- Centre for Ecology and Conservation, University of Exeter, Cornwall, United Kingdom
The expansion of urban areas affects wild animals in a variety of ways. Why members of closely-related species respond differently to urbanisation is often unclear, but an understanding of the factors that lead to urban habitat use or avoidance will have important implications for conservation. Previous research has suggested that urban habitats could favour larger-brained, behaviourally flexible species, which can more readily cope with the novel challenges imposed by urbanisation. However, the opportunity species have to colonise urban areas, and similarities between urban areas and species’ natural habitats, may also explain urban habitat use. We use phylogenetic path analysis to investigate factors that could promote urban breeding and foraging in the gull subfamily, a group with several urbanised species. While we find little evidence to support a relationship between brain size and urban foraging, we reveal an indirect relationship between brain size and urban breeding: cliff-nesting species have relatively larger brains and these species are more likely to breed in urban areas. We show that cliff nesting in gulls is a derived trait and may therefore reflect plasticity in breeding habitat choice, facilitating the use of buildings as nesting sites. Finally, we show that urbanised gull species are less likely to be of international conservation concern or decreasing in population size, exposing the need for more research on the causes and consequences of urban habitat use.
1 Introduction
Urbanisation of habitats can create both challenges and opportunities for wild animals (McKinney, 2006). Explaining why certain species choose to use urban areas, and how they manage to thrive in these habitats while others are unable to do so, is essential for fully understanding humans’ impact on wildlife. While it is evident that some species, such as foxes (Vulpes vulpes) and raccoons (Procyon lotor), fare better in urban areas than they do in rural ones (Chace and Walsh, 2006; Bateman and Fleming, 2012), urbanisation is generally associated with negative consequences for wild animals, such as greater exposure to toxicants and parasites (Murray et al., 2019), fatal collisions with buildings (Loss et al., 2014) and traffic-induced mortality (Glista et al., 2008). Furthermore, species that use urban areas are sometimes considered a nuisance due to unwanted interactions with humans, creating conflict between prioritising human wellbeing and preserving wildlife (Lyytimäki et al., 2008; Barrett et al., 2019). It is therefore not always clear whether urban living can promote stable populations of wild animals over the long term. On the other hand, species that avoid urban areas may be at a disadvantage as towns and cities continue to expand and suitable undeveloped habitat becomes increasingly difficult to find (Rayner et al., 2015).
To persist in urban areas, animals must tolerate human disturbance, avoid traffic and potentially adopt novel food sources (Sol et al., 2013). Urban habitat use has been linked to larger relative brain sizes across bird species (Maklakov et al., 2011; Sayol et al., 2020). Brain size is used as a proxy for cognitive abilities and, although the relationship between brain size and cognitive abilities has often been assumed without prior validation (Healy and Rowe, 2007), recent work on birds has demonstrated a link between brain size, the number of neurons in the brain, and behavioural flexibility (in the form of innovativeness; Sol et al., 2022). Behavioural flexibility could facilitate urban habitat use through, for example, increasing an animal’s ability to select atypical resources for breeding or foraging (Sol et al., 2013), or to enable categorisation of harmful and non-harmful anthropogenic objects (Lee and Thornton, 2021).
In recent decades, some bird species, such as house sparrows (Passer domesticus) and feral pigeons (Columba livia), have become closely associated with urban areas. These are often dietary generalists (Palacio, 2020), allowing them to consume anthropogenic food. Dietary generalism is positively associated with brain size as well as innovativeness, which could be an indication that behavioural flexibility enables flexibility in diet (Ducatez et al., 2015). As well as foraging in urban habitats, many species of birds use buildings for nesting. Gulls are unusual among seabirds in that several species in various parts of the world readily breed and/or forage in urban areas (e.g. Brousseau, Lefebvre and Giroux, 1996; Ludynia, Garthe and Luna-Jorquera, 2005; Auman, Meathrel and Richardson, 2008; Shaffer et al., 2017; Spelt et al., 2019). They represent a relatively recent clade, arising after the emergence of Homo sapiens, with some species diverging in the last 20,000 years (Jetz et al., 2012; Hublin et al., 2017). Therefore, gulls have potentially been influenced by human activity throughout their history, although urbanisation of habitats is a relatively modern phenomenon of the last 200 years (Ritchie and Roser, 2018).
Gull species tend to have relatively large brains for their body size (Morand-Ferron et al., 2007; Fristoe et al., 2017) and exhibit advanced cognitive skills, such as observational learning (Obozova et al., 2011), tool use (Henry and Aznar, 2006) and the use of human cues when foraging (Goumas et al., 2020; Feist et al., 2023). Gulls are also kleptoparasites (Morand-Ferron et al., 2007), and the tendency of some individuals to steal food from people, combined with widespread nesting of these large and noisy birds on roofs, is often considered problematic (Trotter, 2019).
While the cognitive abilities afforded by a large brain could be one plausible explanation for urban habitat use by gulls, such behaviour might also be explained by a species’ ecology and either work in concert with, or not necessarily require, behavioural flexibility (Dale et al., 2015). For example, buildings may resemble cliffs, which could consequently facilitate breeding by cliff-nesting species. Likewise, foraging ecology could explain gulls’ use of urban areas for feeding. Gulls as a clade are considered dietary generalists, with none showing a high degree of specialisation, and all species use marine resources (Malling Olsen, 2018). However, certain species, such as kittiwakes (Rissa; two species), have a more pelagic lifestyle (Daunt et al., 2002), requiring more time spent airborne and away from terrestrial habitats and their associated food resources. Such traits may predispose these species against urban foraging.
A species’ foraging lifestyle (or locomotory niche while foraging; Tobias et al., 2022) is related to a measure of wing pointedness called hand-wing index (HWI), with larger indices associated with a more aerial, rather than terrestrial, lifestyle (Weeks et al., 2022). Foraging lifestyle may relate to urban habitat use in two ways. Firstly, because longer, pointier wings are more efficient for long-distance flight (Sullivan et al., 2019), species that spend a lot of time flying have evolved wings with a higher HWI, and these species may spend more time out at sea than in terrestrial environments where they would otherwise encounter urban environments by chance. Secondly, a lower HWI, indicative of relatively shorter, broader wings, permits greater manoeuvrability and a more efficient take-off (Sullivan et al., 2019), which may be better suited to urban areas, where there are confined spaces and high levels of disturbance.
Like many seabirds, some gull species are of conservation concern, either at the international level (e.g. Audouin’s Gull, Larus audouinii; BirdLife International, 2020) or nationally (e.g. European Herring Gull, L. argentatus, in the UK; Stanbury et al., 2021). Understanding whether urban habitat use is related to population status (i.e. whether the species is declining and/or considered potentially vulnerable to extinction) would provide an insight into how urbanisation could be impacting this taxon. Here, we consider the factors associated with urban habitat use across all species of the gull subfamily (Larinae). These could be cognitive, with relatively larger-brained species more likely to breed and/or forage in urban areas, and ecological: cliff nesting may predispose species to urban breeding, and a more terrestrial, less aerial foraging lifestyle (for which HWI is a proxy; Weeks et al., 2022) may facilitate urban foraging. We hypothesise that brain size, cliff-nesting and terrestrial foraging ecology have a positive effect on urban habitat use. However, urban habitat use may also be opportunistic, explained by the extent to which each species’ range overlaps with urban areas, or could be an artefact of higher research effort in urban areas. As these factors may influence each other and/or have indirect effects on urban habitat use, we use path analysis to infer the most probable causal pathways. Finally, to gain a better understanding of the conservation implications of urban habitat use, we assess whether it is related to population status at the species level.
2 Methods
2.1 Predictors of urban habitat use
We collected data on urban breeding and urban foraging separately, since these distinct behaviours may be explained by different factors (Ducatez et al., 2015). We defined urban breeding as the use of buildings as nesting sites, and urban foraging as the use of built-up areas to obtain food (whether anthropogenic, i.e. produced by humans, or naturogenic, such as earthworms in parks). We used the citation databases Web of Science and Google Scholar with the following search terms: for urban breeding, ALL=(“[binomial species name]” AND (roof OR buildings OR urban) AND (breed* OR nest*)), and for urban foraging, ALL=(“[binomial species name]” AND (towns OR cities OR urban) AND (feed* OR forag*)). In cases where nomenclature has changed (for example, Larus ridibundus is now Chroicocephalus ridibundus), the search included current and previous species names in an OR statement. We checked sources to ensure that urban breeding or foraging by the focal species was reported. The search was completed on 4th October 2022.
Brain mass data were taken from Fristoe et al. (2017), with additional data for three species from Daniel Sol (pers comm). Residual brain mass was calculated using the phyl.resid function in the R package phytools (Revell, 2012), which corrects for phylogenetic relationships when regressing log brain mass against log body mass. The phylogenetic tree was obtained from Jetz et al. (2012).
To assess whether breeding ecology explains urban breeding, we grouped species into cliff-nesters and non-cliff-nesters, based on whether species were described as cliff-nesting by Olsen’s Gulls of the World (2018) and/or Cornell Lab of Ornithology (2023). For the urban foraging analysis, we assessed whether urban foraging was related to a species’ hand-wing index (HWI, as a proxy for foraging lifestyle) using data from the AVONET database (Tobias et al., 2022).
Urban habitat use could be explained by the opportunity each species has to colonise urban areas. We therefore obtained complete species range maps (“resident”, “breeding”, “non-breeding” and “passage”) from BirdLife International and Handbook of the Birds of the World (2021) and a global map of urban settlements from HILDA+ (Winkler et al., 2020). Using QGIS (QGIS Development Team, 2022), we calculated the overlap, in km2, between urban areas and (a) breeding and resident ranges, for the urban breeding analyses, and (b) all ranges, for the urban foraging analyses. From this we obtained the proportion of a species’ breeding and foraging range that encompasses urban areas (abbreviated to PBU and PFU respectively). These measurements were log-transformed to aid model fit.
Presence/absence data may be contingent on research effort (Ducatez and Lefebvre, 2014), with a considerable potential for false negatives. Our analyses therefore included, as the measure of research effort, the total number of articles returned for a given species on Web of Science and Google Scholar (completed on 7th March 2022). Web of Science and Google Scholar differ vastly in the size of their databases; therefore, instead of choosing one or the other, we used a scaled mean of both values. This value was then log-transformed.
2.2 Statistical analyses
Brain mass data were available for 30 of the 50 gull species. We therefore analysed this subset and then analysed the full sample without the brain data to assess whether the effects of the other variables remained similar at the larger sample size.
We used phylogenetic path analysis (von Hardenberg and Gonzalez-Voyer, 2013) with the phylopath (van der Bijl, 2018) package in R to conduct phylogenetically-controlled analyses assessing the effect of our predictor variables on the binary characteristics of ‘urban breeding’ and ‘urban foraging’. Phylogenetic path analysis allows alternative predictions to be made about the directionality of variables, aiding inference about causality. It allows the prediction of more than one variable simultaneously. A maximum clade credibility tree of the Lari (the suborder of which gulls are part) was taken from Jetz et al. (2012).
We used the C statistic Information Criterion corrected for small sample sizes (CICc), which is a modification of Akaike Information Criterion (AIC) designed to incorporate path analysis and can be used analogously to assess model fit. Furthermore, the C statistic has an associated p value which informs how well the path model fits the data: a significant p value (i.e. p < 0.05) would indicate a poor fit, with overlooked relationships between supplied variables (van der Bijl, 2018). As we hypothesised that each of our variables could have a biologically meaningful effect on our dependent variables and we were interested in understanding how they all related to urban habitat use, our models included all variables (depicted in Figure 1; see Supplementary Materials for lists of all models analysed). We drew inferences from the coefficients and their statistical significance in the best-supported model; see Figure 1 for hypothesised pathways between variables. In the case of research effort, it was not clear which direction the causal path might take, since proximity to urban settlements could increase research effort. Path analysis, like regular regression, cannot model feedback loops, so we ran alternative models where urban breeding/foraging was the predictor of research effort with which to compare our original models. We also included models containing relationships between predictor variables that we hypothesised may exist: a relationship between residual brain mass and the two ecological variables (cliff nesting and HWI) in addition to a direct effect of residual brain mass, and simpler models with only an indirect effect of residual brain mass via the ecological variables (see Figure 1). As with AIC, the best-supported model is that with the lowest CICc score, and models within 2 CICc points of the best-supported model should also be considered if they are not simply more complex cases of a simpler model (Arnold, 2010), provided that their C statistics have non-significant p values.
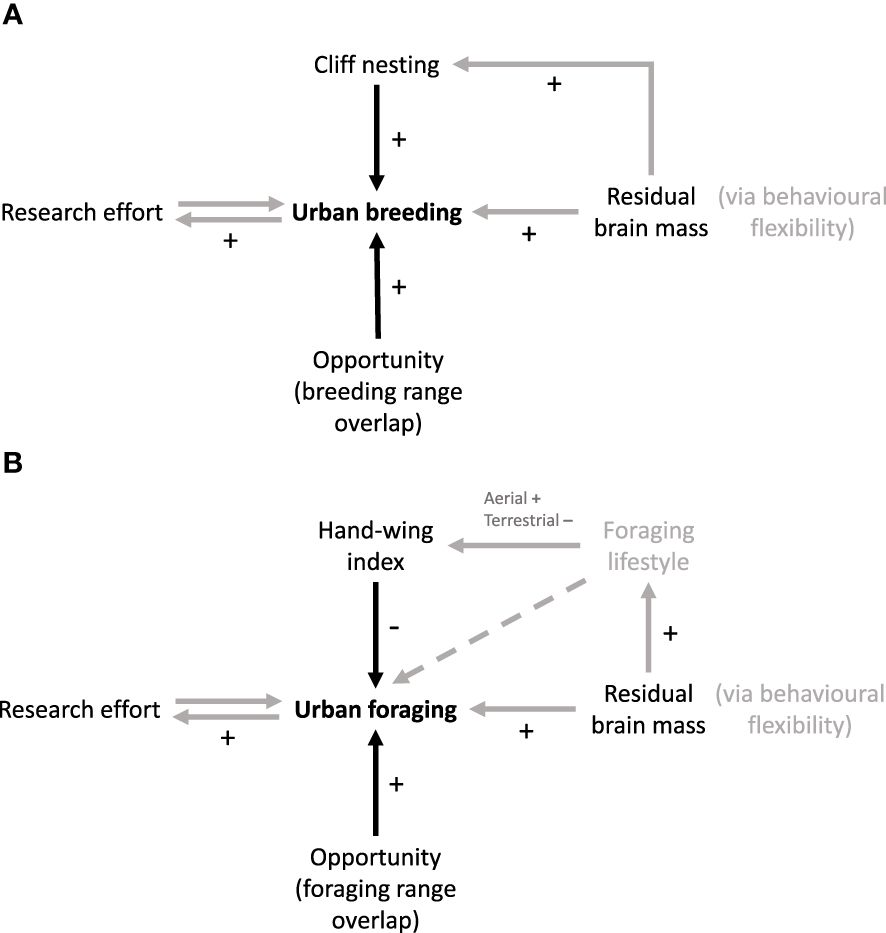
Figure 1 Directed acyclic graphs (DAGs) depicting the hypothesised relationships between variables in our models of (A) urban breeding and (B) urban foraging. Arrows indicate the direction of effects via a hypothesised causal pathway, with signs indicating whether correlations are expected to be positive or negative. Arrows in black show variables that are fixed in each model, whereas arrows in grey show alternative pathways to be tested and a dashed line indicates a relationship that is assumed but unmodelled. Labels in grey signify variables that are not directly included in our model.
2.3 Reconstructing ancestral states of cliff nesting
Because we revealed an interesting effect of cliff nesting, we decided to explore its distribution among the 50 gull species to better understand its evolutionary history. We used the ace function in the package ape (Paradis and Schliep, 2019) to calculate the likelihood of cliff nesting being present at each internal node (representing the ancestral species) on the Larinae phylogenetic tree.
2.4 Population status
Lastly, we obtained information from the International Union for Conservation of Nature (IUCN, 2023) to assess the relationship between urban habitat use and two measures of population status. We first compared the number of species for which urban habitat use has been reported (breeding and/or foraging) in species categorised as “Threatened” or “Near Threatened” with those categorised as “Least Concern”. We then compared urban habitat use in species with a “decreasing” population trend to those with a “stable” or “increasing” population trend. As we had data for each species in the gull subfamily and were not seeking to extrapolate our results to other species outside our sample, we present these results descriptively.
3 Results
Thirteen of the 50 gull species have been recorded breeding in urban areas, and 13 have been recorded foraging in urban areas. Of these, nine have been recorded both breeding and foraging in urban areas. Ten of 19 (53%) cliff-nesting gull species breed in urban settlements, whereas only three of 28 (11%) non-cliff-nesting species do so. The proportion of overlap between breeding ranges and urban areas (PBU) ranged from 0 (Red-legged Kittiwake, Rissa brevirostris) to 0.163 (Saunders’s Gull, Saundersilarus saundersi), with a median of 0.005 and a mean of 0.018. The proportion of overlap between foraging ranges and urban areas (PFU) ranged from 2.62 x 10-6 (Swallow-tailed Gull, Creagrus furcatus) to 0.213 (S. saundersi), with a median of 0.009 and a mean of 0.016.
3.1 Urban breeding
3.1.1 Gull species with residual brain mass data
Our phylogenetic path analysis indicated that the best model (weight = 0.46, p = 0.54) was one where residual brain mass predicted cliff nesting and cliff nesting predicted urban breeding (Table 1). The second-best model (weight = 0.44, p = 0.78, ΔCICc = 0.07) was identical to the best-supported model other than one additional parameter, a direct relationship between residual brain mass and urban breeding. The third-best model’s ΔCICc was 3.51 (see Supplementary Table 1 for the list of models analysed). The best-supported model estimated that the odds of cliff nesting were predicted to increase by a factor of 24 for each standard deviation increase in residual brain mass. In addition, the model estimated that the odds of urban breeding were 12.58 times higher for cliff-nesters than non-cliff-nesters. Urban breeding was also related to the proportion of overlap between breeding ranges and urban areas (PBU): the odds of urban breeding were predicted to increase by 3.08 times for each standard deviation increase in log PBU. Additionally, urban breeding was estimated to be a better predictor of research effort than vice versa; log research effort was 1.16 standard deviations higher in urban-breeding species compared to non-urban-breeding species.
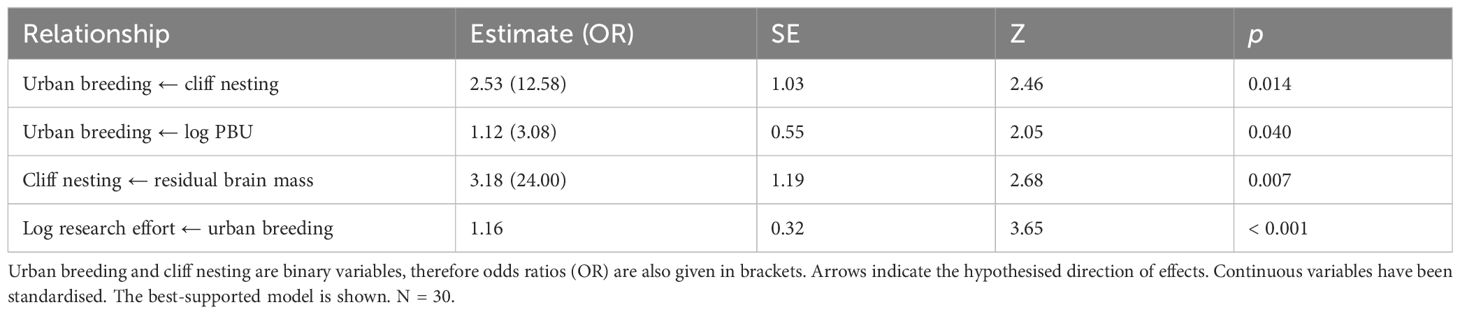
Table 1 Results of phylogenetic path analysis of the relationship between urban breeding, residual brain mass, cliff nesting, research effort, and the proportion of overlap between breeding ranges and urban areas (log PBU).
3.1.2 Urban breeding across the gull subfamily
When considering the larger sample without residual brain mass data, the model where urban breeding predicted research effort was again better supported than the model where research effort predicted urban breeding (weight = 0.99, p = 0.44, next model ΔCICc = 12.8). Cliff nesting remained a statistically significant predictor of urban breeding (Table 2). The alternative model can be viewed in Supplementary Table 2.

Table 2 Results of phylogenetic path analysis of the relationship between urban breeding, cliff nesting, research effort, and the proportion of overlap between breeding ranges and urban areas (log PBU) across the entire gull subfamily.
The best-supported model estimated that the odds of cliff-nesting species breeding in urban areas were 10.83 times higher than those of non-cliff-nesting species. The size and direction of the effects of PBU on urban breeding and of urban breeding on research effort were similar to those in the brain mass subset. Figure 2 represents the model of urban breeding implied by our analyses, showing the relationships and posited causal pathways between the tested variables.
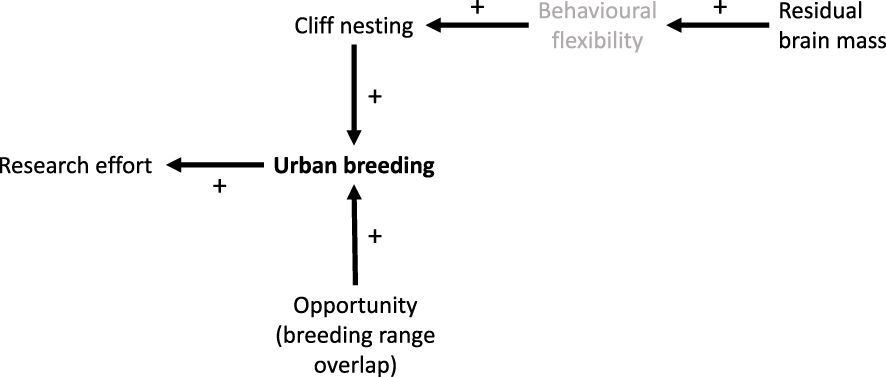
Figure 2 A representation of the best-supported model of urban breeding in the gull subfamily, as determined by phylogenetic path analysis. Arrows indicate the direction of effects, and the positive effects of all predictors are denoted by ‘+’ signs. Behavioural flexibility was not directly measured, hence it is depicted in grey.
3.1.3 The evolution of cliff nesting
We reconstructed the ancestral state of cliff nesting versus exclusive ground nesting. The estimated likelihood of cliff nesting was 0.40 at the root of the phylogenetic tree; one of the two species most distantly related to all other gulls is a present-day cliff nester (Creagrus furcatus; Figure 3). At the next split, the likelihood was estimated to be 0.35. The genus Larus has a high proportion of cliff nesters, but the estimated likelihood of their common ancestor being a cliff nester was only 0.07.
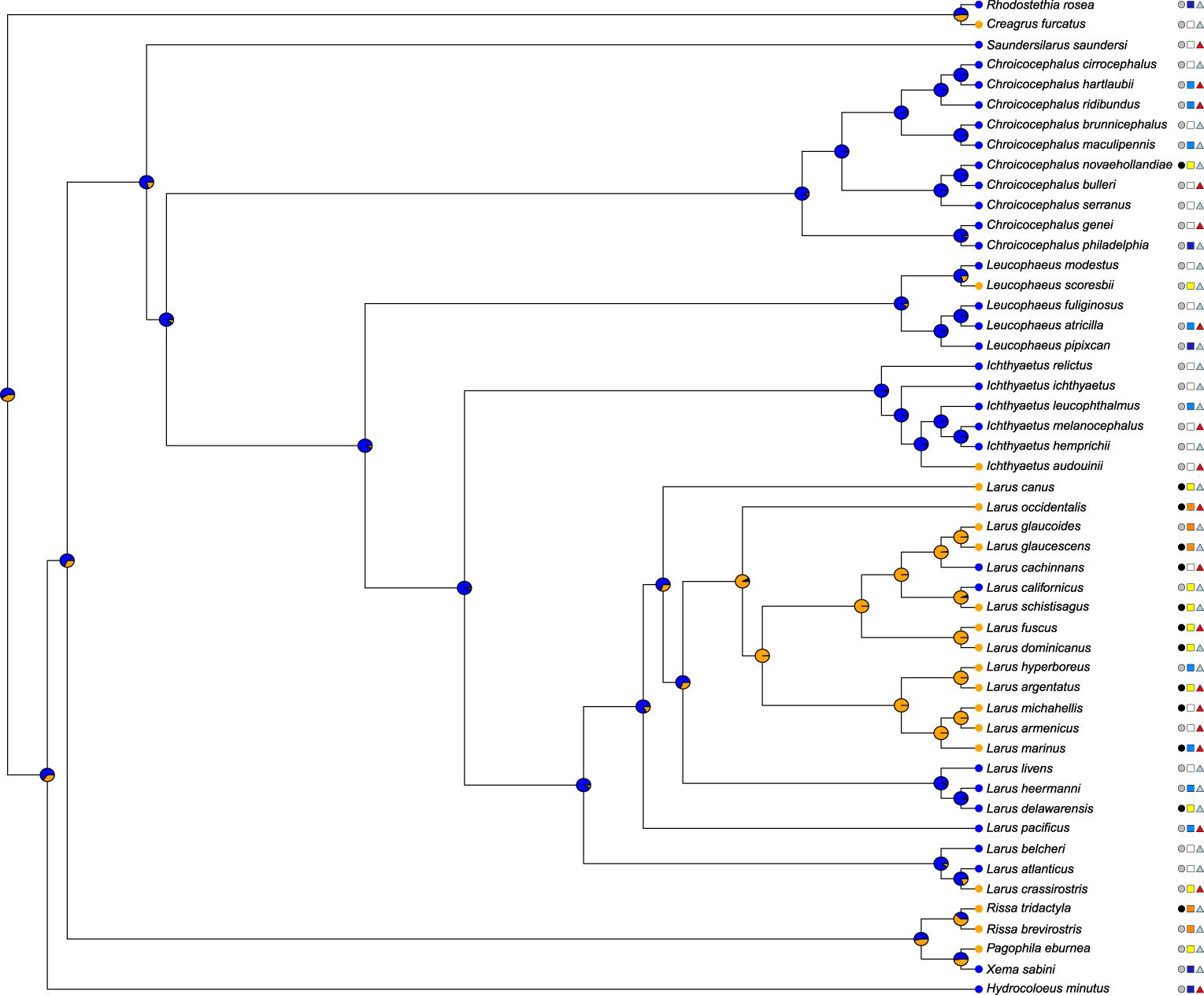
Figure 3 Phylogenetic tree of the gull subfamily, showing the maximum likelihood estimation of the ancestral state of breeding ecology (orange = cliff nesting present, blue = cliff nesting absent), with current states at the tips. Urban breeding status (black circles: urban breeding present, grey circles: urban breeding not present) and an indication of residual brain mass (dark blue squares: < -1 SD, light blue squares: -1 - 0 SD, yellow squares: 0 - 1 SD, orange squares: > 1 SD) and urban breeding range overlap (pale blue triangles: < 1% urban overlap, red triangles: ≥ 1% urban overlap) are also shown.
3.2 Urban foraging
3.2.1 Model including residual brain mass
Two candidate models were identified in the analysis of urban foraging and residual brain mass; one where urban foraging predicted research effort (best-supported, weight = 0.46, p = 0.84), and one where research effort predicted urban foraging (ΔCICc = 1.14, weight = 0.26, p = 0.75; Table 3). The next model had a ΔCICc of 3.20 (Supplementary Table 3). The relationship between residual brain mass and urban foraging in the two top models was positive but not statistically significant. Hand-wing index (HWI) had a weakly negative relationship with urban foraging, but this relationship was also non-significant. Neither of the top models featured a relationship between residual brain mass and HWI.
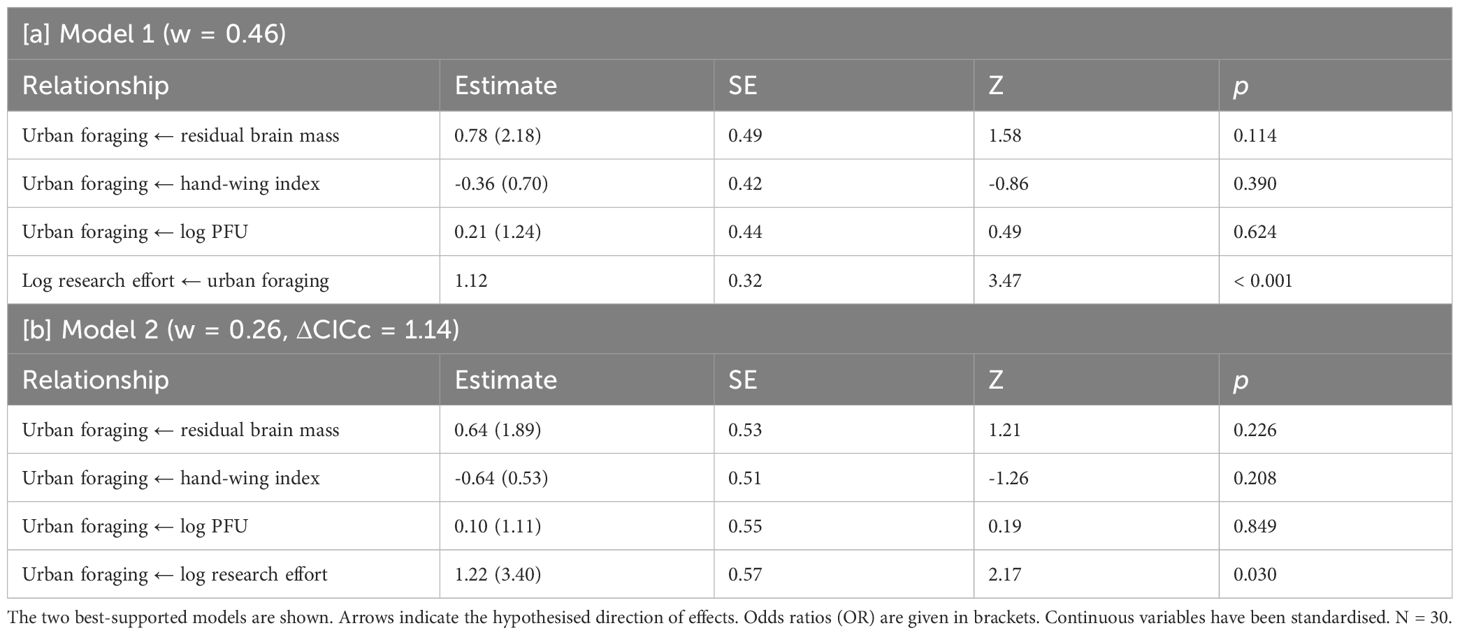
Table 3 Results of phylogenetic path analysis of the relationship between urban foraging, residual brain mass, hand-wing index, research effort, and the proportion of overlap between foraging ranges and urban areas (PFU).
3.2.2 Urban foraging across the gull subfamily
Our urban foraging analysis of all 50 species of gull showed that the only statistically significant relationship was between urban foraging and research effort, but it was unable to distinguish between the direction of effects. The best-supported model (weight = 0.72, p = 0.22) indicated that urban foraging was predicted by research effort, while the other model (ΔCICc = 1.90, weight = 0.28, p = 0.12) placed urban foraging as a predictor of research effort (Table 4). The best-supported model indicated that, although HWI was negatively associated with urban foraging, with the odds of urban foraging predicted to decrease by a factor of 0.43 for each standard deviation increase in HWI, this relationship was not statistically significant (Table 4A). Furthermore, the effect of this non-significant relationship was negligible in the alternative model (Table 4B).
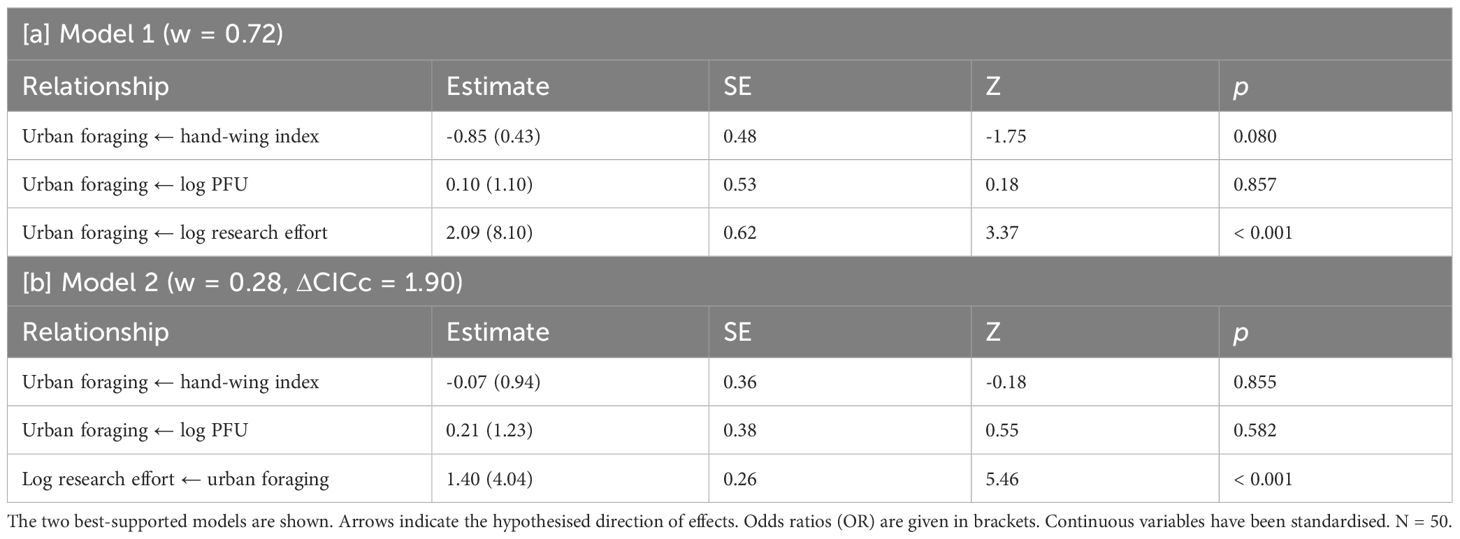
Table 4 Results of phylogenetic path analysis of the relationship between urban foraging, hand-wing index, research effort, and the proportion of overlap between foraging ranges and urban areas (PFU) when considering the entire gull subfamily.
3.3 The relationship between urban habitat use and population status
Ten of the 50 gull species are listed by IUCN as Threatened (six species) or Near Threatened (four species). Of these, only one (Black-legged Kittiwake, Rissa tridactyla) is known to use urban habitats, whereas the other 16 species known to use urban habitats are listed as Least Concern. This means that gulls with a Least Concern conservation status are four times more likely to have been recorded using urban areas for breeding or foraging than those that are listed as Threatened or Near Threatened (Table 5). In addition, eleven species (six of which are Threatened or Near Threatened) are listed as decreasing in population. Of these, two (European Herring Gull and Black-legged Kittiwake) use urban habitats. Excluding those with an “unknown” population trend (N = 11), species whose global populations are stable or increasing are more than twice (2.16 times) as likely to have been recorded using urban habitats than those that are decreasing.
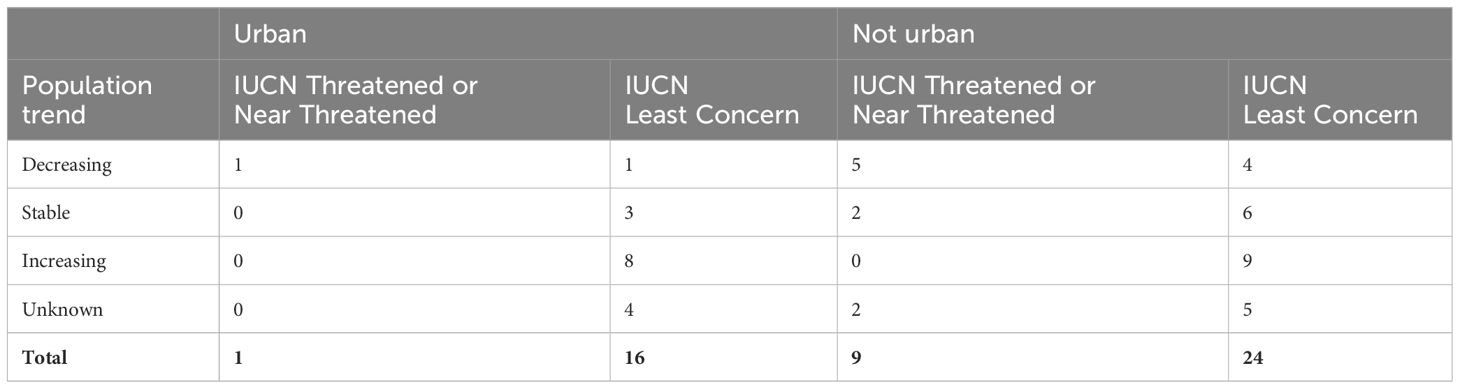
Table 5 Urban habitat use, global population status and population trend for the 50 species in the gull subfamily, as listed by the International Union for Conservation of Nature (IUCN).
4 Discussion
Understanding the traits that cause species to use urban areas, and the potential effects of urban habitat use on wildlife population metrics, has important implications for urban planning, human-wildlife conflict mitigation and conservation actions. We conducted a comparative analysis to identify some of the factors that facilitate urban breeding and foraging among the gull subfamily, and assessed whether urban habitat use is related to their population status. We found that breeding ecology and the opportunity to colonise urban areas were important factors explaining urban breeding, but urban foraging was more difficult to explain.
We found that gull species with relatively larger brains appear to be more likely to exploit urban areas for breeding, which is consistent with previous avian comparative analyses (Maklakov et al., 2011; Sayol et al., 2020). However, this relationship is not direct and is mediated by breeding ecology. The strong relationship between residual brain mass and cliff nesting suggests that breeding habitat choice could be a result of cognitive factors. Our analysis of ancestral states revealed that cliff nesting is likely to be a derived trait in gulls. One potential hypothesis is that behavioural flexibility, afforded by a relatively larger brain, allowed the use of novel breeding habitats. Interestingly, few gull species nest exclusively on cliffs (Smith, 1966; Burger, 1974), indicating that there is flexibility in the choice of breeding habitat type within species. Our model of the relationship between cliff nesting and urban breeding across the gull subfamily supported the existence of a strong relationship between these two traits. It is plausible that buildings may not only be chosen by gulls for their functional similarity to cliffs, but as a result of behavioural flexibility inherent in the cliff-nesting species. Obtaining brain size estimates for the remaining gull species and quantifying the variation in breeding habitat choice at a finer scale would provide a test of this hypothesis. Furthermore, many other species breed in urban areas, and it would be pertinent to investigate whether a relationship between urban breeding and habitat flexibility is evident more widely.
It is, however, important to note that residual brain mass may not necessarily equate to cognitive ability (Schoenemann et al., 2000; Deaner et al., 2007). Brain size is used as a convenient proxy for behavioural flexibility, but the causal pathway between brain size and behavioural flexibility is not simple (Logan et al., 2018). Moreover, brain size estimates are based on small sample sizes for each species, and species estimates can vary widely, creating uncertainty in the results of comparative analyses (Hooper et al., 2022). Cognitive tests, some of which have already shown behavioural flexibility in a small number of relatively large-brained gull species (Obozova et al., 2011; Lamarre and Wilson, 2021; Inzani et al., 2023), could provide a greater insight into cognitive abilities, but would need to be implemented more widely across the subfamily. Cognitive tests would also need to be directly relevant to the questions at hand. Responses to humans, anthropogenic objects and novelty can be expected to affect successful exploitation of urban areas (Lee and Thornton, 2021). A high degree of behavioural flexibility, potentially facilitated by enhanced memory and discriminative abilities, is likely to allow species to cope with urban challenges more effectively.
We did not find a significant relationship between brain size and urban foraging. This might be because urban foraging does not necessarily require behavioural flexibility, because our brain mass data do not adequately explain behavioural flexibility in foraging, or perhaps because urban foraging has been underreported. Although the relationship between urban foraging and hand-wing index (HWI, or wing pointedness) was negative as expected, the evidence that gulls with a high HWI were less likely to use urban areas for foraging was weak. Wing shape therefore does not appear to constrain urban foraging by gulls. Relatedly, if foraging lifestyle is indeed closely linked to HWI, and HWI is reflective of the type of habitat frequented by species and the type of diet consumed, there is little evidence to suggest it has a role in promoting the use of urban areas for feeding. This may not be surprising considering that gulls are relatively similar in their foraging niche: as a group they are considered dietary generalists and the majority of species have a terrestrial foraging component (AVONET database, Tobias et al., 2022). Data on the finer details of each species’ dietary breadth are, to our knowledge, not currently available, but might provide a better understanding of the causes of urban foraging in gulls. HWI may also not be a perfect proxy for foraging lifestyle, and it is sometimes used instead as a proxy for dispersal ability (e.g. Weeks et al., 2023). An analysis examining the effect of HWI, framed as dispersal ability, on the degree of urban habitat use in a larger sample of birds found a slightly positive relationship between these two variables (Neate-Clegg et al., 2023). These differing results perhaps expose the limitations of using proxies and indicate the need for a well-formulated hypothesis of how physical traits could affect habitat use.
Research effort was consistently an important variable in our models of urban breeding and foraging. In some models, a pathway from urban habitat use to research effort was predicted, while in others, research effort better predicted urban habitat use. The direction of causality is, however, likely to be bidirectional: while research effort may increase the reporting of instances of urban habitat use, urban habitat use entails close proximity to humans and therefore ease of access for study, which could result in greater research effort. This is probably why our models, which cannot incorporate bidirectionality, were not always consistent in predicting the direction of effects. The idea that urban habitat use could explain research effort has been suggested before in a study where urban mammals were found to have more documented parasites than non-urban species (Albery et al., 2022). Our results highlight the importance of considering how research effort relates to biological effects and the utility of measuring this variable for revealing species in need of greater study.
Finally, we considered the population status of urban and non-urban gull species. We found that species that were of international conservation concern or decreasing in population size were less likely to have been recorded using urban habitats. These figures contrast with another study assessing the relationship between national conservation status and urban habitat use in a wider variety of animal and plant taxa in Australia (Ives et al., 2016). There may be divergent effects on different taxa in different geographical areas, and the stage of urbanisation and cause of the population declines could be important. However, it should be recognised that, while species populations are routinely monitored, these categories do not necessarily capture the true population status of a species. Some gull species are not of conservation concern internationally, as recognised by IUCN, but are of conservation concern nationally. This is the case for the European Herring Gull, which is on the Red list of Birds of Conservation Concern in the United Kingdom because of its population declines (Stanbury et al., 2021). Other species may have received less attention and not be adequately monitored at a local level. Furthermore, some species classed as Least Concern may not have been recently or fully assessed, as indicated by nine Least Concern gull species having an “unknown” population trend. As an example, recent research has recommended that the Great Black-backed Gull (whose population trend is presently listed by IUCN as “unknown”) should be classed as Vulnerable (Lopez et al., 2023). It is likely that other species have received even less attention than this widespread, urbanised species, and are facing similar, but undocumented, population declines. Therefore these categorisations are an imperfect measure of a species’ population status. Nevertheless, it is important to recognise the implications of a relationship between population status and urban habitat use. If this relationship is still evident with better data, it raises the question why. Are species that can tolerate urban areas also more resilient to other changes in the environment, perhaps because they have a wider dietary breadth and/or consider a range of breeding sites (as suggested by, e.g., Sol et al., 2005)? Or could urban areas be acting as a refuge, buffering individuals from detrimental changes in their original and increasingly degraded habitats? Is this pattern observed in other taxa, and is there any evidence for a causal effect?
Our study adds to a growing literature on urban birds, but comparative studies seeking to understand how other taxonomic groups, such as mammals and reptiles, respond to urbanisation are greatly lacking (Lee and Thornton, 2021). It is probable that some of the factors that explain urban habitat use will be generalisable across taxa, but there could be substantial differences. These differences may also determine whether urban areas are positive or negative for individual species, and whether they act as a safe place or an ecological trap, attracting animals to their peril (Zuñiga-Palacios et al., 2021). For example, mesocarnivores such as coyotes and bobcats avoid large carnivores like wolves and cougars, and tend to prefer to reside close to human settlements since these apex predators avoid human activity; however, a study found that far more mesocarnivores were killed intentionally by humans than by large carnivores (Prugh et al., 2023). This is an instance of urbanised areas being an ecological trap, and, for some species, persecution by humans may outweigh any positive effects of urban living. In many cases, the habitat loss caused by urban expansion will have a detrimental impact on species (Simkin et al., 2022), but, for some, suitable habitat will be created (Lowry et al., 2013).
Presently, there is relatively little information on urban habitat use within gull species, although the body of research is steadily growing (e.g. Pais de Faria et al., 2021; Dalla Pria et al., 2022; Langley et al., 2023). Therefore, our analysis focused on the presence and absence of urban breeding and foraging at the species level. The extent to which each species uses urban habitats and the related topography would undoubtedly reveal finer-scale information on the factors underlying these behaviours. Tracking studies have shown that, despite gulls being generalists at the species or population level, this is largely underpinned by individual specialisation for certain diets and habitat types, and therefore gulls’ selection of urban habitats may be a means of lessening intraspecific competition (Masello et al., 2013; Navarro et al., 2017; Shaffer et al., 2017; Maynard and Ronconi, 2018; Jordan et al., 2021). There are likely to be key differences among individuals or subpopulations that will help in identifying the causes, and the effects, whether positive, negative or neutral, of urban habitat use. This will be invaluable to our understanding of urban ecology, but will require greater research effort.
Data availability statement
The original contributions presented in the study are included in the article/Supplementary Material. Further inquiries can be directed to the corresponding author.
Author contributions
MG: Conceptualization, Data curation, Formal analysis, Investigation, Methodology, Project administration, Visualization, Writing – original draft, Writing – review & editing. CB: Conceptualization, Data curation, Investigation, Methodology, Writing – review & editing. CR: Data curation, Writing – review & editing. NB: Conceptualization, Funding acquisition, Supervision, Writing – review & editing.
Funding
The author(s) declare financial support was received for the research, authorship, and/or publication of this article. This work was funded by a Royal Society Research Fellow Enhanced Research Expenses Grant (RF\ERE\210119) awarded to NB.
Conflict of interest
The authors declare that the research was conducted in the absence of any commercial or financial relationships that could be construed as a potential conflict of interest.
Publisher’s note
All claims expressed in this article are solely those of the authors and do not necessarily represent those of their affiliated organizations, or those of the publisher, the editors and the reviewers. Any product that may be evaluated in this article, or claim that may be made by its manufacturer, is not guaranteed or endorsed by the publisher.
Supplementary material
The Supplementary Material for this article can be found online at: https://www.frontiersin.org/articles/10.3389/fevo.2024.1256911/full#supplementary-material
References
Albery G. F., Carlson C. J., Cohen L. E., Eskew E. A., Gibb R., Ryan S. J., et al. (2022). Urban-adapted mammal species have more known pathogens. Nat. Ecol. Evol. 6, 794–801. doi: 10.1038/s41559-022-01723-0
Arnold T. W. (2010). Uninformative parameters and model selection using akaike’s information criterion. J. Wildlife Manage. 74, 1175–1178. doi: 10.1111/j.1937-2817.2010.tb01236.x
Auman H. J., Meathrel C. E., Richardson A. (2008). Supersize Me: Does Anthropogenic Food Change the Body Condition of Silver Gulls? A Comparison Between Urbanized and Remote, Non-urbanized Areas. Waterbirds 31, 122–126. doi: 10.1675/1524-4695(2008)31[122:smdafc]2.0.co;2
Barrett L. P., Stanton L. A., Benson-Amram S. (2019). The cognition of “nuisance” species. Anim. Behav. 147, 167–177. doi: 10.1016/j.anbehav.2018.05.005
Bateman P. W., Fleming P. A. (2012). Big city life: Carnivores in urban environments. J. Zoology 287, 1–23. doi: 10.1111/j.1469-7998.2011.00887.x
BirdLife International (2020) IUCN Red List of Threatened Species: Larus audouinii. In: IUCN Red List of Threatened Species. Available at: https://www.iucnredlist.org/en (Accessed 16 June 2023).
BirdLife International and Handbook of the Birds of the World (2021). Bird species distribution maps of the world. Version 2021.1. (BirdLife International). Available at: http://datazone.birdlife.org/species/requestdis.
Brousseau P., Lefebvre J., Giroux J.-F. (1996). Diet of Ring-Billed Gull Chicks in Urban and Non-Urban Colonies in Quebec. Colonial Waterbirds 19, 22–30. doi: 10.2307/1521803
Burger J. (1974). Breeding adaptations of Franklin’s gull (larus pipixcan) to a marsh habitat. Anim. Behav. 22, 521–567. doi: 10.1016/S0003-3472(74)80001-1
Chace J. F., Walsh J. J. (2006). Urban effects on native avifauna: a review. Landscape Urban Plann. 74, 46–69. doi: 10.1016/j.landurbplan.2004.08.007
Cornell Lab of Ornithology (2023). Birds of the World - Comprehensive life histories for all bird species and families. Available at: https://birdsoftheworld.org/bow/home (Accessed 17 February 2023).
Dale S., Lifjeld J. T., Rowe M. (2015). Commonness and ecology, but not bigger brains, predict urban living in birds. BMC Ecol. 15, 12. doi: 10.1186/s12898-015-0044-x
Dalla Pria C., Cawkwell F., Newton S., Holloway P. (2022). City living: nest-site selection preferences in urban herring gulls, larus argentatus. Geographies 2, 161–172. doi: 10.3390/geographies2020011
Daunt F., Benvenuti S., Harris M. P., Dall1Antonia L., Elston D. A., Wanless S. (2002). Foraging strategies of the black-legged kittiwake Rissa tridactyla at a North Sea colony: evidence for a maximum foraging range. Mar. Ecol. Prog. Ser. 245, 239–247. doi: 10.3354/meps245239
Deaner R. O., Isler K., Burkart J., van Schaik C. (2007). Overall brain size, and not encephalization quotient, best predicts cognitive ability across non-human primates. Brain Behav. Evol. 70, 115–124. doi: 10.1159/000102973
Ducatez S., Clavel J., Lefebvre L. (2015). Ecological generalism and behavioural innovation in birds: technical intelligence or the simple incorporation of new foods? J. Anim. Ecol. 84, 79–89. doi: 10.1111/1365-2656.12255
Ducatez S., Lefebvre L. (2014). Patterns of research effort in birds. PloS One 9, e89955. doi: 10.1371/journal.pone.0089955
Feist F., Smith K., Graham P. (2023). Inter-species stimulus enhancement: herring gulls (Larus argentatus) mimic human food choice during foraging. Biol. Lett. 19, 20230035. doi: 10.1098/rsbl.2023.0035
Fristoe T. S., Iwaniuk A. N., Botero C. A. (2017). Big brains stabilize populations and facilitate colonization of variable habitats in birds. Nat. Ecol. Evol. 1, 1706–1715. doi: 10.1038/s41559-017-0316-2
Glista D. J., DeVault T. L., DeWoody J. A. (2008). Vertebrate road mortality predominantly impacts amphibians. Herpetological Conserv. Biol. 3, 77–87.
Goumas M., Boogert N. J., Kelley L. A. (2020). Urban herring gulls use human behavioural cues to locate food. R. Soc. Open Sci. 7. doi: 10.1098/rsos.191959
Healy S. D., Rowe C. (2007). A critique of comparative studies of brain size. Proc. R. Soc. B: Biol. Sci. 274, 453–464. doi: 10.1098/rspb.2006.3748
Henry P.-Y., Aznar J.-C. (2006). Tool-use in charadrii: active bait-fishing by a herring gull. Waterbirds 29, 233–234. doi: 10.1675/1524-4695(2006)29[233:TICABB]2.0.CO;2
Hooper R., Brett B., Thornton A. (2022). Problems with using comparative analyses of avian brain size to test hypotheses of cognitive evolution. PloS One 17, e0270771. doi: 10.1371/journal.pone.0270771
Hublin J.-J., Ben-Ncer A., Bailey S. E., Freidline S. E., Neubauer S., Skinner M. M., et al. (2017). New fossils from Jebel Irhoud, Morocco and the pan-African origin of Homo sapiens. Nature 546, 289–292. doi: 10.1038/nature22336
Inzani E. L., Kelley L. A., Boogert N. J. (2023). Object neophilia in wild herring gulls in urban and rural locations. J. Avian Biol. 2023, e03028. doi: 10.1111/jav.03028
IUCN (2023). IUCN Red List, IUCN Red List of Threatened Species. Available at: https://www.iucnredlist.org/en (Accessed 24 February 2023).
Ives C. D., Lentini P. E., Threlfall C. G., Ikin K., Shanahan D. F., Garrard G. E., et al. (2016). Cities are hotspots for threatened species. Global Ecol. Biogeography 25, 117–126. doi: 10.1111/geb.12404
Jetz W., Thomas G. H., Joy J. B., Hartmann K., Mooers A. O. (2012). The global diversity of birds in space and time. Nature 491, 444–448. doi: 10.1038/nature11631
Jordan D. W., Gehring T. M., Seefelt N. E., Alm E. W. (2021). Use of great lakes recreational beaches and human-waste sites by ring-billed gulls. Northeastern Nat. 28, 127–136. doi: 10.1656/045.028.0202
Lamarre J., Wilson D. R. (2021). Waterbird solves the string-pull test. R. Soc. Open Sci. 8, 211343. doi: 10.1098/rsos.211343
Langley L. P., Bearhop S., Burton N. H.K., Banks A. N., Frayling T., Thaxter C. B., et al. (2023). Urban and coastal breeding lesser black-backed gulls (Larus fuscus) segregate by foraging habitat. Ibis 165, 214–230. doi: 10.1111/ibi.13109
Lee V. E., Thornton A. (2021). Animal cognition in an urbanised world. Front. Ecol. Evol. 9. doi: 10.3389/fevo.2021.633947
Logan C. J., Avin S., Boogert N., Buskell A., Cross F. R., Currie A., et al. (2018). Beyond brain size: Uncovering the neural correlates of behavioral and cognitive specialization. Comp. Cogn. Behav. Rev. 13, 55–89. doi: 10.3819/ccbr.2018
Lopez S. L., Bond A. L., O’Hanlon N. J., Wilson J. M., Vitz A., Mostello C. S., et al. (2023). Global population and conservation status of the Great Black-backed Gull Larus marinus. Bird Conserv. Int. 33, e23. doi: 10.1017/S0959270922000181
Loss S. R., Will T., Loss S. S., Marra P. P. (2014). Bird–building collisions in the United States: Estimates of annual mortality and species vulnerability. Condor 116, 8–23. doi: 10.1650/CONDOR-13-090.1
Lowry H., Lill A., Wong B. B. M. (2013). Behavioural responses of wildlife to urban environments. Biol. Rev. 88, 537–549. doi: 10.1111/brv.12012
Ludynia K., Garthe S., Luna-Jorquera G. (2005). Seasonal and Regional Variation in the Diet of the Kelp Gull in Northern Chile. Waterbirds 28, 359–365. doi: 10.1675/1524-4695(2005)028[0359:SARVIT]2.0.CO;2
Lyytimäki J., Petersen L. K., Normander B., Bezák P. (2008). Nature as a nuisance? Ecosystem services and disservices to urban lifestyle. Environ. Sci. 5, 161–172. doi: 10.1080/15693430802055524
Maklakov A. A., Immler S., Gonzalez-Voyer A., Rönn J., Kolm N. (2011). Brains and the city: big-brained passerine birds succeed in urban environments. Biol. Lett. 7, 730–732. doi: 10.1098/rsbl.2011.0341
Masello J. F., Wikelski M., Voigt C. C., Quillfeldt P. (2013). Distribution patterns predict individual specialization in the diet of dolphin gulls. PloS One 8, e67714. doi: 10.1371/journal.pone.0067714
Maynard L. D., Ronconi R. A. (2018). Foraging behaviour of great black-backed gulls larus marinus near an urban centre in atlantic Canada: evidence of individual specialization from GPS tracking. Mar. Ornithology 46, 27–32.
McKinney M. L. (2006). Urbanization as a major cause of biotic homogenization. Biol. Conserv. 127, 247–260. doi: 10.1016/j.biocon.2005.09.005
Morand-Ferron J., Sol D., Lefebvre L. (2007). Food stealing in birds: brain or brawn? Anim. Behav. 74, 1725–1734. doi: 10.1016/j.anbehav.2007.04.031
Murray M. H., Sánchez C. A., Becker D. J., Byers K. A., Worsley-Tonks K. E., Craft M. E. (2019). City sicker? A meta-analysis of wildlife health and urbanization. Front. Ecol. Environ. 17, 575–583. doi: 10.1002/fee.2126
Navarro J., Grémillet D., Ramirez F. J., Afán I., Bouten W., Forero M. G. (2017). Shifting individual habitat specialization of a successful predator living in anthropogenic landscapes. Mar. Ecol. Prog. Ser. 578, 243–251. doi: 10.3354/meps12124
Neate-Clegg M. H. C., Tonelli B. A., Youngflesh C., Wu J. X., Montgomery G. A., Şekercioğlu Ç. H., et al. (2023). Traits shaping urban tolerance in birds differ around the world. Curr. Biol. 33, 1677–1688. doi: 10.1016/j.cub.2023.03.024
Obozova T. A., Smirnova A. A., Zorina Z. A. (2011). Observational learning in a glaucous-winged gull natural colony. Int. J. Comp. Psychol. 24, 226–234. doi: 10.46867/IJCP.2011.24.02.02
Pais de Faria J., Paiva V. H., Veríssimo S., Gonçalves A. M.M., Ramos J. A. (2021). Seasonal variation in habitat use, daily routines and interactions with humans by urban-dwelling gulls. Urban Ecosyst. 24, 1101–1115. doi: 10.1007/s11252-021-01101-x
Palacio F. X. (2020). Urban exploiters have broader dietary niches than urban avoiders. Ibis 162, 42–49. doi: 10.1111/ibi.12732
Paradis E., Schliep K. (2019). A’pe 5.0: an environment for modern phylogenetics and evolutionary analyses in R. Bioinformatics 35, 526–528. doi: 10.1093/bioinformatics/bty633
Prugh L. R., Cunningham C. X., Windell R. M., Kertson B. N., Ganz T. R., Walker S. L., et al. (2023). Fear of large carnivores amplifies human-caused mortality for mesopredators. Science 380, 754–758. doi: 10.1126/science.adf2472
QGIS Development Team (2022). QGIS Geographic Information System. Available at: http://qgis.osgeo.org.
Rayner L., Ikin K., Evans M. J., Gibbons P., Lindenmayer D. B., Manning A. D. (2015). Avifauna and urban encroachment in time and space. Diversity Distributions 21, 428–440. doi: 10.1111/ddi.12293
Revell L. J. (2012). phytools: An R package for phylogenetic comparative biology (and other things). Methods Ecol. Evol. 3, 217–223. doi: 10.1111/j.2041-210X.2011.00169.x
Ritchie H., Roser M. (2018). Urbanization. In: Our World in Data. Available at: https://ourworldindata.org/urbanization (Accessed 17 February 2023).
Sayol F., Sol D., Pigot A. L. (2020). Brain size and life history interact to predict urban tolerance in birds. Front. Ecol. Evol. 8. doi: 10.3389/fevo.2020.00058
Schoenemann P. T., Budinger T. F., Sarich V. M., Wang W. S.-Y. (2000). Brain size does not predict general cognitive ability within families. Proc. Natl. Acad. Sci. 97, 4932–4937. doi: 10.1073/pnas.97.9.4932
Shaffer S. A., Cockerham S., Warzybok P., Bradley R. W., Jahncke J., Clatterbuck C. A., et al. (2017). Population-level plasticity in foraging behavior of western gulls (Larus occidentalis). Movement Ecol. 5, 27. doi: 10.1186/s40462-017-0118-9
Simkin R. D., Seto K. C., McDonald R. I., Jetz W. (2022). Biodiversity impacts and conservation implications of urban land expansion projected to 2050. Proc. Natl. Acad. Sci. 119, e2117297119. doi: 10.1073/pnas.2117297119
Smith N. G. (1966). Adaptations to cliff-nesting in some arctic gulls (larus). Ibis 108, 68–83. doi: 10.1111/j.1474-919X.1966.tb07252.x
Sol D., Duncan R. P., Blackburn T. M., Cassey P., Lefebvre L. (2005). Big brains, enhanced cognition, and response of birds to novel environments. Proc. Natl. Acad. Sci. 102, 5460–5465. doi: 10.1073/pnas.0408145102
Sol D., Olkowicz S., Sayol F., Kocourek M., Zhang Y., Marhounová L., et al. (2022). Neuron numbers link innovativeness with both absolute and relative brain size in birds. Nat. Ecol. Evol. 6, 1381–1389. doi: 10.1038/s41559-022-01815-x
Sol D., Lapiedra O., González-Lagos C. (2013). Behavioural adjustments for a life in the city. Anim. Behav. 85, 1101–1112. doi: 10.1016/j.anbehav.2013.01.023
Spelt A., Williamson C., Shamoun-Baranes J., Shepard E., Rock P., Windsor S. (2019). Habitat use of urban-nesting lesser black-backed gulls during the breeding season. Sci Rep 9, 10527. doi: 10.1038/s41598-019-46890-6
Stanbury A., Eaton M., Aebischer N., Balmer D., Brown A., Douse A., et al. (2021). The status of our bird populations: the fifth Birds of Conservation Concern in the United Kingdom, Channel Islands and Isle of Man and second IUCN Red List assessment of extinction risk for Great Britain. Br. Birds 114, 723–747. Available at: https://www.bto.org/our-science/publications/birds-conservation-concern/status-our-bird-populations-fifth-birds
Sullivan T. N., Meyers M. A., Arzt E. (2019). Scaling of bird wings and feathers for efficient flight. Sci. Adv. 5, eaat4269. doi: 10.1126/sciadv.aat4269
Tobias J. A., Sheard C., Pigot A. L., Devenish A. J.M., Yang J., Sayol F., et al. (2022). AVONET: morphological, ecological and geographical data for all birds. Ecol. Lett. 25, 581–597. doi: 10.1111/ele.13898
Trotter S. (2019). The regulation of urban gulls in the UK: a study of control measures. Br. Birds 112, 282–292.
van der Bijl W. (2018). phylopath: Easy phylogenetic path analysis in R. PeerJ 6, e4718. doi: 10.7717/peerj.4718
von Hardenberg A., Gonzalez-Voyer A. (2013). Disentangling evolutionary cause-effect relationships with phylogenetic confirmatory path analysis. Evolution 67, 378–387. doi: 10.1111/evo.2013.67.issue-2
Weeks B. C., O’Brien B. K., Chu J. J., Claramunt S., Sheard C., Tobias J. A. (2022). Morphological adaptations linked to flight efficiency and aerial lifestyle determine natal dispersal distance in birds. Funct. Ecol. 36, 1681–1689. doi: 10.1111/1365-2435.14056
Weeks T. L., Betts M. G., Pfeifer M., Wolf C., Banks-Leite C., Barbaro L., et al. (2023). Climate-driven variation in dispersal ability predicts responses to forest fragmentation in birds. Nat. Ecol. Evol., 1–13. doi: 10.1038/s41559-023-02077-x
Winkler K., Fuchs R., Rounsevell M. D.A., Herold M. (2020). HILDA+ Global Land Use Change between 1960 and 2019 (PANGAEA). doi: 10.1594/PANGAEA.921846
Keywords: gulls, behavioural flexibility, urban habitat use, breeding ecology, foraging ecology
Citation: Goumas M, Berkin CR, Rayner CW and Boogert NJ (2024) From the sea to the city: explaining gulls’ use of urban habitats. Front. Ecol. Evol. 12:1256911. doi: 10.3389/fevo.2024.1256911
Received: 11 July 2023; Accepted: 05 April 2024;
Published: 25 April 2024.
Edited by:
Yanina Benedetti, Czech University of Life Sciences Prague, CzechiaReviewed by:
Simon Ducatez, University of Cambridge, United KingdomTom Gehring, Central Michigan University, United States
Copyright © 2024 Goumas, Berkin, Rayner and Boogert. This is an open-access article distributed under the terms of the Creative Commons Attribution License (CC BY). The use, distribution or reproduction in other forums is permitted, provided the original author(s) and the copyright owner(s) are credited and that the original publication in this journal is cited, in accordance with accepted academic practice. No use, distribution or reproduction is permitted which does not comply with these terms.
*Correspondence: Madeleine Goumas, bS5nb3VtYXNAZXhldGVyLmFjLnVr