- Biological Sciences, School of Natural Sciences. University of Hull, Hull, United Kingdom
Chemosensory science, the study of how organisms produce and assess olfactory information, is central to our understanding of how organisms interact and gain information about their environment. Signaling cue identification in aquatic systems lags behind our knowledge in terrestrial insects due to analytical challenges in aqueous environments. Unambiguous, reliable, and fast behavioral assays to evaluate the biological activity and function of a chemosensory cue are critical to understand aquatic signaling systems and enable research into their ecology, evolution, and threats in a changing environment. Yet, a range of anthropomorphic assumptions made in this research field create additional challenges to interpret data generated. Here, we evaluate common challenges including assumed readiness of individuals to respond, lack of information on the animals’ physiological and social status, their pre-experimental cue exposure, the innate or learned character of the responses, the animals’ acclimation and habituation status, and the impact of the animals upon their own environment. These factors lead to significant variability in animals’ responses in bioassays, both in the field and in laboratory setups. In the light of our limited knowledge of aquatic chemosensory cues’ chemical structure, active concentrations in samples, and undetermined response thresholds, we evaluate methods of mitigation to minimize differences between studies. We conclude that currently it is nearly impossible to compare results from chemosensory behavioral studies undertaken in different ecosystems, laboratories, and time points. There is an urgent need for the standardization of behavioral methods, recording of environmental conditions, and individuals’ physiology, physical, and social status, to avoid conflicting and contradicting results when comparing studies. Including these parameters in experimental design and data interpretation will provide a deeper understanding of chemosensory communication, reduce unconscious bias in studies, and can help to explain the substantial individuality in animals’ responses to chemosensory cues and their acclimation to environmental stress.
Introduction
The study of animal chemosensory systems has a long-standing tradition. Humans have been fascinated with how animals communicate, but the chemical nature of the compounds used has proved elusive until the late 1950s, when Nobel laureate Butenandt’s group (Butenandt et al., 1959) achieved the purification of Bombykol from the silkworm moth Bombyx mori. Chemical signals are known as the oldest and most widespread form of communication and were postulated as the “language of life” (Wyatt, 2010; Wyatt, 2014). Interestingly, our knowledge of chemosensory cues in aquatic environments is still in its infancy, despite being commonly found in aquatic habitats, where it can be used to overcome the challenges of complex and dynamic environments. This includes, e.g., reduced light levels, turbid water, vision, and obscuring vegetation (Bublitz et al., 2008), particularly in nocturnal organisms, such as crustaceans, where most behaviors and animal interactions occur in darkness (Hayden et al., 2007). To date, many chemosensory cue compounds have been identified in insects and terrestrial vertebrates, often driven by the replacement of traditional pesticides/insecticides using natural cues in integrated pest management (Witzgall et al., 2010).
In recent years, technological advances in the chemical analysis of unknown cues using mass spectrometry (GC-MS/MS, LC-MS/MS) and nuclear magnetic resonance spectroscopy (NMR) and new solid phase extraction (SPE; Li et al., 2018) have laid the foundations for a major increase in our knowledge of chemical signals. In addition, metabolomics and genomic molecular tools have enabled linking odor profiles with metabolic state, fitness, and health status in animals (Kimball et al., 2016). Major advances in research of chemoreceptors and their evolution have shed light on the cellular mechanisms of odor reception and transmission. This enabled the linking of odor profiles with animal physiology and the expression of different types of receptors, including gustatory receptors (GRs), odorant receptors (ORs), and ionotropic receptors (IRs), in a diverse range of organisms, terrestrial and marine ones (see review by Mollo et al., 2022). Consequently, the evolution of ORs has been hypothesized to be associated with insect’s colonization of land through enabling the detection of volatile compounds in air (Mollo et al., 2014). It should be noted here that some of the ambiguity and anthropomorphic bias in chemosensory science could be related to the fact that the dichotomy, odor (smell) vs. gustation(taste), is quite different from the vision that we have as humans. The detection of chemical stimuli involves a complex interplay between receptors and ligands that varies between receptor types and in different environmental conditions.
Odor profiles are used for the development of sensor-driven applications (Brattoli et al., 2011) in ecotoxicology, and research on environmental change (Roggatz et al., 2022), including “olfactory disruption” through Ocean Acidification (OA) (Briffa et al., 2012; Schirrmacher et al., 2021).
While there is now increasing evidence for the use of chemical signals both for distance signaling (smell and odor) and for the sense of taste (gustation) in marine systems, our understanding of the chemical nature of the cues involved continues to be far behind our understanding of insect, vertebrate, and microbial cues (Wyatt, 2014). Despite the technological advances, the challenges associated with purification of olfactory cues from saltwater (with cues often occurring in very low concentrations) are still significant. Key to progress in the marine environment is the development of unambiguous and fast behavioral assays (Hardege and Terschak, 2011), especially when complex, small changes upon signaling in cue-controlled behavior are to be examined, such as impacts of pH or toxicants (Porteus et al., 2021; Roggatz et al., 2022). The complexity of interpretation of small changes to animal behavior is evident in the recent controversial discussion on the effects of OA upon olfactory systems (Clements et al., 2022; Munday, 2022; Esbaugh, 2023). While it is widely accepted that pH impacts and often alters animal behavior (Clements and Hunt, 2015; Draper and Weissburg, 2019), there exists a significant repeatability problem of previous studies on fish olfaction and OA that is at least in part based on difficulties in the interpretation of animal behavior and the lack of quantifiable cues (Porteus et al., 2021; Roggatz et al., 2022; Esbaugh, 2023).
Here, we review existing literature on factors impacting animal behavior and responses to chemosensory cues (Figure 1), and evaluate common methodological challenges and potential pitfalls in behavioral studies, aiming to help the development of standardized methodology and interpretation of chemosensory behavior in aquatic systems. We also use examples from marine chemosensory research using crustaceans and polychaete worms as models to suggest ways to mitigate and to “de-anthropomorph” this type of research.
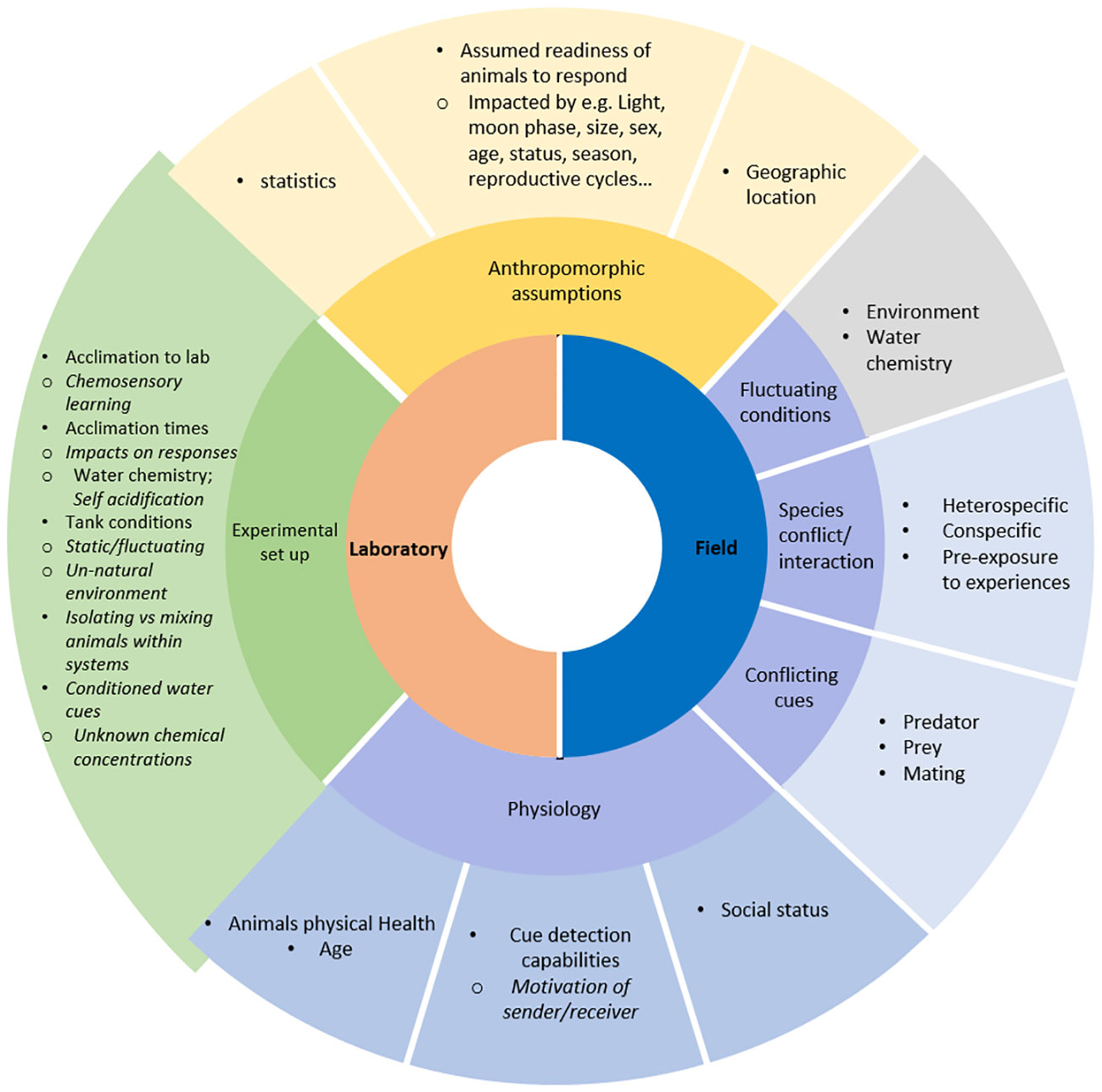
Figure 1 Factors that influence animal behavior in chemosensory assays, indicating factors for field and laboratory experiments, biotic and abiotic factors, and those impacting bioassays and affecting animal physiology pre-behavioral studies.
Methods
Hediste (Nereis) diversicolor culture
Hediste (Nereis) diversicolor ragworms were hand collected on the shores of the Humber Estuary (53.7144° N, 0.4458° W) and transported to the aquariums at the University of Hull. The animals were maintained in flow-through seawater aquariums measuring 75 cm × 150 cm each, kept at a salinity of 20–21 ppt and a temperature of 14°C and exposed to ambient photoperiod regimes. The water in the system was pH adjusted to mimic diurnal fluctuations using the slow inflow of CO2 controlled by Lolligo system capCTRL software (pH 8.2 in the day and pH 7.9 at night). They were fed on crushed Tetra Tabimin 3 × weekly and starved 3 days prior to bioassays. For the bioassays, N. diversicolor (n = 20) were transferred to individual beakers (diameter = 70 mm) with fresh washed (with filtered water) sand and water taken from the tank and left to burrow, which happened within 1–2 min. Seawater was used for a negative control to observe animal response to the input flow and 1 mL of feeding solution made using 2× Tetra Tabimin dissolved in 200 mL of water as a positive control. The bioassays were recorded using a mounted GoPro 10 and results were analyzed using SPSS 13.
To create heatmaps of activity shore crabs, Carcinus maenas were collected by hand from Bridlington pier (54.081235° N, −0.19314° W) in Yorkshire and transported to the aquariums at the University of Hull. The animals were stored in flow through natural sea water aquariums. The salinity was kept at 35 ppt, pH at 8.0–8.18, and temperatures between 15°C and 18°C. They were fed weekly on Mytilus edulis. For the bioassay, Carcinus (n = 20) were placed into the testing tanks (60 cm × 30 cm) and left to acclimate for 2 min. Circular filter paper was soaked in micro plastic odor (polyethylene) and artificial seawater for 1 min, before being placed into opposite ends of the tank. The video footage started after 2 min of acclimatization and ran for 5 min per bioassay. The footage was recorded using a mounted iPhone camera and results were analyzed using LoliTRACK automatic tracking software.
For the acclimation experiment, Hediste (Nereis) diversicolor were tested directly in the field at the Humber Estuary. Animals (n = 20) were hand collected from the estuarine mud and placed into a beaker, and time was recorded at the start of burrowing (head segments). Animals were transported back to the aquariums at Hull University and maintained as described above. At weekly intervals, randomly selected individuals were removed from the aquarium by hand and transferred into individual beakers (diameter, 70 mm) with fresh sterile seawater washed sand, seawater was added, and burrowing time was recorded. This was repeated for a series of 6 weeks. Bioassays were undertaken by the same observer to minimize any observational discrepancies.
For the study on responses of Alitta (Nereis) succinea males to the female sex pheromone Nereithione, individual worms were collected by hand as described previously (see Hardege et al., 2004) at four sampling sites (Roath Docks, Cardiff, UK; Orø Beach, Isefjord, DK; Salton Sea State Park, California, USA; and Eel Pond, Woods Hole, Massachusetts, USA). Sexually active, swimming male heteronereids (n = 40 per population) were transferred into 100-mL glass beakers filled with sterile seawater of the appropriate salinity for their populations (Cardiff 34 ppt, Woods Hole 34 ppt, Salton Sea 56 ppt, Isefjord 22 ppt). A dilution series of cysteinyl-glutathione (CSSG, Nereithione) was prepared using sterile seawater from the sites (0.2 µm filtered) at 10−6 M to 10−9 M concentrations and added to the swimming worms using a micropipette until male worms were initiated to swim in narrow circles and release sperm as described by Hardege et al. (2004) and the response threshold noted. Seawater was used as control, exposure order of experiments was randomized, and manual recording was undertaken blind, with the observer not aware of the nature of the samples.
Results and discussion
Animal behavior, especially when observed in the field, is complex to interpret, as a range of environmental factors that are difficult to control and, in many publications, not even determined or reported (Figure 1), impact organisms’ responses. In addition, organisms are also exposed simultaneously to multiple, often competing sensory cues, such as predator and prey cues that regulate biotic interactions (Weissburg et al., 2014). Very little, if any, information is often available on the physiology, social status, and potential pre-exposure of the organism tested. Similarly, quantification of chemical signals used in studies is almost impossible in the field, and in laboratory setups, it is restricted to those chemosensory compounds where the chemical structure has been identified, both representing major weaknesses in chemosensory research (Roggatz et al., 2022). Exploring small differences between animal responses, when there is significant variance in behavioral responses between individuals, often with a limited number of specimens used, requires statistical proof and the use of sufficient numbers of replicates. The challenge here is that environmental conditions influencing responses (Figure 1) should either be kept identical for all treatments or at least be examined statistically for example using multiparametric analysis (Serdobolskii, 2018). Reproducibility levels of behavioral studies has been reported as similarly low (Locey, 2020) as that of life sciences as a whole where Baker (2016) reported that only 24% of over 1,500 authors interviewed were able to publish a successful replication of published research.
Challenge 1: Will all animals that are used in a behavioral assay respond when a stimulus is added?
An assumption often made in aquatic chemosensory studies is that all animals randomly selected from a pool of individuals will respond to a stimulus (or make a choice upon exposure to multiple stimuli), underestimating the fact that very little information is available on the physiology, social status, and potential pre-exposure of the individuals tested. The readiness to respond to a cue cannot be assessed easily, for example, through a visual check and requires pre-main experiment tests of every individual in a tank to establish their responsiveness—this, though, potentially creates a bias as individuals may, for example, learn from the experience. Selecting individuals from an experimental pool or stock of individuals therefore carries a significant level of uncertainty about the suitability of the individuals selected for the bioassay. Most behavioral studies show substantial levels of result variance and often huge error bars due to lack of signal reliability and environmental uncertainty (McLinn and Stephens, 2006). Undertaking behavioral assays “blind”, with the observer not aware of the identity of the samples to be tested, including negative and positive control, as well as picking the appropriate statistical tests, is therefore key to reduce bias in interpreting the data collected. Simply assuming readiness to respond to chemosensory cues though can lead to false interpretations of behaviors observed. Specific behaviors such as the attraction and mating stance in crustaceans require the receiver of a message to be in the correct physiological state to detect and respond (Hardege et al., 2002; Hardege et al., 2011). In decapod crustaceans, where mating is closely linked to the female molt (Havens and McConaugha, 1990), the receiver, here the male responding to a female sex pheromone, must be sexually active (Hardege et al., 2002). This responsiveness status cannot be simply assumed even in the middle of the reproductive season (Hayden et al., 2007), nor can one determine this by inspecting an individual visually, but usually requires pre-experiment tests, which, in turn, could create complications if cue recognition is not innate but animals can learn odors as shown in fish to alter predator–prey chemosensory interactions. Asterropteryx semipunctatus goby learn to recognize a novel chemical cue and can associate it with danger after a single simultaneous exposure to a cue and a conspecific alarm signal. Rapid learning of novel chemical cues is a potentially important mechanism for odor recognition (Larson and McCormick, 2005). Similarly, tadpoles (Lucon-Xiccato et al., 2018) learned to recognize the odor of novel predator species that were simultaneously paired with alarm cues.
Using paired tests with pre–post-exposure stats on responses to signals can improve levels of significance in simple behavioral tests, as it eliminates unrelated variation (Roggatz et al., 2019), but bears the potential criticism of re-usage of organisms that may have learned from pre-exposure to chemosensory cues (Davies et al., 2019). When analyzing behavioral data, one can look at differences between treatments or samples, or relationships with independent variables, explore whether data are parametric or non-parametric including post-hoc tests, look at means and variances, and include corrections for outliers. There is a large set of statistical tests available and a plethora of commercial and free software packages to help scientists in this task. Power analysis and advanced statistics can potentially help to reduce the number of replicates (Guo et al., 2013) and to analyze complex multiple parameters simultaneously. Using R programming, Yamamoto et al. (2018) demonstrate how multiparametric cluster and factor analysis can be used to analyze and to categorize 33 different behaviors of mice recorded non-interrupted for 11 days. Their data analysis enabled them to interpret behavioral changes that are associated with the effect of social isolation, intermittent socialization, and re-introduction to a familiar home cage. Despite the use of advanced statistics though, the challenges on recording and interpreting behavior remain. In addition, Oza (2023) reports on a study that highlights that when using the same dataset for statistical analysis, 246 biologists calculated a huge spread of results.
While pseudo-replication due to the re-use of animals can be compensated for using Bayesian statistics (Lazic et al., 2020), an often-underestimated effect of animal re-use is negative associative learning. For example, in repeated use of animals for behavioral assays, while testing the avoidance of predator odor, a prey organism potentially habituates by ignoring the predator cue, when a threat is no longer associated with the odor. Memory of negative experiences, such as loss of a fight with a conspecific (Fletcher and Hardege, 2009), and memory of painful events exist in marine invertebrates, but, without reinforcement, only last short periods of time, such as 1 day in hermit crabs, Pagurus bernhardus (Appel and Elwood, 2009). This phenomenon of negative learning has hampered the use of deterrent odors in integrated pest management of insects for decades as these cues quickly lose their activity in field trials (Witzgall et al., 2010). Other examples for effects of learning include habituation in laboratory cultures towards feeding stimulants given to animals with food (Derby et al., 2001) and conditioning to behavior assay tanks, both affecting chemosensory responses such as the time crustaceans require to forage in olfactometers (Davies et al., 2019). Both a positive and a negative control are required in assays to produce robust results that can be included in statistical testing. Unfortunately, many chemosensory studies lack these controls as researchers are anthropomorphizing that all individuals randomly selected and then tested will respond in the same way without evidencing this using previous studies.
The number of replicates should then be carefully decided based on such previous experiments with the chosen model organisms. Responses to cues can vary significantly between species even when almost identical assay methods are applied. For example, in cue choice chambers, a cryptic decapod characterized by low movement probably needs a different number of replicates to have a statistically different behavioral response if compared to a species characterized by faster and sometimes chaotic movement such as predatory decapods. The condition-dependent immense variability in animal behavior determines their responses to chemosensory cues to vary so much that most experiments could be described as “unique”—developing at least a minimal level of standardization would be a major improvement. Including a method development stage should, as such, become a key part of a well-structured experimental plan strictly related to a statistics strategy decided a priori.
Challenge 2: How do we select individuals that will respond to chemosensory cues?
“False positives” and “false negatives” significantly impact the statistical significance of behavioral tests. The observer is usually not able to guarantee that all animals tested will respond as their physiological and olfactory pre-exposure state is unknown (McLinn and Stephens, 2006). Many studies eliminate, for example, non-responders in their statistical analysis and focus on those individuals that showed a response, either negative or positive. Figure 2A shows the effects of removing non-responding shore crabs (C. maenas) in a trial to examine the effects of predator odor. Here, neither group represents crabs that do not move but hide, a response even stronger than choosing the control side. Figure 2B shows that ragworms (Hediste diversicolor) leave their burrows without any chemosensory cue added in studies examining the foraging towards food cues. The box plot shows that although there is a significant difference in responses to a negative and positive feeding cue, there is clear variance in the animals’ behavior, with individuals still responding to a negative control, due to worms leaving their burrows without being stimulated with a chemosensory cue.
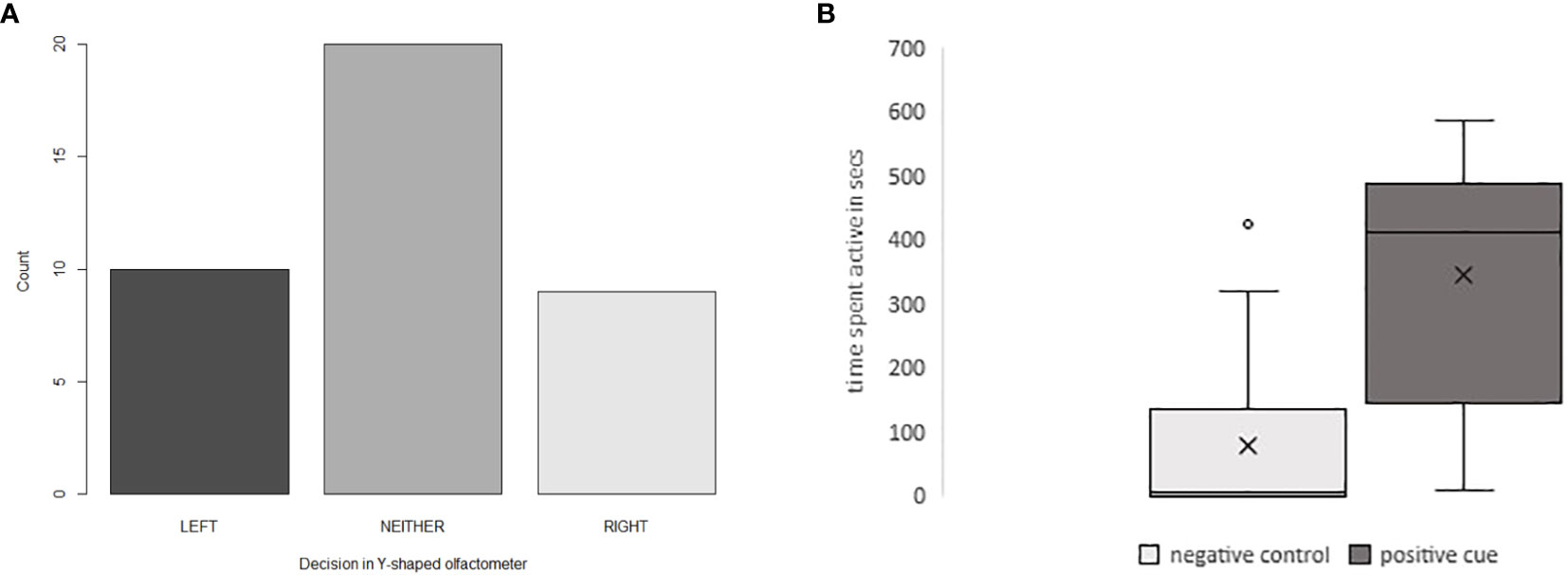
Figure 2 (A) The effect of non-responding animals on the statistical significance in Y-shape olfactometers. Reaction of shore crabs, Carcinus maenas exposed to control (seawater), and a feeding cue (GSH), n = 40. Statistics including all data: χ2 (2, N = 40) = 6.65, p = 0.035, statistically significant. Statistics not including the Carcinus that did not choose either arm (“Neither” on A): χ2 (2, N = 19) = 0.181, p = 0.67, not statistically significant. (B) The effect of animals responding regardless of whether a negative control or a positive sample is added. Foraging activity (leaving the burrow, staying in the open tank) of ragworms; Hediste diversicolor towards a negative control (seawater) and a feeding cue (fish food); N = 20.
Mitigation measures could be taken to ensure that most if not all individuals tested are potentially responding to a chemosensory cue. An example of this is in the sex pheromone of the shore crab, where Hardege et al. (2002) were able to increase the likelihood of male crabs responding by only selecting male crabs that were collected in mating pairs in the field. This way, the observer could assume that the female had released the pheromone, and the male was responsive towards the cue. In behavioral assays with males, approximately 90% of males responded to the natural female cue as well as its synthetic analogue uridine-di-phosphate (Hardege et al., 2011), while in all randomly collected males from the field, response rates never significantly exceeded 50% of the males tested (Hardege et al., 2002).
There are very few examples of aquatic chemosensory cues that have been tested with response rates that are near 100% though, as not every individual selected may be in the correct physiological state to respond. Mating in ragworms, Platynereis dumerilii, only occurs after the individuals have undergone a metamorphosis into a reproductive form that has only one purpose—the release of gametes after which it dies (Özpolat et al., 2021). Such heteronereids exclusively respond to sex pheromones, utilizing no other sensory modes, have a clear, simple reproductive behavior known as nuptial dance that culminates in the release of gametes, and spawn in moonlight-controlled mass events. Not surprisingly, when a worm spawns, and commits itself to die afterwards, all individuals respond to the pheromones of the opposite sex (Zeeck et al., 1988). This enabled simple bioassays (release of sperm yes/no) with response rates of >99% to the cue and under 1% to controls, and the only variance being the compound threshold required to initiate the release of gametes in individuals.
Randomly selecting individuals from a group in a culture or in the field is good practice for statistical reasons, but unless individuals are truly isolated from each other in their culture tanks so that no sensory contact is possible, pre-experimental contact/experiences cannot be ruled out. In the field, such pre-exposure to cues is impossible to determine, but keeping experimental animals in individual tanks with individual non-shared water bodies in the laboratory is rarely reported in the methods of chemosensory research papers.
In the crustacean example, interacting crabs form social and dominance hierarchies reinforced through chemosensory signals (Breithaupt and Eger, 2002). The animal’s status as winner or loser of previous aggressive interactions (fights) significantly impacts the response to female sex pheromones (Fletcher and Hardege, 2009), with the loser either not responding at all or responding only after an elongated time, and even winners taking longer to engage with female sex pheromones. Boldness is a personality trait in animals often studied to describe the way an individual reacts to risk, for example, to predator odors, as such describing their “risk-coping strategy” with hermit crabs showing no evidence that boldness and performance capacity (as measure of fitness) are linked (Courtene-Jones and Briffa, 2023). Used as a criterion to describe intra-individual behavioral variance, a common index of boldness is the latency to recover from a negative experience (startling stimulus), such as the re-emergence from a shelter (Stamps et al., 2012). With such individuality and traits impacting behavioral responses, large sample sizes, repeated measures, linked or paired data, and careful planning of pre-behavioral test culture regimes are paramount to reduce the huge intraindividual variability in animal responses to olfactory cues (Stamps et al., 2012). It is often difficult to determine, though, whether external factors such as seasonality, simple differences such as sex, and complex social interactions or a combination of these impose the strongest pressures leading to physiological status and/or response grade variance. It is as such recommended to reduce the impact of as many of these factors contributing to variability as possible, for example, by restricting experiments to defined times of the season, by careful selection of individuals, and by clearly documenting all these in the method description of any behavioral studies. Preliminary pre-testing animal’s responsiveness and variance for method development is a key element for this process.
Challenge 3: Develop quantifiable testing with high repeatability and selection of the right cues.
Added complications in statistical evaluation of behavioral data include that the behavior definitions (parameters) chosen to grade a response are not always linear. Most papers on crustacean chemosensory behaviors (Hayden et al., 2007) include response-level scales (ethograms) that are not directly correlated mathematically and so require independent statistical analysis for each grade defined, as grades cannot be directly compared.
In recent years, a controversial discussion has arisen about the effects of OA upon olfactory behaviors in fish with research teams evaluating the degree by which olfactory systems are affected differently (Esbaugh, 2023). The controversy is based mainly on differences in the statistical meta-analysis of publications describing the effects of OA upon olfaction that either shows a decline in the severity of the effects in articles published over the past decade (Clements et al., 2022) or is not changing (Munday, 2022). The discussion is at least in part based on the challenging nature of the quantification of the chemosensory cues added during the experiments. In the absence of chemical structural knowledge of the cues used, “conditioned water” with unquantifiable animal odors is often utilized as a proxy for predator or prey odor (Munday et al., 2009; Porteus et al., 2021 for review). For sufficient numbers of chemoreceptors to be activated to enable a behavioral answer, an exposure to cues above a defined signal-to-noise threshold is required (Atema, 2012). This creates significant challenges for the use of unknown compounds in chemosensory research. Changes in olfactory behavior are greatest when around the detection threshold, as levels of signaling compounds may fall below or above the threshold in a small concentration range. The fact that large effects do only occur in a narrow range of cue concentration has huge implications for behavioral science. Changing the quantity of cues outside this small window of detection has a very small effect (Figure 3). Since animals are exposed to a multitude of sensory cues simultaneously, signal reliability and environmental uncertainty influence animal decisions to respond to signaling cues. Often, a minimum reaction-specific signal-to-background noise ratio is required and environmental stressors, such as reduced pH in OA studies, can decrease the bioavailability of signaling cues below this very small concentration window (Roggatz et al., 2022). It has been demonstrated in many species across all realms that a bunch of chemical cues can trigger different behavioral reactions in different target species. The question arises if this is related to the perception of different infochemicals at the different administered concentrations. When based on perception at a threshold, this explains how changes to compound ratios in a cluster of compounds can lead to altered behaviors as described for algae copepod interactions (Maibam et al., 2015). Such ecological observations, for example, on volatile infochemicals (Mutalipassi et al., 2022) could also explain why organisms are attracted or repulsed by the same mix of compounds according to concentrations.
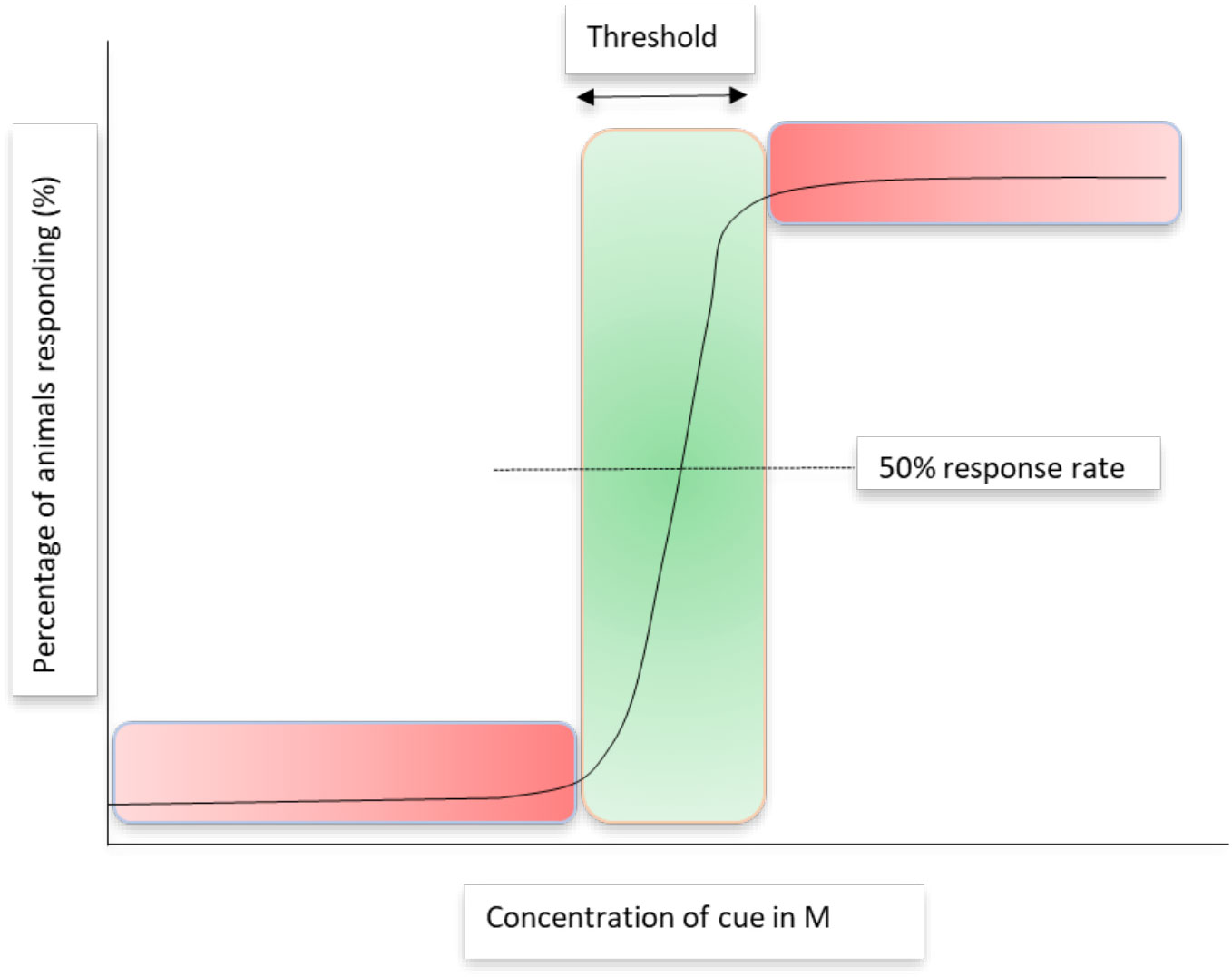
Figure 3 Percentage of animals responding to a chemosensory cue over a concentration range. The green area shows the small range over which adding/reducing the concentration of a cue around the detection threshold will alter the % of animals responding. The red areas describe concentrations of the cue where altering the concentration of signaling cues will not change the % of animals responding.
The use of unknown quantities of chemically unidentified chemosensory cues is extremely unlikely to yield data that fall into this small window, especially since cues in “conditioned water” can vary dramatically in quantity and quality—predator odor, for example, may depend on the physiological state of the predator, the time, quality and quantity of feeding the predator, its size, sex, and the entire list of environmental factors affecting animal fitness described earlier (see Figure 1).
Not surprisingly, repeatability of many chemosensory cue studies for the olfactory disruption topic has been poor; the discussion leads to claim/counterclaim at regular intervals (Clements et al., 2022; Munday, 2022), culminating in problems with the integrity of the data published (Enserink, 2022). The discussion highlights that the diversity of statistical methods and packages can lead to scientists using different statistical tests that are controversially discussed in the process of publication of data with contrasting responses between authors and reviewers or even between reviewers. Although statistical analysis is vital to prove significance in datasets, statistics is a “secondary” mechanism that relies on the chosen behavioral tests to be unambiguous and clear in the first instance, as even agreed conventions on p-values can create irreproducible results if used to reduce the n of replicates too dramatically (Halsey et al., 2015).
In many studies on aquatic chemosensory systems, a common misconception on the chemical nature of cues is that aquatic infochemicals must be water soluble, which excludes many lipophilic (hydrophobic), volatile compounds that subsequently have been found to function as aquatic chemosensory cues (Bartels-Hardege et al., 1996). Mollo et al. (2014) challenged this view, describing how hydrophobic compounds play a key role as chemo-attractants as shown in hermaphroditic shrimps (Zhang et al., 2011). Mollo et al. (2014) rather suggest that hydrophobicity may be the predominant characteristic of marine chemical cues, therefore facilitating a seamless transition to olfaction on land that did not require the selection and subsequent adaptation of olfactory receptors.
Release of chemosensory cues as temporal and spatial intermittent pulsed odors significantly improves the tracking of cues in aquatic organisms in turbulent plumes (Hardege et al., 2002; Michaelis et al., 2020). Most chemosensory cue studies in aquatic systems describe the release of cues in a constant flow though. Chemical cues rely on diffusion, passive transport (currents), or active transport as seen in crustaceans that utilize fan organs to reach the receiver of a message (Breithaupt, 2001), and signal strength should decrease dramatically over distance as the signal-to-noise ratio decreases (Atema, 2012). Low diffusion, however, is essential for animals that lay trail cues, such as female ragworms, Alitta (Nereis) succinea, using a peptide cue to attract males during their spawning (Ram et al., 2008). On land, mice Mus musculus release volatile pheromones in a slow-release matrix, the mouse urinary proteins (MUPS), which enable volatiles to be used as territorial markers and a diversity of functions (Thoss et al., 2015). Despite significant improvements of our understanding of the chemical structure of chemosensory cues, the effective concentrations released into the environment by a sender of a signal and the active thresholds at detection are virtually unstudied. The recommended use of synthetic analogues of known infochemicals is more quantifiable than conditioned water samples and natural extracts, but future studies should determine the effective concentrations in the environment to increase reproducibility.
Challenge 4: Record, store, and analyze data in a transparent manner.
Recording of behavioral tests via video analysis is thought to improve data handling, analysis, and storage, reduce cheating, and increase the repeatability of studies and has become standard practice (Luxem et al., 2023 for review). Most journals now require original datasets to be deposited/submitted to ensure compliance with data management and handling. Video analysis enables documenting and reduces human error, as it includes automated analysis of data, such as the time an animal spent in an area of a tank, its acceleration towards a cue, lag time in response, or quantification in tanks with multiple organisms. Data can be stored and visually compared, for example, via heatmaps, albeit individual heatmaps may show very different results to the average animal’s response (Figure 4).
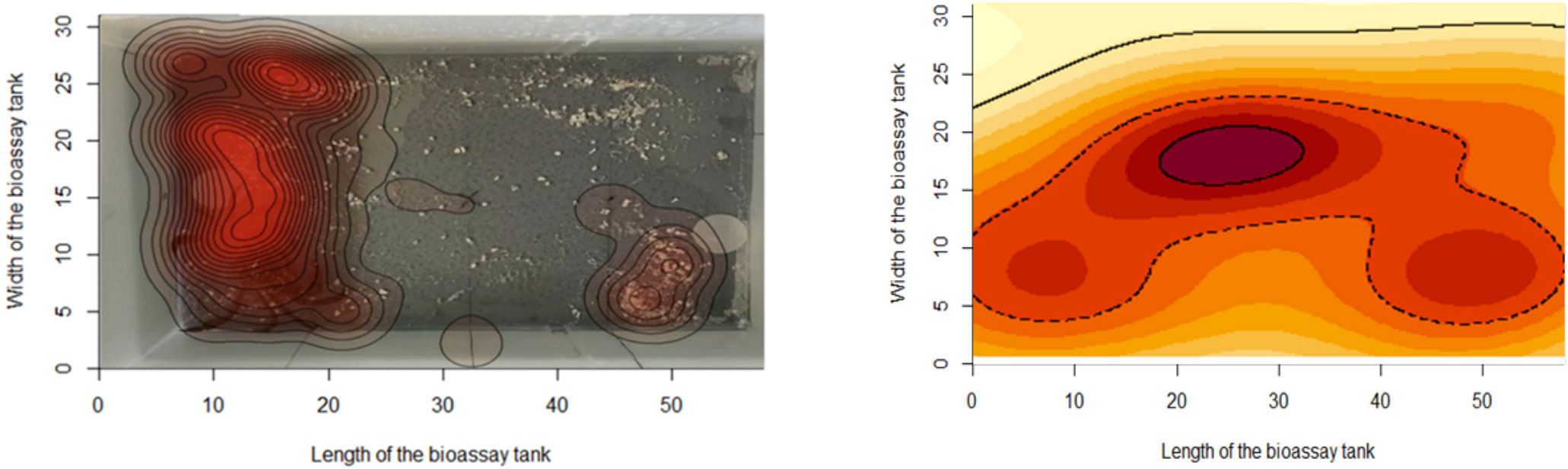
Figure 4 Left: Heatmap of an individual Carcinus maenas movement over a 5-min period with a cue and control present at opposite ends of the tank. Right: Heatmap showing the average movements of n = 20 Carcinus over a 5-min period with a micro plastic odor (polyethylene) cue and control (Artificial Seawater) present.
Technical problems include imaging quality, light, and angle of recording, and most importantly, all analysis of data still relies on the behavior recorded to be a biologically relevant behavior and linked to chemosensory cues; for example, when an animal moves towards a cue in an olfactometer, the motivation of the animal to engage in the behavior is not always clear, potentially tracking a nonspecific response (Hardege et al., 2002). Attraction, for example, of a male crab towards a chemosensory cue of a female in an olfactometer should demonstrate a sexual response, but attraction to a cue or the source of a cue could also be driven by different motivations of the receiver. These include cannibalism, gregariousness, dominance behavior, or fighting (Zi et al., 2023)—interpretation and use of attraction as a sole behavioral trait are as such challenging in chemosensory research (Hardege et al., 2002).
In theory, through photo and video documentation, incidents of data manipulation should decline, but the idea that cheating is still possible has been highlighted by a recently retracted article that was originally published in Science. In the paper by Lönnstedt and Eklöv (2016) on the effects of microplastic on marine fish, ecophysiology and chemosensory behavior was well documented and data were recorded but were found to be fraudulent (Enserink, 2017) and consequently retracted, highlighting the need for ethical handling of data creation and analysis beyond statistics and photo/video documentation.
Challenge 5: Establish a laboratory culture minimizing acclimation/habituation impacts upon behavioral outcomes.
Experiments in the field rarely allow for environmental impacts upon chemosensory science (Figure 1) to be recorded or controlled; it is a common anthropomorphic misconception that laboratory studies are easier as we can control all parameters. In principle, laboratory studies consist of two key phases, the culture/keeping of animals before a behavioral test and the bioassay itself. Undertaking both phases in a laboratory/aquarium setting enables the control of many environmental conditions and thus enables a researcher to reduce such variables, but comes at a potential cost: it can lead to situations that are no longer representative of the field and, as such, to potentially unrepresentative results (McLinn and Stephens, 2006).
Controlling culture conditions prior to a chemosensory assay represents a major challenge, as the list of factors influencing an animal’s physiology and, with this, its ability to respond naturally to a cue are so complex and diverse (Figure 1). To reduce environmental influences, cultures are usually kept in simple scenarios such as the setup of tanks in either glass or plastic with single-species cultures and limited shelters, often with a single source food supply and biofiltration units to control essential water chemistry (Sordo et al., 2016). This minimization of variance and disturbance creates conditions that do not reflect the complexity of ecosystems, and most studies therefore include a period of acclimation to laboratory conditions (Blewett et al., 2022).
Keeping animals in individual tanks but in shared water bodies leaves chemicals dissolved in recirculating systems being distributed to all individuals potentially influencing each other. Isolated prawns Macrobrachium rosenbergii grow more than twice as fast as runts raised with the dominant, blue-clawed males that releases dominance cues impacting subdominants (Karplus et al., 1992). Metabolic excretions accumulating in closed recirculating culture systems include sex hormones and pheromones that are known to impact maturation (Stacey et al., 2003), sexual status, and social status. When individuals are kept in higher densities or are allowed to interact in aggressive and dominance fights, stress and anxiety in such cultured populations increase as shown in cichlid fish (Aires et al., 2015) and a range of crustaceans (Colby and Jackson, 2011). Exposure to abiotic stressors such as reduced pH or increased temperature can lead to the release of metabolites by individuals that propagate the stress to others in the vicinity, leading to a behavioral stress response (Feugere et al., 2021). Fights between cultured individuals can cause injury or cannibalism that activates alarm signals in conspecifics (Toa et al., 2004). Keeping animals in communal tanks prior to experimental tests also carries the potential criticism of creating pseudo replicates as discussed in the section on statistical analysis. The variability in responsiveness to chemosensory cues based on social interactions and hierarchies that are not fixed (Blewett et al., 2022) can change in a difficult-to-predict manner, leading to a major challenge in selection of animals to be tested and interpretation of behavioral experimental data (Nancollas and McGaw, 2021).
In a culture system, apart from obvious factors (such as water temperature, salinity and pH, daylength, and moon phase to simulate seasonality), an additional fundamental decision is to select whether these conditions are kept static or fluctuating. Although this may be surprising, given that most marine life exists in near-shore, often estuarine conditions, where environmental fluctuations are common and define life activities, very few laboratory studies reflect such variable conditions (Kroeker et al., 2020). Schunter et al. (2021) showed that physiology and fish molecular responses to OA differ dramatically between static and fluctuating seawater pH conditions. Temperature and salinity fluctuations cause physiological stress to marine life, which, in turn, will impact responses of animals in chemosensory studies (Feugere et al., 2021). Many of the waste products accumulating in circulating seawater systems over time affect the water alkalinity and pH, especially ammonia and the CO2 excreted, if animals are kept for extended periods of time requiring regular seawater changes using pH-adjusted clean seawater (Munday et al., 2014).
It is widely accepted that the choice of culture conditions for kept specimens represents a major challenge that potentially impacts results of any behavioral and physiological assay undertaken; however, no serious attempts have been made to standardize this type of research. Melvin et al. (2017) demonstrated that for every one of the 165 studies on fish behavior they analyzed, acclimation and behavioral observation periods differed. In contrast, for OA research, there are standardized guidelines (Riebesell et al., 2011) to ensure the comparability of chemical data obtained. Arbitrary time spans for acclimation represent a very different percentage of the lifespan of a species, which we postulate would be a better, more relevant measure to standardize such studies.
Acute exposure to chemosensory cues from animals, for example, collected in the field, is often described as problematic, as handling stress (Wilson et al., 2021) may impact responses to chemosensory cues. O’Neill et al. (2018) found no direct correlation between behavioral repeatability and observation time in guppy, Poecilia reticulata, and in tadpoles, disturbance cues were not associated with learning, but formed part of the background risk, and no acclimation was found (Rivera-Hernández et al., 2022). This correlates with the fact that if the field animals need to respond again and again to a variety of cues and filter out essential chemosensory information like predator cues, not re-using animals for experiments, even after direct handling, makes no ecological sense. Interpretation of data gained from acute exposure studies to make “long-term” predictions, for example, upon future impacts of climate change stressors upon chemosensory signals, is equally too speculative.
We investigated the impact of acclimation and habituation to laboratory conditions using polychaete worms, Hediste (Nereis) diversicolor. Figure 5 shows that burrowing time (escape behavior) in worms tested in the field is significantly shorter (Kruskal–Wallis rank sum test: chi-squared = 47.804, df = 7, p-value = 3.89e-08) than once the worms were settled into a culture tank after an initial “shock” at day 1. The initiation of burrowing does not change much over the following 6 weeks of culture, presumably due to habituation to the lack of anxiety, as no predators can be detected by the worms. Habituation to shadows and movement no longer suggests a predator such as seabirds (Nanninga et al., 2017). Interestingly, we see a huge variability between individuals in this study, suggesting personality traits (bold/shy) as known in crustaceans (Briffa, 2020).
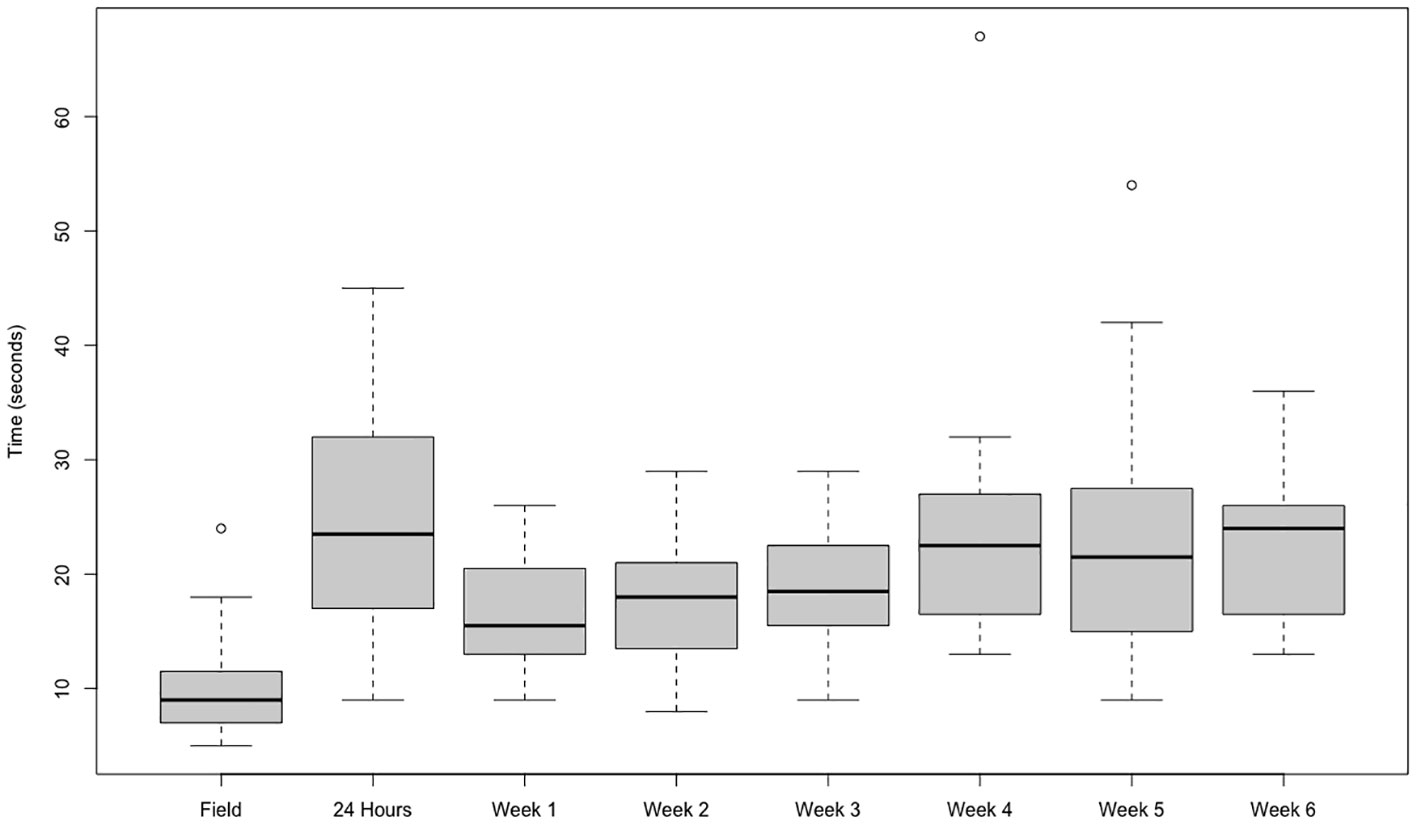
Figure 5 Time taken in seconds for Hediste (Nereis) diversicolor (n = 20) to initiate burrowing of the head segments. Bioassays undertaken with animals in the field and at a range of times within an aquaculture environment from 24 h to 6 weeks.
Challenge 6: Design a bioassay procedure that is unambiguous.
Attraction to a chemosensory signal is frequently used as a type of bioassay to test the effects of cues such as food or prey, gregariousness, or mates. The use of olfactometers testing “attraction” towards olfactory cues is a good example of how small design differences may influence the results, as binary olfactometers are criticized to force animals to make a binary choice (Jutfelt et al., 2017). Apart from flow through flumes or olfactometers, the recirculating systems also suffer from changing and often fast increasing background odor levels, when the wastewater (including the cues to be tested) is recirculated with the clean water (Hardege et al., 2002; Jutfelt et al., 2017). In attraction chambers, the motivation of an animal to move towards a chemosensory cue is not unambiguous (Bamber and Naylor, 1996). More unambiguous reaction-specific behavioral end points of animal responses such as release of gametes or a stereotyped mating stance are essential (Hardege et al., 2002).
Keeping water quality close to natural levels in cultures using top ups and mechanical, biological, and chemical filters is good practice in any aquarium cultures (Sordo et al., 2016). To acclimate individuals to new environments, such as the behavioral test arenas for chemosensory research, animals are kept in these tanks for extended periods of time (hours or even days) prior to experiments, often without water change, filtration pumps, or oxygenation as these would create physical disturbance and currents. This methodology is based on anthropomorphic assumptions that animals require a period of resting time to feel happy and respond to chemical cues after handling stress such as the placement into the behavioral arena (Wilson et al., 2021). Placement in static, often small behavioral tanks without shelter can lead to the release of stress metabolites (Feugere et al., 2021), and oxygen consumption, respiration, and the release of metabolites such as ammonia are potentially changing the chemical characteristics of the environment in which the bioassays take place. The short-term impacts of metabolic waste products in animal behavior tanks, including olfactometers, are poorly studied, and future studies should evaluate these, as we know from OA research that, for example, small chemical changes in seawater pH can alter animal chemosensory responses (Porteus et al., 2021; Roggatz et al., 2022).
Challenge 7: Examining chemosensory behavior in the field.
Examining chemosensory behavior in the field is suggested as more relevant to assess ecosystem impacts and ecological end points (Wyatt, 2014 for review). Studies on animal behavior in the field, such as the open ocean, are challenging though, both technically and in the experimental design (Calosi et al., 2013), as many environmental and ecological factors may influence animal behavior (Figure 1). Environmental variables that cannot be controlled or customized for, may include, among others, the effects of seasonality (Hayden et al., 2007), daily rhythms, and weather conditions [including sunshine, rain, wind, turbulence, currents and their direction and strength, and cloud cover (Hardege et al., 1998)]. Differences in ecosystem complexity range from availability of shelter, vegetation, and topography to interactions with other species including predators, potential prey, competitors, or mating partners, all impacting decision-making in aquatic organisms (Figure 1). Animal behavior observed in the field is as such extremely difficult to quantify and compare, as we usually have little information about the physiological status of individuals, such as their hormonal status, position in hierarchies, or boldness and shyness of individuals (Figure 1). Even basic information such as an animal’s size, weight, sex, or age is often not available when chemosensory responses are evaluated.
An example of the difficulties faced when comparing datasets produced by different research teams based on different locations globally is the lack of information on the local adaptations and acclimations in terms of chemosensory cues. While it is well studied in insects that even geographically very close, but isolated populations, use different ratios or blends of compounds, for example, for sex pheromones (Löfstedt, 1993), relatively little is known on geographic variability in aquatic pheromones. Figure 6 shows that the threshold at which male ragworms, Alitta (Nereis) succinea, respond upon exposure to the female-produced sex pheromone Nereithione (Ram et al., 1999) differs significantly between males from four populations examined. In the light of the recent debate on impacts of climate change on chemosensory cues discussed above (Esbaugh, 2023), this highlights that small changes in animal sensitivity to the exact same cue, when examined in stress research, require careful evaluation of the cue threshold, chemical nature, and quantity of cue(s) added (Figure 3) to obtain true comparisons between samples.
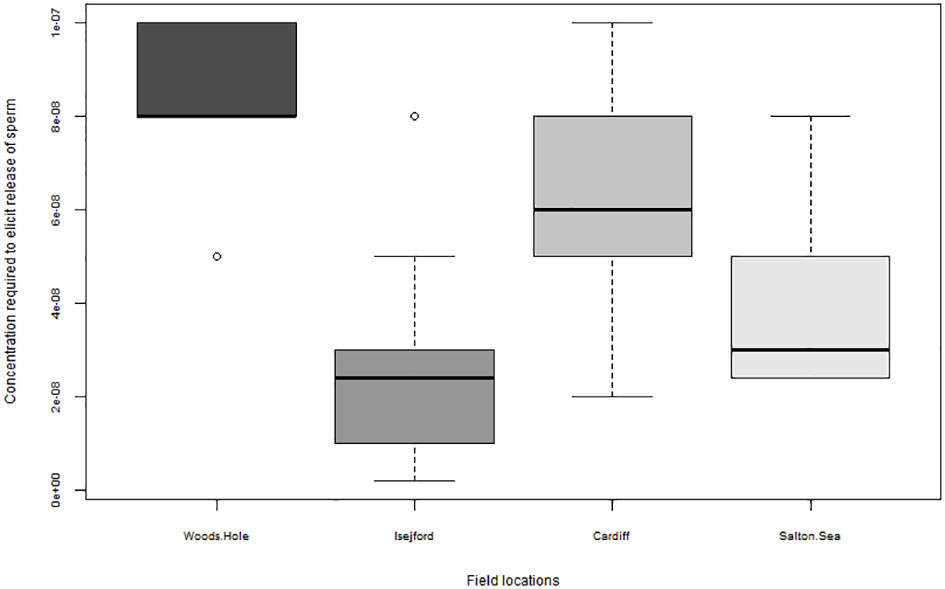
Figure 6 Response threshold to elicit sexual behavior (nuptial dance swimming in narrow circles, see Hardege et al., 2004) in Alitta (Nereis) succinea. Response threshold in male worms upon sex pheromone cysteinyl-glutathione (CSSG) in different ecosystems: Woods Hole, Massachusetts, USA; Isefjord, Denmark; Cardiff Roath docks, UK; Salton Sea, USA N = 40 per population.
While field studies allow for direct examination of chemosensory cues in an ecosystem with all the complexity this entails, not surprisingly, the huge complexity and diversity of factors impacting animal behavior led science for many years to utilize laboratory studies, often using individuals that were kept in culture systems. How results of chemosensory cue experiments can be extrapolated between species, over time or between different locations, is debatable, as the multitude of factors impacting an individual’s response to a cue is so diverse and changes over time (Figure 1). Selecting specimens from one population selects for local acclimation, and when animals come into culture conditions and stay there for weeks, months, or even generations, these acclimations may or may not change. As such, we recommend avoiding comparing studies from different geographic locations or at least acknowledging the high-level uniqueness of field-based experiments in discussions.
Conclusions
1. A wide range of anthropomorphic bias and predetermined assumptions of how animals will behave in experiments and how to interpret such behaviors can make evaluation of chemosensory studies challenging and highlight the need for more standardization similar to those in OA research.
2. To improve the comparability of datasets, future studies should record biotic and abiotic parameters that existed prior to and during a behavioral test.
3. As many biotic and abiotic factors impacting behavior as possible should be kept constant between repeats. Time of day used for bioassays, light levels, moon phase, temperature, size, sex, feeding status, social status, and age are among those parameters.
4. Recording and control of parameters represents an opportunity to discover unexpected correlations between behaviors observed and can help to understand functional traits and behavioral plasticity.
5. Standardization will help to make behavioral studies more transparent and easier to compare, reducing potential conflicts between research teams when studies seem to be not reproducible.
Data availability statement
The raw data supporting the conclusions of this article will be made available by the authors, without undue reservation.
Ethics statement
The manuscript presents research on animals that do not require ethical approval for their study. All collections and experiments were conducted following national and/or institutional guidelines. Approval was granted by the Ethics Committee of the University of Hull (No. UO20).
Author contributions
JH: Conceptualization, Supervision, Writing – original draft, Writing – review & editing, Funding acquisition, Methodology, Validation. NF: Data curation, Formal analysis, Investigation, Visualization, Writing – original draft, Methodology. JB: Data curation, Formal analysis, Investigation, Methodology, Visualization, Writing – original draft. HO: Data curation, Formal analysis, Investigation, Methodology, Visualization, Writing – review & editing. HB: Conceptualization, Methodology, Supervision, Validation, Writing – review & editing.
Funding
The author(s) declare financial support was received for the research, authorship, and/or publication of this article. We are grateful for financial support from the National Environmental Research Council, NERC grant no. NE/T001577/1.
Acknowledgments
The authors thank Daniel Lucas and Bethany Howard for help in data collection and experiments.
Conflict of interest
The authors declare that the research was conducted in the absence of any commercial or financial relationships that could be construed as a potential conflict of interest.
Publisher’s note
All claims expressed in this article are solely those of the authors and do not necessarily represent those of their affiliated organizations, or those of the publisher, the editors and the reviewers. Any product that may be evaluated in this article, or claim that may be made by its manufacturer, is not guaranteed or endorsed by the publisher.
References
Aires R. F., Oliveira G. A., Oliveira T. F., Ros A. F. H., Oliveira R. F. (2015). Dear enemies elicit lower androgen responses to territorial challenges than unfamiliar intruders in a cichlid fish. PloS One 10 (9), e0137705. doi: 10.1371/journal.pone.0137705
Appel M., Elwood R. W. (2009). Gender differences, responsiveness and memory of a potentially painful event in hermit crabs. Anim. Behav. 78, 1373–1379. doi: 10.1016/j.anbehav.2009.09.008
Atema J. (2012). “Aquatic odour dispersal fields: opportunities and limits of detection, communication, and navigation,” in Chemical Ecology in Aquatic Systems. Eds. Brönmark C., Hansson L.-A. (Oxford: Oxford Academic).
Bamber S. D., Naylor E. (1996). Chemical communication and behavioural interactions between sexually mature male and female shore crabs, Carcinus maenas. J. Mar. Biol. Ass. U.K. 76, 691–699. doi: 10.1017/S0025315400031398
Bartels-Hardege H. D., Hardege J. D., Zeeck E., Müller C., Wu B. L., Zhu M. Y. (1996). Sex pheromones in marine polychaetes V: a biologically active volatile compound from the coelomic fluid of female Nereis (Neanthes) japonica. J. Exp. Mar. Biol. Ecol. 201, 275–284. doi: 10.1016/0022-0981(96)00009-3
Blewett T. A., Binning S. A., Weinrauch A. M., Ivy C. M., Rossi G. S., Borowiec B. G., et al. (2022). Physiological and behavioural strategies of aquatic animals living in fluctuating environments. J. Exp. Biol. 225, jeb242503. doi: 10.1242/jeb.242503
Brattoli M., de Gennaro G., de Pinto V., Loiotile A. D., Lovascio S., Penza M. (2011). Odour detection methods: olfactometry and chemical sensors. Sensors (Basel) 11 (5), 5290–5322. doi: 10.3390/s110505290
Breithaupt T. (2001). Fan organs of crayfish enhance chemical information flow. Biol. Bull. 200, 150–154. doi: 10.2307/1543308
Breithaupt T., Eger P. (2002). Urine makes the difference: chemical communication in fighting crayfish made visible. J. Exp. Biol. 205, 1221–1231. doi: 10.1242/jeb.205.9.1221
Briffa M. (2020). “Animal personality and investment in reproduction: hermit crabs and other crustaceans as model organisms,” in Reproductive Biology: The Natural History of the Crustacea, Volume 6. Eds. Cothran R., Thiel M. (New York: Oxford Academic).
Briffa M., de la Haye K., Munday P. L. (2012). High CO2 and marine animal behaviour: Potential mechanisms and ecological consequences. Mar. Poll. Bull. 64, 1519–1528. doi: 10.1016/j.marpolbul.2012.05.032
Bublitz R., Sainte-Marie B., Newcomb-Hodgetts C., Fletcher N., Smith M., Hardege J. (2008). Interspecific activity of the sex pheromone of the European shore crab (Carcinus maenas). Behav 145, 1465–1478. doi: 10.1163/156853908785765872
Butenandt A., Groschel U., Karlson P., Ziliig W. (1959). N-acetyl tyramine, its isolation from Bombyx coccons and its chemical & biological properties. Arch. Biochem. Biophys. 83, 76–83. doi: 10.1016/0003-9861(59)90011-6
Calosi P., Rastrick S. P., Lombardi C., de Guzman H. J., Davidson L., Jahnke M., et al. (2013). Adaptation and acclimatization to ocean acidification in marine ectotherms: an in situ transplant experiment with polychaetes at a shallow CO2 vent system. Phil. Trans. R. Soc Lond. B. 368 (1627), 20120444. doi: 10.1098/rstb.2012.0444
Clements J. C., Hunt H. L. (2015). Marine animal behaviour in a high CO2 Ocean. Mar. Ecol. Progr. Ser. 536, 259–279. doi: 10.3354/meps11426
Clements J. C., Sundin J., Clark T. D., Jutfelt F. (2022). Meta-analysis reveals an extreme “decline effect” in the impacts of ocean acidification on fish behaviour. PloS Biol. 20 (2), e3001511. doi: 10.1371/journal.pbio.3001511
Colby J. T., Jackson A. L. (2011). The combination of social and personal contexts affects dominance hierarchy development in shore crabs, Carcinus maenas. Anim. Behav. 82, 1185–1192. doi: 10.1016/j.anbehav.2011.09.004
Courtene-Jones W., Briffa M. (2023). Boldness is not associated with dynamic performance capacity in hermit crabs. Biol. Lett. 19, 20230224. doi: 10.1098/rsbl.2023.0224
Davies R., Gagen M. H., Bull J. C., Pope E. C. (2019). Maze learning and memory in a decapod crustacean. Biol. Lett. 31, 20190407. doi: 10.1098/rsbl.2019.0407
Derby C. D., Steullet P., Horner A. J., Cate H. S. (2001). The sensory basis of feeding behaviour in the Caribbean spiny lobster, Panulirus argus. Mar. Fresh. Res. 52, 1339–1350. doi: 10.1071/MF01099
Draper A. M., Weissburg M. J. (2019). Impacts of global warming and elevated CO2 on sensory behavior in predator-prey interactions: A review and synthesis. Front. Ecol. Evol. 7, 72. doi: 10.3389/fevo.2019.00072
Enserink M. (2017). Fishy business. Science 355 (6331), 1254–1257. doi: 10.1126/science.355.6331.1254
Enserink M. (2022). Star marine ecologist guilty of misconduct, university says. Science 377 (6607), 699–700. doi: 10.1126/science.ade3374
Esbaugh A. J. (2023). Recalibrating the significance of the decline effect in fish ocean acidification research. PloS Biol. 21 (5), e3002113. doi: 10.1371/journal.pbio.3002113
Feugere L., Angell L., Fagents J., Nightingale R., Rowland K., Skinner S., et al. (2021). Behavioural stress propagation in benthic invertebrates caused by acute pH drop-induced metabolites. Front. Mar. Sci. 8, 773870. doi: 10.3389/fmars.2021.773870
Fletcher N., Hardege J. D. (2009). The cost of conflict: agonistic encounters influence responses to chemical signals in the shore crab, Carcinus maenas. Anim. Behav. 77, 357–361. doi: 10.1016/j.anbehav.2008.10.007
Guo Y., Logan H. L., Glueck D. H., Muller K. E. (2013). Selecting a sample size for studies with repeated measures. BMC Med. Res. Methodol 13, 100. doi: 10.1186/1471-2288-13-100
Halsey L., Curran-Everett D., Vowler S., Drummond G. B. (2015). The fickle P value generates irreproducible results. Nat. Methods 12, 179–185. doi: 10.1038/nmeth.3288
Hardege J. D., Bartels-Hardege H., Müller C. T., Beckmann M. (2004). Peptide pheromones in female Nereis succinea. Peptides 25, 1517–1522. doi: 10.1016/j.peptides.2003.11.029
Hardege J. D., Jennings A., Hayden D., Müller C. T., Pascoe D., Bentley M. G., et al. (2002). Novel behavioural assay and partial purification of a female-derived sex pheromone in Carcinus maenas. Mar. Ecol. Progr. Ser. 244, 179–189. doi: 10.3354/meps244179
Hardege J. D., Müller C. T., Beckmann M., Bartels-Hardege H., Bentley M. G. (1998). Timing of reproduction in marine polychaetes: The role of sex pheromones. Ecoscience 5, 395–404. doi: 10.1080/11956860.1998.11682477
Hardege J. D., Rotchell J. M., Terschak J., Greenway G. M. (2011). Analytical challenges and the development of biomarkers to measure and to monitor the effects of ocean acidification. TrAC Trends Anal. Chem. 30, 1320–1326. doi: 10.1016/j.trac.2011.07.004
Hardege J., Terschak J. (2011). “Identification of crustacean sex pheromones,” in Chemical communication in crustaceans. Ed. Breithaupt T & Thiel M. (New York: Springer), 373–392.
Havens K. J., McConaugha J. R. (1990). Molting in the mature female blue-crab, callinectes-sapidus rathbun. Bull. Mar. Sci. 46, 37–47.
Hayden D., Jennings A., Muller C., Pascoe D., Bublitz R., Webb H., et al. (2007). Sex-specific mediation of foraging in the shore crab, Carcinus maenas. Horm. Behav. 52, 162–168. doi: 10.1016/j.yhbeh.2007.03.004
Jutfelt F., Sundin J., Raby G. D., Krang A. S., Clark T. D. (2017). Two-current choice flumes for testing avoidance and preference in aquatic animals. Methods Ecol. Evol. 8, 379–390. doi: 10.1111/2041-210X.12668
Karplus I., Hulata G., Zafrir S. (1992). Social control of growth in Macrobrachium rosenbergii. iv. the mechanism of growth suppression in runts. Aquaculture 106, 275–283. doi: 10.1016/0044-8486(92)90259-N
Kimball B., Wilson D., Wesson D. (2016). Alterations of the volatile metabolome in mouse models of Alzheimer’s disease. Sci. Rep. 6, 19495. doi: 10.1038/srep19495
Kroeker K. J., Bell L. E., Donham E. M., Hoshijima U., Lummis S., Troy J. A., et al. (2020). Ecological change in dynamic environments: Accounting for temporal environmental variability in studies of ocean change biology. Glob. Change Biol. 26, 54–67. doi: 10.1111/gcb.14868
Larson J. K., McCormick M. I. (2005). The role of chemical alarm signals in facilitating learned recognition of novel chemical cues in a coral reef fish. Anim. Behav. 69, 51–57. doi: 10.1016/j.anbehav.2004.04.005
Lazic S. E., Mellor J. R., Ashby M. C., Munafo M. R. (2020). A Bayesian predictive approach for dealing with pseudoreplication. Sci. Rep. 10, 2366. doi: 10.1038/s41598-020-59384-7
Li K., Buchinger T. J., Li W. (2018). Discovery and characterization of natural products that act as pheromones in fish. Nat. Prod. Rep. 35, 501–513. doi: 10.1039/c8np00003d
Locey M. L. (2020). The evolution of behavior analysis: toward a replication crisis? Perspect. Behav. Sci. 12, 655–675. doi: 10.1007/s40614-020-00264-w
Löfstedt C. (1993). Moth pheromone genetics and evolution. Phil. Trans. R. Soc Lond. B. 340, 167–177. doi: 10.1098/rstb.1993.0055
Lönnstedt O. M., Eklöv P. (2016). RETRACTED: Environmentally relevant concentrations of microplastic particles influence larval fish ecology. Science 352 (6290), 1213–1216. doi: 10.1126/science.aad8828
Lucon-Xiccato T., Ferrari M. C. O., Chivers D. P., Bisazza A. (2018). Odour recognition learning of multiple predators by amphibian larvae. Anim. Behav. 140, 199–205. doi: 10.1016/j.anbehav.2018.04.022
Luxem K., Sun J. J., Bradley S. P., Krishnan K., Yttri E., Zimmermann J., et al. (2023). Open-source tools for behavioral video analysis: Setup, methods, and best practices. eLife 12, e79305. doi: 10.7554/eLife.79305
McLinn C. M., Stephens D. W. (2006). What makes information valuable: signal reliability and environmental uncertainty. Anim. Behav. 71, 1119–1129.
Maibam C., Fink P., Romano G., Buia M. C., Butera E., Zupo V. (2015). Centropages typicus (Crustacea, Copepoda) reacts to volatile compounds produced by planktonic algae. Mar. Ecol. 36, 819–834. doi: 10.1111/maec.12254
Melvin S. D., Petit M. A., Duvignacq M. C., Sumpter J. P. (2017). Towards improved behavioural testing in aquatic toxicology: Acclimation and observation times are important factors when designing behavioural tests with fish. Chemosphere 180, 430–436. doi: 10.1016/j.chemosphere.2017.04.058
Michaelis B. T., Leathers K. W., Bobkov Y. V., Ache B. W., Principe J. C., Baharloo R., et al. (2020). Odor tracking in aquatic organisms: the importance of temporal and spatial intermittency of the turbulent plume. Sci. Rep. 10, 7961. doi: 10.1038/s41598-020-64766-y
Mollo E., Boero F., Peñuelas J., Fontana A., Garson M. J., Roussis V., et al. (2022). Taste and Smell: A unifying chemosensory theory. Quarter. Rev. Biol. 97, 69–94. doi: 10.1086/720097
Mollo E., Fontana A., Roussis V., Polese G., Amodeo P., Ghiselin M. T. (2014). Sensing marine biomolecules: Smell, taste, and the evolutionary transition from aquatic to terrestrial life. Front. Chem. 2, 1–6. doi: 10.3389/fchem.2014.00092
Munday P. L. (2022). Reanalysis shows there is not an extreme decline effect in fish ocean acidification studies. PloS Biol. 20, e3001809. doi: 10.1371/journal.pbio.3001809
Munday P. L., Dixson D. L., Donelson J. M., Jones G. P., Pratchett M. S, Devitsina G. V., et al (2009). Ocean acidification impairs olfactory discrimination and homing ability of a marine fish. Proc Natl Acad Sci USA 106, 1848–1852. doi: 10.1073/pnas.0809996106
Munday P. L., Watson S.-A., Chung W. S., Marshall N. J., Nilsson G. E. (2014). Response to ‘The importance of accurate CO2 dosing and measurement in ocean acidification studies’. J. Exp. Biol. 217, 1828–1829. doi: 10.1242/jeb.105890
Mutalipassi M., Mazzella V., Schott M., Fink P., Glaviano F., Porzio L., et al. (2022). Ocean acidification affects volatile infochemicals production and perception in fauna and flora associated with Posidonia oceanica (L.) Delile. Front. Mar. Sci. 9. doi: 10.3389/fmars.2022.809702
Nancollas S. J., McGaw I. J. (2021). Acclimation to tidal conditions alters the physiological responses of the green shore crab, Carcinus maenas, to subsequent emersion. J. Exp. Biol. 224 (15), jeb242220. doi: 10.1242/jeb.242220
Nanninga G. B., Côté I. M., Beldade R., Mills S. C. (2017). Behavioural acclimation to cameras and observers in coral reef fishes. Ethology 123, 705–711. doi: 10.1111/eth.12642
O’Neill S. J., Williamson J. E., Tosetto L., Brown C. (2018). Effects of acclimatisation on behavioural repeatability in two behaviour assays of the guppy Poecilia reticulata. Behav. Ecol. Sociobio. 72, 166. doi: 10.1007/s00265-018-2582-7
Oza A. (2023). Reproducibility goes on trial in ecology. Nature 622, 677–678. doi: 10.1038/d41586-023-03177-1
Özpolat B. D., Randel N., Williams E. A., Bezares-Calderón L. A., Andreatta G., Balavoine G., et al. (2021). The Nereid on the rise: Platynereis as a model system. Evodevo 27, 10. doi: 10.1186/s13227-021-00180-3
Porteus C., Roggatz C. C., Velez Z., Hubbard P., Hardege J. D. (2021). The effects of ocean acidification on the olfaction of aquatic organisms;. J. Exp. Biol. 224 (14), jeb237941. doi: 10.1242/jeb.237941
Ram J. L., Fei X., Danaher S. M., Lu S., Breithaupt T., Hardege J. D. (2008). Finding females: Pheromone-guided reproductive tracking behavior by male Nereis succinea in the marine environment. J. Exp. Biol. 211, 757–765. doi: 10.1242/jeb.012773
Ram J. L., Müller C. T., Beckmann M., Hardege J. D. (1999). The spawning pheromone cysteine-glutathione disulfide (‘nereithione’) arouses a multicomponent nuptial behavior and electrophysiological activity in Nereis succinea males. FASEB J. 13, 945–952. doi: 10.1096/fasebj.13.8.945
Riebesell U., Fabry V. J., Hansson L., Gattuso J.-P. (2011). Guide to best practices for ocean acidification research and data reporting (Luxembourg: Publications Office of the European Union), 260.
Rivera-Hernández I. A. E., Crane A. L., Pollock M. S., Ferrari M. C. O. (2022). Disturbance cues function as a background risk cue but not as an associative learning cue in tadpoles. Anim. Cogn. 25, 881–889. doi: 10.1007/s10071-022-01599-4
Roggatz C. C., Kenningham N., Bartels-Hardege H. D. (2019). “Taking current climate change research to the classroom—The “Will hermit crabs go hungry in future oceans?” Project,” in Climate Change and the Role of Education. Climate Change Management. Eds. Leal Filho W., Hemstock S. (Cambridge: Springer). doi: 10.1007/978-3-030-32898-6_15
Roggatz C. C., Saha M., Blanchard S., Schirrmacher P., Fink P., Verheggen F., et al. (2022). Becoming nose-blind—Climate change impacts on chemical communication. Glob. Change Biol. 28, 4495–4505. doi: 10.1111/gcb.16209
Schirrmacher P., Roggatz C. C., Benoit D. M., Hardege J. D. (2021). Ocean acidification amplifies the olfactory response to 2-phenylethylamine: altered cue reception as a mechanistic pathway? J. Chem. Ecol. 47, 859–876. doi: 10.1007/s10886-021-01276-9
Schunter C., Jarrold M. D., Munday P. L., Ravasi T. (2021). Diel pCO2 fluctuations alter the molecular response of coral reef fishes to ocean acidification conditions. Mol. Ecol. 30, 5105–5118. doi: 10.1111/mec.16124
Serdobolskii V. I. (2018). Multiparameter methods are the new field in statistics. J. Math. Sci. 228, 533–542. doi: 10.1007/s10958-017-3642-7
Sordo L., Santos R., Reis J., Shulika A., Silva J. (2016). A direct CO2 control system for ocean acidification experiments: testing effects on the coralline red algae. Phymatolithon lusitanicum. Peer J. 4, e2503. doi: 10.7717/peerj.2503
Stacey N., Chojnacki A., Narayanan A., Cole T., Murphy C. (2003). Hormonally derived sex pheromones in fish: exogenous cues and signals from gonad to brain. Can. J. Physiol. Pharm. 81, 329–341. doi: 10.1139/y03-024
Stamps J. A., Briffa M., Biro P. A. (2012). Unpredictable animals: individual differences in intraindividual variability. Anim. Behav. 83, 1325–1334. doi: 10.1016/j.anbehav.2012.02.017
Thoss M., Luzynski K. C., Ante M., Miller I., Penn D. J. (2015). Major urinary protein (MUP) profiles show dynamic changes rather than individual “barcode” signatures. Front. Ecol. Evol. 3, 71. doi: 10.3389/fevo.2015.00071
Toa D. G., Afonso L. O. B., Iwama G. K. (2004). Stress response of juvenile rainbow trout (Oncorhynchus mykiss)to chemical cues released from stressed conspecifics. Fish Physiol. Biochem. 30, 103–108. doi: 10.1007/s10695-005-0266-5
Weissburg M., Smee D. L., Ferner M. C. (2014). The sensory ecology of non-consumptive predator effects. Am. Nat. 184, 141–157. doi: 10.1086/676644
Wilson C. H., Nancollas S. J., Rivers M. L., Spicer J. I., McGaw I. J. (2021). Effects of handling during experimental procedures on stress indices in the green shore crab, Carcinus maenas. Mar. Fresh. Behav. Physiol. 54, 65–85. doi: 10.1080/10236244.2021.1923369
Witzgall P., Kirsch P., Cork A. (2010). Sex pheromones and their impact on pest management. J. Chem. Ecol. 36, 80–100. doi: 10.1007/s10886-009-9737-y
Wyatt T. D. (2010). Pheromones and signature mixtures: defining species-wide signals and variable cues for identity in both invertebrates and vertebrates. J. Comp. Physiol. A 196, 685–700. doi: 10.1007/s00359-010-0564-y
Wyatt T. D. (2014). Pheromones and animal behavior: chemical signals and signatures (Cambridge University Press).
Yamamoto K., Gris K. V., Sotelo Fonseca J. E., Gharagozloo M., Mahmoud S., Simard C., et al. (2018). Exhaustive multi-parametric assessment of the behavioral array of daily activities of mice using cluster and factor analysis. Front. Behav. Neurosci. 30. doi: 10.3389/fnbeh.2018.00187
Zeeck E., Hardege J., Bartels-Hardege H., Wesselmann G. (1988). Sex pheromone in a marine polychaete: determination of the chemical structure. J. Exp. Zool. 246, 285–292. doi: 10.1002/jez.1402460308
Zhang D., Terschak J. A., Harley M. A., Lin J., Hardege J. D. (2011). Simultaneously hermaphroditic shrimp use lipophilic cuticular hydrocarbons as contact sex pheromones. PloS One 6 (4), e17720. doi: 10.1371/journal.pone.0017720
Keywords: olfactory cues, challenges, bioassay, acclimation, habituation, bias, standardization
Citation: Hardege JD, Fletcher N, Burnett JW, Ohnstad H and Bartels-Hardege HD (2024) Bioassay complexities—exploring challenges in aquatic chemosensory research. Front. Ecol. Evol. 11:1293585. doi: 10.3389/fevo.2023.1293585
Received: 13 September 2023; Accepted: 23 November 2023;
Published: 03 January 2024.
Edited by:
Ernesto Mollo, National Research Council (CNR), ItalyReviewed by:
Olivier P. Thomas, University of Galway, IrelandMirko Mutalipassi, Stazione Zoologica Anton Dohrn, Italy
Copyright © 2024 Hardege, Fletcher, Burnett, Ohnstad and Bartels-Hardege. This is an open-access article distributed under the terms of the Creative Commons Attribution License (CC BY). The use, distribution or reproduction in other forums is permitted, provided the original author(s) and the copyright owner(s) are credited and that the original publication in this journal is cited, in accordance with accepted academic practice. No use, distribution or reproduction is permitted which does not comply with these terms.
*Correspondence: Jorg D. Hardege, ai5kLmhhcmRlZ2VAaHVsbC5hYy51aw==