- 1Department of Biological Sciences, Auburn University, Auburn, AL, United States
- 2Department of Biology, Texas A&M University, College Station, TX, United States
Introduction: Upside-down jellyfish (Cassiopea sp.) are highly tolerant to multiple abiotic stressors, including fluctuating temperatures associated with shallow marine habitats. This resilience may underlie the ability of Cassiopea sp. to inhabit a wide variety of tropical habitats across the globe. Additionally, Cassiopea sp. are marked by a conspicuous array of appendage coloration; individual medusae vary in the hue and number of oral appendages, which are often strikingly blue. The function of this coloration is not understood. We aimed to understand how extrinsic (temperature, location) and intrinsic (host color) factors may shape thermal tolerance.
Methods: Adult Cassiopea xamachana were collected from two sites that vary in daily temperature range within the Florida Keys and were subjected to acute lethal heat stress. To quantify a whole-organism response to heat, we measured changes in bell pulsation, which likely plays a role in feeding, oxygen exchange, and symbiont uptake. Finally, color morphs were acclimated at either ambient (26°C) or elevated (33°C) temperatures.
Results: C. xamachana from two locations that vary in thermal range do not exhibit different responses to heat, suggesting that temperature fluctuations do not prime individuals for higher thermal tolerance. Additionally, C. xamachana with blue appendages survived significantly higher temperatures and exhibited less change in bell pulsation rates compared to non-blue individuals. We found that acclimation at 33°C, as well as appendage color in each treatment, led to higher survival under acute heat stress.
Discussion: These findings highlight the importance of temperature and coloration in Cassiopea xamachana resilience during heat stress.
1 Introduction
As global change continues to threaten marine ecosystems, rising sea surface temperature is the dominant stressor for many shallow water marine species (Filbee-Dexter et al., 2020; Lang et al., 2023; Mellin et al., 2019; Pörtner et al., 2019; Strydom et al., 2020). Ectotherms are particularly vulnerable to environmental variation, as even small temperature changes (1–2 °C; Pinsky et al., 2019) can affect physiology and behavior (Lagerspetz and Vainio, 2006; Lang et al., 2023). In response to environmental change, ectothermic organisms can adjust physiological traits (e.g., respiration rate, metabolism, and reproduction; Guderley, 1990; Seebacher et al., 2015) through phenotypic plasticity (Ghalambor et al., 2007; Hartl and Conner, 2004; Jardeleza et al., 2022). This plasticity may facilitate survival during short-term heatwave events, which can help maintain genetic diversity on which selection can act and shape the population (Botero et al., 2015; Gunderson and Stillman, 2015; Huey et al., 2012; Palumbi et al., 2019; Somero, 2010). Given the rapid pace of sea surface temperature increase, it is critical to understand the role of phenotypic plasticity in maintaining variation within and among populations of marine species, and how plasticity contributes to organismal persistence in the face of environmental stress.
Plastic phenotypes are generally favored when environmental variability is predictable (DeWitt et al., 1998; Goldstein and Ehrenreich, 2021; Moran, 1992), and the costs associated with adjusting the phenotype are minimal (Gavrilets and Scheiner, 1993; Pigliucci and Schlichting, 1998; Scheiner, 2018). As with most traits, plasticity itself can vary within and among populations, which may further affect the distribution of resulting phenotypes (Fuller et al., 2022). Natural experiments in coral reef systems indicate that individuals that live in environments characterized by high temperature variability (e.g., back-reef pools and intertidal/shallow reefs) exhibit enhanced thermal tolerance (Palumbi et al., 2014; Rivest et al., 2017; Safaie et al., 2018; Schoepf et al., 2022). Increases in thermal tolerance due to plasticity may also facilitate individual persistence in response to climate change (Schaum et al., 2022).
One of the most strikingly variable phenotypes in marine invertebrates is coloration. In general, color can be produced in one of three ways: structural coloration (i.e., microstructures that selectively interfere with light; Parker, 1998); pigments, which can be either synthesized by the organism or obtained externally; or chromoproteins (Haddock and Dunn, 2015). In some cases, color variation has been linked to physiological processes such as thermoregulation and stress response (Umbers, 2013). For example, sea cucumbers (Apostichopus japonicus), with either green or red coloration exhibit differential thermotolerance (Dong et al., 2010). Physiological roles for color-associated proteins have also been identified in several cnidarian species. Cnidarians exhibit particularly diverse coloration patterns, which are typically the result of either pigment-derived proteins (e.g., carotenoproteins) or fluorescent proteins (FPs; Matz et al., 2002; Shagin et al., 2004). Several Acropora species display polymorphism in fluorescent protein expression which have been hypothesized to contribute to acclimatization potential (Dove, 2004; Gittins et al., 2015; Jarett et al., 2017; Kelmanson, 2003; Paley, 2014; Takahashi-Kariyazono et al., 2018). Several pigments have functions associated with regulating light available for photosynthetic algal symbionts. In reef-building corals, FPs can enhance or dissipating light, thereby modulating light available for symbionts and increasing resistance to bleaching during warming events (Salih et al., 2000). Non-fluorescent chromoproteins generated by the host can have photoprotective roles (Smith et al., 2013; Ferreira et al., 2023). However, cnidarian pigments have also been implicated in a wide variety of physiological functions, including thermal dissipation (Lyndby et al., 2016), antioxidant activity (Bou-Abdallah et al., 2006), and symbiont attraction (Aihara et al., 2019; Hollingsworth et al., 2005). Each of these could contribute to survival in stress conditions (Ferreira et al., 2023).
Studies investigating links between coloration and ecological or evolutionary processes in marine invertebrates have primarily focused on the most common colors: reds, greens, and oranges (Muñoz-Miranda and Iñiguez-Moreno, 2023). In contrast, work on blue colors has been somewhat limited, largely due to the rarity of this color in marine habitats (Lawley et al., 2021; Newsome et al., 2014). Blue coloration has been shown to affect cnidarian physiology; for example, in the scleractinian coral Acropora aspera, individuals with blue pigmentation maintained higher algal densities and photosynthetic efficiency compared to those that lack blue pigment in response to UV light (Dove, 2004).
One of the most striking examples of blue coloration in marine invertebrates is the upside-down jellyfish (Cassiopea sp.; Arai et al., 2017; Gamero-Mora et al., 2022; Medina et al., 2021; Ohdera et al., 2018). Cassiopea xamachana individuals exhibit a striking diversity of color variation, with many different patterns of deep blues and greens in oral appendages and throughout the mesoglea. This unique blue is produced by a chromoprotein known as Cassio Blue (Phelan et al., 2006), which has orthologs in several scyphozoan corals and jellyfish (Bulina et al., 2004; Lawley et al., 2021). Cassio Blue is highly expressed in C. xamachana, comprising up to 6% of the total animal protein (Phelan et al., 2006). Transition metals (Ag, Cu, Mg, Ca, Fe, and Zn) have been recovered with the Cassio Blue protein, indicating that it may serve as a ligand for metal complexation (Phelan et al., 2006). Lawley et al. (2021) suggests that the blue may serve a photoprotective function, however, physiological roles have not yet been identified for blue color or Cassio Blue in Cassiopea sp.
Cassiopea sp. are exceptionally robust in response to environmental variability and evidence suggests that their range is expanding globally across tropical coastal marine habitats (Bayha and Graham, 2014; Holland et al., 2004; Morandini et al., 2017; Stampar et al., 2020). Cassiopea sp. primarily inhabit shallow coastal ecosystems, where temperatures vary considerably among seasons and even within days (Aljbour et al., 2019; Kayal et al., 2012; Rowe et al., 2022). Studies show that C. andromeda are highly tolerant to stress and may even benefit from acute heat stress (Banha et al., 2020). In C. xamachana, this resilience appears to be independent of their symbiotic algae. Cassiopea xamachana that inhabit the Florida Keys are almost exclusively dominated by Symbiodinium microadriaticum (LaJeunesse, 2001). Although S. microadriaticum is generally considered to be thermally susceptible (Iglesias-Prieto et al., 1992; Iglesias-Prieto and Trench, 1997; Warner et al., 1999), Symbiodinium exhibits high levels of intraspecific variation in the response to temperature (Mansour et al., 2018). Understanding the underlying physiological mechanisms that enable C. xamachana to thrive under temperature stress may provide unique insights into the impact of global climate change on marine invertebrates.
Despite their benthic lifestyle in shallow, low water flow habitats, Cassiopea sp. can generate exceptional biogenic mixing via bell pulsation, which can help facilitate ecologically important nutrient mixing within these environments (Durieux et al., 2021). Through continuous cycles of contracting and relaxing their bell, Cassiopea sp. can drive water and small prey around the subumbrellar cavity for feeding along the oral arms (Santhanakrishnan et al., 2012); this cyclical movement also has the potential to provide mixing of nutrients in what would normally be a low flow ecosystem (Durieux et al., 2021). Bell pulsation may also play a role in oxygen exchange and temperature regulation, such that rates of bell pulsation typically increase with rising temperatures (Arai, 1996; Béziat and Kunzmann, 2022), and decline rapidly as lethal levels are reached (Dillon, 1977). In Cassiopea sp., bell pulsation rates have been suggested to reflect metabolic rates (Fitt et al., 2021; Mangum et al., 1972; McClendon, 1917). Thus, this can be used as an observable, whole-organism, ecologically relevant phenotype for assessing organismal response during acute heat stress.
Here, we investigate how environmental history, appendage color, and temperature acclimation influence C. xamachana medusae responses to heat stress. Similarly, as in other cnidarians, we find that C. xamachana responds to acute heat stress by increasing bell pulsation rates until ~37 °C, at which point pulsation rates slow until lethality (38–41 °C). Daily temperature ranges that varied between collection sites had no effect on rates of bell pulsation or lethal temperature. However, we present evidence that C. xamachana can acclimate to increased temperatures and that survival is associated with the presence of blue appendages. This work is the first to demonstrate a link between color phenotype and resilience to thermal stress in Cassiopea xamachana and begins to fill the knowledge gap concerning the novel role for chromoproteins in thermal physiology.
2 Materials and methods
2.1 Collection sites and environmental data
For all experiments, C. xamachana medusae were collected by hand from two sites in Key Largo, FL, USA. The “Atlantic” site (25.086669, −80.453382) is near-shore and receives water flow from the Atlantic Ocean. The “Bay” site (25.079426, −80.453009) is located off docks leading into the Florida Bay (Supplementary Figure 1). Water temperature was measured at collection sites using pendant HOBO® data loggers (MX2201) were deployed from March 12 to September 9, 2022, at a depth of 1.5 m to reflect the depth at which C. xamachana are regularly observed. Daily mean temperatures and ranges (maximum - minimum) were calculated using 15-minute interval data (Figures 1A, B). Statistical differences between mean daily temperature and daily temperature were determined using t-tests in R with the stats package (v. 4.3.0; R Core Team, 2023).
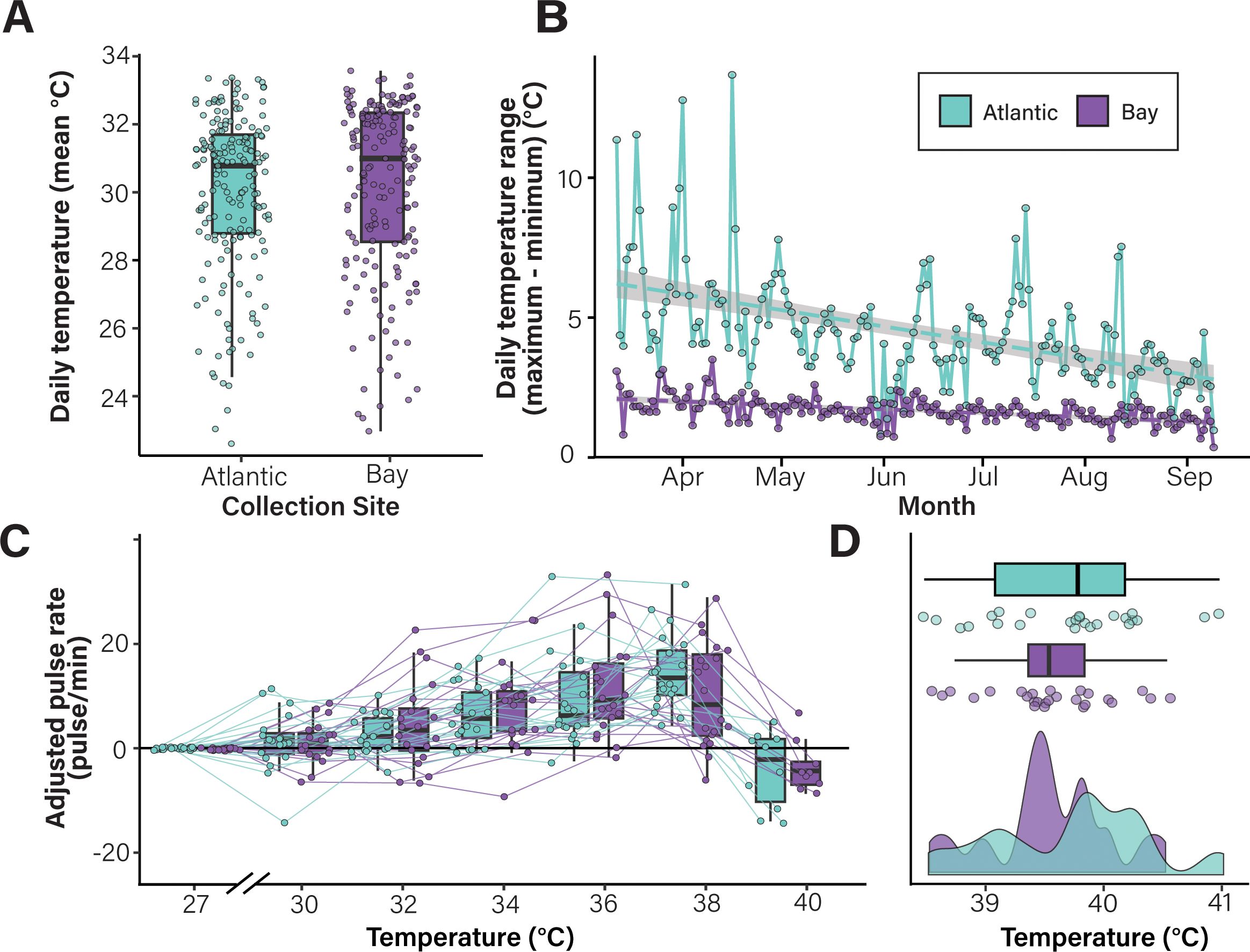
Figure 1. Environmental history does not affect C. xamachana bell pulsation rate or lethal temperature during acute heat stress. (A, B) Cassiopea xamachana that inhabit the Atlantic site experience greater environmental variation than those in the Bay site. Daily mean temperatures (A) and temperature ranges (B) are shown for the Atlantic (teal) and Bay (purple) collection sites. Box plots show the median value for each group as a thick line; boxes represent the interquartile range and whiskers signify the minimum and maximum values. Statistical analyses indicate that the two locations have similar daily mean temperatures (pt-test = 0.378) but significantly variation in daily temperature (daily temperature range; pt-test < 0.0001). (C, D) Cassiopea xamachana collected from the two sites do not differ in their response to an acute lethal heat stress. Each point represents data from a single individual. Data are shown as adjusted pulse rate (the difference in bell pulsation rate (pulses per minute) between the baseline temperature (27°C) and the temperature indicated). Lines connect measurements collected from individual medusa. A density plot of lethal temperatures is shown in (D) Statistical analysis indicates that collection site does not influence lethal temperature during acute heat stress (pLMM = 0.356).
2.2 Experiment 1: Assessing the role of environmental variability on C. xamachana heat stress physiology
In May 2021, approximately 50 C. xamachana medusae (28–95 mm) were collected from each of the two sites (Supplementary Figure 1) and transported to Auburn University where they were acclimated for two weeks in a closed-sump aquarium system under ambient conditions (26 °C ± 1 °C; 36 ppt salinity). Animals were maintained on a 12:12 light:dark cycle and fed Artemia three times per week. Morphological characteristics (bell diameter, sex, and appendage morphology) were recorded, and animals were re-acclimated for one week to compensate for handling stress.
Acute heat stress trials were performed such that 10 animals were monitored simultaneously, and trials were repeated five times with different individuals (10 animals x 5 blocks = 50 total animals measured). Within each block, five individuals from each collection site were chosen at random. The evening before each trial, individual medusae were placed into 500 mL containers with mesh sides in the experimental tank (water conditions were identical to acclimation tank). Water temperature was controlled using two submersible rod heaters (Finnex Deluxe Titanium Tube Heater, 800W) connected to a digital temperature controller (SunTHIN WiFi Digital Temperature Controller 1250W, 10A). Experimental tank temperatures were monitored using pendant HOBO® data loggers (MX2201) recording temperatures at 15-minute intervals. Heat stress trials were performed at the same time each day to reduce potential variability associated with photoperiod (Nath et al., 2017). Water temperature was increased 1°C/hour; 30 minutes after each temperature increase, 1-minute videos were recorded in triplicate using Blink security cameras (Amazon) suspended above the experimental tank. Trials were complete when all medusae reached lethality, defined here as either 0.5 x baseline pulsation rate or < 10 pulses/minute. This criterion corresponded with tissue disintegration and was often accompanied by a separation of arms from bell tissue (Toullec et al., 2024). Dead animals were immediately removed from the experimental tank. Lethal temperatures and bell diameters at time of death were recorded for each individual.
2.3 Experiment 2: Testing the role of temperature acclimation on C. xamachana heat stress physiology
In May 2022, approximately 50 C. xamachana medusae of each color morph (blue and uncolored/brown) were collected from the Atlantic site (Supplementary Figure 1) and transported to Auburn University where they were randomly placed into two sump systems, each with 4 individual tanks, and maintained as described above. After a five-day acclimation period, morphological characteristics (bell diameter, sex, and appendage morphology) were recorded. Individuals were photographed at a standard height (0.5 m) using a Canon Rebel X camera with a size and color standard. Tissue samples were collected from arm tips to measure symbiont density (stored at 4°C) and isolate genomic DNA (flash-frozen in liquid nitrogen and stored at −80°C). Animals were acclimated for three days to compensate for handling stress.
The temperature in one of the two sump systems was increased 2°C per day and then maintained at 33°C (± 1 °C) for 30 days (N =43; these animals are referred to as “elevated”). Individuals in the remaining tanks (N = 42) were maintained in ambient conditions (26 °C ± 1°C). Temperatures were monitored using pendant HOBO® data loggers (MX2201). After the acclimation period, bell diameters were measured, and arm tissue was sampled to quantify symbiont density (stored at 4°C).
Acute heat stress trials were performed on medusae three days after post-acclimation measurements. Trials were performed as described in Experiment 1, with the additional parameters that 1) animals of each color morph were evenly distributed among the blocks; and 2) “elevated” animals were transferred to the experimental tank when the temperature matched that of their acclimation tank (33 °C).
2.4 Bell pulsation analysis
Rates of bell pulsation were quantified using the videos captured during acute heat stress experiments. Videos were viewed once per individual within the camera view. Pulsation rate was calculated as the number of pulses per minute. Bell pulsation rates vary uniquely among Cassiopea sp. individuals (Rowe et al., 2022). To account for this variation and assess the influence of heat stress on bell pulsation, baseline pulsation rates were calculated in each individual in the absence of any experimental manipulation. For all experiments in 2021 and the medusae that were acclimated at ambient temperatures in 2022, baseline rates were calculated after the animals were transferred to the experimental tanks and allowed to acclimate overnight to account for handling stress. In medusae acclimated at elevated temperatures (2022 trials), baseline rates were calculated in the experimental tank at 32°C. For all data shown, the individual baseline pulsation rate was subtracted from the pulsation at each temperature. All raw data, including the baseline pulsation rates and un-adjusted rates are available at https://github.com/mem0294/Maloneyetal2024.
To test the effects of collection site or acclimation status, appendage color, and temperature on unadjusted bell pulsation rates, a generalized linear mixed-effect model (GLMM) with a Poisson link-log function was conducted in R using the package lme4 (v.1.1-7, Bates et al., 2015). In these designs, the location or acclimation state, appendage color, and temperature during heat stress were fixed effects, and individuals and the date of the experiment (block) were coded as random effects. To test the effects of location or acclimation, appendage color, and heat stress temperature on the change in bell pulsation rates from baseline rates, a linear mixed effect model (LMM) was conducted with the R package lme4 (v.1.1-7, Bates et al., 2015). A LMM was used to examine differences in lethal temperature between location/acclimation and appendage colors. In this design, location/acclimation and appendage color were fixed effects; the date of the heat stress trial (block) was a random effect.
For all analyses, assumptions of normality and homogeneity of variance were graphically assessed using histograms, residual plots, and quantile-quantile plots. When there was a significant main effect (p < 0.05), Tukey’s post hoc mean comparisons were conducted using the multcomp package (v.1.4-25, Hothorn et al., 2008).
2.5 Analyzing color, saturation and morphology of medusae appendages
Images taken before the acclimation treatment were analyzed in Adobe Photoshop CC to quantify number of pigmented appendages, color information, and blue coverage (percent). The number of pigmented appendages was quantified using FIJI (Schindelin et al., 2012) using the “Cell Counter” plugin (O’Brien et al., 2016). Appendages were counted if they met any of the following criteria: 1) contained a color dissimilar to that of the bell or frilled sections (this includes but is not limited to: blue, green, yellow, purple) or 2) morphologically matched that of a typical “large vesical” (as described in Hummelinck, 1968). The presence of “pigmented” appendages was not used to distinguish blue vs. brown individuals.
RGB (red, blue, and green) and HSB (hue, saturation, brightness) values were quantified using the Color Sampler tool. Hue refers to true color (e.g., red, green, or blue) and is measured as degrees on a color wheel, such that 0° (and 360°) is red. Saturation is the intensity or richness of color and is measured by a percentage, such that 0% indicates “no color” and 100% indicates “intense color”. Brightness is also measured as a percentage such that 0–100% corresponds to black–white. Four measurements were recorded for each individual: 1) a pigmented vesicle (if present); 2) a non-pigmented oral arm appendage; 3) the bell tissue; and 4) the blue color standard that was included in each image and served as a control for variation in imaging. To normalize across images, the HSB values of the blue color standard were subtracted from each of the jellyfish measurements. A Pearson correlation was conducted in R to assess the relationship between lethal temperature and adjusted saturation percent.
The coverage of blue appendages present in individual medusae was quantified using the number of pixels (in percent) associated with blue in the R package countcolors (v.0.9.1, Weller and Westneat, 2019). Image backgrounds were removed in Adobe Photoshop and replaced with white; images were manually edited to ensure that the entire medusa was included. The countcolors package quantifies all pixels in an image according to RGB values translated to a 0–1 scale, rather than the traditional 0–255 (Weller and Westneat, 2019). The package was run as follows: ignore white (RGB [1, 1, 1]) to exclude the background pixels and identify the “blue” pixels (RGB [0.33, 0.30, 0.25] with an extended radius of 0.1. This color range was determined by quantifying RGB values for pigmented appendages sampled across all blue jellyfish and averaging the value. Note that, because these organisms are not bleached, the background tissue color is light brown due to the presence of the algal endosymbionts. Therefore, the color values of the pigmented appendages fall within a brownish gray, rather than pure blue. The countcolors package subsequently exports a new image in which pixels within the range are masked as magenta (RGB [1, 0, 1]). The accuracy of the masked images was verified manually and adjusted as necessary. Finally, countcolors was used on the masked images to calculate the percentage of magenta pixels in the image. A Pearson correlation was conducted in R to assess the relationship between lethal temperature and amount of blue coloration.
2.6 Quantifying symbiont density
Medusa arm tip pieces were added to pre-weighed 1.5 ml centrifuge tubes and weighed to determine wet mass. Frozen tissue was homogenized in 500-700 μL of artificial seawater (Instant Ocean) using a disposable pestle and an electric homogenizer. To separate host tissue from the Symbiodiniaceae cells, homogenized samples were centrifuged at 800 x g for 10 minutes. Algal pellets were washed in 500 µl artificial seawater, vortexed to mix and re-homogenized as above. Samples were washed three times in artificial seawater (400 x g for 10 minutes) and resuspended in 150 µl Z-Fix (ANATECH). Symbionts were counted using an Improved Neubauer hemocytometer and normalized to the wet mass to calculate density. Eight counts were performed for each individual. A repeated measures ANOVA in R was used to examine symbiont density before and acclimation. In this design, acclimation treatment and appendage color were categorized as fixed effects; individual medusa ID was used as the repeated measure, and therefore the random effect.
2.7 Verifying Cassiopea species
DNA from arm tissue was extracted using the E.Z.N.A. Tissue DNA Kit (Omega Bio-Tek) according to manufacturer’s instructions. DNA concentrations were quantified using the dsDNA Broad-Range Qubit assay (Invitrogen). The mitochondrial gene cytochrome c oxidase subunit I [COI] was amplified using custom-designed primers (Supplementary Table 1; Ye et al., 2012). Thermal cycling conditions were 3 min at 95°C for initial denaturation, followed by 35 amplification cycles (denaturation at 95°C for 35 s, annealing at 49°C for 40 s and extension at 72°C for 50 s) and a final extension for 7 min at 72°C. Amplified products were cleaned (Zymo Research Genomic DNA Clean and Concentrator-10 kit) and quantified using the dsDNA Broad-Range Qubit assay (Invitrogen). Purified products were sequenced using Sanger chemistry with custom primer MM1 (Supplementary Table 1). COI sequences were aligned to publicly available sequences from C. xamachana (Genbank accession NC_016466.1; Ohdera et al., 2019) and C. andromeda (OP503353.1 and OP503325.1; Kayal et al., 2012) using Geneious v. 2022.2.2.
2.8 Identifying Symbiodiniaceae type using ITS2 amplicon sequencing
Medusae collected from the Bay (n = 6) and Atlantic sites (n= 5) were used to characterize symbiont communities. Tissues samples were collected from arms, stored in ethanol and used for DNA extractions as described. ITS-2 regions were amplified using GoTaq MasterMix (Promega), and Symbiodiniaceae-specific primers (final concentration 400 nM) with adaptors for Illumina sequencing (Supplementary Table 1). Reactions were amplified as follows: 95°C for 3 min, 25 cycles of 95°C for 30 sec, 55°C for 30 sec, 72°C for 30 sec, and 72°C for 5 min. Samples were purified with the Genomic DNA Clean and Concentrator-10 kit (Zymo Research) and sequenced on the Illumina MiSeq platform (150 bp paired-end reads; Georgia Genomics).
The R package dada2 (Callahan et al., 2016) was used to identify and quantify the Symbiodiniaceae taxa present in each sample. After removing chimeric sequences, valid amplicon sequence variants (ASVs) were identified and enumerated. To identify the proportion and identity of the symbiont community in each sample, we created a BLAST database containing annotated ITS2 sequences from the SymPortal framework (https://symportal.org).
3 Results
3.1 Animals used in this study are C. xamanchana
Given the morphological variation and potential for cryptic species among Cassiopea sp., (Muffett and Miglietta, 2023), the species identity of collected animals was confirmed. DNA isolated from medusae used for the 2022 acute heat stress experiment was used to amplify the COI gene for sequencing. All 30 sequences exhibited >99.6% sequence identity with the Cassiopea xamachana COI sequence (Kayal et al., 2012; Supplementary Figure 2A). Further, 11 representative animals from the collection sites were selected for ITS-2 symbiont typing. These medusae were overwhelmingly dominated by Symbiodinium A1 (Supplementary Figure 2B), which is consistent with studies showing strong host-symbiont fidelity between C. xamachana and S. microadriaticum (LaJeunesse, 2017). Thus, despite the observed morphological variation among individuals, medusae used in these studies are C. xamachana.
3.2 Daily temperature variation does not affect rates of bell pulsation or lethal temperatures during acute heat stress
To determine if environmental history affects how C. xamachana responds to heat stress, medusae were collected from two sites that vary significantly in daily temperature range (“Atlantic” and “Bay” sites; Supplementary Figure 1). Temperature data collected from the two sites revealed that, although these locations had similar average daily temperatures (30.1°C in the Bay and 29.9°C in the Atlantic; Figure 1A), the Atlantic site water temperatures were significantly more variable than those in the Bay site (pt-test < 0.001, Figure 1B). On average, water temperatures at the Atlantic site varied 4.55°C per day compared to 1.73°C at the Bay site. This variability may reflect changes in water depth due to tidal influx.
Animals from each site were exposed to an acute lethal heat stress (starting from ambient temperature and increasing 1°C/hour until lethality). Bell pulsation rates were used to monitor organismal response. Notably, no significant differences were observed in the change in bell pulsation rates among animals collected from the two sites (Figure 1C; Supplementary Figure 3; Supplementary Table 2). The rates of bell pulsation increased ~3.1 pulses per minute for each 1°C increase in water temperature (pGLMM < 0.001, Supplementary Table 3) until 37°C, at which point pulsation rates dropped dramatically just prior to mortality. Post-hoc analyses identified statistical differences in the change in pulsation rates at several temperatures relative to baseline (Supplementary Table 4). Additionally, no significant difference was observed in average lethal temperatures of C. xamachana collected from the Atlantic (39.81°C), and Bay sites (39.52°C; pLMM = 0.356, Figure 1D, Supplementary Table 5). Notably, despite surviving to 40°C, we observed no evidence of symbiont loss (bleaching) in medusa from either collection site. Together, these data indicate that, despite the difference in daily temperature range and variability between collection sites, C. xamachana from both locations displayed similar physiological phenotypes in response to acute heat stress.
3.3 Temperature acclimation influences bell pulsation and lethal temperature
To determine if C. xamachana can acclimate to elevated temperatures, medusae (N=100) were collected from the Atlantic site and maintained at either ambient (26°C) or elevated (32°C) temperature for 30 days (Supplementary Figure 4A). The different acclimation temperatures did not cause significant differences in bell diameter or symbiont density (Supplementary Figure 4B, C). In fact, medusae in both treatment conditions exhibited reduced bell diameters and increased symbiont density (Supplementary Figure 4B, C), which may reflect the transition to the artificial aquarium system. Following acclimation, 40 individuals (Nambient = 20, Nelevated = 20) were randomly selected for heat stress trials.
Following the 30-day acclimation period and after the first group of animals was subjected to experimental heat stress, an unexpected mechanical failure in the ambient tank (26°C) caused a temperature decrease of 5°C for 24 hours. The remaining individuals were thus treated as “cold-shocked”. To account for the possibility that this affected subsequent responses, shock status was added as a fixed effect for all models. Notably, no significant interaction of shock status was observed with any other treatment factors. We have therefore interpreted fixed effects (temperature, acclimation treatment, and appendage color) equally.
Animals were exposed to an acute heat stress starting from the acclimation temperature and increasing 1°C/hour until lethality. As in the initial study, rates of bell pulsation steadily increased with temperature until animals approached lethality (Figure 2; Supplementary Figure 5). Acclimation temperature had a significant effect on the change of bell pulsation rates from the start of heat stress (pLMM = 0.008, Figure 2A, Supplementary Table 6). Notably, medusae acclimated in elevated conditions survived to higher temperatures compared to those acclimated at ambient temperatures. The average lethal temperature for C. xamachana acclimated at ambient temperature was 39.97°C compared with animals acclimated at elevated temperatures (41.2°C, pLMM < 0.0001; Figure 2B; Supplementary Table 8). This data show that C. xamachana can acclimate to a consistent period of elevated temperatures and that this acclimation is beneficial in the context of acute heat stress.
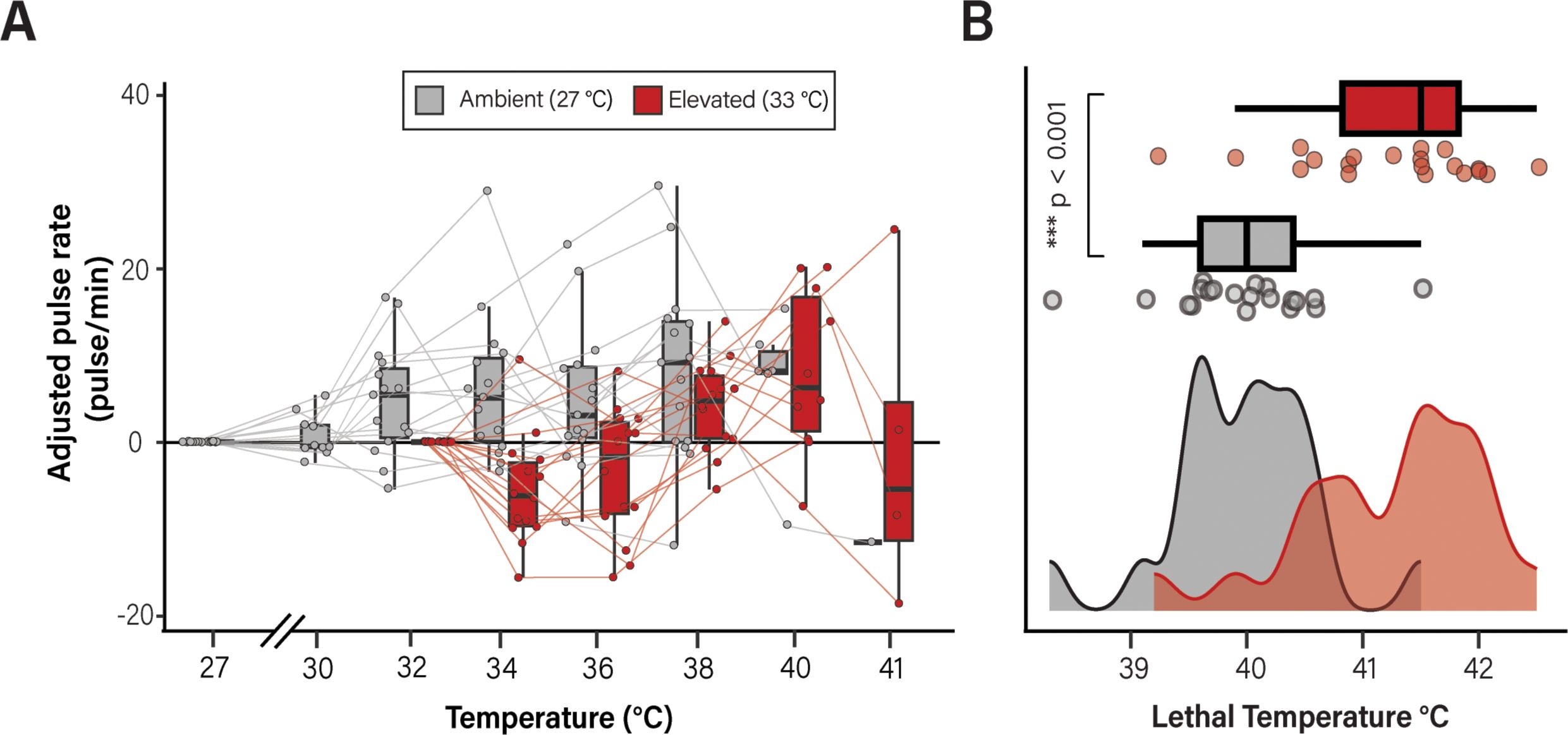
Figure 2. Temperature acclimation significantly affects bell pulsation rate and lethality. Individual medusae were acclimated at either 27°C (ambient; shown in red) or 32°C (elevated; shown in black) and subsequently exposed to an acute lethal temperatures stress and monitored for rates of bell pulsation (A) and temperature at which death occurred (B). Data are shown as adjusted pulse rates such that, for each animal, the baseline rate (measured at either 27°C for ambient or 32°C for elevated individuals) was subtracted from the pulsation rate at the temperature indicated. Pulsation rates are shown as pulses per minute. Lines connect measurements collected from individual medusa. Data in B are shown as a histogram of lethal temperatures during the acute heat stress trial. A significant difference (pLMM < 0.001) was observed in the lethal temperature of C. xamachana acclimated at ambient vs. elevated conditions. Box plots show the median value for each group as a thick line; boxes represent the interquartile range and whiskers signify the minimum and maximum values.
3.4 Appendage color is associated with C. xamachana survival during acute heat stress
Given the lack of significant differences in medusae collected from two sites (Figure 1), we were interested in identifying other factors that might influence C. xamachana physiology during heat stress. We therefore investigated if the variation in color phenotypes is associated with survival during heat stress. Using the data from both experiments, C. xamachana individuals were categorized as either “blue” or “brown” based on the predominant color of their oral appendages and all data were re-analyzed in the context of appendage color (Figure 3). Importantly, no relationship was observed between appendage color and either bell diameter or symbiont density (Supplementary Figure 6). The change in rate of bell pulsation during the acute heat stress experiments was not significantly different between medusae with blue appendages or brown appendages in either experimental year (2021 experiment, pLMM = 0.699, Figure 3A; 2022 experiment, pLMM = 0.681, Figure 3B). However, in both experiments, medusae with blue appendages survived significantly higher temperatures than those without (Figure 3C; 2021 experiment, pLMM = 0.003, Supplementary Table 5; 2022 experiment, pLMM < 0.007, Supplementary Table 8). This effect was also observed in medusae collected from both sites in 2021, such that individuals with blue appendages from the Atlantic site survived to significantly higher temperatures than those from the Bay site (pLMM = 0.0013; Figure 3D; Supplementary Table 9). In both blue and brown individuals, elevated acclimation treatment was significantly associated with higher survival (pLMER < 0.0001; Figure 3D).
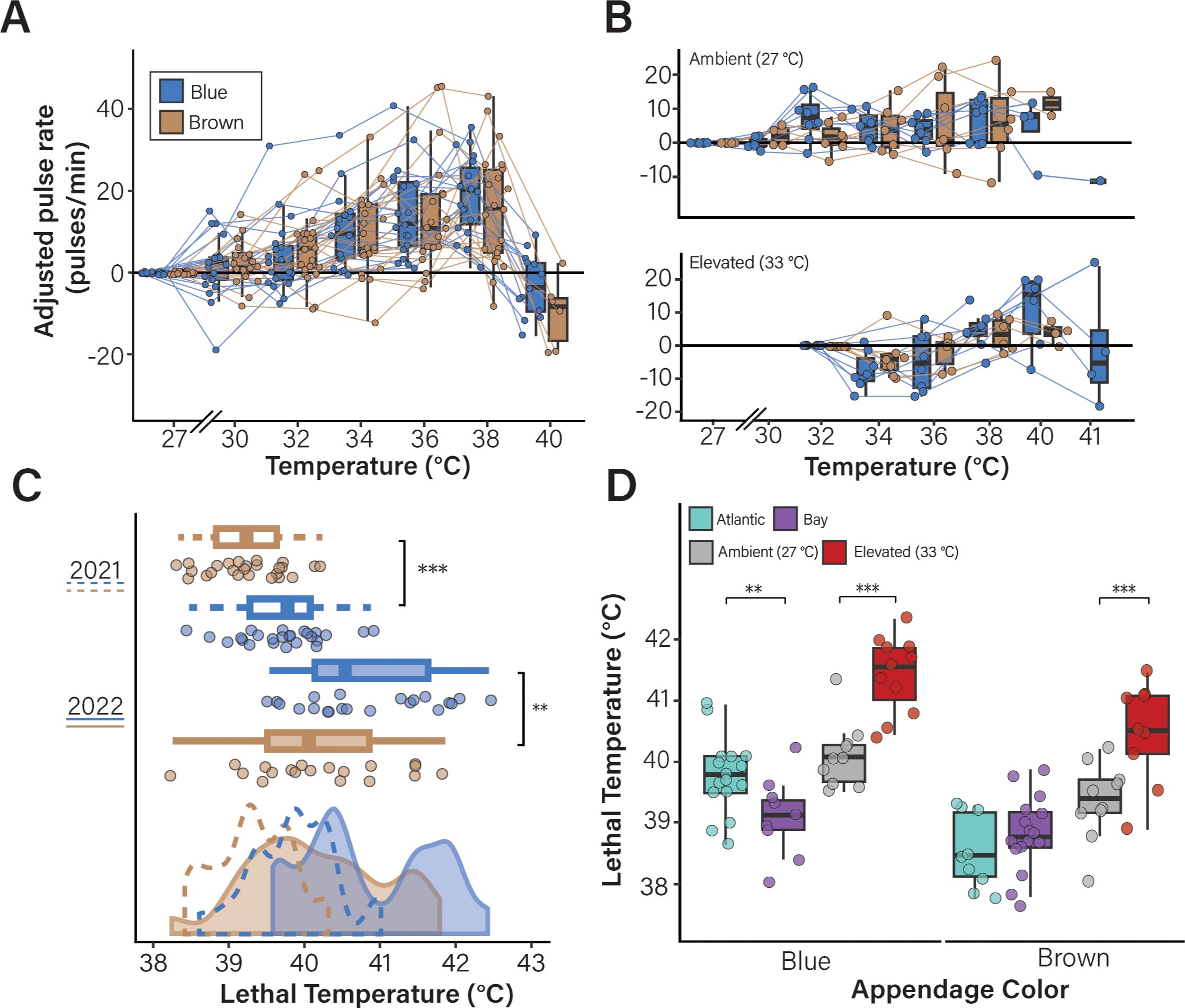
Figure 3. The presence of blue appendages is associated with C. xamachana survival during acute heat stress. Data from the experiments described in Figures 1, 2 were re-analyzed in the context of oral appendage color. Animals were classified as either “blue” or “brown” based on the predominant appendage color. No significant difference was observed in the adjusted pulsation rats of blue vs. brown animals in either experiment (2021 collection site data is shown in (A) pLMM = 0.44; 2022 acclimation data is shown in (B) pLMM = 0.94). Lines connect individual medusa between measurement points. Black dots indicate the final pulsation rate measured before lethality. (C, D) Appendage color affects lethal temperature during acute heat stress. Lethal temperatures in the context of appendage color are shown for the medusae collected in 2021 (C; pLMM = 0.0013) and 2022 (pLMM = 0.004) with significant differences denoted with asterisks (**, p < 0.05; ***, p < 0.001). (D) The effect of appendage color on lethal temperature in the context of collection location (pLMM = 0.0007, Atlantic = teal, Bay = purple) and acclimation treatment (pLMM = 0.004, gray = ambient, red = elevated) are shown. Box plots show the median value for each group as a thick line; boxes represent the interquartile range and whiskers signify the minimum and maximum values.
To further investigate if there is a relationship between the amount of blue coloration coverage/saturation of pigmented appendages and lethal temperature during an acute heat stress, appendage morphology was quantified as the number of pixels within the average RGB of blue appendages. A significant, positive correlation was observed between the percentage of blue coverage within a medusa and lethal temperature (Figure 4A, R = 0.39; p = 0.032). Similarly, we found a significant, positive correlation between the adjusted saturation of the appendage and lethal temperature (Figure 4B, R = 0.41, p = 0.024). We however found no significant relationship between the number of pigmented appendages and lethal temperature (Figure 4C, R = 0.26, p = 0.15). Analysis of the acclimation treatment data revealed positive correlations between percent blue coloration and lethal temperature in medusae acclimated in either temperature treatment, although this effect was stronger in ambient conditions (R = 0.55, p = 0.034), relative to elevated (R = 0.36, p = 0.19, Supplementary Figure 7A). Positive correlations were also present for adjusted saturation (Supplementary Figure 7B). Representative medusa and their corresponding results for blue coverage, adjusted saturation, number of appendages, RGB and HSB are shown in Figure 4D.
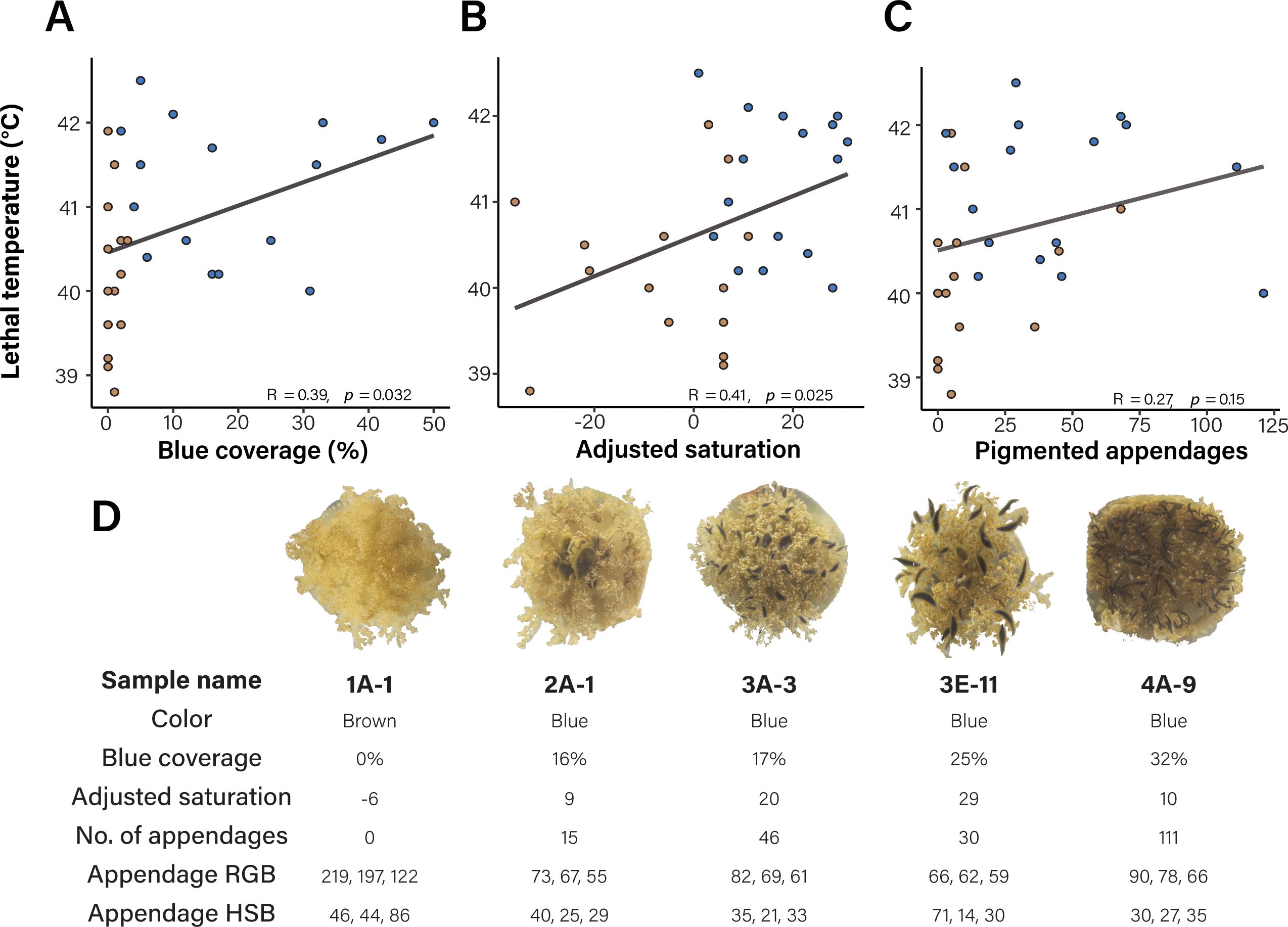
Figure 4. Appendage coverage and saturation correlate with survival during acute heat stress. Blue appendage coverage (A, R = 0.39; p = 0.032), and adjusted saturation (B, R = 0.41, p = 0.024) had a positive and significant association with lethal temperature in medusa subjected to an acute heat stress, while number of appendages (C) was not significant (R = 0.26, p = 0.15). Points represent individual medusae and are colored according to their manual color classification (either brown or blue), lines represent the Pearson correlation between lethal temperature and appendage metric. (D) Representative medusae with distinct morphologies are shown. For each medusa, the color morph, blue coverage, adjusted saturation values, number of pigmented appendages, RGB and HSB scores are indicated.
4 Discussion
Here, we present results that highlight how temperature regimes and coloration shape the ability of upside-down jellyfish (Cassiopea xamachana) to respond to thermal stress, revealing mechanisms underlying the remarkable resilience of this species. We find that animals with different environmental history, specifically differences in daily temperature range, do not exhibit variation in their thermal stress responses. However, short-term acclimation and the presence of blue appendages was associated with enhanced survival in C. xamachana. Ultimately, these findings highlight a novel link between blue coloration and resilience in this marine invertebrate.
4.1 Temperature influences the rate of bell pulsation in Cassiopea xamanchana
Among symbiotic marine cnidarians, Cassiopea appears to be uniquely capable of thriving in a range of environmental conditions (Purcell, 2012; Toullec et al., 2024). Specifically, C. andromeda exhibits exceptional tolerance to repeated, intense (26–34°C) heat stress episodes (Banha et al., 2020; Béziat and Kunzmann, 2022). Here, using rates of bell pulsation as a measure of organismal physiology, we find that C. xamachana maintain homeostatic bell pulsation over a broad, but ecologically-relevant, temperature range (26–34°C). This contrasts with several species of tropical cnidarians in which bell pulsation rates change dramatically beyond a narrow temperature range (Béziat and Kunzmann, 2022; Gatz et al., 1973). In Cassiopea sp., bell pulsations create water currents that facilitate food capture (Gohar and Eisawy, 1960; Hamlet et al., 2011; Klein et al., 2016) and oxygenate the surrounding water (Rowe et al., 2022). In the context of temperature stress, increased rates of bell pulsation may serve as a mechanism to enhance metabolic processes during stress (Fitt et al., 2021; Mangum et al., 1972; McClendon, 1917). This phenomenon has been observed in other marine invertebrates responding to acute temperature stresses (e.g., crayfish, Ern et al., 2015; gastropods, Valles-Regino et al., 2022). In response to temperature fluctuations, Cassiopea sp. exhibit increased superoxide dismutase (SOD) activity (McCord and Fridovich, 1969), signs of oxidative stress (Regoli and Giuliani, 2014), and altered metabolic rates (Aljbour et al., 2019; Fridovich, 1973; Lesser, 2006; McCord and Fridovich, 1969; Regoli and Giuliani, 2014). Stress conditions, such as increased temperature beyond physiological limits, may increase the demand for metabolic energy and, in turn, increase reactive oxygen species (ROS) (Boveris and Chance, 1973; Speakman and Selman, 2011). This ability to overcome oxidative stress may underlie C. xamachana resilience to thermal stress. Future work should aim to clarify the physiological and metabolic benefits or costs of increasing bell pulsation and how this contributes to the remarkable thermotolerance of C. xamachana.
4.2 Environmental history does not shape how C. xamachana respond to acute heat stress
It has been proposed that high environmental variability can result in increased phenotypic plasticity (Lande, 2014). This theory is supported by several empirical studies in marine invertebrates, demonstrating that organisms living in fluctuating environments (e.g., drastic fluctuations in temperature or salinity) exhibit increased resistance to temperature stress associated with climate change (Burton et al., 2022; Godefroid et al., 2023; Kapsenberg and Cyronak, 2019; Rivest et al., 2017; Safaie et al., 2018). In contrast, here we demonstrate that C. xamachana collected from a site with high daily fluctuations in temperature exhibit the same physiological (bell pulsation rate) and survival (lethal temperature) response during heat stress than individuals collected from a site with stable daily temperatures. Similar experiments in other cnidarian species have produced contradicting results. For example, temperature fluctuations on daily or tidal timescales were suggested to be sufficient to promote thermal tolerance of some corals via acclimation, while high temperatures were short enough to avoid lethality (Castillo and Helmuth, 2005; Oliver and Palumbi, 2011; Safaie et al., 2018). However, the effect of temperature variability on heat tolerance is likely species-specific (Putnam and Edmunds, 2011; Schoepf et al., 2022; Voolstra et al., 2020).
Here we show that C. xamanchana are robust to thermal stress, and that this was not likely explained by inhabiting environments with variable daily temperatures. Several potential factors may contribute to this result. First, the temperature fluctuations between the two sites may not be sufficiently different to elicit distinct physiological effects. Corals from habitats such as the back-reef pools in American Samoa experience daily temperature fluctuations up to 5.6°C, even reaching daily extremes of >35°C (Barshis et al., 2010); whereas the nearby, less-variable forereef experiences seasonal maximum daily temperature fluctuations of 1.8°C (Barshis et al., 2018; Craig et al., 2001). These corals have phenotypic differences consistent with local adaptation of thermal performance (Palumbi et al., 2014). Similarly, either the temperature or the duration of daily maximum temperature in the Atlantic site may be insufficient to induce increased thermal tolerance (either via acclimation or local adaptation). While our COI data revealed that all animals used in this study are C. xamanchana (consistent with Muffett and Miglietta, 2023), these data are unable to resolve population-level differences or assess local adaptation. Thus, it is possible that C. xamachana sampled from both sites represent the same population and are thus robust to stable and fluctuating temperatures regardless of the site they are found. These results are consistent with the overall observed robustness of C. xamachana relative to other marine invertebrates (Aljbour et al., 2019; Banha et al., 2020; Béziat and Kunzmann, 2022; Fitt et al., 2021; McGill and Pomory, 2008; Medina et al., 2021; Ohdera et al., 2018; Rowe et al., 2022). In fact, Cassiopea sp. thrive in human-disturbed environments (Stoner et al., 2011, 2016) and are expanding their habitat range. Our results may therefore reflect the underlying thermal tolerance of C. xamachana rather than environmental conditions of specific collection sites.
4.3 Acclimation allows C. xamachana to survive elevated temperatures
As oceans warm for more prolonged periods due to global change, marine organisms must adjust their physiology to tolerate greater extremes (Coumou and Rahmstorf, 2012; Lopez et al., 2018; Morley et al., 2019). Short-term temperature stress can lead to subsequent increases in thermal tolerance in cnidarians (Baker et al., 2008; Kenkel and Matz, 2016; Kirk et al., 2018). The work presented here indicates that acclimation to a warmer environment may promote survival under acute heat stress and thus may prepare C. xamachana medusae for future warming conditions. Given the rapid heating of shallow water environments, C. xamachana may have an advantage if previous warming allows them to acclimate; it is possible that the previously encountered temperature “extreme” in our acclimation experiment could have buffered the effect of the acute heat stress, thereby allowing them to survive to higher lethal temperatures (i.e., stress memory; Crisp et al., 2016; Li et al., 2020).
These results, while novel for C. xamachana., are not uncommon among marine ectotherms. In natural experiments, corals of the Great Barrier Reef pre-exposed to thermal stress exhibited protection from subsequent heat waves (Ainsworth et al., 2016; DeMerlis et al., 2022). It is hypothesized, that an organismal thermal threshold might be a plastic trait, in which some individuals are able to extend the upper thermal limit over time (Buckley and Huey, 2016; Leung et al., 2021). These observations are consistent with the laboratory-based experiments presented here and by others investigating cnidarian thermal tolerance (Ainsworth et al., 2016; Bay and Palumbi, 2015; Bellantuono et al., 2012a, 2012b; Middlebrook et al., 2008). Cassiopea appear to be resilient to temperature stress, which may be the result of a hardening capacity, or the ability to increase thermotolerance due to experiencing a prior non-lethal temperature stress (Sgrò et al., 2010). The results presented here emphasize Cassiopea’s ability to acclimate to warming temperatures but may also predict their invasion potential and survival in more diverse environments. Some studies have already investigated Cassiopea’s invasion into more temperate environments (Rowe et al., 2022), however it is possible, due to their ability to acclimate, they may expand their range into warmer habitats.
4.4 Appendage color is associated with survival in warming environments
How temperature-dependent processes differ among color morphs could impact species survival and influence how polymorphisms evolve and/or persist (Thompson et al., 2023). In the present study, we observed differences in C. xamachana response to heat stress in medusae containing blue appendages compared to those lacking blue appendages. This finding was consistent across two years of experimentation using individuals collected from different sites, which demonstrates the strength of our hypothesis that pigmented appendages may benefit C. xamachana during extreme heating events. This is the first record of appendage color contributing to fitness traits in C. xamachana. While blue appendage color is common among some Cassiopea species, other color morphs are also common, including red, blue, purple, green, brown, and white (Lampert et al., 2012). Cassiopea sp. also vary dramatically in the level of pigmentation, appendage shape and appendage number (Lampert et al., 2012; Rowe et al., 2022). Color has been associated with camouflage, sexual selection, and social interactions in many organisms (Cuthill et al., 2017), although these roles are unlikely in Cassiopea. Given their habitat (shallow, clear seawater), and ecology (epibenthic, with brown coloration mimicking the substrate), bright blue coloration makes individuals more obvious. Additionally, Cassiopea males freely release sperm into the seawater and females brood larvae and therefore do not undergo coloration-based mate selection. Based on the results presented here, we hypothesize that the Cassio Blue protein provides Cassiopea with an advantage in thermal stress conditions at the level of cellular physiology.
Several hypotheses on the function of Cassio Blue have been proposed. One possibility is that Cassio Blue facilitates optimal photosynthesis via mitigation of solar radiation (Phelan et al., 2006), although Cassio Blue pigmentation does not strongly co-localize in tissue with photosymbionts. Other chromoproteins have been proposed to absorb excess light and thus optimize the environment for algal symbionts (Ferreira et al., 2023; Salih et al., 2000; Smith et al., 2013). It has also been hypothesized that the pigmented areas in Cassiopea provide a refuge for the symbiotic algae and that certain colors correlate with specific algal strains. However, data presented here and by others (Lampert et al., 2012) indicate that this is not the case. Different color morphs harbor identical symbiont communities (Supplementary Figure 2), and symbionts were present in both pigmented and non-pigmented tissues. Furthermore, an ortholog of Cassio Blue is present in the blue jellyfish, Rhizostoma pulmo, which does not harbor symbionts, making it likely that the primary role for this protein is related to host, rather than symbiont, physiology (Bulina et al., 2004; Lawley et al., 2021).
The Cassio Blue protein is composed of Frizzled and Kringle domains, neither of which have been previously associated with pigmentation or thermal stress responses (Lawley et al., 2021). However, based on physiological roles for fluorescent proteins in other cnidarians and chemical properties of Cassio Blue, we propose two hypotheses for how this protein may promote survival in heat stress. In Cassiopea sp., heat stress induces signs of oxidative stress (Aljbour et al., 2019) and increased production of reactive oxygen species (ROS). To attenuate potential damage from ROS, some cnidarians express proteins with antioxidant properties. For example, fluorescent proteins expressed in several species of scleractinian corals serve as scavengers for ROS (Palmer et al., 2008). As an alternate hypothesis, Cassio Blue is associated with transition metals, including Cu, Fe, and Zn (Phelan et al., 2006). It is therefore possible that this protein serves as an important reservoir for these trace metals, which are required for metabolic processes such as the protein synthesis and enzyme activity needed for physiological acclimation (Reich et al., 2021). Metals have also been implicated in several aspects of coral physiology, specifically relating to the ability of algal symbionts to photosynthesize (Ferrier-Pagès et al., 2018; Reich et al., 2021, 2023). Symbiodiniaceae have higher metal requirements to initiate electron transfer between photosystems (Camp et al., 2022; Raven et al., 1999; Reich et al., 2020; Rodriguez et al., 2016). These hypotheses are not mutually exclusive; Cassio Blue may be involved in both functions. Future work will be necessary to examine the mechanistic role of Cassio Blue in C. xamachana physiology.
Trade-offs between fitness benefits and energetic costs of producing pigmentation may impact organismal physiology (Calsbeek et al., 2010), however these factors may not be relevant during acute heat stress in Cassiopea. In some organisms, selection pressures for coloration may result in variation in color traits (Zajitschek et al., 2012). However, in C. xamachana, blue appendages do not appear to influence whole-animal performance traits, such as bell pulsation, but may instead contribute to overall survival through alternative mechanisms (Husak and Fox, 2008; Irschick et al., 2008; Zajitschek et al., 2012). Blue color in many organisms can be achieved by combining a chromophore with an apoprotein (Bulina et al., 2004), which is not a common phenomenon due to the complex structure of blue pigments. Although the chromophore for Cassio Blue has not yet been identified, it is likely that the benefits of blue pigmentation outweigh the potential costs of producing the protein.
Color and fluorescent proteins are extremely common in Cnidaria and have been associated with immunity functions, growth enhancement, and photoprotection (D’Angelo et al., 2012; Palmer et al., 2008, 2009; Salih et al., 1997; Smith et al., 2013). While functional studies on blue pigmentation in Cassiopea sp. have been somewhat limited, considerable insights can be gained from work in corals. Populations of the stony coral Montastraea cavernosa, which have green and red color morphs, possess the same gene, but exhibit differences in mRNA abundance between morphs (Ferreira et al., 2023; Kelmanson, 2003). According to a study by Gittins et al. (2015), copy number and expression level of the RFP gene was found to be an important factor in the intensity of red fluorescence in Acropora millepora (Ferreira et al., 2023). Like what has been studied in corals (Bollati et al., 2022; Dove, 2004; Lyndby et al., 2016; Salih et al., 2000), Cassiopea sp. appendage colors other than blue could generate different light microenvironments, which may be important for photoprotection in shallow waters (Lyndby et al., 2023).
5 Conclusions
In the present study, we assessed the impact of environmental history, temperature acclimation and appendage color on the rate of bell pulsation and survival during an acute heat stress in Cassiopea jellyfish. Overall, we demonstrate that C. xamachana medusae increase bell pulsation rates during an acute heat stress until temperatures reach ~37°C, at which point the rates sharply decline as the animals approach lethality. Surprisingly, we found that environmental history did not affect bell pulsation or survival, but pre-conditioning C. xamachana to warmer temperatures enables acclimation and survival to more extreme temperatures. Finally, our data show for the first time that the presence of blue appendages corresponds to survival of C. xamachana under warming climates. Together, these data hint at novel physiological mechanisms controlling the organismal response to thermal stress. Understanding the mechanisms that allow species to survive environmental stressors has become increasingly important as climate change continues to threaten marine taxa. This work contributes to our broader understanding of how phenotypic plasticity and color variability can shape organismal survival.
Data availability statement
The datasets generated and analyzed for this study can be found in the GitHub repository: https://github.com/mem0294/Maloneyetal2024.
Ethics statement
The manuscript presents research on animals that do not require ethical approval for their study.
Author contributions
MM: Data curation, Formal analysis, Investigation, Methodology, Visualization, Writing – original draft, Writing – review & editing, Validation. KB: Conceptualization, Funding acquisition, Investigation, Project administration, Resources, Supervision, Validation, Visualization, Writing – review & editing. MS: Conceptualization, Funding acquisition, Investigation, Project administration, Resources, Supervision, Writing – review & editing.
Funding
The author(s) declare financial support was received for the research, authorship, and/or publication of this article. Funding was secured via KMB and MES through Auburn University Department of Biological Sciences start up.
Acknowledgments
We thank Emily Wilkins, Sammy Kutsch, Sarah Leinbach Cole Moquin, and Andrew Meyer for field/lab support, and Dr. Bill Fitt for housing support in the Florida Keys. Research and collection was completed under permits issued by Florida Fish and Wildlife.
Conflict of interest
The authors declare that the research was conducted in the absence of any commercial or financial relationships that could be construed as a potential conflict of interest.
Publisher’s note
All claims expressed in this article are solely those of the authors and do not necessarily represent those of their affiliated organizations, or those of the publisher, the editors and the reviewers. Any product that may be evaluated in this article, or claim that may be made by its manufacturer, is not guaranteed or endorsed by the publisher.
Supplementary material
The Supplementary Material for this article can be found online at: https://www.frontiersin.org/articles/10.3389/fevo.2024.1409379/full#supplementary-material
References
Aihara Y., Maruyama S., Baird A. H., Iguchi A., Takahashi S., Minagawa J. (2019). Green fluorescence from cnidarian hosts attracts symbiotic algae. Proc. Natl. Acad. Sci. 116, 2118–2123. doi: 10.1073/pnas.1812257116
Ainsworth T. D., Heron S. F., Ortiz J. C., Mumby P. J., Grech A., Ogawa D., et al. (2016). Climate change disables coral bleaching protection on the Great Barrier Reef. Science 352, 338–342. doi: 10.1126/science.aac7125
Aljbour S. M., Zimmer M., Al-Horani F. A., Kunzmann A. (2019). Metabolic and oxidative stress responses of the jellyfish Cassiopea sp.to changes in seawater temperature. J. Sea Res. 145, 1–7. doi: 10.1016/j.seares.2018.12.002
Arai M. N. (1996). A Functional Biology of Scyphozoa, 1st ed. (Dordrecht Netherlands: Springer). doi: 10.1007/978-94-009-1497-1
Arai Y., Gotoh R., Yokoyama J., Sato C., Okuizumi K., Hanzawa N. (2017). Phylogenetic relationships and morphological variations of upside-down jellyfishes, Cassiopea spp. inhabiting Palau Islands. Biogeography 19, 133–141. doi: 10.11358/biogeo.19.133
Baker A. C., Glynn P. W., Riegl B. (2008). Climate change and coral reef bleaching: An ecological assessment of long-term impacts, recovery trends and future outlook. Estuarine Coast. Shelf Sci. 80, 435–471. doi: 10.1016/j.ecss.2008.09.003
Banha T. N. S., Mies M., Güth A. Z., Pomory C. M., Sumida P. Y. G. (2020). Juvenile Cassiopea andromeda medusae are resistant to multiple thermal stress events. Mar. Biol. 167, 173. doi: 10.1007/s00227-020-03792-w
Barshis D. J., Birkeland C., Toonen R. J., Gates R. D., Stillman J. H. (2018). High-frequency temperature variability mirrors fixed differences in thermal limits of the massive coral Porites lobata. J. Exp. Biol. 221 (24), jeb188581. doi: 10.1242/jeb.188581
Barshis D. J., Stillman J. H., Gates R. D., Toonen R. J., Smith L. W., Birkeland C. (2010). Protein expression and genetic structure of the coral Porites lobata in an environmentally extreme Samoan back reef: does host genotype limit phenotypic plasticity? Mol. Ecol. 19, 1705–1720. doi: 10.1111/J.1365-294X.2010.04574.X
Bates D., Mächler M., Bolker B., Walker S. (2015). Fitting linear mixed-effects models using lme4. J. Stat. Software 67 (1), 1–48. doi: 10.18637/jss.v067.i01
Bay R. A., Palumbi S. R. (2015). Rapid acclimation ability mediated by transcriptome changes in reef-building corals. Genome Biol. Evol. 7, 1602–1612. doi: 10.1093/GBE/EVV085
Bayha K. M., Graham W. M. (2014). Nonindigenous Marine Jellyfish: Invasiveness, Invasibility, and Impacts. In: Pitt K., Lucas C. (eds) Jellyfish Blooms. (Dordrecht: Springer). doi: 10.1007/978-94-007-7015-7_3
Bellantuono A. J., Granados-Cifuentes C., Miller D. J., Hoegh-Guldberg O., Rodriguez-Lanetty M. (2012a). Coral thermal tolerance: tuning gene expression to resist thermal stress. PloS One 7, e50685. doi: 10.1371/JOURNAL.PONE.0050685
Bellantuono A. J., Hoegh-Guldberg O., Rodriguez-Lanetty M. (2012b). Resistance to thermal stress in corals without changes in symbiont composition. Proc. R. Soc. B: Biol. Sci. 279, 1100–1107. doi: 10.1098/RSPB.2011.1780
Béziat P., Kunzmann A. (2022). Under pressure: Cassiopea andromeda jellyfish exposed to increasing water temperature or lead, cadmium and anthropogenic gadolinium contamination. Mar. Biol. Res. 18(1–2), 48–63. doi: 10.1080/17451000.2022.2066132
Bollati E., Lyndby N. H., D’Angelo C., Kühl M., Wiedenmann J., Wangpraseurt D. (2022). Green fluorescent protein-like pigments optimise the internal light environment in symbiotic reef-building corals. ELife 11, e73521. doi: 10.7554/eLife.73521
Botero C. A., Weissing F. J., Wright J., Rubenstein D. R. (2015). Evolutionary tipping points in the capacity to adapt to environmental change. Proc. Natl. Acad. Sci. 112, 184–189. doi: 10.1073/pnas.1408589111
Bou-Abdallah F., Chasteen N. D., Lesser M. P. (2006). Quenching of superoxide radicals by green fluorescent protein. Biochim. Biophys. Acta (BBA) - Gen. Subj. 1760, 1690–1695. doi: 10.1016/j.bbagen.2006.08.014
Boveris A., Chance B. (1973). The mitochondrial generation of hydrogen peroxide. General properties and effect of hyperbaric oxygen. Biochem. J. 134, 707–716. doi: 10.1042/bj1340707
Buckley L. B., Huey R. B. (2016). How extreme temperatures impact organisms and the evolution of their thermal tolerance. Integr. Comp. Biol. 56, 98–109. doi: 10.1093/ICB/ICW004
Bulina M. E., Lukyanov K. A., Yampolsky I. V., Chudakov D. M., Staroverov D. B., Shcheglov A. S., et al. (2004). New class of blue animal pigments based on frizzled and kringle protein domains. J. Biol. Chem. 279, 43367–43370. doi: 10.1074/jbc.C400337200
Burton T., Ratikainen I. I., Einum S. (2022). Environmental change and the rate of phenotypic plasticity. Global Change Biol. 28, 5337–5345. doi: 10.1111/gcb.16291
Callahan B. J., McMurdie P. J., Rosen M. J., Han A. W., Johnson A. J. A., Holmes S. P. (2016). DADA2: High-resolution sample inference from Illumina amplicon data. Nat. Methods 13, 581–583. doi: 10.1038/nmeth.3869
Calsbeek B., Hasselquist D., Clobert J. (2010). Multivariate phenotypes and the potential for alternative phenotypic optima in wall lizard (Podarcis muralis) ventral colour morphs. J. Evolutionary Biol. 23, 1138–1147. doi: 10.1111/J.1420-9101.2010.01978.X
Camp E. F., Nitschke M. R., Clases D., Gonzalez de Vega R., Reich H. G., Goyen S., et al. (2022). Micronutrient content drives elementome variability amongst the Symbiodiniaceae. BMC Plant Biol. 22, 184. doi: 10.1186/s12870-022-03512-0
Castillo K. D., Helmuth B. S. T. (2005). Influence of thermal history on the response of Montastraea annularis to short-term temperature exposure. Mar. Biol. 148, 261–270. doi: 10.1007/s00227-005-0046-x
Coumou D., Rahmstorf S. (2012). A decade of weather extremes. Nat. Climate Change 2, 491–496. doi: 10.1038/nclimate1452
Craig P., Birkeland C., Belliveau S. (2001). High temperatures tolerated by a diverse assemblage of shallow-water corals in American Samoa. Coral Reefs 20, 185–189. doi: 10.1007/S003380100159/METRICS
Crisp P. A., Ganguly D., Eichten S. R., Borevitz J. O., Pogson B. J. (2016). Reconsidering plant memory: Intersections between stress recovery, RNA turnover, and epigenetics. Sci. Adv. 2, e1501340. doi: 10.1126/sciadv.1501340
Cuthill I. C., Allen W. L., Arbuckle K., Caspers B., Chaplin G., Hauber M. E., et al. (2017). The biology of color. Science 357, eaan0221. doi: 10.1126/science.aan0221
D’Angelo C., Smith E. G., Oswald F., Burt J., Tchernov D., Wiedenmann J. (2012). Locally accelerated growth is part of the innate immune response and repair mechanisms in reef-building corals as detected by green fluorescent protein (GFP)-like pigments. Coral Reefs 31, 1045–1056. doi: 10.1007/s00338-012-0926-8
DeMerlis A., Kirkland A., Kaufman M. L., Mayfield A. B., Formel N., Kolodziej G., et al. (2022). Pre-exposure to a variable temperature treatment improves the response of Acropora cervicornis to acute thermal stress. Coral Reefs 41, 435–445. doi: 10.1007/S00338-022-02232-Z/TABLES/6
DeWitt T. J., Sih A., Wilson D. S. (1998). Costs and limits of phenotypic plasticity. Trends Ecol. Evol. 13, 77–81. doi: 10.1016/S0169-5347(97)01274-3
Dillon T. M. (1977). Effects of acute changes in temperature and salinity on pulsation rates in ephyrae of the scyphozoan Aurelia aurita. Mar. Biol. 42, 31–35. doi: 10.1007/BF00392011
Dong Y.-W., Ji T.-T., Meng X.-L., Dong S.-L., Sun W.-M. (2010). Difference in thermotolerance between green and red color variants of the Japanese sea cucumber, apostichopus japonicus selenka: hsp70 and heat-hardening effect. Biol. Bull. 218, 87–94. doi: 10.1086/BBLv218n1p87
Dove S. (2004). Scleractinian corals with photoprotective host pigments are hypersensitive to thermal bleaching. Mar. Ecol. Prog. Ser. 272, 99–116. doi: 10.3354/meps272099
Durieux D. M., Du Clos K. T., Lewis D. B., Gemmell B. J. (2021). Benthic jellyfish dominate water mixing in mangrove ecosystems. Proc. Natl. Acad. Sci. 118 (30), e2025715118. doi: 10.1073/pnas.2025715118
Ern R., Huong D. T. T., Phuong N. T., Madsen P. T., Wang T., Bayley M. (2015). Some like it hot: Thermal tolerance and oxygen supply capacity in two eurythermal crustaceans. Sci. Rep. 5, 10743. doi: 10.1038/SREP10743
Ferreira G., Bollati E., Kühl M. (2023). The role of host pigments in coral photobiology. Front. Mar. Sci. 10. doi: 10.3389/fmars.2023.1204843
Ferrier-Pagès C., Sauzéat L., Balter V. (2018). Coral bleaching is linked to the capacity of the animal host to supply essential metals to the symbionts. Global Change Biol. 24, 3145–3157. doi: 10.1111/gcb.14141
Filbee-Dexter K., Wernberg T., Grace S. P., Thormar J., Fredriksen S., Narvaez C. N., et al. (2020). Marine heatwaves and the collapse of marginal North Atlantic kelp forests. Sci. Rep. 10, 13388. doi: 10.1038/s41598-020-70273-x
Fitt W. K., Hofmann D. K., Kemp D. W., Ohdera A. H. (2021). Different Physiology in the Jellyfish Cassiopea xamachana and C. frondosa in Florida Bay. Oceans 2, 811–821. doi: 10.3390/oceans2040046
Fridovich I. (1973). Superoxide dismutases: 877. Available online at: www.annualreviews.org (Accessed February 26, 2024).
Fuller R. C., McGhee K. E., Sandkam B., Schrader M., Travis J. (2022). Polyphenisms and polymorphisms: Genetic variation in plasticity and color variation within and among bluefin killifish populations. Evolution 76, 1590–1606. doi: 10.1111/evo.14511
Gamero-Mora E., Collins A. G., Boco S. R., Geson S. M., Morandini A. C., Wilson N., et al. (2022). Revealing hidden diversity among upside-down jellyfishes (Cnidaria: Scyphozoa: Rhizostomeae: Cassiopea): distinct evidence allows the change of status of a neglected variety and the description of a new species. Invertebrate Systematics 36, 63–89. doi: 10.1071/IS21002
Gatz A. J., Kennedy V. S., Mihursky J. A. (1973). Effects of temperature on activity and mortality of the scyphozoan medusa, chrysaora quinquecirrha. Chesapeake Sci. 14, 171–180. doi: 10.2307/1350603
Gavrilets S., Scheiner S. M. (1993). The genetics of phenotypic plasticity. VI. Theoretical predictions for directional selection. J. Evolutionary Biol. 6, 49–68. doi: 10.1046/J.1420-9101.1993.6010049.X
Ghalambor C. K., McKay J. K., Carroll S. P., Reznick D. N. (2007). Adaptive versus non-adaptive phenotypic plasticity and the potential for contemporary adaptation in new environments. Funct. Ecol. 21, 394–407. doi: 10.1111/J.1365-2435.2007.01283.X
Gittins J. R., D’Angelo C., Oswald F., Edwards R. J., Wiedenmann J. (2015). Fluorescent protein-mediated colour polymorphism in reef corals: multicopy genes extend the adaptation/acclimatization potential to variable light environments. Mol. Ecol. 24, 453–465. doi: 10.1111/mec.13041
Godefroid M., Gouveia A., Otero-Ferrer F., Espino F., Tuya F., Dubois P. (2023). Higher daily temperature range at depth is linked with higher thermotolerance in antipatharians from the canary islands. J. Thermal Biol. 115, 103593. doi: 10.1016/j.jtherbio.2023.103593
Gohar H., Eisawy A. (1960). The development of Cassiopea andromeda (Scyphomedusae). Publications Mar. Biol. Station Al-Ghardaqa 11, 148–190.
Goldstein I., Ehrenreich I. M. (2021). “Genetic variation in phenotypic plasticity,” in Pfennig D.W. (Ed.). Phenotypic Plasticity & Evolution: Causes, Consequences, Controversies (1st ed.). (Boca Raton: CRC Press). doi: 10.1201/9780429343001
Guderley H. (1990). Functional significance of metabolic responses to thermal acclimation in fish muscle. Am. J. Physiol. 259, R245–R252. doi: 10.1152/ajpregu.1990.259.2.R245
Gunderson A. R., Stillman J. H. (2015). Plasticity in thermal tolerance has limited potential to buffer ectotherms from global warming. Proc. R. Soc. B: Biol. Sci. 282, 20150401. doi: 10.1098/rspb.2015.0401
Haddock S. H. D., Dunn C. W. (2015). Fluorescent proteins function as a prey attractant: experimental evidence from the hydromedusa OlIndias formosus and other marine organisms. Biol. Open 4, 1094–1104. doi: 10.1242/bio.012138
Hamlet C., Santhanakrishnan A., Miller L. A. (2011). A numerical study of the effects of bell pulsation dynamics and oral arms on the exchange currents generated by the upside-down jellyfish Cassiopea xamachana. J. Exp. Biol. 214, 1911–1921. doi: 10.1242/JEB.052506
Holland B. S., Dawson M. N., Crow G. L., Hofmann D. K. (2004). Global phylogeography of Cassiopea (Scyphozoa: Rhizostomeae): Molecular evidence for cryptic species and multiple invasions of the Hawaiian Islands. Mar. Biol. 145, 1119–1128. doi: 10.1007/S00227-004-1409-4
Hollingsworth L. L., Kinzie R. A., Lewis T. D., Krupp D. A., Leong J.-A. C. (2005). Phototaxis of motile zooxanthellae to green light may facilitate symbiont capture by coral larvae. Coral Reefs 24, 523–523. doi: 10.1007/s00338-005-0063-8
Hothorn T., Bretz F., Westfall P. (2008). Simultaneous inference in general parametric models. Biometrical Journal 50(3), 346–363. doi: 10.1002/bimj.200810425
Huey R. B., Kearney M. R., Krockenberger A., Holtum J. A. M., Jess M., Williams S. E. (2012). Predicting organismal vulnerability to climate warming: roles of behaviour, physiology and adaptation. Philos. Trans. R. Soc. B: Biol. Sci. 367, 1665–1679. doi: 10.1098/rstb.2012.0005
Hummelinck P. W. (1968). Caribbean scyphomedusae of the genus cassiopea. Stud. Fauna Curaçao Other Caribbean Islands 25, 1–57.
Husak J. F., Fox S. F. (2008). Sexual selection on locomotor performance. Evol. Ecol. Res. 10, 213–228.
Iglesias-Prieto R., Matta J. L., Robins W. A., Trench R. K. (1992). Photosynthetic response to elevated temperature in the symbiotic dinoflagellate Symbiodinium microadriaticum in culture. Proc. Natl. Acad. Sci. 89, 10302–10305. doi: 10.1073/pnas.89.21.10302
Iglesias-Prieto R., Trench R. K. (1997). Acclimation and adaptation to irradiance in symbiotic dinoflagellates. II. Response of chlorophyll-protein complexes to different photon-flux densities. Mar. Biol. 130, 23–33. doi: 10.1007/S002270050221/METRICS
Irschick D. J., Meyers J. J., Husak J. F., Le Galliard J.-F. (2008). How does selection operate on whole-organism functional performance capacities? A review and synthesis. Evol. Ecol. Res. 10, 177–196.
Jardeleza M. G., Koch J. B., Pearse I. S., Ghalambor C. K., Hufbauer R. A. (2022). The roles of phenotypic plasticity and adaptation in morphology and performance of an invasive species in a novel environment. Ecol. Entomology 47, 25–37. doi: 10.1111/een.13087
Jarett J. K., MacManes M. D., Morrow K. M., Pankey M. S., Lesser M. P. (2017). Comparative genomics of color morphs in the coral montastraea cavernosa. Sci. Rep. 7, 16039. doi: 10.1038/s41598-017-16371-9
Kapsenberg L., Cyronak T. (2019). Ocean acidification refugia in variable environments. Global Change Biol. 25, 3201–3214. doi: 10.1111/GCB.14730
Kayal E., Bentlage B., Collins A. G., Kayal M., Pirro S., Lavrov D. V. (2012). Evolution of linear mitochondrial genomes in medusozoan cnidarians. Genome Biol. Evol. 4, 1–12. doi: 10.1093/gbe/evr123
Kelmanson I. V. (2003). Molecular basis and evolutionary origins of color diversity in great star coral montastraea cavernosa (Scleractinia: faviida). Mol. Biol. Evol. 20, 1125–1133. doi: 10.1093/molbev/msg130
Kenkel C. D., Matz M. V. (2016). Gene expression plasticity as a mechanism of coral adaptation to a variable environment. Nat. Ecol. Evol. 1, 1–6. doi: 10.1038/s41559-016-0014
Kirk N. L., Howells E. J., Abrego D., Burt J. A., Meyer E. (2018). Genomic and transcriptomic signals of thermal tolerance in heat-tolerant corals ( Platygyra daedalea) of the Arabian/Persian Gulf. Mol. Ecol. 27, 5180–5194. doi: 10.1111/mec.14934
Klein S. G., Pitt K. A., Carroll A. R. (2016). Reduced salinity increases susceptibility of zooxanthellate jellyfish to herbicide toxicity during a simulated rainfall event. Environ. pollut. 209, 79–86. doi: 10.1016/j.envpol.2015.11.012
Lagerspetz K. Y. H., Vainio L. A. (2006). Thermal behaviour of crustaceans. Biol. Rev. 81, 237. doi: 10.1017/S1464793105006998
LaJeunesse T. C. (2001). INVESTIGATING THE BIODIVERSITY, ECOLOGY, AND PHYLOGENY OF ENDOSYMBIOTIC DINOFLAGELLATES IN THE GENUS SYMBIODINIUM USING THE ITS REGION: IN SEARCH OF A “SPECIES” LEVEL MARKER. J. Phycology 37, 866–880. doi: 10.1046/J.1529-8817.2001.01031.X
LaJeunesse T. C. (2017). Validation and description of Symbiodinium microadriaticum, the type species of Symbiodinium (Dinophyta). J. Phycology 53, 1109–1114. doi: 10.1111/JPY.12570
Lampert K. P., Bürger P., Striewski S., Tollrian R. (2012). Lack of association between color morphs of the Jellyfish Cassiopea andromeda and zooxanthella clade. Mar. Ecol. 33, 364–369. doi: 10.1111/j.1439-0485.2011.00488.x
Lande R. (2014). Evolution of phenotypic plasticity and environmental tolerance of a labile quantitative character in a fluctuating environment. J. Evolutionary Biol. 27, 866–875. doi: 10.1111/JEB.12360
Lang B. J., Donelson J. M., Bairos-Novak K. R., Wheeler C. R., Caballes C. F., Uthicke S., et al. (2023). Impacts of ocean warming on echinoderms: A meta-analysis. Ecol. Evol. 13, e10307. doi: 10.1002/ece3.10307
Lawley J. W., Carroll A. R., McDougall C. (2021). ). Rhizostomins: A novel pigment family from rhizostome jellyfish (Cnidaria, scyphozoa). Front. Mar. Sci. 8. doi: 10.3389/fmars.2021.752949
Lesser M. P. (2006). OXIDATIVE STRESS IN MARINE ENVIRONMENTS: biochemistry and physiological ecology. Annu. Rev. Physiol. 68, 253–278. doi: 10.1146/annurev.physiol.68.040104.110001
Leung J. Y. S., Russell B. D., Coleman M. A., Kelaher B. P., Connell S. D. (2021). Long-term thermal acclimation drives adaptive physiological adjustments of a marine gastropod to reduce sensitivity to climate change. Sci. Total Environ. 771, 145208. doi: 10.1016/j.scitotenv.2021.145208
Li H., Huang X., Zhan A. (2020). Stress memory of recurrent environmental challenges in marine invasive species: ciona robusta as a case study. Front. Physiol. 11. doi: 10.3389/FPHYS.2020.00094/BIBTEX
Lopez H., West R., Dong S., Goni G., Kirtman B., Lee S.-K., et al. (2018). Early emergence of anthropogenically forced heat waves in the western United States and Great Lakes. Nat. Climate Change 8, 414–420. doi: 10.1038/s41558-018-0116-y
Lyndby N. H., Kühl M., Wangpraseurt D. (2016). Heat generation and light scattering of green fluorescent protein-like pigments in coral tissue. Sci Rep 6, 26599. doi: 10.1038/srep26599
Lyndby N. H., Murray M. C., Trampe E., Meibom A., Kühl M. (2023). The mesoglea buffers the physico-chemical microenvironment of photosymbionts in the upside-down jellyfish Cassiopea sp. Front. Ecol. Evol. 11. doi: 10.3389/FEVO.2023.1112742/FULL
Mangum C. P., Oakes M. J., Shick J. M. (1972). Rate-temperature responses in scyphozoan medusae and polyps. Mar. Biol. 15, 298–303. doi: 10.1007/BF00401389/METRICS
Mansour J. S., Pollock F. J., Díaz-Almeyda E., Iglesias-Prieto R., Medina M. (2018). Intra- and interspecific variation and phenotypic plasticity in thylakoid membrane properties across two Symbiodinium clades. Coral Reefs 37, 841–850. doi: 10.1007/s00338-018-1710-1
Matz M. V., Lukyanov K. A., Lukyanov S. A. (2002). Family of the green fluorescent protein: Journey to the end of the rainbow. BioEssays 24, 953–959. doi: 10.1002/BIES.10154
McClendon J. F. (1917). The direct and indirect calorimetry of Cassiopea xamachana: the effect of stretching on the rate of the nerve impulse. J. Biol. Chem. 32, 275–296. doi: 10.1016/S0021-9258(18)86650-9
McCord J. M., Fridovich I. (1969). Superoxide Dismutase: an enzymic function for Erythrocuprein (Hemocuprein). J. Biol. Chem. 244, 6049–6055. doi: 10.1016/S0021-9258(18)63504-5
McGill C. J., Pomory C. M. (2008). Effects of bleaching and nutrient supplementation on wet weight in the jellyfish Cassiopea xamachana (Bigelow) (Cnidaria: Scyphozoa). Mar. Freshw. Behav. Physiol. 41, 179–189. doi: 10.1080/10236240802369899
Medina M., Sharp V., Ohdera A., Bellantuono A., Dalrymple J., Gamero-Mora E., et al. (2021). “The upside-down jellyfish cassiopea xamachana as an emerging model system to study cnidarian–algal symbiosis,” in Handbook of Marine Model Organisms in Experimental Biology (Boca Raton: CRC Press), 149–171. doi: 10.1201/9781003217503-9
Mellin C., Matthews S., Anthony K. R. N., Brown S. C., Caley M. J., Johns K. A., et al. (2019). Spatial resilience of the Great Barrier Reef under cumulative disturbance impacts. Global Change Biol. 25, 2431–2445. doi: 10.1111/gcb.14625
Middlebrook R., Hoegh-Guldberg O., Leggat W. (2008). The effect of thermal history on the susceptibility of reef-building corals to thermal stress. J. Exp. Biol. 211, 1050–1056. doi: 10.1242/JEB.013284
Moran N. A. (1992). The evolutionary maintenance of alternative phenotypes. Am. Nat. 139, 971–989. doi: 10.1086/285369
Morandini A. C., Stampar S. N., Maronna M. M., Da Silveira F. L. (2017). All non-indigenous species were introduced recently? The case study of Cassiopea (Cnidaria: Scyphozoa) in Brazilian waters. J. Mar. Biol. Assoc. United Kingdom 97, 321–328. doi: 10.1017/S0025315416000400
Morley S. A., Peck L. S., Sunday J. M., Heiser S., Bates A. E. (2019). Physiological acclimation and persistence of ectothermic species under extreme heat events. Global Ecol. Biogeography 28, 1018–1037. doi: 10.1111/geb.12911
Muffett K., Miglietta M. P. (2023). Demystifying Cassiopea species identity in the Florida Keys: Cassiopea xamachana and Cassiopea andromeda coexist in shallow waters. PloS One 18, e0283441. doi: 10.1371/JOURNAL.PONE.0283441
Muñoz-Miranda A. L., Iñiguez-Moreno M. (2023). An extensive review of marine pigments: sources, biotechnological applications, and sustainability. Aquat. Sci. 85, 68. doi: 10.1007/s00027-023-00966-8
Nath R. D., Bedbrook C. N., Abrams M. J., Basinger T., Bois J. S., Prober D. A., et al. (2017). The jellyfish cassiopea exhibits a sleep-like state. Curr. Biol. 27, 2984–2990.e3. doi: 10.1016/J.CUB.2017.08.014
Newsome A. G., Culver C. A., van Breemen R. B. (2014). Nature’s palette: the search for natural blue colorants. J. Agric. Food Chem. 62, 6498–6511. doi: 10.1021/jf501419q
O’Brien J., Hayder H., Peng C. (2016). Automated quantification and analysis of cell counting procedures using imageJ plugins. J. Visualized Experiments 117, e54719. doi: 10.3791/54719
Ohdera A. H., Abrams M. J., Ames C. L., Baker D. M., Suescún-Bolívar L. P., Collins A. G., et al. (2018). Upside-down but headed in the right direction: Review of the highly versatile Cassiopea xamachana system. Front. Ecol. Evol. 6. doi: 10.3389/FEVO.2018.00035/BIBTEX
Ohdera A., Ames C. L., Dikow R. B., Kayal E., Chiodin M., Busby B., et al. (2019). Box, stalked, and upside-down? Draft genomes from diverse jellyfish (cnidaria, acraspeda) lineages: Alatina alata (cubozoa), calvadosia cruxmelitensis (staurozoa), and cassiopea xamachana (scyphozoa). GigaScience 8, giz069. doi: 10.1093/gigascience/giz069
Oliver T. A., Palumbi S. R. (2011). Do fluctuating temperature environments elevate coral thermal tolerance? Coral Reefs 30, 429–440. doi: 10.1007/s00338-011-0721-y
Paley A. (2014). Colour polymorphism and its role in stress tolerance in the coral Acropora millepora in the Great Barrier Reef. [The Degree of Doctor of Philosophy]. (Townsville: James Cook University). The Degree of Doctor of Philosophy.
Palmer C. V., Modi C. K., Mydlarz L. D. (2009). Coral fluorescent proteins as antioxidants. PloS One 4, e7298. doi: 10.1371/journal.pone.0007298
Palmer C. V., Mydlarz L. D., Willis B. L. (2008). Evidence of an inflammatory-like response in non-normally pigmented tissues of two scleractinian corals. Proc. R. Soc. B: Biol. Sci. 275, 2687–2693. doi: 10.1098/RSPB.2008.0335
Palumbi S. R., Barshis D. J., Traylor-Knowles N., Bay R. A. (2014). Mechanisms of reef coral resistance to future climate change. Science 344, 895–898. doi: 10.1126/science.1251336
Palumbi S. R., Evans T. G., Pespeni M. H., Somero G. N. (2019). Present and future adaptation of marine species assemblages: DNA-based insights into climate change from studies of physiology, genomics, and evolution. Oceanography 32, 83–93. doi: 10.5670/OCEANOG.2019.314
Parker A. R. (1998). The diversity and implications of animal structural colours. J. Exp. Biol. 201, 2343–2347. doi: 10.1242/jeb.201.16.2343
Phelan M. A., Matta A. J. L., Reyes A. Y. M., Fernando R., Boykins A. R. A., Blanquet A. R. S. (2006). Associations between metals and the blue mesogleal protein of Cassiopea xamachana. Mar. Bio. 149, 307–312. doi: 10.1007/s00227-005-0189-9
Pigliucci M., Schlichting C. (1998). Reaction norms of Arabidopsis. V. Flowering time controls phenotypic architecture in response to nutrient stress. J. Evolutionary Biol. 11 (3), 285–301. doi: 10.1046/j.1420-9101.1998.11030285.x
Pinsky M. L., Eikeset A. M., McCauley D. J., Payne J. L., Sunday J. M. (2019). Greater vulnerability to warming of marine versus terrestrial ectotherms. Nature 569, 108–111. doi: 10.1038/s41586-019-1132-4
Pörtner H.-O., Roberts D. C., Masson-Delmotte V., Zhai P., Tignor M., Poloczanska E., et al. (2019). IPCC special report on the ocean and cryosphere in a changing climate. IPCC Intergovernmental Panel Climate Change: Geneva Switzerland 1, 1–755. doi: 10.1017/9781009157964
Purcell J. (2012). Jellyfish and ctenophore blooms coincide with human proliferations and environmental perturbations. Annu. Rev. Mar. Sci. 4, 209–235. doi: 10.1146/annurev-marine-120709-142751
Putnam H. M., Edmunds P. J. (2011). The physiological response of reef corals to diel fluctuations in seawater temperature. J. Exp. Mar. Biol. Ecol. 396, 216–223. doi: 10.1016/J.JEMBE.2010.10.026
R Core Team. (2023). R: A language and environment for statistical computing. R Foundation for Statistical Computing. Vienna, Austria. Available at: https://www.R-project.org/.
Raven J. A., Evans M. C. W., Korb R. E. (1999). The role of trace metals in photosynthetic electron transport in O2-evolving organisms. Photosynthesis Res. 60, 111–150. doi: 10.1023/A:1006282714942
Regoli F., Giuliani M. E. (2014). Oxidative pathways of chemical toxicity and oxidative stress biomarkers in marine organisms. Mar. Environ. Res. 93, 106–117. doi: 10.1016/J.MARENVRES.2013.07.006
Reich H. G., Camp E. F., Roger L. M., Putnam H. M. (2023). The trace metal economy of the coral holobiont: supplies, demands and exchanges. Biol. Rev. 98, 623–642. doi: 10.1111/brv.12922
Reich H. G., Rodriguez I. B., LaJeunesse T. C., Ho T.-Y. (2020). Endosymbiotic dinoflagellates pump iron: differences in iron and other trace metal needs among the Symbiodiniaceae. Coral Reefs 39, 915–927. doi: 10.1007/s00338-020-01911-z
Reich H. G., Tu W., Rodriguez I. B., Chou Y., Keister E. F., Kemp D. W., et al. (2021). Iron availability modulates the response of endosymbiotic dinoflagellates to heat stress. J. Phycology 57, 3–13. doi: 10.1111/jpy.13078
Rivest E. B., Comeau S., Cornwall C. E. (2017). The role of natural variability in shaping the response of coral reef organisms to climate change. Curr. Climate Change Rep. 3, 271–281. doi: 10.1007/S40641-017-0082-X/FIGURES/2
Rodriguez I. B., Lin S., Ho J., Ho T.-Y. (2016). Effects of trace metal concentrations on the growth of the coral endosymbiont symbiodinium kawagutii. Front. Microbiol. 7. doi: 10.3389/fmicb.2016.00082
Rowe C. E., Keable S. J., Ahyong S. T., Figueira W. F. (2022). Physiological responses of the upside-down jellyfish, Cassiopea (Cnidaria: Scyphozoa: Cassiopeidae) to temperature and implications for their range expansion along the east coast of Australia. J. Exp. Mar. Biol. Ecol. 554, 151765. doi: 10.1016/j.jembe.2022.151765
Safaie A., Silbiger N. J., McClanahan T. R., Pawlak G., Barshis D. J., Hench J. L., et al. (2018). High frequency temperature variability reduces the risk of coral bleaching. Nat. Commun. 9, 1671. doi: 10.1038/s41467-018-04074-2
Salih A., Hoegh-Guldberg O., Cox G. (1997). Photoprotection of symbiotic dinoflagellates by fluorescent pigments in reef corals.
Salih A., Larkum A., Cox G., Kühl M., Hoegh-Guldberg O. (2000). Fluorescent pigments in corals are photoprotective. Nature 408, 850–853. doi: 10.1038/35048564
Santhanakrishnan A., Dollinger M., Hamlet C. L., Colin S. P., Miller L. A. (2012). Flow structure and transport characteristics of feeding and exchange currents generated by upside-down Cassiopea jellyfish. J. Exp. Biol. 215, 2369–2381. doi: 10.1242/jeb.053744
Schaum C. E., Buckling A., Smirnoff N., Yvon-Durocher G. (2022). Evolution of thermal tolerance and phenotypic plasticity under rapid and slow temperature fluctuations. Proc. R. Soc. B 289, 20220834. doi: 10.1098/RSPB.2022.0834
Scheiner S. M. (2018). The genetics of phenotypic plasticity. XVI. Interactions among traits and the flow of information. Evolution 72, 2292–2307. doi: 10.1111/EVO.13601
Schindelin J., Arganda-Carreras I., Frise E., Kaynig V., Longair M., Pietzsch T., et al. (2012). Fiji: an open-source platform for biological-image analysis. Nat. Methods 9, 676–682. doi: 10.1038/nmeth.2019
Schoepf V., Sanderson H., Larcombe E. (2022). Coral heat tolerance under variable temperatures: Effects of different variability regimes and past environmental history vs. current exposure. Limnology Oceanography 67, 404–418. doi: 10.1002/LNO.12000
Seebacher F., White C. R., Franklin C. E. (2015). Physiological plasticity increases resilience of ectothermic animals to climate change. Nat. Climate Change 5, 61–66. doi: 10.1038/nclimate2457
Sgrò C. M., Overgaard J., Kristensen T. N., Mitchell K. A., Cockerell F. E., Hoffmann A. A. (2010). A comprehensive assessment of geographic variation in heat tolerance and hardening capacity in populations of Drosophila melanogaster from eastern Australia. J. Evolutionary Biol. 23, 2484–2493. doi: 10.1111/J.1420-9101.2010.02110.X
Shagin D. A., Barsova E. V., Yanushevich Y. G., Fradkov A. F., Lukyanov K. A., Labas Y. A., et al. (2004). GFP-like proteins as ubiquitous metazoan superfamily: evolution of functional features and structural complexity. Mol. Biol. Evol. 21, 841–850. doi: 10.1093/molbev/msh079
Smith E. G., D’Angelo C., Salih A., Wiedenmann J. (2013). Screening by coral green fluorescent protein (GFP)-like chromoproteins supports a role in photoprotection of zooxanthellae. Coral Reefs 32, 463–474. doi: 10.1007/s00338-012-0994-9
Somero G. N. (2010). The physiology of climate change: how potentials for acclimatization and genetic adaptation will determine ‘winners’ and ‘losers.’. J. Exp. Biol. 213, 912–920. doi: 10.1242/jeb.037473
Speakman J. R., Selman C. (2011). The free-radical damage theory: Accumulating evidence against a simple link of oxidative stress to ageing and lifespan. BioEssays 33, 255–259. doi: 10.1002/BIES.201000132
Stampar S. N., Gamero-Mora E., Maronna M. M., Fritscher J. M., Oliveira B. S. P., Sampaio C. L. S., et al. (2020). The puzzling occurrence of the upside-down jellyfish Cassiopea (Cnidaria: Scyphozoa) along the Brazilian coast: a result of several invasion events? Zoologia 37, 1–10. doi: 10.3897/zoologia.37.e50834
Stoner E. W., Layman C. A., Yeager L. A., Hassett H. M. (2011). Effects of anthropogenic disturbance on the abundance and size of epibenthic jellyfish Cassiopea spp. Mar. pollut. Bull. 62, 1109–1114. doi: 10.1016/j.marpolbul.2011.03.023
Stoner E. W., Sebilian S. S., Layman C. A. (2016). Densidad de zooxantelas presentes en la medusa invertida, Cassiopea xamachana, en los hábitats costeros de Las Bahamas. Rev. Biol. Marina y Oceanografia 51, 203–208. doi: 10.4067/S0718-19572016000100022
Strydom S., Murray K., Wilson S., Huntley B., Rule M., Heithaus M., et al. (2020). Too hot to handle: Unprecedented seagrass death driven by marine heatwave in a World Heritage Area. Global Change Biol. 26, 3525–3538. doi: 10.1111/gcb.15065
Takahashi-Kariyazono S., Sakai K., Terai Y. (2018). Presence–absence polymorphisms of highly expressed FP sequences contribute to fluorescent polymorphisms in acropora digitifera. Genome Biol. Evol. 10, 1715–1729. doi: 10.1093/gbe/evy122
Thompson A., Kapsanaki V., Liwanag H. E. M., Pafilis P., Wang I. J., Brock K. M. (2023). Some like it hotter: Differential thermal preferences among lizard color morphs. J. Thermal Biol. 113, 306–4565. doi: 10.1016/j.jtherbio.2023.103532
Toullec G., Rädecker N., Pogoreutz C., Banc-Prandi G., Escrig S., Genoud C., et al. (2024). Host starvation and in hospite degradation of algal symbionts shape the heat stress response of the Cassiopea-Symbiodiniaceae symbiosis. Microbiome 12, 42. doi: 10.1186/s40168-023-01738-0
Umbers K. D. L. (2013). On the perception, production and function of blue colouration in animals. J. Zoology 289, 229–242. doi: 10.1111/jzo.12001
Valles-Regino R., Mouatt P., Yee L. H., Benkendorff K. (2022). Ocean warming and heat stress impact molecules of keystone significance in a predatory marine gastropod. Front. Mar. Sci. 9. doi: 10.3389/FMARS.2022.830425/BIBTEX
Voolstra C. R., Buitrago-López C., Perna G., Cárdenas A., Hume B. C. C., Rädecker N., et al. (2020). Standardized short-term acute heat stress assays resolve historical differences in coral thermotolerance across microhabitat reef sites. Global Change Biol. 26, 4328–4343. doi: 10.1111/GCB.15148
Warner M. E., Fitt W. K., Schmidt G. W. (1999). Damage to photosystem II in symbiotic dinoflagellates: a determinant of coral bleaching. Proc. Natl. Acad. Sci. United States America 96, 8007–8012. doi: 10.1073/PNAS.96.14.8007
Weller H. I., Westneat M. W. (2019). Quantitative color profiling of digital images with earth mover’s distance using the R package colordistance. PeerJ 7, e6398. doi: 10.7717/peerj.6398
Ye J., Coulouris G., Zaretskaya I., Cutcutache I., Rozen S., Madden T. L. (2012). Primer-BLAST: a tool to design target-specific primers for polymerase chain reaction. BMC Bioinf. 13, 134. doi: 10.1186/1471-2105-13-134
Keywords: Cassiopea, heat stress, blue coloration, acclimation, climate change, bell pulsation
Citation: Maloney ME, Buckley KM and Strader ME (2024) Appendage pigmentation and temperature acclimation correlate with survival during acute heat stress in the upside-down jellyfish, Cassiopea xamachana. Front. Ecol. Evol. 12:1409379. doi: 10.3389/fevo.2024.1409379
Received: 29 March 2024; Accepted: 19 August 2024;
Published: 09 September 2024.
Edited by:
Jose A. Masero, University of Extremadura, SpainReviewed by:
Patrick Buerger, Macquarie University, AustraliaChristine Schnitzler, University of Florida, United States
Copyright © 2024 Maloney, Buckley and Strader. This is an open-access article distributed under the terms of the Creative Commons Attribution License (CC BY). The use, distribution or reproduction in other forums is permitted, provided the original author(s) and the copyright owner(s) are credited and that the original publication in this journal is cited, in accordance with accepted academic practice. No use, distribution or reproduction is permitted which does not comply with these terms.
*Correspondence: Megan E. Maloney, bWVtMDI5NEBhdWJ1cm4uZWR1
†These authors have contributed equally to this work and share last authorship