- Department of Geology and Geophysics, Texas A&M University, College Station, TX, United States
Mangroves create unique and highly productive wetland communities in intertidal zones of tropical and subtropical coastlines. Despite their many ecosystem services, such as carbon sequestration, mangroves remain threatened by climate change, sea-level rise, and human development. The inclusion of conservation paleobiology and long-term perspectives on how these ecosystems have responded to past environmental change can inform current policy and lead to more effective conservation and restoration management strategies for modern mangrove communities. In South Florida, humified plant debris, or peat, in mangroves provides this historical record. Our research takes a novel paleobiological approach by using plant organ- and taxon-based measures to describe the influence of the taphonomically active zone (TAZ: the zone near the surface of the substrate where taphonomic processes actively formation and degrade accumulated detritus) on the decomposition of mangrove peat with depth. This allows us to understand the taphonomic biases imposed on mangrove peat as it is sequestered into the sedimentological record and provides us with the paleoecological context to better interpret preserved peats and reconstruct past mangrove sub-habitats from peat cores. Accordingly, we collected modern surficial peat cores from two contrasting mangrove sub-habitats in Barnes Sound, FL. These surficial cores were characterized and compared to historical, deep cores from other South Florida mangrove peat deposits. By comparing the proportional abundance of mangrove peat constituents in these samples, we established modern analogs needed to interpret changes in the depositional environment of historical mangrove peats found in sediment cores, which is critical for understanding shoreline responses of mangroves to sea-level rise and anthropogenic change. We demonstrate that (1) leaf mat thickness may be a relative indicator of surficial peat decomposition rates because it correlates with the degree of tidal activity and detritivore access to the leaf litter layer; (2) root percentages are valid tools to differentiate between peats at depth, and can be used as relative indicators for the distance of in situ peat from shorelines; and (3) organismal signals, such as foraminifera and insect parts, provide a means for deciphering precursor mangrove sub-habitats from sequestered peats.
Introduction
The taphonomically active zone (TAZ) is the zone at the surface of the substrate where physical, chemical, and microbial processes facilitate the breakdown and decomposition of accumulated detritus (Davies et al., 1989). Paleobiologists model the TAZ in marine depositional environments to describe the fossilization process of benthic fauna at the sediment–water interface (Davies et al., 1989; Sadler, 1993; Olszewski, 1999, 2004; Walker and Goldstein, 1999; Tomašových et al., 2014, 2019). Further, the TAZ has been extended to include the dissolution of fauna through the pelagic zone (Petró et al., 2018). In the terrestrial realm, Noto (2011) modeled the TAZ and discussed its implications for vertebrate paleobiology. In this study, we introduce a novel paleobotanical approach and characterize the TAZ in mangrove environments to better understand how taphonomic processes shape accumulated plant litter as it is humified into peat and sequestered into the sedimentological record. This is important because as peat is buried and sequestered with depth, information about the precursor mangrove environment is reduced, or even lost, which may skew interpretations on the succession of mangroves and lead to imprecise reconstructions of mangrove communities.
In mangroves, the peat-forming process starts when leaf litter and other aerial plant organs (e.g., propagules, stems, etc.) are deposited on the substrate surface. As with marine invertebrates in shallow shelf deposits, these plant constituents must pass through the TAZ, and it is here that they will degrade and compact to form peat. The degree of peat decomposition is dependent on the amount of time constituents spend in the TAZ (Davies et al., 1989). Sadler (1993) suggested that the amount of time required for constituents to pass through the TAZ depends on (1) frequency and depth of reworking, (2) degree of bioturbation, and (3) thickness of the TAZ. Easily degradable constituents, such as plant debris, are less likely to survive the TAZ unless they are quickly sequestered, or the rates of decomposition and bioturbation are negligible (Davies et al., 1989). If sequestered plant debris is returned to the surface through peat loss or bioturbation, it likely would not survive a second cycle through the TAZ. Because the probability of exhumation decreases with depth (Sadler, 1993; Olszewski, 2004), buried peats enter the depth of final burial (DFB: Olszewski, 2004). It is here that the coalification process (the peat-to-coal transition) begins (Dai et al., 2020). Because the thickness of the TAZ reflects the depth of the water table (Clymo, 1984), the degree of peat decomposition can be used to interpret past hydrological and other environmental conditions that occurred within the mangrove community during the peat-forming process (Drzymulska, 2016). Generally, the rate of peat decomposition decreases during wet climate conditions when water levels are high and increases during dry climate conditions when water levels are low (Clymo, 1984). In peat-accumulating mangroves, these wet-dry conditions are reflective of the daily tide cycles where the peat decomposition rate accelerates during low tide when the water table depth has retreated and the wetland surface is aerated, and the decomposition rate decreases during high tide when the wetland is inundated with tidal waters limiting oxic decomposition of accumulated plant debris. These fluctuations in the water table facilitate peat decomposition with depth by inducing bacterial respiration processes such as methanogenesis, and the reduction of nitrate, manganese, iron, and sulfate (Aller, 1982; Canfield and Thamdrup, 2009).
Not all mangroves are peat-accumulating and the presence of peat in mangroves depends on the balance between the rate of sediment influx and the rate of organic matter accumulation including root growth (Cohen et al., 1987; Covington and Raymond, 1989; Parkinson et al., 1994; Raymond et al., 2010). On siliciclastic substrates, the rate of sediment influx is high relative to the rate of organic matter accumulation, resulting in allochthonous, organic-rich mud substrates (Woodroffe, 1983). In these environments, pollen data may be more useful to reconstruct past mangroves (Anderson and Muller, 1975; Ishman et al., 1998; Urrego et al., 2009; Willard and Bernhardt, 2011). On carbonate platforms, such as those in Florida, autochthonous mangrove peat forms because the rate of organic matter accumulation exceeds the rates of decomposition and sediment influx (Woodroffe, 1983; Cohen et al., 1987; Parkinson et al., 1994). In these environments, accumulated peat reflects the modern mangrove community, and the burial of modern peat provides a sedimentary archive that can be analyzed to understand how environmental trends, such as shifts in rainfall and sea-level rise, affect the mangrove community (McKee et al., 2007; Ezcurra et al., 2016). The occurrence of mangrove peat in sediment cores thus signals the presence of past mangroves and is commonly used to identify intertidal environments and track relative sea-level change (Cohen and Spackman, 1972, 1977; Davies and Cohen, 1989; Ishman et al., 1998; McKee and Faulkner, 2000; McKee et al., 2007; Ellison, 2008; Jones et al., 2019; Meeder et al., 2021).
Mangrove species zonation patterns coupled with geomorphology of the substrate form distinct mangrove sub-habitats that produce characteristic peat types (Lugo and Snedaker, 1974; Davies and Cohen, 1989; Snedaker, 1989; Woodroffe, 1992; Ewel et al., 1998; McKee and Faulkner, 2000). Each mangrove sub-habitat is characterized by flora, fauna, and organismal-peat interactions reflective of the salinity gradient, tidal inundation, distance from the shoreline, and other environmental factors (Odum and Heald, 1975; Haines and Montague, 1979; Zieman et al., 1984; Fleming et al., 1990; Duke, 1992, 2017; Bouillon et al., 2008). In fringe sub-habitats, the red mangrove (Rhizophora mangle) occurs in monospecific stands along the coastline of mangrove communities, and these sites experience high wave activity, tidal scouring, and the wet-dry conditions of daily tide cycles. Further inland, R. mangle may occur in mixed stands with the black mangrove (Avicennia germinans) in basin sub-habitats, and these sub-habitats experience limited tidal activity and remain inundated with standing pools of water after the tide retreats.
Particle size and the organ composition of plant debris in peats provide a means to measure the rate and infer the processes of peat decomposition in the TAZ (Boelter, 1969; Cohen and Spackman, 1977; Raymond et al., 2001). This is important because the degree of peat decomposition in historical peats can be used to identify and interpret precursor mangrove sub-habitats. Rhizophora-dominated mangrove peats that form in fringe sub-habitats with a more active TAZ have smaller-sized peat particles and more decayed plant tissues compared to mangrove peats formed in basin sub-habitats, which have larger-sized peat particles and more recognizable plant tissue because of a reduced TAZ. Further, characterization of surficial peats from contrasting mangrove sub-habitats may provide necessary information to infer the distance of the depositional environment from the shoreline in sequestered peats, where sequestered fringe peats indicate peats formed on the periphery of the mangrove whereas sequestered basin peats indicate peats formed in the interior of the mangrove.
Although mangroves occupy approximately 1.5% of global tropical and subtropical coastlines with a smaller percentage of these accumulating peat (Davis, 1940; Teas, 1977; Woodroffe, 1983; Tomlinson, 1986; Duke, 1992, 2017; Kathiresan and Bingham, 2001; Nagelkerken et al., 2008; Luther and Greenberg, 2009; Daru et al., 2013; Alongi, 2015, 2018), they are declining at an estimated 0.16% per year globally and 0.22% per year in the United States (Friess et al., 2019; Alongi, 2022). Mangrove communities in the United States are the largest in Florida and it is here that their total coverage has declined ~25% from historical estimates of 260,000 to 200,000 ha between 1985 and 2001 (Lewis, 2001). Sea-level rise, increased storm frequency, changes in rainfall patterns, and anthropogenic alteration of freshwater influx significantly affect mangrove ecosystems (Cahoon et al., 2006; Day et al., 2008; Krauss et al., 2011, 2014; Smith et al., 2013; Koh et al., 2018), and these factors may reduce the potential for mangroves to accumulate peat and store atmospheric CO2 underground via carbon sequestration (Ezcurra et al., 2016; Alongi, 2020, 2022). As for all coastal communities, the inclusion of conservation paleobiology and historical research into conservation management strategies can inform modern restoration efforts for mangrove ecosystems (Dietl and Flessa, 2011; Dietl et al., 2015; Savarese, 2018). These long-term perspectives provide conservation and restoration practitioners with expanded temporal scales on the vegetation and sea-level history of mangrove communities. Not only do these expanded temporal scales provide information on how mangroves responded to past environmental change, but they also are used to predict how mangroves may respond to anthropogenically-induced climate change (Alongi, 2008; Dahdouh-Guebas and Koedam, 2008; Ellison, 2008; Koh et al., 2018; Ong and Ellison, 2021).
Here, we differentiate between peat-accumulating mangrove sub-habitats and track the influence of changing environmental conditions on peat decomposition by using plant organ- and taxon-based measures to determine the presence and the proportional abundance of constituents in mangrove peats from two mangrove sub-habitats in South Florida: (1) a flooded basin site and (2) a tidally influenced fringe site. This will allow us to characterize mangrove peat types with depth, interpret precursor mangrove sub-habitats of deposition, and trace the environmental history of sequestered peats. Analyses into the fate of mangrove peat constituents as they become integrated into the sedimentological record enable us to not only identify the presence of mangroves on past landscapes but also to trace changes in mangrove sub-habitats with time. These establish modern analogs needed to interpret changes in the depositional environments of historical mangrove peats found in sediment cores. This information is critical because it provides insights for resource managers and urban planners to understand the responses of mangrove coasts to sea-level rise and to make management decisions that will enhance coastal resiliency in the decades ahead.
Materials and methods
Study site
We analyzed mangrove peat within a mangrove forest at the southwest corner of Barnes Sound, Florida (Figure 1A), which is the southernmost basin of the Biscayne Bay and the southeastern Everglades ecosystem (Smith, 2001; USACE and USDOI, 2020). Mangrove peats in Barnes Sound are an estimated 1–2 meters thick and mostly organic with few inclusions of fine-grained sand and silt sediments (Wanless, 1974). Destruction of mangroves occurred near our field sites with the construction of the original Jewfish Creek Bridge in 1907 for the Florida Overseas Railroad. Continued alteration to this mangrove community occurred when the bridge was restructured from a railway to a highway in 1944 and when it was converted from a drawbridge to a fixed bridge in 2008 (Garbin and Mann, 2010; Benson and Yuhr, 2016). Over time, this construction may have altered hydrological patterns within the mangrove community that affected peat accumulation within water-logged basins near the construction site. Anthropogenic diversion of freshwater from the coast coupled with sea-level rise has increased salinity over the past 150 years in Barnes Sound (Ishman et al., 1998; Ross et al., 2000; USACE and USDOI, 2020; Wingard et al., 2022). For example, the opening of the C-111 canal in 1968 diverted freshwater flow from naturally entering Barnes Sound, resulting in the shift from historically brackish to hypersaline conditions (Ishman et al., 1998). Because increasing saline conditions can shift the structure and composition of accumulated mangrove peats from brackish to marine (Davies and Cohen, 1989; Chambers et al., 2016; Meeder et al., 2021), we decided to characterize sub-habitats within this mangrove community that experience various levels of tidal exposure. We thus sampled peat from two contrasting mangrove sub-habitats: (1) a Rhizophora-dominated sheltered mangrove fringe site (Figure 1B) that is located behind the seaward mangrove periphery of the wetland where the peat experiences semidiurnal tidal activity and is shielded from direct wave activity; and (2) an interior, mixed forest basin site (Figure 1C), which is generally flooded, experiences microtidal activity, and has an accumulated thick leaf mat. Henceforth, we refer to the mixed forest basin site as ‘basin’ and the sheltered mangrove fringe site as ‘fringe’ sites.
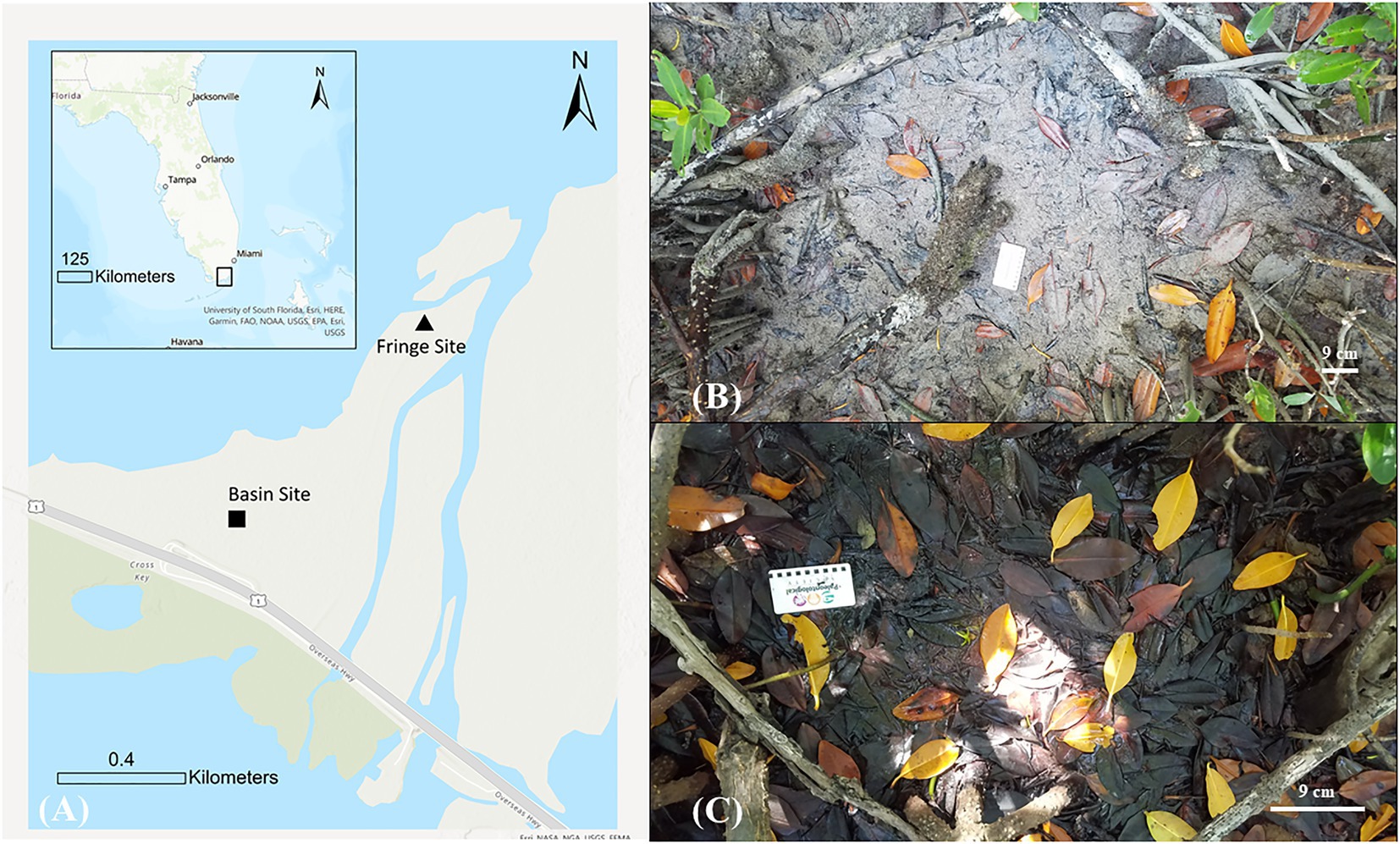
Figure 1. (A) Map of our field sites within a mangrove forest on the southwest shore of Barnes Sound, Florida. Aerial view of the peat surface from each site: (B) a sheltered mangrove fringe site with a sparse leaf mat that is tidally exposed; and (C) an interior basin site with a thick leaf mat.
Core collection and sampling
Clymo (1988) estimated the TAZ, or acrotelm, in peat bogs occurs at ≤20 cm depths. Cohen (1968) collected and characterized peat cores from South Florida and his shallowest mangrove peat sample occurred at ~18 cm depth. To provide a more informed understanding of the plant debris-to-peat transition as surficial litter is degraded and formed into peats within the upper TAZ, we targeted shallower, surficial mangrove peats not observed by Cohen (1968). In May 2018, we collected five surficial mangrove peat cores (15 cm depth × 15 cm diameter) every four meters along a 20 m transect from our field sites. We followed the coring protocol of Schultz (2015), gently hammering the coring device into the peat substrate. Because large woody debris and roots extended beyond the edge of the coring device, we used a small hand saw to cut through them to facilitate core extraction and placed a hand underneath the core to prevent peat loss during extraction. We extruded the cores into nylon mesh sleeves with the same dimensions as the coring device to prevent diagenetic reactions between the edge of the cored peat and the interior walls of the metal core canister, to retain the shape of the core, and to allow the core to drain. Although the edges of most cores had some surficial debris carried to deeper depths by the coring process, examination of the cores showed that peat in the interior of cores remained undistorted. After most of the water had drained from the peat, we sealed extruded cores in plastic bags and stored them in a temperature-controlled cooler within a dark, air-conditioned room until we could process them. Cores were processed within 6 months of collection.
Leaf mat thickness
We measured the thickness of the surficial leaf mat by counting the number of accumulated, stacked leaves above the peat substrate (Cintra, 1997; Schultz, 2015). We reported leaf mat thickness in terms of stacked leaves because it enables comparisons between modern leaf mats formed from different broad-leaved plant communities, and between modern and ancient leaf mats (Raymond, 1987; Gastaldo and Staub, 1999; Schultz, 2015). Leaf mat thickness reflects the decomposition rate of leaf litter, with thicker accumulated leaf mats indicating slower rates of decomposition (Raymond, 1987; Schultz, 2015). To measure leaf mat thickness, we inserted a wire probe into the center of the substrate and counted the number of stacked leaves with intact margins and large leaf fragments (≥1 cm2) that touched the needle (Cintra, 1997; Schultz, 2015). We stopped counting leaves when they were consistently less than 1 cm2. Because the peat surface is exposed at low tide, we measured leaf mat thickness at the fringe site every meter along a 20 m transect, yielding 21 leaf-mat counts. Because the waterlogged nature of the basin site made it too difficult to make a clear measure of leaf mat thickness in the field, we determined leaf mat thickness in the lab from our collected basin peat cores. We increased our basin sample to 10 measures of leaf mat thickness by supplementing these data with comparable data from Schultz (2015), who conducted research at the same basin site during contemporaneous months. Although sampled during different years, measures of leaf mat thickness from our study and Schultz (2015) yielded similar leaf mat counts. We compared the differences in leaf mat thickness between mangrove sites using the Mann–Whitney U test, performed using the function wilcox.test in the “stats” package of R version 4.1.3 (R Core Team, 2021).
Proportional abundance of peat constituents
In this study, we used the wet-sieve method as a cost-effective and time-sensitive tool to analyze the proportional abundance of peat constituents in mangrove peats from basin and fringe mangrove sub-habitats. In basin cores, the cohesive leaf mat overlies the intact peat; the leaf mat at the fringe site was thin and sparse and was not sampled in fringe cores. We thus removed the intact leaf mat from basin cores. All cores were divided into three levels at 5 cm depth intervals and sampled: upper (0–5 cm), middle (5–10 cm), and base (10–15 cm). The upper level differed from other sampled core depths because it consisted of identifiable plant debris, such as fragmented leaves (<1 cm2), twigs, and rootlets. We thus equated the upper level as the incohesive leaf mat, which was located below the leaf mat in basin cores and at the surface of the peat substrate in fringe cores.
We analyzed peat subsamples (12.5 cm3) from each core to determine the proportional abundance of peat constituents at four levels, including the cohesive leaf mat, for the basin cores and three levels for the fringe cores. For each core, we took two replicate peat subsamples from each core level. We submerged subsamples in 150 mL of water for 12–16 h to disaggregate them before wet sieve processing (Levesque and Dinel, 1977). Disaggregated subsamples were wet-sieved in eight fractions: 8.0 mm, 4.0 mm, 2.0 mm, 1.0 mm, 500 μm, 250 μm, 150 μm, and 106 μm. We used a hand-held Büchner funnel to collect particles that were < 106 μm from the collected liquid.
After wet-sieving, point counts of peat constituents were made for each size fraction. All constituents on the larger sieves (≥1,000 μm) were counted and identified. Because constituents on sieves <1,000 μm were too numerous to count, we made point counts (n = 300) on sieved size fractions between 106 and 1,000 μm using a digital grid that overlays the field of view on a high-resolution dissecting microscope at 10× magnification. The digital grid overlay corresponded with the mesh size of each sieved fraction. For example, we used a 500 μm digital grid on the sieve fraction with a 500 μm mesh. We counted constituents that corresponded with the cross-sections of the digital grid, and we increased the magnification on the microscope to identify them.
Previous studies in South Florida used thin sections to track the proportional abundance of peat constituents to describe peats and measure the particle size distribution of peat (Cohen, 1968; Cohen and Spackman, 1977). These authors divided peat particles into two size fractions: framework (F: plant organs having one dimension ≥100 μm that provide fibrous structure to the peat) and matrix (M: plant tissue remains and particles with dimensions <100 μm) and determined framework percentages of 49–54% for mangrove peat in South Florida. Because of easily available sieve sizes, we defined framework as plant organ particles having one dimension ≥106 μm, and matrix as plant tissue remains and particles with all dimensions <106 μm. The wet-sieve method used here allows us to easily track and compare the size of framework to matrix particles. Since particle size has been used as a proxy to assess the amount of decomposition, the proportion of framework to matrix particles (F:M ratio: as determined by point counts described above) in this study was used to indicate the sub-habitat of deposition in sequestered peats.
Cohen (1968) separated framework peat constituents into roots and subaerial organs. Instead of ‘subaerial organs,’ we used the term ‘aerial organs’ to describe fallen leaf litter and other aerial plant organs deposited on the peat surface. Following Cohen (1968), we used root percentage to refer to the percentage of all framework constituents composed of root debris (see also Cohen and Spackman, 1977). Numerous studies have reported the root percentage, or root-shoot ratio, of peat (Raymond, 1987; Esterle et al., 1989; Middleton and McKee, 2001), enabling comparisons of modern and ancient peat deposits. We also used the term ‘total percent of roots,’ which is the percentage of roots relative to all peat constituents.
Matrix constituents included all constituents that would eventually degrade into small particles (<106 μm). Matrix constituents thus included all particles that were cells, fecal pellets, and miscellaneous debris. Our cells category consisted of plant tissues that were reduced to individual cells, cell fragments, and sheets of cells that were < 500 μm. Miscellaneous debris consisted of sediment grains and unidentifiable particles. We also tracked the occurrence of organismal peat constituents, which include insect parts, foraminifera, and mollusk shells.
After making point counts, we dry-sieved samples for 24 h at 50°C and weighed all constituents on each sieve to estimate their proportional abundance within the peat. Because our data consist of subsample replicates, we averaged our constituent proportional abundance values at each core level for each site. To compare framework to matrix particles among and between field sites, we calculated dry weight ratios of framework particles (≥ 106 μm) to matrix particles (<106 μm). We compared our data for the proportional abundance of peat constituents between sites and depth using a two-way ANOVA with post-hoc Tukey tests to differentiate the significance between depth levels. We used the anova function for the two-way ANOVA and the Tukey function for the post-hoc Tukey tests in the statistical package “stats” of R version 4.1.3 (R Core Team, 2021).
Historical data analyses
To investigate whether peat from different mangrove sub-habitats can be distinguished based on the proportional abundance of peat constituents, we compared our data with the historical mangrove peat data from Cohen (1968). Cohen (1968) categorized peat types based on the relative percentage of root constituents: root-dominated peat (<5% leaf litter and other aerial organs) and sedimentary peat (≥5% leaf litter and other aerial organs). We applied these established peat types to our surficial peat data and created a new category: leaf mat (≥40% leaf litter and other aerial organs).
The peat constituent categories directly comparable between our data and the data of Cohen (1968) were framework, roots, and aerial organs. Cohen (1968) and Cohen and Spackman (1977) included fecal pellets in their matrix category. Accordingly, we combined our fecal pellet data with our miscellaneous debris data to create a comparable fine granular debris category. Because we did not differentiate between cells and cell fragments, and we did not track cell inclusions in our data, we combined these categories from Cohen (1968) into our cells category for comparison. The cells and fine granular debris categories were combined and included in our total matrix category. We were not able to include insect parts or foraminifera proportional abundances in our comparison study because Cohen (1968) did not track their proportional abundances, but instead lumped these data in the matrix category.
For this study, linear discriminant analysis (LDA) was applied to a combined mangrove peat dataset of our modern data and the historical data of Cohen (1968). We used LDA to reduce the dimensionality of the quantified peat properties (proportional abundances of roots, aerial organs, and matrix particles) and determine the combination of peat constituents that best differentiate between peat types. These selected categories form the basis for the LDA that classified each subsample into a peat type: leaf mat, sedimentary peat, and root peat. To test the accuracy of our peat categories, we performed the leave-one-out cross-validation with the combined dataset. Variables for the LDA were z-score transformed before running the test. For the LDA, we use the lda function in the statistical package “MASS” of R version 4.1.3 (Venables and Ripley, 2002).
We used a detrended correspondence analysis (DCA) as an indirect gradient analysis to summarize the primary gradients in peat composition for the different peat types (Kent and Coker, 2011). The DCA has been used in numerous modern and historical studies to place samples along an environmental gradient based on their constituents (McCune et al., 2002; Kleinebecker et al., 2007; Sharon et al., 2021). We z-score transformed the data using the decostand function and performed the DCA using the function decorana from the statistical package “VEGAN” of R version 4.1.3 (Oksanen et al., 2020). Because the scores on DCA axis 1 explain the most variance in the data (Kent and Coker, 2011), we focused on the first axis to determine if the primary variation in peat composition was related to a core depth gradient. We computed the Spearman correlation between the DCA axis 1 score of a peat sample and the core depth from which it was collected. We used the cor.test function for the Spearman correlation in the “stats” package of R version 4.1.3 (R Core Team, 2021).
Results
Leaf mat thickness
The leaf mat in the basin site was significantly thicker than that of the fringe site based on the Mann–Whitney U test (W = 0, p < 0.01). The leaf mat in the fringe site ranged from 0 to 4 stacked leaves accumulated on the peat surface, with a median of zero and a mean of 0.76 (SD = ± 1.04) stacked leaves, whereas the thicker leaf mat in the basin site ranged from 19 to 37 stacked leaves, with a median of 25.5, and mean of 26.75 (SD = 6.39) stacked leaves (Figure 2). Leaves displaying mostly entire margins occurred at the top of cores from the basin site. In all basin cores, the leaf mat consisted of leaves and leaf fragments from R. mangle and A. germinans, non-woody rootlets, wood, bark, A. germinans pneumatophores, and fragments of R. mangle propagules. Well-preserved micro-arthropod fecal pellets and snail fecal strings occurred between and on the surface of leaves. Other important animal remains within the leaf mat included insect parts, foraminifera, and mollusk shells. We identified mollusk shells in the leaf mat and within cores as Melampus coffea, an endemic snail of South Florida that is common in both basin and fringe mangrove sub-habitats (Proffitt et al., 1993; Proffitt and Devlin, 2005). Although shells of the marine snail, Phrontis vibex were observed on the periphery of the fringe site, we did not observe them in the leaf mat nor within cores.
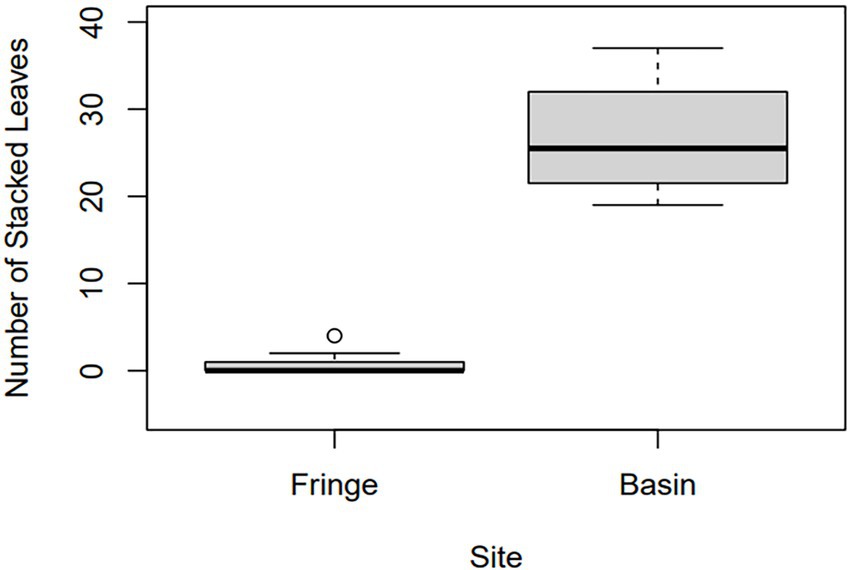
Figure 2. Boxplot depicting leaf mat thickness for fringe and basin sites. Leaf mat thickness is defined as the number of stacked leaves that overlie the peat substrate. Leaf mat thickness data for the basin site was collected from surficial cores (n = 10), whereas that for the fringe site was collected along a 20 m transect at every meter (n = 21).
Proportional abundance of peat constituents
Framework constituents (≥106 μm) accounted for 51.6–68.7% and 41.3–72.3% of peat cores at basin and fringe sites, respectively (Table 1; Figure 3). Fringe cores had a higher root percentage (i.e., percent of all framework constituents that are roots) than basin cores (41 vs. 34%), with the highest proportion of roots concentrated at 10 cm depth (48%). The highest root percentage in basin sites occurred at the base of the core (45%). Even though basin sites exhibited a statistically significantly thicker leaf mat compared to fringe sites, there was no significant difference in the proportion of aerial organs between sites below the surface (Table 1; Figure 3D). In both sites, the proportion of roots and aerial organs increased significantly with depth, specifically between 5 and 10 cm depths (Table 2; Figures 3C,D).
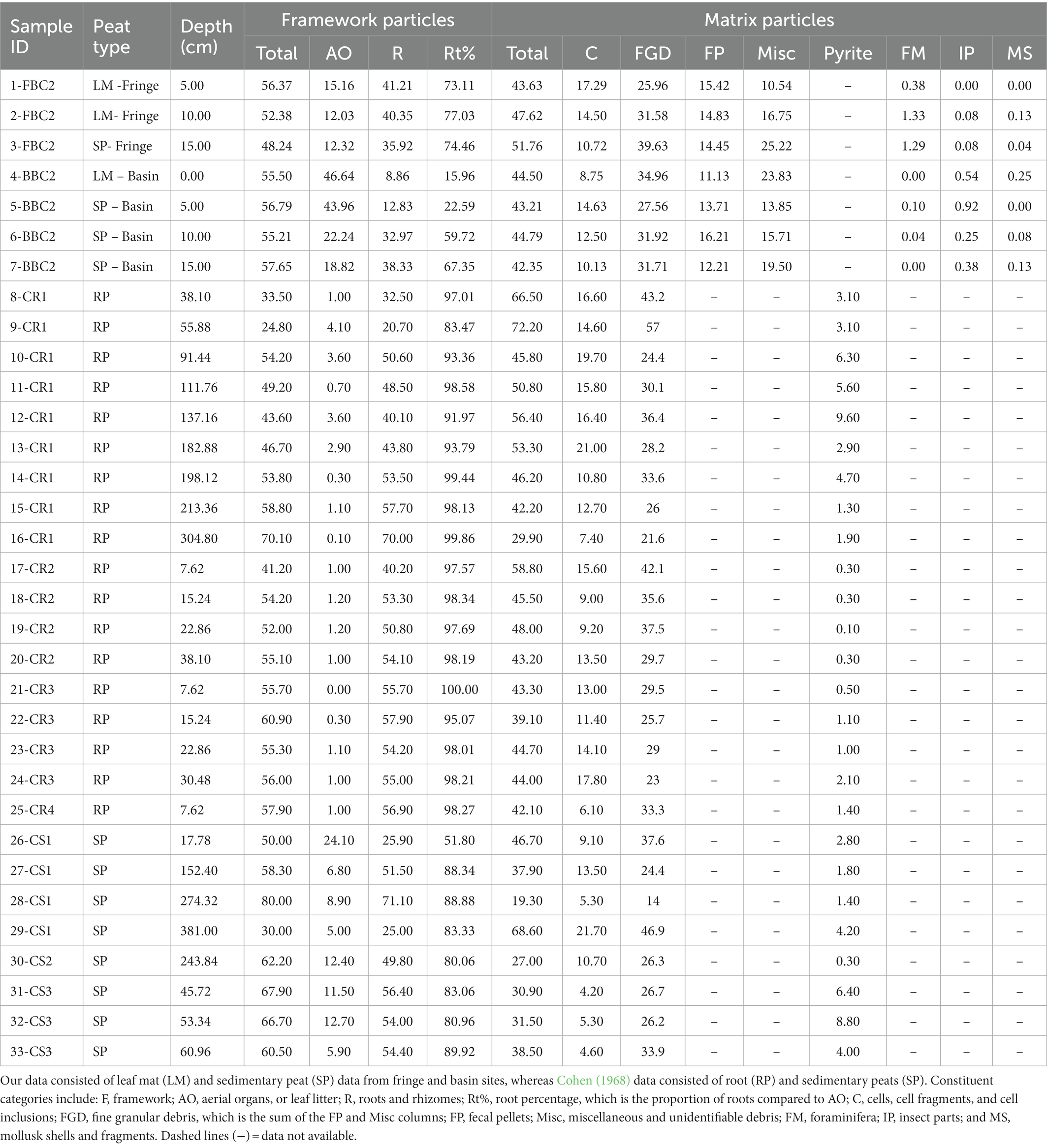
Table 1. Proportional abundances of organic and inorganic mangrove peat constituents within our modern samples and the historical samples from Cohen (1968).
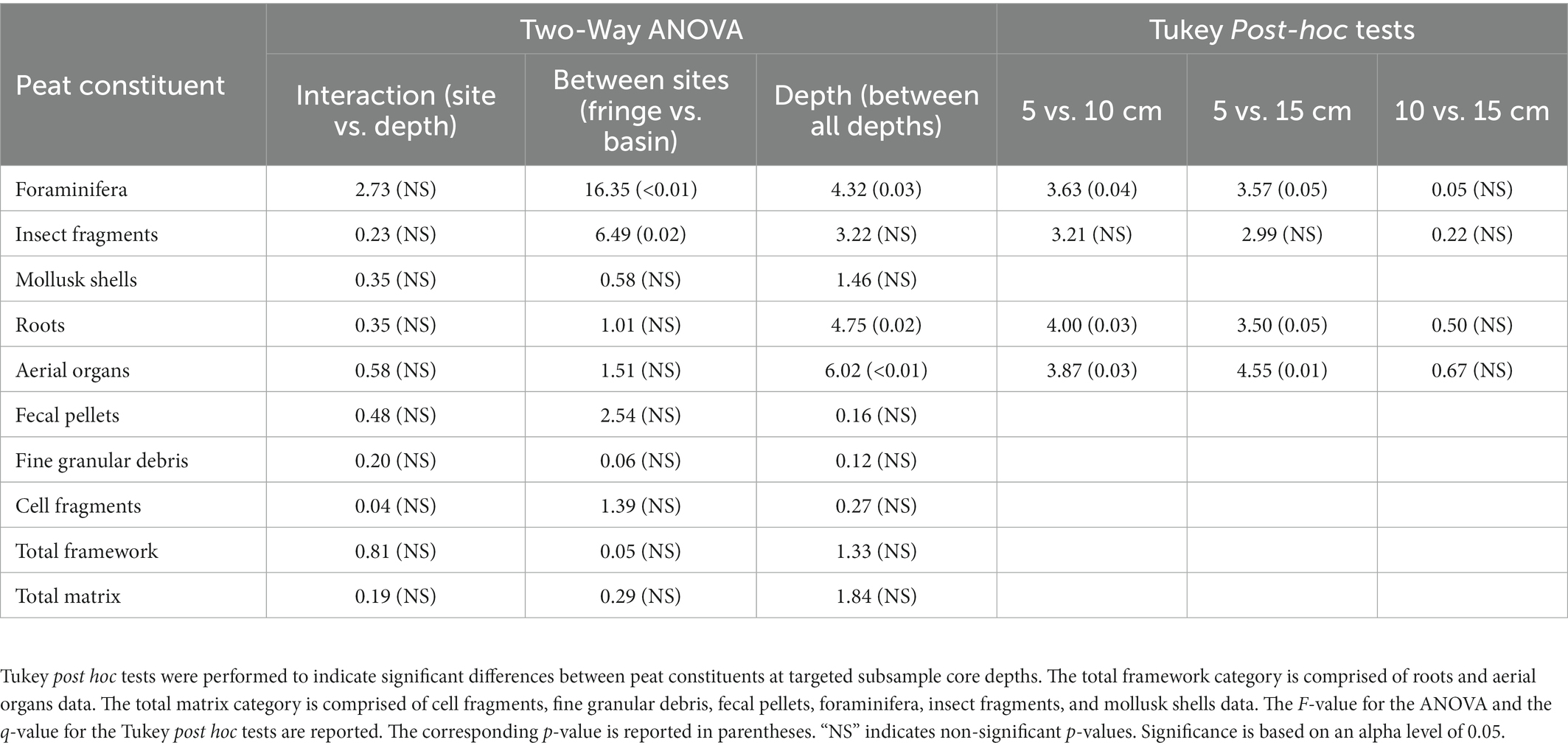
Table 2. Importance of peat constituents with characterizing and differentiating mangrove peat between sites (fringe vs. basin) and sampled depths, based on a Two-Way ANOVA.
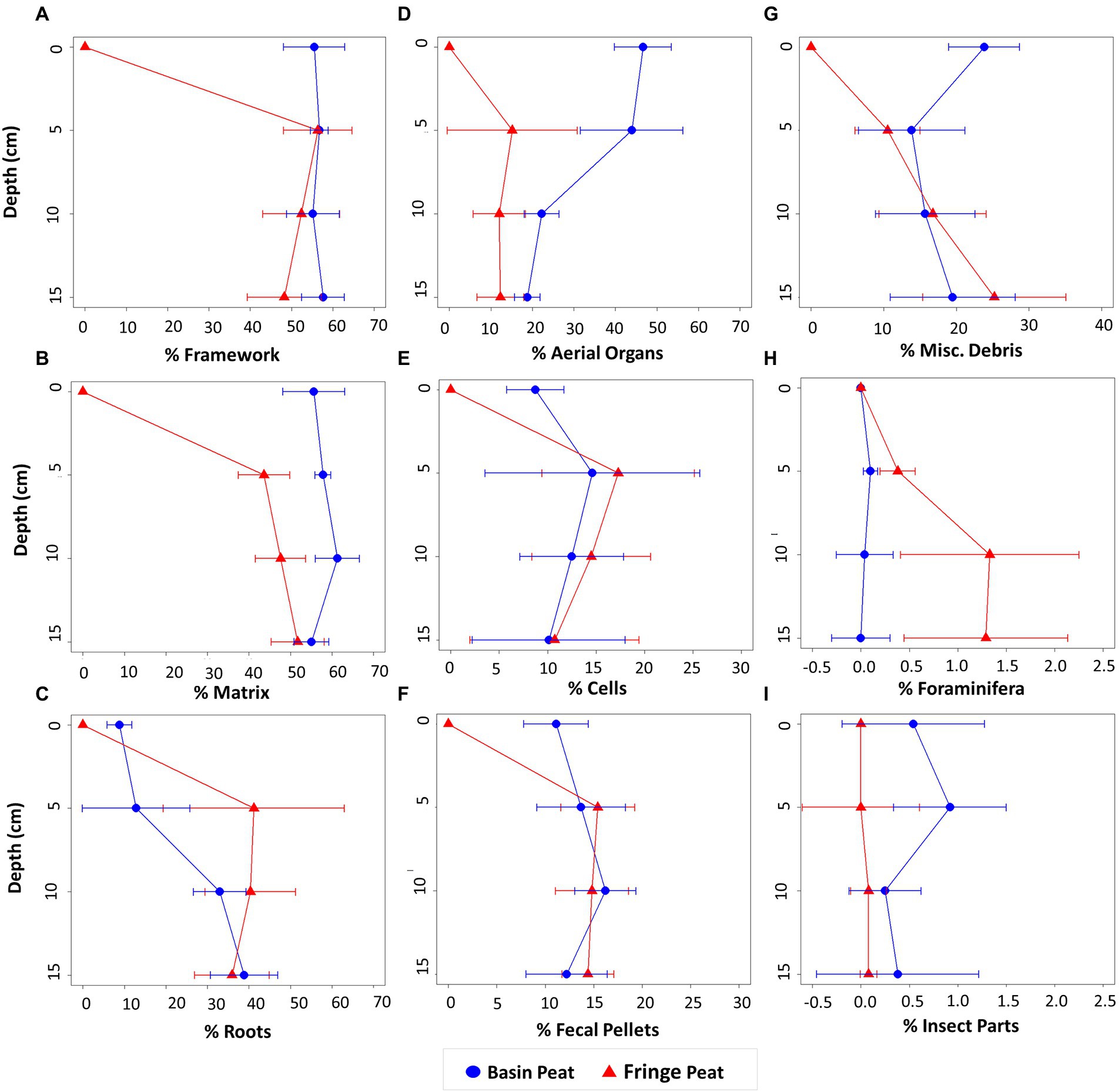
Figure 3. Proportional abundance of peat constituents at depth (cm) for our sampled fringe and basin peats: (A) framework; (B) matrix; (C) roots; (D) aerial organs; (E) cells and cell fragments; (F) fecal pellets; (G) miscellaneous (misc.) debris; (H) foraminifera; and (I) insect parts. There are 10 data points per site at each depth interval (e.g., for each site, five cores were collected, and two subsamples were collected at each depth level = 10 data points). These data points were averaged for each depth level.
Based on a two-way ANOVA, the proportional abundance of foraminifera and insect parts were significantly different between sites: the fringe site had a higher abundance of foraminifera whereas the basin site had a higher abundance of insect parts (Table 3). There was no significant difference in the proportional abundance of mollusk shells found within cores between sites. The interaction of the proportional abundance of mollusk shells between sites and with depth was not significant (Table 3).
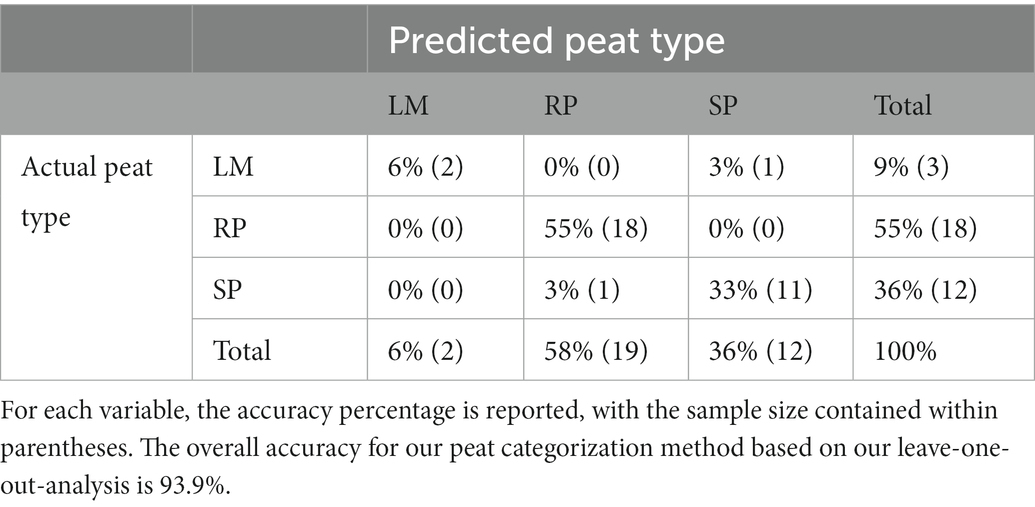
Table 3. Confusion matrix for different peat types: leaf mat (LM), root peat (RP), and sedimentary peat (SP).
Comparison with historical data
LD1 and LD2 had the greatest proportion of trace values (LD1 = 85.80%; LD2 = 14.20%) and sample scores on these two axes provided the best discrimination among peat types. On LD1, root peats had higher discriminate scores, whereas leaf mat peats had lower discriminate scores (Figure 4). Samples with a higher proportional abundance of roots and matrix constituents had higher LD1 scores than samples with a higher proportional abundance of aerial organs. Our basin leaf mat peats had the most negative values on LD1 indicating lower root and higher aerial organs percentages (Figure 4). Sedimentary peats had intermediate values on LD2; these samples had lower percentages of matrix particles and higher percentages of aerial organs compared to root peats (Figure 4). The overall accuracy of distinguishing between peat types was 93.9% (Table 3). Our method of categorizing peat types by the proportional abundance of aerial organs correctly identified root peats (100%) but misidentified one leaf mat sample and one sedimentary peat sample. Thus, our fringe leaf mat sample (1 – FBC2) was reassigned as a sedimentary peat, whereas a sedimentary peat sample (29 – CS1) from the Cohen (1968) data was reassigned as root peat.
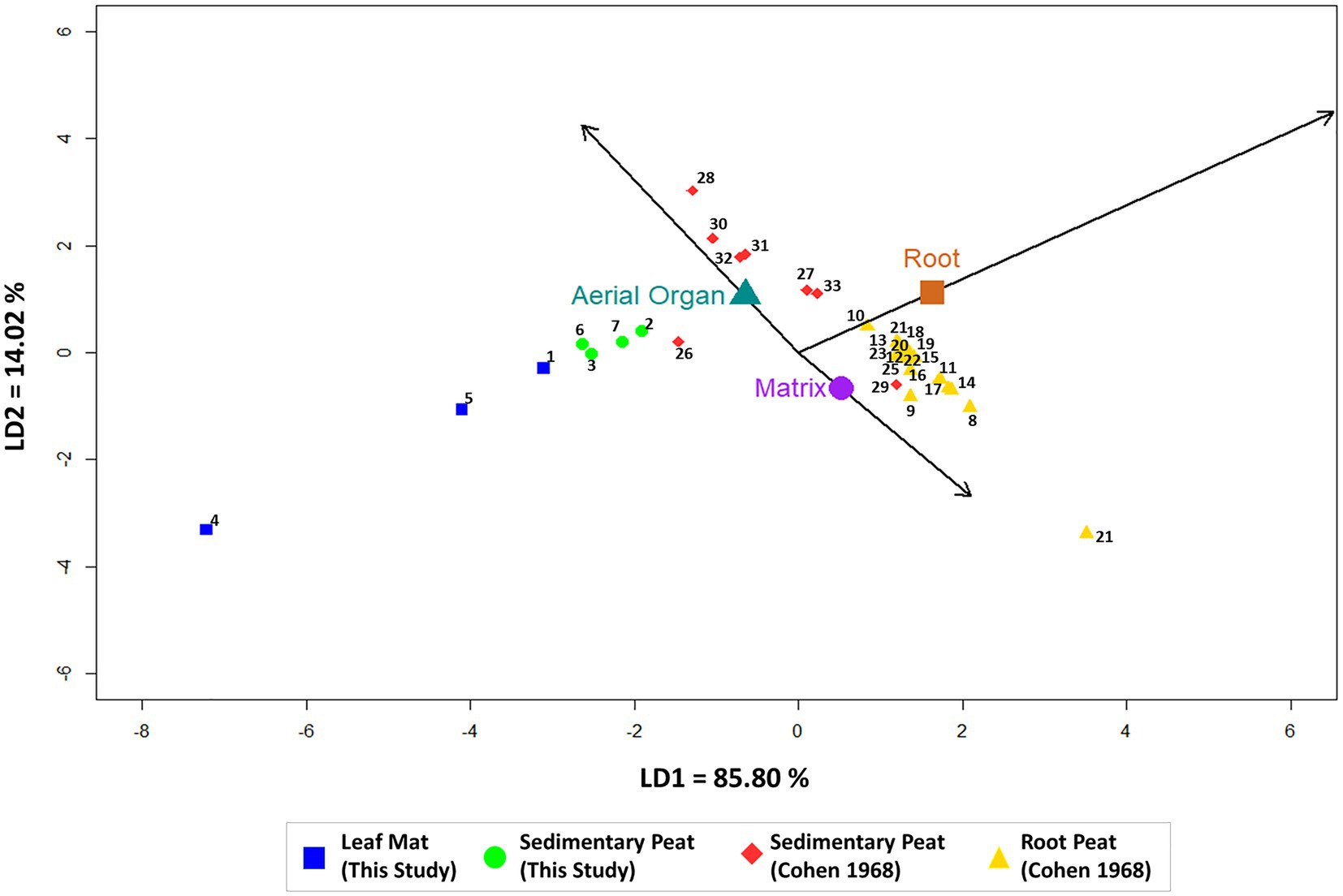
Figure 4. Linear discriminant analysis of mangrove peat types from South Florida, based on proportional abundance data of constituents within sampled peats: aerial organs, roots, and matrix. Our data consisted of leaf mat and sedimentary peat data from basin and fringe mangrove sub-habitats, whereas Cohen (1968) data consisted of sedimentary and root peats. Data labels correspond with the Sample ID number in Table 1.
DCA Axis 1 returned 75.84% of the variance among peat samples. Samples with higher scores on DCA axis 1 had a higher percentage of aerial organs; samples with lower scores had a higher percentage of matrix particles (Figure 5A). Because the percentage of aerial organs in peat declines with depth, sample scores on DCA axis 1 showed a significant covariance with depth, with shallow samples having higher DCA axis 1 scores than deeper samples (rho = −0.96; Figure 5B). DCA axis 2 summarized 8.11% of the variance of peat constituents among sites. Peats with higher DCA axis 2 scores had lower root percentages, whereas those with lower DCA axis 2 scores had higher root percentages.
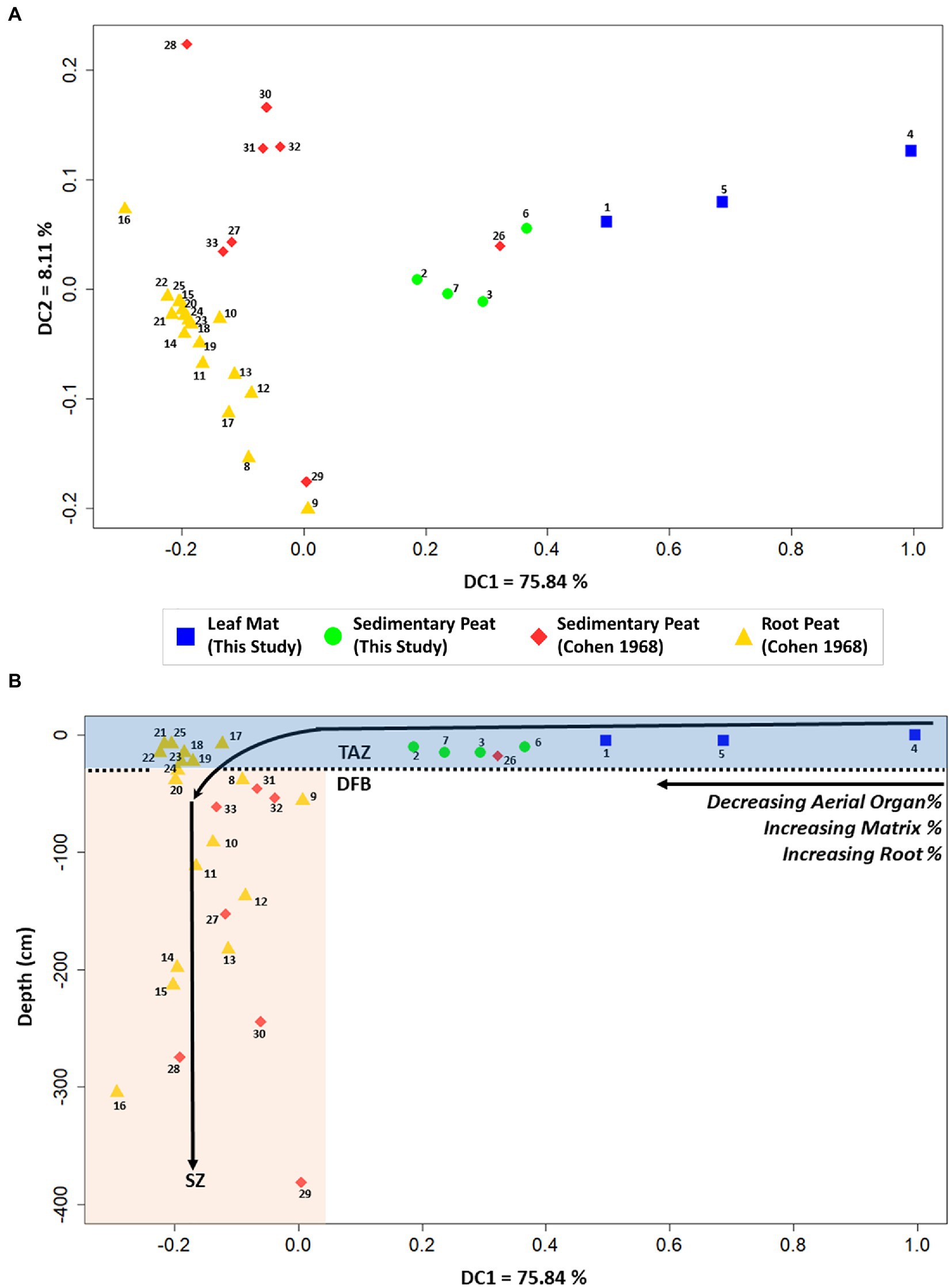
Figure 5. Detrended correspondence analyses (DCA) for the different peat types: (A) DCA axis 1 vs. DCA axis 2 scores of samples; (B) DCA axis 1 vs. core depth (cm). Our data consisted of leaf mat and sedimentary peat data from basin and fringe mangrove sub-habitats, whereas Cohen (1968) data consisted of sedimentary and root peats. Data labels correspond with the Sample ID number in Table 1.
Discussion
Mangrove peats retain a wealth of historical data about the composition and interactions within mangrove communities. By characterizing surficial mangrove peats with depth from contrasting mangrove sub-habitats, we were able to assess the influence of the taphonomically active zone on the peat-forming process and create modern analogs that allow us to better decipher historical mangrove peats that were sequestered into the sedimentological record. Through our analyses, we extrapolated downcore trends in a novel paleobotanical approach that allows us to interpret and reconstruct precursor mangrove habitats from cores. The insights provided from these interpretations of historical peats allow us to better understand past responses to change, which in turn provides decision-makers with information to make long-term decisions about coastal resiliency and mangrove conservation. Here, we use the TAZ model to interpret our results and discuss leaf mat thickness and percentages of aerial organs, roots, and faunal constituents in terms of their use for deciphering mangrove peats with depth.
Locating the TAZ in mangrove peat
Because peat types reflect a strong depth gradient (Figure 5B), we interpret the TAZ - DFB transition as the gradational downcore transition from surficial peat with abundant leaf litter and other aerial organs to sequestered peat that is root- and matrix-rich. Concomitant with this downcore transition is the gradual decrease in decomposition rates with depth (Drzymulska, 2016). Although we did not encounter root peat in our surficial samples (≤15 cm depth), it was identified by Cohen (1968) to occur at approximately 8 cm depth. This suggests that the TAZ – DFB transition for surficial root peats may occur closer to the peat surface in wave-dominated fringe sites that experience rapid decomposition and tidal scouring, a depositional environment unlike the mangrove sites that we sampled. This is significant because a shallower TAZ coupled with increased tidal activity would increase the rate of decomposition, leading to faster degradation of aerial plant debris resulting in the peat constituent signature of root peat. Our surficial samples were identified as leaf mats and sedimentary peats that are rich in leaf litter and other aerial organs. These surficial peat types occur in depositional environments with relatively lower decomposition rates and limited tidal influence, such as in the sheltered fringe and basin sites of this study. At these sites, the TAZ – DFB transition occurs at deeper depths than wave-dominated fringe sites. Cohen’s (1968) shallowest sedimentary peat sample (26 – CS1), which has an aerial organs percentage of 24%, occurs near the peat surface (17.78 cm depth) and has as much aerial debris as our deepest basin sedimentary peat (15 cm depth). Taken together, these samples suggest that the base of the TAZ for sedimentary peats occurs at least 18 cm depth. Further, R. mangle produces an abundant root mat at 20–30 cm depth (Wanless, 1974), which suggests that the TAZ extends to this depth of live horizontal rooting. This depth could be deeper in basin sub-habitats that have a higher abundance of A. germinans, which has a deeper rooting depth than R. mangle (Davies and Cohen, 1989). Moreover, fossorial crabs have been observed to burrow to deep depths in mangroves (<1.2 m: Kristensen, 2008). These deep burrows, which introduce oxygen at depth, further extend the depth of the TAZ. The proportional abundance of roots increases with depth in the deep cores (>1 m) from Cohen (1968), suggesting that differential compaction and microbial decomposition of aerial plant debris relative to fibrous rootlets continue to alter peat below the TAZ.
Leaf litter and aerial organs
The leaf mat at the basin site is significantly thicker than at the fringe site, suggesting differences in litterfall dynamics and soil processes lead to leaf mat formation Although mangroves continuously shed leaves throughout the year (Gladstone-Gallagher et al., 2014), litterfall is not uniform (Odum and Heald, 1975); increased litterfall occurs seasonally during hurricanes and other extreme weather events (Kathiresan and Bingham, 2001; Davis et al., 2004; Zhao et al., 2021). Thicker leaf mats occur in supratidal mangrove sub-habitats that experience reduced tidal export compared to those on the seaward periphery of the mangrove (Odum and Heald, 1975; Cohen and Spackman, 1977; Davies and Cohen, 1989; Primavera, 1998). Many studies suggested the disparity in surficial leaf mat thickness among mangrove sub-habitats correlates with slower rates of decomposition in basin sites relative to fringe sites (Woodroffe, 1992; Rivera-Monroy et al., 1995; Reis et al., 2017). Twilley et al. (1986) reported that accumulated leaf litter has longer residence times in interior mangrove sites when compared to sites closer to the seaward periphery of the mangrove, and that leaf litter in sites <100 m from the seaward periphery in mixed forest stands have longer residence times than leaf litter in monospecific stands. This suggests that leaf litter in our fringe site has higher leaf turnover rates than leaf litter in our basin site and could explain the disparity in leaf mat thickness between these sites. Raymond et al. (2001) attributed extremely thick leaf mats in Paleozoic permineralized peat to reduced rates of decomposition. Further, the condition and identity of plant organs in the cohesive leaf mat of peat from Barnes Sound is similar to that described by Raymond et al. (2012) in the permineralized leaf mats of Pennsylvanian (~299–323 Ma) fossilized peat. Although leaves are sporadically present on the peat surface in the fringe site, we expected our uppermost fringe sample (1 – FBC2) to plot with the incohesive leaf mat basin sample (5 – BBC2: 0–5 cm depth). Instead, it was recategorized as a sedimentary peat in the leave-one-out analysis of our LDA. This suggests that the fringe site has neither a cohesive nor an incohesive leaf mat. Both the sparse leaf mat and the lack of an incohesive leaf mat at this site suggest that leaf debris and other aerial organs are exported via tidal surges or decompose rapidly once they fall to the substrate. Leaf litter in the fringe site may fragment and decompose quickly in part because exposure to the substrate surface and the delivery of oxygenated water into the wetland during each tidal cycle increases aerobic decomposition; thus, preventing peat accumulation above the tide line (Benner and Hodson, 1985; Davies and Cohen, 1989).
Invertebrate detritivores could influence the accumulation of a cohesive leaf mat (Swift et al., 1979; Middleton and McKee, 2001; Proffitt and Devlin, 2005; Kristensen, 2008; Santonja et al., 2018). Detritivory is tied to tidal influence because tides and topography control the distribution of bioturbators and aerobic invertebrate detritivores that consume leaf litter and other aerial plant debris (Fell et al., 1975; Davies and Cohen, 1989; Camilleri, 1992; Lavelle et al., 1993; Bouillon et al., 2008). For example, the detritivorous pulmonate snail Melampus coffea is estimated to consume approximately 40.5% of detrital leaf debris in mangroves of South Florida (Proffitt and Devlin, 2005). In the basin site, high standing water levels limit access of air-breathing detritivores, such as M. coffea, to the peat surface and restrict their feeding behaviors to non-flooded areas, which could play a key role in the accumulation of thick leaf mats.
Sedimentary peat, interpreted here as peat formed within the TAZ below the leaf mat, occurs at variable depths throughout Cohen’s (1968) deep cores (Figure 5B), and its presence indicates preserved basin depositional environments that likely accumulated thick, surficial leaf mats. The preservation of cohesive leaf mats at depth in peat deposits would thus require their rapid removal from the TAZ. In both freshwater and estuarine wetlands, flooding and storm events can transport enough sediment to bury the surficial leaf mat below the TAZ (Wanless, 1974; Scheihing and Pfefferkorn, 1984; Wnuk and Pfefferkorn, 1987; Thomas and Cleal, 2015). In modern and ancient peat deposits, comminuted plant debris at the base of an inorganic sediment layer may be the only record of the surficial leaf mat. Exceptions include airborne ash deposits, which conceivably could bury the surficial leaf mat without disruption, and rapid permineralization, which has preserved surficial leaf mats in Pennsylvanian coal deposits from Euramerica (Snigirevskaya, 1972; Raymond et al., 2001), Triassic coal deposits from Antarctica (Slater et al., 2015), and Eocene coal deposits of North America (Schoenhut et al., 2004).
Davies and Cohen (1989) suggested the preservation of Avicennia-Rhizophora sedimentary peats, interpreted here as equivalent to basin peats, results from the rapid accumulation of overlying detrital marine carbonates, which could occur during storm events. Although our samples do not contain evidence of high sediment loading, the presence of large amounts of inorganic sediment in peat cores from hurricane-impacted areas may correlate with a storm event that transported marine sediment into the wetland (Risi et al., 1995; Smith et al., 2009; Whelan et al., 2009; Wingard et al., 2020). The transport and deposition of marine sediments into mangroves promote carbon sequestration via the rapid removal of leaf litter and other aerial organs from the TAZ (Day et al., 2008; Smith et al., 2009), leading to their preservation in peats at deeper depths. Further, marine sediments lead to an increase in mangrove productivity because they provide necessary nutrients that promote new growth as the living mangrove forest recovers from catastrophic events (Smith et al., 2009; Castañeda-Moya et al., 2020).
Wanless (1974) reported that inorganic debris within accumulated peat from Barnes Sound, FL, contains abundant quartz sand. However, shallow cores from both our sites contained negligible amounts of quartz sand, which we included in our miscellaneous debris category. This suggests that our modern peat cores did not experience marine sediment overloading from hurricanes. In the absence of sediment overloading, which could preserve leaf litter and other aerial organs, saltwater encroachment will likely increase the decomposition of accumulated sedimentary peats, altering them to become more indicative of Rhizophora root peats with high root percentages. This could explain why Davies and Cohen (1989) observed that Avicennia-Rhizophora sedimentary peat and Rhizophora root peat, interpreted here as basin and fringe peats respectively, rarely occur in the same core. However, Clymo (1987) reported layers of well-preserved peat surrounded by highly decayed peat in freshwater Sphagnum bogs, which suggests that changes in wetland hydrology may play a pivotal role in carbon sequestration and preservation of aerial plant debris in the absence of inorganic sedimentation.
Root percentages
In both ancient and modern peats, root percentages have been used to indicate the amount of peat decomposition (Cohen and Spackman, 1977; Covington and Raymond, 1989; DiMichele and Phillips, 1994; Hoyos-Santillan et al., 2015). The historical mangrove peats of Cohen (1968) are dominated by roots (Table 1; see also Davies and Cohen, 1989). This suggests that few aerial organs survive the TAZ to become incorporated into the sequestered peat. The root percentages of the historical peats are greater than our surficial sedimentary peats (Table 1: 60–67% at the basin and 73–77% at the fringe site), which have higher abundances of aerial organs. Cohen (1968) considered mangrove peat with leaf percentages as low as 5% as sedimentary peats; however, 26–71% of his samples consists of root debris, with root percentages ranging from 52 to 90% (Table 1). Raymond (1987) reported average root percentages for the upper 35 cm of mangrove peat at five fringe mangrove localities in the Ten Thousand Islands section of the Everglades. At these sites, the average root percentage of mangrove peat varied from 77 to 94%, and individual core increments of 2.5 cm ranged from 61 to 100% root. Gladstone-Gallagher et al. (2014) demonstrated that decomposition rates of leaves and woody root debris decreased with burial depth. The percentage of roots in peat thus is expected to be higher deep in the subsurface than near the surface. However, our observations of horizontal rootlets growing through the surficial leaf mat and relatively high root percentages in surficial cores suggest that mangrove taxa target the upper part of the TAZ to obtain nutrients from quickly decaying leaves. Because aerial organs have a faster decomposition rate than roots and are more likely to succumb to the taphonomic processes within the TAZ (Drzymulska, 2016), fragile leaf debris and other aerial organs will degrade into unidentifiable matrix particles and root percentages will increase in sedimentary peats with depth. Sedimentary peats will thus transition into root peats with depth, creating a taphonomic bias of root peats in the sedimentological record. This could explain why Rhizophora root peat is the most common peat type observed by Cohen (1968) and Raymond (1987).
Additionally, preserved mangrove roots in sequestered peats can be identified at the species level, allowing for reconstructions of the living mangrove community at the time of peat formation (Cohen, 1968; Cohen and Spackman, 1977; Raymond, 1987; Davies and Cohen, 1989; McKee and Faulkner, 2000). Roots may be more abundant in deeper peats because vertical roots may bypass the upper part of the TAZ as they grow into sequestered peat and will decompose more slowly than aerial plant debris once they die (Raymond, 1987, 1988; Raymond et al., 2001). Davies and Cohen (1989) warned that roots alter the characterization of sequestered peats and paleoenvironmental reconstructions because intruded roots from living trees may inflate the root percentages in the sequestered peat at depth. They warned Avicennia communities growing on sequestered Rhizophora root peats could create the false impression of a mixed forest community, particularly because Avicennia is a deeper rooting tree than Rhizophora. Furthermore, other botanical non-root constituents that could be used to define Avicennia peats are rare in sequestered peats, indicating a rapid decomposition of these plant constituents (Davies and Cohen, 1989).
Faunal constituents
Cohen (1973) observed that non-botanical constituents, including foraminifera, insect parts, and fecal pellets, are relatively common in surficial peats. Our data show that foraminifera provide a taphonomically robust signal: higher abundances of foraminifera indicate tidally influenced fringe sites, whereas lower abundances or their absences indicate inundated basin sites. Davies and Cohen (1989) also noted that foraminifera are common in Rhizophora root peats, which experience scouring from daily tides, but rarely occur in Avicennia-dominated basin peats. Foraminifera are common in sequestered sediments of acidic and hypersaline mangrove environments (Woodroffe et al., 2005; Fiorini et al., 2019). If their calcareous tests dissolve, foraminifera in sequestered mangrove peats can be identified by the organic linings of their tests (Tyszka et al., 2021). Further, the identification of foraminifera specimens to their lowest taxonomic rank could provide more detailed information about the mangrove sub-habitat of deposition. Cohen (1973) stated that insect parts have no trend in their depositional environments. However, our data show that insect parts are relatively common in basin peats, and seldom occur in fringe peats. Few research studies track the presence of insect parts in deep cores, and it is possible that these constituents do not survive the TAZ (Martı́nez-Delclòs et al., 2004).
The combined percentage of fecal pellets and other matrix categories in our short cores is very similar to the total matrix percentages reported by Cohen (1968). Cohen (1968) counted all fecal pellets, regardless of size, as matrix, and did not report the proportional abundance of fecal pellets with depth. This approach makes sense for studies of the peat-to-coal transition. Hower et al. (2011) identified coal macerals derived from invertebrate fecal pellets. As peat metamorphoses to coal, the boundaries of individual fecal pellets may disappear, making it difficult to distinguish the finely comminuted debris of the fecal pellet from the matrix. Although Cohen and Bailey (1997) reported that on average 6% of Rhizophora peat contains fecal pellets, they suggested that microbial decomposition may destroy fecal pellets in deeply buried peats. We found that the percentage of miscellaneous debris, which included unidentifiable constituents, increases with depth in the fringe site, but not in the basin site (Figure 3G). This may indicate different microbial decomposition pathways between frequently drained fringe peats as opposed to flooded basin peats.
Unlike Cohen (1968), we report fecal pellet abundance as a unique category because we observed that counting fecal pellets as matrix provides minimal information about the paleoecology of the peat and may inflate the percent of fine granular debris. The proportional abundance of fecal pellets in peat provides insight into detritivore-peat interactions, particularly with estimating the size of detritivores because fecal pellet size directly correlates with the body size of the tracemaker (Paffenhöfer and Knowles, 1979; Wotton and Malmqvist, 2001). In Australia, Camilleri (1992) reported that leaf-shredding crabs, amphipods, and isopods produced fecal pellets that ranged in size from 20 to 1,284 μm. In our South Florida peat samples, the average percent of fecal pellets within our cores from both sites was 11–16%, with most fecal pellets falling in the size category between 150 and 500 μm (Table 1). Although the proportional abundance of fecal pellets does not differ significantly between fringe and basin mangrove sub-habitats (Figure 3F), fecal pellets could provide useful ichnological information about the ecology of the TAZ in mangrove peats.
Applications for mangrove conservation
Current management strategies for mangrove restoration aim to improve coastal resiliency and promote the natural succession of coastal wetland habitats by restoring natural freshwater flow into Barnes Sound and other coastal environments in South Florida (Ishman et al., 1998; Gaiser et al., 2006; NOAA, 2019; USACE, 2019; USACE and USDOI, 2020; Wingard et al., 2022). Identification and delineation of coastal wetlands in sediment cores are crucial tasks for informing management strategies because these tasks provide the historical context necessary for monitoring environmental shifts and the responses of coastal wetlands to current climate change and anthropogenic impacts (Tiner, 2016), particularly in tracking the retreat or expansion of mangrove communities (Davies and Cohen, 1989; Alongi, 2015; Woodroffe et al., 2016). There are successes in localized efforts for mangrove restoration in Florida (USACE, 2019; USACE and USDOI, 2020). Krauss et al. (2011) reported mangrove coverage in the Ten Thousand Islands National Wildlife Refuge from 1927 to 2005 increased by 35%, attributing this increase to the construction of waterways that promoted propagule dispersal and establishment of mangroves. Because environmental factors create complex patterns in mangrove community structure, Kaly and Jones (1998) suggested that mangrove conservation should focus on integrative restoration strategies with well-defined aims that re-establish the self-sustainability of the entire mangrove ecosystem rather than restoration efforts that target precise ecosystem services, such as simply planting monospecific stands of mangroves to promote shoreline stabilization. Several recent studies echoed this call for more informed restoration strategies (Barnuevo et al., 2017; Lee et al., 2019; Gatt et al., 2022).
The plant organ- and taxon-based methods presented in this study demonstrate that historical data in peat from sediment cores provide expanded temporal scales for land managers to reconstruct precursor mangrove sub-habitats that allow them to delineate wetland habitats through time, which could be used to establish restoration targets for specific mangrove sub-habitats. Because accumulation and decomposition are critical components during the peat-forming process, the methods presented here allow for more informed interpretations of the depositional environments for sequestered historical peats. Further, the incorporation of these methods into coastal restoration goals could provide useful insights to understand the long-term effects of hurricanes and other natural disasters on peat collapse, carbon sequestration, and the rebound of functional mangal communities (Cahoon et al., 2003; Smith et al., 2009; Barr et al., 2012; Griffiths and Mitsch, 2021; Peneva-Reed et al., 2021). Although our study highlights mangroves by characterizing and deciphering mangrove sub-habitats from historical peats, the plant organ- and taxon-based methods described here can be applied to other peat-accumulating environments (see Cohen (1968) and Davies and Cohen (1989) for descriptions of other South Florida peats). The identification of distinct peat types thus provides insights into the spatial succession and zonation of plant communities in cores. Here, we applied a conservation paleobiology approach to the analysis of mangrove peats and developed methods to track changes within mangroves communities of South Florida:
1. Root percentages, or root–shoot ratios, in peats are reliable indicators of the relative rate of peat decomposition because roots are more resistant to decay than plant litter. We found that root percentages of surficial peats from fringe and basin mangrove sub-habitats are reflected in their respective sequestered peats, where fringe, or Rhizophora root, peats will have higher root percentages than the basin, or sedimentary, peats.
2. Accumulated leaf litter that overlies mangrove peats enables measurement of leaf mat thickness, which is a tool that can be used to indicate depositional environments that differ in surficial decomposition processes and accumulation rates. For example, we observed that thicker leaf mats accumulate in interior basin mangrove sub-habitats that are sheltered from tidal activity and storm surges. These accumulated leaf mats are likely transformed into sedimentary peats once they pass through the TAZ; thus, the presence of sedimentary peats in cores signals basin sub-habitats. The lack of leaf mats in fringe mangrove sub-habitats and the lower proportional abundance of leaf litter in their sequestered root peats may indicate more rapid decomposition of leaf litter and environments that are more susceptible to tidal scouring and coastal erosion.
3. To differentiate between mangrove sub-habitats in peat cores, we suggest tracking the proportional abundances of invertebrate communities, particularly insect parts and foraminifera. Although insect parts may disappear with depth in peat cores, foraminifera may dissolve into and persist as foraminiferal organic linings (Cohen, 1968). Foraminifera in sequestered peats thus enables us to distinguish basin from fringe peats: their presence indicates tidally influenced fringe mangrove sub-habitats, whereas their absence indicates basin sub-habitats.
Conclusion
We took a paleobotanical approach and developed novel techniques using peat cores to decipher changes in the distribution of mangroves through time that can be incorporated into management strategies and the long-term monitoring of mangrove ecosystems. We demonstrate that the TAZ model is appropriate to characterize taphonomic biases imposed on mangrove peat with depth, which provides insight into the paleoecological context of peat formation. This context allows us to make informed interpretations of sequestered peats and identify mangrove sub-habitats in cores. The identification of mangrove sub-habitats from cores is important because it provides conservation managers with historical evidence that can be used to develop targeted goals and informed management strategies for restoring functional mangrove wetlands within the broader coastal ecosystem and to anticipate and plan for future changes.
Data availability statement
The original contributions presented in the study are included in the article/supplementary material, further inquiries can be directed to the corresponding author.
Author contributions
SN and AR contributed to conception and design of the study. SN performed the statistical analysis and wrote the first draft of the manuscript. AR wrote sections of the manuscript. All authors contributed to manuscript revision, read, and approved the submitted version.
Funding
This research was partially supported by the Antoinette Lierman Medlin Award to SN from the Energy Division of the Geological Society of America.
Acknowledgments
Thank you to our reviewers and editor for the comments and suggestions that greatly improved the quality and clarity of this manuscript. We thank C. Belanger for insightful comments on statistical methods, and T. Solis for assisting with the processing of peat samples.
Conflict of interest
The authors declare that the research was conducted in the absence of any commercial or financial relationships that could be construed as a potential conflict of interest.
Publisher’s note
All claims expressed in this article are solely those of the authors and do not necessarily represent those of their affiliated organizations, or those of the publisher, the editors and the reviewers. Any product that may be evaluated in this article, or claim that may be made by its manufacturer, is not guaranteed or endorsed by the publisher.
References
Aller, R. C. (1982). Carbonate dissolution in nearshore terrigenous muds: the role of physical and biological reworking. J. Geol. 90, 79–95. doi: 10.1086/628652
Alongi, D. M. (2008). Mangrove forests: resilience, protection from tsunamis, and responses to global climate change. Estuar. Coast. Shelf Sci. 76, 1–13. doi: 10.1016/j.ecss.2007.08.024
Alongi, D. M. (2015). The impact of climate change on mangrove forests. Curr. Clim. Change Rep. 1, 30–39. doi: 10.1007/s40641-015-0002-x
Alongi, D. M. (2018). “Mangrove forests” in Blue Carbon. ed. D. M. Alongi (Berlin, Germany: Springer), 23–36.
Alongi, D. M. (2020). Global significance of mangrove blue carbon in climate change mitigation. Science 2:67. doi: 10.3390/sci2030067
Alongi, D. M. (2022). Impacts of climate change on blue carbon stocks and fluxes in mangrove forests. Forests 13:149. doi: 10.3390/f13020149
Anderson, J. A. R., and Muller, J. (1975). Palynological study of a Holocene peat and a Miocene coal deposit from NW Borneo. Rev. Palaeobot. Palynol. 19, 291–351. doi: 10.1016/0034-6667(75)90049-4
Barnuevo, A., Asaeda, T., Sanjaya, K., Kanesaka, Y., and Fortes, M. (2017). Drawbacks of mangrove rehabilitation schemes: lessons learned from the large-scale mangrove plantations. Estuar. Coast. Shelf Sci. 198, 432–437. doi: 10.1016/j.ecss.2017.02.015
Barr, J. G., Engel, V., Smith, T. J., and Fuentes, J. D. (2012). Hurricane disturbance and recovery of energy balance, CO2 fluxes and canopy structure in a mangrove forest of the Florida Everglades. Agric. For. Meteorol. 153, 54–66. doi: 10.1016/j.agrformet.2011.07.022
Benner, R., and Hodson, R. E. (1985). Microbial degradation of the leachable and lignocellulosic components of leaves and wood from Rhizophora mangle in a tropical mangrove swamp. Mar. Ecol. Prog. Ser. 23, 221–230. doi: 10.3354/meps023221
Benson, R. C., and Yuhr, L. B. (2016). “Site characterization along bridge alignment” in Site Characterization in Karst and Pseudokarst Terraines: Practical Strategies and Technology for Practicing Engineers, Hydrologists and Geologists. eds. R. C. Benson and L. B. Yuhr (Dordrecht: Springer), 365–384.
Boelter, D. H. (1969). Physical properties of peats as related to degree of decomposition. Soil Sci. Soc. Am. J. 33, 606–609. doi: 10.2136/sssaj1969.03615995003300040033x
Bouillon, S., Connolly, R. M., and Lee, S. Y. (2008). Organic matter exchange and cycling in mangrove ecosystems: recent insights from stable isotope studies. J. Sea Res. 59, 44–58. doi: 10.1016/j.seares.2007.05.001
Cahoon, D. R., Hensel, P., Rybczyk, J., McKee, K. L., Proffitt, C. E., and Perez, B. C. (2003). Mass tree mortality leads to mangrove peat collapse at Bay Islands, Honduras after Hurricane Mitch. J. Ecol. 91, 1093–1105. doi: 10.1046/j.1365-2745.2003.00841.x
Cahoon, D. R., Hensel, P. F., Spencer, T., Reed, D. J., McKee, K. L., and Saintilan, N. (2006). “Coastal wetland vulnerability to relative sea-level rise: wetland elevation trends and process controls” in Wetlands and Natural Resource Management Wetlands and Natural Resource Management. eds. J. T. A. Verhoeven, B. Beltman, R. Bobbink, and D. F. Whigham (Berlin, Heidelberg: Springer), 271–292.
Camilleri, J. C. (1992). Leaf litter processing by invertebrates in a mangrove forest in Queensland. Mar. Biol. 114, 139–145. doi: 10.1007/BF00350863
Canfield, D. E., and Thamdrup, B. (2009). Towards a consistent classification scheme for geochemical environments, or, why we wish the term ‘suboxic’ would go away. Geobiology 7, 385–392. doi: 10.1111/j.1472-4669.2009.00214.x
Castañeda-Moya, E., Rivera-Monroy, V. H., Chambers, R. M., Zhao, X., Lamb-Wotton, L., Gorsky, A., et al. (2020). Hurricanes fertilize mangrove forests in the Gulf of Mexico (Florida Everglades, USA). Proc. Natl. Acad. Sci. 117, 4831–4841. doi: 10.1073/pnas.1908597117
Chambers, L. G., Guevara, R., Boyer, J. N., Troxler, T. G., and Davis, S. E. (2016). Effects of salinity and inundation on microbial community structure and function in a mangrove peat soil. Wetlands 36, 361–371. doi: 10.1007/s13157-016-0745-8
Cintra, R. (1997). Leaf litter effects on seed and seedling predation of the palm Astrocaryum murumuru and the legume tree Dipteryx micrantha in Amazonian Forest. J. Trop. Ecol. 13, 709–725. doi: 10.1017/S0266467400010889
Clymo, R. S. (1984). The limits to peat bog growth. Philos. Trans. R. Soc. B 303, 605–654. doi: 10.1098/rstb.1984.0002
Clymo, R. S. (1987). Rainwater-fed peat as a precursor of coal. Geol. Soc. Lond. Spec. Publ. 32, 17–23. doi: 10.1144/GSL.SP.1987.032.01.03
Clymo, R. S. (1988). A high-resolution sampler of surface peat. Funct. Ecol. 2, 425–431. doi: 10.2307/2389416
Cohen, A. D. (1968). The Petrology of Some Peats of Southern Florida (With Special Reference to the Origin of Coal). Ph.D. Dissertation. State College, PA: Pennsylvania State University.
Cohen, A. D. (1973). Petrology of some Holocene peat sediments from the Okefenokee swamp-marsh complex of southern Georgia. Bull. Geol. Soc. Am. 84, 3867–3878. doi: 10.1130/0016-7606(1973)84%3C3867:POSHPS%3E2.0.CO;2
Cohen, A. D., and Bailey, A. M. (1997). Petrographic changes induced by artificial coalification of peat: comparison of two planar facies (Rhizophora and Cladium) from the Everglades-mangrove complex of Florida and a domed facies (Cyrilla) from the Okefenokee swamp of Georgia. Int. J. Coal Geol. 34, 163–194. doi: 10.1016/S0166-5162(97)00022-0
Cohen, A. D., and Spackman, W. (1972). Methods in peat petrology and their application to reconstruction of paleoenvironments. Geol. Soc. Am. Bull. 83, 129–142. doi: 10.1130/0016-7606(1972)83[129:MIPPAT]2.0.CO;2
Cohen, A. D., and Spackman, W. (1977). Phytogenic organic sediments and sedimentary environments in the Everglades-mangrove complex. II - the origin, description and classification of the peats of southern Florida. Palaeontographica 162, 71–114.
Cohen, A. D., Spackman, W., and Raymond, R. (1987). Interpreting the characteristics of coal seams from chemical, physical and petrographic studies of peat deposits. Geol. Soc. Lond. Spec. Publ. 32, 107–125. doi: 10.1144/GSL.SP.1987.032.01.08
Covington, D., and Raymond, A. (1989). Taxonomic uniformitarianism: the problem with shoot/root ratios of peats. Rev. Palaeobot. Palynol. 58, 85–94. doi: 10.1016/0034-6667(89)90058-4
Dahdouh-Guebas, F., and Koedam, N. (2008). Long-term retrospection on mangrove development using transdisciplinary approaches: a review. Aquat. Bot. 89, 80–92. doi: 10.1016/j.aquabot.2008.03.012
Dai, S., Bechtel, A., Eble, C. F., Flores, R. M., French, D., Graham, I. T., et al. (2020). Recognition of peat depositional environments in coal: a review. Int. J. Coal Geol. 219:103383. doi: 10.1016/j.coal.2019.103383
Daru, B. H., Yessoufou, K., Mankga, L. T., and Davies, T. J. (2013). A global trend towards the loss of evolutionarily unique species in mangrove ecosystems. PLoS One 8:e66686. doi: 10.1371/journal.pone.0066686
Davies, T. D., and Cohen, A. D. (1989). Composition and significance of the peat deposits of Florida Bay. Bull. Mar. Sci. 44, 387–398.
Davies, D. J., Powell, E. N., and Stanton, R. J. Jr. (1989). Relative rates of shell dissolution and net sediment accumulation—a commentary: can shell beds form by the gradual accumulation of biogenic debris on the sea floor? Lethaia 22, 207–212. doi: 10.1111/j.1502-3931.1989.tb01683.x
Davis, J. H. (1940). The ecology and geologic role of mangroves in Florida. Publications Carnegie Institution Washington 517, 303–412.
Davis, S. E. III, Cable, J. E., Childers, D. L., Coronado-Molina, C., Day, J. W. Jr., Hittle, C. D., et al. (2004). Importance of storm events in controlling ecosystem structure and function in a Florida gulf coast estuary. J. Coast. Res. 204, 1198–1208. doi: 10.2112/03-0072R.1
Day, J. W., Christian, R. R., Boesch, D. M., Yañez-Arancibia, A., Morris, J., Twilley, R. R., et al. (2008). Consequences of climate change on the ecogeomorphology of coastal wetlands. Estuar. Coasts 31, 477–491. doi: 10.1007/s12237-008-9047-6
Dietl, G. P., and Flessa, K. W. (2011). Conservation paleobiology: putting the dead to work. Trends Ecol. Evol. 26, 30–37. doi: 10.1016/j.tree.2010.09.010
Dietl, G. P., Kidwell, S. M., Brenner, M., Burney, D. A., Flessa, K. W., Jackson, S. T., et al. (2015). Conservation paleobiology: leveraging knowledge of the past to inform conservation and restoration. Annu. Rev. Earth Planet. Sci. 43, 79–103. doi: 10.1146/annurev-earth-040610-133349
DiMichele, W. A., and Phillips, T. L. (1994). Paleobotanical and paleoecological constraints on models of peat formation in the late carboniferous of Euamerica. Palaeogeogr. Palaeoclimatol. Palaeoecol. 106, 39–90. doi: 10.1016/0031-0182(94)90004-3
Drzymulska, D. (2016). Peat decomposition–shaping factors, significance in environmental studies and methods of determination; a literature review. Andean Geol. 22, 61–69. doi: 10.1515/logos-2016-0005
Duke, N. C. (1992). “Mangrove floristics and biogeography” in Tropical Mangrove Ecosystems. eds. A. I. Robertson and D. M. Alongi (Washington, DC: American Geophysical Union), 63–100.
Duke, N. C. (2017). “Mangrove floristics and biogeography revisited: further deductions from biodiversity hot spots, ancestral discontinuities, and common evolutionary processes” in Mangrove Ecosystems: A Global Biogeographic Perspective. eds. V. Rivera-Monroy, S. Lee, E. Kristensen, and R. Twilley (Cham: Springer)
Ellison, J. C. (2008). Long-term retrospection on mangrove development using sediment cores and pollen analysis: a review. Aquat. Bot. 89, 93–104. doi: 10.1016/j.aquabot.2008.02.007
Esterle, J. S., Ferm, J. C., and Yiu-Liong, T. (1989). A test for the analogy of tropical domed peat deposits to “dulling up” sequences in coal beds—preliminary results. Org. Geochem. 14, 333–342. doi: 10.1016/0146-6380(89)90060-0
Ewel, K. C., Twilley, R. R., and Ong, J. E. (1998). Different kinds of mangrove forests provide different goods and services. Glob. Ecol. Biogeogr. 7, 83–94. doi: 10.1111/j.1466-8238.1998.00275.x
Ezcurra, P., Ezcurra, E., Garcillán, P. P., Costa, M. T., and Aburto-Oropeza, O. (2016). Coastal landforms and accumulation of mangrove peat increase carbon sequestration and storage. Proc. Natl. Acad. Sci. 113, 4404–4409. doi: 10.1073/pnas.1519774113
Fell, J. W., Cefalu, R. C., Master, I. M., and Tallman, A. S. (1975). “Microbial activities in the mangrove (Rhizophora mangle) leaf detrital system” in Proceedings of the International Symposium on the Biology and Management of Mangroves. eds. G. E. Walsh, S. C. Snedaker, and H. J. Teas (Gainesville, FL: Institute of Food and Agricultural Sciences), 661–679.
Fiorini, F., Lokier, S. K., Garrison, T., and Kaminski, M. A. (2019). Agglutinated foraminifera from recent mangrove environments of the United Arab Emirates (UAE). Micropaleontology 65, 301–304. doi: 10.47894/mpal.65.4.03
Fleming, M., Lin, G., and Sternberg, L. (1990). Influence of mangrove detritus in an estuarine ecosystem. Bull. Mar. Sci. 47, 663–669.
Friess, D. A., Rogers, K., Lovelock, C. E., Krauss, K. W., Hamilton, S. E., Lee, S. Y., et al. (2019). The state of the world’s mangrove forests: past, present, and future. Annu. Rev. Environ. Resour. 44, 89–115. doi: 10.1146/annurev-environ-101718-033302
Gaiser, E. E., Zafiris, A., Ruiz, P. L., Tobias, F. A., and Ross, M. S. (2006). Tracking rates of ecotone migration due to salt-water encroachment using fossil mollusks in coastal South Florida. Hydrobiologia 569, 237–257. doi: 10.1007/s10750-006-0135-y
Garbin, E., and Mann, J. A. (2010). Mass stabilization for environmentally sensitive projects in Florida. Transp. Res. Rec. 2201, 62–69. doi: 10.3141/2201-08
Gastaldo, R. A., and Staub, J. R. (1999). A mechanism to explain the preservation of leaf litter lenses in coals derived from raised mires. Paleogeogr. Palaeoclimatol. Palaeoecol. 149, 1–14. doi: 10.1016/S0031-0182(98)00188-6
Gatt, Y. M., Andradi-Brown, D. A., Ahmadia, G. N., Martin, P. A., Sutherland, W. J., Spalding, M. D., et al. (2022). Quantifying the reporting, coverage and consistency of key indicators in mangrove restoration projects. Front. For. Glob. Change 5:720394. doi: 10.3389/ffgc.2022.720394
Gladstone-Gallagher, R. V., Lundquist, C. J., and Pilditch, C. A. (2014). Mangrove (Avicennia marina subsp. australasica) litter production and decomposition in a temperate estuary. N. Z. J. Mar. Freshw. Res. 48, 24–37. doi: 10.1080/00288330.2013.827124
Griffiths, L. N., and Mitsch, W. J. (2021). Estimating the effects of a hurricane on carbon storage in mangrove wetlands in Southwest Florida. Plan. Theory 10:1749. doi: 10.3390/plants10081749
Haines, E. B., and Montague, C. L. (1979). Food sources of estuarine invertebrates analyzed using 13C/12C ratios. Ecology 60, 48–56. doi: 10.2307/1936467
Hower, J. C., O’Keefe, J. M. K., Eble, C. F., Raymond, A., Valentim, B., Volk, T. J., et al. (2011). Notes on the origin of inertinite macerals in coal: evidence for fungal and arthropod transformations of degraded macerals. Int. J. Coal Geol. 86, 231–240. doi: 10.1016/j.coal.2011.02.005
Hoyos-Santillan, J., Lomax, B., Large, D., Turner, B., Boom, A., Lopez, O., et al. (2015). Getting to the root of the problem: litter decomposition and peat formation in lowland Neotropical peatlands. Biogeochemistry 126, 115–129. doi: 10.1007/s10533-015-0147-7
Ishman, S. E., Cronin, T. M., Brewster-Wingard, G. L., Willard, D. A., and Verardo, D. J. (1998). A record of ecosystem change. Manatee Bay, Barnes, Sound, Florida. J. Coastal Res. 26, 125–138.
Jones, M. C., Wingard, G. L., Stackhouse, B., Keller, K., Willard, D., Marot, M., et al. (2019). Rapid inundation of southern Florida coastline despite low relative sea-level rise rates during the late-Holocene. Nat. Commun. 10:3231. doi: 10.1038/s41467-019-11138-4
Kaly, U. L., and Jones, G. P. (1998). Mangrove restoration: a potential tool for coastal management in tropical developing countries. Ambio 27, 656–661.
Kathiresan, K., and Bingham, B. L. (2001). Biology of mangroves and mangrove ecosystems. Adv. Mar. Biol. 40, 81–251. doi: 10.1016/S0065-2881(01)40003-4
Kent, M., and Coker, P. (2011). Vegetation Description and Data Analysis: A Practical Approach. Hoboken, NJ: John Wiley & Sons, Incorporated.
Kleinebecker, T., Hölzel, N., and Vogel, A. (2007). Gradients of continentality and moisture in South Patagonian ombrotrophic peatland vegetation. Folia Geobot. 42, 363–382. doi: 10.1007/BF02861700
Koh, H. L., Teh, S. Y., Kh’ng, X. Y., and Raja Barizan, R. S. (2018). Mangrove forests: protection against and resilience to coastal disturbances. J. Trop. For. Sci. 30, 446–460. doi: 10.26525/jtfs2018.30.5.446460
Krauss, K. W., From, A. S., Doyle, T. W., Doyle, T. J., and Barry, M. J. (2011). Sea-level rise and landscape change influence mangrove encroachment onto marsh in the Ten Thousand Islands region of Florida, USA. J. Coast. Conserv. 15, 629–638. doi: 10.1007/s11852-011-0153-4
Krauss, K. W., McKee, K. L., Lovelock, C. E., Cahoon, D. R., Saintilan, N., Reef, R., et al. (2014). How mangrove forests adjust to rising sea level. New Phytol. 202, 19–34. doi: 10.1111/nph.12605
Kristensen, J. (2008). Mangrove crabs as ecosystem engineers; with emphasis on sediment processes. J. Sea Res. 59, 30–43. doi: 10.1016/j.seares.2007.05.004
Lavelle, P., Blanchart, E., Martin, A., and Martin, S. (1993). A hierarchical model for decomposition in terrestrial ecosystems: application to soils of the humid tropics. Biotropica 25, 130–150. doi: 10.2307/2389178
Lee, S. Y., Hamilton, S., Barbier, E. B., Primavera, J., and Lewis, R. R. (2019). Better restoration policies are needed to conserve mangrove ecosystems. Nat. Ecol. Evol. 3, 870–872. doi: 10.1038/s41559-019-0861-y
Levesque, M., and Dinel, H. (1977). Fiber content, particle-size distribution and some related properties of four peat materials in eastern Canada. Can. J. Soil Sci. 57, 187–195. doi: 10.4141/cjss77-023
Lewis, R. R. (2001). Mangrove restoration—costs and benefits of successful restoration. Proceedings Mangrove Valuation Workshop, Universiti Sains Malaysia, Penang, Penang, Malaysia.
Lugo, A. E., and Snedaker, S. C. (1974). The ecology of mangroves. Annu. Rev. Ecol. Syst. 5, 39–64. doi: 10.1146/annurev.es.05.110174.000351
Luther, D. A., and Greenberg, R. (2009). Mangroves: a global perspective on the evolution and conservation of their terrestrial vertebrates. Bioscience 59, 602–612. doi: 10.1525/bio.2009.59.7.11
Martı́nez-Delclòs, X., Briggs, D. E. G., and Peñalver, E. (2004). Taphonomy of insects in carbonates and amber. Palaeogeogr. Palaeoclimatol. Palaeoecol. 203, 19–64. doi: 10.1016/S0031-0182(03)00643-6
McCune, B., Grace, J. B., and Urban, D. L. (2002). Analysis of Ecological Communities. Gleneden Beach, OR: MjM Software Design.
McKee, K. L., Cahoon, D. R., and Feller, I. C. (2007). Caribbean mangroves adjust to rising sea level through biotic controls on change in soil elevation. Glob. Ecol. Biogeogr 16, 545–556. doi: 10.1111/j.1466-8238.2007.00317.x
McKee, K. L., and Faulkner, P. (2000). Restoration of biogeochemical function in mangrove forests. Restor. Ecol. 8, 247–259. doi: 10.1046/j.1526-100x.2000.80036.x
Meeder, J. F., Parkinson, R. W., Ogurcak, D., Ross, M. S., and Kominoski, J. S. (2021). Changes in sediment organic carbon accumulation under conditions of historical sea-level rise, Southeast Saline Everglades, Florida, USA. Wetlands 41, 1–12. doi: 10.1007/s13157-021-01440-7
Middleton, B. A., and McKee, K. L. (2001). Degradation of mangrove tissues and implications for peat formation in Belizean island forests. J. Ecol. 89, 818–828. doi: 10.1046/j.0022-0477.2001.00602.x
Nagelkerken, I., Blaber, S. J. M., Bouillon, S., Green, P., Haywood, M., Kirton, L. G., et al. (2008). The habitat function of mangroves for terrestrial and marine fauna: a review. Aquat. Bot. 89, 155–185. doi: 10.1016/j.aquabot.2007.12.007
NOAA (2019). 2015–2020 Biscayne Bay Habitat Focus Area Implementation Plan. Biscayne Bay Habitat Focus Area. Available at: https://www.habitatblueprint.noaa.gov/habitat-focus-areas/biscayne-bay-florida/
Noto, C. R. (2011). “Hierarchical control of terrestrial vertebrate taphonomy over space and time: discussion of mechanisms and implications for vertebrate paleobiology” in Taphonomy: Process and Bias Through Time. eds. P. A. Allison and D. J. Bottjer (Dordrecht: Springer), 287–336. doi: 10.1007/978-90-481-8643-3_8
Odum, W. E., and Heald, E. J. (1975). “Mangrove forests and aquatic productivity” in Coupling of Land and Water System. ed. A. D. Hasler (New York, NY: Springer), 129–136. doi: 10.1007/978-3-642-86011-9_5
Oksanen, J., Blanchet, F. G., Friendly, M., Kindt, R., Legendre, P., McGlinn, D., et al. (2020). vegan: Community Ecology Package. R package version 2.5-7.
Olszewski, T. D. (1999). Taking advantage of time-averaging. Paleobiology 25, 226–238. doi: 10.1017/S009483730002652X
Olszewski, T. D. (2004). Modeling the influence of taphonomic destruction, reworking, and burial on time-averaging in fossil accumulations. Palaios 19, 39–50. doi: 10.1669/0883-1351
Ong, W. J., and Ellison, J. C. (2021). “A framework for the quantitative assessment of mangrove resilience” in Dynamic Sedimentary Environments of Mangrove Coasts. eds. F. Sidik and D. A. Friess (Cambridge, MA: Elsevier), 513–538.
Paffenhöfer, G. A., and Knowles, S. C. (1979). Ecological implications of fecal pellet size, production and consumption by copepods. J. Mar. Res. 37, 35–49.
Parkinson, R. W., DeLaune, R. D., and White, J. R. (1994). Holocene sea-level rise and the fate of mangrove forests within the wider Caribbean region. J. Coast. Res. 10, 1077–1086.
Peneva-Reed, E. I., Krauss, K. W., Bullock, E. L., Zhu, Z., Woltz, V. L., Drexler, J. Z., et al. (2021). Carbon stock losses and recovery observed for a mangrove ecosystem following a major hurricane in Southwest Florida. Estuar. Coast. Shelf Sci. 248:106750. doi: 10.1016/j.ecss.2020.106750
Petró, S. M., do Nascimento Ritter, M. A. T. I. A. S., Pivel, M. A. G., and Coimbra, J. C. (2018). Surviving in the water column: defining the taphonomically active zone in pelagic systems. Palaios 33, 85–93. doi: 10.2110/palo.2017.032
Primavera, J. H. (1998). Mangroves as nurseries: shrimp populations in mangrove and non-mangrove habitats. Estuar. Coast. Shelf Sci. 46, 457–464. doi: 10.1006/ecss.1997.0275
Proffitt, C. E., and Devlin, D. J. (2005). Grazing by the intertidal gastropod Melampus coffeus greatly increases mangrove leaf litter degradation rates. Mar. Ecol. Prog. Ser. 296, 209–218. doi: 10.3354/meps296209
Proffitt, C. E., Johns, K. M., Cochrane, C. B., Devlin, D. J., Reynolds, T. A., Payne, D. L., et al. (1993). Field and laboratory experiments on the consumption of mangrove leaf litter by the macrodetritivore Melampus coffeus L. (Gastropoda: Pulmonata). Florida Sci. 56, 211–222.
R Core Team (2021). R: A Language and Environment for Statistical Computing. R Foundation for Statistical Computing: Vienna, Austria.
Raymond, A. (1987). Interpreting ancient swamp communities: can we see the forest in the peat? Rev. Palaeobot. Palynol. 52, 217–231. doi: 10.1016/0034-6667(87)90055-8
Raymond, A. (1988). The paleoecology of a coal-ball deposit from the Middle Pennsylvanian of Iowa dominated by cordaitalean gymnosperms. Rev. Palaeobot. Palynol. 53, 233–250. doi: 10.1016/0034-6667(88)90034-6
Raymond, A., Cutlip, P., and Sweet, M. (2001). “Rates and processes of terrestrial nutrient-cycling in the Paleozoic: the world before beetles, termites and flies” in Evolutionary Paleoecology. eds. W. Allmon and D. Bottier (New York, NY: Columbia University Press), 235–283. doi: 10.7312/allm10994-012
Raymond, A., Guillemette, R., Jones, C. P., and Ahr, W. M. (2012). Carbonate petrology and geochemistry of Pennsylvanian coal balls from the Kalo formation of Iowa. Int. J. Coal Geol. 94, 137–149. doi: 10.1016/j.coal.2012.01.007
Raymond, A., Lambert, L. L., Costanza, S. H., Slone, E. J., and Cutlip, P. C. (2010). Cordaiteans in paleotropical wetlands: an ecological re-evaluation. Int. J. Coal Geol. 83, 248–265. doi: 10.1016/j.coal.2009.10.009
Reis, C. R. G., Nardoto, G. B., Rochelle, A. L. C., Vieira, S. A., and Oliveira, R. S. (2017). Nitrogen dynamics in subtropical fringe and basin mangrove forests inferred from stable isotopes. Oecologia 183, 841–848. doi: 10.1007/s00442-016-3789-9
Risi, J. A., Wanless, H. R., Tedesco, L. P., and Gelsanliter, S. (1995). Catastrophic sedimentation from Hurricane Andrew along the Southwest Florida coast. J. Coast. Res. 21, 83–102.
Rivera-Monroy, V. H., Day, J. W., Twilley, R. R., Vera-Herrera, F., and Coronado-Molina, C. (1995). Flux of nitrogen and sediment in a fringe mangrove forest in Terminos Lagoon, Mexico. Estuar. Coast. Shelf Sci. 40, 139–160. doi: 10.1016/S0272-7714(05)80002-2
Ross, M. S., Meeder, J. F., Sah, J. P., Ruiz, P. L., and Telesnicki, G. J. (2000). The southeast saline Everglades revisited: 50 years of coastal vegetation change. J. Veg. Sci. 11, 101–112. doi: 10.2307/3236781
Sadler, P. M. (1993). “Models of time-averaging as a maturation process: how soon do sedimentary sections escape reworking?” in Taphonomic Approaches to Time Resolution in Fossil Assemblages. Short Courses in Paleontology. eds. S. M. Kidwell and A. K. Behrensmeyer (Knoxville, TN: Paleontological Society), 188–209.
Santonja, M., Pellan, L., and Piscart, C. (2018). Macroinvertebrate identity mediates the effects of litter quality and microbial conditioning on leaf litter recycling in temperate streams. Ecol. Evol. 8, 2542–2553. doi: 10.1002/ece3.3790
Savarese, M. (2018). “Effectively connecting conservation paleobiological research to environmental management: examples from Greater Everglades’ restoration of Southwest Florida”, In Marine Conservation Paleobiology, Eds. C. Tyler and C. Schneider, Springer: Cham, 55–73.
Scheihing, M. H., and Pfefferkorn, H. W. (1984). The taphonomy of land plants in the Orinoco Delta: a model for the incorporation of plant parts in clastic sediments of late carboniferous age of Euramerica. Rev. Palaeobot. Palynol. 41, 205–240. doi: 10.1016/0034-6667(84)90047-2
Schoenhut, K., Vann, D. R., and LePage, B. A. (2004). Cytological and ultrastructural preservation in Eocene Metasequoia leaves from the Canadian high Arctic. Am. J. Bot. 91, 816–824. doi: 10.3732/ajb.91.6.816
Schultz, E. (2015). Characterizing the taphonomically active zone in subtropical peat from Barnes Sound in Key Largo, Florida. Master’s Thesis. College Station, TX: Texas: A&M University.
Sharon,, Belanger, C., du, J., and Mix, A. (2021). Reconstructing paleooxygenation for the last 54,000 years in the Gulf of Alaska using cross-validated benthic foraminiferal and geochemical records. Paleoceanogr. Paleoclimatol. 36:e2020PA003986. doi: 10.1029/2020PA003986
Slater, B. J., McLoughlin, S., and Hilton, J. (2015). A high-latitude Gondwanan lagerstätte: the Permian permineralised peat biota of the Prince Charles Mountains, Antarctica. Gondwana Res. 27, 1446–1473. doi: 10.1016/j.gr.2014.01.004
Smith, N. P. (2001). Tides of Biscayne Bay, card sound, Barnes Sound, and Manatee Bay, Florida. Florida Sci. 64, 224–236.
Smith, T. J., Anderson, G. H., Balentine, K., Tiling, G., Ward, G. A., and Whelan, K. R. T. (2009). Cumulative impacts of hurricanes on Florida mangrove ecosystems: sediment deposition, storm surges and vegetation. Wetlands 29, 24–34. doi: 10.1672/08-40.1
Smith, T. J., Foster, A. M., Tiling-Range, G., and Jones, J. W. (2013). Dynamics of mangrove-marsh ecotones in subtropical coastal wetlands: fire, sea-level rise, and water levels. Fire Ecol. 9, 66–77. doi: 10.4996/fireecology.0901066
Snedaker, S. S. (1989). Overview of ecology of mangroves and information needs for Florida Bay. Bulletin of Marine Science 44, 341–347.
Snigirevskaya, N. S. (1972). Studies of coal balls of the Donets Basin. Rev. Palaeobot. Palynol. 14, 197–204. doi: 10.1016/0034-6667(72)90019-X
Swift, M. J., Heal, O. W., Anderson, J. M., and Anderson, J. M. (1979). Decomposition in Terrestrial Ecosystems. Berkeley, CA: University of California Press.
Teas, H. J. (1977). Ecology and restoration of mangrove shorelines in Florida. Environ. Conserv. 4, 51–58. doi: 10.1017/S0376892900025042
Thomas, B. A., and Cleal, C. J. (2015). Cyclones and the formation of plant beds in late carboniferous tropical swamps. Palaeobiodivers. Palaeoenviron. 95, 531–536. doi: 10.1007/s12549-015-0191-2
Tiner, R. W. (2016). Wetland Indicators: A Guide to Wetland Formation, Identification, Delineation, Classification, and Mapping. Boca Raton, FL: CRC Press.
Tomašových, A., Kidwell, S. M., Alexander, C. R., and Kaufman, D. S. (2019). Millennial-scale age offsets within fossil assemblages: result of bioturbation below the taphonomic active zone and out-of-phase production. Paleoceanogr. Paleoclimatol. 34, 954–977. doi: 10.1029/2018PA003553
Tomašových, A., Kidwell, S. M., Barber, R. F., and Kaufman, D. S. (2014). Long-term accumulation of carbonate shells reflects a 100-fold drop in loss rate. Geology 42, 819–822. doi: 10.1130/G35694.1
Twilley, R. W., Lugo, A. E., and Patterson-Zuc, C. (1986). Litter production and turnover in basin mangrove forests in Southwest Florida. Ecology 67, 670–683. doi: 10.2307/1937691
Tyszka, J., Godos, K., Goleń, J., and Radmacher, W. (2021). Foraminiferal organic linings: functional and phylogenetic challenges. Earth Sci. Rev. 220:103726. doi: 10.1016/j.earscirev.2021.103726
Urrego, L. E., Bernal, G., and Polanía, J. (2009). Comparison of pollen distribution patterns in surface sediments of a Colombian Caribbean mangrove with geomorphology and vegetation. Rev. Palaeobot. Palynol. 156, 358–375. doi: 10.1016/j.revpalbo.2009.04.004
USACE (2019). 2019 Everglades System Status Report: Assessment Period of 2012–2017. Comprehensive Everglades Restoration Plan, Restoration Coordination and Verification (RECOVER) Program Report to Congress. Available at: https://www.saj.usace.army.mil/Missions/Environmental/Ecosystem-Restoration/RECOVER/2019-System-Status-Report/
USACE and USDOI (2020). Biscayne Bay and Southeastern Everglades Ecosystem Restoration Project Management Plan (PMP). Biscayne Bay and Southeastern Everglades Ecosystem Restoration (BBSEER) Project. Available at: https://www.saj.usace.army.mil/BBSEER/
Venables, W. N., and Ripley, B. D. (2002). Modern Applied Statistics With S. New York, NY: Springer.
Walker, S. E., and Goldstein, S. T. (1999). Taphonomic tiering: experimental field taphonomy of molluscs and foraminifera above and below the sediment-water interface. Palaeogeogr. Palaeoclimatol. Palaeoecol. 149, 227–244. doi: 10.1016/S0031-0182(98)00203-X
Wanless, H. R. (1974). “Mangrove sedimentation in geological perspective” in Environments of South Florida: Present and Past, Memoir 2. ed. P. J. Gleason (Miami, FL: Miami Geological Society), 190–200.
Whelan, R. T., Smith, T. J., Anderson, G. H., and Ouellette, M. L. (2009). Hurricane Wilma’s impact on overall soil elevation and zones within the soil profile in a mangrove forest. Wetlands 29, 16–23. doi: 10.1672/08-125.1
Willard, D. A., and Bernhardt, C. E. (2011). Impacts of past climate and sea level change on Everglades wetlands: placing a century of anthropogenic change into a late-Holocene context. Clim. Chang. 107, 59–80. doi: 10.1007/s10584-011-0078-9
Wingard, G. L., Bergstresser, S. E., Stackhouse, B. L., Jones, M. C., Marot, M. E., Hoefke, K., et al. (2020). Impacts of Hurricane Irma on Florida Bay Islands, Everglades National Park, USA. Estuar. Coasts 43, 1070–1089. doi: 10.1007/s12237-019-00638-7
Wingard, G. L., Stackhouse, B. L., and Daniels, A. M. (2022). Using mollusks as indicators of restoration in nearshore zones of South Florida’s estuaries. Bull. Mar. Sci. 98, 351–380. doi: 10.5343/bms.2022.0004
Wnuk, C., and Pfefferkorn, H. W. (1987). A Pennsylvanian-age terrestrial storm deposit; using plant fossils to characterize the history and process of sediment accumulation. J. Sediment. Res. 57, 212–221. doi: 10.1306/212F8AE9-2B24-11D7-8648000102C1865D
Woodroffe, C. D. (1983). “Development of mangrove forests from a geological perspective” in Biology and Ecology of Mangroves. ed. H. J. Teas (Dordrecht: Springer), 1–17.
Woodroffe, C. D. (1992). “Mangrove sediments and geomorphology” in Tropical Mangrove Ecosystems: Coastal and Estuarine Studies. eds. A. I. Robertson and D. M. Alongi (Washington, DC: American Geophysical Union), 7–41. doi: 10.1029/CE041p0007
Woodroffe, S. A., Horton, B. P., Larcombe, P., and Whittaker, J. E. (2005). Intertidal mangrove foraminifera from the central great barrier Reef shelf, Australia: implications for sea-level reconstruction. J. Foraminifer. Res. 35, 259–270. doi: 10.2113/35.3.259
Woodroffe, C. D., Rogers, K., McKee, K. L., Lovelock, C. E., Mendelssohn, I. A., and Saintilan, N. (2016). Mangrove sedimentation and response to relative sea-level rise. Annu. Rev. Mar. Sci. 8, 243–266. doi: 10.1146/annurev-marine-122414-034025
Wotton, R. S., and Malmqvist, B. (2001). Feces in aquatic ecosystems: feeding animals transform organic matter into fecal pellets, which sink or are transported horizontally by currents; these fluxes relocate organic matter in aquatic ecosystems. Bioscience 51, 537–544. doi: 10.1641/0006-3568(2001)051[0537:FIAE]2.0.CO;2
Zhao, X., Rivera-Monroy, V. H., Farfán, L. M., Briceño, H., Castañeda-Moya, E., Travieso, R., et al. (2021). Tropical cyclones cumulatively control regional carbon fluxes in Everglades mangrove wetlands (Florida, USA). Sci. Rep. 11:13927. doi: 10.1038/s41598-021-92899-1
Keywords: taphonomically active zone, mangrove conservation, peat formation, coastal resilience, sea-level rise, leaf mat thickness
Citation: Neely SH and Raymond A (2023) The influence of the taphonomically active zone on peat formation: Establishing modern peat analogs to decipher mangrove sub-habitats from historical peats. Front. Ecol. Evol. 11:981537. doi: 10.3389/fevo.2023.981537
Edited by:
G. Lynn Wingard, United States Geological Survey (USGS), United StatesReviewed by:
Carlos A. Coronado, South Florida Water Management District, United StatesMiriam Jones, United States Geological Survey (USGS), United States
Copyright © 2023 Neely and Raymond. This is an open-access article distributed under the terms of the Creative Commons Attribution License (CC BY). The use, distribution or reproduction in other forums is permitted, provided the original author(s) and the copyright owner(s) are credited and that the original publication in this journal is cited, in accordance with accepted academic practice. No use, distribution or reproduction is permitted which does not comply with these terms.
*Correspondence: Samuel H. Neely, ✉ c2FtdWVsaG5lZWx5QHRhbXUuZWR1