- 1College of Geographical Science, Harbin Normal University, Harbin, China
- 2State Key Laboratory of Black Soils Conservation and Utilization, Northeast Institute of Geography and Agroecology, Chinese Academy of Sciences, Changchun, China
- 3Heilongjiang Academy of Forestry, Harbin, China
- 4School of Geography, Geomatics and Planning, Jiangsu Normal University, Xuzhou, China
- 5Shandong Key Laboratory of Eco-Environmental Science for Yellow River Delta, Binzhou University, Binzhou, China
Introduction: Soil salinity–alkalinity has emerged as a global problem affecting many ecosystems, including grassland. Plants evolve into different ecotypes to adapt to various environments. Leymus chinensis widely distributed in the eastern Eurasian steppe, has evolved into two main ecotypes: yellow–green(YG) and gray–green (GG). Studies on the adaption mechanisms of both ecotypes in response to saline–alkaline stress are limited.
Methods: In this study, the growth and physiological traits of ecotypes YG and GG in soils with different salinity–alkalinity levels—severe saline–alkaline soil (SS), moderate saline–alkaline soil (MS), and light saline–alkaline soil (LS)—were studied. After exposure to saline–alkaline stress for 15 months, the L. chinensis ecotypes exhibited significant differences in the growth characteristics.
Results: The specific leaf area, individual tiller biomass, net photosynthetic rate, and potassium content of the two ecotypes under MS conditions were significantly higher than or similar to those under LS conditions. This indicates that L. chinensis showed a certain degree of tolerance to saline–alkaline environments under MS conditions. Saline–alkaline stress increased the tillers by 56% in GG and reduced them by 26% in YG, and did not alter the SLA and the number of individual tiller leaves of GG but reduced that of YG. Moreover, with increasing levels of saline–alkaline stress, ecotype GG exhibited an increase in net photosynthetic rate (Pn), while ecotype YG showed insignificant changes. Under SS, GG exhibited higher Pn and chlorophyll content than YG. Additionally, with the increase in the saline–alkaline stress level, the Na+ content increased, but GG exhibited a significantly lower Na+ content than YG. Conversely, the K+ and Ca2+ contents and the K+/Na+ and Ca2+/Na+ ratios decreased, but GG exhibited higher values than YG.
Discussion: GG reduced Na+ by absorption by increasing tillers. Additionally, GG absorbed more K+ and Ca2+ ions, thereby maintaining higher K+/Na+ and Ca2+/Na+ ratios than YG under saline–alkaline stress. These adaptive strategies enable ecotype GG to grow and maintain normal physiological functions under high saline–alkaline stress. The findings of this study hold practical significance for enhancing the economic and ecological value of saline–alkaline grasslands.
1 Introduction
Soil salinity–alkalinity is an important factor influencing the degradation of grassland ecosystems and has become a global environmental problem (Kemp et al., 2013; Shabala, 2013; Sun et al., 2017; Wang et al., 2020a). The diminishing soil protection capacity and excessive soil moisture evaporation relative to precipitation in degraded grasslands result in the continuous accumulation of neutral and alkaline salts in the soil (Yang et al., 2016; Gang et al., 2018; Chen et al., 2019). Soil salinity–alkalinity constrains the absorption of water and nutrients by plants, threatening their survival (Kitajima and Fenner, 2000; El-Keblawy and Al-Rawai, 2005). Grassland degradation and soil salinity–alkalinity form a detrimental cycle, severely limiting agricultural and pastoral production and development. Bioremediation measures such as the cultivation of plants tolerant to saline–alkaline conditions are commonly employed to treat saline–alkaline soil (Maggio et al., 2000; Tipirdamaz et al., 2006). Hence, understanding the adaptation of plant growth to saline–alkaline soil is crucial.
Saline–alkaline stress exerts a strong inhibitory effect on plant growth (Zhang et al., 2017). Saline–alkaline soil contains high concentrations of neutral salts and alkaline salts i.e., Na2CO3 and NaHCO3 (Doula et al., 2016). Compared with plants subjected to neutral salt stress, plants under saline–alkaline stress absorb a large number of ions through root tip contact, resulting in ion toxicity and osmotic stress; moreover, they are affected by high pH and its combination with other stressors (Wang et al., 2017). Saline–alkaline stress can significantly reduce plant height, leaf number, stem length, and aboveground biomass (AB) (Liu et al., 2015a) and increase tillering and stem biomass in plants. Moreover, different species exhibit different responses to saline–alkaline stress. Light saline–alkaline stress can promote the growth of plants tolerant to salinity–alkalinity conditions, such as Phragmites australis, Chloris virgata, and Setaria viridis; however, high saline–alkaline stress inhibits the growth of such plants (Munns et al., 1999). Additionally, some plants may evolve into different ecotypes through several strategies, including the adjustment of growth, morphology, and reproduction patterns, to thrive under diverse stresses (Hoffmann and Sgrò, 2011; Anderson et al., 2012; Sun et al., 2022a). Previous studies have focused on the effects of salt stress on grassland plants, and the research objects of saline–alkaline stress are mainly crops and cultivated land (Hu and Schmidhalter, 2023; Rao et al., 2023; Zhou et al., 2023).
The growth morphology of plants serves as a visible indicator of their response to stress. Sun et al. (2022a) divided these indicators into horizontal and vertical aspects. The horizontal aspects include plant height, while the vertical aspects include leaf density, leaves, and tillers (Zeng et al., 2013; Wang et al., 2022). El-Hendawy et al. (2005) demonstrated that different wheat and rice genotypes exhibited various responses to salinity according to three agronomic variables: tiller number, leaf number, and leaf area per plant. Salt-tolerant wheat exhibited more tillers than salt-sensitive wheat (El-Hendawy et al., 2005). In addition, Poorter et al. (2012) and Dolezal et al. (2021) revealed that plants were more likely to adjust biomass allocation than to change the morphology of tissues and organs. This adaptation serves as a survival strategy for coping with stress (Dolezal et al., 2021; Sun et al., 2022b). Cortois et al. (2016) reported that photosynthesis is a functional feature that affects plant growth and reproductive adaptability. Other studies have reported that photosynthesis reflects the characterization of plants in different environments (Lawlor, 2009; Zhou et al., 2014). Zhou et al. (2014) reported that different ecotypes exhibited different photosynthesis intensities. In another study, photosynthetic area and solar utilization efficiency were mainly affected by leaf morphology, and they exhibited high sensitivity and adaptability to changes in the external environment (Xu et al., 2009). In addition, Zhou et al. (2014) reported that leaf water use efficiency, chlorophyll content, and inorganic ions were key factors for evaluating the environmental suitability and production performance of plants. Therefore, the observation and measurement of the growth characteristics and functional characteristics of plants enable a more direct exploration of the tolerance mechanism of plants to saline–alkaline stress conditions.
Leymus chinensis is one of the main forage grasses and dominant plant species in the grasslands of eastern Eurasia (Liu et al., 2011; Zhou et al., 2014; Liu et al., 2019b). This perennial gramineous plant exhibits resistance to cold, drought, and salinity, with a broad ecological niche (Ma et al., 2015, Ma et al., 2019). L. chinensis primarily relies on root reproduction, with seeds serving as supplementary reproduction under stress conditions (Ma et al., 2021b; Sun et al., 2022a). The long-term growth of L. chinensis in various environmental conditions leads to the formation of individuals or populations of the same species with ecological differences due to adaptive divergence. Moreover, two ecotypes of this species exist: gray–green (GG) and yellow–green (YG) (Bai et al., 2012; Zhou et al., 2014; Sun et al., 2022a), which differ in morphology and physiology (Chen and Wang, 2009). The GG ecotype is distributed mainly in the soda saline–alkaline grassland of the Songnen Plain in Northeast China, while the of YG ecotype is distributed mainly in the natural grasslands of Inner Mongolia (Liu et al., 2019b; Ma et al., 2019). Some studies have focused on the differences in ecotypes, such as leaf color (Liu et al., 2019b), leaf photosynthesis (Zhou et al., 2014), and seed germination (Ma et al., 2021a); however, research on the differences and mechanisms of stress tolerance between ecotypes GG and YG is limited.
In this study, two ecotypes of L. chinensis ramets were transplanted from natural Songnen Plain grasslands to pots containing different ratios of saline–alkaline soil in 2021 and cultured for 15 months. In June 2022, L. chinensis individuals from both GG and YG ecotypes were examined. The aim of this study was to investigate (1) the growth characteristics, functional traits, and physiological traits of the GG and YG ecotypes in response to different levels of soil salinity–alkalinity and (2) the mechanisms of saline–alkaline tolerance from the aspects of the ion and photosynthesis characteristics.
2 Materials and methods
2.1 Plant and saline–alkaline soil samples
The experiment was conducted in the canopy of the Northeast Institute of Geography and Agroecology, Chinese Academy of Sciences, Changchun City, Jilin Province (125.38°E, 43.98°N, altitude 190 m). The experimental site is situated in a temperate continental monsoon climate. From 2010 to 2016, the average daily temperature ranged from 2°C to 6°C, with the highest daily temperature reaching 35°C and the lowest dropping to −20°C. The annual precipitation ranged from 350 mm to 650 mm, with 75% of the precipitation concentrated from June to August (Zhao et al., 2019; Wang et al., 2020b). The soil type was black soil, with a soil layer thickness of 0.30 m. L. chinensis individuals from both GG and YG ecotypes in the experimental nursery were selected. Light saline–alkaline (LS, pH: 7.1–8.5), moderate saline–alkaline (MS, pH: 8.5–9.5), and severe saline–alkaline (SS, pH: > 9.5) soils were mixtures of pure alkaline and non-alkaline soils at ratios of 1:4, 3:2, and 4:1, respectively, and the salinity–alkalinity intensity of the experimental soil was determined according to the pH of the mixed soil. The alkaline soil with a soil pH range of 8.5–11 and the non-alkaline soil were collected from the Songnen Plain.
2.2 Pot culture test
The experiment was conducted from May 2021 to August 2022. The two ecotypes of L. chinensis used in the experiment were all collected from the Da’an Sodic Land Experiment Station (45° 35′58″ – 45° 36′ 28″ N, 123° 50′ 27″ – 123° 51′ 31″ E), in the western part of the Songnen Plain, Northeast China. The GG and YG ecotypes utilized in the experiment were sourced from the same experimental field, ensuring uniform growth environments. In May 2021, 36 tillers of L. chinensis with similar growth potential (2 leaves, average plant height of 10 cm) were obtained from GG and YG, respectively. Each group consisted of four tillers of L. chinensis, and GG and YG were each divided into nine groups. Three groups of L. chinensis were transplanted into three pots (H:D = 20 cm:15 cm) containing the same type of saline–alkaline soil, resulting in a total of 18 pots. GG and YG ecotypes of L. chinensis in pots were cultivated under a canopy. The same amount of water was applied every 3 days to maintain soil moisture. To eliminate the impact of litter on the regreening of L. chinensis in the second year, AB was cleared in September 2021, leaving a stubble height of approximately 5 cm. By the end of July 2022, the photosynthetic efficiency and growth indices (plant height, tillers, and leaf morphology) of L. chinensis were assessed. In August 2022, the aboveground parts of L. chinensis were collected for the determination of leaf cation content, biomass, and biomass allocation. During the culture experiment, the L. chinensis ecotype and the soil used were the only two variables.
2.3 Plant growth, photosynthetic characteristics, and leaf cations
The growth characteristic indices of this experiment mainly included height, tiller number (T), leaf biomass of individual plants (LBI), stem biomass of individual plants (SB) and leaf sheath biomass of individual plants (LSB). During the vigorous growth period, tiller (T) counts were conducted in the pots, and the height of six vegetative plants was measured in each treatment. Additionally, two plant samples were collected from each pot, with each treatment comprising six tillers. From each treatment, six healthy and non-destructive vegetative stems were selected, and two plant samples were collected from each pot. These samples were immediately transported back to the laboratory, with the stems, leaves, and sheaths placed separately into envelopes. Subsequently, the six vegetative stems were dried at 75°C for 48 hours and weighed (Zhou et al., 2014).
The leaf morphological traits were measured, including leaf thickness (LT), leaf biomass (LM), and specific leaf area (SLA). Secondary leaves from six healthy and non-destructive vegetative strains at vigorous growth stage were selected to measure LT, and leaf area was determined using a leaf area meter. The leaves were placed in an envelope and dried to determine LM. The SLA was calculated as the ratio of leaf area to leaf biomass.
The photosynthetic indices included net photosynthetic rate (Pn), transpiration rate (Tr), stomatal conductance (Gs), and instantaneous water use efficiency (WUE). The physiological and biochemical indices included chlorophyll (Chl, chlorophyll a, chlorophyll b), sodium ion (Na+), potassium ion (K+), calcium ion (Ca2+), and magnesium ion (Mg2+) content. Photosynthesis measurements were conducted from 9:00 to 11:00. Six fully expanded healthy secondary leaves were selected for each treatment (Liu et al., 2019a). Pn, Tr, and Gs were measured using an open gas exchange system photosynthesis system (LI-6400, LI-COR, Lincoln, USA). WUE was calculated using the formula: (Dewar, 1997). Chlorophyll a and chlorophyll b were measured through the Arnon method (Esteban et al., 2018), with each pot containing two plant samples (Fargašová, 1996), and each treatment comprising six tillers. The contents of Na+, K+, Ca2+, and Mg2+ in leaves were determined through inductively coupled plasma-atomic emission spectrometry (ICP-AES; inductively coupled plasma optical emission spectrometry, Japan) (Cuin and Shabala, 2005). After the experiment, the aboveground parts of L. chinensis were collected, then dried at 75°C for 48 hours (Zhou et al., 2014). The AB was measured. The individual tiller biomass (IB) was determined as follows:
2.4 Soil physical and chemical properties
Highly alkaline soil and natural non-alkaline soil were mixed at ratios of 0:1, 1:1, and 4:1 (weight/weight) to obtain LS, MS, and SSs, respectively. Samples of experimental soils were collected to analyze their physical and chemical properties, with each soil type replicated three times. Soil and water were mixed at a ratio of 1:5 (weight/volume), and then pH and electrical conductivity (EC) were determined by potentiometry. Soil organic carbon (SOC) content was determined through thermal oxidation using H2SO4-K2Cr2O7 (Zhou et al., 2014). CH3COONH4 solution was added to dry soil samples, and then available potassium (AK) was determined through ICP-AES (Li et al., 1995). After the dry soil sample was digested with a catalyst and concentrated H2SO4, total nitrogen (TN) was determined using a continuous flow analytical system (SKALAR SAN++). The dry soil samples were digested using 4 mL H2SO4-1 mL HClO4, and total phosphorus (TP) was determined using the continuous flow analytical system. The NO3−-N content of fresh soil samples extracted with KCl was determined using the continuous flow analytical system. The HCO3− content of soil samples was determined through the neutralization titration method. The SO42− content of soil samples was determined through spectrophotometry. The Cl− content soil samples was determined via ion chromatography. The Na+ and Mg2+ contents of soil samples were determined through (ICP-AES, ICPS-7500).
2.5 Statistical analysis
The differences in experimental soil physical and chemical properties were calculated through one-way analysis of variance (ANOVA). The effects of ecotypes (E), saline–alkaline stress (S), and their interaction (S×E) on the traits of L. chinensis were analyzed through two-way (ANOVA). Tukey’s post-hoc tests were employed to determine the differences among the ecotypes of L. chinensis and among treatments. The “ggpair” function was utilized to generate the correlation matrix of growth characteristics, photosynthesis of YG and GG ecotype L. chinensis and leaf cation contents, soil pH and EC. All statistical analyses were conducted using R version 4.1.3.
3 Results
3.1 Soil physical and chemical properties
The physical and chemical properties of the soils are presented in Tables 1, 2. With the increase in the percentage of alkaline soil, the pH increased from 8.02 to 9.50, and EC increased from 120.9 to 612.02 μS/cm. LS exhibited higher SOC, TN, TP, and AN contents than SS and MS, while no significant difference in these parameters existed between MS and SS. SS exhibited higher Na+, Mg2+, HCO3−, SO42−, and NO3−-N contents than MS and LS, while the contents in MS were higher or not significantly different from those in LS (p > 0.05). No significant differences in Ca2+, K+, Cl−, TK, AP, AK, and NH4+-N contents existed between the different saline–alkaline soils (p > 0.05). Among the soil anions, the HCO3− content was the highest, reaching 1628.7 mg/kg in SS. The three experimental soils significantly differed in nutrient composition and soil physical and chemical properties.
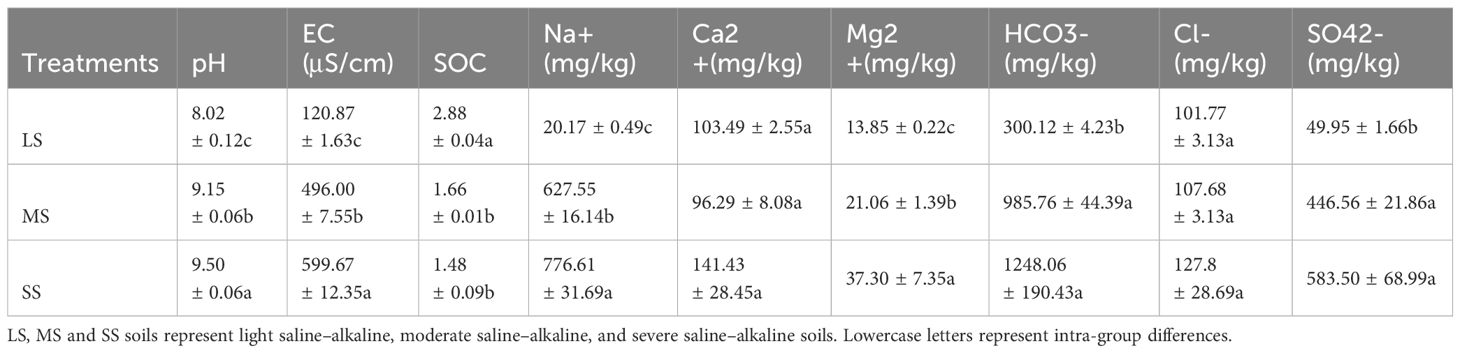
Table 1 Soil physical and chemical properties of LS, MS and SS soils before planting Leymus chinensis.
3.2 Growth characteristics of Leymus chinensis with different ecotypes under saline–alkaline stress
The different ecotypes of L. chinensis exhibited significant differences in height, tillers, LT, SLA, and IB under various saline–alkaline stress conditions (p< 0.05, Figure 1). Height was significantly affected by ecotypes (F1, 30 = 6.86, p< 0.05). Tillers were affected by the interaction between ecotypes and saline–alkaline stress (F2, 3 = 8.61, p< 0.01). LT was affected by ecotypes (F1, 30 = 8.12, p< 0.001), saline–alkaline stress (F2, 30 = 25.46, p< 0.01) and their interaction (F2, 30 = 7.59, p< 0.01). SLA was affected by ecotypes (F1, 30 = 5.03, p< 0.05) and saline–alkaline stress (F2, 30 = 4.41, p< 0.05). The two ecotypes exhibited no significant difference in AB, but the IB was affected by saline–alkaline stress (F2, 30 = 8.60, p< 0.05). Saline–alkaline stress increased the tillers by 56% in GG and reduced them by 26% in YG. The saline–alkaline stress did not alter the SLA of GG but reduced that of YG by 20.1% (Figure 1). Under SS conditions, GG exhibited larger tillers numbers, LT, and SLA than YG. Ecotypes GG and YG exhibited lower IB under SS compared with the other stress conditions, but GG showed higher IB than YG (although the difference was significant).The average individual tiller biomass of YG decreased from 3.1896 g under LS to 2.1226 g under SS. In contrast, GG exhibited 25.72% under SS compared with LS. Saline–alkaline stress reduced the leaf biomass allocation of L. chinensis. GG exhibited a higher leaf biomass allocation than YG (Figure 2). Under the different saline–alkali stress levels, GG exhibited no significant difference in the number of tiller leaves produced by a individual tiller, while YG exhibited a significant decrease in the number of individual tiller leaves with increasing stress levels (F2,30 = 5.24, p< 0.05, Figure 2).
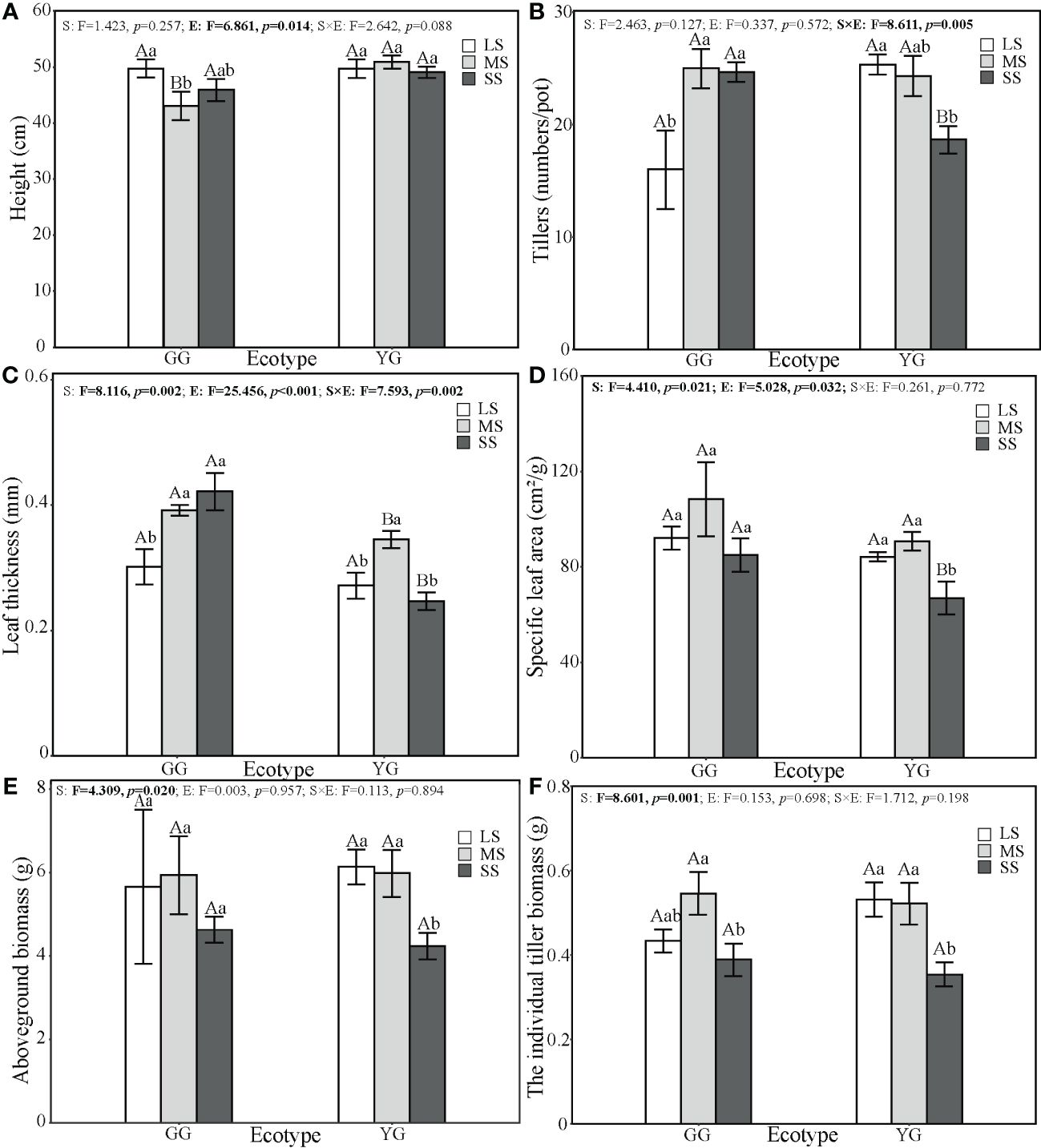
Figure 1 Different ecotypes of L. chinensis show significant differences in growth characteristics under saline–alkaline stress. The growth characteristics include the Height (A), Tillers per pot (B), Leaf thickness (C), Specific lesf area (D), Aboveground biomass (E), and the individual tiller biomass (F). The values represent mean ± se. The F value represents the ratio of between-group variability to within-group variability, and the p value indicates the significance level of this F value. Significant effects (p < 0.05) are in bold and marginally significant effects (0.05 < (p < 0.1) are in italics. Capital letters indicate the significance of differences between ecotypes. Lowercase letters indicate the significance of differences under different saline-alkaline stresses.
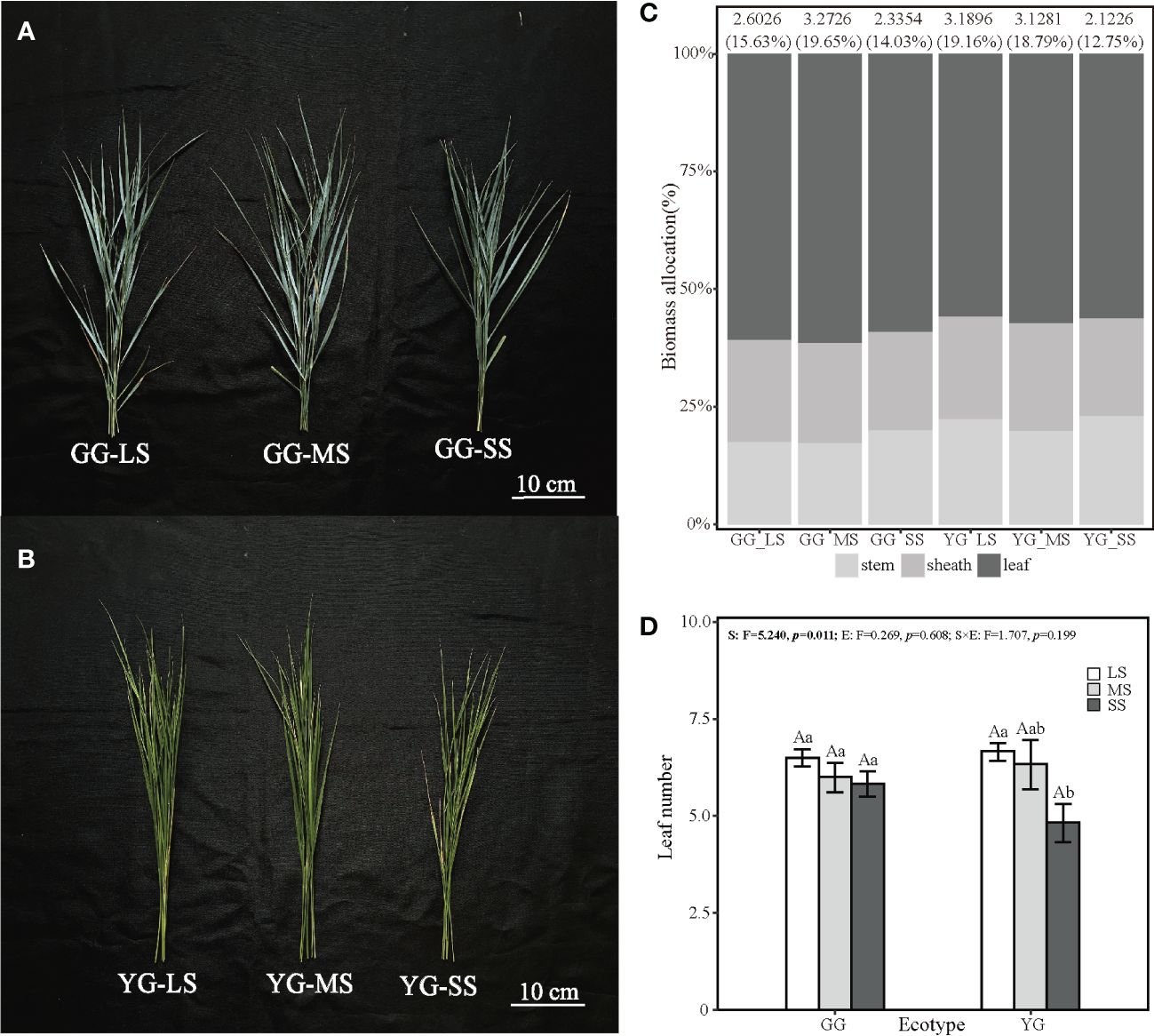
Figure 2 The changes in biomass allocation of GG and YG under saline–alkaline stress. The number and percentage on each columns represent the average biomass individual tiller and the proportion of the average biomass in the total biomass individual tiller. (A, B) represent the aboveground parts of 6 GG and YG tillers under LS, MS and SS (from lift to right). (C, D) represent biomass allocation percentage and leaf number of one tiller in Leymus chinensis under treatment.
3.3 Photosynthetic capability of different ecotypes of Leymus chinensis under saline–alkaline stress
Saline–alkaline stress exhibited different negative impacts on the photosynthetic characteristics of ecotypes GG and YG (Figure 2). The Pn and chlorophyll content of L. chinensis were affected by ecotypes (F1,46 = 3.50, p = 0.064; F1,30 = 62.63, p< 0.001), saline–alkaline stress (F2,46 = 2.91, p = 0.068; F2,3 = 6.10, p< 0.01), and their interaction (F2,46 = 2.90, p = 0.065; F2,30 = 3.63, p< 0.05), and Tr and Gs were affected by saline–alkaline stress (F2,46 = 10.95, p = 0.001; F2,46 = 18.99, p< 0.001). Saline–alkaline stress increased the Pn of GG by 50% but did not alter that of YG; reduced the Gs of GG and YG by 26% and 51%, respectively; and reduced the Tr of GG and YG by 11% and 46%, respectively. Moreover, saline–alkaline stress increased the WUE of GG and YG by 27% and 11%, respectively. Under SS conditions, GG exhibited a higher Pn than YG. Moreover, under SS conditions, GG exhibited a significantly higher leaf chlorophyll content than YG, despite YG showing a higher chlorophyll content under moderate saline–alkaline stress (p< 0.01, Figure 3).
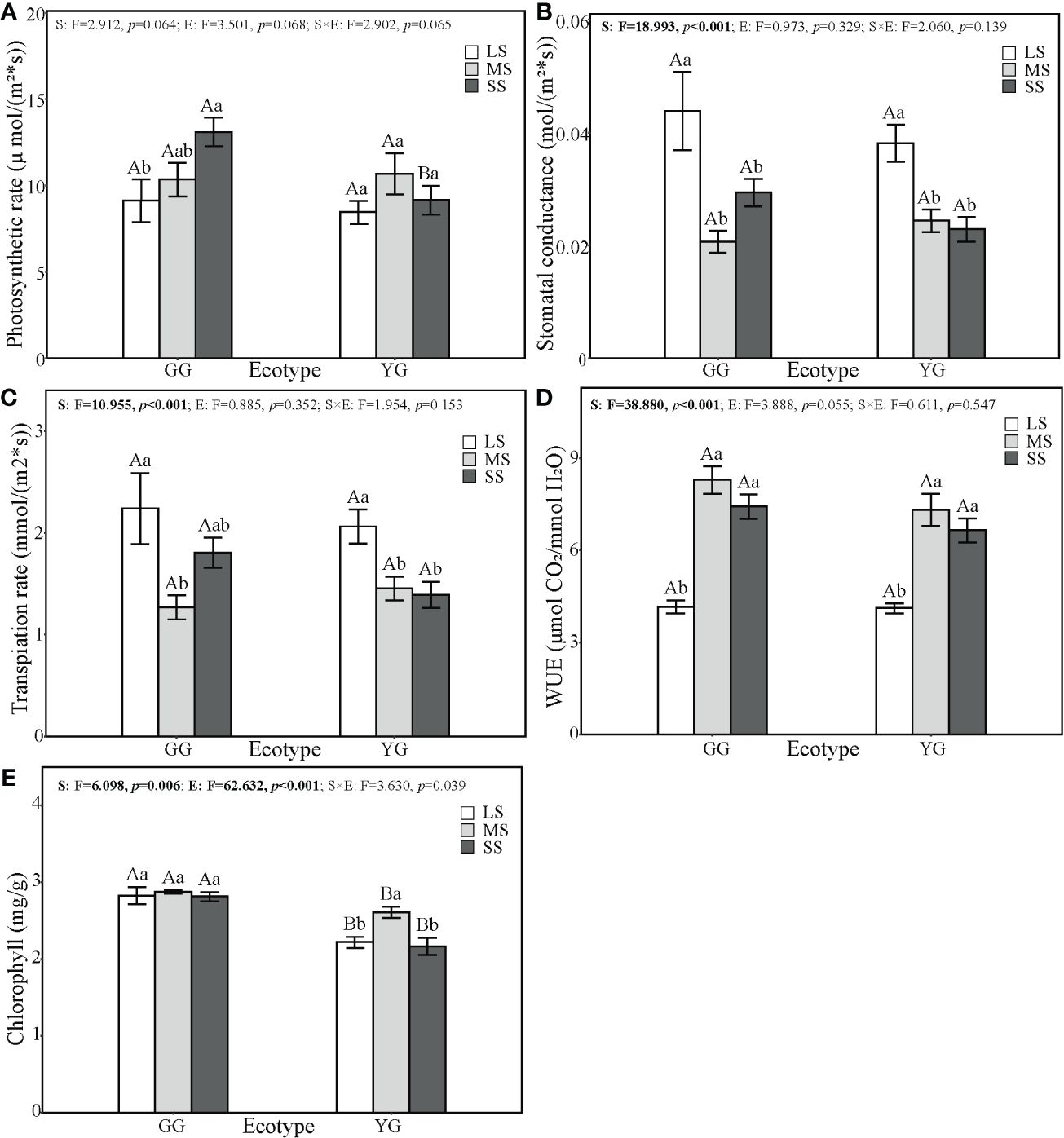
Figure 3 Effects of saline–alkaline stress on the photosynthetic characteristics of Leymus chinensis. The photosynthetic characteristics include Photosynthetic rate (A), Stomatal conductance (B), Transpiation rate (C), water use efficiency (WUE, D), and Chlorophyll (the sum of chlorophyll a and chlorophyll b, E). The values represent mean ± se. The F value represents the ratio of between-group variability to within-group variability, and the p value indicates the significance level of this F value. Significant effects (p < 0.05) are in bold and marginally significant effects (0.05 < p < 0.1) are in italics. Capital letters indicate the significance of differences between ecotypes. Lowercase letters indicate the significance of differences under different saline–alkaline stresses.
3.4 Response of leaf ions of Leymus chinensis to saline–alkaline stress
The Na+ and K+ contents of L. chinensis were significantly correlated with ecotypes (F1, 30 = 30.73, p< 0.001; F1, 30 = 11.07, p< 0.01) and saline–alkaline stress (F2, 30 = 133.04, p< 0.001; F2, 30 = 3.46, p< 0.05), and the Ca2+ content of L. chinensis was significantly correlated with saline–alkaline stress (F2, 30 = 23.05, p< 0.001, Figure 3). The ratios of K+/Na+ and Ca2+/Na+ in L. chinensis leaves were significantly influenced by ecotype (F1, 30 = 6.83, p< 0.05; F1, 30 = 7.62, p = 0.01), saline–alkaline stress (F2, 30 = 7.46, p< 0.001; F2, 30 = 8.17, p< 0.01), and their interaction (F2, 30 = 6.364, p< 0.01; F2, 30 = 7.04, p< 0.01). No significant difference in Mg2+ content existed among the treatments. Saline–alkaline stress increased the Na+ contents of GG and YG by 4352% and 472%, respectively, while the K+ content remained unchanged in GG and decreased by 15% in YG (Figure 4). Moreover, saline–alkaline stress reduced the Ca2+ content by 33% and 34% in GG and YG, respectively. The ratios of K+/Na+ and Ca2+/Na+ in GG decreased by 99% and 85%, respectively, while those in YG decreased by 99% and 88%, respectively. YG exhibited a lower leaf K+ content than GG. Moreover, GG exhibited significantly higher K+/Na+ and Ca2+/Na+ ratios than YG.
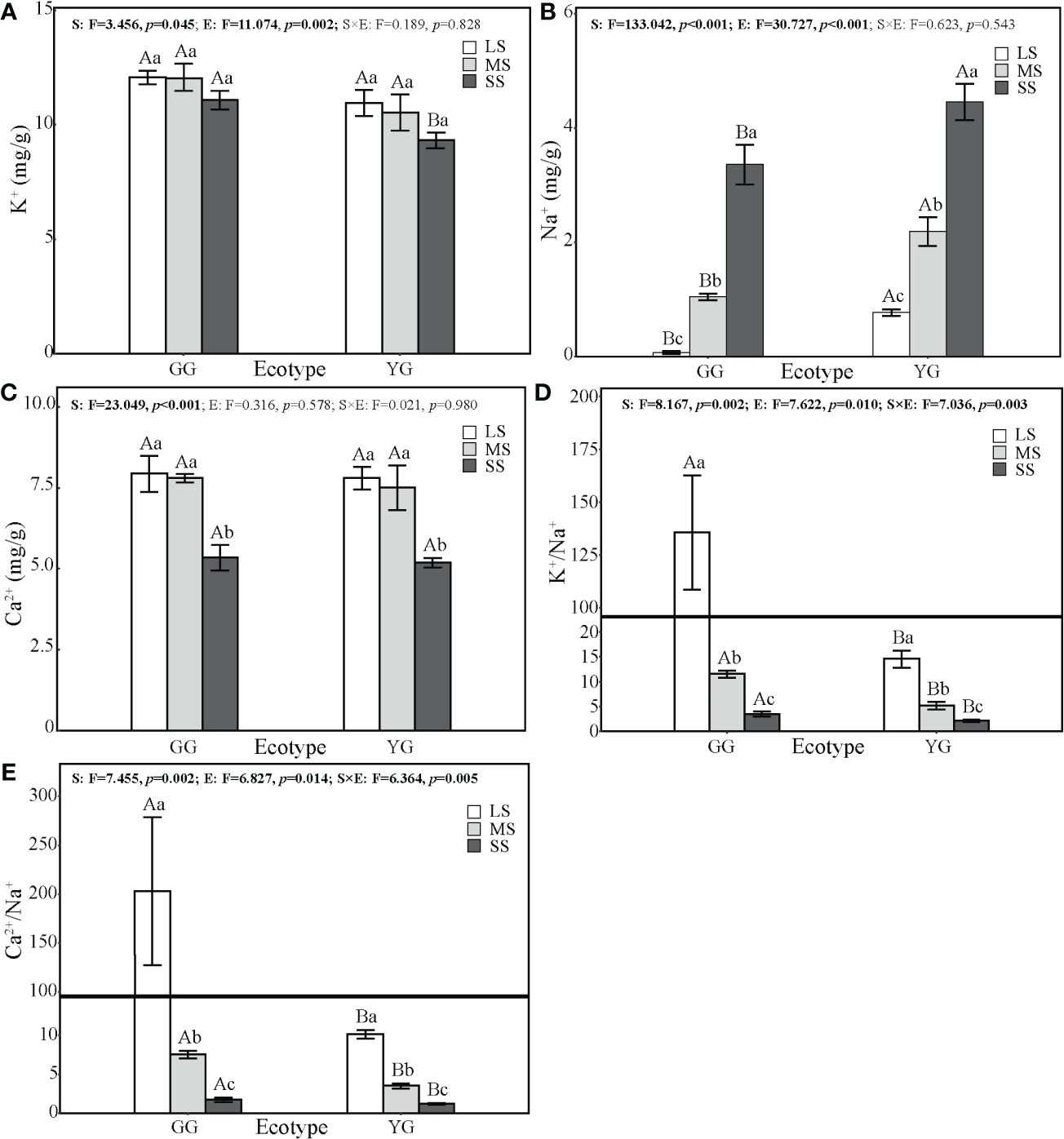
Figure 4 Effects of saline–alkaline stress on cation content in leaves of two ecotypes of Leymus chinensis. the leaf cation content including K+ cation (A), Na+ cation (B), Ca2+ cation (C), the ratio of K+/Na+ (D) and the ratio of Ca2+/Na+ (E) of leaves. The values represent mean ± se. The F value represents the ratio of between-group variability to within-group variability, and the p value indicates the significance level of this F value. Significant effects (p < 0.05) are in bold and marginally significant effects (0.05 < p < 0.1) are in italics. Capital letters indicate the significance of differences between ecotypes. Lowercase letters indicate the significance of differences under different saline–alkaline stresses.
3.5 Correlation between soil pH, EC, leaf cation contents, and growth characteristics, and photosynthesis of Leymus chinensis
With the increase in saline–alkaline stress, both ecotypes exhibited similar patterns in the leaf cation content, although the extent of increase or decrease varied. To explore the mechanisms underlying the influence of saline–alkaline stress on the growth and photosynthesis of L. chinensis, we conducted Pearson correlation analysis on the original data of soil pH, EC, leaf cation, growth characteristics, and photosynthesis. Soil pH and EC were positively correlated with Na+ content and negatively correlated with the K+ and Ca2+ contents. Soil pH, EC, and leaf cations exhibited significant effects on the growth characteristics of L. chinensis under saline–alkaline stress (Figure 5). SB exhibited a significant negative correlation with Ca2+ (p< 0.001) and K+ (p< 0.01) and a significant positively correlated with pH (p< 0.05), EC (p< 0.01), Na+ (p< 0.001). Leaf biomass (LB) showed the opposite trend compared with SB. SLA was negatively correlated with Na+ (p< 0.05) but positively correlated with K+ (p< 0.05) and Ca2+ (p< 0.05). IB was positively correlated with Ca2+ (p< 0.05). A correlation existed between plant growth characteristic indexes. Leaf biomass (LB) exhibited a significant negative correlation SB (p< 0.001), and LB and LT were positively correlated with SLA (p< 0.01). LB exhibited the opposite trend compared with SB. IB was negatively correlated with SB (p< 0.05).
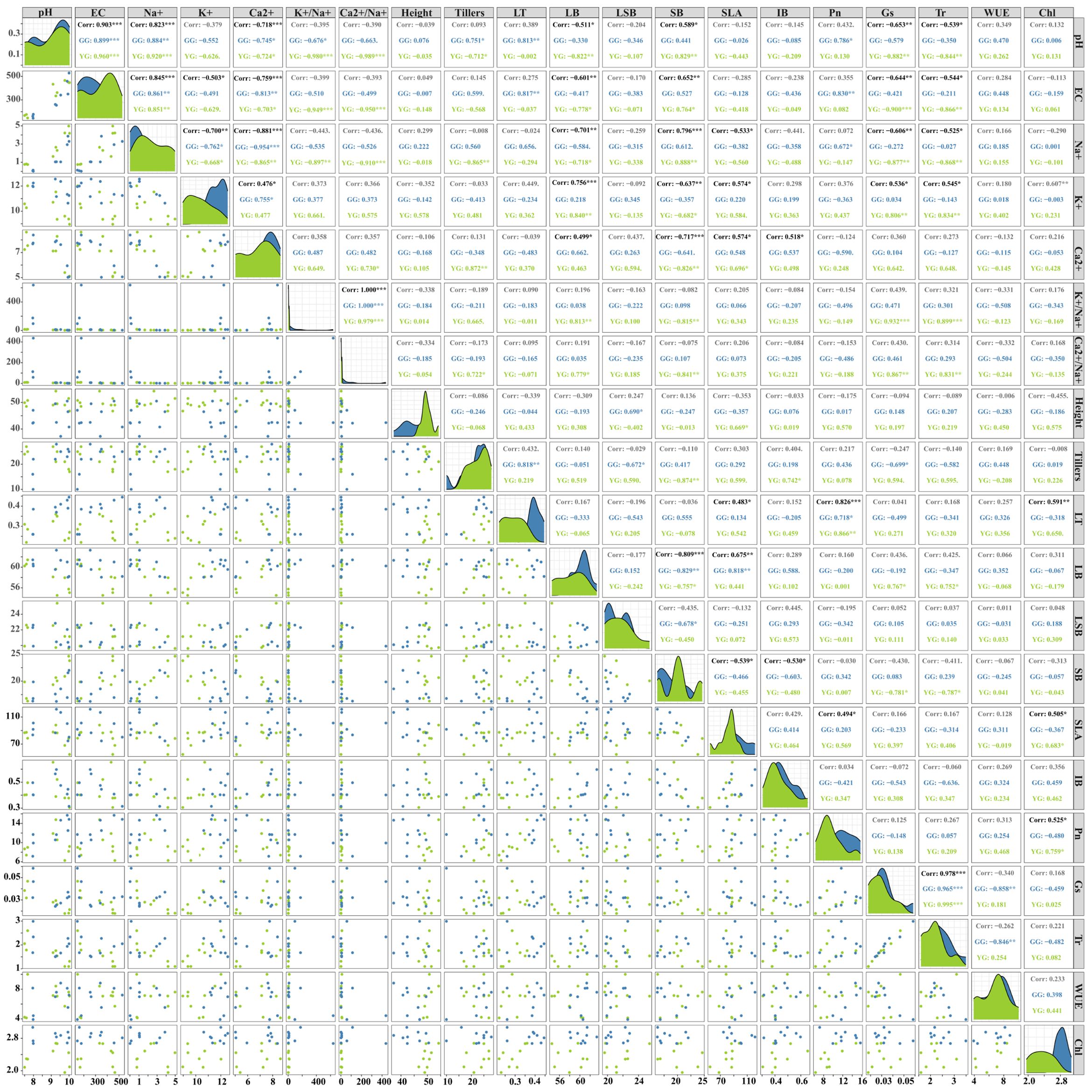
Figure 5 Pearson correlation among traits of Leymus chinensis and soil pH and EC. Morphological traits are including to K+, Na+, Ca2+, K+/Na+, Ca2+/Na+ of leaf. Growth Indicators are including to Height, Trillers, leaf thickness (LT), leaf biomass individual plant (LB), leaf sheath biomass individual plant (LSB), stem biomass individual plant (SB), specific leaf area (SLA) and IB (individual plant biomass). Physiological traits are including to photosynthesis rate (Pn), stomatal conductivity (Gs), transpiration rate (Tr) and water use efficiency (WUE), chlorophyll (Chl, sum of chlorophyll a and chlorophyll b). Green dots, regions and numbers represent YG, blue dots, regions and numbers represent GG, and black bold numbers represent two factors that are significantly correlated. The green dots, regions and numbers represent YG ecotype of Leymus chinensis, the blue dots, regions and numbers represent GG ecotype of Leymus chinensis, and the bold black numbers represent a significant correlation between the two factors. *, **, *** indicate significant correlation between two factors at 0.05, 0.01, and 0.001 level respectively.
Under saline–alkaline stress, soil pH, EC, and leaf cations exhibited significant effects on the photosynthesis of L. chinensis (Figure 5). Stomatal conductivity and transpiration rate were negatively correlated with pH, EC, and Na+ (p< 0.05) but positively correlated with K+. Photosynthesis rate was positively correlated with SLA, LT, and chlorophyll content. Chlorophyll content was positively correlated with K+. Additionally, chlorophyll content was positively correlated with SLA and LT. Na+ was negatively correlated with K+ and Ca2+, and K+/Na+ was positively correlated with Ca2+/Na+.
4 Discussion
Consistent with previous research results, different ecotypes of L. chinensis exhibited different responses under saline–alkaline stress (Zhou et al., 2014; Ma et al., 2015; Ma et al., 2021a; Sun et al., 2022b). Saline–alkaline stress increased the tillers in GG and reduced them in YG, and did not alter the SLA, the number of individual tiller leaves, and K+ content of GG but reduced that of YG. The decrease in leaf biomass proportion, individual tiller biomass, Gs, and Tr of GG caused by saline–alkaline stress was lower than that of YG. The increase in water use efficiency caused by saline–alkaline stress was higher in GG compared to YG. Ecotype GG exhibited more tillers; larger IB, LT, and SLA; enhanced photosynthesis, higher chlorophyll, K+, and Ca2+ contents; and higher K+/Na+ and Ca2+/Na+ ratios. Variations in sensitivity to saline–alkaline stress were associated with ecotype identity and the saline–alkaline concentration, consistent with findings from previous studies (Megdiche et al., 2007). The growth morphology, biomass, and photosynthetic advantages of ecotype GG indicate that it was more tolerant to saline–alkaline conditions than YG, owing to the accumulation of K+ and Ca+ in the former.
Ecotype GG demonstrated greater resilience to saline–alkaline stress than YG, as evidenced by the growth morphology. The saline–alkaline stress severely disrupted the normal growth, development, and physiological and biochemical metabolism of L. chinensis (Fang et al., 2021; Rao et al., 2023). First, sodium ions enter the cell directly through channel and carrier proteins, causing ion toxicity (Neverisky and Abbott, 2015; Fang et al., 2021). Subsequently, the high ion concentration outside the cell reduces the osmotic potential, which drives water molecules out of the cell, leading to physiological drought. Both of these aspects can cause plant metabolic disorders (Fang et al., 2021). Under saline–alkaline stress, the accumulation of oxidative metabolites in L. chinensis can inhibit normal growth and seed germination (Ma et al., 2021b; Yan et al., 2023). Moreover, saline–alkaline stress can alter apoplastic pH and intracellular pH environment (Geilfus, 2017; Rao et al., 2023), thereby affecting photosynthetic efficiency (Ye et al., 2019). Fitness of clonal plants can be determined by tiller production (Pilson, 2000). In terms of gene expression, Jin et al. (2006) identified a higher number of putative abiotic stress-related genes from the leaf cDNA library of Leymus chinensis grown in alkaline soil compared to the root cDNA library. Sun et al. (2022b) studied the correlation between gene expression flexibility and physiological performance of L. chinensis under salt–alkaline stress. The DEGs and activated or silenced genes identified from each of the pairwise transcriptomic comparisons were found to enrich in highly condition-specific functional KEGG pathways of secondary metabolites and GO terms that are known to be involved in responses to the respective environmental stresses (Fang et al., 2021; Sun et al., 2022b). The KEGG pathways in L. chinensis under salt–alkaline stress showed enrichment of upregulated and downregulated DEGs in the leaves. These KEGG pathways are mainly involved in the biosynthesis and/or metabolism of secondary metabolites related to plant growth and stress response. For example, pyruvate metabolism, which produces soluble sugars in plants grown in saline–alkaline soil, showed the highest and most concentrated soluble sugar content. Additionally, the findings of (Sun et al. 2022b) indicate that although roots are directly in contact with the soil, gene expression in both above-ground and root tissues is greatly influenced by soil type, including the DEGs and their functional relevance. Soluble sugars, such as glucose, sucrose, and fructose, are closely associated with plant tolerance to abiotic stress (Rathinasabapathi, 2000). These metabolites are essential for the normal growth and photosynthesis of L. chinensis (Liu et al., 2015b; Ma et al., 2021a). Under salt–alkaline stress, leaf ions directly or indirectly participate in the process of photosynthesis, thereby affecting the production of soluble sugars and the normal growth of L. chinensis (Fang et al., 2021; Sun et al., 2022b). In our study, the T, LT, SLA and IB values of L. chinensis increased or decreased with increasing saline–alkaline stress. Ecotype GG exhibited different patterns from YG. YG exhibited lower T, LT, and SLA in SS compared with the other soils. GG exhibited higher T and LT in MS and SS than LS, with no significant difference in SLA among the soils. Ecotype GG demonstrated a more robust resistance breeding strategy compared with YG. The larger LT indicates that ecotype GG leaves could preserve more water. SLA is an important indicator of plant stress tolerance (Kumar et al., 2012; Zhou et al., 2023). These findings demonstrate that ecotype GG exhibited greater resistance to saline–alkaline stress than ecotype YG. The results are attributable to the reduced generation and accumulation of reactive oxygen species and the increased production of antioxidant enzymes and antioxidants in ecotype GG, which mitigated the impact of saline–alkaline stress on the morphology of GG. Moreover, the increased tillers of ecotype GG possibly alleviated the adverse effects of saline–alkaline stress on its aboveground parts.
Soil pH and EC elucidate the physiological regulatory strategies of ecotype GG in adapting to highly saline–alkaline environments through the leaf ions content. To mitigate the stress imposed by saline–alkaline soils on individual plant organs (root), the roots gradually transmit stress information to the aboveground parts (Anil et al., 2005; Krishnamurthy et al., 2009). Leaf ion content reflects the ability of plants to regulate the absorption of soil ions (Fang et al., 2021; Wang et al., 2022). The leaf Na+ content can serve as an indicator of the plant’s capacity to mitigate ion stress in saline–alkaline stress environments (An et al., 2017; Zhang et al., 2017). Additionally, the K+ concentration is closely related to the regulation of osmosis, the membrane potential, and enzyme activity in plants (Falhof et al., 2016; Hasanuzzaman et al., 2018). Appropriate Ca2+ content is beneficial for maintaining K+ homeostasis under saline–alkaline stress. Moreover, supplementing Ca2+ can counteract plasma membrane depolarization induced by salt stress, thereby enhancing K+ absorption (Bacha et al., 2015). High K+/Na+ and Ca2+/Na+ ratios are necessary for the normal growth and development of plants under saline–alkaline stress (Fang et al., 2021), consistent with our findings. In our study, Na+ accumulation increased in L. chinensis, while the K+/Na+ and Ca2+/Na+ ratios decreased under saline–alkaline stress. This indicates that L. chinensis was affected by saline–alkaline stress. The Ca2+ and K+ contents maintained a certain concentration in LS and MSs, which proves that L. chinensis exhibited a certain degree of saline–alkaline tolerance. However, ecotypes GG and YG differed in tolerance ability. GG showed a lower Na+ content than YG, and GG exhibited higher the K+/Na+ and Ca2+/Na+ ratios than YG. The K+ content of GG under SS conditions was comparable to those under LS and MS conditions but higher than YG. The stronger saline–alkaline tolerance of ecotype GG compared with that of YG is attributable to the ability of GG to maintain stable K+ and Ca2+ contents in cells and reduce Na+ absorption, thereby maintaining higher K+/Na+ and Ca2+/Na+ ratios.
Saline–alkaline stress negatively impacts the chloroplast structure, leading to a decrease in chlorophyll content and affecting photosynthetic efficiency (Ye et al., 2019; An et al., 2021). Moreover, studies have reported that salt stress led to stomatal closure, reduced intercellular carbon dioxide concentration, and thereby reduced photosynthesis (Zhou et al., 2014; Wang et al., 2017). Photosynthesis primarily occurs in plant leaves, and thus, leaf morphology influences plant photosynthesis (Lawlor, 2009; Cortois et al., 2016). K+ ions play a direct role in the formation and stability of chloroplasts by influencing leaf expansion and stomatal opening and closing. They also regulate the activity of photosynthetic enzymes, ultimately affecting photosynthesis either directly or indirectly (Falhof et al., 2016; Hasanuzzaman et al., 2018). By regulating the activity of enzymes in chloroplasts and participating in the synthesis of photosynthetic pigments, Ca2+ ions affect photosynthesis and the synthesis of photosynthetic products (Bacha et al., 2015). Therefore, plants can maintain high potassium and calcium contents under stress to ensure normal photosynthetic function. Furthermore, SLA and LT exhibited a positive correlation with photosynthetic rate under saline–alkaline stress. Moderate and severe saline–alkaline stress significantly reduced the transpiration rate and stomatal conductance of L. chinensis, indicating its susceptibility to saline–alkaline stress. However, this reduction did not impair the photosynthetic functions of ecotypes GG and YG, substantiating that L. chinensis possesses a certain tolerance to saline–alkaline conditions. Moreover, under severe saline–alkaline stress, the photosynthetic rate of ecotype GG increased, while that of YG decreased. Therefore, the superior photosynthetic performance of GG compared with YG under saline–alkaline stress is due to the ability of GG to regulate the absorption of higher levels of K+ and Ca2+, thereby mitigating ion toxicity and maintaining elevated chlorophyll levels. This interpretation is supported by our correlation analysis findings.
We investigated the adaptation mechanism of the aboveground parts of different ecotypes of L. chinensis under saline–alkaline stress. Ecotype GG exhibited lower Na+ absorption, higher K+ and Ca2+ absorption, and higher K+/Na+ and Ca2+/Na+ ratios than ecotype YG under saline–alkaline stress. Future research can explore rhizome characteristics and metabolic processes to further elucidate the differences in clonal integration strategies among different ecotypes of L. chinensis under saline–alkaline stress.
Data availability statement
The original contributions presented in the study are included in the article/supplementary material. Further inquiries can be directed to the corresponding authors.
Author contributions
Y-XL: Conceptualization, Data curation, Formal analysis, Writing – original draft, Writing – review & editing. H-YM: Conceptualization, Funding acquisition, Methodology, Writing – review & editing. H-WN: Conceptualization, Writing – review & editing. S-YL: Data curation, Investigation, Writing – review & editing. XL: Writing – review & editing. M-DS: Writing – review & editing, Investigation. W-WQ: Writing – review & editing. D-DZ: Writing – review & editing.
Funding
The authors declare financial support was received for the research, authorship, and/or publication of this article. This research was supported by the National Key Research and Development Program (2022YFF1300601), the Strategic Priority Research Program of the Chinese Academy of Sciences (XDA28110300), the Provincial Natural Science Foundation of Jilin (20220101280JC; 20220203023SF), the Provincial Natural Science Foundation of Shandong (ZR2022QD106), the Joint Found of the National Natural Science Foundation of China (U23A2004) and Innovation Team Project of Northeast Institute of Geography and Agroecology, Chinese Academy of Sciences (2023CXTD02).
Acknowledgments
The authors thank the reviewers and editor for their insightful comments and constructive suggestions.
Conflict of interest
The authors declare that the research was conducted in the absence of any commercial or financial relationships that could be construed as a potential conflict of interest.
Publisher’s note
All claims expressed in this article are solely those of the authors and do not necessarily represent those of their affiliated organizations, or those of the publisher, the editors and the reviewers. Any product that may be evaluated in this article, or claim that may be made by its manufacturer, is not guaranteed or endorsed by the publisher.
References
An D., Chen J. G., Gao Y. Q., Li X., Chao Z. F., Chen Z. R., et al. (2017). Athkt1 drives adaptation of arabidopsis thaliana to salinity by reducing floral sodium content. PLoS Genet. 13, e1007086. doi: 10.1371/journal.pgen.1007086
An Y., Gao Y., Tong S. Z., Liu B. (2021). Morphological and physiological traits related to the response and adaption of bolboschoenus planiculmis seedlings grown under salt-alkaline stress conditions. Front. Plant Sci. 12. doi: 10.3389/fpls.2021.567782
Anderson J. T., Panetta A. M., Mitchell-Olds T. (2012). Evolutionary and ecological responses to anthropogenic climate change: Update on anthropogenic climate change. Plant Physiol. 160, 1728–1740. doi: 10.1104/pp.112.206219
Anil V. S., Krishnamurthy P., Kuruvilla S., Sucharitha K., Thomas G., Mathew M. K. (2005). Regulation of the uptake and distribution of na+ in shoots of rice (oryza sativa) variety pokkali: Role of ca2+ in salt tolerance response. Physiologia plantarum. 124, 451–464. doi: 10.1111/j.1399-3054.2005.00529.x
Bacha H., Ródenas R., López-Gómez E., García-Legaz M. F., Nieves-Cordones M., Rivero R. M., et al. (2015). High ca2+ reverts the repression of high-affinity k+ uptake produced by na+ in solanum lycopersycum l. (var. Microtom) plants. J. Plant Physiol. 180, 72–79. doi: 10.1016/j.jplph.2015.03.014
Bai D., LI J. D., Yang Y. F., Zhang Z., Zhou C. (2012). Analysis of sexual reproduction of leymus chinensis with different leaf colors under heterogeneous habitat. Acta Agrestia Sinica. 20, 213–220. doi: 10.11733/j.issn.1007-0435.2012.02.004
Chen L., Wang R. Z. (2009). Anatomical and physiological divergences and compensatory effects in two leymus chinensis (poaceae) ecotypes in northeast China. Agriculture Ecosyst. Environment. 134, 46–52. doi: 10.1016/j.agee.2009.05.015
Chen T., Bao A. M., Jiapaer G., Guo H., Zheng G. X., Jiang L. L., et al. (2019). Disentangling the relative impacts of climate change and human activities on arid and semiarid grasslands in central asia during 1982-2015. Sci. Total Environment. 653, 1311–1325. doi: 10.1016/j.scitotenv.2018.11.058
Cortois R., Schröder-Georgi T., Weigelt A., Putten W. H., De Deyn G. B. (2016). ). Plant–soil feedbacks: Role of plant functional group and plant traits. J. Ecol. 104, 1608–1617. doi: 10.1111/1365-2745.12643
Cuin T. A., Shabala S. (2005). Exogenously supplied compatible solutes rapidly ameliorate nacl induced potassium efflux from barley roots. Plant Cell Physiol. 46, 1924–1933. doi: 10.1093/pcp/pci205
Dewar R. (1997). A simple model of light and water use evaluated for pinus radiata. Tree Physiol. 17, 259–265. doi: 10.1093/treephys/17.4.259
Dolezal J., Jandova V., Macek M., Liancourt P. (2021). Contrasting biomass allocation responses across ontogeny and stress gradients reveal plant adaptations to drought and cold. Funct. Ecology. 35, 32–42. doi: 10.1111/1365-2435.13687
Doula M. K., Sarris A., Poulopoulos S. G., Inglezakis V. J. (Eds.) (2016). “Chapter 4 - soil environment,” in Environment and development (Elsevier, Amsterdam). doi: 10.1016/B978-0-444-62733-9.00004-6
El-Hendawy S. E., Hu Y., Yakout G. M., Awad A. M., Hafiz S. E., Schmidhalter U. (2005). Evaluating salt tolerance of wheat genotypes using multiple parameters. Eur. J. Agron. 22, 243–253. doi: 10.1016/j.eja.2004.03.002
El-Keblawy A., Al-Rawai A. (2005). Effects of salinity, temperature and light on germination of invasive prosopis juliflora (sw.) dc. J. Arid Environments. 61, 555–565. doi: 10.1016/j.jaridenv.2004.10.007
Esteban R., García-Plazaola J. I., Hernández A., Fernández-Marín B. (2018). On the recalcitrant use of arnon's method for chlorophyll determination. New Phytologist. 217, 474–476. doi: 10.1111/nph.14932
Falhof J., Pedersen J. T., Fuglsang A. T., Palmgren M. (2016). Plasma membrane h(+)-atpase regulation in the center of plant physiology. Mol. Plant 9, 323–337. doi: 10.1016/j.molp.2015.11.002
Fang S. M., Hou X., Liang X. L. (2021). Response mechanisms ofplants under saline-alkali stress. Front. Plant Science. 12. doi: 10.3389/fpls.2021.667458
Fargašová A. (1996). Inhibitive effect of organotin compounds on the chlorophyll content of the green freshwater alga scenedesmus quadricauda. Bull. Environ. Contamination Toxicology. 57, 99–106. doi: 10.1007/s001289900161
Gang C. C., Zhao W., Zhao T., Zhang Y., Gao X. R., Wen Z. M. (2018). The impacts of land conversion and management measures on the grassland net primary productivity over the loess plateau, northern China. Sci. Total Environment. 645, 827–836. doi: 10.1016/j.scitotenv.2018.07.161
Geilfus C. M. (2017). The ph of the apoplast: Dynamic factor with functional impact under stress. Mol. Plant 10, 1371–1386. doi: 10.1016/j.molp.2017.09.018
Hasanuzzaman M., Bhuyan M. H. M., Nahar K., Hossain M. S., Mahmud J. A., Hossen M., et al. (2018). Potassium: a vital regulator of plant responses and tolerance to abiotic stresses. Agronomy 8, 31. doi: 10.3390/agronomy8030031
Hoffmann A. A., Sgrò C. M. (2011). Climate change and evolutionary adaptation. Nature. 470, 479–485. doi: 10.1038/nature09670
Hu Y. C., Schmidhalter U. (2023). Opportunity and challenges of phenotyping plant salt tolerance. Trends Plant Science. 28, 552–566. doi: 10.1016/j.tplants.2022.12.010
Jin H., Plaha P., Park J. Y., Hong C. P., Lee I. S., Yang Z. H., et al. (2006). Comparative est profiles of leaf and root of leymus chinensis, a xerophilous grass adapted to high ph sodic soil. Plant Science. 170, 1081–1086. doi: 10.1016/j.plantsci.2006.01.002
Kemp D. R., Han G. D., Hou X. Y., Michalk D. L., Hou F. J., Wu J. P., et al. (2013). Innovative grassland management systems for environmental and livelihood benefits. Proc. Natl. Acad. Sci. United States America. 110, 8369–8374. doi: 10.1073/pnas.1208063110
Kitajima K., Fenner M. (2000). “Ecology of seedling regeneration,” in Seeds: The ecology of regeneration in plant communities (CABI Publishing, UK). doi: 10.1079/9780851994321.0331
Krishnamurthy P., Ranathunge K., Franke R., Prakash H. S., Schreiber L., Mathew M. K. (2009). The role of root apoplastic transport barriers in salt tolerance of rice (oryza sativa l.). Planta. 230, 119–134. doi: 10.1007/s00425-009-0930-6
Kumar U., Singh P., Boote K. J. (2012). “Effect of climate change factors on processes of crop growth and development and yield of groundnut (arachis hypogaea l.),” in Advances in agronomy (Elsevier). doi: 10.1016/B978-0-12-394277-7.00002-6
Lawlor D. W. (2009). Musings about the effects of environment on photosynthesis. Ann. Botany. 103, 543–549. doi: 10.1093/aob/mcn256
Li X., Coles B. J., Ramsey MH and Thornton I. (1995). Sequential extraction of soils for multielement analysis by icp-aes. Chem. Geology. 124, 109–123. doi: 10.1016/0009-2541(95)00029-L
Liu S. H., Kang Y. H., Wan S. Q., Wang Z. C., Liang Z. W., Sun X. J. (2011). Water and salt regulation and its effects on leymus chinensis growth under drip irrigation in saline-sodic soils of the songnen plain. Agric. Water Management. 98, 1469–1476. doi: 10.1016/j.agwat.2011.04.016
Liu B., Kang C., Wang X and Bao G. (2015b). Physiological and morphological responses of leymus chinensis to saline-alkali stress. Grassland Science. 61, 217–226. doi: 10.1111/grs.12099
Liu G., Li X., Zhang Q. (2019b). Sheepgrass (leymus chinensis): An environmentally friendly native grass for animals (Singapore: Springer Singapore). doi: 10.1007/978-981-13-8633-6
Liu Z. P., Liu G. S., Li X. X., Zhang Q. F. (Eds.) (2019a). “Distribution and germplasm phenotypic diversity of sheepgrass (leymus chinensis),” in Sheepgrass (leymus chinensis): An environmentally friendly native grass for animals (Springer Singapore, Singapore). doi: 10.1007/978-981-13-8633-6_2
Liu L. P., Long X. H., Shao H. B., Liu Z. P., Tao Y., Zhou Q. S., et al. (2015a). Ameliorants improve saline–alkaline soils on a large scale in northern Jiangsu province, China. Ecol. Eng. 81, 328–334. doi: 10.1016/j.ecoleng.2015.04.032
Ma H. Y., Liu G. S., Li X. X., Zhang Q. F. (Eds.) (2019). “Saline–alkaline resistance: Physiological and ecological characteristics of sheepgrass (leymus chinensis),” in Sheepgrass (leymus chinensis): An environmentally friendly native grass for animals (Springer Singapore, Singapore). doi: 10.1007/978-981-13-8633-6_6
Ma M. Y., Ma H. Y., Wang L., Qi W. W., Li S. Y., Zhao D. D. (2021a). Differences in the seed germination of leymus chinensis (poaceae) ecotypes reveal sistinct strategies for coping with salinity stress: A common garden experiment. Front. Ecol. Evol. 9. doi: 10.3389/fevo.2021.703287
Ma M.-Y., Ma H.-Y., Wang L., Qi W.-W., Li S-Y and Zhao D.-D. (2021b). Differences in the seed germination of leymus chinensis (poaceae) ecotypes reveal distinct strategies for coping with salinity stress: A common garden experiment. Front. Ecol. Evol. 9. doi: 10.3389/fevo.2021.703287
Ma H. Y., Yang H. Y., Lü X. T., Pan Y. P., Wu H. T., Liang Z. W., et al. (2015). Does high ph give a reliable assessment of the effect of alkaline soil on seed germination? A case study with leymus chinensis (poaceae). Plant Soil. 394, 35–43. doi: 10.1007/s11104-015-2487-4
Maggio A., Reddy M. P., Joly R. J. (2000). Leaf gas exchange and solute accumulation in the halophyte salvadora persica grown at moderate salinity. Environamental And Exp. Botany. 44, 31–38. doi: 10.1016/S0098-8472(00)00051-4
Megdiche W., Amor N. B., Debez A., Hessini K., Ksouri R., Zuily-Fodil Y., et al. (2007). Salt tolerance of the annual halophyte cakile maritima as affected by the provenance and the developmental stage. Acta Physiologiae Plantarum. 29, 375–384. doi: 10.1007/s11738-007-0047-0
Munns R., Cramer G. R., Ball M. C., Luo Y. Q., Mooney H. A. (Eds.) (1999). “5 - interactions between rising co2, soil salinity, and plant growth,” in Carbon dioxide and environmental stress (Academic Press, San Diego). doi: 10.1016/B978-012460370-7/50006-1
Neverisky D. L., Abbott G. W. (2015). Ion channel-transporter interactions. Crit. Rev. Biochem. Mol. Biol. 51, 257–267. doi: 10.3109/10409238.2016.1172553
Pilson D. (2000). The evolution of plant response to herbivory: Simultaneously considering resistance and tolerance in brassica rapa. Evolutionary Ecol. 14, 4–6. doi: 10.1023/A:1010953714344
Poorter H., Niklas K. J., Reich P. B., Oleksyn J., Poot P., Mommer L. (2012). Biomass allocation to leaves, stems and roots: Meta-analyses of interspecific variation and environmental control. New Phytologist. 193, 30–50. doi: 10.1111/j.1469-8137.2011.03952.x
Rao Y., Peng T., Xue S. W. (2023). Mechanisms of plant saline-alkaline tolerance. J. Plant Physiol. 281, 153916. doi: 10.1016/j.jplph.2023.153916
Rathinasabapathi B. (2000). Metabolic engineering for stress tolerance: Installing osmoprotectant synthesis pathways. Ann. Botany. 86, 709–716. doi: 10.1006/anbo.2000.1254
Shabala S. (2013). Learning from halophytes: Physiological basis and strategies to improve abiotic stress tolerance in crops. Ann. Botany. 112, 1209–1221. doi: 10.1093/aob/mct205
Sun H. J., Lu H. Y., Chu L., Shao H. B., Shi W. M. (2017). Biochar applied with appropriate rates can reduce n leaching, keep n retention and not increase nh3 volatilization in a coastal saline soil. Sci. Total Environment. 575, 820–825. doi: 10.1016/j.scitotenv.2016.09.137
Sun Y. F., Wang Y. P., Yan Z. B., He L. S., Ma S. H., Feng Y. H., et al. (2022a). Above- and belowground biomass allocation and its regulation by plant density in six common grassland species in China. J. Plant Res. 135, 41–53. doi: 10.1007/s10265-021-01353-w
Sun Y., Zhang X., Wang S. N., Wu X. F., Wang Y. K., Wang X. F., et al. (2022b). Phenotypic plasticity couples with transcriptomic flexibility in leymus chinensis under diverse edaphic conditions. Environ. Exp. Botany. 197, 104838. doi: 10.1016/j.envexpbot.2022.104838
Tipirdamaz R., Gagneul D., Duhazé C., Aïnouche A., Monnier C., Özkum D., et al. (2006). Clustering of halophytes from an inland salt marsh in Turkey according to their ability to accumulate sodium and nitrogenous osmolytes. Environ. Exp. Botany. 57, 139–153. doi: 10.1016/j.envexpbot.2005.05.007
Wang X. S., Ren H. L., Wei Z. W., Wang Y. W., Ren W. B. (2017). Effects of neutral salt and alkali on ion distributions in the roots, shoots, and leaves of two alfalfa cultivars with differing degrees of salt tolerance. J. Integr. Agriculture. 16, 1800–1807. doi: 10.1016/S2095-3119(16)61522-8
Wang Y. J., Shen X. J., Jiang M., Lu X. G. (2020b). Vegetation change and its response to climate change between 2000 and 2016 in marshes of the songnen plain, northeast China. Sustainability. 12, 3569. doi: 10.3390/su12093569
Wang D. L., Wang L., Xin X. P., Li L. H., Tang H. J. (2020a). Systematic restoration for degraded grasslands: Concept, mechanisms and approaches. Scientia Agricultura Sinica. 53, 2532–2540. doi: 10.3864/j.issn.0578-1752.2020.13.002
Wang R., Yang J., Liu H., Sardans J., Zhang Y., Wang X., et al. (2022). Nitrogen enrichment buffers phosphorus limitation by mobilizing mineral-bound soil phosphorus in grasslands. Ecology. 103, e3616. doi: 10.1002/ecy.3616
Xu F., Guo W. H., Xu W. H., Wei Y. H., Wang R. Q. (2009). Leaf morphology correlates with water and light availability: What consequences for simple and compound leaves? Prog. Natural Science. 19, 1789–1798. doi: 10.1016/j.pnsc.2009.10.001
Yan G., Shi Y. J., Mu C. S., Wang J. F. (2023). Differences in organic solute and metabolites of leymus chinensis in response to different intensities of salt and alkali stress. Plants. 12, 1916. doi: 10.3390/plants12091916
Yang Y., Wang Z. Q., Li J. L., Gang C. C., Zhang Y. Z., Zhang Y., et al. (2016). Comparative assessment of grassland degradation dynamics in response to climate variation and human activities in China, Mongolia, Pakistan and Uzbekistan from 2000 to 2013. J. Arid Environments. 135, 164–172. doi: 10.1016/j.jaridenv.2016.09.004
Ye L., Zhao X., Bao E. C., Cao K., Zou Z. R. (2019). Effects of arbuscular mycorrhizal fungi on watermelon growth, elemental uptake, antioxidant, and photosystem ii activities and stress-response gene expressions under salinity-alkalinity stresses. Front. Plant Sci. 10. doi: 10.3389/fpls.2019.00863
Zeng F. J., Song C., Guo H. F., Liu B., Luo W. C., Gui D. W., et al. (2013). Responses of root growth of alhagi sparsifolia shap. (fabaceae) to different simulated groundwater depths in the southern fringe of the taklimakan desert, China. J. Arid Land. 5, 220–232. doi: 10.1007/s40333-013-0154-2
Zhang H., Liu X. L., Zhang R. X., Yuan H. Y., Wang M. M., Yang H. Y., et al. (2017). Root damage under alkaline stress is associated with reactive oxygen species accumulation in rice (oryza sativa l.). Front. Plant Sci. 8. doi: 10.3389/fpls.2017.01580
Zhao D. D., Ma H. Y., Wei J. P., Li Y., Wang Z. C. (2019). Effects of water and nutrient additions on functional traits and aboveground biomass of leymus chinensis. Chin. J. Plant Ecol. 43, 501–511. doi: 10.17521/cjpe.2019.0041
Zhou H. P., Shi H. F., Yang Y. Q., Feng X. X., Chen X., Xiao F., et al. (2023). Insights into plant salt stress signaling and tolerance. J. Genet. Genomics 51, 16–34. doi: 10.1016/j.jgg.2023.08.007
Keywords: ecotypes, Leymus chinensis, salinity–alkalinity, adaptation mechanisms, leaf cations, photosynthesis
Citation: Li Y-X, Ma H-Y, Ni H-W, Li S-Y, Xu L, Sun M-D, Qi W-W and Zhao D-D (2024) Adaptation responses of different ecotypes of Leymus chinensis to saline–alkaline stress. Front. Ecol. Evol. 12:1361124. doi: 10.3389/fevo.2024.1361124
Received: 25 December 2023; Accepted: 12 April 2024;
Published: 24 April 2024.
Edited by:
Wen-Chao Liu, Guangdong Ocean University, ChinaReviewed by:
Ren Wei Bo, Chinese Academy of Agricultural Sciences, ChinaJianyong Wang, Northeast Normal University, China
Guowen Cui, Northeast Agricultural University, China
Martin Šimon, University of Ljubljana, Slovenia
Copyright © 2024 Li, Ma, Ni, Li, Xu, Sun, Qi and Zhao. This is an open-access article distributed under the terms of the Creative Commons Attribution License (CC BY). The use, distribution or reproduction in other forums is permitted, provided the original author(s) and the copyright owner(s) are credited and that the original publication in this journal is cited, in accordance with accepted academic practice. No use, distribution or reproduction is permitted which does not comply with these terms.
*Correspondence: Hong-Yuan Ma, mahongyuan@neigae.ac.cn; Hong-Wei Ni, nihongwei2000@163.com