster layers. We coded used GPS collar locations from collared bighorn sheep as successes (1) and a random sample of available locations within a 95% minimum convex polygon drawn around all GPS collar locations for a given herd unit as failures (0). We used a ratio of 100 available locations to each used location, because diagnostic plots showed that this sampling ratio accurately captured the variation in availability. Beta coefficients for slope and herbaceous biomass from each binomial generalized linear model were used to exponentiate Euler’s number to yield a relative selection coefficient. We averaged repeated measures of the same bighorn sheep, when present, to account for serial autocorrelation. Additionally, we removed one outlier that was >3.5 standard deviations from the next closest observation. We then used the relative selection coefficients as the response variable in a Bayesian general linear model to test if selection for gentler terrain and higher forage biomass was related positively to conspecific abundance. We included availability of slope, or biomass as appropriate, as a fixed effect term in our models, to account for functional responses in habitat selection, that would have otherwise masked the relationship between population size and selection strength. We specified the non-informative priors βj~N(0, 1,000,000) and σb2~N(0, 1,000,000) for our models. For both models, we estimated parameters using 3 chains of 105,000 draws each. Our models had an initial burn-in period of 5,000 draws and we retained every tenth draw for a sample of 10,000 draws per chain. Our model to test if selection for gentler terrain was related negatively to population abundance had the following form:
and our model testing if selection for higher forage biomass was related positively to population abundance had the following form:
We surmised that models had converged properly, if trace plots showed even mixing of the chains, all parameters had unimodal posterior distributions, and the Gelman-Rubin convergence diagnostic was <1.1 (Gelman and Rubin, 1992; Brooks and Gelman, 1997). To provide readers with an easily interpretable metric of confidence, we report probability of direction values, which is simply the probability that the relationship between an independent and dependent variable is positive or negative. We used the R package brms to run all models, unless otherwise noted, via a Hamiltonian Monte Carlo algorithm within Stan (version 2.26.1; Bürkner, 2017). We ran all statistical analyses in the R computing environment (version 4.2.1) and QGIS (version 3.16.5).
3 Results
Estimated forage biomass was related negatively to terrain steepness across the 14 herd units that Sierra Nevada bighorn sheep inhabit (n = 100,000, Probability of Direction = 100%). Mean estimated forage biomass ranged from 18 kg/ha at a terrain steepness of 78 degrees to 179 kg/ha on level ground–a 10-fold increase in forage with a concomitant 30-fold increase in predicted predation risk (n = 804, Probability of Direction = 100%). Group size increased with number of female bighorn sheep (a proxy for population size; n = 6,459, Probability of Direction = 100%). Mean group size grew from 6 at a minimum count of 1 female bighorn sheep to 10 at a minimum count of 55 females.
Steepness of terrain used by bighorn sheep decreased by 0.02 degrees for each additional sheep in the group (n = 10,485, Probability of Direction = 95.03%, Figure 1), thereby increasing useable area by 9% and the nutritional carrying capacity by 13% (Figure 2). Furthermore, estimated forage biomass at locations used by bighorn sheep increased from 38 kg/ha at a group size of 1 to 52 kg/ha at a group size of 65 (n = 10,485, Probability of Direction = 100%, Figure 1). Lastly, the expansion in habitat used, along with changes in group size as population abundance grew (indexed using minimum counts of female bighorn sheep), resulted in a change in habitat selection (Figure 2). A combination of resource selection analyses and Bayesian general linear regression revealed that relative strength of selection for steep terrain by bighorn sheep was related negatively to population abundance in both winter (n = 344, β = -0.006, Probability of Direction = 99.30%) and summer (n = 262, β = -0.011, Probability of Direction = 99.99%, Figure 3). Relative strength of selection for estimated forage biomass by bighorn sheep was related positively to population abundance during the winter (n = 344, β = 0.004, Probability of Direction = 99.13%), but not during the summer (n = 262, β = -0.001, Probability of Direction = 68.63%, Figure 3).
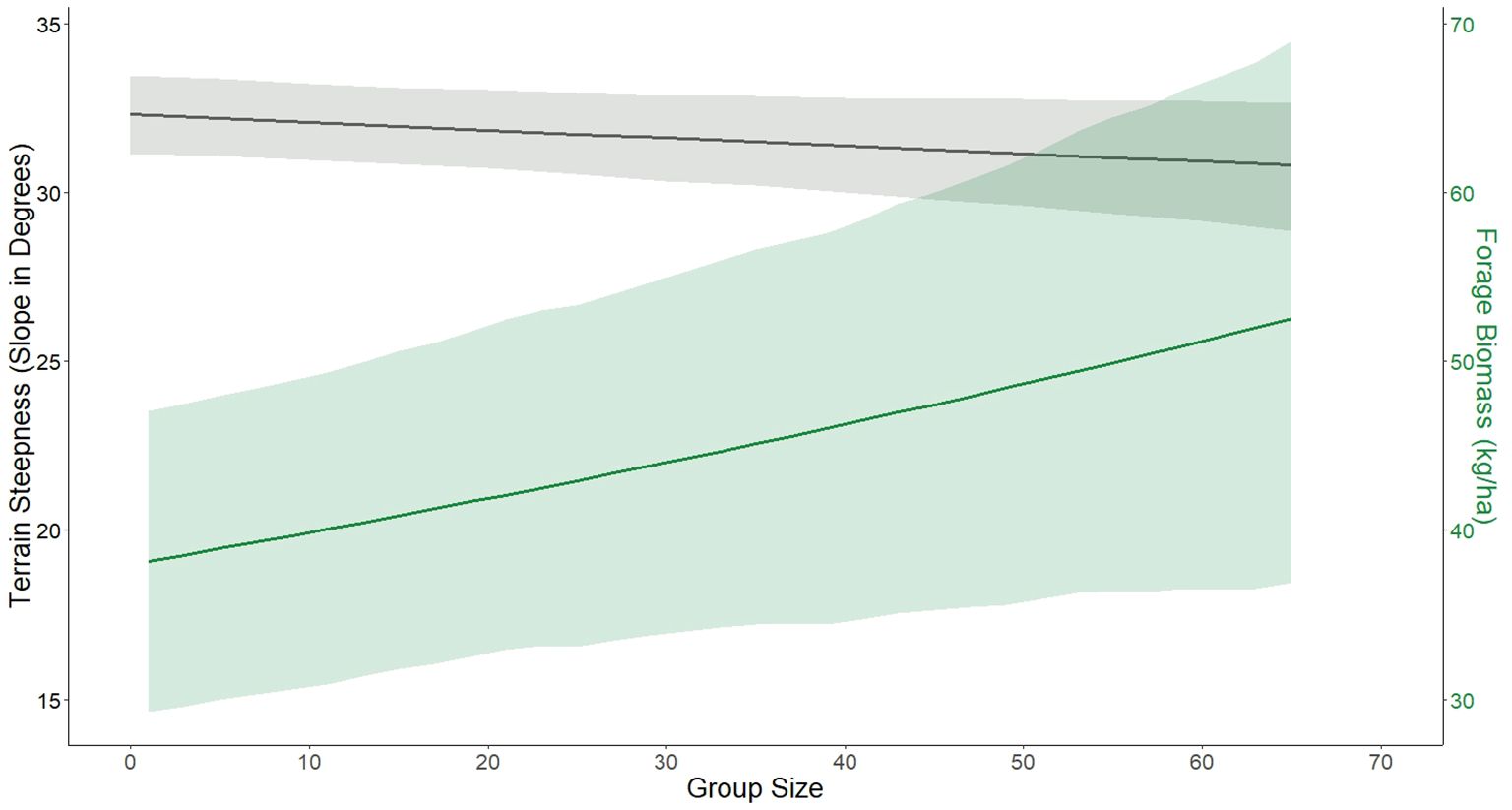
Figure 1. GPS collared bighorn sheep in the Sierra Nevada mountains of California, USA, increased their use of risky areas (quantified by terrain steepness with less steep terrain being riskier; black) with more forage (green) as group size grew larger. Lines represent estimated marginal mean prediction (± 95% CI) from a Bayesian linear mixed-effects model (black; terrain steepness – n = 10,485, Probability of Direction = 95.03%) and a Bayesian Tweedie generalized linear mixed-effects model (green; forage biomass – n = 10,485, Probability of Direction = 100%).
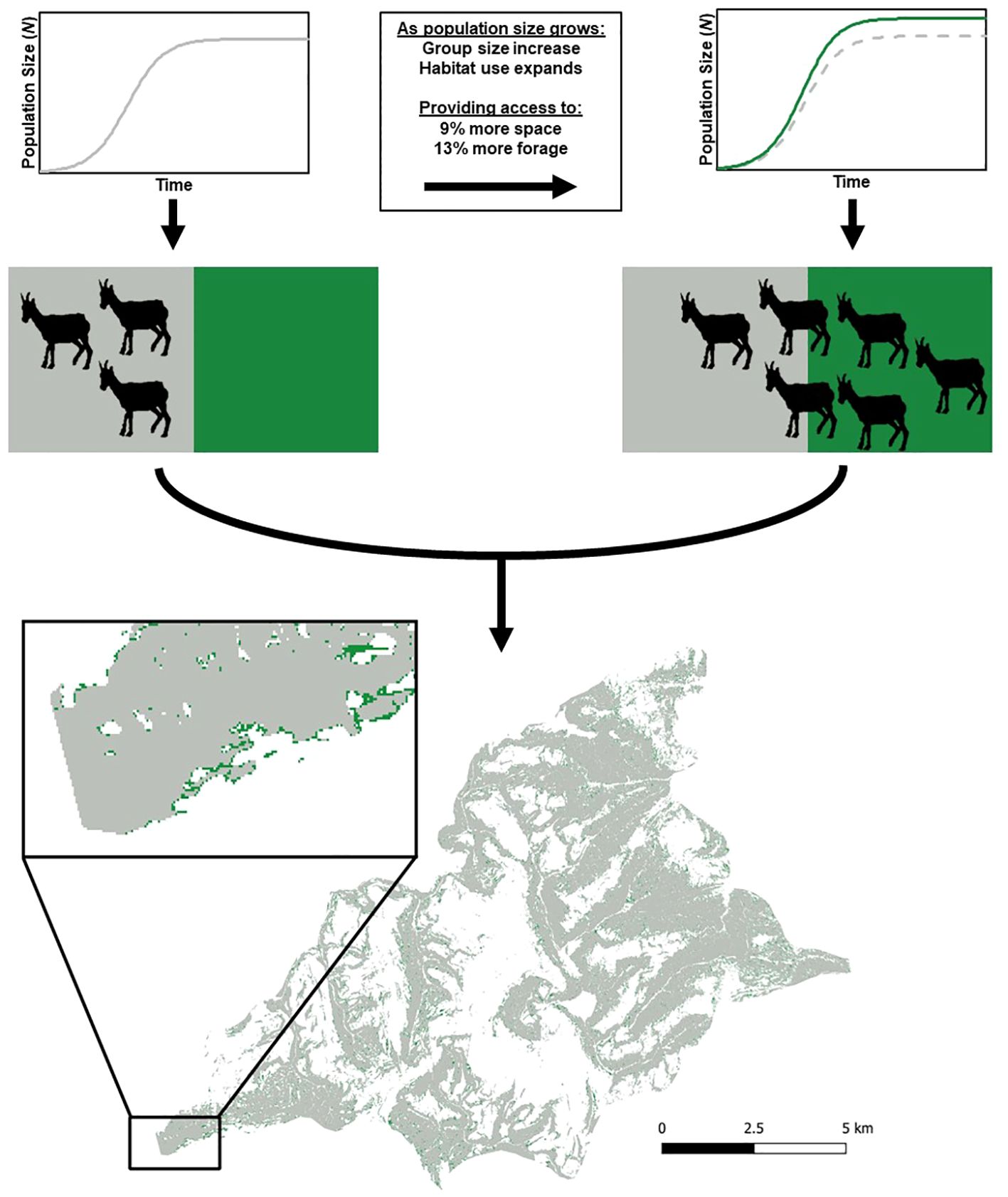
Figure 2. There was a tradeoff between safety and forage resources, which allowed bighorn sheep habitat use to expand (from gray to both green and gray areas) as population size increased and was mediated through changes in group size. The ability to expand into the areas depicted in green increased the useable space by 9% but came with a 13% increase in the amount of forage, because of the exponential relationship between safety and forage on the landscape. Results are a synthesis of the multiple Bayesian regression analyses. The example map at bottom was the Sawmill Canyon Herd Unit, which was 1 of 14 herd units included in the analyses and was in California, USA.
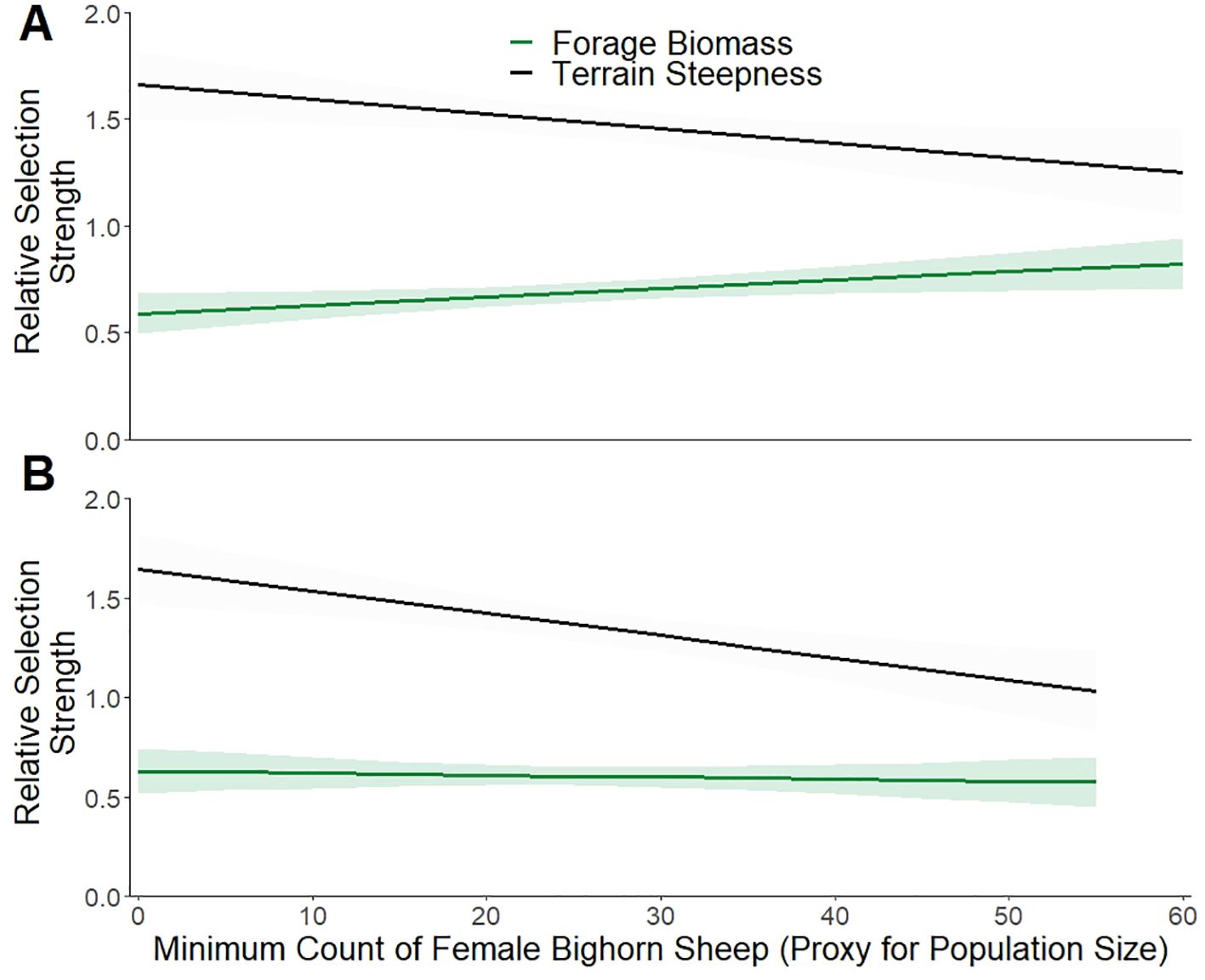
Figure 3. Relative selection strength for areas with high risk (quantified by terrain steepness; black; n = 344, β = -0.006, Probability of Direction = 99.30%) and plentiful forage resources (green; n = 344, β = 0.004, Probability of Direction = 99.13%) by bighorn sheep increased as population size grew in the winter (A). In the summer (B) relative selection strength for areas with high risk (black; n = 262, β = -0.011, Probability of Direction = 99.99%) increased as population size grew, but there was no change in relative selection strength for forage biomass (green; n = 262, β = -0.001, Probability of Direction = 68.63%). Lines represent estimated marginal mean predictions (± 95% CI) from Bayesian linear mixed-effects models. Resource selection analyses used data from GPS collared Sierra Nevada bighorn sheep collected between 2004 and 2021 inhabiting California, USA.
4 Discussion
Habitat is defined as the components of the environment that provide animals with the food, water, cover, and environmental conditions that are necessary for survival, growth, and reproduction (Morrison et al., 2006). The components of habitat also must be accessible to animals, rather than simply present on the landscape (Dwinnell et al., 2019); both risk and sociality interact with the abiotic landscape to shape the habitat that is functionally available for an animal (Figure 1). Risk may alter habitat use in diverse taxa ranging from mountain lions to brown anoles (Anolis sagrei) and have fitness costs linked to energy and mate acquisition (Steinberg et al., 2014; Nickel et al., 2021). Response to real or perceived risk (i.e., fear) can affect areas that animals are willing to use on the landscape (Brown et al., 1999). Although risk altered habitat use of bighorn sheep, they use group size to modulate the risk. Thus, changes in sociality, which often has been ignored in the context of wildlife management and conservation, affects where group-living animals, such as ungulates (Figure 1), primates (Eppley et al., 2022), and fishes (Orpwood et al., 2008), will forage. Reductions in group size associated with declines in abundance can reduce the amount of useable space and consequently, render previously useable habitat functionally unavailable with potential implications for vital rates in small populations (Allee and Bowen, 1932). Behaviorally induced habitat loss (Figure 2) can hold insidious consequences as an underrecognized problem (Dwinnell et al., 2019), that may occur in protected areas frequently thought to be strongholds for endangered species conservation (Anderson et al., 2023).
The social context of nutritional carrying capacity, while having potentially large ramifications, remains underappreciated and rarely quantified. Nevertheless, changes in behavior may restrict habitat use, and therefore, functionally affect carrying capacity (Dwinnell et al., 2019). All habitat is not created equally and small changes in behavior can have outsized impacts on the carrying capacity of an area (Figure 2). Although there will always be an upper limit on population growth, the ability to exploit previously unavailable resources as a population grows and group sizes increase will dampen the effect of intraspecific competition for forage. Sociality in the form of group size modulating the amount of forage bighorn sheep have access to is a specific example of access to food resources being buffered by behavior, which is in line with the general theory outlined in the ecology of fear framework (Brown et al., 1999). Although we only examined how sociality reduces the negative consequences of density-dependent competition for food, we expect other behaviors, such as timing and propensity to migrate may also affect intraspecific competition for food (Mysterud et al., 2011). Our study was observational in nature; therefore, we were unable to determine if bighorn sheep used high risk and high reward habitat because they could form large groups, or if they grouped together to use riskier habitat and alleviate competition. Regardless of the underlying cause, the positive relationships among population abundance, group size, and increased access to forage have the same impact on carrying capacity and should apply to terrestrial (Figure 2), arboreal (Eppley et al., 2022), and aquatic (Orpwood et al., 2008) group-living animals.
The finding that group size, and thereby population size, modulate habitat use in group-living ungulates holds potential insight into understanding the conundrum that exists between historical reports and more recent research on the distribution and habitat use of bighorn sheep throughout western North America. For example, historical reports from early European explorers, described groups of more than 1,000 bighorn sheep, that ventured into the plains surrounding steep terrain (Buechner, 1960). In contrast, modern research based on GPS collar locations indicates bighorn sheep are dependent on steep terrain (Lula et al., 2020; Forshee et al., 2022). A conundrum that has led some biologists to suggest that some now extinct subspecies of bighorn sheep had phenotypic differences more suitable for gentler terrain (Buechner, 1960). Alternatively, we suggest differences in habitat use by bighorn sheep arise from declines in bighorn sheep populations since the onset of European colonization. Although estimates are imprecise, it is thought between 1,500,000 and 2,000,000 bighorn sheep inhabited North America around the year 1800, but rapidly declined to <20,000 in the United States around 1960 (Buechner, 1960; Whiting et al., 2023). Certainly, a change in population size large enough to create a dramatic shift in habitat use.
Bighorn sheep and their closest relatives, Dall’s sheep (O. dalli) and snow sheep (O. nivicola), have evolutionary adaptations for predator evasion in steep terrain with short vegetation (Geist, 1971). Predation is considered one of the most important factors shaping the realized niche of bighorn sheep in the wild (Mckinney et al., 2006; Lula et al., 2020). Like other sub-species of bighorn sheep (Jones et al., 2022), Sierra Nevada bighorn sheep were more likely to be preyed upon by mountain lions in gentler terrain compared with steep terrain. Mountain lions are currently the primary predator of adult Sierra Nevada bighorn sheep and bighorn sheep select habitat (e.g., steep escape terrain) where the relative probability of encountering mountain lions is lessened (Gammons et al., 2021; Forshee et al., 2022). Although the predator guild that Sierra Nevada bighorn sheep co-occur with now differ from the predators they evolved with (Zielinski et al., 2005; Dellinger et al., 2021; Mychajliw et al., 2024), we expect the relationship between steep terrain and predation risk would be even stronger if coursing predators (e.g., wolves) were still present in the system (Festa-Bianchet, 1991). Furthermore, bighorn sheep inhabiting areas that historically had the same predators found currently in our study system have thriving populations of bighorn sheep that rely on steep terrain for predator evasion (Carroll et al., 2006; Cox, 2021; Marcus et al., 2022; but see Rominger, 2018). Thus, we consider it reasonable to presume that bighorn sheep we studied possess similar physical adaptations and behaviors for predator avoidance to bighorn sheep in other locales and time periods, meaning the patterns we observed are likely broadly generalizable.
In accordance with selfish herd theory, predation risk is related negatively to group size (Hamilton, 1971; Bowyer et al., 2020). Consistent with that theory, areas with greater risk of predation by bighorn sheep were more likely to be used by larger groups than were safer places (Figure 1). Although group living comes with a cost to fitness through increased competition for limited resources and greater exposure to pathogenic organisms (Manlove et al., 2014; Markham et al., 2015), corresponding benefits through access to more forage and reductions in per capita risk of predation outweigh the costs in some scenarios. Theoretically, there should be an optimal group size that balances the cost and benefits of group living (Markham et al., 2015). Group sizes <5 represent a safety threshold for bighorn sheep, where increased vigilance, and changes in habitat use, are unable to compensate for increased predation risk (Mooring et al., 2004).
Fitness enhancing tradeoffs, including the tradeoff created by residing in a group, are hypothesized to be a mechanism through which genetic diversity and biodiversity are maintained (Darwin, 1859; Agrawal et al., 2010). Tradeoffs, such as the one between resource acquisition and safety, can give rise to divergent evolutionary stable strategies (Darwin, 1859; Agrawal et al., 2010). Bighorn sheep can exploit more space and access more forage when traveling in larger groups, as opposed to smaller groups (Figure 2). Some bighorn sheep remain in small groups, however, even at the largest population sizes we observed. Restricting their movements to steeper terrain allows bighorn sheep in small groups to lessen their exposure to predation risk and intraspecific competition for resources, but also comes at a cost to resource acquisition. Presumably, neither behavioral strategy produces consistently higher fitness, as both behaviors are common in populations of bighorn sheep and is potentially an example of divergent evolutionary stable strategies. Natural selection can only act on existing phenotypic variation; meaning natural variation in expressed traits, including behavior, are important for species persistence as environmental conditions change.
Herein we demonstrated that nutritional carrying capacity encompasses both the physical supply of forage and the access to said forage. Consequently, nutritional carrying capacity of group-living animals increases with animal abundance and is mediated through changes in group size and behavior (Figure 2). By expanding selfish herd theory to incorporate the tradeoff between resource acquisition and predation risk, we identified a behavioral paradigm that modulates access to forage and functionally increases the carrying capacity of an area as the population grows. The same behavior simultaneously restricts the suitable habitat of animals that live in groups in dwindling populations, further exacerbating the risk of extinction.
Data availability statement
The original contributions presented in the study are publicly available. This data can be found here: https://zenodo.org/records/10779205.
Ethics statement
The animal study was approved by University of Montana Institutional Animal Care and Use Committee (024-07MHWB-071807 and 46-11), California Department of Fish and Wildlife Animal Welfare Policy (Sierra Nevada Bighorn Sheep Capture Plan 2006-10-2018-10), and United States Fish and Wildlife Service Recovery Permit TE050122-6. The study was conducted in accordance with the local legislation and institutional requirements.
Author contributions
SR: Conceptualization, Data curation, Formal analysis, Investigation, Methodology, Software, Visualization, Writing – original draft, Writing – review & editing. TS: Conceptualization, Data curation, Funding acquisition, Investigation, Methodology, Project administration, Resources, Supervision, Writing – review & editing. KM: Conceptualization, Funding acquisition, Methodology, Resources, Writing – review & editing.
Funding
The author(s) declare financial support was received for the research, authorship, and/or publication of this article. Funding for data collection was provided by the Federal Assistance in Wildlife Restoration grants, Endangered Species Conservation and Recovery (Section 6) grants, California Department of Fish and Wildlife, California Wild Sheep Foundation, Yosemite Conservancy, Sierra Nevada Bighorn Sheep Foundation, Wild Sheep Foundation, and U.S. Fish and Wildlife Service.
Acknowledgments
We thank the staff and volunteers with the California Department of Fish and Wildlife for their assistance with data collection. In particular, We thank K. Anderson, V. Bleich, L. Bowermaster, D. Bowie, E. Brandell, M. Brown, G. Cadwallader, T. Calfee, G. Chadwick, K. Chang, J. Davis, M. Davis, V. Davis, T. Del Bosco, M. Dodd, K. Ellis, A. Ellsworth, J. Erlenbach, A. Evans, A. Feinberg, A. Few, G. Foote, S. Forshee, J. Fusaro, L. Gable, D. Gammons, D. German, T. Glenner, B. Gonzales, L. Greene, L. Harrison, B. J. Harvey, Hatfield, B. Haverstock, A. Howell, R. Ianniello, D. Jensen, H. Johnson, J. Johnson, P. Johnston, D. Jensen, M. Kane, M. Kiner, J. Kolek, L. Konde, A. Lawrence, J. Leary, J. Leonard Cahn, W. Livingston, W. Loeper, S. Maple, C. Massing, L. P. McGrath, Milan, S. Miller, S. Morano, M. Morrison, K. Nelson, C. Noear, B. O’Connor, J. Ostergard, E. Otto, A. Peet, B. Pierce, P. Prentice, B. Regan, D. Rivers, J. Runcie, C. Schroeder, Y. Shakeri, E. Shockley, E. Siemion, R. Spaulding, D. Spitz, A. Stephenson, S. Stock, A. Sturgill, B. Teagle, J. Villepique, J. Wehausen, J. Weissman and S. Yeager for data collection. We thank M. Breiling, S. DeJesus, G. Pope, J. Pope, G. Schales, M. Shelton and R. Swisher for assistance with capturing animals for collaring.
Conflict of interest
The authors declare that the research was conducted in the absence of any commercial or financial relationships that could be construed as a potential conflict of interest.
Publisher’s note
All claims expressed in this article are solely those of the authors and do not necessarily represent those of their affiliated organizations, or those of the publisher, the editors and the reviewers. Any product that may be evaluated in this article, or claim that may be made by its manufacturer, is not guaranteed or endorsed by the publisher.
References
Acasuso-Rivero C., Murren C. J., Schlichting C. D., Steiner U. K. (2019). Adaptive phenotypic plasticity for life-history and less fitness-related traits. Proc. R. Soc B: Biol. Sci. 286, 20190653. doi: 10.1098/rspb.2019.0653
Agrawal A. A., Conner J. K., Rasmann S. (2010). “Tradeoffs and negative correlations in evolutionary ecology,” in Evolution After Darwin: The First 150 Years. Eds. Bell M., Eanes W., Futuyma D., Levinton J. (Sinauer Associates, Sunderland), 243–268.
Allee W. C., Bowen E. S. (1932). Studies in animal aggregations: Mass protection against colloidal silver among goldfishes. J. Exp. Zool. 61, 185–207. doi: 10.1002/jez.1400610202
Anderson A. K., Waller J. S., Thornton D. H. (2023). Partial COVID-19 closure of a national park reveals negative influence of low-impact recreation on wildlife spatiotemporal ecology. Sci. Rep. 13, 687. doi: 10.1038/s41598-023-27670-9
Beck J. L., Peek J. M., Strand E. K. (2006). Estimates of elk summer range nutritional carrying capacity constrained by probabilities of habitat selection. J. Wildl. Manage. 70, 283–294. doi: 10.2193/0022-541X(2006)70[283:EOESRN]2.0.CO;2
Becker J. A., Hutchinson M. C., Potter A. B., Park S., Guyton J. A., Abernathy K., et al. (2021). Ecological and behavioral mechanisms of density-dependent habitat expansion in a recovering African ungulate population. Ecol. Monogr. 91, e01476. doi: 10.1002/ecm.1476
Bell A. M., Hankison S. J., Laskowski K. L. (2009). The repeatability of behaviour: a meta-analysis. Anim. Behav. 77, 771–783. doi: 10.1016/j.anbehav.2008.12.022
Bowyer R. T., McCullough D. R., Rachlow J. L., Ciuti S., Whiting J. C. (2020). Evolution of ungulate mating systems: Integrating social and environmental factors. Ecol. Evol. 10, 5160–5178. doi: 10.1002/ece3.6246
Brooks S. P., Gelman A. (1997). General methods for monitoring convergence of iterative simulations. J. Comput. Graph. Stat. 7, 434–455. doi: 10.1080/10618600.1998.10474787
Brown J. S., Laundré J. W., Gurung M. (1999). The ecology of fear: Optimal foraging, game theory, and trophic interactions. J. Mammal. 80, 285–399. doi: 10.2307/1383287
Buechner H. K. (1960). The bighorn sheep in the United States, its past, present, and future. Wildl. Monogr. 4, 1–174. Available at: https://about.jstor.org/terms.
Bürkner P. C. (2017). brms: An R package for Bayesian multilevel models using Stan. J. Stat. Software 80, 1–28. doi: 10.18637/jss.v080.i01
Caraco T., Giraldeau L.-A. (1991). Social foraging: Producing and scrounging in a stochastic environment. J. Theor. Biol. 153, 559–583. doi: 10.1016/S0022-5193(05)80156-0
Carroll C., Phillips M. K., Lopez-Gonzalez C. A., Schumaker N. H. (2006). Defining recovery goals and strategies for endangered species: The wolf as a case study. Bioscience 56, 25–37. doi: 10.1641/0006-3568(2006)056[0025:DRGASF]2.0.CO;2
Cleary M. B., Pendall E., Ewers B. E. (2008). Testing sagebrush allometric relationships across three fire chronosequences in Wyoming, USA. J. Arid. Environ. 72, 285–301. doi: 10.1016/j.jaridenv.2007.07.013
Clement M. J., Hervert J. J., Bright J. L. (2022). Telemetry-based aerial surveys for estimating abundance of sparse populations. Wildl. Soc Bull. 46, e1253. doi: 10.1002/wsb.1253
Clutton-Brock T. H., Major M., Albon S. D., Guinness F. E. (1987). Early development and population dynamics in red deer. I. Density-dependent effects on juvenile survival. J. Anim. Ecol. 56, 53–67. doi: 10.2307/4799
Conner M. M., Stephenson T. R., German D. W., Monteith K. L., Few A. P., Bair E. H. (2018). Survival analysis: Informing recovery of Sierra Nevada bighorn sheep. J. Wildl. Manage. 82, 1442–1458. doi: 10.1002/jwmg.21490
Cox M. (2021). “Status of bighorn sheep in Nevada 2019–2020,” in 2021 Desert Bighorn Council Transactions, vol. 56 . Eds. Cain J. W. III., Gonzalez C. E., 98–104. Online - Virtual. Available at: https://www.desertbighorncouncil.com/transactions/download-past-dbc-transactions/.
Darwin C. (1859). On the Origin of Species By Means of Natural Selection, or the Preservation of Favoured Races in the Struggle for Life (London: John Murray).
Dellinger J. A., Laudon K., Figura P. (2021). Summer diet of California’s recolonizing gray wolves. Calif. Fish Wildl. 107, 140–146. doi: 10.51492/cfwj.hwisi.1
DeYoung R. W., Hellgren E. C., Fulbright T. E., Robbins W. F., Humphreys I. D. (2000). Modeling nutritional carrying capacity for translocated desert bighorn sheep in Western Texas. Restor. Ecol. 8, 57–65. doi: 10.1046/j.1526-100x.2000.80066.x
Ducatez S., Sol D., Sayol F., Lefebvre L. (2020). Behavioural plasticity is associated with reduced extinction risk in birds. Nat. Ecol. Evol. 4, 788–793. doi: 10.1038/s41559-020-1168-8
Dwinnell S. P. H., Sawyer H., Randall J. E., Beck J. L., Forbey J. S., Fralick G. L., et al. (2019). Where to forage when afraid: Does perceived risk impair use of the foodscape? Ecol. Appl. 29, e01972. doi: 10.1002/eap.1972
Eppley T. M., Hoeks S., Chapman C. A., Ganzhorn J. U., Hall K., Owen M. A., et al. (2022). Factors influencing terrestriality in primates of the Americas and Madagascar. Proc. Nati. Acad. Sci. U.S.A. 119, e2121105119. doi: 10.1073/pnas
Festa-Bianchet M. (1991). The social system of bighorn sheep: grouping patterns, kinship and female dominance rank. Anim. Behav. 42, 71–82. doi: 10.1016/S0003-3472(05)80607-4
Forshee S. C., Mitchell M. S., Stephenson T. R. (2022). Predator avoidance influences selection of neonatal lambing habitat by Sierra Nevada bighorn sheep. J. Wildl. Manage. 86, e22311. doi: 10.1002/jwmg.22311
Gammons D. J., Davis J. L., German D. W., Denryter K., Wehausen J. D., Stephenson T. R. (2021). Predation impedes recovery of Sierra Nevada bighorn sheep. Calif. Fish Wildl. 107, 444–470. doi: 10.51492/cfwj.cesasi.27
Geist V. (1971). Mountain sheep: A study in behavior and evolution (Chicago: The University of Chicago Press).
Gelman A., Rubin D. B. (1992). Inference from iterative simulation using multiple sequences. Stat. Sci. 7, 457–511. doi: 10.1214/ss/1177011136
Glass D. M., Prentice P. R., Evans A. D., Schmitz O. J. (2022). Local differences in maximum temperature determine water use among desert bighorn sheep populations. J. Wildl. Manage. 86, e22313. doi: 10.1002/jwmg.22313
Hamilton W. D. (1971). Geometry for the selfish herd. J. Theor. Biol. 31, 295–311. doi: 10.1016/0022-5193(71)90189-5
Horn B. K. P. (1981). Hill shading and the reflectance map. Proc. IEEE 69, 14–47. doi: 10.1109/PROC.1981.11918
Johnson H. E., Mills L. S., Stephenson T. R., Wehausen J. D. (2010). Population-specific vital rate contributions influence management of an endangered ungulate. Ecol. Appl. 20, 1753–1765. doi: 10.1890/09-1107.1
Jones M. O., Robinson N. P., Naugle D. E., Maestas J. D., Reeves M. C., Lankston R. W., et al. (2021). Annual and 16-Day rangeland production estimates for the western United States. Rangel. Ecol. Manage. 77, 112–117. doi: 10.1016/j.rama.2021.04.003
Jones A. S., Rubin E. S., Clement M. J., Harding L. E., Mesler J. I. (2022). Desert bighorn sheep habitat selection, group size, and mountain lion predation risk. J. Wildl. Manage. 86, e22173. doi: 10.1002/jwmg.22173
Kissell J. R.E. (1996). Population dynamics, food habits, seasonal habitat use, and spatial relationships of bighorn sheep, mule deer, and feral horses in the Pryor Mountains, Montana/Wyoming (Bozeman: Montana State University).
Kvalnes T., Saether B. E., Engen S., Roulin A. (2022). Density-dependent selection and the maintenance of colour polymorphism in barn owls. Proc. R. Soc B: Biol. Sci. 289, 20220296. doi: 10.1098/rspb.2022.0296
Lecomte J. B., Benoît H. P., Ancelet S., Etienne M. P., Bel L., Parent E. (2013). Compound Poisson-gamma vs. delta-gamma to handle zero-inflated continuous data under a variable sampling volume. Methods Ecol. Evol. 4, 1159–1166. doi: 10.1111/2041-210X.12122
Lula E. S., Lowrey B., Proffitt K. M., Litt A. R., Cunningham J. A., Butler C. J., et al. (2020). Is habitat constraining bighorn sheep restoration? A Case study. J. Wildl. Manage. 84, 588–600. doi: 10.1002/jwmg.21823
Manlove K. R., Frances Cassirer E., Cross P. C., Plowright R. K., Hudson P. J. (2014). Costs and benefits of group living with disease: A case study of pneumonia in bighorn lambs (Ovis canadensis). Proc. R. Soc B: Biol. Sci. 281, 20142331. doi: 10.1098/rspb.2014.2331
Marcus W. A., Meacham J. E., Rodman A. W., Steingisser A. Y., Menke J. T., West R. (2022). Atlas of Yellowstone. 2nd Edn (Berkley: University of California Press).
Markham A. C., Gesquiere L. R., Alberts S. C., Altmann J. (2015). Optimal group size in a highly social mammal. Proc. Nati. Acad. Sci. U.S.A. 112, 14882–14887. doi: 10.1073/pnas.1517794112
McCullough D. R. (1979). The George Reserve deer herd: population ecology of a K-selected species (Ann Arbor: University of Michigan Press).
Mckinney T., Devos J. J.C., Ballard W. B., Boe S. R. (2006). Mountain lion predation of translocated desert bighorn sheep in Arizona. Wildl. Soc Bull. 34, 1255–1263. doi: 10.2193/0091-7648(2006)34[1255:MLPOTD]2.0.CO;2
McLellan B. N., Serrouya R., Wittmer H. U., Boutin S. (2010). Predator mediated Allee effects in multi-prey systems. Ecol. 91, 286–292. doi: 10.1890/09-0286.1
Monteith K. L., Bleich V. C., Stephenson T. R., Pierce B. M., Conner M. M., Kie J. G., et al. (2014). Life-history characteristics of mule deer: Effects of nutrition in a variable environment. Wildl. Monogr. 186, 1–62. doi: 10.1002/wmon.1011
Mooring M. S., Fitzpatrick T. A., Nishihira T. T., Reisig D. D. (2004). Vigilance, predation risk, and the Allee effect in desert bighorn sheep. J. Wildl. Manage. 68, 519–532. doi: 10.2193/0022-541X(2004)068[0519:VPRATA]2.0.CO;2
Moran N. P., Sánchez-Tójar A., Schielzeth H., Reinhold K. (2021). Poor nutritional condition promotes high-risk behaviours: a systematic review and meta-analysis. Biol. Rev. 96, 269–288. doi: 10.1111/brv.12655
Morrison M. L., Marcot B. G., Mannan R. W. (2006). Wildlife–habitat Relationships. 3rd Edn (Washington, D.C: Island Press).
Mychajliw A. M., Adams A. J., Brown K. C., Campbell B. T., Hardesty-Moore M., Welch Z. S., et al. (2024). Coupled social and ecological change drove the historical extinction of the California grizzly bear (Ursus arctos californicus). Proc. R. Soc B: Biol. Sci. 291, 20230921. doi: 10.1098/rspb.2023.0921
Mysterud A., Loe L. E., Zimmermann B., Bischof R., Meisingset E., Mysterud A., et al. (2011). Partial migration in expanding red deer populations at northern latitudes-a role for density dependence? Oikos 120, 1817–1825. doi: 10.1111/j.1600-0706.2010.19439.x
Nickel B. A., Suraci J. P., Nisi A. C., Wilmers C. C. (2021). Energetics and fear of humans constrain the spatial ecology of pumas. Proc. Nati. Acad. Sci. U.S.A. 118, 2004592118. doi: 10.1073/pnas.2004592118/-/DCSupplemental
Orpwood J. E., Magurran A. E., Armstrong J. D., Griffiths S. W. (2008). Minnows and the selfish herd: effects of predation risk on shoaling behaviour are dependent on habitat complexity. Anim. Behav. 76, 143–152. doi: 10.1016/j.anbehav.2008.01.016
Pulliam H. R. (1973). On the advantages of flocking. J. Theor. Biol. 38, 419–422. doi: 10.1016/0022-5193(73)90184-7
Rigge M., Homer C., Cleeves L., Meyer D. K., Bunde B., Shi H., et al. (2020). Quantifying western U.S. rangelands as fractional components with multi-resolution remote sensing and in situ data. Remote Sens. (Basel) 12, 412. doi: 10.3390/rs12030412
Robbins C. T. (1973). The Biological Basis for the Determination of Carrying Capacity (Ithaca: Cornell University).
Robinson N. P., Jones M. O., Moreno A., Erickson T. A., Naugle D. E., Allred B. W. (2019). Rangeland productivity partitioned to sub-pixel plant functional types. Remote Sens. (Basel) 11, 1427. doi: 10.3390/rs11121427
Rominger E. M. (2018). The Gordian knot of mountain lion predation and bighorn sheep. J. Wildl. Manage. 82, 19–31. doi: 10.1002/jwmg.21396
Schroeder C. A., Bowyer R. T., Bleich V. C., Stephenson T. R. (2010). Sexual segregation in Sierra Nevada bighorn sheep, Ovis canadensis sierrae: Ramifications for conservation. Arct. Antarct. Alp. Res. 42, 476–489. doi: 10.1657/1938-4246-42.4.476
Smith W. P. (1991). Ontogeny and adaptiveness of tail-flagging behavior in white-tailed deer. Am. Nat. 138, 190–200. doi: 10.1086/285211
Smith T. S. (1992). The Bighorn Sheep of Bear Mountain: Ecological Investigations and Management Recommendations (Provo: Brigham Young University).
Smith B. J., MacNulty D. R., Stahler D. R., Smith D. W., Avgar T. (2023). Density-dependent habitat selection alters drivers of population distribution in northern Yellowstone elk. Ecol. Lett. 26, 245–256. doi: 10.1111/ele.14155
Smythe S. E., Sanchez D. M., Epps C. W. (2019). Contrasting winter moose nutritional carrying capacity models on a dynamic landscape. J. Fish. Wildl. Manage. 10, 163–179. doi: 10.3996/122017-JFWM-104
Steinberg D. S., Losos J. B., Schoener T. W., Spiller D. A., Kolbe J. J., Leal M. (2014). Predation-associated modulation of movement-based signals by a Bahamian lizard. Proc. Nati. Acad. Sci. U.S.A. 111, 9187–9192. doi: 10.1073/pnas.1407190111
van Beest F. M., McLoughlin P. D., vander Wal E., Brook R. K. (2014). Density-dependent habitat selection and partitioning between two sympatric ungulates. Oecologia 175, 1155–1165. doi: 10.1007/s00442-014-2978-7
van Deventer A., Shrader A. M. (2021). Predation risk and herd position influence the proportional use of antipredator and social vigilance by impala. Anim. Behav. 172, 9–16. doi: 10.1016/j.anbehav.2020.11.019
Villepique J. T., Bleich V. C., Pierce B. M., Stephenson T. R., Botta R. A., Bowyer R. T. (2008). Evaluating GPS collar error: A critical evaluation of Televilt Posrec-scienceTM collars and a method for screening location data. Calif. Fish Game 94, 155–168. Available at: https://nrm.dfg.ca.gov/FileHandler.ashx?DocumentID=47353&inline=1.
Wehausen J. D. (1980). Sierra Nevada bighorn sheep: History and population ecology (Ann Arbor: University of Michigan).
Wheeler B. C. (2008). Selfish or altruistic? An analysis of alarm call function in wild capuchin monkeys, Cebus apella nigritus. Anim. Behav. 76, 1465–1475. doi: 10.1016/j.anbehav.2008.06.023
Whiting J. C., Bleich V. C., Bowyer R. T., Epps C. W. (2023). Restoration of bighorn sheep: History, successes, and remaining conservation issues. Front. Ecol. Evol. 11. doi: 10.3389/fevo.2023.1083350
Xian G., Homer C., Rigge M., Shi H., Meyer D. (2015). Characterization of shrubland ecosystem components as continuous fields in the northwest United States. Remote Sens. Environ. 168, 286–300. doi: 10.1016/j.rse.2015.07.014
Zhang Y. (2013). Likelihood-based and Bayesian methods for Tweedie compound Poisson linear mixed models. Stat. Comput. 23, 743–757. doi: 10.1007/s11222-012-9343-7
Keywords: density-dependence, nutritional carrying capacity, Ovis canadensis sierrae, population growth, selfish herd theory, Sierra Nevada bighorn sheep, tradeoff, ungulates
Citation: Rankins ST, Stephenson TR and Monteith KL (2024) Sociality modulates nutritional carrying capacity of an endangered species. Front. Ecol. Evol. 12:1417970. doi: 10.3389/fevo.2024.1417970
Received: 15 April 2024; Accepted: 31 July 2024;
Published: 20 August 2024.
Edited by:
Aurelio F. Malo, University of Alcalá, SpainReviewed by:
Stephan T. Leu, University of Adelaide, AustraliaJorge Lozano, Complutense University of Madrid, Spain
Copyright © 2024 Rankins, Stephenson and Monteith. This is an open-access article distributed under the terms of the Creative Commons Attribution License (CC BY). The use, distribution or reproduction in other forums is permitted, provided the original author(s) and the copyright owner(s) are credited and that the original publication in this journal is cited, in accordance with accepted academic practice. No use, distribution or reproduction is permitted which does not comply with these terms.
*Correspondence: Seth T. Rankins, c2V0aC50LnJhbmtpbnNAZ21haWwuY29t