- Harmon-Threatt Lab, Department of Entomology, University of Illinois at Urbana-Champaign, Urbana, IL, United States
The neurotoxic insecticide class of neonicotinoids has become one of the most widely used groups of pesticides globally. Their long half-lives and high water solubility increase their potential to linger and affect numerous organisms long after application. A prominent concern associated with residual contamination is the negative impact that neonicotinoids can have on beneficial arthropods such as bees and certain groups of beetles. Many studies have looked at the effects neonicotinoids have on arthropod communities in lab settings; however, comparatively few studies have looked at these groups in neonicotinoid-contaminated restored prairie habitats. These habitats are often restored from or located near agriculture and are almost ubiquitously contaminated with neonicotinoids. Our one-year manipulated field study compared native bee nesting rates and beetle community assemblages between paired clothianidin-contaminated and non-contaminated restored prairie plots. Native bee nesting probability and nesting abundance increased by 46% and 172%, respectively, in sites contaminated with clothianidin. Conversely, we observed no significant differences in beetle family assemblages, abundance, or richness between clothianidin-contaminated and control sites. These results suggest that neonicotinoid contamination of natural habitats can have numerous environmental consequences for arthropods and that these effects are not always consistent between taxa. Understanding how neonicotinoid contamination affects beneficial groups such as bees and arthropod community assemblages is crucial for characterizing the risks these chemicals pose to ecologically imperative taxa.
1 Introduction
Insects account for three-quarters of global animal and plant species, occupy a vast array of ecological niches (Chapman, 2009), and support countless ecosystem functions, such as nutrient cycling, soil formation, decomposition, water purification, and pollination, which have an outsized impact on the global economy. As insects decline, ecosystem structure and overall human well-being are expected to be adversely affected (Ameixa et al., 2018). The pervasive use of pesticides, specifically neonicotinoids, which is the most commonly used class of pesticide, is considered a major contributor to the decline of insects due to the impact that they have on non-target organisms (Godfray et al., 2014; Pisa et al., 2014; Hladik et al., 2018; Wagner et al., 2021). Yet, we still lack a fundamental understanding of how neonicotinoid contamination broadly affects non-target arthropods, as most neonicotinoid studies have focused on managed insect species that are not representative of the larger insect community.
Neonicotinoids have become ubiquitous in agricultural, industrial, and urban landscapes because of their low mammalian toxicity, application versatility, and tendency to contaminate adjacent habitats (Jeschke et al., 2011). The most prominent application method for neonicotinoids are seed coatings on agricultural commodities such as cereal grains and oilseeds (Jeschke et al., 2011). This method was developed to provide more targeted pest control, as neonicotinoids can be systemically incorporated into growing plants (Bonmatin et al., 2015). The incorporation of neonicotinoids into plant tissues has led to concerns about non-target exposure to pollinators in pollen and nectar (Rundlöf et al., 2015), but the proportion of the pesticide absorbed by target plants is small, ranging from 0.7-20% in several crop species (Sur and Stork, 2003). The remainder of the pesticide usually ends up in the soil, where it can persist for long periods of time. Clothianidin, the neonicotinoid with the longest environmental persistence, exhibits a half-life between 148-6931 days (Rexrode et al., 2003). This contamination can negatively impact the beneficial arthropods that natural habitats foster (Main et al., 2020; Kuechle et al., 2022). As neonicotinoids move into natural areas embedded in agroecosystems, these habitats could unintentionally attract beneficial insects to locations contaminated with harmful neonicotinoid insecticides.
Two beneficial insect groups that are often targeted in restoration efforts for their role in providing arthropod-mediated ecosystem services (AMES) (Isaacs et al., 2009) are bees and beetles. Both bees and beetles generally exhibit increases in richness and abundance in these restoration settings compared to agricultural fields (Purvis et al., 2020); however, due to differences in life history traits, bees and beetles are likely differentially affected by neonicotinoid contamination in these habitats. While numerous groups of beetles (Pfiffner and Luka, 2000) and the vast majority of bee species are ground-dwelling (Harmon-Threatt, 2020), the feeding guilds that they occupy vary (Losey and Vaughan, 2006). A majority of bee species are considered pollinators, while beetles occupy comparatively more feeding guilds including predators (Labrie et al., 2003; Menalled et al., 2004), pollinators (Young, 1986; Maia et al., 2013), detritivores (Wicklow et al., 1988; Topp et al., 2008), omnivores (Wäckers et al., 2005; Blubaugh et al., 2016), and herbivores (Alyokhin et al., 2008; Gray et al., 2009). The abundance and composition of these feeding guilds can have drastic impacts on ecosystem structure and function (Whiles and Charlton, 2006), including plant composition and biomass (Mulder et al., 1999), nutrient cycling and soil health (Stanton, 1988), and pest suppression (Moran and Hurd, 1997). Because these functional groups have evolved due to different evolutionary pressures in their ecological niche and have different routes of exposure to contamination, they are likely to respond differently to neonicotinoid exposure, therefore potentially shifting ecosystem structure and services.
Numerous studies have shown that neonicotinoid exposure can have negative consequences on cognitive and motor function (Alkassab and Kirchner, 2017; Tooming et al., 2017), reproduction and development (Alkassab and Kirchner, 2017; Wu-Smart and Spivak, 2018; Feng et al., 2019; Fortuin et al., 2021), and longevity (Alkassab and Kirchner, 2017; Feng et al., 2019). However, most neonicotinoid bee and beetle studies have been conducted in a lab setting with managed species. Because these managed species have either different social organization or nesting habits than most native ground-nesting species, potential effects and routes of exposure should not be broadly applied to all bees (Mayack and Boff, 2019). Furthermore, neonicotinoid studies rarely compare responses to insects in other orders, which would provide a more thorough community-level response. By looking at ground bee nesting and beetle functional group abundances in response to neonicotinoid contamination, this study will provide a more comprehensive picture of how beneficial insects are utilizing restorations from agriculture and the potential exposure risks for each group. Findings from this study could also be used to provide a more realistic account of the proposed benefits to beneficial insect communities associated with habitat restoration initiatives. The objectives of this study are to (i) evaluate differential ground bee nesting rates and (ii) beetle feeding guild assemblages between clothianidin-contaminated and non-contaminated soils of natural areas intended to augment conservation biological control systems.
2 Materials and methods
2.1 Study sites
Five fields located in Champaign and Vermilion Counties, IL, were utilized in the study. Each field site was separated by at least 500 m to ensure site independence. All field sites were previously in row crop agriculture but were removed from production for at least 17 years (Supplementary Table S1). Within each field site, a pair of 100m2 plots separated by 50m were established and assigned as either control or neonicotinoid treatment.
In May of 2018, one of the paired plots at each site was treated with the granular, clothianidin-based insecticide Arena 0.25G (Valent U.S.A. Corporation, Walnut Creek, CA) at a rate of 80g of active ingredient per acre. This application rate was chosen based on manufacturer recommendations for row crops. Because neonicotinoid levels in restoration habitats are notoriously heterogeneous and rates of persistence are dependent on other environmental variables (Felsot et al., 1998; Donnarumma et al., 2011; Sharma et al., 2014; Fletcher et al., 2018), we selected an application rate to reflect a worst-case scenario of clothianidin soil contamination in field margins or prairies recently restored from conventional agriculture; areas that are designed to attract and be a refuge for beneficial insect communities (Lagerlöf et al., 1992; Winfree, 2010). This application rate yielded soil contamination rates higher (Supplementary Table S4) than some studies in the neonicotinoid soil contamination literature (Hladik et al., 2017; Main et al., 2020). These higher rates were chosen to prevent treatment effects from being masked by higher variance typical of field-based studies, ultimately providing more confidence in the directionality and intensity of the treatment effect. While not traditionally used in row crop agriculture, Arena 0.25G was chosen as a delivery method to mimic pesticide soil deposition associated with seed coatings. It was selected over alternatives such as killed, treated seeds due to the ease of application and control of confounding variables. As most coated seeds contain other pesticides and the physical seed represents a carbon addition, Arena 0.25G was an appropriate application technique to control for clothianidin accurately.
All sites were sampled for arthropods, bare ground, floral abundance, and floral richness during June, July, and August 2018 as detailed below.
2.2 Bee and beetle sampling
During each sampling event, nine emergence tents (BugDorm, Taichung, Taiwan; model BT2006) were deployed in each plot (18 per site, 9 in control and 9 in treated plots) in a three-by-three grid with adjacent tents separated by 2m. Tent corners were staked down, and soil was packed on the edges of tent flaps to prevent insect movement in or out of the tent. A collection jar, located at the top of the tent at the end of a mesh funnel, was filled with soapy water to collect any insects emerging from the soil and vegetation beneath the tent. Tents were installed between 1800 and 2000 when diurnal insect movement decreases and retrieved after 72 hours, which was previously found to be sufficient to maximize captures of actively nesting female bees (Pane and Harmon-Threatt, 2017). Captured specimens were removed and placed into 70% ethanol. Bees were identified to species using keys created by Michael Arduser (unpublished, 2015) and Discover Life (Orr et al., 2021). These data were used to generate two separate response variables - bee abundance and bee nesting probability - in the models described below. Beetles captured were identified to at least family using American Beetles Volume II (Thomas et al., 2002), Beetles of Eastern North America (Evans, 2014), and A Field Guide to the Beetles of North America (White and Peterson, 1998). Feeding guilds (predator, herbivore, omnivore, and detritivore) for each beetle were assigned using the same resources. From these data, beetle richness, beetle abundance and abundance of each feeding guild were determined and used as responses in models described below.
2.3 Environmental covariate quantification
Environmental characteristics that influence ground bee nesting (O’Toole and Raw, 1991; Potts et al., 2005) and beetle abundance (Woodcock et al., 2008b; Diehl et al., 2012; Egerer et al., 2017) were quantified during each sampling session. Quantification was conducted using 16 independent 0.25 m2 quadrats, evenly spaced within each plot in a four-by-four grid and separated by 2m. Bare ground cover (exposed soil), floral abundance, and floral richness were recorded for all quadrats. For each sampling period, all 16 quadrat observations made within the same plot were averaged to provide more representative plot-scale metrics as model covariates.
2.4 Statistical analysis
All statistical modeling was conducted in R v4.3.1 (R Core Team, 2023). Generalized linear mixed effects models (GLMMs) were fitted using the glmer function in lme4 (v1.1-2 Bates et al., 2015) to establish global models for model selection. Average bare ground cover, average floral abundance, and average floral richness were considered as covariates in the model selection process. Site was included as a random effect in all models to account for the paired nature of the experimental design. Beetle abundance and richness data were pooled across months to deal with issues associated with low abundance for certain feeding guilds, heteroskedasticity, and model overfitting. Bee abundance and nesting probability did not have these issues and therefore were not pooled.
A model selection process utilizing AICc (Akaike information criterion with correction for small sample sizes) comparisons was used to identify the most predictive model(s) for bees and beetles. Models that had a delta AICc less than two were included as potential candidate models. Candidate models for bee nesting probability, bee abundance, beetle omnivore abundance and beetle detritivore abundance were used to create model averages using the model.avg function in the MuMIn package (v1.47.5 Bartoń, 2023) in order to account for numerous candidate models falling within our delta AICc cutoff of two (Johnson and Omland, 2004). Multicollinearity among independent variables was assessed by calculating variance inflation factors (VIF). VIF scores from all models showed low correlation between predictor variables, indicating that our covariates were sufficiently independent. Residuals from all models were assessed visually and via Shapiro-Wilk tests of normality. Heteroskedasticity was assessed visually and via Breusch-Pagan tests for all models.
GLMMs with binomial distributions were used to assess bee nesting probability. The probability of bee nesting was designated as the number of tents that captured one or more bees divided by the total number of tents. Because many ground-nesting species exhibit varying degrees of sociality and have been found to gregariously nest (Eickwort, 1975; Wcislo, 1992; Wuellner, 1999; Smith et al., 2003), analyzing bee nesting as tent success accounts for conspecifics that may otherwise be double counted. GLMMs with Poisson distributions were used to assess nesting bee abundance. Unlike the previous model, bee abundance is comprised of all captured females, therefore accounting for potential size variations in nest aggregations. In both bee nesting probability and bee abundance models, male and above-ground nesting bees (Harmon-Threatt, 2020) were omitted from analyses as they do not participate in nest construction (Antoine and Forrest, 2021). Due to low capture rates common with this sampling method, bee richness measures were not evaluated.
Generalized linear mixed effects models (GLMMs) with Poisson distributions were used to assess total beetle richness and abundance, as well as abundances for predator, herbivore, omnivore, and detritivore feeding guilds. In addition to environmental variables, models looking at individual feeding guilds included all other feeding guilds as possible covariates. These parameters were included in order to account for interspecific interactions. All model covariates were scaled in order to account for large eigenvalues observed during the model selection process. Residuals for the omnivore abundance models were not normally distributed and the issue could not be resolved using other model family residual methods. Furthermore, numerous beetle models exhibited symptoms of model overfitting. As a result, model predictions were tested using predicted residual error sum of squares (PRESS) and predictive R2 cross validation (Mediavilla et al., 2008). These methods showed that beetle herbivore and omnivore abundance models were drastically overfitting the data and therefore were not very predictive (Table 1). These models were therefore not used for further data interpretation.
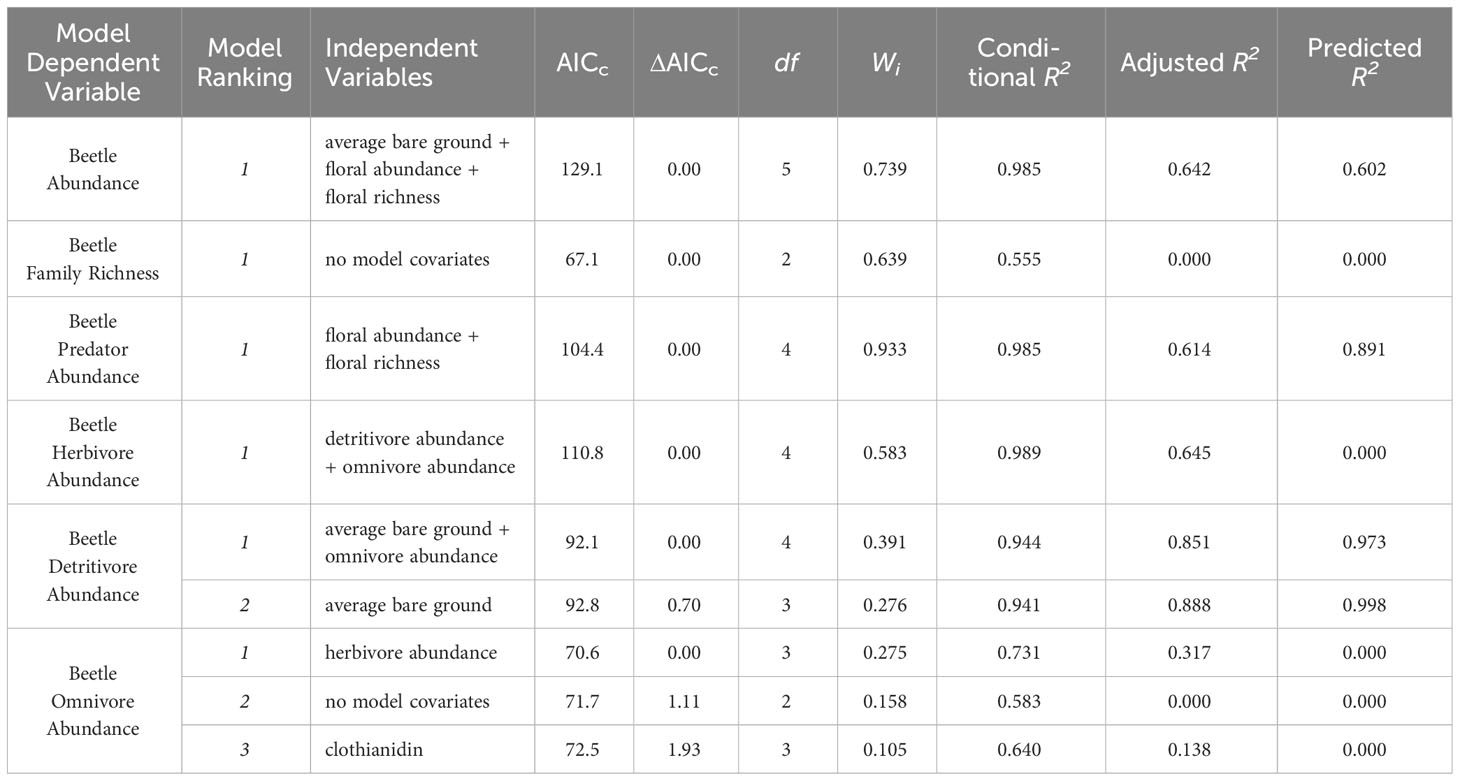
Table 1 Summary of model selection parameters and goodness of fit for evaluating overall beetle abundance, richness, and feeding guild models.
3 Results
3.1 Bee response to clothianidin
We caught 50 ground-nesting bee females from 11 species across the five sites and three sampling periods. A greater number of bees were seen in clothianidin-treated plots (8.6 ± 2.874) compared to control plots (1.4 ± 0.510), as seen in Figure 1B. Similarly, the average tent capture success was greater in clothianidin-treated plots (1.33 ± 0.187) than in control plots (0.47 ± 0.192) (Figure 1A).
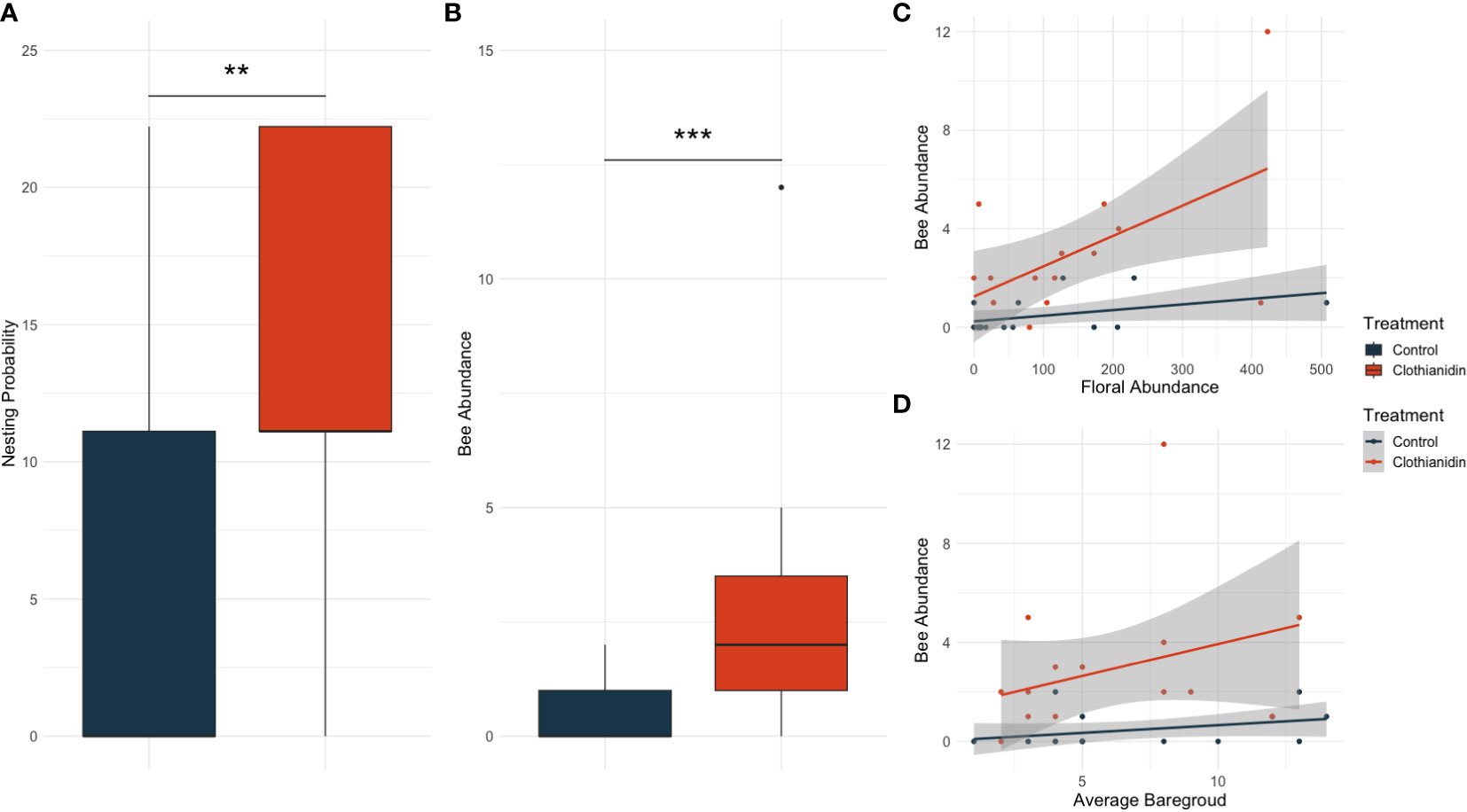
Figure 1 Significant predictors of the averaged top models for bee nesting probability (A) and bee abundance (B–D) in prairie restorations. Non-significant predictors that were present in our top models can be found in Table 2 and Supplementary Table S2. Asterisks indicate significance levels with two asterisks highlight p-values <0.01 and three asterisks highlighting p-values <0.001.
Four candidate models were similarly predictive for estimating the probability of bee nesting at the tent level. The averaged model included the independent variables clothianidin (Z = 2.326, P = 0.020), floral abundance (Z = 1.216, P = 0.224), floral richness (Z = 1.061, P = 0.289), and average bare ground (Z = 1.049, P = 0.294). (Table 2). Full model selection and estimates can be found in Table 2 and Supplementary Table S2, respectively.
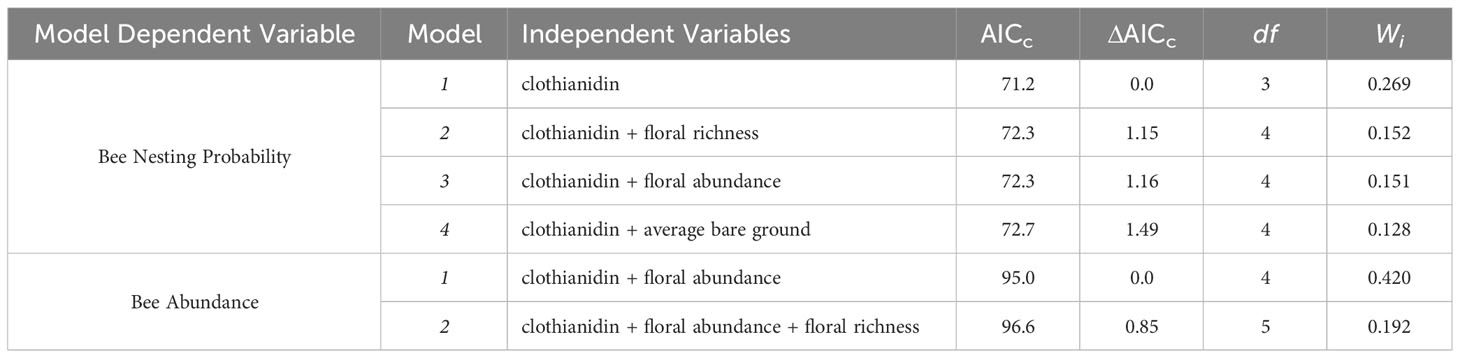
Table 2 Summary of model selection parameters for evaluating bee nesting percentage and bee abundance.
Likewise, four candidate models were similarly predictive of bee abundance and were averaged. The averaged model included the independent variables clothianidin (Z = 3.912, P < 0.001), floral abundance (Z = 2.099, P = 0.036), floral richness (Z = 1.799, P = 0.072), and average bare ground (Z = 2.011, P = 0.044) (Figures 1B–D). Full model selection and estimates for bees can be found in Table 2 and Supplementary Table S2, respectively.
3.2 Beetle response to clothianidin
We captured 2,613 beetles from 34 families across the sites and samples. Beetle abundance by family was dominated by Staphylinidae (792) and Chrysomelidae (652). Other prevalent families included Latridiidae (139), Carabidae (130), Mordellidae (118), and Phalacridae (111). Average ( ± SE) beetle abundance per site was similar between control plots (268.8 ± 71.639) and clothianidin plots (313.4 ± 82.622). Despite clothianidin not being a significant predictor of pooled beetle abundance, it was frequently present as a covariate in our top candidate models based on AICc comparisons. Full model selections and estimates for beetles can be found in Table 1 and Supplementary Table S3, respectively. Top model dependent variables and regression coefficients are compared visually using incident rate ratios and confidence intervals in Figure 2.
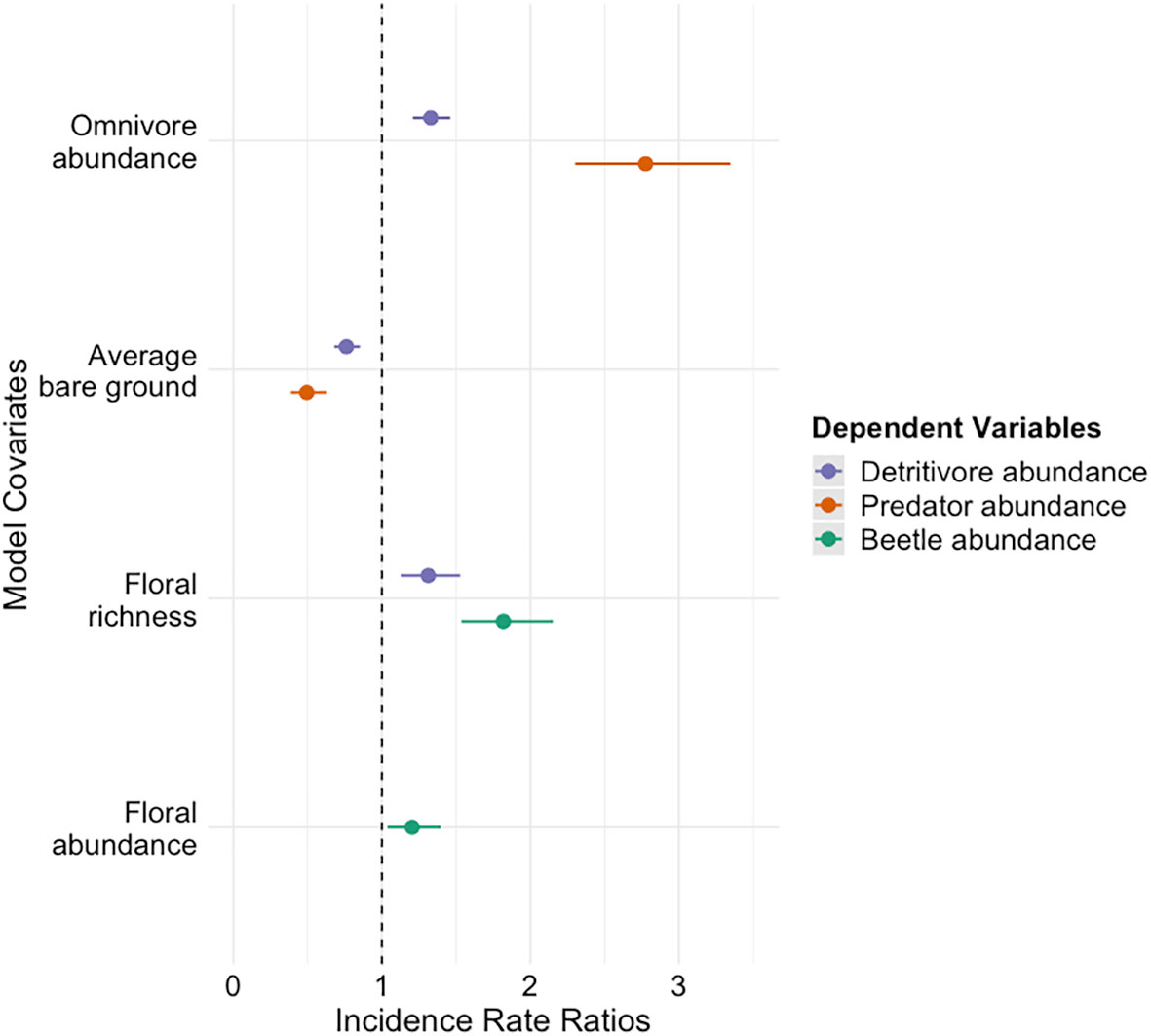
Figure 2 Top model dependent variables and regression coefficients are compared visually using incident rate ratios and confidence intervals (95%). Incident rate ratios provide a multiplying coefficient to describe direction and intensity of the effect that regression coefficients have on model dependent variables. Values in between zero and one indicate a negative relationship and numbers more than one indicate a positive relationship. Only models that are not overfit are shown.
Our most predictive model for beetle abundance included the scaled explanatory variables: floral abundance (Z = 5.876, P = <0.001), floral richness (Z = -4.678, P = <0.001), and average bare ground (Z = 3.491, P = <0.001). Our most predictive model for estimating beetle family richness did not include any model covariates. Significant predictors from top models are depicted in Figure 3.
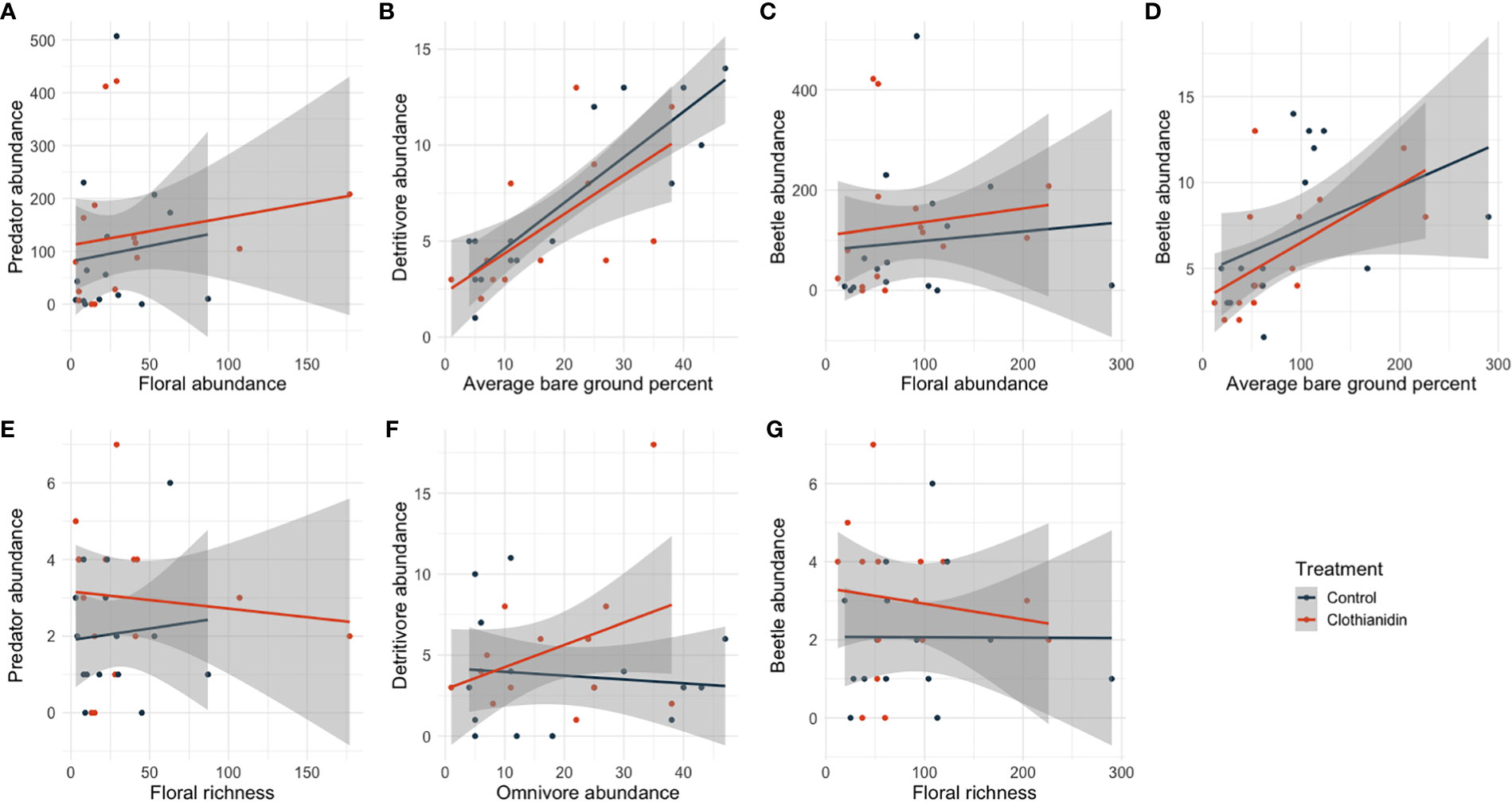
Figure 3 Significant regression coefficient predictors of overall beetle abundance (C, D, G), beetle predator abundance (A, E), and beetle detritivore abundance (B, F) in prairie restorations. Significant predictors were taken from all candidate models and not just model averages, which are often more conservative. Non-significant predictors that were present in our top models can be found in Table 1 and Supplementary Table S3.
Predacious beetles were highly abundant across sites and treatments with a total of 963 individuals across six families captured. A single predictive model of the abundance of predacious beetles included floral abundance (Z = 10.685, P = <0.001) and floral richness (Z = -5.657, P = <0.001) as explanatory variables. Significant predictors from any of the top models are depicted in Figure 3.
Beetle detritivores had a total of 554 individuals from 17 families. Two candidate models were averaged and include the explanatory variables: average bare ground (Z = 5.747, P = <0.001) and omnivore abundance (Z = 1.962, P = 0.050)Herbaceous beetles were also very common across sites and treatments with a total of 919 individuals from twelve families and omnivorous beetles had a total of 116 individuals. Both models showed symptoms of overfitting and were therefore not used for further interpretation.
4 Discussion
The results from our study indicate that high levels (Supplementary Table S4) of clothianidin in the soil of natural areas can have a profound yet inconsistent effect on the insect taxa found in those habitats. Ground bee abundance and nesting probability models showed a strong positive correlation with clothianidin contamination, while clothianidin contamination did not have an effect on beetle abundance, richness, or feeding guild abundances. Instead, beetle metrics were far more influenced by environmental factors. These differences outline the disproportionate effect that clothianidin contamination can have on insect communities in restoration ecosystems.
To our knowledge, this is the first study looking at ground bee nesting rates relative to clothianidin soil contamination in a restoration field experiment. These results contradict the findings of Willis Chan and Raine (2021), which showed a significant decrease in the nesting rates of the hoary squash bee (Eucera pruinosa) when exposed to different systematic insecticides in a manipulated agricultural setting. It is of note that herein we applied a single high dose of insecticides to restored prairies and examined immediate responses in active nest initiation and excavation, whereas in the Willis Chan and Raine (2021) study, different neonicotinoids were applied in a variety of application methods, and at different time points throughout the growing season. These differences in experimental design may influence the disparity in ground bee nesting rates in response to neonicotinoid contamination. Regardless, it suggests that many factors can contribute to differences in bee responses to contamination, including differences in insecticide type and application rate, experimental setting (agricultural vs. prairie restoration), or taxa (no bees from the genus Eucera were collected in our field experiment). In a follow-up study looking at bee emergence the year after application, so few bees were caught that data analysis could not be conducted (Tetlie and Harmon-Threatt unpublished work). This indicates that there could be high variability year to year and highlights the need for longitudinal work looking at bee communities over time in relation to neonicotinoid contamination.
While our results did not align with the only other nesting study on bees, they did have similarities with studies on oral exposure to neonicotinoids, which found that managed or semi-managed species do not avoid food sources containing neonicotinoid insecticides (Kessler et al., 2015; Arce et al., 2018). Furthermore, Kessler et al. (2015) found that honey and bumble bees consumed greater amounts of sucrose solutions that had been laced with neonicotinoids than sucrose alone. Similar results were seen by Singaravelan et al. (2005), who found that free-flying honey bees prefer to collect sucrose solutions containing low nicotine concentrations, the plant phytochemical from which the neonicotinoid class of insecticides is derived. The increases in feeding behavior from previous studies and the increase in nesting behavior seen in our data can likely be attributed to the mode of action of neonicotinoids themselves. Neonicotinoids target nicotinic acetylcholine receptors (nAChRs) throughout the brain, including the mushroom bodies required for learning and memory (Dupuis et al., 2011; Palmer et al., 2013). Sublethal doses of these neonicotinoids may provide an associated positive stimulus, therefore incentivizing congruent behaviors. It is however important to note that bee attraction to neonicotinoids is not ubiquitous (Kang and Jung, 2017; Fortuin and Gandhi, 2021); further highlighting the importance of additional variables which could augment bee behavior.
The increase in ground bee nesting behavior in contaminated soils that we have seen in our study and the affinity of various bee species to feed on neonicotinoid-laced foods could have significant negative consequences on bee communities. Previous work has shown that chronic exposure to neonicotinoids can affect larval mass, development speed, sex ratios, fecundity, and adult longevity in a variety of bee species (Laycock et al., 2012; Anderson and Harmon-Threatt, 2019; Strobl et al., 2019; Willis Chan and Raine, 2021). This is particularly concerning as many conservation efforts have focused on converting conventional agricultural fields and adjacent margins into pollinator habitats (M’Gonigle et al., 2015; Williams et al., 2015; Harmon-Threatt and Chin, 2016). Therefore, pollinator restorations can become ecological traps (Robertson and Hutto, 2006). Furthermore, sublethal doses of neonicotinoids have been shown to negatively interact with other factors connected to bee declines, such as pathogens and viruses (Alaux et al., 2010; Doublet et al., 2015), meaning that there could be compounding adverse effects associated with neonicotinoid exposure.
In contrast to bees, beetle abundance, richness, and feeding guild abundances were driven by environmental factors and not clothianidin contamination. These findings are contradictory to previous work indicating a negative impact of clothianidin on arthropod abundance (Main et al., 2018), beetle herbivores (Dembilio et al., 2015; Harmon et al., 2023) and predators (Pisa et al., 2017; Harmon et al., 2023). It is important to note that very few studies examine the effects of neonicotinoids on feeding guilds in restoration habitats – many being conducted in laboratory settings – and most studies focus on a specific family or species and not the broader community. That being said, numerous studies have shown that neonicotinoids can have negative effects on behavior (Kunkel et al., 2001; Moser and Obrycki, 2009; Tooming et al., 2017) and reproduction (Smith and Krischik, 1999; Papachristos and Milonas, 2008; Khani et al., 2012). While declines resulting from neonicotinoid contamination in overall beetle and feeding guild abundances were not observed in this study, sublethal exposure could lead to declines in subsequent years. Interestingly, the absence of an effect of clothianidin seen on detritivores in this study, is consistent with previous work (Main et al., 2018). While it is possible that sublethal effects could be seen in future years, some work indicates that detritivores are far more influenced by fungicides (Sánchez-Bayo et al., 2016), highlighting the importance of looking at multiple factors and the broader insect community when assessing the environmental impacts of agricultural inputs.
In their 2023 arthropod community-level study, Harmon et al. (2023)saw a reduction in arthropod herbivore and predator biomass in response to clothianidin contamination in a prairie restoration. These reductions in herbivore and predator biomass were inconsistent with our findings, but they could be indicative of trends that were not captured in our study. In the Harmon et al., 2023 study, insect sampling was done two years after the sites were restored to prairie from row crop agriculture and in both years prior to sampling, the sites were treated with clothianidin. This repeated exposure, could be causing negative generational effects on the arthropod community. Furthermore, the reduction in biomass could be a result of decreased body mass rather than reduced abundance; an observed phenomenon in numerous insect-neonicotinoid studies (Shi et al., 2017; Wu et al., 2021). Additional factors that could explain this discrepancy in findings include differences in the scope of taxa being analyzed (beetles vs. Arthropods) and differences in sampling technique (sweep netting vs. emergence tent sampling). Regardless, these differing results highlight the complexity of these study systems and the need for further community-level analysis in the neonicotinoid-arthropod literature.
Overall beetle abundance and detritivore abundance were significantly positively influenced by bare ground cover. These positive effects could be a symptom of the sampling method (i.e. the more bare ground present under a sampling tent, the less obscured the collection jar), however numerous studies indicate that beetle abundance and richness are positively influenced by habitat management practices such as periodical burns and grazing (Reed, 1997; Woodcock et al., 2008a). While not equivalent, these management practices create disturbance and temporarily increase the amount of bare ground. It is possible that a similar phenomenon is being observed in our study.
Overall beetle abundance and predator abundance were influenced by both floral abundance and richness. In both cases, floral abundance had a significantly positive effect on beetle and predator abundance, and floral richness had a significant negative effect. While positive associations between beetle and floral abundance are well represented in the literature (Miller, 2021; Killewald et al., 2023), the observed reductions in overall beetle and predator abundance in relation to increased floral richness is contradictory to much of the existing literature (Varchola and Dunn, 1999; Jonsson et al., 2009; Cook-Patton et al., 2011), however these findings are not unprecedented (Koricheva et al., 2000; Zou et al., 2013). It is also important to note that we measured flowering plant richness and not total plant richness. Not all beetle species have floral associations so this metric may not have been representative of the plant community diversity as a whole.
Despite their prophylactic application and ubiquity of use, the efficacy of neonicotinoid seed coatings on common pests of cereal crops, such as the western corn rootworm (Diabrotica virgifera) on corn (Zea mays), is limited. Studies by Boetel et al. (2003); Witmer et al. (2003), and Furlan et al. (2006), have shown that prophylactic neonicotinoid seed coatings do not manage or reduce corn rootworm population levels. While seed coatings have been effective in controlling other pest species on various crops (Krupke et al., 2017), the discrepancy in effectiveness against pests and the potential negative consequences to beneficial arthropod groups, brings the ubiquitous use of neonicotinoids as seed coatings into question. The findings from this study along with an abundance of neonicotinoid research, indicate that non-target organisms are differentially affected by neonicotinoids. Yet, very few studies examine these trends in field realistic settings and even fewer compare findings across taxa. The lack of consensus in the literature examining the negative effects of neonicotinoid contamination on beneficial arthropods, highlights the importance of field-based research, community level analysis, and longitudinal studies; which provide important environmental and long-term context. We believe that further examination of these factors is imperative to comprehensively assess the risks associated with neonicotinoid contamination of natural habitats and will allow for more informed management and policy decisions.
Data availability statement
The original contributions presented in the study are publicly available. Specimens collected in this experiment are available from the Illinois Data Bank: https://doi.org/10.13012/B2IDB-1762625_V1.
Ethics statement
Ethical approval was not required for the study involving animals in accordance with the local legislation and institutional requirements because research on insects typically does not require ethical approval. Special permitting is occasionally required, but only when the study involves an endangered species. This study did not involve any endangered species.
Author contributions
JT: Conceptualization, Data curation, Formal analysis, Funding acquisition, Investigation, Methodology, Project administration, Resources, Software, Validation, Visualization, Writing – original draft, Writing – review & editing. AH: Conceptualization, Funding acquisition, Project administration, Resources, Supervision, Validation, Writing – review & editing.
Funding
The author(s) declare financial support was received for the research, authorship, and/or publication of this article. This research was supported by USDA/NIFA award number 2018-67013-27537, the Prairie Biotic Research Corporation, and the School of Integrative Biology at the University of Illinois at Urbana-Champaign (Fred H. Schmidt Summer Scholars Award, Francis M. and Harlie M. Clark Research Support Grant, and Harley J. Van Cleave Research Award). The funders were not involved in the study design, collection, analysis, interpretation of data, the writing of the article or the decision to submit it for publication.
Acknowledgments
The authors would like to thank Adam Dolezal and Anthony Yannarell for their input in the establishment of this project, Tommy McElrath and Morgan Mackert for their assistance with beetle and bee identification and processing, Jamie Ellis and Nate Hudson for their assistance in site scouting and establishment of the sites used in this project, and Nick Anderson, Marissa Chase, Scott Clem, Brenna Decker, Ryan Leonard, Karl Roeder, Annaliese Wargin, Virginia Roberts, Crissy Gallick, Kristine Schoenecker, Jamilyn Martin, and Vi Aldunate for providing feedback on the manuscript.
Conflict of interest
The authors declare that the research was conducted in the absence of any commercial or financial relationships that could be construed as a potential conflict of interest.
Publisher’s note
All claims expressed in this article are solely those of the authors and do not necessarily represent those of their affiliated organizations, or those of the publisher, the editors and the reviewers. Any product that may be evaluated in this article, or claim that may be made by its manufacturer, is not guaranteed or endorsed by the publisher.
Supplementary material
The Supplementary Material for this article can be found online at: https://www.frontiersin.org/articles/10.3389/fevo.2024.1347526/full#supplementary-material
References
Alaux C., Brunet fr J.L., Dussaubat C., Mondet F., Tchamitchan S., Cousin M., et al. (2010). Interactions between Nosema microspores and a neonicotinoid weaken honeybees (Apis mellifera). Environ. Microbiol. 12, 774–782. doi: 10.1111/j.1462-2920.2009.02123.x
Alkassab A. T., Kirchner W. H. (2017). Sublethal exposure to neonicotinoids and related side effects on insect pollinators: honeybees, bumblebees, and solitary bees. J. Plant Dis. Prot. 124, 1–30. doi: 10.1007/s41348-016-0041-0
Alyokhin A., Baker M., Mota-Sanchez D., Dively G., Grafius E. (2008). Colorado potato beetle resistance to insecticides. Am. J. Potato Res. 85, 395–413. doi: 10.1007/s12230-008-9052-0
Ameixa O., Soares A. O., Soares A., Lillebø A. I. (2018). “Ecosystem services provided by the little things that run the world,” in Selected studies in biodiversity (London: IntechOpen), 267–302.
Anderson N. L., Harmon-Threatt A. N. (2019). Chronic contact with realistic soil concentrations of imidacloprid affects the mass , immature development speed , and adult longevity of solitary bees. Sci. Rep. 1–9. doi: 10.1038/s41598-019-40031-9
Antoine C. M., Forrest J. R. K. (2021). Nesting habitat of ground-nesting bees: a review. Ecol. Entomol. 46, 143–159. doi: 10.1111/een.12986
Arce A. N., Rodrigues A. R., Yu J., Colgan T. J., Wurm Y., Gill R. J. (2018). Foraging bumblebees acquire a preference for neonicotinoid-treated food with prolonged exposure. Proc. R. Soc B Biol. Sci. 285, 8–11. doi: 10.1098/rspb.2018.0655
Bates D., Mächler M., Bolker B. M., Walker S. C. (2015). Fitting linear mixed-effects models using lme4. J. Stat. Software 67, 1–48. doi: 10.18637/jss.v067.i01
Blubaugh C. K., Hagler J. R., Machtley S. A., Kaplan I. (2016). Cover crops increase foraging activity of omnivorous predators in seed patches and facilitate weed biological control. Agric. Ecosyst. Environ. 231, 264–270. doi: 10.1016/j.agee.2016.06.045
Boetel M. A., Fuller B. W., Evenson P. D. (2003). Emergence of adult northern and western corn rootworms (Coleoptera: chrysomelidae) following reduced soil insecticide applications. J. Econ. Entomol. 96, 714–729. doi: 10.1093/jee/96.3.714
Bonmatin J. M., Giorio C., Girolami V., Goulson D., Kreutzweiser D. P., Krupke C., et al. (2015). Environmental fate and exposure; neonicotinoids and fipronil. Environ. Sci. pollut. Res. 22, 35–67. doi: 10.1007/s11356-014-3332-7
Chapman A. (2009). Numbers of living species in Australia and the world (Canberra, Australia). doi: 10.1111/j.1398-9995.1975.tb00205.x
Cook-Patton S. C., Mcart S. H., Parachnowitsch A. L., Thaler J. S., Agrawal A. A. (2011). A direct comparison of the consequences of plant genotypic and species diversity on communities and ecosystem function. Ecology 92, 915–923. doi: 10.1890/10-0999.1
Dembilio Ó., Riba J. M., Gamón M., Jacas J. A. (2015). Mobility and efficacy of abamectin and imidacloprid against Rhynchophorus ferrugineus in Phoenix canariensis by different application methods. Pest Manage. Sci. 71, 1091–1098. doi: 10.1002/ps.3889
Diehl E., Wolters V., Birkhofer K. (2012). Arable weeds in organically managed wheat fields foster carabid beetles by resource- and structure-mediated effects. Arthropod. Plant Interact. 6, 75–82. doi: 10.1007/s11829-011-9153-4
Donnarumma L., Pulcini P., Pochi D., Rosati S., Lusco L., Conte E. (2011). Preliminary study on persistence in soil and residues in maize of imidacloprid. J. Environ. Sci. Heal. - Part B Pestic. Food Contam. Agric. Wastes 46, 469–472. doi: 10.1080/03601234.2011.583848
Doublet V., LabaRussias M., de Miranda J. R., Moritz R. F. A., Paxton R. J. (2015). Bees under stress: Sublethal doses of a neonicotinoid pesticide and pathogens interact to elevate honey bee mortality across the life cycle. Environ. Microbiol. 17, 969–983. doi: 10.1111/1462-2920.12426
Dupuis J. P., Gauthier M., Raymond-Delpech V. (2011). Expression patterns of nicotinic subunits α2, α7, α8, and β1 affect the kinetics and pharmacology of ACh-induced currents in adult bee olfactory neuropiles. J. Neurophysiol. 106, 1604–1613. doi: 10.1152/jn.00126.2011
Egerer M. H., Bichier P., Philpott S. M. (2017). Landscape and local habitat correlates of lady beetle abundance and species richness in urban agriculture. Ann. Entomol. Soc Am. 110, 97–103. doi: 10.1093/aesa/saw063
Eickwort G. C. (1975). Gregarious nesting of the mason bee hoplitis anthocopoides and the evolution of parasitism and sociality among megachilid bees. Evol. (N. Y). 29, 142. doi: 10.2307/2407147
Evans A. V. (2014). Beetles of eastern north america (Princeton: Princeton University Press). Available at: https://books.google.com/books?id=72SYDwAAQBAJ.
Felsot A. S., Cone W., Yu J., Ruppert J. R. (1998). Distribution of imidacloprid in soil following subsurface drip chemigation. Bull. Environ. Contam. Toxicol. 60, 363–370. doi: 10.1007/s001289900635
Feng W.B., Bong L. J., Dai S. M., Neoh K. B. (2019). Effect of imidacloprid exposure on life history traits in the agricultural generalist predator Paederus beetle: Lack of fitness cost but strong hormetic effect and skewed sex ratio. Ecotoxicol. Environ. Saf. 174, 390–400. doi: 10.1016/j.ecoenv.2019.03.003
Fletcher E., Morgan K. T., Qureshi J. A., Leiva J. A., Nkedi-Kizza P. (2018). Imidacloprid soil movement under micro-sprinkler irrigation and soil-drench applications to control Asian citrus psyllid (ACP) and citrus leafminer (CLM). PloS One 13, 1–16. doi: 10.1371/journal.pone.0192668
Fortuin C. C., Gandhi K. J. K. (2021). Mason bees (Hymenoptera: megachilidae) exhibit no avoidance of imidacloprid-treated soils. Environ. Entomol. 50, 1438–1445. doi: 10.1093/ee/nvab083
Fortuin C. C., McCarty E., Gandhi K. J. (2021). Acute contact with imidacloprid in soil affects the nesting and survival success of a solitary wild bee, Osmia lignaria (Hymenoptera: Megachilidae). Chemosphere 264, 128572. doi: 10.1016/j.chemosphere.2020.128572
Furlan L., Canzi S., Di Bernardo A., Edwards C. R. (2006). The ineffectiveness of insecticide seed coatings and planting-time soil insecticides as Diabrotica virgifera virgifera LeConte population suppressors. J. Appl. Entomol. 130, 485–490. doi: 10.1111/j.1439-0418.2006.01103.x
Godfray H. C. J., Blacquière T., Field L. M., Hails R. S., Petrokofsky G., Potts S. G., et al. (2014). A restatement of the natural science evidence base concerning neonicotinoid insecticides and insect pollinators. Proc. R. Soc B Biol. Sci. 281. doi: 10.1098/rspb.2014.0558
Gray M. E., Sappington T. W., Miller N. J., Moeser J., Bohn M. O. (2009). Adaptation and invasiveness of western corn rootworm: Intensifying research on a worsening pest. Annu. Rev. Entomol. 54, 303–321. doi: 10.1146/annurev.ento.54.110807.090434
Harmon G. T., Harmon-Threatt A. N., Anderson N. L. (2023). Changes in predator biomass may mask the negative effects of neonicotinoids on primary consumers in field settings. Insect Conserv. Divers. 16, 298–305. doi: 10.1111/icad.12625
Harmon-Threatt A. (2020). Influence of nesting characteristics on health of wild bee communities. Annu. Rev. Entomol. 65, 39–56. doi: 10.1146/annurev-ento-011019-024955
Harmon-Threatt A., Chin K. (2016). Common methods for tallgrass prairie restoration and their potential effects on bee diversity. Nat. Areas J. 36, 400–411. doi: 10.3375/043.036.0407
Hladik M. L., Bradbury S., Schulte L. A., Helmers M., Witte C., Kolpin D. W., et al. (2017). Neonicotinoid insecticide removal by prairie strips in row-cropped watersheds with historical seed coating use. Agric. Ecosyst. Environ. 241, 160–167. doi: 10.1016/j.agee.2017.03.015
Hladik M. L., Main A. R., Goulson D. (2018). Environmental risks and challenges associated with neonicotinoid insecticides. Environ. Sci. Technol. 52, 3329–3335. doi: 10.1021/acs.est.7b06388
Isaacs R., Tuell J., Fiedler A., Gardiner M., Landis D. (2009). Maximizing arthropod-mediated ecosystem services in agricultural landscapes: The role of native plants. Front. Ecol. Environ. 7, 196–203. doi: 10.1890/080035
Jeschke P., Nauen R., Schindler M., Elbert A. (2011). Overview of the status and global strategy for neonicotinoids. J. Agric. Food Chem. 59, 2897–2908. doi: 10.1021/jf101303g
Johnson J. B., Omland K. S. (2004). Model selection in ecology and evolution. Trends Ecol. Evol. 19, 101–108. doi: 10.1016/j.tree.2003.10.013
Jonsson M., Yeates G. W., Wardle D. A. (2009). Patterns of invertebrate density and taxonomic richness across gradients of area, isolation, and vegetation diversity in a lake-island system. Ecography (Cop.). 32, 963–972. doi: 10.1111/j.1600-0587.2009.05784.x
Kang M., Jung C. (2017). Avoidance Behavior of Honey bee, Apis mellifera from Commonly used Fungicides, Acaricides and Insecticides in Apple Orchards. J. Apic. 32, 295–302. doi: 10.17519/apiculture.2017.11.32.4.295
Kessler S. C., Tiedeken E. J., Simcock K. L., Derveau S., Mitchell J., Softley S., et al. (2015). Bees prefer foods containing neonicotinoid pesticides. Nature 521, 74–76. doi: 10.1038/nature14414
Khani A., Ahmadi F., Ghadamyari M. (2012). Side effects of imidacloprid and abamectin on the mealybug destroyer , cryptolaemus montrouzieri. Trakia J. Sci. 10, 30–35.
Killewald M. F., Costamagna A. C., Lawley Y., Gulden R. H., Gibbs J. (2023). Floral strips adjacent to Manitoba crop fields attract beneficial insects shortly after establishment regardless of management type or landscape context. Agric. For. Entomol. 26, 18–37. doi: 10.1111/afe.12595
Koricheva J., Mulder C. P. H., Schmid B., Joshi J., Huss-Danell K. (2000). Numerical responses of different trophic groups of invertebrates to manipulations of plant diversity in grasslands. Oecologia 125, 271–282. doi: 10.1007/s004420000450
Krupke C. H., Alford A. M., Cullen E. M., Hodgson E. W., Knodel J. J., McCornack B., et al. (2017). Assessing the value and pest management window provided by neonicotinoid seed treatments for management of soybean aphid (Aphis glycines Matsumura) in the Upper Midwestern United States. Pest Manage. Sci. 73, 2184–2193. doi: 10.1002/ps.4602
Kuechle K. J., Webb E. B., Mengel D., Main A. R. (2022). Seed treatments containing neonicotinoids and fungicides reduce aquatic insect richness and abundance in midwestern USA–managed floodplain wetlands. Environ. Sci. pollut. Res. 29, 45261–45275. doi: 10.1007/s11356-022-18991-9
Kunkel B. A., Held D. W., Potter D. A. (2001). Lethal and sublethal effects of bendiocarb, halofenozide, and imidacloprid on Harpalus pennsylvanicus (Coleoptera: Carabidae) following different modes of exposure in Turfgrass. J. Econ. Entomol. 94, 60–67. doi: 10.1603/0022-0493-94.1.60
Labrie G., Prince C., Bergeron J. M. (2003). Abundance and developmental stability of Pterostichus melanarius (Coleoptera: Carabidae) in organic and integrated pest management orchards of Québec, Canada. Environ. Entomol. 32, 123–132. doi: 10.1603/0046-225X-32.1.123
Lagerlöf J., Stark J., Svensson B. (1992). Margins of agricultural fields as habitats for pollinating insects. Agric. Ecosyst. Environ. 40, 117–124. doi: 10.1016/0167-8809(92)90087-R
Laycock I., Lenthall K. M., Barratt A. T., Cresswell J. E. (2012). Effects of imidacloprid, a neonicotinoid pesticide, on reproduction in worker bumble bees (Bombus terrestris). Ecotoxicology 21, 1937–1945. doi: 10.1007/s10646-012-0927-y
Losey J. E., Vaughan M. (2006). The economic value of ecological services provided by insects. Bioscience 56, 311. doi: 10.1641/0006-3568(2006)56[311:tevoes]2.0.co;2
Maia A. C. D., Gibernau M., Dötterl S., Do Amaral Ferraz Navarro D. M., Seifert K., Müller T., et al. (2013). The floral scent of Taccarum ulei (Araceae): Attraction of scarab beetle pollinators to an unusual aliphatic acyloin. Phytochemistry 93, 71–78. doi: 10.1016/j.phytochem.2013.03.005
Main A. R., Webb E. B., Goyne K. W., Mengel D. (2018). Neonicotinoid insecticides negatively affect performance measures of non-target terrestrial arthropods: a meta-analysis. Ecol. Appl. 28, 1232–1244. doi: 10.1002/eap.1723
Main A. R., Webb E. B., Goyne K. W., Mengel D. (2020). Reduced species richness of native bees in field margins associated with neonicotinoid concentrations in non-target soils. Agric. Ecosyst. Environ. 287, 106693. doi: 10.1016/j.agee.2019.106693
Mayack C., Boff S. (2019). LD50 values may be misleading predictors of neonicotinoid toxicity across different bee species. Uludag Aricilik Derg. 19, 19–33. doi: 10.31467/uluaricilik.568251
Mediavilla F. A. M., Landrum F., Shah V. (2008). A comparison of the coefficient of predictive power, the coefficient of determination and AIC for linear regression. J. Appl. Bus. Econ. 8, 1261–1266. Available at: http://www.digitalcommons.www.na-businesspress.com/JABE/MendezWeb.pdf.
Menalled F. D., Landis D. A., Dyer L. E. (2004). Research and extension supporting ecologically based IPM systems. J. Crop Improv. 11, 153–174. doi: 10.1300/J411v11n01_08
M’Gonigle L. K., Ponisio L. C., Cutler K., Kremen C. (2015). Habitat restoration promotes pollinator persistence and colonization in intensively managed agriculture. Ecol. Appl. 25, 1557–1565. doi: 10.1890/14-1863.1
Miller R. B. (2021). Management and landscape effects on beneficial tallgrass prairie insects - a study of bees and beetles. 1–160.
Moran M. D., Hurd L. E. (1997). A trophic cascade in a diverse arthropod community caused by a generalist arthropod predator. Oecologia 113, 126–132. doi: 10.1007/s004420050360
Moser S. E., Obrycki J. J. (2009). Non-target effects of neonicotinoid seed treatments; mortality of coccinellid larvae related to zoophytophagy. Biol. Control 51, 487–492. doi: 10.1016/j.biocontrol.2009.09.001
Mulder C. P. H., Koricheva J., Huss-Danell K., Högberg P., Joshi J. (1999). Insects affect relationships between plant species richness and ecosystem processes. Ecol. Lett. 2, 237–246. doi: 10.1046/j.1461-0248.1999.00070.x
Orr M. C., Hughes A. C., Chesters D., Pickering J., Zhu C. D., Ascher J. S. (2021). Global patterns and drivers of bee distribution. Curr. Biol. 31, 451–458.e4. doi: 10.1016/j.cub.2020.10.053
Palmer M. J., Moffat C., Saranzewa N., Harvey J., Wright G. A., Connolly C. N. (2013). Cholinergic pesticides cause mushroom body neuronal inactivation in honeybees. Nat. Commun. 4, 1–8. doi: 10.1038/ncomms2648
Pane A. M., Harmon-Threatt A. N. (2017). An assessment of the efficacy and peak catch rates of emergence tents for measuring bee nesting. Appl. Plant Sci. 5, 1700007. doi: 10.3732/apps.1700007
Papachristos D. P., Milonas P. G. (2008). Adverse effects of soil applied insecticides on the predatory coccinellid Hippodamia undecimnotata (Coleoptera: Coccinellidae). Biol. Control 47, 77–81. doi: 10.1016/j.biocontrol.2008.06.009
Pfiffner L., Luka H. (2000). Overwintering of arthropods in soils of arable fields and adjacent semi-natural habitats. Agric. Ecosyst. Environ. 78, 215–222. doi: 10.1016/S0167-8809(99)00130-9
Pisa L. W., Amaral-Rogers V., Belzunces L. P., Bonmatin J. M., Downs C. A., Goulson D., et al. (2014). Effects of neonicotinoids and fipronil on non-target invertebrates. Environ. Sci. Pollut. Res. 22, 68–102. doi: 10.1007/s11356-014-3471-x
Pisa L., Goulson D., Yang E. C., Gibbons D., Sánchez-Bayo F., Mitchell E., et al. (2017). An update of the Worldwide Integrated Assessment (WIA) on systemic insecticides. Part 2: impacts on organisms and ecosystems. Environ. Sci. Pollut. Res. 28, 1–49. doi: 10.1007/s11356-017-0341-3
Potts S. G., Vulliamy B., Roberts S., O’Toole C., Dafni A., Ne’eman G., et al. (2005). Role of nesting resources in organising diverse bee communities in a Mediterranean landscape. Ecol. Entomol. 30, 78–85. doi: 10.1111/j.0307-6946.2005.00662.x
Purvis E. E. N., Vickruck J. L., Best L. R., Devries J. H., Galpern P. (2020). Wild bee community recovery in restored grassland-wetland complexes of prairie North America. Biol. Conserv. 252, 108829. doi: 10.1016/j.biocon.2020.108829
R Core Team (2023)R: A language and environment for statistical computing. Available at: https://www.r-project.org/.
Reed C. C. (1997). Responses of prairie insects and other arthropods to prescription burns. Nat. Areas J. 17 (4), 380–385.
Rexrode M., Barrett M., Ellis J., Patrick G., Vaughan A., Felkel J., et al. (2003). EFED risk assessment for the seed treatment of clothianidin 600FS on corn and canola 1–91.
Robertson B., Hutto R. (2006). A framework for understanding ecological traps and an evaluation of existing evidence. Ecology 87, 1075–1085. doi: 10.1890/0012-9658(2006)87[1075:AFFUET]2.0.CO;2
Rundlöf M., Andersson G. K. S., Bommarco R., Fries I., Hederström V., Herbertsson L., et al. (2015). Seed coating with a neonicotinoid insecticide negatively affects wild bees. Nature 521, 77–80. doi: 10.1038/nature14420
Sánchez-Bayo F., Goulson D., Pennacchio F., Nazzi F., Goka K., Desneux N. (2016). Are bee diseases linked to pesticides? - A brief review. Environ. Int.. 89–90, 7–11. doi: 10.1016/j.envint.2016.01.009
Sharma S., Singh B., Gupta V. K. (2014). Biodegradation of imidacloprid by consortium of two soil isolated bacillus sp. Bull. Environ. Contam. Toxicol. 93, 637–642. doi: 10.1007/s00128-014-1386-3
Shi T. F., Wang Y. F., Liu F., Qi L., Yu L. S. (2017). Sublethal effects of the neonicotinoid insecticide thiamethoxam on the transcriptome of the honey bees (Hymenoptera: apidae). J. Econ. Entomol. 110, 2283–2289. doi: 10.1093/jee/tox262
Singaravelan N., Nee'man G., Inbar M., Izhaki I. (2005). Feeding responses of free-flying honeybees to secondary compounds mimicking floral nectars. J. Chem. Ecol. 31, 2791–2804. doi: 10.1007/s10886-005-8394-z
Smith S. F., Krischik V. A. (1999). Effects of systemic imidacloprid on Coleomegilla maculata (Coleoptera: Coccinellidae). Environ. Entomol. 28, 1189–1195. doi: 10.1093/ee/28.6.1189
Smith A. R., Wcislo W. T., O’Donnell S. (2003). Assured fitness returns favor sociality in a mass-provisioning sweat bee, Megalopta genalis (Hymenoptera: Halictidae). Behav. Ecol. Sociobiol. 54, 14–21. doi: 10.1007/s00265-003-0589-0
Stanton N. L. (1988). The underground in grasslands. Annu. Rev. Ecol. Syst. 19, 573–589. doi: 10.1146/annurev.ecolsys.19.1.573
Strobl V., Albrecht M., Radford S., Wolf S., Neumann P. (2019). A short note on extreme sex ratio in solitary bees Osmia cornuta in semi-field trials testing the impact of neonicotinoids. J. Apic. Res. 58, 469–470. doi: 10.1080/00218839.2018.1552238
Sur R., Stork A. (2003). Uptake, translocation and metabolism of imidacloprid in plants. Bull. Insectology 56, 35–40.
Thomas M. C., Skelley P. E., Frank J. H. (2002). American Beetles, Volume II: Polyphaga: Scarabaeoidea through Curculionoidea (Boca Raton: CRC Press). Available at: https://books.google.com/books?id=YiPNBQAAQBAJ.
Tooming E., Merivee E., Must A., Merivee M. I., Sibul I., Nurme K., et al. (2017). Behavioural effects of the neonicotinoid insecticide thiamethoxam on the predatory insect Platynus assimilis. Ecotoxicology 26, 902–913. doi: 10.1007/s10646-017-1820-5
Topp W., Kappes H., Rogers F. (2008). Response of ground-dwelling beetle (Coleoptera) assemblages to giant knotweed (Reynoutria spp.) invasion. Biol. Invasions 10, 381–390. doi: 10.1007/s10530-007-9137-6
Varchola J. M., Dunn J. P. (1999). Changes in ground beetle (Coleoptera: Carabidae) assemblages in farming systems bordered by complex or simple roadside vegetation. Agric. Ecosyst. Environ. 73, 41–49. doi: 10.1016/S0167-8809(99)00009-2
Wäckers F. L., van Rijn P. C. J., Bruin J. (2005). Plant-Provided Food for Carnivorous Insects: A Protective Mutualism and its Applications (Cambridge: Cambridge University Press). Available at: https://books.google.com/books?id=7j1WyqPAARwC.
Wagner D. L., Grames E. M., Forister M. L., Berenbaum M. R., Stopak D. (2021). Insect decline in the Anthropocene: Death by a thousand cuts. Proc. Natl. Acad. Sci. U. S. A. 118, 1–10. doi: 10.1073/PNAS.2023989118
Wcislo W. T. (1992). Nest localization and recognition in a solitary bee, lasioglossum (Dialictus) figueresi wcislo (Hymenoptera: halictidae), in relation to sociality. Ethology 92, 108–123. doi: 10.1111/j.1439-0310.1992.tb00952.x
Whiles M. R., Charlton R. E. (2006). The ecological significance of tallgrass prairie arthropods. Annu. Rev. Entomol. 51, 387–412. doi: 10.1146/annurev.ento.51.110104.151136
White R. E., Peterson R. T. (1998). A Field Guide to the Beetles of North America. (New York: Houghton Mifflin). Available at: https://books.google.com/books?id=S9IK9OMjuNEC.
Wicklow D. T., Dowd P. F., Tepaske M. R., Gloer J. B. (1988). Sclerotial metabolites of Aspergillus Flavus toxic to a detritivorous maize insect (Carpophilus Hemipterus, Nitidulidae). Trans. Br. Mycol. Soc 91, 433–438. doi: 10.1016/s0007-1536(88)80119-0
Williams N. M., Ward K. L., Pope N., Isaacs R., Wilson J., May E. A., et al. (2015). Native wildflower plantings support wild bee abundance and diversity in agricultural landscapes across the United States. Ecol. Appl. 25, 2119–2131. doi: 10.1890/14-1748.1
Willis Chan D. S., Raine N. E. (2021). Population decline in a ground-nesting solitary squash bee (Eucera pruinosa) following exposure to a neonicotinoid insecticide treated crop (Cucurbita pepo). Sci. Rep. 11, 1–11. doi: 10.1038/s41598-021-83341-7
Winfree R. (2010). The conservation and restoration of wild bees. Ann. N. Y. Acad. Sci. 1195, 169–197. doi: 10.1111/j.1749-6632.2010.05449.x
Witmer J. E., Hough-Goldstein J. A., Pesek J. D. (2003). Ground-dwelling and foliar arthropods in four cropping systems. Environ. Entomol. 32, 366–376. doi: 10.1603/0046-225X-32.2.366
Woodcock B. A., Edwards A. R., Lawson C. S., Westbury D. B., Brook A. J., Harris S. J., et al. (2008a). Contrasting success in the restoration of plant and phytophagous beetle assemblages of species-rich mesotrophic grasslands. Oecologia 154, 773–783. doi: 10.1007/s00442-007-0872-2
Woodcock B. A., Westbury D. B., Tscheulin T., Harrison-Cripps J., Harris S. J., Ramsey A. J., et al. (2008b). Effects of seed mixture and management on beetle assemblages of arable field margins. Agric. Ecosyst. Environ. 125, 246–254. doi: 10.1016/j.agee.2008.01.004
Wu C., Zhang H., He M., Dong F., Xu J., Wu X., et al. (2021). Toxicity of neonicotinoid insecticides on key non-target natural predator the larvae of Coccinella septempunctata in environmental. Environ. Technol. Innov. 23, 101523. doi: 10.1016/j.eti.2021.101523
Wuellner C. T. (1999). Nest site preference and success in a gregarious, ground-nesting bee Dieunomia triangulifera. Ecol. Entomol. 24, 471–479. doi: 10.1046/j.1365-2311.1999.00215.x
Wu-Smart J., Spivak M. (2018). Effects of neonicotinoid imidacloprid exposure on bumble bee (Hymenoptera: Apidae) queen survival and nest initiation. Environ. Entomol. 47, 55–62. doi: 10.1093/ee/nvx175
Young H. J. (1986). Beetle pollination of dieffenbachia longispatha (Araceae). Am. J. Bot. 73, 931. doi: 10.2307/2444306
Keywords: ground-nesting bees, beetle feeding guilds, clothianidin, restored prairie, agricultural contamination
Citation: Tetlie J and Harmon-Threatt A (2024) Neonicotinoid contamination in conservation areas affects bees more sharply than beetles. Front. Ecol. Evol. 12:1347526. doi: 10.3389/fevo.2024.1347526
Received: 30 November 2023; Accepted: 25 January 2024;
Published: 19 February 2024.
Edited by:
Kris Braman, University of Georgia, United StatesReviewed by:
Lewis J. Bartlett, University of Georgia, United StatesChristine Fortuin, Mississippi State University, United States
Copyright © 2024 Tetlie and Harmon-Threatt. This is an open-access article distributed under the terms of the Creative Commons Attribution License (CC BY). The use, distribution or reproduction in other forums is permitted, provided the original author(s) and the copyright owner(s) are credited and that the original publication in this journal is cited, in accordance with accepted academic practice. No use, distribution or reproduction is permitted which does not comply with these terms.
*Correspondence: Alexandra Harmon-Threatt, aht@illinois.edu