- 1Department of Behavioral and Cognitive Biology, University of Vienna, Vienna, Austria
- 2Department of Forest and Soil Sciences, Institute of Soil Research, University of Natural Resources and Life Sciences, Tulln, Austria
- 3Department of Biology, University of Bern, Bern, Switzerland
The avian vampire fly is an invasive species on the Galapagos Islands, where its hematophagous larvae utilize nestling birds as hosts. It poses a serious threat to populations and species of Darwin finches and other avian species. Prior studies suggest that the avian vampire fly larvae develop more rapidly in nests of small tree finches compared to sympatric green warbler finch nests. Here we investigate factors that may differentially influence larval growth in the two host species, including nest microclimate and nestling diet. We collected nest base temperature and humidity data as proxies for nest microclimate. As indicators of dietary composition and quality, we measured stable isotope δ13C and δ15N composition of nestling blood, used the C:N ratios as an indicator of relative protein content, and assessed the amino acid composition of the blood. The hematophagous larvae living off nestling blood were analyzed for the same parameters except amino acid composition. The effect of nest base humidity on nest base temperature significantly depended on bird species: at low humidity levels nest base temperature was higher in the small tree finch, and declined in both species as nest base humidity increased, which suggests higher stable heat capacity of small tree finch nests. The effect of age group based on the predicted onset of thermoregulation was marginally significant. Nest base temperatures were significantly higher in small tree finch nests during the predicted female brooding period before fully effective thermoregulation. Stable isotope δ13C levels were significantly higher in the blood of small tree finch nestlings compared to warbler finch nestlings, suggesting a difference in nestling food composition and source, while. δ15N levels were similar. The C:N ratios and amino acid composition of nestling blood were not significantly different between the two species, suggesting similar dietary quality. Thus, the faster development of larvae in small tree finch nests may be due to higher nest base temperatures during the early nestling phase rather than host blood quality parameters.
1 Introduction
Host suitability and host quality are important factors in parasite life history, development and parasite population growth (e.g. Tschirren et al., 2007; Bize et al., 2008; Lange et al., 2014; Manzoli et al., 2018). Parasites may choose hosts based on many factors, such as habitat, host species, (e.g., Manzoli et al., 2021), host distribution, body size, abundance, host defense, and/or nutritional status (e.g., Walker and Rotherham, 2011; Seppälä and Leicht, 2015; Stokke et al., 2018). For generalist polyphagous parasites, fitness may vary depending on specific host qualities (e.g., Tschirren et al., 2007; Lange et al., 2014; Manzoli et al., 2021). Specifically for nest parasites, nest climate and diet quality of hosts (such as protein levels) may be important factors in both parasite selection of hosts and ultimately, in parasite reproductive success (Heeb et al., 2000; Tschirren et al., 2007; Cantarero et al., 2013). Variation in host quality may be particularly pronounced in newly introduced parasites on remote archipelagos, where no coevolution between hosts and parasites occurred yet.
A species that exemplifies host selection is the invasive avian vampire fly, Philornis downsi, which has been introduced to the Galapagos Islands and parasitizes at least 21 host species in Galapagos, including passerines and doves (Fessl et al., 2018; Coloma et al., 2020; Anchundia and Fessl, 2021). The avian vampire flies oviposit in host nests during both incubation and nestling phases, with greater parasite loads in the nestling phase (Cimadom et al., 2014; Pike et al., 2021; McNew and Clayton, 2018; Common et al., 2020). In the birds’ incubation phase, the avian vampire fly larvae feed on the underside of brooding adult females and retreat into the nest material between feeding bouts. During the nestling phase, newly hatched larvae initially develop in the nasal cavities and ear canals of nestlings, while the 2nd and 3rd instar larval stages generally reside in the nest base during daylight hours and feed on nestling blood and tissue at night (Fessl et al., 2006). Variation in numbers and parasite growth and development among its different host species suggest that hosts vary in profitability for the parasite (Manzoli et al., 2021). Host preference by Philornis sp. has been suggested in other Philornis-host systems in South America, as certain host species have much higher Philornis nest parasitism than other host species in the same sites (Manzoli et al., 2021; Ramirez et al., 2022). However, a better understanding of factors influencing the quality of different host species of the avian vampire fly in Galapagos is still lacking. Prior studies reported differences in avian vampire fly parasitism between the small tree finch and the green warbler finch, suggesting a difference in host profitability (how much a parasite benefits from a host, including resources/energy acquired from the host) between host species (Bize et al., 2008; Lemoine et al., 2011; Cimadom et al., 2014; Cimadom and Tebbich, 2021). Furthermore, parasite levels in the two species changed over a period of 15 years: compared to data from the early 2000s, mean avian vampire fly parasitism decreased in green warbler finch nests but increased in small tree finch nests during 2012-2017 (Cimadom and Tebbich (2021). This may suggest a potential change in host preference of the avian vampire fly for the small tree finch compared to the green warbler finch due to host profitability. Additionally, Cimadom and Tebbich (2021) reported that among all larval stages the proportion of 3rd instar larvae and pupae increased at a faster rate in small tree finch nests compared to those in the green warbler finch nests suggesting faster larval growth, maturation and completion of larval stages. It suggests that the small tree finch may be a higher quality host and/or provides a more suitable habitat for the avian vampire fly than the green warbler finch. In this study, we aimed to determine whether the two host species differ in factors that may influence larval development and thus could explain the difference found in larval development and growth, and therefore the apparent current host preference. A variety of natural, biotic conditions/factors related to hosts may influence larval growth and maturation in insects, depending on their life history. In the case of the avian vampire fly, these factors may include microclimate of the nest base and overall nutritional quality of the hosts’ blood, however, studies are lacking on how these factors vary between host species in the Galapagos Islands.
Nest microclimate, including nest temperature and humidity, are influenced by many factors, including ambient weather conditions, parent and nestling mass, brood size, brooding rate of female parents, among other factors. Studies have investigated the effects of ambient temperature on nest ectoparasite abundance (e.g., Mennerat et al., 2021; Castaño-Vázquez and Merino, 2022), but field studies on the effects of direct nest temperature on larval development are limited. Prior studies have experimentally shown that black soldier fly, Hermetia illucens, larvae and Protocalliphora blowfly larvae may develop faster at higher temperatures (Bennett and Whitworth, 1991; Palma et al., 2019). Nest microclimate can be influenced by nest composition (e.g., Michielsen et al., 2019). As the green warbler finch is smaller than the small tree finch and their nests differ in size, material, and mean nest height (Kleindorfer and Dudaniec, 2009; ST pers. obs.; CP unpublished data), they potentially have different nest microclimates that may influence larval growth. Nest humidity has also been shown to be an important factor in parasitism in an experimental study where nest humidity influenced the prevalence of hematophagous bird parasites, and vice versa parasite prevalence affected nest humidity (Heeb et al., 2000). We predict that small tree finch nests have higher nest temperatures than green warbler finches, which may be mediated by nest humidity and ambient climate.
Nest base temperatures are likely influenced by both female brooding behavior after hatching and the onset of thermoregulation in nestlings. The precise onset of thermoregulation is not known for the two bird species but can be estimated from multifactorial comparative studies of altricial birds (e.g. Dunn, 1975). Before active thermoregulation, metabolic rates and growth of nestlings depend on nest temperature and hence the brooding behavior of females. After nestling thermoregulation occurs, various factors could still create variation in nest climate, such as the number of nestlings, nestling size and metabolic rate of the nestlings. Thus, female brooding behavior may continue for this short time period of transition and hence affect nest base temperatures.
Nutritional levels in the diet are essential for adequate larval development in insects. Natural food sources of hematophagous larvae may vary in nutritional composition. Prior research demonstrated that protein concentrations in food sources, measured for example as C:N ratios, have profound impacts on larval growth of insects, including mosquitoes, black soldier flies and fruit flies (Balagawi et al., 2014; Hood-Nowotny et al., 2012; Palma et al., 2019). More specifically, larval growth can be positively influenced by lower C:N ratios (i.e. higher protein contents) of larval diet alone in the mosquito Anopheles arabiensis or the melon fly Bactrocera cucurbita (Hood-Nowotny et al., 2012; UlHaq et al., 2010), or can act in conjunction with higher temperatures to improve growth (Palma et al., 2019). However, a previous experimental study on Aedes aegypti found that larvae fed a diet consisting of 61-77% carbohydrates and 20-33% proteins developed faster compared to those on both lower or higher protein-to-carbohydrate diets (van Schoor et al., 2020). This suggests that there may be optimal carbohydrate levels for larval growth.
Previous studies of parasite-host feeding systems demonstrated that stable isotope analysis is an effective method for determining dietary sources and is commonly used in ecological/entomological research (Hood-Nowotny and Knols, 2007; Quinby et al., 2020). The stable isotopes composition δ13C and δ15N may vary depending on the diet per se and the trophic position of hosts and their ectoparasites. δ13C can reflect dietary choices by individuals and their prey, specifically which plant types they consume (with either C3 or C4 photosynthetic pathways). Thus, higher δ13C values can suggest that birds or their prey consume more C4 versus C3 plants (Staddon, 2004). δ15N is also dependent on the trophic level of the study organism, with higher tissue δ15N values indicating consumption of organisms higher up the trophic ladder. In the case of insectivorous or omnivorous hosts, higher tissue δ15N may be driven by more arthropods compared to seeds or arthropods further up the carnivorous food chain, such as spiders, in the host diet (Meyhoff et al., 2023; Hood-Nowotny et al., 2023). Additionally, lower C:N ratio of the host species blood or parasite indicates more protein-N per molecule of carbon. Schmidt et al. (2011) reported carbon and nitrogen stable isotope ratios in blood-feeding larvae of the tick, Ixodes ricinus, were reflective of the differing diets of the two host species. Ticks that fed on rabbit hosts with lower δ13C blood values than gerbil hosts also had significantly lower δ13C values than ticks that fed on gerbil hosts (Schmidt et al., 2011). Green warbler finch nestlings feed on a more insectivorous diet than small tree finch nestlings (Filek et al., 2018) and we thus predict a more protein-rich blood composition in green warbler finch nestlings, demonstrated by both a lower C:N ratio and higher δ15N levels. However, we do not expect to see significant differences in blood δ13C levels between the two host species, as most plants in our study site use C3 photosynthesis (RCHN, pers obs).
Amino acids are equally essential factors in the growth and development of insects. Prior studies on dietary amino acids in insects have reported specific amino acids that are essential for the completion of larval developmental stages (Singh and Brown, 1957; Davis, 1975; Chang, 2004). Insect larvae lacking specific non-essential amino acids in their diet may survive, but larval growth may be delayed (e.g., Davis, 1975; Chang, 2004). Additionally, an excess of specific amino acids may also slow or hinder larval growth (Hinton et al., 1951). Thus, investigating amino acid composition in natural blood diets of avian vampire fly larvae may provide insight into the nutritional requirements of avian vampire fly larval development and additionally host blood quality. Given this, we predicted that there would be significant differences in the percent composition of at least one amino acid in small tree finch nestling blood compared to green warbler finch nestling blood that may play a role in the observed differences in larval development. This may include, but is not limited to lower isoleucine levels for small tree finches, as high isoleucine levels can inhibit larval development (Hinton et al., 1951).
We aimed to answer the following questions: (1) Is there a significant difference in nest base temperature or humidity levels during the nestling phase between our two focal host species, the small tree finch and green warbler finch? (2) Does dietary quality of nestling blood differ between the two host species?, and (3) Do the hematophagous avian vampire fly larvae living off nestling blood reflect host dietary differences?. We thus monitored climatic variables in nests of both the green warbler finch and small tree finch and measured carbon/nitrogen levels and stable isotope composition (δ13C and δ15N) in the nestling blood and larvae from nests of both species. Furthermore, we quantified amino acid composition in the blood of both focal host species for comparison.
2 Materials and methods
2.1 Study site and focal species
This study took place in Los Gemelos (0°37’50” S, 90°23’25” W, 569 - 634 m a.s.l.), a protected area within the Galapagos National Park on Santa Cruz Island in the Galapagos Islands, Ecuador. This study site is a humid forest mainly characterized by the endemic daisy tree, Scalesia pedunculata, along with other trees and shrubs, such as the endemic guayabillo tree, Psidium galapageium. Additionally, the field site includes introduced plant species, such as the guava tree, P. guajava, and the highly invasive raspberry, Rubus niveus, among others (Rentería et al., 2012; Jäger et al., 2017; Walentowitz et al., 2021); however, in our study area, there has been long-term management of R. niveus by the Galapagos National Park (Cimadom et al., 2019).
Samples were collected from two avian host species, the green warbler finch, Certhidia olivacea, and the small tree finch, Camarhyncus parvulus. Nests of these two host species were monitored in 2021-2022, from January to May, during the incubation and nestling/feeding phases. Nests were visited every 2-3 days to confirm activity and hatching date. Nestlings were considered zero days old on hatch date, when the first egg hatched. All nests were monitored until activity ceased, which was confirmed by visual observations and filming with an endoscopic camera. Once a nest was no longer active, it was collected and avian vampire fly larvae and pupae were quantified.
2.2 Nest climate data collection
We used data loggers to measure temperature and humidity in the nest base of green warbler finch and small tree finch nests between 6 February - 2 May 2021 and 16 February - 21 April 2022. Hygrometers (iButton® DS1923, Maxim Integrated), recording both temperature and humidity, were placed in the nest base of eight green warbler finch nests and 11 small tree finch nests in 2021 and four green warbler finch and five small tree finch nests in 2022. Additionally, thermochrons (iButton® DS1925, Maxim Integrated), measuring temperature only, were placed in two green warbler finch nests and one small tree finch nest in 2021 and in four green warbler finch and two small tree finch nests in 2022, due to a limited number of hygrometers. All data loggers were placed in the nest base under the top base layer where avian vampire fly larvae commonly develop and pupate. Climate data was recorded every 30 min. during the nestling phase. Data loggers were removed from each nest after termination of breeding activity. All data was downloaded via the OneWireViewer software. Percent relative humidity (RH) data were automatically compensated for temperature and saturation drift within each data logger. We only used data points from data loggers when we could confirm at least one nestling was still alive in the nest.
2.3 Blood and larvae field collections
2.3.1 Nestlings
Blood samples were collected between 12 February to 3 May 2021 from 36 green warbler finch nestlings of 21 nests and 16 small tree finch nestlings of nine nests at the age of 5-6 days. Nestlings were removed from their nest for a maximum of 15 minutes. Simultaneously, standard body measurements (bill head, tarsus, and wing length) and weight of each nestling were recorded. Each nestling was also checked for effects of avian vampire fly larval parasitism. One to two drops of blood (<30 μL) were collected from the brachial vein in the left wing of each nestling using a new non-heparinized microcapillary tube. Immediately, the blood from each nestling was transferred onto separate fiber glass filter discs (the size of a hole punch circle) and stored in 1.5 mL Eppendorf tubes. Each fiber glass filter disc with blood was dried at approximately 40°C for one hour. After drying, the Eppendorf tubes were stored in a cool, dry place until exportation.
2.3.2 Larvae from natural nests
From 15 February - 3 May 2021, we collected avian vampire fly larvae from green warbler finch and small tree finch nests once nest activity ceased. All larvae were collected from nests in which nestling blood samples were taken for stable isotope analysis. One to five whole larvae per nest were collected and stored in separate 1.5 mL Eppendorf tubes with 70% ethanol. In total, 32 avian vampire fly larvae from eight green warbler finch nests and 32 larvae from seven small tree finch nests were collected. All larvae were 2nd and 3rd instar stages. These samples were stored at room temperature until stable isotope analysis was conducted in Austria.
2.4 Stable isotope analysis
Stable isotope analyses of all samples (nestling blood and avian vampire fly larvae) were conducted at BOKU, Tulln, Austria. Total C and N analysis and bulk 13C and 15N isotope analysis, were conducted by elemental isotope ratio mass spectrometry. For samples stored in alcohol samples were repeatedly (3 times) washed and dried at 40°C to remove traces of alcohol. These and other samples were then dried (40°C for 72 h), either accurately weighed (3-5 mg) or just put into (in the case of the fiberglass filter discs) 9 by 5 mm tin cups. Samples were analyzed using a Thermo Flash 2000 Organic Elemental analyzer, linked to a Thermo Delta V Advantage automated isotope ratio mass spectrometer (IRMS) (Thermo, Bremen, DE). A full complement of internal and external standards was run with the samples to calculate isotopic ratios and %C and %N values. The isotope ratios were expressed as parts per thousand per mille (‰) or δ deviation from the internationally recognized standards Vienna Pee Dee Belemnite (VPDB) and ambient air.
2.5 Amino acid analysis
2.5.1 Stock amino acids
Amino acid stock solutions for standards were made from purchased pure amino acids and dissolved in Millipore water. Standards included 0.1M concentrations of l-alanine, l- leucine, l-arginine, l-proline, l-cysteine, l-asparagine, d-serine, l-glycine, l-threonine, l-lysine, l-phenylalanine and l-aspartic acid, 0.05M concentration of l-valine, 0.02M concentration of l- isoleucine and l-methionine, and a 0.005M concentration of l-glutamic acid and l-tyrosine. All of these amino acids are related to protein synthesis, and thus informative for larval development.
2.5.2 Sample preparation - bird blood digestion for amino acid determination
Half of a disc of quartz filter containing a drop of the host blood was used for analysis. Blood samples from 18 green warbler finch and 10 small tree finch nestlings were analyzed to quantify amino acid levels. To each disc sample, 500 μL 6M HCl solution was added and samples incubated at 110°C for 24 hours in sealed glass vials. After incubation, once samples reached room temperature, they were exposed to nitrogen (N2) gas for up to 24 hours to evaporate the HCl, and then diluted in 500 μL of deionized water.
2.5.3 Derivatization of amino acids
Derivatization of samples was conducted following protocols from Bertrand et al. (2008). In brief, 300 μL of either amino acid standard stock solution or bird blood sample was added to a 1 ml glass vial. Then, 300 μL alcohol EtOH was added to each tube, and vortexed 10 s. Then, 75 μL pyridine was added to each tube, followed by 165 μL 0.5 M NaOH, and vortexed for 10s after each addition. After, 100 μL ethyl chloroformate was added to each tube, followed by 60 μL ethanol-stable chloroform. This solution was then left for 5 min. for the CO2 to evaporate. Finally, 750 μL of ethanol-stable chloroform was added and each tube was vortexed. All reagents used were of gas chromatography quality and all necessary health and safety precautions adhered to (e.g., all activities were conducted under the fume hood).
The organic chloroform layer was extracted from each sample, placed in a new tube and evaporated using N2 gas for generally 2-3 hours until dry and 200 μL of iso-octane was added to each sample to resuspend it. For each sample, 100 μL of the resuspension was decanted into a 1.5 mL glass vial containing a ~150 μL glass insert for gas chromatography.
2.5.4 Gas chromatography
Amino acid samples were run on an HP 5890 Series II Plus gas chromatograph. Samples were analyzed by injecting 1uL of the organic layer. A non-chiral RTX1701 column (60 m long x 0.25 mm diameter, 1 µm thickness; Restek) was used. Helium was used as the carrier gas with a flow rate of 1.2 mL min−1. The oven program consisted of an initial temperature set for 120°C for 10 min, followed by a ramp of 20.0°C/min, with a final temperature of 275°C for 10 min. Total run time was 27.75 min. Using the ChemStation program (Agilent Technologies, USA), peaks of amino acids were identified by comparing with retention times from reference amino acid peaks in the chromatograms (Supplementary Table 1).
2.6 Statistical analysis
2.6.1 Nestling size
We conducted a Mann-Whitney U test (n = 59) to determine if there was a difference in body mass (g) between the two host species.
2.6.2 Nest climate
For this study, 13,045 data points were included in the analysis for temperature and 9,407 data points included for percent humidity. Descriptive statistics were obtained using describe.by function in the psych package (Revelle, 2022). Daily mean values for nest base temperature and humidity (% RH) were calculated for days with complete data (24 hrs). Daily mean ambient temperature and humidity (% RH) values from the “El Carmen” weather station near the study site were also used in the analysis (CDF, unpublished data). To account for the estimated age at which nestlings begin to thermoregulate (Dunn, 1976, 1979; Ricklefs and Hainsworth, 1968), each data point was classified into one of two age groups, based on the age of nestlings when the data was collected: 0-6 days old and 7-14 days old.
Initially, individual linear mixed models (fit with REML) were run using lme4 package (Bates et al., 2015) and included temperature and humidity as dependent variables. To account for non-normality and/or heteroscedasticity, final robust linear mixed models were run using the robustlmm package (Koller, 2016) for both nest base temperature and humidity. Overall, 194 daily mean values for nest base temperature and 130 daily mean values for nest base humidity were used in the models. For the initial nest base temperature and humidity models, fixed effects included species and age group before and after the predicted onset of thermoregulation 6 days after hatching. Additional fixed effects included year, number of nestlings alive each day, nest height (m), number of parasites, and ambient temperature or humidity, as these factors may affect the nest base climate in field conditions. Additionally, interaction terms in each model included: 1) species*age group, 2) species*number of parasites, and 3) species*ambient humidity in the humidity model. We included multiple interaction terms, which consisted of variables of interest and species, to account for potential species-specific differences in how each variable of interest may affect nest temperature and nest humidity. In the temperature models, the interaction term nest humidity*species was also included, to account for a potential evaporative cooling effect of humid conditions. An interaction of ambient temperature and species was not included in the temperature model, to improve model fit (see models compared in Supplementary Tables 2, 3). In addition, a second model with nest base temperature as dependent variable was run that includes data from day 0-7 after hatching, that is from hatching to the predicted onset of thermoregulatory activity plus an additional day to account for the transition phase from exothermy to fully effective endothermy, where females are thus still expected to partially brood nestlings (eg., Heyer et al., 2021; CP, unpublished data). This model included the same variables as the initial temperature model except age group and the age group*species interaction. Each model also included iButtonID/NestID as a nested random factor. All continuous variables were mean centered and scaled before running models and the log of the number of parasites was taken and used in the analyses.
2.6.3 Stable isotopes
Six separate linear mixed models (fit with REML) were run using the lme4 package to analyze the effect of species on mean δ13C, mean δ15N, and mean C:N ratios of nestling blood and larval values, with δ13C, δ15N, and C:N ratio of nestling blood and larvae as the dependent variables. Species, blood sampling date (as food availability may vary throughout the season), and number of days the nestlings lived after sampling (as an indicator of nestling starvation, as this can affect the stable isotope values and protein levels in the blood) were included as fixed effects in all models. Nest ID was included as a random factor to control for relatedness between nestlings or larvae in the same nest. Due to non-normal residuals and heteroscedasticity, robust linear mixed models for nestling blood δ15N and C:N values were run using the robustlmm package. Due to non-normal residuals, a generalized linear mixed model with a gamma family with log link was run for δ15N larval values.
Mean δ13C, mean δ15N, and mean C:N were calculated for nestlings and larvae separately for each nest. Separate correlation tests were run in R Studio to test for correlations of δ13C, δ15N, and C:N values between nestlings and larvae from each nest using the corr.test function. For testing all values of both species together, Spearman correlation tests were run due to non-normally distributed nestling values. For testing correlations using data from each species separately, Pearson correlation tests were run for green warbler finch δ13C and C:N values and small tree finch δ13C and δ15N values. For green warbler finch δ15N and small tree finch C:N, Spearman correlation tests were run due to non-normally distributed nestling values.
2.6.4 Amino acids
We analyzed all amino acids that were possible to amplify and identify using gas chromatography. All of these amino acids are related to protein synthesis, and thus informative for larval development. Based on the chromatograms from gas chromatography, we quantified the area for each identified amino acid peak per sample using the ChemStation program. We then summed up a group of defined pre-selected amino acid peak areas per sample for a total area. Peak areas of amino acids that had two peaks close together (methionine, phenylalanine, cysteine, asparagine) within the expected range of the amino acid were added together. For glutamic acid, the peak area of the expected peak was added with the area of its secondary peaks. Next, for each amino acid, individual peak area was divided by total area per sample to obtain a percent composition value or relative abundance measure, a method routinely used for analyzing amino acid composition. All calculations were performed in Microsoft Excel.
Generalized linear mixed models were conducted with percent composition of each amino acid as the dependent variable. Species and blood sampling date were included as fixed effects and nest ID as a random effect. All models were run using the glmmTMB function from the glmmTMB package (Brooks et al., 2017) using the beta family and logit link function.
All analyses were conducted in R Studio (R Core Team, 2021). A variance inflation test was done for all models using the vif function in the car package (Fox and Weisberg, 2019) to confirm the fixed effects were not highly correlated (all VIF values were < 3.57, Supplementary Tables 4-13). For each linear mixed model, the residuals were inspected for normality using QQ plots, histograms, and a Pearson standardized versus residual plot. Each model was also inspected for heteroskedasticity using scaled residual plots. All beta models were assessed for overdispersion using the DHARMa package (Hartig, 2022). All dispersion values were < 1.0. The lmerTest package (Kuznetsova et al., 2017) was used to obtain p-values for the linear mixed models and generalized linear mixed model with the gamma family. The summary function and tab_model function in the sjPlot package (Lüdecke, 2021) were used to obtain p-values for the robust linear mixed models. For all statistical analyses, p ≤ 0.05 was considered significant. All figures were made using the ggplot2 package (Wickham, 2016) in R Studio.
3 Results
3.1 Nestling size
Body mass of 5-6 days old nestlings was not significantly different between the small tree finch and green warbler finch (U = 266, p = 0.267, n = 59).
3.2 Nest climate
Small tree finch and green warbler finch nest base temperatures differed significantly in relation to nest base humidity. At lower nest base humidity levels (e.g., 60%), nest base temperature was higher in the small tree finch nests than in the green warbler finch nests. Small tree finch nest temperature declined more than green warbler finch nest base temperature as nest humidity levels increased (significant nest base humidity*species interaction, t = 2.01, p = 0.031; Table 1, Figure 1). As nest base humidity reached 100%, nest base temperatures for both species were similar (Figure 1). There was also a significant age-group effect (t = 2.01, p = 0.044; Table 1), mainly due to the higher nest base temperatures in small tree finch nests before the predicted onset of thermoregulation (Figure 2A). There was no significant effect of year or nest height on nest temperature (Table 1). Small tree finch nests showed a lower minimum humidity (% RH) and a greater humidity range than green warbler finch nests (Table 2). Since nest base humidity slightly but non-significantly increased in the small tree finch after the onset of thermoregulation and it decreased non-significantly in the green warbler finch, this led to a marginally significant age group*species interaction (t = -1.81, p = 0.07; Table 3, Figure 2B). In the second nest temperature model with nest temperature data for the predicted female brooding period before thermoregulation plus the transition from exothermy to fully effective endothermy, nest base temperature was significantly lower in green warbler finch nests compared to small tree finch nests (t = -2.55, p = 0.01; Table 4). Additionally, nest base temperature decreased significantly, as nest base humidity increased (t = -12.51, p < 0.001; Table 4).
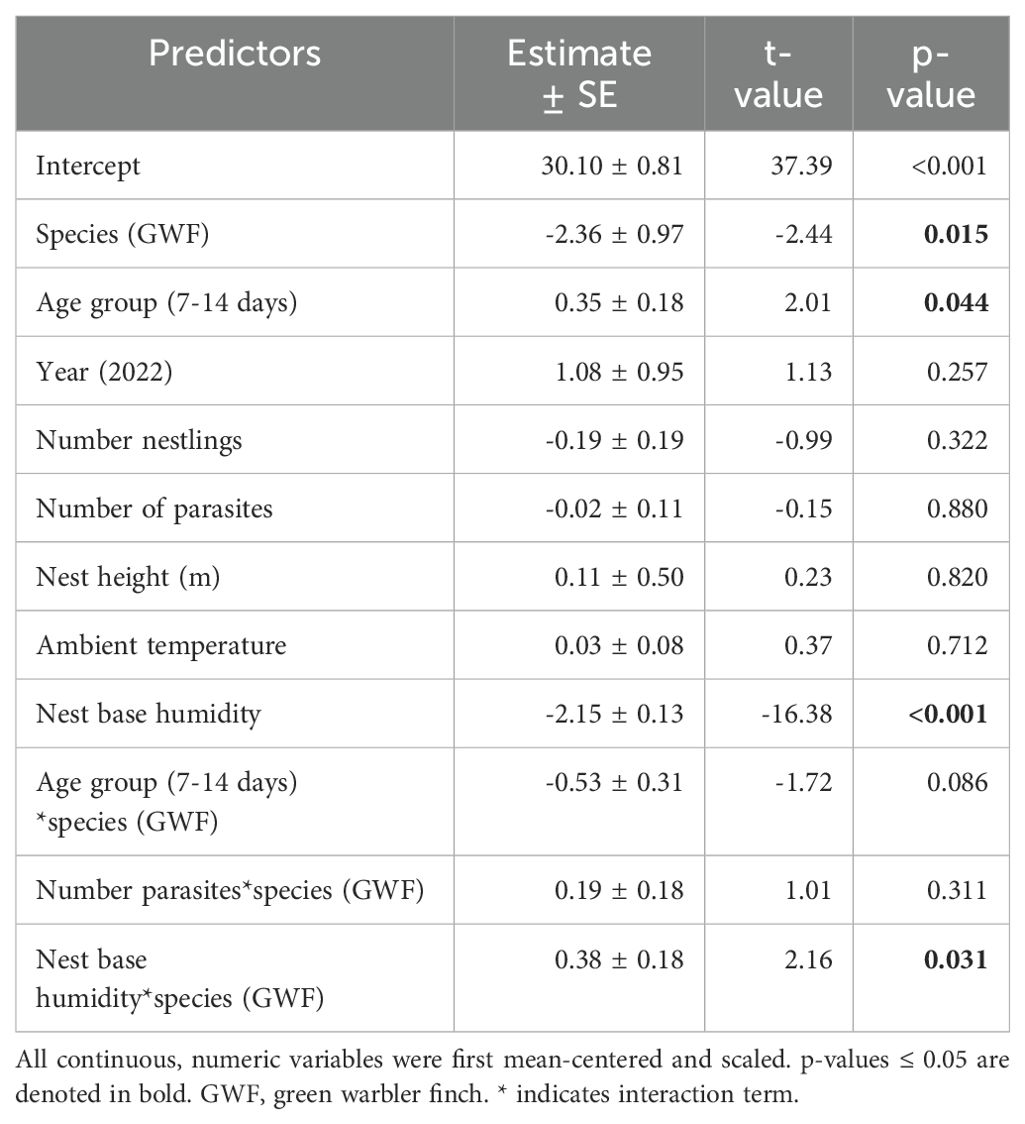
Table 1. Model output of the initial robust linear mixed model for nest base temperature as the dependent variable, including age group as a factor.
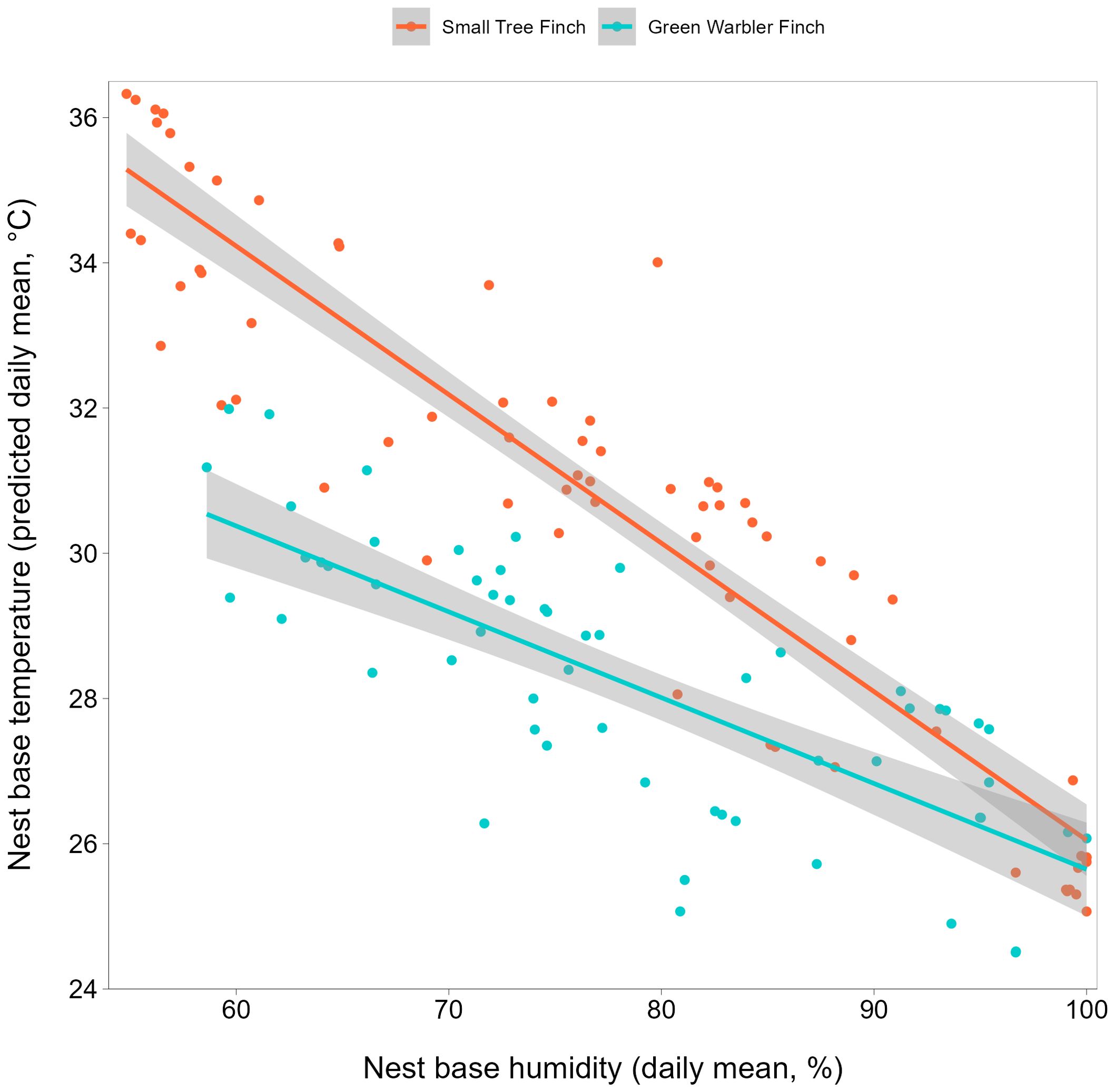
Figure 1. Nest base temperature relative to nest base humidity, for the small tree finch and green warbler finch. Raw data for daily mean temperature values (°C) are displayed as dots. Lines shown are model predicted values for daily mean nest base temperature with 95% confidence intervals in grey.
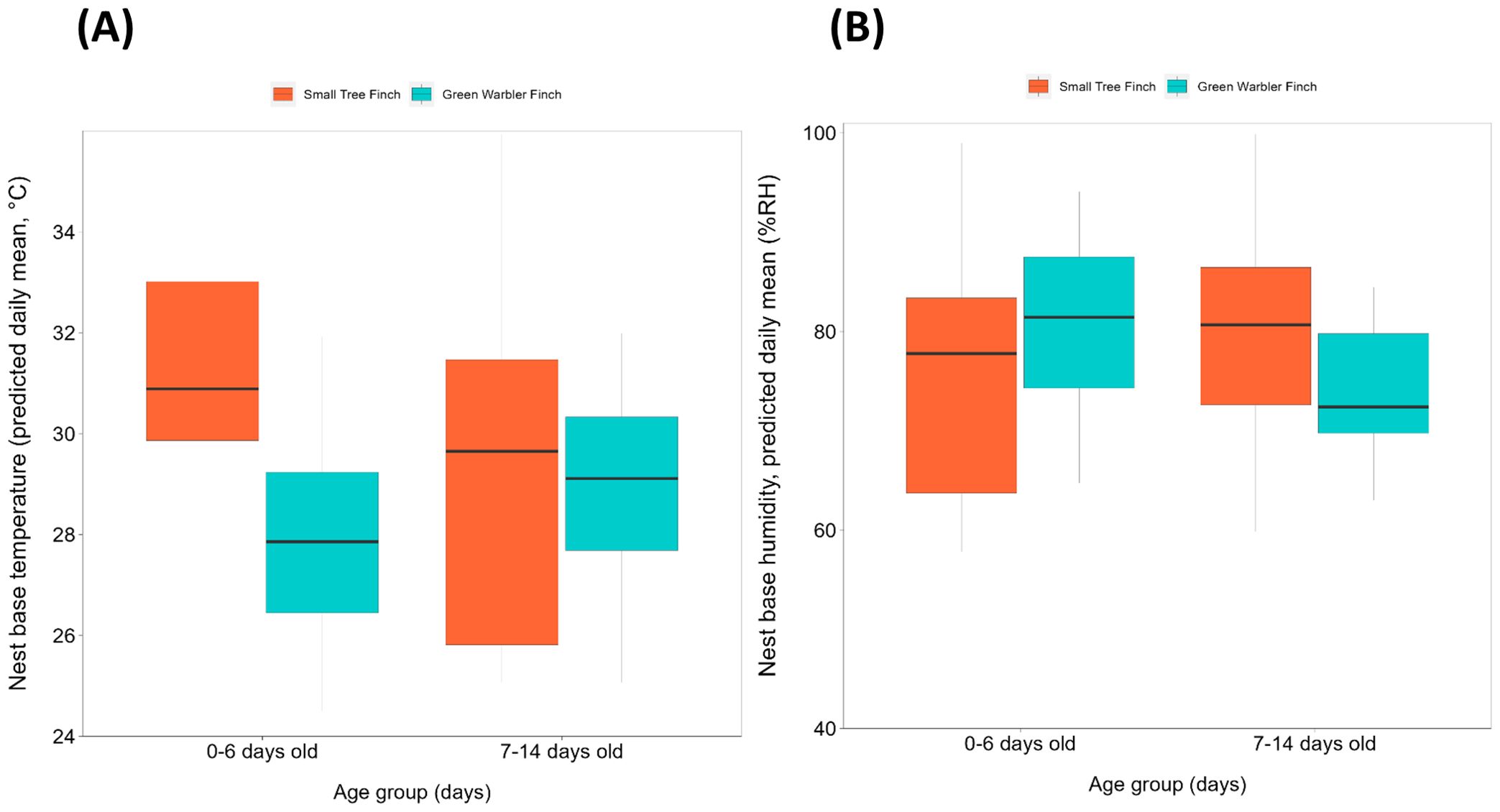
Figure 2. Nest base climate relative to age group (days), per species, for (A) nest base temperature (°C) and (B) nest base humidity (% RH). Species include the small tree finch and the green warbler finch. Both nest base temperature and humidity are displayed as daily means. The box indicate the values within the interquartile range (within 25-75%). The black line inside the boxplot indicates the median. Whisker lines represent values outside the interquartile range, with dots indicating outliers.
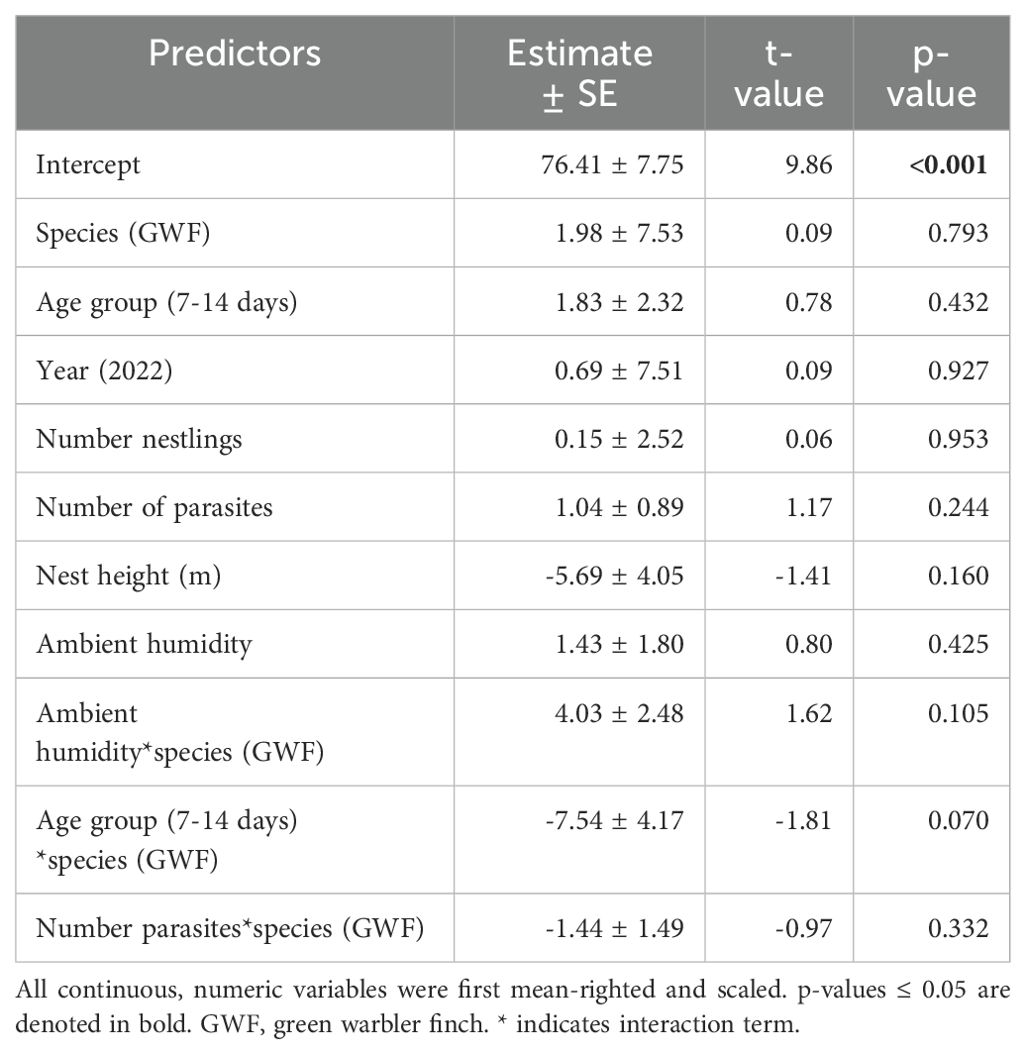
Table 3. Model output of the robust linear mixed model for percent humidity as the dependent variable.
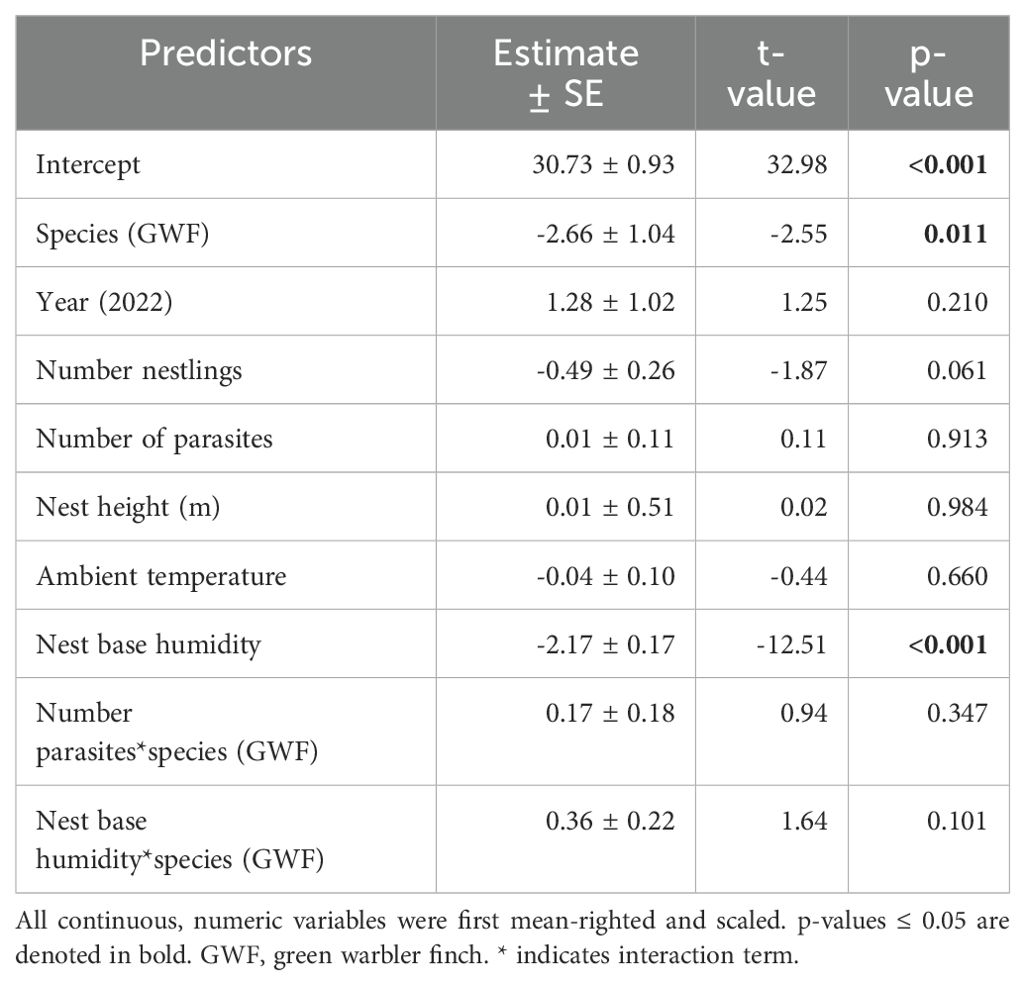
Table 4. Model output of the second robust linear mixed model for nest base temperature as the dependent variable, only including data during the initial days to account for female brooding (0-7 days old nestlings).
3.3 Stable isotopes in nestling blood
Small tree finch nestlings showed significantly higher δ13C levels than green warbler finch nestling (t = -3.57, p = 0.0013; Tables 5, 6, Figure 3A). In contrast, δ15N levels were only marginally lower in small tree finch nestlings compared to green warbler finch nestlings (t = 1.90, p = 0.058; Table 6, Figure 3B). The C:N ratio of nestling blood was not significantly different between the two species (Table 6, Figure 3C). Both the δ15N levels and the C:N ratios were lower in nestlings that survived longer.
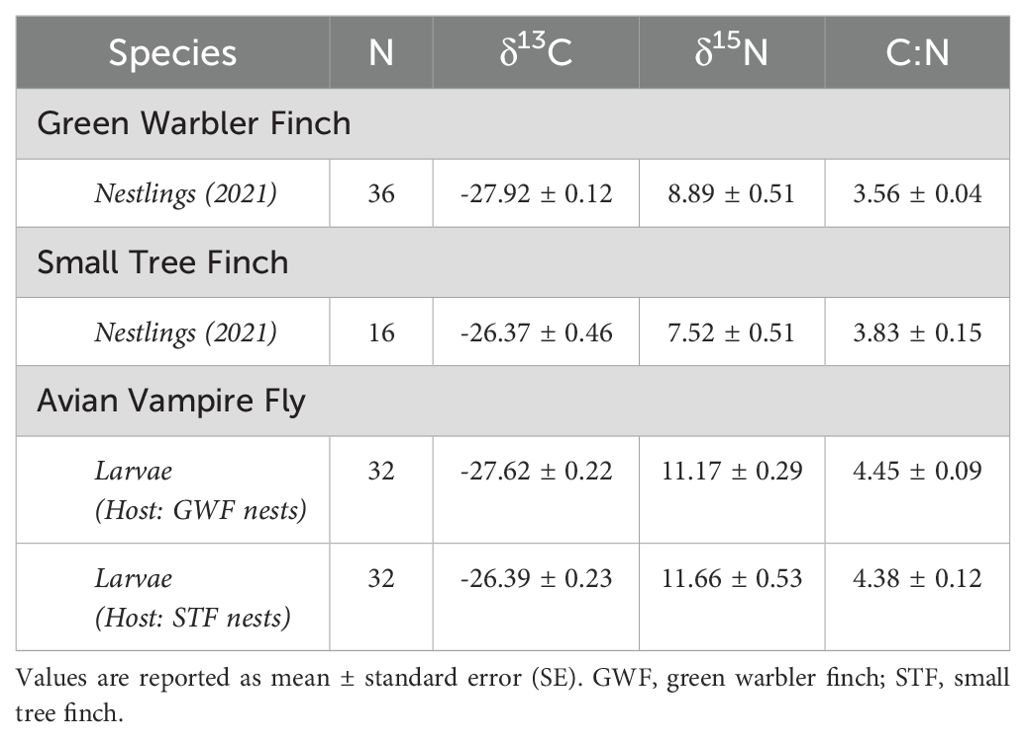
Table 5. Values of stable isotopes for both hosts and parasites including nestling and adult host life stages and larval stage of the avian vampire fly.
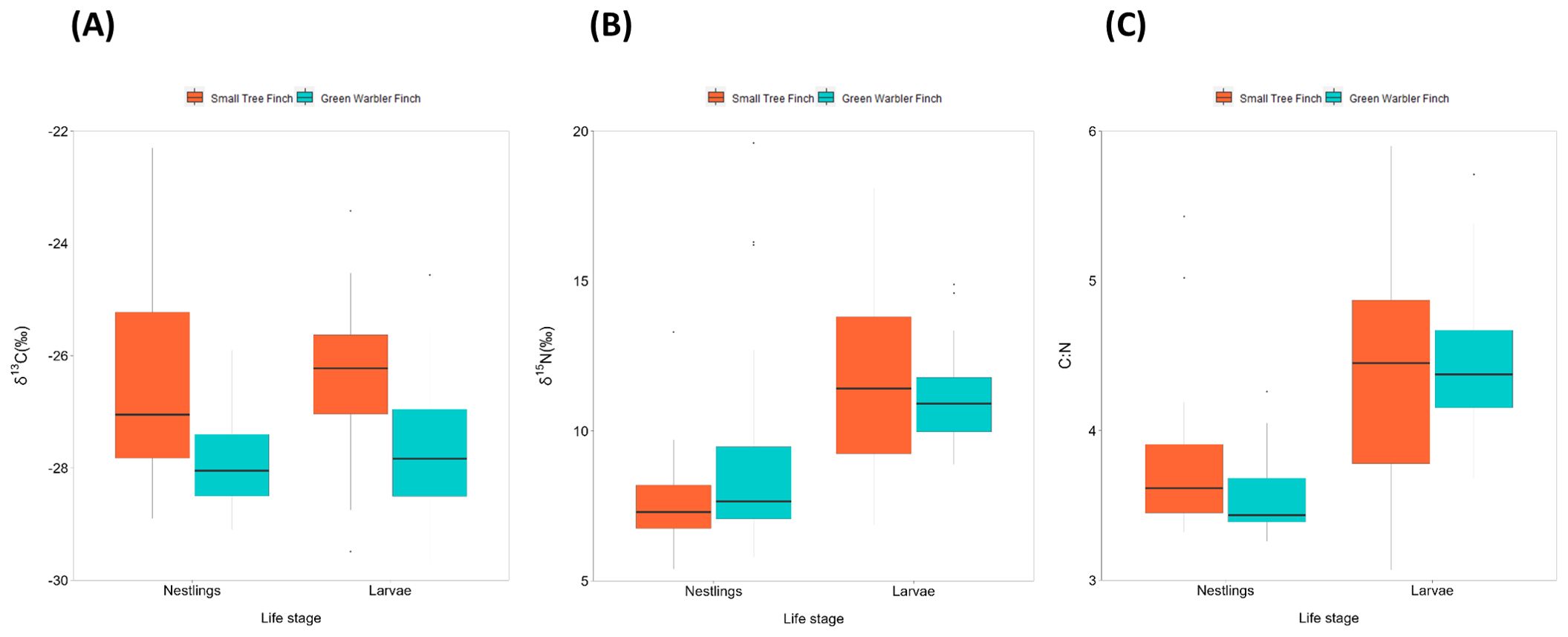
Figure 3. Boxplot of nestling blood and avian vampire fly larvae stable isotope values for (A) δ13C, (B) δ15N, and (C) C:N for the small tree finch and the green warbler finch. The box indicate the values within the interquartile range (within 25-75%). The black line inside the boxplot indicates the median. Whisker lines represent values outside the interquartile range, with dots indicating outliers.
3.4 Amino acids
Amino acid percent composition did not significantly differ between the two host species (Table 7, Figure 4). The day of year each sample was collected, included in the models as a covariate, had a significant effect on percent composition for alanine (z = 2.05, p = 0.04), serine (z = 2.28, p = 0.023), and glutamic acid (z = -2.70, p = 0.007; Table 7).
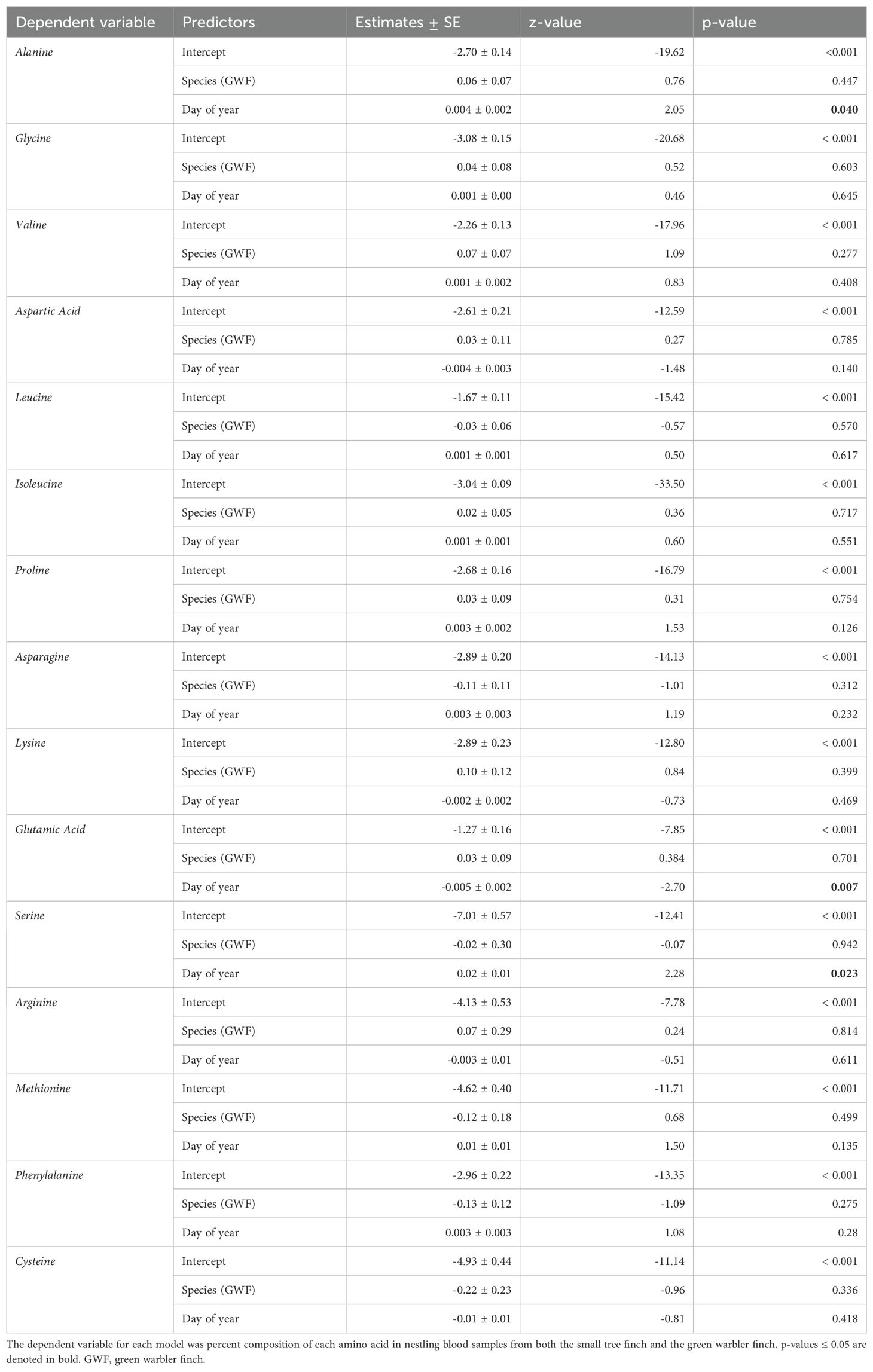
Table 7. Results of a generalized linear mixed model with beta family and logit link, with each amino acid as a separate dependent variable, species and day of year as fixed effects and NestID as a random factor.
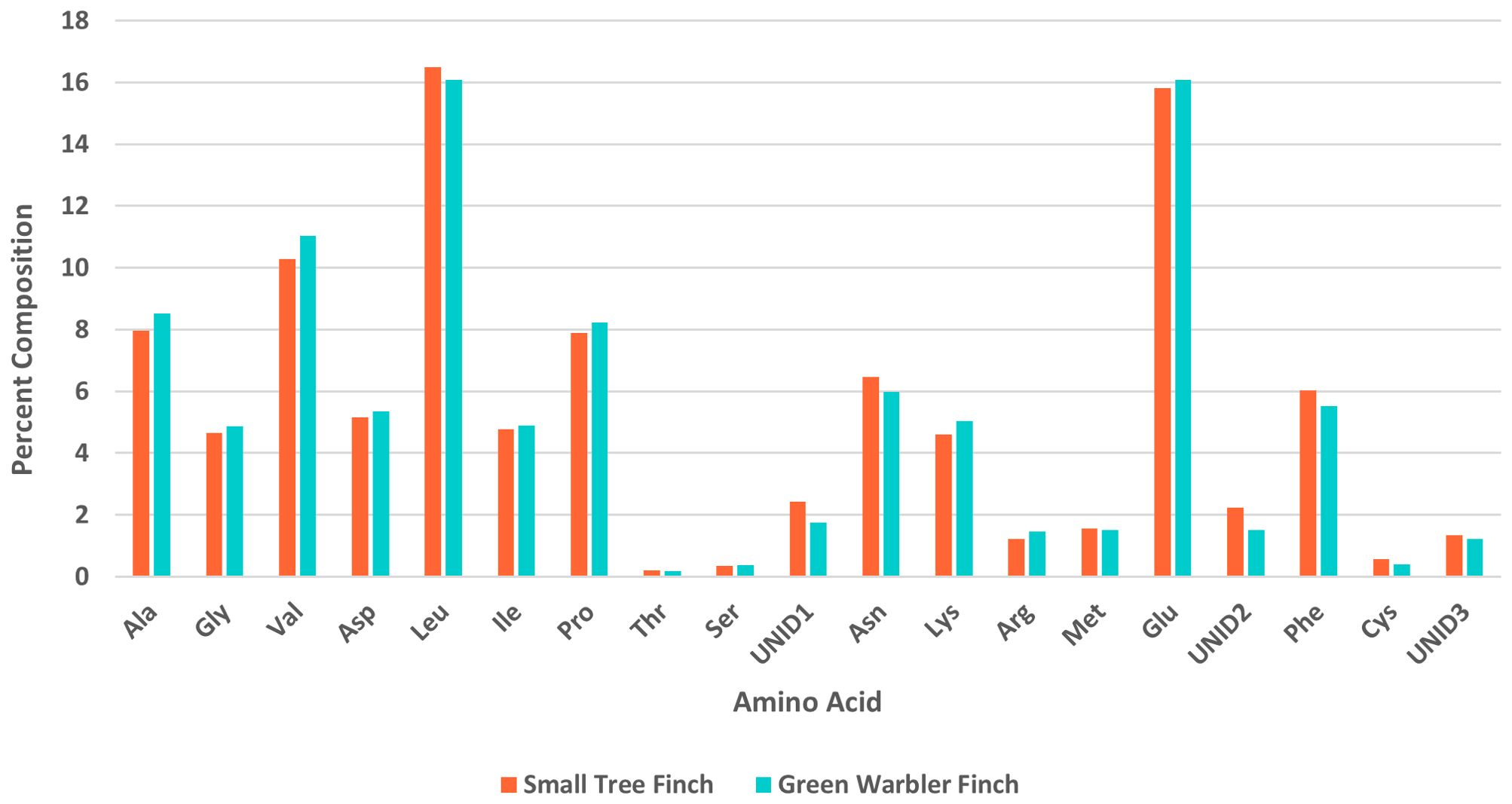
Figure 4. Amino acid composition (%) in nestling blood for the green warbler finch and small tree finch. Ala, alanine; Gly, glycine; Val, valine; Asp, aspartic acid; Leu, leucine; Ile, isoleucine; Pro, proline; Thr, threonine; Ser, serine; UNID1, first unidentified peak; Asn, asparagine; Lys, lysine; Arg, arginine; Met, methionine; Glu, glutamic acid; UNID2, second unidentified peak; Phe, phenylalanine; Cys, cysteine; UNID3, third unidentified peak.
3.5 Stable isotopes in avian vampire fly larvae
δ13C levels were significantly higher in larvae collected from small tree finch nests, compared to larvae from green warbler finch nests (t = -2.50, p = 0.032; Table 8, Figure 3A). δ13C levels increased with day of year (t = 2.61, p = 0.028) and were lower in nests where nestlings survived longer (t = -2.69, p = 0.024) (Table 8). There were no significant differences of larval δ15N nor C:N ratios in larvae collected from the two host species (Table 8, Figure 3).
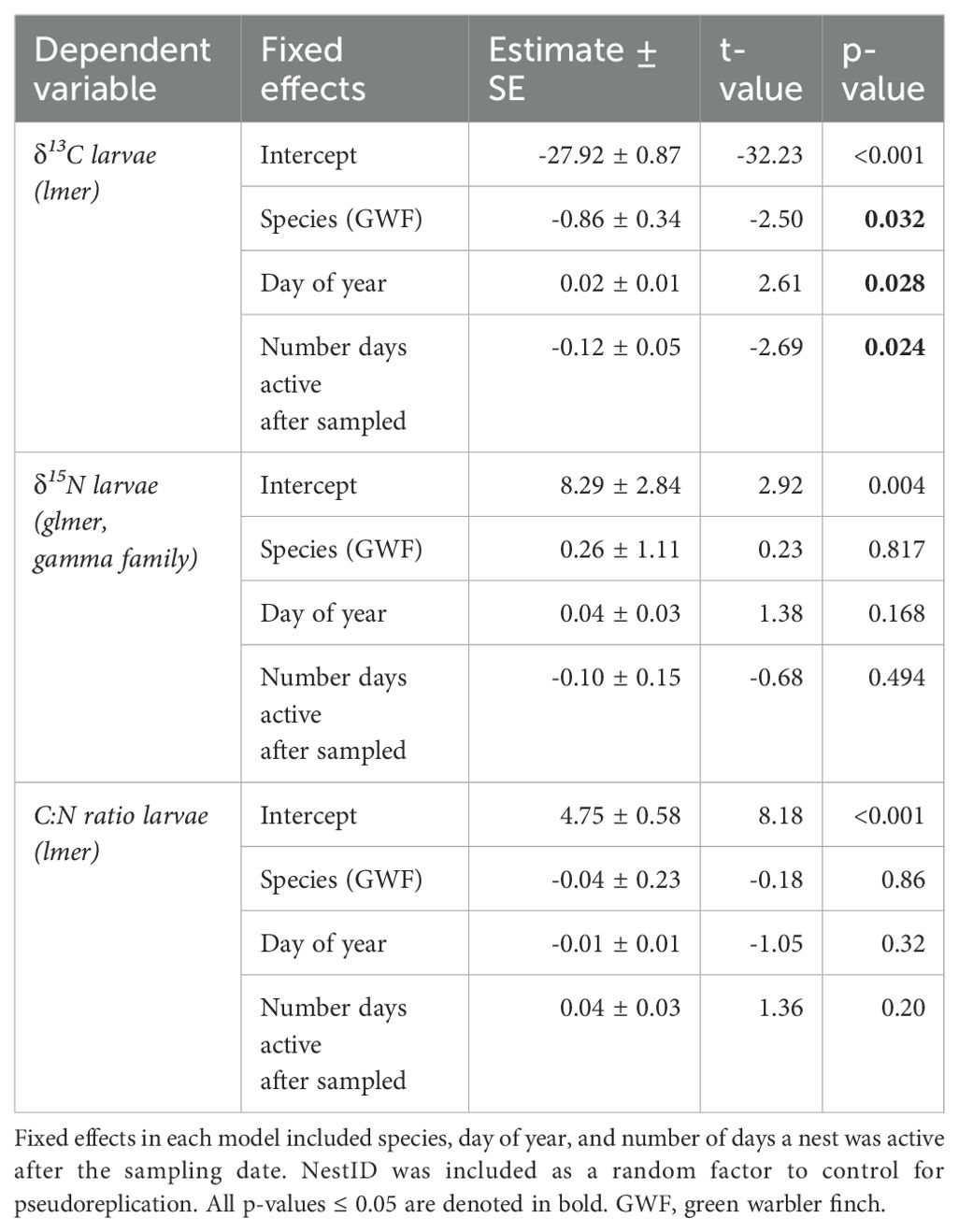
Table 8. Linear mixed models for δ13C and C:N ratio larval values as dependent variables and of a robust linear mixed model for δ15N larval values as dependent variable.
Stable isotope values showed no significant correlation between nestling blood and larvae (pooled values for both species, Spearman correlation test, δ13C: ρ(13) = 0.45, p = 0.094; δ15N: ρ(13) = 0.404, p(13) = 0.137; C:N: ρ(13) = -0.342-, p = 0.212, n = 15; Figure 5). δ15N for green warbler finches and larvae were significantly correlated (ρ(6) = 0.81, p = 0.022, n = 7) and C:N values for small tree finches and larvae marginally (ρ(5) = -0.709, p = 0.074, n = 8) correlated.
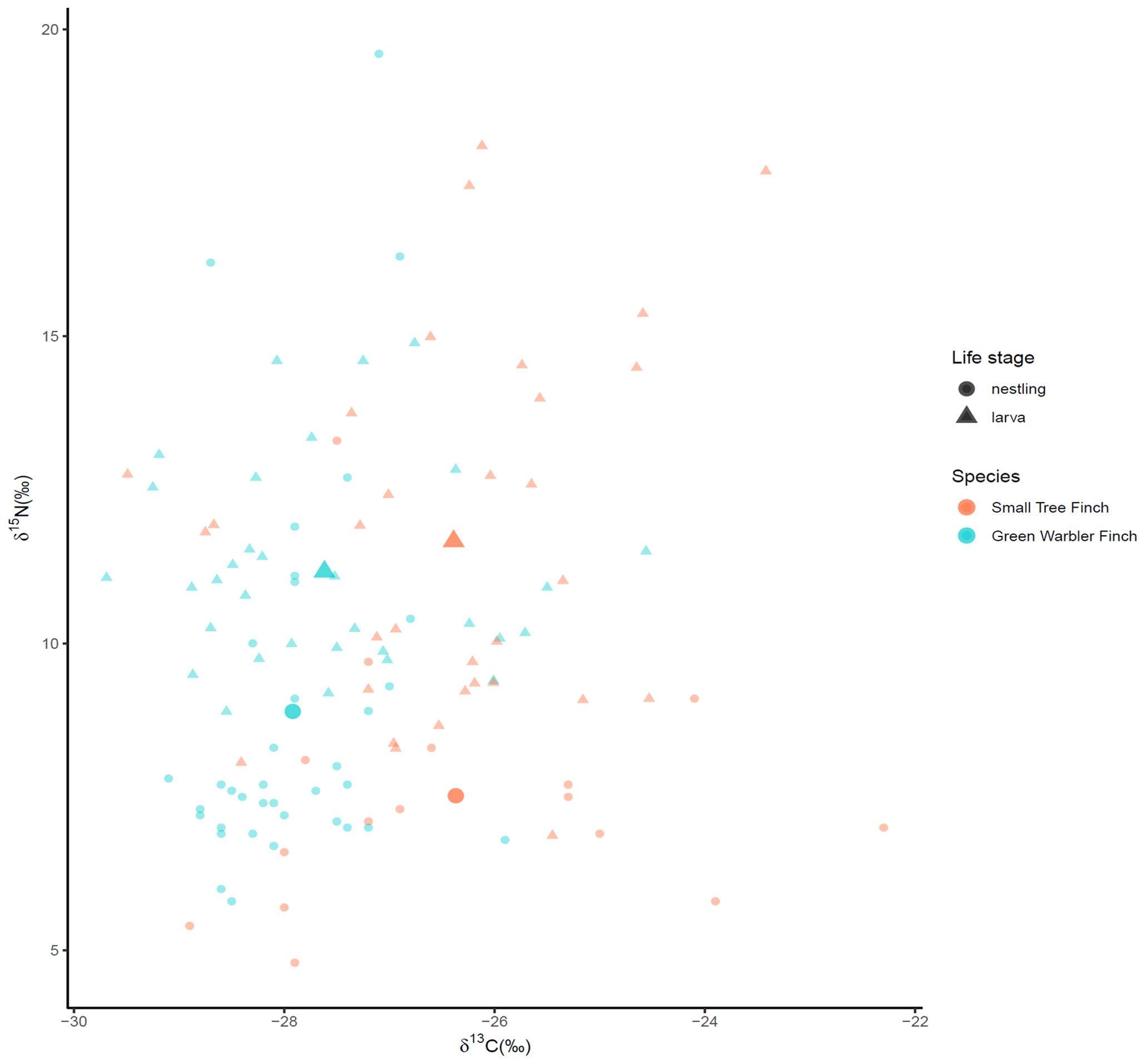
Figure 5. Nestling blood and larval δ13C vs δ15N values. Data are displayed as small diamonds (nestlings) and triangles (larvae) with mean values displayed as large diamonds and triangles.
4 Discussion
Recent studies demonstrated higher parasite levels and faster larval development of hematophagous avian vampire fly larvae in nests of the small tree finch compared to the green warbler finch, two endemic Darwin finch species in the Galapagos (Cimadom et al., 2014; Cimadom and Tebbich, 2021). In this study, we assessed the contribution of two main factors for the growth of the hematophagous larvae, nest climate and the nutritional quality of the host blood. Our results indicate that nest base temperatures were influenced by nest base humidity, with small tree finch females maintaining significantly higher nest base temperatures before predicted fully effective thermoregulation. The nutritional quality of the blood reflected host diet, with small tree finch nestling blood having significantly higher δ13C; however, no significant difference in δ15N, C:N, or amino acid composition was observed. Our data therefore indicate that higher nest temperatures for the small tree finches, rather than blood quality, favor faster development of the hematophagous larvae.
Nest base daily mean temperatures were significantly different between species in relation to daily mean nest base humidity. At lower nest base humidity levels, the small tree finch nests had higher temperatures than the green warbler finch nests; however, as nest base humidity levels increased, the nest base temperature of small tree finches decreased more than that of green warbler finches. At higher humidity levels, it seems neither species can maintain a higher temperature. Small tree finch nests may be better insulated and have lower thermal conductance than green warbler finch nests. This may be potentially due to differences in nest material and/or nest structure, such as nest wall thickness and/or nest hole diameter (Skowron and Kern, 1980; Windsor et al., 2013; Heenan and Seymour, 2011), as the small tree finch nests are larger than green warbler finch nests (ST, CP pers. obs.). At higher humidity levels, evaporative cooling may prevent the maintenance of higher nest base temperatures, which may be aggravated with exposure to wind.
Before predicted fully effective thermoregulation, the presence of females with body temps of typically around 40°C will strongly affect mean nest base temperature and its variation, and hence also favor growth conditions for the parasites. Other potential factors like number of nestlings and nestling size and hence resting metabolic rates (Bushuev et al., 2018) may be of lesser importance given that nestling number and size were not significantly different between species. During the incubation phase, small tree finch females have longer bout durations than green warbler finch females (BK, unpublished data) but it is unknown if higher nest attendance continues into the brooding phase. Beyond nest attendance, small tree finch females are larger than green warbler finch females, and thus may be more efficient for covering the nest base and also for transmitting heat to the nestlings via the brood patch. This difference in temperature and humidity levels in the nest base may affect larval growth and development of nest parasites like the avian vampire fly. The higher temperature in small tree finch nests at an early age may positively influence larval development, accounting for the observed differences in larval stage proportions in Cimadom and Tebbich (2021). Currently, at the CDRS laboratory, 2nd instar avian vampire fly larvae are reared at 30-31°C and 50-70% relative humidity (RH) (CDF, unpublished data) after adjustments from 36°C and 80-95% RH (Lahuatte et al., 2016), which improved larval production. Other studies on insects have reported faster larval growth and development in lab conditions ranging from 32-35°C and at 75% RH compared to lower temperatures and 55% RH (e.g., lesser mealworm: Kotsou et al., 2021; mealworm: Machona et al., 2023; nest fly: Bennett and Whitworth, 1991; black soldier fly: Palma et al., 2019). Additionally, lower temperatures (e.g. 20°C) may in fact stunt larval development, as reported for the mealworm Tenebrio molitor (Machona et al., 2023). Our two focal species had mean percent RH values within or near the range that the avian vampire fly lab currently uses for rearing the avian vampire fly.
To date there is no systematic study on the optimal temperature and humidity levels required for optimal growth of the other larval stages of the avian vampire fly in the laboratory. Further studies should be conducted on how temperature and humidity affect larval development and growth in the avian vampire, these are necessary to determine optimal developmental conditions. Additionally, further information on how nest climate varies between other host species in similar and different habitats in Galapagos would assist us in understanding the avian vampire fly’s thermal thresholds and the ranges for larval development in the wild and hence inform conservation measures related to parasite control.
Another factor that could influence larval development and growth is the quality of the host blood. Contrary to our prediction, blood C:N ratios were not significantly different between the two bird species, but most interestingly, nestlings with lower C:N ratios survived longer. Nestlings with more larval parasites may also have had depleted protein levels (and therefore higher blood C:N) due to parasitism, weakening them and causing these nestlings to die at an earlier age than those with lower C:N blood ratios. Alternatively, or additionally, diet composition may interact with other biotic factors such as the larval microbiota that influence larval development. Bacterial communities in avian vampire fly larvae collected from green warbler finch nests versus tree finch nests including the small tree finch, were significantly different in a prior study (Ben-Yosef et al., 2017). In this study, larvae from green warbler finch nests had microbial communities commonly with Serratia and Acinetobacter bacteria, while larvae from small tree finches had differing genera of bacteria (Ben-Yosef et al., 2017). These bacterial communities may interact with diet composition and inhibit larval development, as reported for tephritid flies with Serratia bacteria (Fitt and O'Brien, 1985).
Dietary differences between small tree finch and green warbler finch were detectable in nestling blood composition and consequently in the hematophagous parasitic larvae. The δ13C values were higher both in the blood of the more omnivorous small tree finch nestlings and in their Philornis larvae. C isotope ratios (δ13C) are also relatively conserved across trophic levels, but vary depending on the photosynthetic pathways and the degree of water stress experienced at the base of the food web (Peterson and Fry, 1987; Still and Powell, 2010; Basu et al., 2015). Thus, higher δ13C values in small tree finch nestling blood suggest that their food web contains more C4 plants comparative to that of green warbler finch nestlings. Other factors, such as the proportion of lipids in the blood, can also affect isotopic δ13C values (Cherel et al., 2005), as lipids have a more negative δ13C than proteins (DeNiro and Epstein, 1977). We did not defat blood samples of either host species; however, variations of the amount of fat in avian diets may affect lipid levels in the blood of certain avian species (Bavelaar and Beynen, 2003; Regar et al., 2019; although see Baighi and Nobakht, 2017). Therefore, the observed differences in δ13C in our host species may, in fact, be partially attributed to blood lipid level differences between the two species.
δ15N generally increases 3-4‰ from diet to consumer, as 15N is more highly retained than 14N (DeNiro and Epstein, 1981). In our study, green warbler finch nestling blood δ15N signatures were on average 1.5‰ higher compared to small tree finch nestling blood, suggesting dietary sources of the green warbler finch are half a level up on the trophic ladder (assuming an average diet to consumer enrichment of 3‰) and therefore more protein rich. The higher δ15N of the green warbler finch is in line with the observation of a more insectivorous diet, compared to the small tree finch who partly also consume seeds and plant materials (Loo et al., 2019).
Despite the suggested difference in trophic levels of small tree finch and green warbler finch nestlings, the hematophagous Philornis larvae in the nests of the two species did not differ significantly in δ15N. Our results indicate a characteristic trophic shift from host to larvae of ~2 – 4‰ δ15N and ~0.02 – 0.3‰ δ13C isotopic enrichment, similar to other studies on host-parasite enrichment (Post, 2002; Gómez-Díaz and González-Solís, 2010). However, the δ13C isotopic enrichment for small tree finch nestlings and their larvae was quite low in our study. The absence of differences in δ15N between larvae from nests of both species could be possibly explained by the fact that the larvae are probably under protein stress or starvation conditions (particularly from nests with dead or dying nestlings), which often increases the delta δ15N signature (Olive et al., 2003), potentially overriding the trophic signature. However, when testing for correlations between nestlings and larvae from their nests, by species separately, we did find green warbler finch and larval δ15N were highly correlated; however, this must be interpreted cautiously due to low sample size (n= 7).
Despite the differences in the dietary composition of the small tree finch and the green warbler finch nestlings observed in previous studies (e.g., Filek et al., 2018) and herein confirmed by the isotopic signatures, there were no significant differences in the amino acid percent composition between species. Additionally, due to limitations in our methodology, some amino acids, including tyrosine, tryptophan, and histidine were not detected/quantified for the analysis of blood amino acid composition. However, we did include three unidentified peaks (probable amino acids) in our percent composition calculations, which may have accounted for some of the missing amino acids. Given C:N in nestling blood was not significantly different between species, it is not surprising that the amino acid composition was also similar between species. Protein deficiencies or increases in protein can influence amino acid concentrations in bird blood (Hebert et al., 2002); however, in a prior study on herring gulls, these differences were only detected between gulls in different habitats, not ones occupying the same habitat. This suggests blood quality of avian vampire fly hosts may also be more influenced by differences in habitat and location than by different hosts occupying the same habitat.
Host quality between the small tree finch and the green warbler finch does not appear to vary significantly in our study, based on the parameters/characteristics investigated. However, these species may still differ in quality for avian vampire fly, in other factors that we did not measure but that directly influence larval development, or other life history factors that may influence oviposition success and/or larval survival. One limitation in our study was that we only measured blood values when nestlings were 5-6 days old. It may be that prey composition differs between species earlier or later in the nestling phase, potentially influencing C:N blood values. Additionally, while we investigated host blood quality, we did not investigate the hosts’ tissue quality. As avian vampire fly larvae also feed on nestlings’ tissue (Fessl et al., 2006), the C:N of host tissue may influence larval development/growth. We also only measured relative abundance of each amino acid and not total amounts or concentrations of each amino acid in the blood as this would have been logistically very difficult. Thus, the small tree finch nestlings may have higher concentrations of amino acids that could influence avian vampire fly larval development, but we could not detect this. Further investigation into C:N and amino acid composition in tissue and host blood and tissue of younger and older nestlings and brooding females may provide additional insight into diet quality for avian vampire fly larval growth and/or development.
This study is the first to investigate directly the differences in nest and blood quality between hosts of the avian vampire fly and the trophic impacts of diet quality on larval values of C and N stable isotopes. This data not only informs about the quality of diet of the larvae of avian vampire fly but also contributes general knowledge on the diet of endemic Darwin’s finches. The avian vampire fly has a wide range of host species; thus, future studies could include investigating the factors related to the avian vampire fly’s host preferences. These studies could include investigating nest conditions, microbiomes and optimal levels of protein in the diet of many other host species of the avian vampire fly larvae. Additionally, immune responses of the hosts may influence larval development, so it may also be beneficial to investigate nestlings’ immune responses. Furthermore, there is currently limited information on the factors controlling larval development and growth in the field, which is important information for parasite control. Therefore, our study provides insights into host quality for an invasive parasite in the Galapagos useful for planning future studies and control initiatives. Additionally, these data will provide insight into natural conditions for larval growth and development that may help in optimizing the laboratory conditions for mass rearing of the avian vampire fly for the purpose of developing other control methods such as the sterile male technique. We therefore suggest building off our study by examining the effects of temperature variation in larval growth and development in experimental conditions, which may inform optimal conditions for mass rearing of avian vampire fly larvae.
Data availability statement
The datasets presented in this study can be found in online repositories. The names of the repository/repositories and accession number(s) can be found below: https://doi.org/10.6084/m9.figshare.25329202.v1, Figshare repository.
Ethics statement
The animal study was approved by Animal Ethics and Experimental Board from the Faculty of Life Sciences at the University of Vienna and the Galapagos National Park Directorate. The study was conducted in accordance with the local legislation and institutional requirements.
Author contributions
CP: Data curation, Formal analysis, Investigation, Project administration, Visualization, Writing – original draft, Writing – review & editing. RH-N: Data curation, Funding acquisition, Investigation, Methodology, Project administration, Resources, Writing – review & editing. BK: Data curation, Formal analysis, Investigation, Visualization, Writing – review & editing. HR: Methodology, Validation, Visualization, Writing – review & editing, Funding acquisition. ST: Conceptualization, Funding acquisition, Methodology, Project administration, Resources, Supervision, Writing – review & editing.
Funding
The author(s) declare financial support was received for the research, authorship, and/or publication of this article. Open access funding provided by Austrian Science Fund (FWF). This study was funded by a grant awarded to ST and RH-N by the Austrian Science Fund (grant number P 32555-B). For open access purposes, the author has applied a CC BY public copyright license to any author accepted manuscript version arising from this submission.
Acknowledgments
We thank our field assistants, Eileen Heyer, Tatiana Torres, Nikolaus Filek, Jefferson Garcia Loor, Sergio Alvarez, and Alexander Schmied for their help and dedication, including long hours in the field. We also thank Charles Darwin Research Station staff, including Charlotte Causton and Birgit Fessl for their assistance with obtaining permits in the Galapagos and David Anchundia for his advice in the field and assistance at the study site. Thank you to the Charles Darwin Foundation and to Rolf Sievers for providing the long-term weather data for Santa Cruz and to Clare Peabody for compiling and analyzing these data. We also thank Elizabeth Ziss for her assistance with stable isotope lab analysis, Stephanie Primisser for her help troubleshooting the gas chromatograph, and Valeria Medoro for her assistance with amino acids in the lab. The content of this manuscript was previously included in the PhD dissertation of CP at the University of Vienna (Pike, 2024). We would like to thank the Galapagos National Park, including Christian Sevilla, counterpart from the Galapagos National Park. This study was conducted with permission from the Galapagos National Park (project permit numbers: PC-11-20, PC-24-20, Anexo de Muestras MAE-DPNG-IA-2020-08, Oficio Nro. MAAE-DPNG/DGA-2020-0685-O, PC-06-21, PC-08-21, Anexo de Muestras MAE-DPNG-IA-2021-05, PC-30-22 and PC-20-22). This publication is contribution number 2547 of the Charles Darwin Foundation for the Galapagos Islands.
Conflict of interest
The authors declare that the research was conducted in the absence of any commercial or financial relationships that could be construed as a potential conflict of interest.
Publisher’s note
All claims expressed in this article are solely those of the authors and do not necessarily represent those of their affiliated organizations, or those of the publisher, the editors and the reviewers. Any product that may be evaluated in this article, or claim that may be made by its manufacturer, is not guaranteed or endorsed by the publisher.
Supplementary material
The Supplementary Material for this article can be found online at: https://www.frontiersin.org/articles/10.3389/fevo.2024.1398353/full#supplementary-material
References
Anchundia D., Fessl B. (2021). The conservation status of the Galapagos Martin Progne modesta: Assessment of historical records and results of recent surveys. Bird Conserv. Int. 31, 129–138. doi: 10.1017/S095927092000009X
Baighi G., Nobakht A. (2017). The effects of different levels of saturated and unsaturated fats and their composition in growing and finishing periods on productive performance and blood lipids of broilers. Iranian J. Appl. Anim. Sci. 7, 329–336.
Balagawi S., Jackson K., Ul Haq I., Hood-Nowotny R., Resch C., Clark A. R. (2014). Nutritional status and the foraging behaviour of Bactrocera tryoni with particular reference to protein bait spray. Physiol. Entomology 39, 33–43. doi: 10.1111/phen.12045
Basu S., Agrawal S., Sanyal P., Mahato P., Kumar S., Sarkar A. (2015). Carbon isotopic ratios of modern C3–C4 plants from the Gangetic Plain, India and its implications to paleovegetational reconstruction. Palaeogeography Palaeoclimatology Palaeoecol. 440, 22–32. doi: 10.1016/j.palaeo.2015.08.012
Bates D., Mächler M., Bolker B., Walker S. (2015). Fitting linear mixed-effects models using lme4. J. Stat. Software 67, 1–48. doi: 10.18637/jss.v067.i01
Bavelaar F. J., Beynen A. (2003). Influence of amount and type of dietary fat on plasma cholesterol concentrations in African grey parrots. J. Appl. Res. Veterinary Med. 1, 1–8.
Bennett G. F., Whitworth T. L. (1991). Studies on the life history of some species of Protocalliphora (Diptera: Calliphoridae). Can. J. Zoology 69, 2048–2058. doi: 10.1139/z91-286
Ben-Yosef M., Zaada D. S. Y., Dudaniec R. Y., Pasternak Z., Jurkevitch E., Smith R. J., et al. (2017). Host-specific associations affect the microbiome of Philornis downsi, an introduced parasite to the Galápagos Islands. Mol. Ecol. 26, 4644–4656. doi: 10.1111/mec.14219
Bertrand M., Chabin A., Brack A., Westall F. (2008). Separation of amino acid enantiomers via chiral derivatization and non-chiral gas chromatography. J. Chromatogr. A 1180, 131–137. doi: 10.1016/j.chroma.2007.12.004
Bize P., Jeanneret C., Klopfenstein A., Roulin A. (2008). What makes a host profitable? Parasites balance host nutritive resources against immunity. Am. Nat. 171, 107–118. doi: 10.1086/523943
Brooks M. E., Kristensen K., van Benthem K. J., Magnusson A., Berg C. W., Nielsen A., et al. (2017). glmmTMB balances speed and flexibility among packages for zero-inflated generalized linear mixed modeling. R J. 9, 378–400. doi: 10.32614/RJ-2017-066
Bushuev A., Tolstenkov O., Zubkova E., Solovyeva E., Kerimov A. (2018). Basal metabolic rate in free-living tropical birds: The influence of phylogenetic, behavioral, and ecological factors. Curr. Zoology 64, 33–43. doi: 10.1093/cz/zox018
Cantarero A., López-Arrabé J., Rodríguez-García V., González-Braojos S., Ruiz-DeCastañeda R., Redondo A. J., et al. (2013). Factors affecting the presence and abundance of generalist ectoparasites in nests of three sympatric hole-nesting bird species. Acta Ornithologica 48, 39–54. doi: 10.3161/000164513X669982
Castaño-Vázquez F., Merino S. (2022). Differential effects of environmental climatic variables on parasite abundances in blue tit nests during a decade. Integrative Zoology 17, 511–29. doi: 10.1111/1749-4877.12625
Chang C. L. (2004). Effect of amino acids on larvae and adults of Ceratitis capitata (Diptera: Tephritidae). Ann. Entomological Soc. America 97, 529–535. doi: 10.1603/0013-8746(2004)097[0529:EOAAOL]2.0.CO;2
Cherel Y., Hobson K. A., Bailleul F., Groscolas R. (2005). Nutrition, physiology, and stable isotopes: New information from fasting and molting penguins. Ecology 86, 2881–2888. doi: 10.1890/05-0562
Cimadom A., Jäger H., Schulze C. H., Hood-Nowotny R., Wappl C., Tebbich S. (2019). Weed management increases the detrimental effect of an invasive parasite on arboreal Darwin’s finches. Biol. Conserv. 233, 93–101. doi: 10.1016/j.biocon.2019.02.025\
Cimadom A., Tebbich S. (2021). Timing of infestation influences virulence and parasite success in a dynamic multi-host-parasite interaction between the invasive parasite, Philornis downsi, and Darwin's finches. Oecologia 195, 249–259. doi: 10.1007/s00442-020-04807-5
Cimadom A., Ulloa A., Meidl P., Zöttl M., Zöttl E., Fessl B., et al. (2014). Invasive parasites, habitat change and heavy rainfall reduce breeding success in Darwin’s finches. PloS One 9, e107518. doi: 10.1371/journal.pone.0107518
Coloma A., Anchundia D., Piedrahita P., Pike C., Fessl B. (2020). Observations on the nesting of the Galapagos Dove Zenaida galapagoensis in Galapagos, Ecuador. Galapagos Res. 69, 34–38.
Common L. K., O’Connor J. A., Dudaniec R. Y., Peters K. J., Kleindorfer S. (2020). Evidence for rapid downward fecundity selection in an ectoparasite (Philornis downsi) with earlier host mortality in Darwin’s finches. J. Evolutionary Biol. 33, 524–533. doi: 10.1111/jeb.13588
Davis G. R. F. (1975). Essential dietary amino acids for growth of larvae of the yellow mealworm, Tenebrio molitor L. J. Nutr. 105, 1071–1075. doi: 10.1093/jn/105.8.1071
DeNiro M. J., Epstein S. (1977). Mechanism of carbon isotope fractionation associated with lipid synthesis. Science 197, 261–263. doi: 10.1126/science.327543
DeNiro M. J., Epstein S. (1981). Influence of diet on the distribution of nitrogen isotopes in animals. Geochimica Cosmochimica Acta 45, 341–351. doi: 10.1016/0016-7037(81)90244-1
Dunn E. H. (1975). The timing of endothermy in the development of altricial birds. Condor. 77, 288–293. doi: 10.2307/1366224
Dunn E. H. (1976). The relationship between brood size and age of effective homeothermy in nestling house wrens. Wilson Bull. 88, 478–482.
Dunn E. H. (1979). Age of effective homeothermy in nestling tree swallows according to brood size. Wilson Bull. 91, 455–457.
Fessl B., Heimpel G. E., Causton C. E. (2018). ““Invasion of an avian nest parasite, Philornis downsi, to the Galapagos Islands: Colonization history, adaptations to novel ecosystems, and conservation challenges,” in Disease ecology: galapagos birds and their parasites. Ed. Parker P. G. (Springer, Cham), 213–266. doi: 10.1007/978-3-319-65909-1_9
Fessl B., Sinclair B. J., Kleindorfer S. (2006). The life-cycle of Philornis downsi (Diptera: Muscidae) parasitizing Darwin's finches and its impacts on nestling survival. Parasitology 133, 739–747. doi: 10.1017/S0031182006001089
Filek N., Cimadom A., Schulze C. H., Jäger H., Tebbich S. (2018). The impact of invasive plant management on the foraging ecology of the Warbler Finch (Certhidea olivacea) and the Small Tree Finch (Camarhynchus parvulus) on Galápagos. J. Ornithology 159, 129–140. doi: 10.1007/s10336-017-1481-4
Fitt G. P., O'Brien R. W. (1985). Bacteria associated with four species of Dacus (Diptera: Tephritidae) and their role in the nutrition of the larvae. Oecologia 67, 447–454. doi: 10.1007/BF00384954
Gómez-Díaz E., González-Solís J. (2010). Trophic structure in a seabird host-parasite food web: Insights from stable isotope analyses. PloS One 5, e10454. doi: 10.1371/journal.pone.0010454
Hartig F. (2022). DHARMa: Residual diagnostics for hierarchical (multi-level / mixed) regression models. R package version 0.4.6. Available online at: https://CRAN.R-project.org/package=DHARMa.
Hebert C. E., Shutt J. L., Ball R. O. (2002). Plasma amino acid concentrations as an indicator of protein availability to breeding Herring Gulls (Larus Argentatus). Auk 119, 185–200. doi: 10.1093/auk/119.1.185
Heeb P., Kölliker M., Richner H. (2000). Bird-ectoparasite interactions, nest humidity and ectoparasite community structure. Ecology 81, 958–968. doi: 10.1890/0012-9658(2000)081[0958:BEINHA]2.0.CO;2
Heenan C. B., Seymour R. S. (2011). Structural support, not insulation, is the primary driver for avian cup-shaped nest design. Proc. R. Soc. B - Biol. Sci. 278, 2924–2929. doi: 10.1098/rspb.2010.2798
Heyer E., Cimadom A., Wappl C., Tebbich S. (2021). Parental care in the Small Tree Finch Camarhynchus parvulus in relation to parasitism and environmental factors. Ibis 163, 137–149. doi: 10.1111/ibi.12845
Hinton T., Theriault Noyes D., Ellis J. (1951). Amino acids and growth factors in a chemically defined medium for Drosophila. Physiol. Zoology 24, 335–353. doi: 10.1086/physzool.24.4.30152141
Hood-Nowotny R., Knols B. G. J. (2007). Stable isotope methods in biological and ecological studies of arthropods. Entomologia Experimentalis Applicata 124, 3–16. doi: 10.1111/j.1570-7458.2007.00572.x
Hood-Nowotny R., Rabitsch I., Cimadom A., Suarez-Rubio M., Watzinger A., Schmidt Yáñez P., et al. (2023). Plant invasion causes alterations in Darwin's finch feeding patterns in Galápagos cloud forests. Sci. Total Environ. 895, 164990. doi: 10.1016/j.scitotenv.2023.164990
Hood-Nowotny R., Schwarzinger B., Schwarzinger C., Soliban S., Madakacherry O., Aigner M., et al. (2012). An analysis of diet quality, how it controls fatty acid profiles, isotope signatures and stoichiometry in the malaria mosquito Anopheles arabiensis. PloS One 7, e45222. doi: 10.1371/journal.pone.0045222
Jäger H., Buchholz S., Cimadom A., Tebbich S., Rodríguez J., Barrera D., et al. (2017). ““Restoration of the blackberry-invaded scalesia forest: impacts on the vegetation, invertebrates, and birds“,” in Galapagos report 2015-2016, eds. GNPS, GCREG, CDF and GC (DPNG, Puerto Ayora), 142–148.
Kleindorfer S., Dudaniec R. Y. (2009). Love thy neighbour? Social nesting pattern, host mass and nest size affect ectoparasite intensity in Darwin's tree finches. Behav. Ecol. Sociobiology 63, 731–739. doi: 10.1007/s00265-008-0706-1
Koller M. (2016). robustlmm: An R package for robust estimation of linear mixed-effects models. J. Stat. Software 75, 1–24. doi: 10.18637/jss.v075.i06
Kotsou K., Rumbos C. I., Baliota G. V., Gourgouta M., Athanassiou C. G. (2021). Influence of temperature, relative humidity and protein content on the growth and development of larvae of the lesser mealworm, Alphitobius diaperinus (Panzer). Sustainability 13, 11087. doi: 10.3390/su131911087
Kuznetsova A., Brockhoff P. B., Christensen R. H. B. (2017). lmerTest package: Tests in linear mixed effects models. J. Stat. Software 82, 1–26. doi: 10.18637/jss.v082.i13
Lahuatte P. F., Lincango M. P., Heimpel G. E., Causton C. E. (2016). Rearing larvae of the avian nest parasite, Philornis downsi (Diptera: Muscidae), on chicken blood-based diets. J. Insect Sci. 16, 84. doi: 10.1093/jisesa/iew064
Lange B., Reuter M., Ebert D., Muylaert K., Decaestecker E. (2014). Diet quality determines interspecific parasite interactions in host populations. Ecol. Evol. 4, 3093–3102. doi: 10.1002/ece3.1167
Lemoine M., Doligez B., Passerault M., Richner H. (2011). Influence of host profitability and microenvironmental conditions on parasite specialization on a main and an alternative hosts. J. Evolutionary Biol. 24, 1212–1225. doi: 10.1111/j.1420-9101.2011.02252.x
Loo W. T., García-Loor J., Dudaniec R. Y., Kleindorfer S., Cavanaugh C. M. (2019). Host phylogeny, diet, and habitat differentiate the gut microbiomes of Darwin's finches on Santa Cruz Island. Sci. Rep. 9, 18781. doi: 10.1038/s41598-019-54869-6
Lüdecke D. (2021). sjPlot: Data Visualization for Statistics in Social Science. R package version 2.8.10. Available online at: https://CRAN.R-project.org/package=sjPlot.
Machona O., Chiweshe T., Chidzwondo F., Mangoyi R. (2023). Investigating the optimal conditions for rearing and breeding Tenebrio molitor in Zimbabwe. J. Biochem. Technol. 14, 27–33. doi: 10.51847/2rdpiKkqm7
Manzoli D. E., Saravia-Pietropaolo M. J., Antoniazzi L. R., Barengo E., Arce S. I., Quiroga M. A., et al. (2018). Contrasting consequences of different defence strategies in a natural multihost–parasite system. Int. J. Parasitolology 48, 445–455. doi: 10.1016/j.ijpara.2017.11.001
Manzoli D. E., Saravia-Pietropaolo M. J., Arce S. I., Percara A., Antoniazzi L. R., Beldomenico P. M. (2021). Specialist by preference, generalist by need: Availability of quality hosts drives parasite choice in a natural multihost–parasite system. Int. J. Parasitol. 51, 527–534. doi: 10.1016/j.ijpara.2020.12.003
McNew SM., Clayton DH. (2018). Alien Invasion: Biology of Philornis Flies Highlighting Philornis downsi, an Introduced Parasite of Galápagos Birds. Annu Rev Entomol. 63, 369–387. doi: 10.1146/annurev-ento-020117-043103
Mennerat A., Charmantier A., Perret P., Hurtrez-Boussès S., Lambrechts M. M. (2021). Parasite intensity is driven by temperature in a wild bird. Peer Community J. 1, e60. doi: 10.1101/323311
Meyhoff S. D., Johnson D. L., Ellert B. H., Lutes K. (2023). Seasonal changes of stable isotope signals in the primary feathers of plains sharp-tailed grouse. Wildlife Soc. Bull. 47, e1412. doi: 10.1002/wsb.1412
Michielsen R., Ausems A., Jakubas D., Pętlicki M., Plenzler J., Shamoun-Baranes J., et al. (2019). Nest characteristics determine nest microclimate and affect breeding output in an Antarctic seabird, the Wilson’s storm-petrel. PloS One 14, e0217708. doi: 10.1371/journal.pone.0217708
Olive P. J. W., Pinnegar J. K., Polunin N. V. C., Richards G., Welch R. (2003). Isotope trophic-step fractionation: a dynamic equilibrium model. J. Anim. Ecol. 72, 608–617. doi: 10.1046/j.1365-2656.2003.00730.x
Palma L., Fernandez-Bayo J., Niemeier D., Pitesky M., VanderGheynst J. S. (2019). Managing high fiber food waste for the cultivation of black soldier fly larvae. NPJ Sci. Food 3, 15. doi: 10.1038/s41538-019-0047-7
Peterson B. J., Fry B. (1987). Stable isotopes in ecosystem studies. Annu. Rev. Ecol. Systematics 18, 293–320. doi: 10.1146/annurev.es.18.110187.001453
Pike C. (2024). Philornis downsi, in different host nests in the Galapagos Islands. [dissertation]. University of Vienna, Vienna (Vienna.
Pike C. L., Ramirez I. E., Anchundia D. J., Fessl B., Heimpel G. E., Causton C. E. (2021). Behavior of the avian parasite Philornis downsi (Diptera: Muscidae) in and near host nests in the Galapagos Islands. J. Insect Behav. 34, 296–311. doi: 10.1007/s10905-021-09789-7
Post D. M. (2002). Using stable isotopes to estimate trophic position: models, methods, and assumptions. Ecology 83, 703–718. doi: 10.1890/0012-9658(2002)083[0703:USITET]2.0.CO;2
Quinby B. M., Creighton J. C., Flaherty E. A. (2020). Stable isotope ecology in insects: a review. Ecol. Entomology 45, 1231–1246. doi: 10.1111/een.12934
Ramirez I. E., Causton C. E., Gutierrez G. A., Mosquera D. A., Piedrahita P., Heimpel G. E. (2022). Specificity within bird–parasite–parasitoid food webs: A novel approach for evaluating potential biological control agents of the avian vampire fly. J. Appl. Ecol. 59, 2189–2198. doi: 10.1111/1365-2664.14235
R Core Team (2021). R: A language and environment for statistical computing (Vienna: R Foundation for Statistical Computing). Available online at: http://www.r.project.org/.
Regar M. N., Tulung B., Londok J. J. M. R., Moningkey S. A. E., Tulung Y. R. L. (2019). Blood lipid profile of broiler chicken as affected by a combination of feed restriction and different crude fiber sources. IOP Conf. Series: Earth Environ. Sci. 387, 012053.
Rentería J. L., Gardener M. R., Panetta F. D., Atkinson R., Crawley M. J. (2012). Possible impacts of the invasive plant Rubus niveus on the native vegetation of the Scalesia forest in the Galapagos Islands. PloS One 7, e48106. doi: 10.1371/journal.pone.0048106
Revelle W. R. (2022). Package ‘psych’: procedures for personality and psychological research. R Package Version 4.2.1. (Evanston, Illinois: Northwestern University).
Ricklefs R. E., Hainsworth F. R. (1968). Temperature regulation in nestling cactus wrens: The development of homeothermy. Condor 70, 121–127. doi: 10.2307/1365955
Schmidt O., Dautel H., Newton J., Gray J. S. (2011). Natural isotopic signatures of host blood are replicated in moulted ticks. Ticks Tick-borne Dis. 2, 225–227. doi: 10.1016/j.ttbdis.2011.09.006
Seppälä O., Leicht K. (2015). Quality attracts parasites: host condition-dependent chemo-orientation of trematode larvae. Funct. Ecol. 29, 791–795. doi: 10.1111/1365-2435.12392
Singh K. R. P., Brown A. W. A. (1957). Nutritional requirements of Aedes aEgypti L. J. Insect Physiol. 1, 199–220. doi: 10.1016/0022-1910(57)90036-7
Skowron C., Kern M. (1980). The insulation in nests of selected North American songbirds. Auk 4, 816–824. doi: 10.1093/auk/97.4.816
Staddon P. (2004). Carbon isotopes in functional soil ecology. Trends Ecol. Evol. 19, 148–154. doi: 10.1016/j.tree.2003.12.003
Still C. J., Powell R. L. (2010). “Continental-scale distributions of vegetation stable carbon isotope ratios,” in Isoscapes: understanding movements, pattern and process on earth through isotope mapping. Eds. West J. B., Bowen G. J., Dawson T. E., Tu K. P. (Dordrecht, Springer), 179–194. doi: 10.1007/978-90-481-3354-3_9
Stokke B. G., Ratikainen I. I., Moksnes A., Røskaft E., Schulze-Hagen K., Leech D. I., et al. (2018). Characteristics determining host suitability for a generalist parasite. Sci. Rep. 8, 6285. doi: 10.1038/s41598-018-24627-1
Tschirren B., Bischoff L. L., Saladin V., Richner H. (2007). Host condition and host immunity affect parasite fitness in a bird-ectoparasite system. Funct. Ecol. 21, 372–378. doi: 10.1111/j.1365-2435.2007.01235.x
UlHaq I., Mayr L., Teal P. E. A., Hendrichs J., Robinson A. S., Stauffer C., et al. (2010). Total body nitrogen and total body carbon as indicators of body protein and body lipids in the melon fly Bactrocera cucurbitae: Effects of methoprene, a juvenile hormone analogue, and of diet supplementation with hydrolyzed yeast. J. Insect Physiol. 56, 1807–1815. doi: 10.1016/j.jinsphys.2010.07.011
van Schoor T., Kelly E. T., Tam N., Attardo G. M. (2020). Impacts of dietary nutritional composition on larval development and adult body composition in the yellow fever mosquito (Aedes aEgypti). Insects 11, 535. doi: 10.3390/insects11080535
Walentowitz A., Manthey M., Bentet Preciado M. B., Chango R., Sevilla C., Jäger H. (2021). Limited natural regeneration of unique Scalesia forest following invasive plant removal in Galapagos. PloS One 16, e0258467. doi: 10.1371/journal.pone.0258467
Walker M. D., Rotherham I. D. (2011). Host selection by the Louse Fly Crataerina pallida, an avian nest ectoparasite of the Common Swift. Apus apus. Exp. Parasitol. 129, 48–54. doi: 10.1016/j.exppara.2011.05.016
Wickham H. (2016). Package ‘ggplot2’: elegant graphics for data analysis. R Package Version 4.2.1. (New York: Springer-Verlag).
Keywords: nest parasite, stable isotopes, amino acids, thermal conditions, host quality, Darwin finches, avian vampire fly, Galapagos Islands
Citation: Pike CL, Hood-Nowotny R, Kofler B, Richner H and Tebbich S (2024) Nest climate and blood quality of two host species of the ectoparasitic Philornis downsi in the Galapagos Islands as factors of larval development. Front. Ecol. Evol. 12:1398353. doi: 10.3389/fevo.2024.1398353
Received: 09 March 2024; Accepted: 07 August 2024;
Published: 30 August 2024.
Edited by:
Simeon Lisovski, Alfred Wegener Institute Helmholtz Centre for Polar and Marine Research (AWI), GermanyReviewed by:
Daniel Becker, University of Oklahoma, United StatesAnia A. Majewska, University of Georgia, United States
Copyright © 2024 Pike, Hood-Nowotny, Kofler, Richner and Tebbich. This is an open-access article distributed under the terms of the Creative Commons Attribution License (CC BY). The use, distribution or reproduction in other forums is permitted, provided the original author(s) and the copyright owner(s) are credited and that the original publication in this journal is cited, in accordance with accepted academic practice. No use, distribution or reproduction is permitted which does not comply with these terms.
*Correspondence: Courtney L. Pike, Q291cnRuZXkuTC5QaWtlQGdtYWlsLmNvbQ==