- State Key Laboratory of Herbage Improvement and Grassland Agro-ecosystems (SKLHIGA), College of Ecology, Lanzhou University, Lanzhou, China
Drought profoundly affects the morpho-physiological responses of desert plants in dryland. To scrutinize the morpho-physiological responses of nitrogen (N)-fixing legumes (Ammopiptanthus mongolicus, Caragana korshinskii), N-fixing non-legumes (Elaeagnus angustifolia, Hippophae rhamnoides), and non-N-fixing plants (Nitraria tangutorum, Haloxylon ammodendron) under varied drought stress levels (75%, 50%, 25% and 5% of soil water holding capacity), a pot experiment was conducted in greenhouse. Following prolonged water deficit, carbon (C) and N stoichiometry, metabolic rates, plant growth, and biomass distribution of unstressed and stressed plants were recorded. Intensified drought significantly reduced stem, root and whole-plant biomass, with no significant changes observed in leaf dry-fresh mass ratio, specific leaf area, intrinsic water use efficiency and root to shoot ratio. However, other traits were impacted differently, reflecting distinct adaptive strategies to drought among three plant functional types (PFTs). Patterns of trait-soil water content (SWC) relationships varied across different PFTs, with N-fixing non-legumes followed by N-fixing legumes displayed greater sensitivity to SWC variations than non-N-fixing plants. This resulted in a shift from a stronger trait-SWC relationship in N-fixing non-legumes and N-fixing legumes to a less correlated relationship in non-N-fixing plants. The diverse responses to drought among PFTs suggest a shift from N limitation to water limitation as SWC decreases.
1 Introduction
Human-induced climate change is anticipated to intensify aridity and the risk of drought, driven by decreased precipitation and/or increased evaporation, which suggest widespread and severe droughts and the expansion of drylands (Dai, 2013; Feng and Fu, 2013). Water is the most limited factor in drylands, when soil water availability reaches critical levels, causing harm to plant morphological characteristics, physiological processes, and even culminating in plant mortality (Ramakrishna and Ravishankar, 2011; Adams et al., 2017; Lauriks et al., 2022). Plant functional traits encompass any measurable morphological, physiological or phenological features at the organ or individual level (Violle et al., 2007). Drought triggers a cascade of adjustments in plant functional traits, indirectly shaping plant performance by impacting plant growth, survival and reproduction (He et al., 2020). In essence, plants adjust their functional traits to adapt the fluctuations in soil water availability (Rodriguez-Alarcon et al., 2022). Over large parts of the earth’s surface, the extent of plant performance is notably controlled by the water supply more than other factors (Kramer and Boyer, 1995; Larcher, 2003; Deng et al., 2006, 2008; Chen et al., 2019; Hu et al., 2021; Sun et al., 2021; Cao et al., 2022; Wei et al., 2022; Xiong et al., 2022). As plants could not escape the dry environment like animals, the impact of drought on plant performance of desert plants throughout their life span is particularly severe (Mukarram et al., 2021).
Drought inflicts significant damage on plant growth and development via various processes (Mukarram et al., 2021), thus, plants must orchestrate series of adjustments, including morphological and physiological responses, to survive in drought while maintaining sufficient resources to sustain the recovery of functions (Morabito et al., 2022; Rowland et al., 2023). Amid periods of water shortage, plants initiate coordinated responses, initially regulating stomatal closure (resulted in typically decline in stomatal conductance, gs) (Bartlett et al., 2016; Trueba et al., 2019; Dayer et al., 2020). This consequently reduces evaporation (evaporation rate, E) to minimize the water loss, but foliar CO2 uptake downregulates simultaneously, leading to a further decline in photosynthesis rate (A) (Yan et al., 2016). Consequently, early stomatal closure may increase leaf intrinsic water use efficiency (iWUE) (Li et al., 2022a). Instantaneous carbon assimilation efficiency (ICAE) calculates the ratio of plant carbon (C) gains to C uptake per unit time, which evaluates capacity of plant C fixation after standardizing plant size and species along soil water gradients, may undergo changes in response to drought (Limousin et al., 2015; Wang et al., 2015). Plant respiration, producing energy for plant growth and tissue maintenance, may increase as a strategy to improve drought tolerance (Huang et al., 2020a, b). Notably, plant growth displays more sensitivity to drought than both photosynthesis and respiration (Muller et al., 2011; Lauriks et al., 2022). Drought manifests as a hindrance to biomass accumulation, reproduction and canopy development (Zang et al., 2020; Jacobs et al., 2021), clearly impeding overall plant growth. Therefore, it remains pivotal for comprehending plant adaptation to arid conditions.
Besides, plants adopt a myriad of adaptive strategies to drought at organ level. Changes in C and nitrogen (N) stoichiometry within tissues reflect as indicators of plant responses to drought (Sardans et al., 2012). For instance, heightened drought conditions lead to an increased leaf C:N of shrubs in the Mediterranean (Sardans et al., 2008a), whereas contrasting reduction were observed in root C:N of Quercus ilex (Sardans et al., 2008b). Moreover, adjustments in biomass partitioning in leaf (new carbon hydrates fixing), stem (plant supporting and material transporting), and root (water and nutrients absorbing) reflect both long-term (evolutionary) and short-term (genotypic or phenotypic) responses of plant to climate change (Poorter et al., 2015; Hanslin et al., 2019; Puglielli et al., 2021; Li et al., 2022b). However, the specific aspects and extent to which organs and whole-plant morphological and physiological traits are affected by the progression of increasing drought stress, remain an open question.
Drought tolerance is of prime importance for desert plants residing in arid habitats, where it stands out as a crucial strategy and frequently used by plant scientists to describe plants that are able to grow in conditions of limited water availability (Proctor and Tuba, 2002; Senapati et al., 2019; Gambetta et al., 2020; Mukarram et al., 2021). Drought tolerance cannot completely mitigate the negative impacts on growth and biomass accumulation, nevertheless, it promotes plant survival during periods of low water availability (Blum, 2005; Correia et al., 2019). In other words, drought tolerance helps plants avoid grave harm, potential death, or even risk of species extinction (Bowles et al., 2021). Researches on diverse responses of drought tolerance among different PFTs are driven by the imperative need to understand drought tolerance strategies, especially in drylands.
Nitrogen is another important limiting factor for plant growth in drylands, which is reported that both inorganic (NH4+ and NO3-) and dissolved amino acids can be directly absorbed by plants (Andersen et al., 2017). Besides taking up N directly from soil, biological nitrogen fixation (BNF) is another major means of N supply (Vance, 2002). The N-fixing symbionts involved in BNF mainly include rhizobial symbioses associating with legumes (family Fabaceae in this study) (Desbrosses and Stougaard, 2011), and Frankia (actinorhizal symbioses) nodulating with actinorhizal plants (family Elaeagnaceae in this study), termed N-fixing non-legumes hereafter (Cooper and Scherer, 2012). Comparison of actinorhizal and leguminous nodules shows that morphology, anatomy, origin, and functioning of nodules are different between these two N-fixing plants categories (Silvester et al., 2008). In other words, there are differences among different N-fixing microbes and plants. However, studies on plant functional traits of N-fixing legumes, N-fixing non-legumes and non-N-fixing plants which are commonly distributed in semi-arid area of north-western China responding to drought are rarely to be compared before.
Here, we integrated measurements related to leaf, stem, root and whole-plant morphological and physiological traits across four levels of soil water availability among three PFTs (N-fixing legumes, N-fixing non-legumes and non-N-fixing plants). In this study, the primary objective was to elucidate the plant drought tolerant mechanisms underlying the responses of seedlings of three PFTs. Findings from this study will add to our knowledge on (1) effects of drought on leaf, stem, root and whole-plant morpho-physiological traits, (2) sensitivity of N-fixing and non-N-fixing plants to soil water availability changes, i.e. if there is a ranking on a stronger coupling between functional traits and soil water content (SWC) in three PFTs. Advancing our understanding of how N-fixing legumes, N-fixing non-legumes and non-N-fixing desert plants respond to drought is important in classifying and characterizing the adaptive capacities of these species to withstand water deficit in drylands, with implications for the survival of desert plants under water stress conditions (Catoni et al., 2013).
2 Materials and methods
2.1 Study site and plant materials
Seedlings acquired from Minqin Desert Experimental Station were cultivated in greenhouse at Yuzhong Experimental Station, Lanzhou University, China (35°56′N, 104°09′E, 1750 m a.s.l.), starting from December 2017 (except Ammopiptanthus mongolicus cultivated from May 2018). Three PFTs (6 woody species, N-fixing legumes: Ammopiptanthus mongolicus, Caragana korshinskii; N-fixing non-legumes: Elaeagnus angustifolia (Bertrand and Lalonde, 1985), Hippophae rhamnoides (Korekar et al., 2012); non-N-fixing plants: Nitraria tangutorum, Haloxylon ammodendron) comprising of first-year seedlings were selected as target plant materials. Each seedling was grown in a plastic pot filled with sand separately without competition.
2.2 Drought treatment and 15N labeling
To clarify the relationship between plant drought stress and varying levels of soil water content, we have referenced the work of Li et al. (2019a, 2019b, 2024) across several studies to provide context for our experimental design. Specifically, Li et al. (2024) analyzed soil samples from 30 locations along a 4500 km east–west transect in the drylands of northern China, varying in aridity index ranged from 0.04 to 0.59, and conduct a laboratory experiment under relative soil water contents of 20%, 40%, and 60%. Complementing this, Li et al. (2019a, 2019b) documented soil water content measurements from the Hexi Corridor and Xinjiang regions within China’s drylands, noting that soil water content varied from 0 to 0.497. Considering these empirical realities and practical constraints of our experimental setup, we opted to conduct our study using the soil water content levels representative of China’s dryland conditions in this study, which are consistent with those described by Li et al. in their researches (Li et al., 2019a, 2019b, 2024). All pots were assigned to one of the four water gradients: 75% soil water holding capacity (SWHC, which absolute water content is 16.7%, marked as control treatment), 50% SWHC (moderate treatment), 25% SWHC (severe treatment) and 5% SWHC (extreme treatment) with 9–15 replicates (variations of replicates were because the death of the specific seedlings) of each water gradient. The drought treatment lasted for at least 3 months before initiating 15N pulse labelling.
15N labeling procedure took place 3–4 hours before separating plants into aboveground and belowground parts (labeling hours were recorded for every plant; Zhang et al., 2020). A stick was used to prick four holes about 1cm away from the stem of a plant and 15cm deep evenly before 1.5mg 15N dissolved in 8ml solution via a syringe injected into the soil. Pots from the same water gradient, one quarter of randomly selected plants were labeled with 15N-KNO3, one quarter with 15N-(NH4)2SO4, one quarter with 13C-15N-Glycine (all three were 99.99 atom% 15N enrichment purchased from Shanghai Chem-Industry Institute), the remaining plants were served as the control treatment and were added with a mixture of 14N-KNO3, 14N-(NH4)2SO4 and 12C-14N-Glycine. For example, when 1.5mg 15N of KNO3 was injected, 1.5mg 14N of (NH4)2SO4 and 1.5mg 14N of Glycine would be injected at the same time for a plant.
15N recovery rate of each N form (NO3-, NH4+, Glycine) from the labeled 15N pool (nmol 15N uptake per gram plant per hour) is calculated as follows:
Where NUR (nmol g-1 h-1) is the plant 15N uptake rate, APE (= atomlabelled% − atomunlabelled%) is the atom% excess (15N atom% difference in plants between the labelled and the unlabeled), Plant [N] (mg g-1) is the whole-plant N content, t (h) is labeling time for plant. N uptake capacity (NUC) is plant total 15N uptake rate, i.e. the sum of plant NUR of NO3-, NH4+, Glycine (Hong et al., 2018).
2.3 Gas exchange measurements and calculation
Whole-plant net photosynthesis and dark respiration were measured using a Li-8100 automated CO2 flux system (LiCor, Nebraska, USA) in a high-lucid organic glass box (0.4×0.4×0.4m; Wang et al., 2015), with triplicates for each pot. The glass box, equipped with two circulating fans, facilitated CO2 concentration variation recorded every 3 minutes during each measurement.
Plants were exposed to sodium lamp (Philips SON-TARGO, Amsterdam, Holland), beyond the glass box, providing a quantum flux density (PAR) of 800–1000 μmol m-2 s-1 for at least 2h to initiate photophosphorylation. Subsequently, plants were placed inside the glass box for the measurement of photosynthetic rate (P). Whole-plant dark respiration (Rdark) was measured approximately after 30 minutes of dark adaption in the dark chamber. Following these measurements, cut the plants in pots at the base of stems to separate the plants into two parts, i.e., aboveground parts (including leaves and stem, termed shoots hereafter) and belowground parts (i.e., roots). Carefully remove soil from the roots to avoid damage to fine roots and root underwent thorough washing with distilled water three times before conducting respiratory measurements on shoots (Rshoot) and roots (Rroot). Afterwards, place the plants in a self-fabricated closed-circuit system which was a cylindrical dark chamber (317.8 cm2 in base area and 4823.9 cm3 in volume) connected to a LI-8100 automated CO2 flux system. Triplicates were recorded for every plant every 3 minutes during the measurement.
Whole-plant ICAE is calculated as follows:
Where ICAE is instantaneous carbon assimilation efficiency, Pnet (Pnet = Pgross + R, nmol s-1, where R = Rshoot + Rroot) is whole-plant net photosynthetic rate per plant, Pgross (Pgross = Rdark + Plight, nmol s-1) is whole-plant gross photosynthetic rate per plant. The unit of gas exchange rate is converted by the formula of gas exchange rate (nmol s-1) = observed gas exchange rate (μmol m-2 s-1)×cross sectional area of the chamber (m2, i.e. 0.16 for glass box and 0.03178 for cylindrical dark chamber in this study)×103 (Wang et al., 2015).gs,A and E were measured at leaf level using a portable open gas-exchange system (LI-6400, LiCor, Lincoln, NE, USA). The gas exchange chamber was supplied with a photosynthetic photon flux density of 1200 μmol m-2 s-1 and equipped with an LED light source.
For iWUE at leaf level, it is calculated as
Where iWUE (μmol mol-1) is intrinsic water use efficiency at leaf level, A (μmol m-2 s-1) is photosynthesis rate at leaf level, gs (mol m-2 s-1) is stomatal conductance.
2.4 Plant and soil sampling
Plant and soil sampling were collected approximately four hours after 15N labeling. After shoot and root respiration measurement, each plant was segmented into components (leaves, stem and root) and measured the fresh mass, and then dried in an oven at 65°C for 48h (to a constant mass), measured the dry mass of components and ground with a Mixer Mill MM400 (Retsch, Haan, Germany). Plant C, N content and 15N atom% were measured using an isotope ratio mass spectrometer coupled with an elemental analyzer (Thermo Fisher Scientific, DELTA VIRMS).
2.5 Statistical analysis
All analyses were carried out using R statistical software v. 4.0.5 (R Core Team, 2021). One-way ANOVA with Tukey’s HSD test (HSD.test function in the agricolae package) was performed to detect the differences in plant traits among N-fixing legumes, N-fixing non-legumes and non-N-fixing plants across SWC gradients by evaluating the pairwise differences at p< 0.05 level. Two-way ANOVA was performed (aov function in the stats package) to test the interaction of SWC and PFTs on plant functional traits at p< 0.05 level.
To further illustrate the different responses of plant traits to drought among N-fixing legumes, N-fixing non-legumes and non-N-fixing plants, correlations between pairwise plant trait and SWC were used separately for each PFT. Relationships between selected plant trait and SWC were tested with linear regression (Couso and Fernandez, 2012). Data of plant traits were log10-transformed to normalize statistical distribution. The figures were generated using the ggplot2 package in R.
3 Results
3.1 Leaf traits responding to drought
Drought significantly altered leaf dry biomass (LDM), leaf fresh biomass (LFM), A, gs and E, with the effects varying among different plant functional types (PFTs) for all leaf traits except gs, while the interaction of PFTs and drought exerted significant influence on leaf dry-fresh mass ratio (LDFR), leaf nitrogen concentration (Leaf [N]) and leaf carbon to nitrogen ratio (Leaf C:N) (Table 1). Drought significantly altered LDM, LFM, Leaf [N], Leaf C:N and E in two N-fixing plants, but not in non-N-fixing plants, while leaf mass fraction (LMF), A and gs only changed with drought in N-fixing non-legumes but no significant changes were observed in N-fixing legumes or non-N-fixing plants. LDFR, specific leaf area (SLA) and iWUE showed insignificant changes along SWC gradients among the three PFTs (Figure 1).
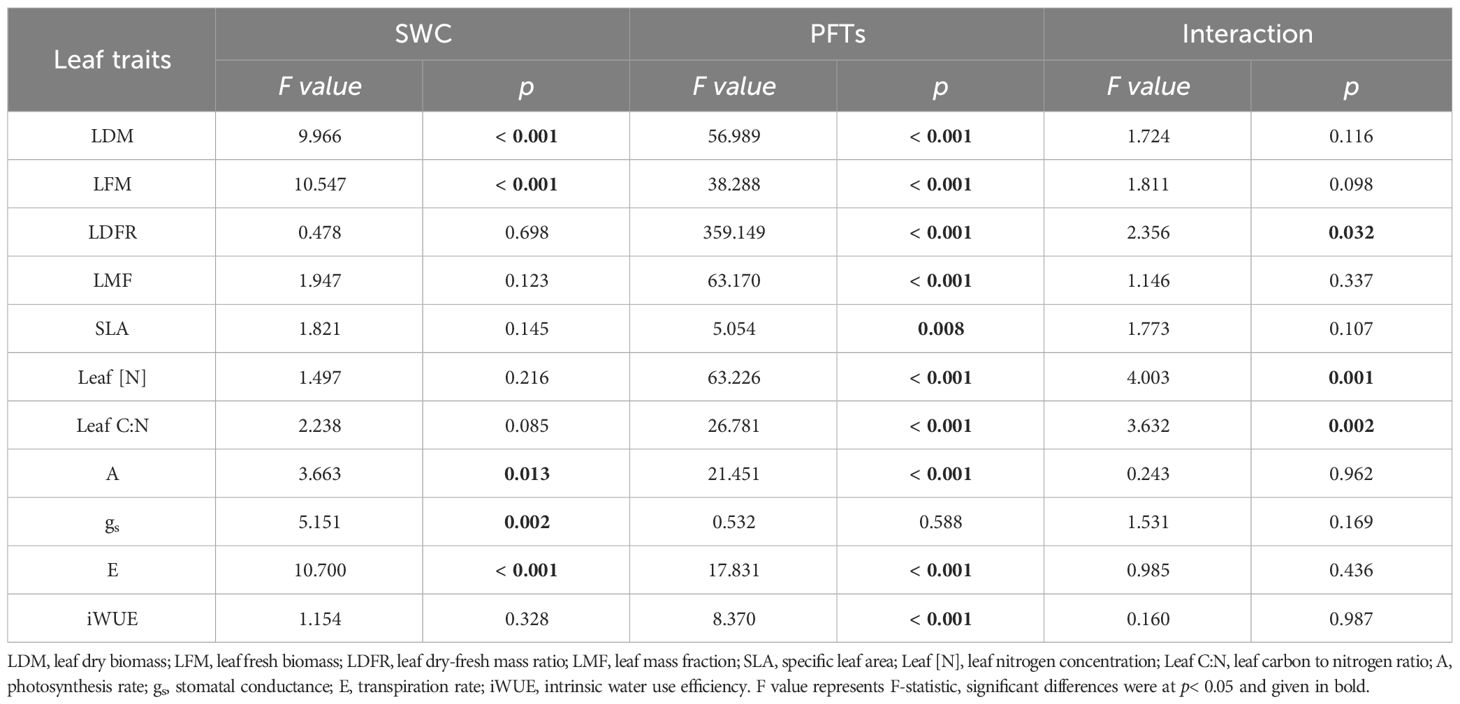
Table 1 Effects of soil water content (SWC), plant functional types (PFTs) and their interactions on leaf traits (F value of two-way ANOVA).
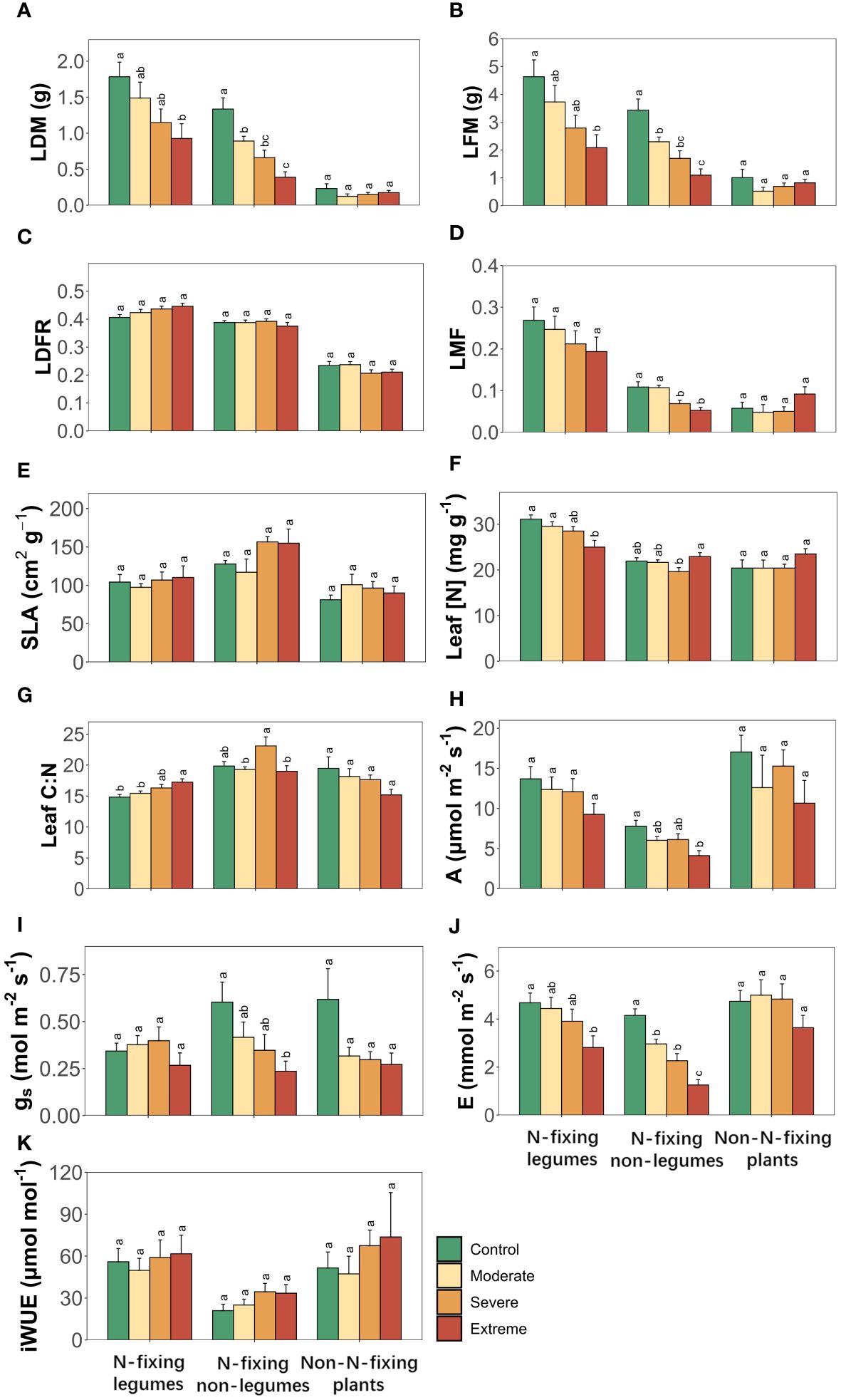
Figure 1 Leaf traits of three plant functional types (N-fixing legumes, N-fixing non-legumes and non-N-fixing plants) responses to drought. (A) Leaf dry biomass, (B) leaf fresh biomass, (C) leaf dry-fresh mass ratio, (D) leaf mass fraction, (E) specific leaf area, (F) leaf nitrogen concentration, (G) leaf carbon to nitrogen ratio, (H) photosynthesis rate, (I) stomatal conductance, (J) transpiration rate, (K) intrinsic water use efficiency. Four soil water gradients (Control, 75% soil water holding capacity (SWHC); Moderate, 50% SWHC; Severe, 25% SWHC; Extreme, 5% SWHC) were distinguished by four different colors. Error bars show SE of mean values. Different letters above error bars indicate significant differences (p< 0.05) among all treatments according to the Tukey’s HSD test.
3.2 Stem traits responding to drought
Drought significantly altered stem dry biomass (SDM), stem fresh biomass (SFM), stem dry-fresh mass ratio (SDFR) and stem nitrogen concentration (Stem [N]), with specific effects being varied to each PFTs for all stem traits, while the combined influence of PFTs and drought was deemed insignificant on all stem traits (Table 2). Drought induced significant alterations in both SDM and SFM of all three PFTs, while Stem [N] showed changes in two N-fixing plants but not in non-N-fixing plants. SDFR only experienced changes in N-fixing legumes while stem mass fraction (SMF) and stem carbon to nitrogen ratio (Stem C:N) were changed in N-fixing non-legumes responding to drought (Figure 2).
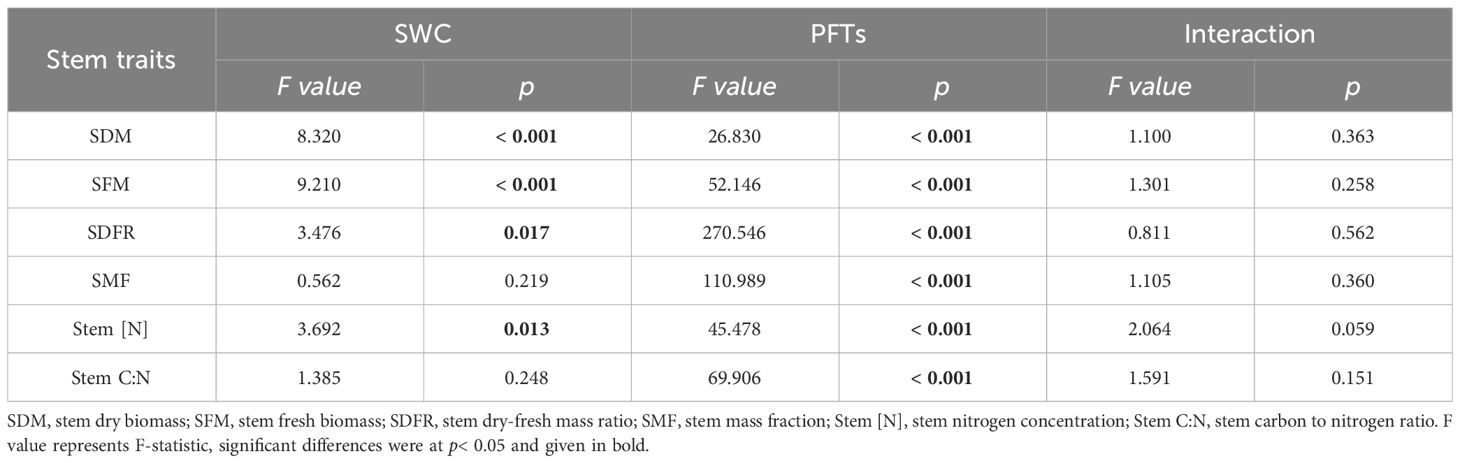
Table 2 Effects of soil water content (SWC), plant functional types (PFTs) and their interactions on stem traits (F value of two-way ANOVA).
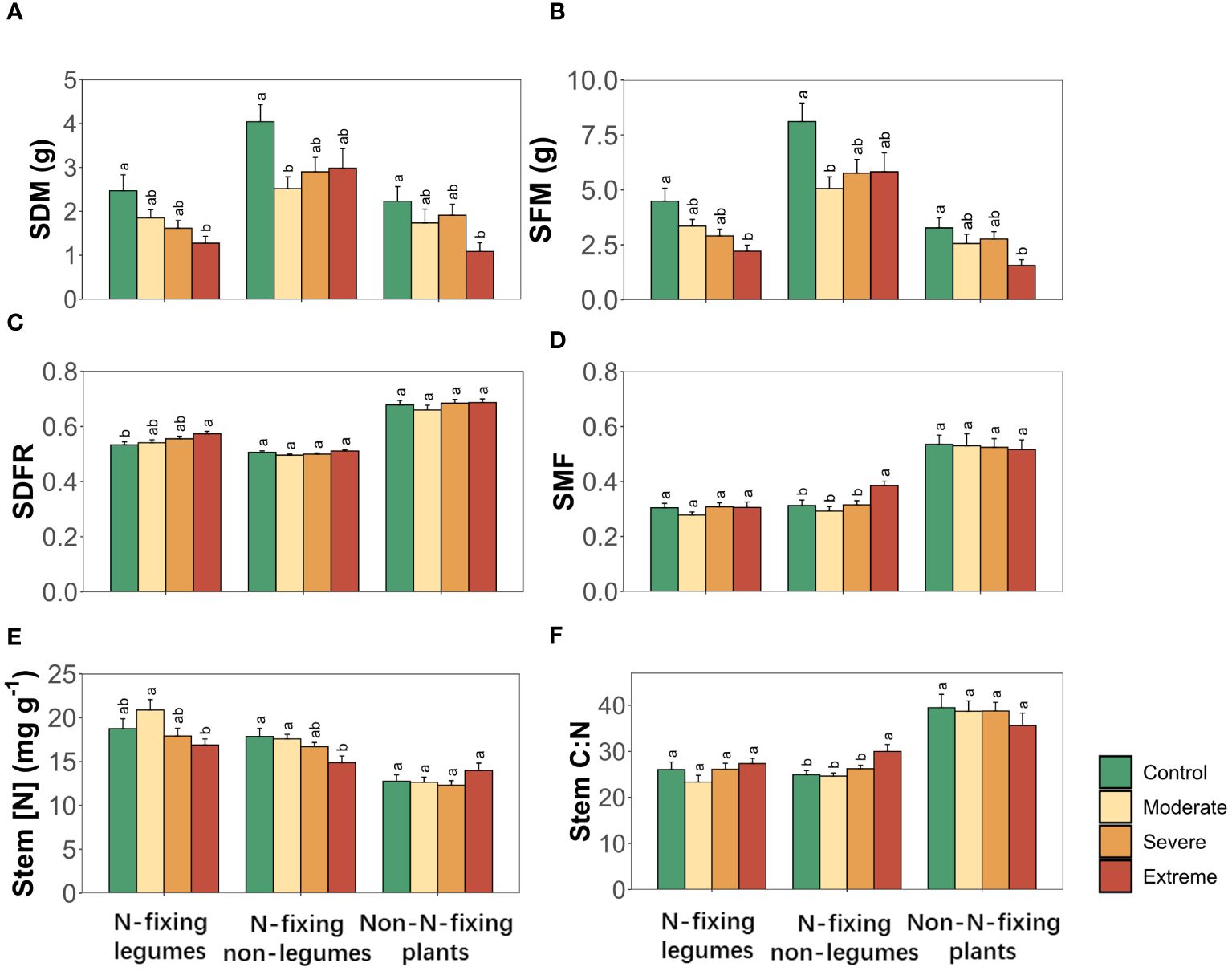
Figure 2 Stem traits of three plant functional types (N-fixing legumes, N-fixing non-legumes and non-N-fixing plants) responses to drought. (A) Stem dry biomass, (B) stem fresh biomass, (C) stem dry-fresh mass ratio, (D) stem mass fraction, (E) stem nitrogen concentration, (F) stem carbon to nitrogen ratio. Four soil water gradients (Control, 75% soil water holding capacity (SWHC); Moderate, 50% SWHC; Severe, 25% SWHC; Extreme, 5% SWHC) were distinguished by four different colors. Error bars show SE of mean values. Different letters above error bars indicate significant differences (p< 0.05) among all treatments according to the Tukey’s HSD test.
3.3 Root traits responding to drought
Drought triggered significant alterations in root dry biomass (RDM), root fresh biomass (RFM), root dry-fresh mass ratio (RDFR) and root nitrogen concentration (Root [N]). These effects were unique to each PFT for all root traits, while the combination of PFTs and drought exerted a significant influence on RDM, RFM, Root [N] and root carbon to nitrogen ratio (Root C:N) (Table 3). The repercussion of drought manifested in significant alterations in both RDM and RFM across all three PFTs. RDFR and Root [N] underwent changes in two N-fixing plants but not in non-N-fixing plants, whereas root mass fraction (RMF) and Root C:N were affected solely in N-fixing non-legumes (Figure 3).
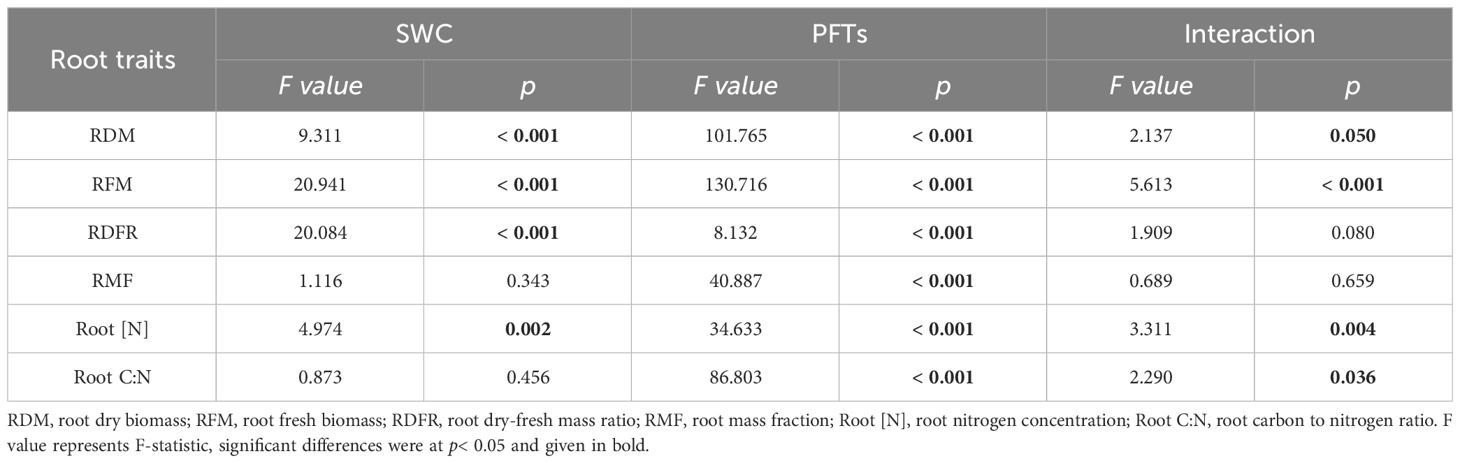
Table 3 Effects of soil water content (SWC), plant functional types (PFTs) and their interactions on root traits (F value of two-way ANOVA).
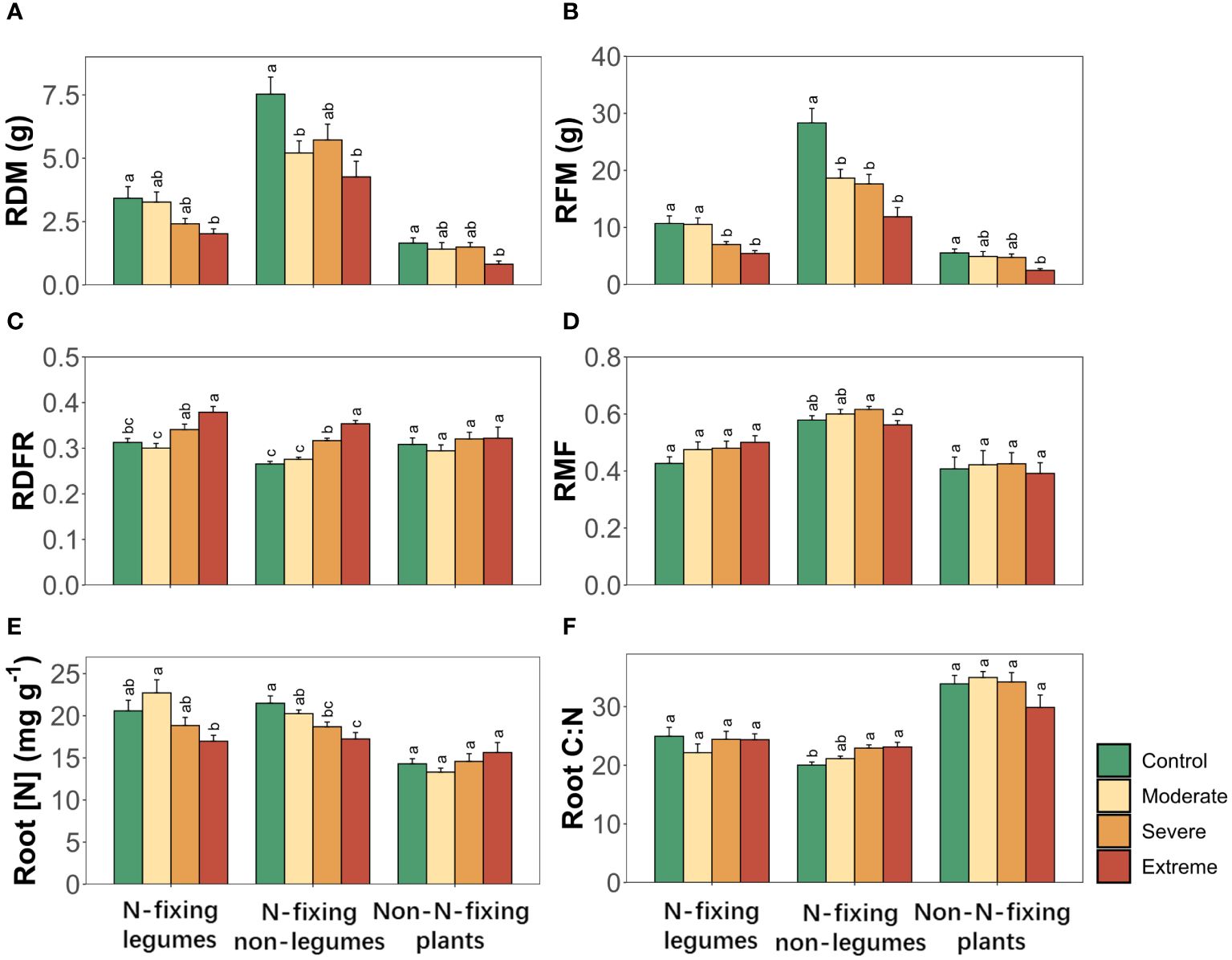
Figure 3 Root traits of three plant functional types (N-fixing legumes, N-fixing non-legumes and non-N-fixing plants) responses to drought. (A) Root dry biomass, (B) root fresh biomass, (C) root dry-fresh mass ratio, (D) root mass fraction, (E) root nitrogen concentration, (F) root carbon to nitrogen ratio. Four soil water gradients (Control, 75% soil water holding capacity (SWHC); Moderate, 50% SWHC; Severe, 25% SWHC; Extreme, 5% SWHC) were distinguished by four different colors. Error bars show SE of mean values. Different letters above error bars indicate significant differences (p< 0.05) among all treatments according to the Tukey’s HSD test.
3.4 Whole-plant traits responding to drought
Drought induced significant modifications in whole-plant dry biomass (TDM), whole-plant fresh biomass (TFM), whole-plant dry-fresh mass ratio (TDFR), Plant [N], whole-plant mass-specific respiration rate (Rmass), ICAE andNUC, with distinct effects to each PFTs for all whole-plant traits. However, the combined influence of PFTs and drought exerted significant influence on TFM, TDFR, Plant [N], whole-plant carbon to nitrogen ratio (Plant C:N), Rmass and NUC (Table 4). Drought induced alterations were also observed in TDM, TFM, Plant [N], Plant C:N and NUC for all three PFTs. Only TDFR changed in two N-fixing plants but not in non-N-fixing plants, while ICAE only displayed changes in N-fixing non-legumes. Rmass, on the other hand, only changed in non-N-fixing plants. Root to shoot ratio (R/S) showed insignificant changes along SWC gradients for all three PFTs (Figure 4).
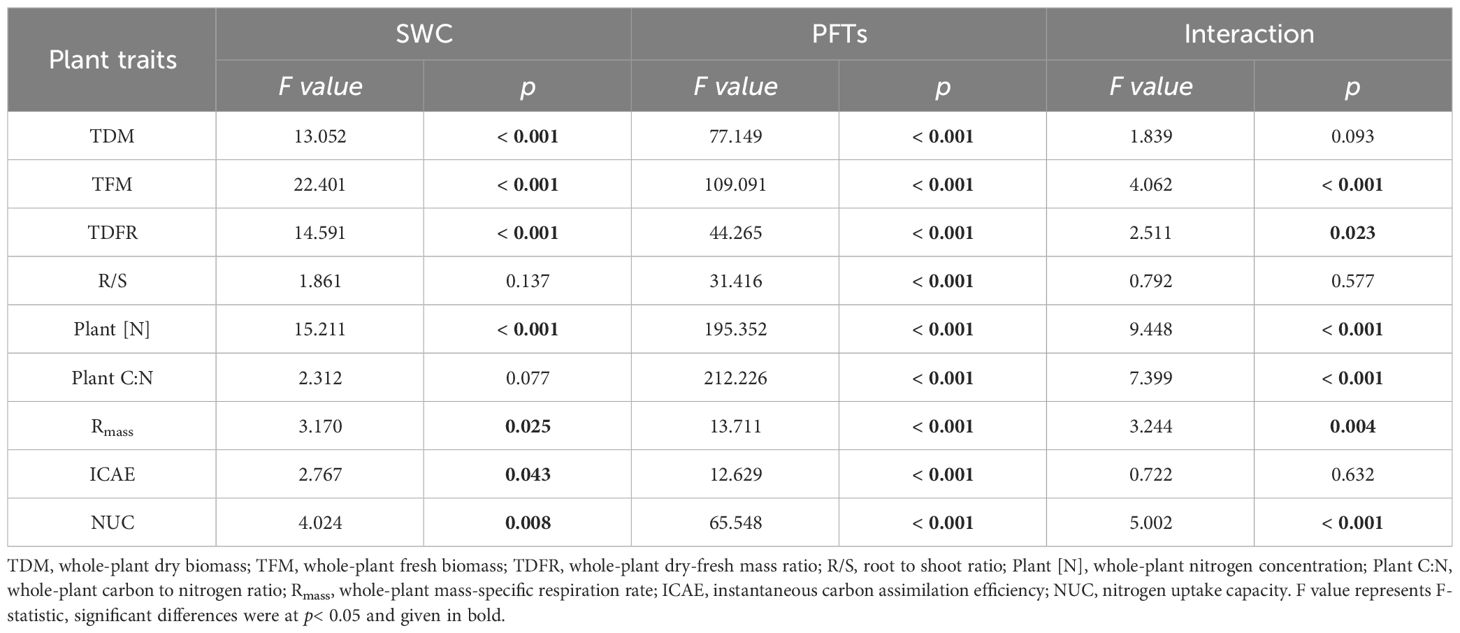
Table 4 Effects of soil water content (SWC), plant functional groups (PFTs) and their interactions on whole-plant traits (F value of two-way ANOVA).
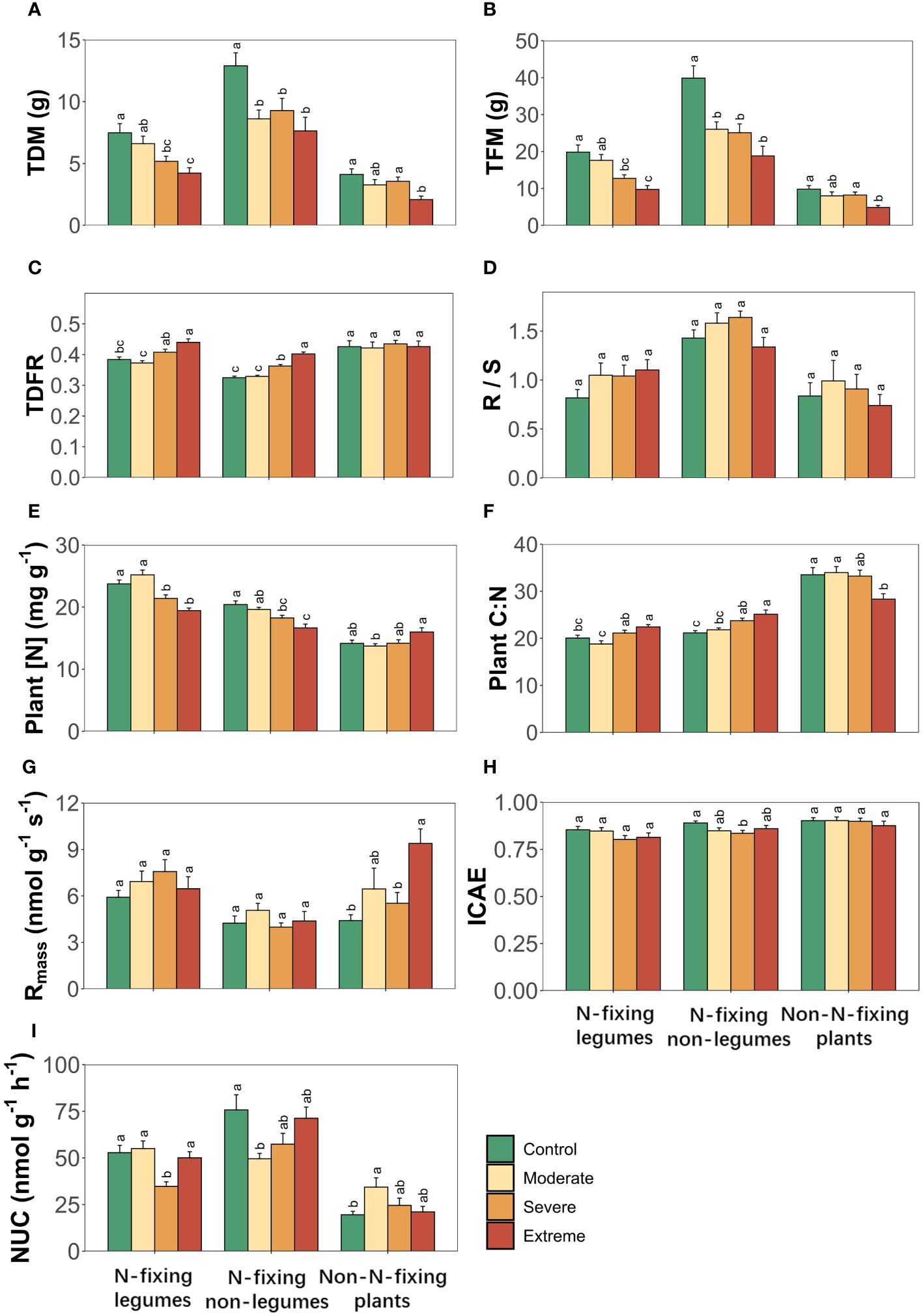
Figure 4 Whole-plant traits of three plant functional types (N-fixing legumes, N-fixing non-legumes and non-N-fixing plants) responses to drought. (A) Whole-plant dry biomass, (B) whole-plant fresh biomass, (C) whole-plant dry-fresh mass ratio, (D) root to shoot ratio, (E) whole-plant nitrogen concentration, (F) whole-plant carbon to nitrogen ratio, (G) whole-plant mass-specific respiration rate, (H) instantaneous carbon assimilation efficiency, (I) nitrogen uptake capacity. Four soil water gradients (Control, 75% soil water holding capacity (SWHC); Moderate, 50% SWHC; Severe, 25% SWHC; Extreme, 5% SWHC) were distinguished by four different colors. Error bars show SE of mean values. Different letters above error bars indicate significant differences (p< 0.05) among all treatments according to the Tukey’s HSD test.
3.5 Variations of trait-SWC relationships among PFTs
In context of leaf traits i.e., LDM and E (Supplementary Figures 1A, J), stem traits i.e., SFM and Stem [N] (Supplementary Figures 2B, E), root traits i.e., RDM, RFM and RDFR (Supplementary Figures 3A–C), and whole-plant traits i.e., TDM, TFM and TDFR (Supplementary Figures 4A–C), the represented absolute slopes of trait-SWC linear regression models in N-fixing non-legumes demonstrated higher values than that in N-fixing legumes, with the lowest values observed in non-N-fixing plants. Similarly, R2 between traits and SWC decreased from N-fixing non-legumes, N-fixing legumes to non-N-fixing plants in LDM, LFM, LMF, A, E, SMF, Stem [N], RDM, RFM, RDFR, Root [N], TFM, TDFR, Plant [N] and Plant C:N (Supplementary Figures 1A, B, D, H, J, 2D, E, 3A–C, E, 4B, C, E, F).
Absolute slopes of trait-SWC linear regression models in N-fixing plants displayed higher values than non-N-fixing plants in LFM, LMF, Root [N], Plant [N], ICAE and NUC (Supplementary Figures 1B, D, 3, 4E, H, I). SLA, gs, SMF and Stem C:N showed greater values in N-fixing non-legumes than non-N-fixing plants, followed by N-fixing legumes in absolute slopes of trait-SWC linear regression models (Supplementary Figures 1E, I, 2D, F). However, LDFR, Leaf [N], SDM, SDFR, RMF and R/S indicated higher values in N-fixing legumes than non-N-fixing plants, followed by N-fixing non-legumes (Supplementary Figures 1C, F, 2A, C, 3, 4D). Notably, for Leaf C:N, A, iWUE, Root C:N, Plant C:N, Rmass, the absolute slopes values in non-N-fixing plants were much greater than N-fixing plants (Supplementary Figures 1G, H, K, 3F, 4F, G).
4 Discussion
4.1 Response of plant traits to drought stress
Plants dynamically adjust their growth and biomass distribution in response to drought conditions. This investigation revealed consistent decreases in the biomass of leaves, stems, roots and whole plants across N-fixing legumes, N-fixing non-legumes and non-N-fixing plants (except LDM and LFM of non-N-fixing plants; Figures 1A, B, 2A, B, 3A, B, 4A, B), in agreement with previous studies (Rodiyati et al., 2005; Gebauer et al., 2020). On the one hand, drought limits biomass accumulation with reduction of SWC which leads to lower nutrients availability (Matías et al., 2011; Usmani et al., 2020). Simultaneously, plants adjust biomass distribution to improve their adaptations to drought (Puglielli et al., 2021). Leaf, stem, root and plant biomass in N-fixing plants were higher than non-N-fixing plants indicating that N supply is the main factor contributing the differences under higher soil water content, however steeply decreasing in biomass of N-fixing plants under lower soil water content in this study. The differences in plant functional types responses to drought suggest a shift from N limitation under sufficient water availability to water limitation under extreme drought (Hofer et al., 2017). In this study, drought stress had a notable impact on the LMF, SMF and RMF of N-fixing non-legumes, but had insignificant effect on R/S of three PFTs and biomass distribution (LMF, SMF, RMF) of N-fixing legumes and non-N-fixing plants (Figures 1–4D). The decline of LMF and the concurrent increase of RMF (particularly evident under extreme treatment compared to control treatment) with drought in N-fixing legumes suggested adaptive adjustments in plant functions and improved ability to obtain limited resources in harsh environment (Freschet et al., 2018; Du et al., 2019). The findings of Tobita et al. (2011) showed R/S of Alnus hirsute (N-fixing non-legumes) responded insignificantly to drought, aligning with the outcomes of this study.
C and N are important nutrients in maintaining plant growth and functionality, with C:N stoichiometry serving as key indicators of plant responses to environmental shifts (Agren, 2008). Results showed that leaf, stem, root and whole-plant nitrogen concentration ([N]) and C:N in N-fixing plants significantly varied (except Stem C:N and Root C:N of N-fixing legumes) with increasing drought severity, while non-N-fixing plants did not changed (except Plant [N] and Plant C:N, Figures 1F, G, 2–4E, F), which illustrated that N concentration and C:N tends to be more stable in non-N-fixing plants compared to their N-fixing counterparts. Leaf [N] and Leaf C:N partly determines plant growth, as changes in protein, chlorophyll and rRNA production influence plant N content because of the cost in plant growth (Agren, 2004; Yan et al., 2019). Drought-induced shifts in plant metabolism may further contribute to changes in N concentration and C:N across different organs and whole plants (Sardans et al., 2012).
Water is a crucial factor in plant growth and closely correlated with photosynthesis and respiration (Huang et al., 2020a, 2020b). The initial response to water deficit is stomatal closure to prevent water loss, leading to a subsequent reduction in transpiration and improvement in water use efficiency. However, this inevitably results in a decrease in photosynthesis due to limited availability of water, CO2 and water deficiency in leaves (Limousin et al., 2015; Yan et al., 2016). In this study, drought significantly decreased A, gs and E of N-fixing non-legumes, only E significantly declined in N-fixing legume, while these parameters remained relatively unchanged in non-N-fixing plants (Figures 1H–J). It indicated non-N-fixing plants are more tolerant to drought compared to N-fixing plants, ensuring maintenance of leaf photosynthesis during drought (Zhao et al., 2020). However, iWUE varied insignificantly among all the three PFTs (Figure 1K), with distinction to Li et al. finding that iWUE was very sensitive to soil moisture variation, suggesting desert plants are most adaptive to arid conditions despite the different PFTs (Li et al., 2022a). This study revealed that drought had no significant effect on the Rmass of N-fixing plants, but led to a rapid increase in non-N-fixing plants (Figure 4G). Plant respiration plays a crucial role in producing energy and C-rich precursors for biomass in physiological processes (Wendering and Nikoloski, 2023). Increasing plant respiration induced by drought may reflect needs of ATP, associated with various physiological aspects like plant growth, cell maintenance, and ion transport, which improved plant tolerance to drought consequently (Atkin and Macherel, 2009; Birami et al., 2020). ICAE allows for the standardized comparison of plant C gains between different PFTs under drought conditions (Limousin et al., 2015). This study found a significant alteration in ICAE for N-fixing non-legumes under drought, but insignificant changes in N-fixing legumes and non-N-fixing plants (Figure 4H).
4.2 Plant sensitivity to drought
Sensitivity of plant traits to drought indicated distinctive responses in leaf, stem, root and whole-plant characteristics among different PFTs (Geng et al., 2012). Drought changes plant morphological and physiological traits, representing a complex of plant adaptation process of different PFTs to drought conditions, often involving a trade-off between trait and fitness (Li et al., 2022c). Some previous studies have shown that increasing drought was more harmful to non-N-fixing plants compared to N-fixing plants (Hofer et al., 2016, 2017). However, our research did not align with this conclusion. Specific significant effects on each PFT for all plant traits (except gs) were observed, demonstrating that drought led to distinct changes in plant traits among three PFTs in this study. In general, the relationship between trait and SWC progressively weakened from N-fixing non-legumes, N-fixing legumes to non-N-fixing plants (Supplementary Figures 1–4). This implied a more sensitive response to decreasing soil water supply in N-fixing non-legumes and N-fixing legumes than non-N-fixing plants. The possible discrepancy between our findings and previous studies may be attributed to the way in which drought treatments were applied, including sustained exposure to low water availability (e.g. decreased precipitation) that may not lead to the climax of biomass loss and persistent severe drought (our study), and the differences between species (Sui et al., 2011; Hofer et al., 2016, 2017). In this study, sharp decline in SWC may rendered N-fixing plants incapable of nodulating and fixing N, potentially leading to plant mortality (Pawlowski and William, 2008; Cooper and Scherer, 2012). This resulted in a substantial reduction in N availability for N-fixing plants, while N availability of non-N-fixing plants remained unaffected by N-fixing microsymbionts, thus N-fixing plants and non-N-fixing plants responded differently to drought stress.
5 Conclusion
Our results underscore that with increasing drought, N-fixing non-legumes, followed by N-fixing legumes, undergo more pronounced changes compared to non-N-fixing counterparts. While there is a little evidence supporting the notion that N-fixing plants display stronger trait-SWC relationships compared to non-N-fixing plants, indicating a potential specialization of N-fixing plants in adapting to escalating drought. The observed trade-off between drought tolerance and trait sensitivity implies that more drought tolerant plant functional group has fewer plastic responses to drought. However, caution is advised in drawing definitive conclusion, particularly when only two species included in each plant functional group, as this may introduce confounding phylogenetic signals that limit the precise quantification of factor effects (Nadia Biruk et al., 2022).
Data availability statement
The datasets presented in this article are not readily available because the raw data supporting the conclusions of this article will be made available by the authors, without undue reservation. Requests to access the datasets should be directed to Jinzhi Ran, cmFuanpAbHp1LmVkdS5jbg==.
Author contributions
DZ: Conceptualization, Data curation, Formal analysis, Investigation, Methodology, Software, Validation, Visualization, Writing – original draft, Writing – review & editing. MW: Data curation, Formal analysis, Investigation, Methodology, Software, Validation, Writing – review & editing. XW: Formal analysis, Methodology, Software, Validation, Writing – original draft. MA: Methodology, Validation, Writing – review & editing. JR: Conceptualization, Project administration, Resources, Supervision, Visualization, Writing – original draft, Writing – review & editing. JD: Conceptualization, Funding acquisition, Project administration, Resources, Supervision, Validation, Visualization, Writing – original draft, Writing – review & editing.
Funding
The author(s) declare financial support was received for the research, authorship, and/or publication of this article. This research was funded by National Key Research and Development Program of China (2023YFF0805600), National Natural Science Foundation of China (32225032, 32001192, 32271597, 42201041), the Innovation Base Project of Gansu Province (2021YFF0703904) and Natural Science Foundation of Gansu Province (21JR1RA138, 22JR5RA525, 23JRRA1157).
Acknowledgments
We thank Lixin Qiao for his assistance in the experiment at Yuzhong Experimental Station, Lanzhou University and Xueyao Zhang for her assistance in the isotopic measurement at Core Facility of the School of Life Sciences, Lanzhou University.
Conflict of interest
The authors declare that the research was conducted in the absence of any commercial or financial relationships that could be construed as a potential conflict of interest.
Publisher’s note
All claims expressed in this article are solely those of the authors and do not necessarily represent those of their affiliated organizations, or those of the publisher, the editors and the reviewers. Any product that may be evaluated in this article, or claim that may be made by its manufacturer, is not guaranteed or endorsed by the publisher.
Supplementary material
The Supplementary Material for this article can be found online at: https://www.frontiersin.org/articles/10.3389/fevo.2024.1407882/full#supplementary-material
References
Adams H. D., Zeppel M. J. B., Anderegg W. R. L., Hartmann H., Landhausser S. M., Tissue D. T., et al. (2017). A multi-species synthesis of physiological mechanisms in drought-induced tree mortality. Nat. Ecol. Evol. 1, 1285–1291. doi: 10.1038/s41559-017-0248-x
Agren G. I. (2004). The C:N:P stoichiometry of autotrophs - theory and observations. Ecol. Lett. 7, 185–191. doi: 10.1111/j.1461-0248.2004.00567.x
Agren G. I. (2008). Stoichiometry and nutrition of plant growth in natural communities. Annu. Rev. Ecol. Evol. Systematics 39, 153–170. doi: 10.1146/annurev.ecolsys.39.110707.173515
Andersen K. M., Mayor J. R., Turner B. L. (2017). Plasticity in nitrogen uptake among plant species with contrasting nutrient acquisition strategies in a tropical forest. Ecology 98, 1388–1398. doi: 10.1002/ecy.1793
Atkin O. K., Macherel D. (2009). The crucial role of plant mitochondria in orchestrating drought tolerance. Ann. Bot. 103, 581–597. doi: 10.1093/aob/mcn094
Bartlett M. K., Klein T., Jansen S., Choat B., Sack L. (2016). The correlations and sequence of plant stomatal, hydraulic, and wilting responses to drought. Proc. Natl. Acad. Sci. United States America 113, 13098–13103. doi: 10.1073/pnas.1604088113
Bertrand L. J., Lalonde M. (1985). In vitro propagation and nodulation by Frankia of actinorhizal Russian Olive (Elaeagnus angustifolia L.). Plant Soil 87, 143–152. doi: 10.1007/BF02277655
Birami B., Naegele T., Gattmann M., Preisler Y., Gast A., Arneth A., et al. (2020). Hot drought reduces the effects of elevated CO2 on tree water-use efficiency and carbon metabolism. New Phytol. 226, 1607–1621. doi: 10.1111/nph.16471
Blum A. (2005). Drought resistance, water-use efficiency, and yield potential - are they compatible, dissonant, or mutually exclusive? Aust. J. Agric. Res. 56, 1159–1168. doi: 10.1071/AR05069
Bowles A. M. C., Paps J., Bechtold U. (2021). Evolutionary origins of drought tolerance in spermatophytes. Front. Plant Sci. 12. doi: 10.3389/fpls.2021.655924
Cao D., Zhang J., Han J., Zhang T., Yang S., Wang J., et al. (2022). Projected increases in global terrestrial net primary productivity loss caused by drought under climate change. Earths Future 10, e2022EF002681. doi: 10.1029/2022ef002681
Catoni R., Varone L., Gratani L. (2013). Variations in leaf respiration across different seasons for Mediterranean evergreen species. Photosynthetica 51, 295–304. doi: 10.1007/s11099-013-0026-1
Chen R., Ran J., Huang H., Dong L., Sun Y., Ji M., et al. (2019). Life history strategies drive size-dependent biomass allocation patterns of dryland ephemerals and shrubs. Ecosphere 10, e02709. doi: 10.1002/ecs2.2709
Cooper J. E., Scherer H. W. (2012). Marschner’s mineral nutrition of higher plants (Oxford, UK: Academic Press).
Correia D. L. P., Bouchard M., Filotas E., Raulier F. (2019). Disentangling the effect of drought on stand mortality and productivity in northern temperate and boreal forests. J. Appl. Ecol. 56, 758–768. doi: 10.1111/1365-2664.13305
Couso L. L., Fernandez R. J. (2012). Phenotypic plasticity as an index of drought tolerance in three Patagonian steppe grasses. Ann. Bot. 110, 849–857. doi: 10.1093/aob/mcs147
Dai A. G. (2013). Increasing drought under global warming in observations and models. Nat. Climate Change 3, 52–58. doi: 10.1038/nclimate1633
Dayer S., Herrera J. C., Dai Z., Burlett R., Lamarque L. J., Delzon S., et al. (2020). The sequence and thresholds of leaf hydraulic traits underlying grapevine varietal differences in drought tolerance. J. Exp. Bot. 71, 4333–4344. doi: 10.1093/jxb/eraa186
Deng J., Li T., Wang G., Liu J., Yu Z., Zhao C., et al. (2008). Trade-offs between the metabolic rate and population density of plants. PloS One 3, e1799. doi: 10.1371/journal.pone.0001799
Deng J., Wang G., Morris E. C., Wei X., Li D., Chen B., et al. (2006). Plant mass-density relationship along a moisture gradient in north-west china. J. Ecol. 94, 953–958. doi: 10.1111/j.1365-2745.2006.01141.x
Desbrosses G. J., Stougaard J. (2011). Root nodulation: A paradigm for how plant-microbe symbiosis influences host developmental pathways. Cell Host Microbe 10, 348–358. doi: 10.1016/j.chom.2011.09.005
Du L., Liu H., Guan W., Li J., Li J. (2019). Drought affects the coordination of belowground and aboveground resource-related traits in Solidago canadensis in China. Ecol. Evol. 9, 9948–9960. doi: 10.1002/ece3.5536
Feng S., Fu Q. (2013). Expansion of global drylands under a warming climate. Atmospheric Chem. Phys. 13, 10081–10094. doi: 10.5194/acp-13-10081-2013
Freschet G. T., Violle C., Bourget M. Y., Scherer-Lorenzen M., Fort F. (2018). Allocation, morphology, physiology, architecture: the multiple facets of plant above- and below-ground responses to resource stress. New Phytol. 219, 1338–1352. doi: 10.1111/nph.15225
Gambetta G. A., Herrera J. C., Dayer S., Feng Q., Hochberg U., Castellarin S. D. (2020). The physiology of drought stress in grapevine: towards an integrative definition of drought tolerance. J. Exp. Bot. 71, 4658–4676. doi: 10.1093/jxb/eraa245
Gebauer R., Plichta R., Urban J., Volařík D., Hájíčková M. (2020). The resistance and resilience of European beech seedlings to drought stress during the period of leaf development. Tree Physiol. 40, 1147–1164. doi: 10.1093/treephys/tpaa066
Geng Y., Wang Z., Liang C., Fang J., Baumann F., Kuehn P., et al. (2012). Effect of geographical range size on plant functional traits and the relationships between plant, soil and climate in Chinese grasslands. Global Ecol. Biogeography 21, 416–427. doi: 10.1111/j.1466-8238.2011.00692.x
Hanslin H. M., Bischoff A., Hovstad K. A. (2019). Root growth plasticity to drought in seedlings of perennial grasses. Plant Soil 440, 551–568. doi: 10.1007/s11104-019-04117-7
He N., Li Y., Liu C., Xu L., Li M., Zhang J., et al. (2020). Plant trait networks: Improved resolution of the dimensionality of adaptation. Trends Ecol. Evol. 35, 908–918. doi: 10.1016/j.tree.2020.06.003
Hofer D., Suter M., Buchmann N., Luscher A. (2017). Nitrogen status of functionally different forage species explains resistance to severe drought and post-drought overcompensation. Agric. Ecosyst. Environ. 236, 312–322. doi: 10.1016/j.agee.2016.11.022
Hofer D., Suter M., Haughey E., Finn J. A., Hoekstra N. J., Buchmann N., et al. (2016). Yield of temperate forage grassland species is either largely resistant or resilient to experimental summer drought. J. Appl. Ecol. 53, 1023–1034. doi: 10.1111/1365-2664.12694
Hong J., Ma X., Yan Y., Zhang X., Wang X. (2018). Which root traits determine nitrogen uptake by alpine plant species on the Tibetan Plateau? Plant Soil 424, 63–72. doi: 10.1007/s11104-017-3434-3
Hu W., Ran J., Dong L., Du Q., Ji M., Yao S., et al. (2021). Aridity-driven shift in biodiversity-soil multifunctionality relationships. Nat. Commun. 12, 5350. doi: 10.1038/s41467-021-25641-0
Huang H., Ran J., Ji M., Wang Z., Dong L., Hu W., et al. (2020a). Water content quantitatively affects metabolic rates over the course of plant ontogeny. New Phytol. 228, 1524–1534. doi: 10.1111/nph.16808
Huang H., Ran J., Li X., Wang Z., Chen R., Wu F., et al. (2020b). A general model for seed and seedling respiratory metabolism. Am. Nat. 195, 534–546. doi: 10.1086/707072
Jacobs M., Rais A., Pretzsch H. (2021). How drought stress becomes visible upon detecting tree shape using terrestrial laser scanning (TLS). For. Ecol. Manage. 489, 118915. doi: 10.1016/j.foreco.2021.118975
Korekar G., Sharma R. K., Kumar R., Bisht N. C., Srivastava R. B., Ahuja P. S., et al. (2012). Identification and validation of sex-linked SCAR markers in dioecious Hippophae rhamnoides L. (Elaeagnaceae). Biotechnol. Lett. 34, 973–978. doi: 10.1007/s10529-012-0852-4
Kramer P., Boyer J. (1995). Plant and soil water relationships. New York and London: Academic Press.
Lauriks F., Salomón R. L., De Roo L., Sobrino-Plata J., Rodríguez-García A., Steppe K. (2022). Limited mitigating effects of elevated CO2 in young aspen trees to face drought stress. Environ. Exp. Bot. 201, 104942. doi: 10.1016/j.envexpbot.2022.104942
Li H., Wei M., Dong L., Hu W., Xiong J., Sun Y., et al. (2022a). Leaf and ecosystem water use efficiencies differ in their global-scale patterns and drivers. Agric. For. Meteorology 319, 108919. doi: 10.1016/j.agrformet.2022.108919
Li J., Pei J., Fang C., Li B., Nie M. (2024). Drought may exacerbate dryland soil inorganic carbon loss under warming climate conditions. Nat. Commun. 15, 617. doi: 10.1038/s41467-024-44895-y
Li P. F., Ma B. L., Guo S., Ding T. T., Xiong Y. C. (2022b). Bottom-up redistribution of biomass optimizes energy allocation, water use and yield formation in dryland wheat improvement. J. Sci. Food Agric. 102, 3336–3349. doi: 10.1002/jsfa.11680
Li X., Shao M. A., Zhao C., Jia X. (2019a). Spatial variability of soil water content and related factors across the Hexi Corridor of China. J. Arid Land 11, 123–134. doi: 10.1007/s40333-018-0123-x
Li X., Shao M. A., Zhao C., Liu T., Jia X., Ma C. (2019b). Regional spatial variability of root-zone soil moisture in arid regions and the driving factors - a case study of Xinjiang, China. Can. J. Soil Sci. 99, 277–291. doi: 10.1139/cjss-2019-0006
Li Y., Jiang Y., Zhao K., Chen Y., Wei W., Shipley B., et al. (2022c). Exploring trait-performance relationships of tree seedlings along experimentally manipulated light and water gradients. Ecology 103, e3703. doi: 10.1002/ecy.3703
Limousin J. M., Yepez E. A., McDowell N. G., Pockman W. T. (2015). Convergence in resource use efficiency across trees with differing hydraulic strategies in response to ecosystem precipitation manipulation. Funct. Ecol. 29, 1125–1136. doi: 10.1111/1365-2435.12426
Matías L., Castro J., Zamora R. (2011). Soil-nutrient availability under a global-change scenario in a Mediterranean mountain ecosystem. Global Change Biol. 17, 1646–1657. doi: 10.1111/gcb.2011.17.issue-4
Morabito C., Orozco J., Tonel G., Cavalletto S., Meloni G. R., Schubert A., et al. (2022). Do the ends justify the means? Impact of drought progression rate on stress response and recovery in Vitis vinifera. Physiologia Plantarum 174, e13590. doi: 10.1111/ppl.13590
Mukarram M., Choudhary S., Kurjak D., Petek A., Khan M. M. A. (2021). Drought: Sensing, signalling, effects and tolerance in higher plants. Physiologia Plantarum 172, 1291–1300. doi: 10.1111/ppl.13423
Muller B., Pantin F., Genard M., Turc O., Freixes S., Piques M., et al. (2011). Water deficits uncouple growth from photosynthesis, increase C content, and modify the relationships between C and growth in sink organs. J. Exp. Bot. 62, 1715–1729. doi: 10.1093/jxb/erq438
Nadia Biruk L., Elena Fernandez M., Veronica Gonzalez C., Guevara A., Rovida-Kojima E., Valeria Giordano C. (2022). High and diverse plastic responses to water availability in four desert woody species of South America. Trees-Structure Funct. 36, 1881–1894. doi: 10.1007/s00468-022-02335-8
Pawlowski K., William E. (2008). Nitrogen-fixing actinorhizal symbioses (Dordrecht, The Netherlands: Springer). doi: 10.1007/978-1-4020-3547-0
Poorter H., Jagodzinski A. M., Ruiz-Peinado R., Kuyah S., Luo Y., Oleksyn J., et al. (2015). How does biomass distribution change with size and differ among species? An analysis for 1200 plant species from five continents. New Phytol. 208, 736–749. doi: 10.1111/nph.13571
Proctor M. C. F., Tuba Z. (2002). Poikilohydry and homoihydry: antithesis or spectrum of possibilities? New Phytol. 156, 327–349. doi: 10.1046/j.1469-8137.2002.00526.x
Puglielli G., Laanisto L., Poorter H., Niinemets U. (2021). Global patterns of biomass allocation in woody species with different tolerances of shade and drought: evidence for multiple strategies. New Phytol. 229, 308–322. doi: 10.1111/nph.16879
Ramakrishna A., Ravishankar G. A. (2011). Influence of abiotic stress signals on secondary metabolites in plants. Plant Signaling Behav. 6, 1720–1731. doi: 10.4161/psb.6.11.17613
R Core Team. (2021). R: A language and environment for statistical computing (Vienna, Austria: R Foundation for Statistical Computing). Available online at: https://www.R-project.org/ (Accessed 6th March, 2024 2024).
Rodiyati A., Arisoesilaningsih E., Isagi Y., Nakagoshi N. (2005). Responses of Cyperus brevifolius (Rottb.) Hassk. and Cyperus kyllingia Endl. to varying soil water availability. Environ. Exp. Bot. 53, 259–269. doi: 10.1016/j.envexpbot.2004.03.018
Rodriguez-Alarcon S., Tamme R., Carmona C. P. (2022). Intraspecific trait changes in response to drought lead to trait convergence between-but not within-species. Funct. Ecol. 36, 1900–1911. doi: 10.1111/1365-2435.14099
Rowland L., Ramirez-Valiente J., Hartley I. P., Mencuccini M. (2023). How woody plants adjust above- and below-ground traits in response to sustained drought. New Phytol. 239, 1173–1189. doi: 10.1111/nph.19000
Sardans J., Penuelas J., Estiarte M., Prieto P. (2008a). Warming and drought alter C and N concentration, allocation and accumulation in a Mediterranean shrubland. Global Change Biol. 14, 2304–2316. doi: 10.1111/j.1365-2486.2008.01656.x
Sardans J., Penuelas J., Ogaya R. (2008b). Experimental drought reduced acid and alkaline phosphatase activity and increased organic extractable P in soil in a Quercus ilex Mediterranean forest. Eur. J. Soil Biol. 44, 509–520. doi: 10.1016/j.ejsobi.2008.09.011
Sardans J., Rivas-Ubach A., Penuelas J. (2012). The C:N:P stoichiometry of organisms and ecosystems in a changing world: A review and perspectives. Perspect. Plant Ecol. Evol. Systematics 14, 33–47. doi: 10.1016/j.ppees.2011.08.002
Senapati N., Stratonovitch P., Paul M. J., Semenov M. A. (2019). Drought tolerance during reproductive development is important for increasing wheat yield potential under climate change in Europe. J. Exp. Bot. 70, 2549–2560. doi: 10.1093/jxb/ery226
Silvester W. B., Berg R. H., Schwintzer C. R., Tjepkema J. D. (2008). Nitrogen fixation: origins, applications, and research progress (Dordrecht, The Netherlands: Springer).
Sui Y., Cui Q., Dong M., He W. (2011). Contrasting responses of legume versus non-legume shrubs to soil water and nutrient shortages in the Mu Us Sandland. J. Plant Ecol. 4, 268–274. doi: 10.1093/jpe/rtq040
Tobita H., Uemura A., Kitao M., Kitaoka S., Maruyama Y., Utsugi H. (2011). Effects of elevated atmospheric carbon dioxide, soil nutrients and water conditions on photosynthetic and growth responses of Alnus hirsuta. Funct. Plant Biol. 38, 702–710. doi: 10.1071/FP11024
Sun Y., Sun Y., Yao S., Akram M. A., Hu W., Dong L., et al. (2021). Impact of climate change on plant species richness across drylands in china: From past to present and into the future. Ecol. Indic. 132, 108288. doi: 10.1016/j.ecolind.2021.108288
Trueba S., Pan R., Scoffoni C., John G. P., Davis S. D., Sack L. (2019). Thresholds for leaf damage due to dehydration: declines of hydraulic function, stomatal conductance and cellular integrity precede those for photochemistry. New Phytol. 223, 134–149. doi: 10.1111/nph.15779
Usmani M. M., Nawaz F., Majeed S., Shehzad M. A., Ahmad K. S., Akhtar G., et al. (2020). Sulfate-mediated drought tolerance in maize involves regulation at physiological and biochemical levels. Sci. Rep. 10, 1147. doi: 10.1038/s41598-020-58169-2
Violle C., Navas M., Vile D., Kazakou E., Fortunel C., Hummel I., et al. (2007). Let the concept of trait be functional! Oikos 116, 882–892. doi: 10.1111/j.2007.0030-1299.15559.x
Wang Z., Ji M., Deng J., Milne R. I., Ran J., Zhang Q., et al. (2015). A theoretical framework for whole-plant carbon assimilation efficiency based on metabolic scaling theory: a test case using Picea seedlings. Tree Physiol. 35, 599–607. doi: 10.1093/treephys/tpv030
Wei M., Li H., Akram M. A., Dong L., Sun Y., Hu W., et al. (2022). Quantifying drought resistance of drylands in northern china from 1982 to 2015: regional disparity in drought resistance. Forests 13, 100. doi: 10.3390/f13010100
Wendering P., Nikoloski Z. (2023). Toward mechanistic modeling and rational engineering of plant respiration. Plant Physiol. 191, 2150–2166. doi: 10.1093/plphys/kiad054
Xiong J., Dong L., Lu J., Hu W., Gong H., Xie S., et al. (2022). Variation in plant carbon, nitrogen and phosphorus contents across the drylands of china. Funct. Ecol. 36, 174–186. doi: 10.1111/1365-2435.13937
Yan W., Zhong Y., Shangguan Z. (2016). A meta-analysis of leaf gas exchange and water status responses to drought. Sci. Rep. 6, 20917. doi: 10.1038/srep20917
Yan Y., Liu Q., Zhang Q., Ding Y., Li Y. (2019). Adaptation of dominant species to drought in the inner Mongolia grassland - species level and functional type level analysis. Front. Plant Sci. 10. doi: 10.3389/fpls.2019.00231
Zang Y., Min X., de Dios V. R., Ma J., Sun W. (2020). Extreme drought affects the productivity, but not the composition, of a desert plant community in Central Asia differentially across microtopographies. Sci. Total Environ. 717, 137251. doi: 10.1016/j.scitotenv.2020.137251
Zhang L., Zhu T., Liu X., Nie M., Xu X., Zhou S. (2020). Limited inorganic N niche partitioning by nine alpine plant species after long-term nitrogen addition. Sci. Total Environ. 718, 137270. doi: 10.1016/j.scitotenv.2020.137270
Keywords: N-fixing legumes, metabolic rate, CN stoichiometry, biomass distribution, drought sensitivity
Citation: Zhao D, Wei M, Wang X, Aqeel M, Ran J and Deng J (2024) Morpho-physiological adaptations to drought stress in nitrogen-fixing and non-nitrogen-fixing plants. Front. Ecol. Evol. 12:1407882. doi: 10.3389/fevo.2024.1407882
Received: 27 March 2024; Accepted: 09 April 2024;
Published: 26 April 2024.
Edited by:
Peijian Shi, Nanjing Forestry University, ChinaCopyright © 2024 Zhao, Wei, Wang, Aqeel, Ran and Deng. This is an open-access article distributed under the terms of the Creative Commons Attribution License (CC BY). The use, distribution or reproduction in other forums is permitted, provided the original author(s) and the copyright owner(s) are credited and that the original publication in this journal is cited, in accordance with accepted academic practice. No use, distribution or reproduction is permitted which does not comply with these terms.
*Correspondence: Jinzhi Ran, cmFuanpAbHp1LmVkdS5jbg==