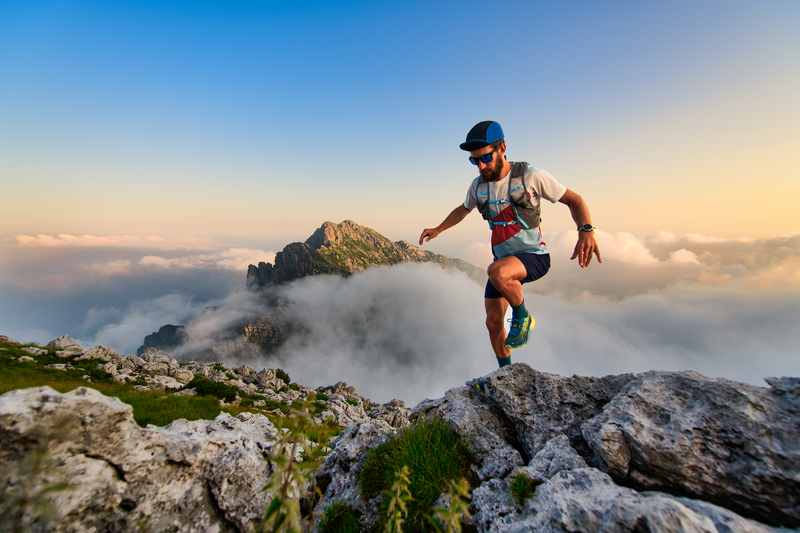
95% of researchers rate our articles as excellent or good
Learn more about the work of our research integrity team to safeguard the quality of each article we publish.
Find out more
ORIGINAL RESEARCH article
Front. Ecol. Evol. , 24 November 2023
Sec. Population, Community, and Ecosystem Dynamics
Volume 11 - 2023 | https://doi.org/10.3389/fevo.2023.1278762
Heat waves have increased in frequency, duration, and magnitude in recent decades, causing mass mortality events in terrestrial and aquatic ecosystems. Arguably, mass mortalities of habitat-forming organisms – i.e., dominant sessile organisms that define habitats via their own physical structure – would be amongst the most dramatic impact of heat waves because of their negative, cascading consequences on their associated biodiversity. However, the resistance of habitat-forming organisms to heat waves can be enhanced if they associate with secondary habitat formers able to tolerate and modulate extreme heat levels. Here we show that a seaweed of the Porphyra/Pyropia (P/P) clade can shield primary habitat-forming mussels, Brachidontes rodriguezii, from the impacts of extreme temperatures in a southwestern Atlantic rocky intertidal shore. By means of P/P removal experiments and surveys, we illustrate that P/P cover (a) buffers temperatures in the understory mussel beds during daytime air exposure periods in the summer, (b) reduces mussel mortality and leads to increased mussel body condition during warm summer periods, and (c) can prevent mass mortality of mussels during the course of a heat wave. Additionally, by means of a mussel removal experiment we illustrate that mussel cover is critical for P/P establishment, which is in consonance with the remarkably higher P/P densities and cover observed in mussel beds relative to exposed rock surfaces across a ~70 km coastal range. Collectively, these findings reveal a facultative mutualism where mussels provide a favorable substrate for P/P colonization and P/P attenuates heat mediated mortality on mussels. The ability of P/P to enhance the resistance of mussel beds to extreme heat events and the occurrence of similar P/P-mussel associations during spring-summer at globally dispersed sites suggests a widespread importance of P/P for the stability of mussel beds and their associated communities under warming climates.
Extreme heat events have increased in frequency, duration, and magnitude in recent decades and this trend is expected to continue under future climate scenarios (Perkins et al., 2012; Perkins-Kirkpatrick and Gibson, 2017). Organisms can be severely impacted by such heat waves, as evidenced by concomitant mass mortality events both in terrestrial and aquatic ecosystems (e.g., Welbergen et al., 2008; Garrabou et al., 2009; Ruthrof et al., 2018; Piatt et al., 2020; Westley, 2020). Intertidal organisms appear particularly susceptible to heat waves, especially during periods of aerial exposure at low tide when body temperatures can exceed their upper tolerance limits (Fields et al., 1993; Stillman, 2002). Heat-mediated mortality poses a particular threat to rocky intertidal communities, where a large proportion of species typically depend on the physical habitat created by one or a few dominant sessile species (e.g., seaweeds, mussels, oysters, barnacles, tunicates; Gutiérrez et al., 2022) which, on the other hand, can be sensitive to extreme heat (e.g., Hanekom, 2013; Kordas et al., 2015; Seuront et al., 2019; Scanes et al., 2020). Thus, the resistance and resilience of these communities to heat waves would strongly depend on the temperatures experienced by these dominant, habitat-forming organisms and their thermal tolerance limits (Jurgens et al., 2022).
Heat waves have been causing mass mortalities of habitat-forming species in intertidal rocky shores worldwide (e.g., seaweeds: Thomsen et al., 2019, mussels; Tsuchiya, 1983, tunicates; Hanekom, 2013, barnacles; Hesketh and Harley, 2023). In most of these cases, however, some cover of the habitat-forming species persists after the heat wave (e.g., Tsuchiya, 1983; Harley, 2008; Hesketh and Harley, 2023). To a significant extent, this occurs because rocky shores are thermally heterogeneous and frequently include microhabitats that are protected from temperatures that are detrimental to the habitat-forming organisms (see Helmuth and Hofmann, 2001; Harley, 2008; Denny et al., 2011; Li et al., 2021). These thermal refugia can prevent the local extirpation of the habitat-forming species during heat waves and assist its subsequent recolonization (e.g., by acting as sources of propagules and recruitment foci, via the lateral expansion of remnant patches attendant to individual growth, via various forms of self-facilitating feedbacks; e.g., Anderson et al., 1997; Alvarado et al., 2001; Angelini et al., 2016; Clausing et al., 2023). Studies on the impact of heat waves on rocky shores have typically emphasized thermal refugia of geomorphological origin (e.g., lower elevations in the intertidal slope, shore zones exposed to wave splash at low tide, shaded topographic elements such as crevices, tidepools, overhangs, and vertical surfaces protected from full sunlight exposure; see Tsuchiya, 1983; Helmuth and Hofmann, 2001; Helmuth et al., 2006; Harley, 2008; Lima et al., 2016; Hesketh and Harley, 2023). On the other hand, biogenic thermal refugia from heat waves have seldom been documented for habitat-forming species in rocky shores (but see Leonard, 2000), even when commonly overgrown by epibionts that could potentially buffer against solar irradiation, as well as temperatures and desiccation during the course of a heat wave.
Mussels are major space occupiers and habitat formers in the intertidal zone of temperate rocky shores across five continents (Suchanek, 1985; Gutiérrez et al., 2003; Gutiérrez et al., 2011) but are also sensitive to extreme heat events (e.g., Tsuchiya, 1983; Harley, 2008; Olabarria et al., 2016; Seuront et al., 2019; Galil et al., 2022; Raymond et al., 2022). Extensive mussel mortality is expected to cause severe and lasting impoverishment of local rocky shore biodiversity as mussel beds support rich species assemblages (including many species that would not otherwise occur in the rocky intertidal zone; see Suchanek, 1985; Tokeshi and Romero, 1995; Borthagaray and Carranza, 2007; Bagur et al., 2016) and can take relatively long to recover (Tsuchiya, 1983; Micheli et al., 2016). Nonetheless, the species inhabiting mussel beds frequently include sessile epibionts that attach to the mussel shells (Lohse, 1993; Tokeshi and Romero, 1995; Miyamoto and Noda, 2004). While epibionts are known to negatively affect their individual mussel hosts in various ways (Dittman and Robles, 1991; O’Connor et al., 2006; O’Connor, 2010), they are also likely to shield them from high solar irradiation and air temperatures at low tide (see Wahl, 2008). Additionally, in the case of epibiotic seaweeds that grow larger than their individual mussel hosts, their thalli could also protect neighboring, unfouled mussels from extreme heat at low tide. However, it remains uninvestigated whether epibiotic seaweeds can act as biogenic thermal refugia for understory mussels and enhance the resistance of mussel beds to extreme heat events.
Foliose red seaweeds originally ascribed to the genus Porphyra (now split into Porphyra and Pyropia based on molecular evidences; see Sutherland et al., 2011) commonly succeed as mussel epibionts in temperate rocky shores worldwide (e.g., Santelices and Martínez, 1988; Miyamoto and Noda, 2004; Aquilino et al., 2009; O’Connor, 2010). Interestingly, many species of Porphyra/Pyropia (hereafter P/P) could be especially suited to protect understory mussels from the impacts of heat waves, as they are known to modulate understory temperatures (e.g., Umanzor et al., 2017) and have been regarded as “stress-tolerant” due to their adaptations to withstand temperatures, light, and desiccation levels that are typically detrimental to other macroalgal species (see Blouin et al., 2011; Contreras-Porcia et al., 2017; Contreras-Porcia et al., 2022). A P/P species (not yet determined) has been observed growing as epibionts on bed-forming mussels, Brachidontes rodriguezii, on rocky shores of Buenos Aires Province, Argentina (Penchaszadeh, 1973; Gutiérrez and Palomo, 2016; Gutiérrez et al., 2019). Remarkably, this species reaches considerable cover (usually more than 20%) and density in mussel beds between mid-October and mid-January (i.e., mid spring to early summer; see Gutiérrez and Palomo, 2016; Gutiérrez et al., 2019 and Figure S1), with peaks of cover that can approach 100% (Figure 1A). This suggests that P/P could provide thermal protection to mussels at the time of the year with maximum solar irradiation (Atanasoska, 2021), and when the maximum annual temperatures often happen (Giampietri and Piccolo, 2000). It is also of note that mussels seem to be a critical substrate for this P/P species, as it is largely restricted to mussel beds and rarely develops on exposed rock surfaces (see Figure 1 and Figure S1). This suggests a facultative mutualism where mussels are critical substrates for P/P colonization and P/P protects the understory mussels from environmental stress.
Figure 1 (A) Rocky shore showing dense and extensive Porphyra/Pyropia (P/P) cover on mid-intertidal mussel beds (Punta Cantera, Argentina, 38°05’ S, 57°32’ W, December 2011). (B) P/P-covered mussel bed surface surrounded by exposed rock surfaces virtually lacking from P/P cover. The ruler at the bottom of the picture is 20 cm long (Las Brusquitas, Argentina, 38°14’S, 57°46’W, January 2016). (C) Rock surface showing nearly 100% cover of P/P on mussel beds. The scale at the bottom right is about 20 cm length (Copacabana, Argentina, 38°14’ S, 57°46’ W, December 2022).
In December 2015, a heat wave conforming to the definition of Perkins and Alexander (2013) (i.e., a period of at least three consecutive days when temperatures exceed the 90th percentile value of historical, calendar day records) occurred on coastal Buenos Aires Province (Figure S2, see also Aldeco et al., 2016) just when a P/P-removal experiment was being run to test whether P/P cover affects the mobile interstitial invertebrate assemblage inhabiting mussel beds (see Gutiérrez et al., 2019). We observed substantial losses of mussel cover in the P/P-removal treatment but not in the control plots with natural P/P cover (see Results), which suggested that P/P overgrowth affords significant thermal protection from heat waves to mussels. To evaluate if the protective effect of P/P on understory mussels is limited to heat wave conditions, we repeated this experiment during late spring/early summer in subsequent years, when we also considered effects on mussel body condition as an indication of non-lethal effects. Moreover, we conducted additional P/P-removal experiments and surveyed natural areas with and without P/P cover to test for modulatory effects of P/P on understory temperatures. Finally, we conducted a mussel removal experiment to test whether mussels facilitate P/P colonization or, instead, whether their spatial association results from shared preferences for particular spots within the shore.
This study was conducted on intertidal cohesive platforms at Las Brusquitas (38°14’43” S, 57°46’33” W), 5 km northeast of Miramar, Buenos Aires Province, Argentina. These platforms are located at the base of an 8–12 m high cohesive cliff, and are primarily composed of reddish-brown, sandy siltstones originating from reworked loess deposits and sparse indurated caliche levels and nodules (Zárate and Fasano, 1989; Isla et al., 2015; Rico et al., 2020). This shore faces southeast, which is the direction of the strongest swells (Fiore et al., 2009), and can be classified as “exposed” according to the MarLin wave exposure categories (i.e., an open coast facing away from prevailing winds but with a long fetch, and where strong winds are frequent; https://www.marlin.ac.uk/glossarydefinition/waveexposurelocated). The tidal regime across this range is semidiurnal and microtidal (0.80 m mean amplitude; Servicio de Hidrografía Naval, Argentina; www.hidro.gov.ar).
Brachidontes rodriguezii forms dense beds (up to 2000 ind. dm−2) at the mid intertidal elevation of these platforms (Gutiérrez et al., 2018; Gutiérrez et al., 2019). This relatively small mytilid (up to 55 mm length, most individuals < 30 mm length) occurs on the Atlantic coast of South America from the state of Rio Grande do Sul (Brazil, 32°S) to Punta Ninfas (Argentina, 42°S; Penchaszadeh, 1973; Arribas et al., 2013; Trovant et al., 2015). Their beds are primarily single-layered with multilayered areas restricted to protected vertical rock surfaces and small-sized (< 50 cm2) sparse hummocks (< 1 per m−2; Gutiérrez et al., 2015).
Porphyra/Pyropia thalli occur in these platforms from late winter (August) to early fall (March; Gutiérrez et al., 2019, see also Results). They mostly occur as mussel epibionts (> 75% of thalli) but colonize just a small fraction of the mussels in the population (< 7%; Gutiérrez and Palomo, 2016). P/P reaches considerable cover (> 20%) in mussel beds between mid-October and mid-January (mid spring to early summer; Gutiérrez and Palomo, 2016; Gutiérrez et al., 2019; see also Results), with peaks of cover that vary in timing across years and sites and sometimes approach 100% (see Figure 1, Table 1, Results, and Supporting Information 1). During its growing season, P/P is the only epibiont that establishes significant cover in mussel beds (other abundant mussel epibionts are either small-sized or occur at other times of the year; e.g., barnacles, Balanus glandula, and Ulva spp., respectively).
Table 1 Dates of execution of P/P removal experiments, mean (SD) initial and final Porphyra/Pyropia (P/P) cover in control plots, and response variables measured in each experimental trial.
In previous studies, P/P specimens sampled at nearby rocky shores were either reported as Porphyra umbilicalis (Penchaszadeh, 1973), Porphyra leucosticta (López Gappa et al., 1990; now in the genus Pyropia following Sutherland et al., 2011), Porphyra pujalsiae (Boraso and Zaixso, 2011) or, simply, Porphyra sp (Becherucci et al., 2014). To date, taxonomic uncertainties remain due to the lack of molecular studies on P/P specimens from our study area. Hence, for the purposes of this paper, we conservatively use the term Porphyra/Pyropia, or P/P, to refer to the specimens found at our study area as well as the broader group of related species they pertain to.
To evaluate the effect of P/P cover on the survival and condition of understory mussels, short-term P/P removal experiments (nearly 3 weeks long) were conducted in 2015, 2017, 2019, and 2020. These experiments were run between early December and mid-January (i.e., late spring to early summer; see Table 1 for exact dates), concurrently with high P/P cover (see Table 1), high temperatures (maximum records during each period were 37, 34, 35, and 35°C, respectively: see Figure S2), and other conditions that favor desiccation (high solar irradiation, long photoperiods). Yet, extreme heat conditions spanning across several consecutive days only occurred during the 2015 experiment (see Figure S2 and Aldeco et al., 2016).
Sixteen 25-cm side, square plots showing 95–100% mussel cover were randomly demarcated in horizontal, single layered areas of the mussel bed on each date (distance between plots > 2 m). P/P thalli at half of these plots were pruned at their base with multipurpose scissors. The remaining plots were left undisturbed as controls (i.e., n = 8). P/P removal plots were checked periodically (every 3–5 days) to remove any newly recruited or regrowing thalli. Since rocky intertidal shores in the study region lack resident predators (see Palomo et al., 2019), our experiment was not designed to control for predators as a cause of differences in mussel mortality in relation to P/P cover (see also Discussion for further evidences on the unimportance of predators as a cause of mussel mortality in this experiment).
After about 3 weeks, mussel cover losses were estimated in each plot with the point-intercept method. For that purpose, a squared 20 cm-side grid (4 cm grid size) was placed centered in the plots and the number of grid interceptions overlapping with mussel-covered surfaces were counted. The grid used here was smaller than the plot to minimize the likelihood of edge effects (e.g., influences of surrounding algae on the mussels occurring in the edge of P/P-removal plots).
Additionally, a cylindrical core sample of the mussel layer (10 cm diameter) was taken from each plot. These samples were taken to the laboratory and sieved (1 mm mesh) to remove sediments, and then the empty, articulated mussel shells in these samples were counted as another measure of individual mussel mortality (i.e., in addition to mussel cover loss). Although these empty, articulated shells may not strictly have come from mussels that died during the experiments (i.e., shells from earlier-dying mussels may also be present), differences in empty shell counts among treatments can be reliably considered as an indication of treatment effects even when they may not reflect actual mortality rates. Finally, three mussels ranging 15–17 mm shell length were taken from each core sample in the 2017, 2019 and 2020 experiments and analyzed for body condition (measured as dry tissue mass/dry shell mass; Sousa et al., 2011). The tissues of these mussels were extracted from their shells after briefly immersing them in boiling water. These shells and tissues were oven-dried to constant weight at 50°C and weighed on an analytical scale (precision: 0.001 g).
A generalized linear model (GLM) with binomial distribution and a logit link function was used to evaluate whether the Treatment (P/P removal vs. control) and the Year when the experiment was executed (2015, 2017, 2019, 2020) explained variations in mussel cover at the end of the experiment (the data modelled was the proportion of 36 grid points overlapping with mussel-covered areas after point-intercept sampling of the experimental plots). A GLM with Poisson distribution and log link function was used to evaluate whether Treatment and Year (2015, 2017, 2019, 2020) explained variation in counts of empty, articulated mussel shells. Generalized Linear Mixed Models (GLMM) with a Gaussian distribution and an identity link function were used to evaluate whether mussel body condition at the end of the experiment varied with Treatment, Year (2017, 2019, 2020) and the experimental Plot from where the mussels were collected (random variable). All statistical analyses were run with Stata, Release 14 (StataCorp, 2015).
The potential of P/P cover to modulate temperatures in the underlying mussel bed during the low-tide air exposure period was evaluated with the two following approaches:
(a) The temperature of the mussel bed surface in natural, P/P-covered and exposed mussel bed areas was evaluated on six dates between December 2015 and March 2016 (10 areas of each type per day, a single measurement per area) by means of an infrared thermometer (Fluke 59 MAX+, Fluke Corporation, Everett, WA, USA; accuracy: ± 1.5°C). This thermometer allows adjusting temperature readings to the emissivity of the target material (here set to 1 as previously assumed for mussels; see Helmuth, 1998). Measurements were made by placing the infrared sensor of the thermometer 15 cm above the mussel bed surface, which gave a circular field of measurement of about 2 cm diameter based on an 8:1 distance to spot ratio (see www.fluke.com). To measure mussel bed temperatures in the P/P-covered areas a few algal thalli were first displaced to expose the underlying mussels and relocated to their original position once temperature was measured. Each date, all temperature measurements were made within a 20-minute timeframe to minimize variations in air temperature and other atmospheric conditions (e.g., cloudiness, solar irradiation) that may influence temperature readings.
(b) Waterproof temperature loggers (Hobo Pendant MX2201, Onset Computer Corporation, Bourne, MA, USA; accuracy: ± 0.5°C) were installed on P/P-removal and control plots such as those described above (see Effects of P/P cover on understory mussels) on two hot days: December 17, 2020, and February 1, 2022. Temperature data were obtained at 15-second intervals over 3 afternoon hours, encompassing the maximum air temperatures occurring each date (33 and 29°C, respectively, as recorded at Mar del Plata Airport; 37°55’ S, 57°34’ W, ~40 km from the study site). The maximum air temperatures on these dates corresponded to those of a typical hot summer day in this area (mean maximum daily temperature = 25°C; Giampietri and Piccolo, 2000). In both cases, logging finished before the platform began to be flooded again by the rising tide.
A single logger was placed in the center of each P/P-removal and control plot (3 plots each) after removing the mussels from an area just sufficiently large to allow it to fit in (logger size ~ 3 × 5 cm). All loggers were covered by a large shell (~ 9 cm) of the mussel Mytilus chilensis obtained from mussels bought from a local fish shop. These shells were used instead of B. rodriguezii shells because the latter are not large enough to fully cover the loggers. The loggers and their overlying shells in the control plots were placed beneath naturally-growing P/P thalli. Although loggers covered by M. chilensis shells are unlikely to provide a measure of the actual temperatures experienced by B. rodriguezii in the field, they should approximate such temperatures better than uncovered loggers receiving direct solar radiation (cf., heat transfer to mussel tissues across their shells) and, ultimately, they are expected to provide a more realistic picture of the effect of P/P cover on the temperatures of underlying shelled invertebrates.
Variations in the temperature of the mussel bed surface as related to P/P cover (Yes/No) and sampling Date (6 different dates; see above) were evaluated with a generalized linear model (GLM) with a Gaussian distribution and an identity link function. Logger data from P/P-removal and control plots was displayed as temperature curves and visually compared.
A mussel-removal experiment was conducted to test whether the tight spatial association between P/P and mussel beds occurs because mussels represent a crucial habitat for P/P or, instead, because of shared preferences for shore spots. Twelve 25-cm side, square plots were demarcated in the mussel bed. Mussels were removed from six of these plots by means of a putty knife and the remaining plots were left undisturbed as controls. These plots were installed in July 2016, right before the beginning of the P/P growing season, and P/P colonization was monthly monitored until March 2017. Monthly data included counts of P/P thalli in the experimental plots as well as P/P cover estimates by means of the point intercept method (squared 20 cm-side grid centered on the 25 cm-side plot, 4 cm grid size; see Effect of P/P cover on understory mussels for detailed methods).
P/P count and cover data were not statistically analyzed as P/P largely failed to colonize the mussel removal plots during its growing season (i.e., most count data and all the cover data were zeroes as only two small thalli recruited to these plots; see Results).
P/P removal led to decreased mussel cover only in the 2015 experiment (14% reduction relative to controls), concurrently with the heat wave (GLM, Treatment x Year interaction: χ2 = 20.46, df = 3, p < 0.001; see Table S1 for full GLM results). No significant difference in mussel cover among treatments was observed in subsequent experiments conducted over non-heat wave periods (i.e., 2017, 2019, 2020 experiments; Figure 2A). Yet, increased densities of empty, articulated shells were found in P/P-removal plots relative to controls across all the above-mentioned experiments (GLM, Treatment effect: χ2 = 23.61, df = 1, p < 0.001; Figure 2B; see Table S2 for full GLM results).
Figure 2 Effect of Porphyra/Pyropia (P/P) removal on (A) percent mussel cover, (B) counts of empty, articulated mussel shells, and (C) mussel body condition (dry tissue mass/dry shell mass) in the different experimental years. Columns and bars represent means and standard deviations, respectively. Asterisks in (A, C) indicate significant effects of P/P removal in particular experimental years after significant interactions among both variables and planned contrasts of marginal linear predictors (not shown in panel (B) as only main effects of P/P removal on empty shell counts were observed).
Mussel body condition (not quantified in the 2015 experiment; see Materials and Methods) was negatively affected by P/P removal in the 2017, 2019, and 2020 non-heat wave experiments (13–32% decreases relative to controls; GLMM, Treatment × Year interaction: χ2 = 6.39, df = 2, p = 0.041; Figure 2C; see Table S3 for full GLMM results).
The temperature of the mussel bed surface (as measured with the infrared thermometer) was lower in P/P-covered areas relative to exposed mussel bed areas irrespective of the date of measurement (GLM, Treatment effect: χ2 = 61.54, df = 1, p < 0.001; Figure 3A; see Table S4 for full GLM results). Temperatures during the low tide air exposure period (obtained from loggers) were up to 7 and 9°C higher in P/P-removal plots relative to controls (December 17, 2020, and February 1, 2022, respectively) with no overlap in temperature records between treatments at any time (Figures 3B, C).
Figure 3 (A) Temperature of sun exposed mussel bed surfaces and those in the understory of Porphyra/Pyropia (P/P) canopies on different sampling dates, as measured by an infrared thermometer. Columns and bars represent means and standard deviations, respectively. Only main effects of P/P cover on temperatures were observed. (B, C) Effect of P/P removal on temperatures registered by shell-covered data loggers during two 3-h air exposure periods in the afternoon of two hot sunny days. Each curve represents the data registered every 15 seconds by temperature loggers installed in three P/P removal plots and three P/P-covered controls. The loggers installed in P/P-covered controls were placed underneath the algal canopy. Maximum air temperatures, as registered by the local weather station (Mar del Plata Airport, 37°55’ S, 57°34’ W, ~40 km from the study site), were 33 and 29°C on December 17, 2020, and February 1, 2022, respectively.
Mussel removal had a negative effect on P/P colonization across its growing season. Whilst P/P reached substantial densities and cover in mussel-covered control plots (up to 47 thalli and 94% cover in a single 0.0625 m2 plot) it remained virtually absent from mussel removal plots (only two small-sized, short-lived thalli were found; Figures 4A, B).
Figure 4 (A) Counts and (B) percent cover of Porphyra/Pyropia (P/P) thalli in mussel removal and control plots measured at monthly intervals over a P/P growing season. Columns and bars represent means and standard deviations, respectively. Note that only two small thalli recruited to mussel removal plots during the course of the experiment.
P/P cover has a protective effect on understory mussels, as illustrated by lethal (reductions in mussel cover, increased empty shell accumulation) and non-lethal negative effects (decreased mussel body condition) of short-term, experimental P/P removal (Figure 2). It is of note that mass mortality of mussels and a concomitant reduction of mussel cover after P/P removal were only observed in the 2015 experiment (Figure 2A), concurrently with a heat wave (Aldeco et al., 2016; Figure S2). This implies that the protective effect of P/P on understory mussels is largely attributable to thermal buffering (see Figure 3). Predators, on the other hand, can be ruled out as a source of mussel cover losses in P/P removal plots as rocky intertidal shores in the study region lack resident predators (Palomo et al., 2019) and any eventual non-resident mussel predator (such as fish or invertebrate predators that could forage in the mussel bed when submersed) would be expected to cause mussel cover losses every year irrespective of heat wave occurrence (see below in this section for further arguments on the general unimportance of mussel predators in this system).
Additionally, the temperatures experienced by mussels during the 2015 heat wave (December 25 to 30) can well explain the mass mortality and concomitant losses of mussel cover observed after the experimental removal of P/P. The daily maximum air temperatures during that period, as registered by the local weather station (Mar del Plata Airport, 37°55’ S, 57°34’ W, ~40 km from the study site), ranged between 33 and 37°C and exceeded the 90 percentile of maximum temperatures registered for these same dates between 1973 and 2021 (see Figure S2). Such temperatures could explain some degree of mussel mortality on their own, as they closely matched those that are known to be lethal for air-exposed intertidal mussels in laboratory experiments (median lethal temperatures ranging between 32 and 38°C depending on species, acclimation, and exposure time; see Denny et al., 2011; Seuront et al., 2019; Sorte et al., 2019) and took place over five consecutive days with a high chance of cumulative impacts (e.g., Seuront et al., 2019). Moreover, the actual temperatures experienced by mussels can be higher than air temperatures if mussels are air exposed during high solar irradiation periods (Helmuth et al., 2002; see also Figures 3B, C for further support). During the 2015 heatwave, sunny conditions prevailed (59 out of 90 daytime hourly records) and, during the first three days of the heat wave (which also were the clearest ones), mussels were air exposed during midday and/or the early afternoon (see Figure S7). This means that mussels were air- and sun-exposed at a time of the day (and the year; see Introduction) when potential solar irradiation is at or about its daily (and annual) maximum. Midday exposure to high air temperatures and high solar irradiation have been predicted to be especially stressful for mussels (Helmuth and Hofmann, 2001; Helmuth et al., 2002). Considering the upper thermal tolerance limits typical of mussels (32–38°C; see above), the maximum air temperatures registered during the heat wave (33–37°C), and the amplifying effects of high solar irradiation on the temperatures actually experienced by mussels, it becomes clear that the mass mortalities of mussels of observed in P/P removal plots in 2015 were heat mediated.
Although mussel mortality was not high enough to cause reductions in mussel cover in the subsequent P/P-removal experiments (2017, 2019, 2020; see Figure 2A), it was still higher in P/P-removal plots relative to controls, as indicated by increased numbers of empty, articulated mussel shells in the former (Figure 2B). As far as we can see, these differences in mortality are also attributable to the thermal buffering effects of P/P. Even though seaweed cover could physically protect understory mussels from the action of predators (e.g., Laudien and Wahl, 1999), mussel predators are largely lacking in the rocky shores of our region (Palomo et al., 2019: see above) and we know of no predator that can consume mussels without removing them from the bed and/or damaging their shells. Disease or aging can neither explain per se the increased numbers of empty mussel shells in the P/P removal treatment, as they are expected to operate irrespective of P/P cover. Thus, heat-mediated mortality seems to be the most compelling cause of increased empty shell accumulation in the P/P removal treatment (though increased disease prevalence in P/P removal plots might still have occurred as a by-product of increased thermal stress; see Green et al., 2019). Even when heat waves as defined here (see Introduction and Perkins and Alexander, 2013) did not occur during these experiments (maximum air temperatures in the lower range of those during the 2015 heatwave only persisted for 2 consecutive days; see Figure S2), heat-mediated mortality of sparse individuals across the mussel layer could still be explained, again, by the amplifying effects of solar irradiation on the temperatures experienced by mussels (Helmuth et al., 2002; see above), and by interindividual differences in heat conduction/storage (e.g., due to variations in mussel bed and rock topography, mussel size, position within the bed, or they degree of contact with neighbors and the underlying rock; Helmuth, 1998; Harley, 2008; Denny et al., 2011; Mislan and Wethey, 2015).
The role of P/P in protecting understory mussels from heat stress is further supported by the observed negative effects of P/P removal on mussel body condition (Figure 2C), which is a common indicator of heat stress in mussels and other intertidal bivalves (e.g., Fitzgerald-Dehoog et al., 2012; Hiebenthal et al., 2012; Taylor et al., 2017). Decreases in mussel body condition may be due to reductions in their energy stores (especially glycogen, which can make up to 35% dry weight of the soft parts; Zwaan and Zandee, 1972) or increased protein degradation both of which occur during heat stress (Hofmann and Somero, 1995; Lesser, 2016).
The protective effects of epibiotic P/P on understory mussels documented here can be attributed to distinct, but co-occurring structure-mediated effects (i.e., ecosystem engineering effects; Jones et al., 1994) on heat transfer processes. P/P thalli are expected to intercept, absorb, and reflect a significant fraction of the incident solar radiation, which otherwise would directly reach and heat understory mussels. On the other hand, direct conduction of heat from heated P/P thalli to understory mussels during air exposure periods will likely be limited given their small contact area (Mussels are mostly oriented with their antero-posterior axis perpendicular to the rock substrate and P/P lays flat over them as if laying on a bed of nails; Gutiérrez and Palomo, 2016; Soria et al., 2022). By intercepting incident solar radiation, P/P also reduces evaporation and maintains higher moisture in the interstitial space of the understory mussel beds (Gutiérrez et al., 2019). That moisture, together with the water retained by the P/P thalli themselves, may increase the efficiency of evaporative cooling (Gowell et al., 2015), and also decrease mussel desiccation stress. Finally, as the air within the mussel bed heats up, we also expect that moisture will evaporate leading to vapor accumulation and condensation at the undersides of P/P thalli (a “condensation trap” analogous to those made by humans for water collection and purification in arid regions; e.g., Hodder, 1970; Kumar and Bai, 2008, see Gutiérrez et al., 2019). This could further contribute to moisture retention, enhanced efficiency of evaporative cooling, and decreased mussel desiccation stress.
P/P thalli in the study site and other rocky shores in our region mostly occur as mussel epibionts and are scarce or absent in the gaps of exposed rock that are usually interspersed within mussel beds (Gutiérrez et al., 2019; see also Figure S1 and Figure 1B). Our findings demonstrate that this co-occurrence pattern happens because mussel cover facilitates P/P establishment, not because of shared habitat preferences. Mussel removal before the P/P growing season virtually inhibited subsequent P/P establishment (Figure 4), which is in consonance with its scarcity in natural, exposed rock gaps (see Figure 1B and Figure S1). The dearth of P/P thalli establishing in the mussel removal plots and its considerable levels of colonization in mussel-covered controls (up to 94% cover and 8 thalli dm−2; see also Figure 4) indicate that mussels play a crucial role in supporting P/P populations on these shores.
As with the protective effects of epibiotic P/P on understory mussels, several structure-mediated, engineering mechanisms are likely to account for the increased abundance of P/P in mussel beds relative to exposed rock gaps. Because of their complex three-dimensional structure, mussel beds could be more effective than relatively flat, exposed rock surfaces in trapping algal propagules (Wangkulangkul et al., 2016) and could provide them with refugia against pulmonate limpets, Siphonaria lessonii (see Hesketh et al., 2021), which are the main grazers in these shores (Palomo et al., 2019) and are known to forage on microalgae, macroalgae – including P/P species – and macroalgal spores and propagules (Santelices and Correa, 1985). Moreover, differences between mussel shells and exposed rock surfaces as regards to physical, chemical, and biological attributes that influence algal adhesion (eg., hardness, free energy, wettability, polarity, organic and microbial films; Fletcher and Callow, 1992; Callow and Fletcher, 1994) could have further accounted for the preferential development of P/P on mussel shells. Even when mussel shell surfaces bear physical or chemical defenses against epibiont adhesion, such as ripple-like microtopography or surface bound compounds (Scardino et al., 2003; Bers et al., 2006), our local P/P species succeed at colonizing mussel shells as is the case of several other P/P species elsewhere (e.g., Santelices and Martínez, 1988; Miyamoto and Noda, 2004; Aquilino et al., 2009; O’Connor, 2010). This suggests that the antifouling defenses of mussels would not necessarily be effective against P/P species or, alternatively, that these seaweeds take advantage of individuals with low immunity and/or abraded/damaged shells (Wahl et al., 1998; Bers et al., 2006). In the same vein, the consumption of algal propagules (which is known for some mussel species) would not severely be limiting P/P recruitment in mussel beds (see Santelices and Martínez, 1988).
Lastly, the mechanisms of moisture retention and cooling described above as beneficial for mussels (e.g., increased evaporative cooling in the understory of P/P, vapor accumulation and condensation at the undersides of P/P thalli; see Section 4.1) could also reduce environmental stresses for P/P and further promote its preferential development onto mussel beds relative to exposed rock gaps.
In summation, our findings illustrate a facultative mutualism where mussels provide a critical substrate for P/P which, in turn, buffers understory mussels from extreme heat and desiccation levels and enhance their resistance to heat waves. Since P/P can maintain significant cover over the mussel beds even during periods of high temperature and solar irradiation (Table 1, Figure S2), it could be a good candidate as a “climate rescuer” – i.e., a species resistant/resilient to climate change providing suitable environmental conditions to species that would otherwise be unable to maintain viable populations under future climate scenarios (Bulleri et al., 2018). A peculiarity of P/P as climate rescuer would be that it is a secondary habitat former, being the primary habitat formers in the system – i.e., the mussels – their direct beneficiaries (cf., Bulleri et al., 2018 focus on primary habitat formers as climate rescuers). Obvious indirect beneficiaries of P/P as a climate rescuer would be the substantial fraction of the rocky shore species that are obligate inhabitants of mussel beds (e.g., infaunal invertebrates that take advantage of the sediments trapped within mussel beds, small invertebrates lacking adhesive structures and prone to be washed-out by waves if outside the interstitial mussel bed habitat; Bagur et al., 2016). In this way, the rescuing effects of P/P on mussels would have cascading positive consequences for the overall rocky shore biodiversity. Considering that mussel cover can take several years to fully recover from disturbance (2+ years in our study system; e.g., Soria et al., 2023), the rescuing effects of P/P could also be contributing to the stability of biodiversity in the long-term.
Whether a “climate rescuer” status applies to P/P will depend on its resistance or adaptation to future environmental conditions and its ability to ameliorate stressful conditions under such circumstances (temperature and desiccation but also other climate-related stressors such as increased UV radiation and changing hydrodynamic regimes: see Bulleri et al., 2018), both of which need to be more comprehensively investigated. In principle, our results are clear in that, under current conditions, P/P cover increases the resistance to heat waves of understory mussel beds and their associate biota, which may be having important implications for their persistence vis-à-vis recent and impending increases in the frequency, magnitude, and duration of heat waves in our region (Barros et al., 2015; Rusticucci et al., 2016).
Certainly, the role of P/P as refugia from heat waves in mussel beds will not necessarily be as effective as documented here since heat waves vary in magnitude, duration, and timing, whereas the temporal dynamics and extent of P/P cover across its growing season also vary between years and sites (see cover data in Table 1 vs. Figure 4; see also site comparisons in Figure S1). Indeed, the substantial protective effects of P/P on mussels during the 2015 heatwave (Figure 2A) occurred at a time of relatively high P/P cover (68–78%, see Table 1). Clearly such protective effects should be more modest if P/P cover had been lower and/or peaked earlier in its growing season (e.g., as in 2014; Figure 4) or if the heat wave had occurred in the mid to late summer (February/March) when P/P cover is generally low or null (Gutiérrez et al., 2019, Figure 4) perhaps because of chronic stress build-up (Flores et al., 2015). It should be noted, however, that even a low P/P cover could contribute the resilience of mussel beds to heat waves by maintaining mussel bed patches that, although small and scattered, could be the germs for subsequent mussel bed recovery (e.g., by acting as recruitment foci and facilitating juvenile survival, via lateral patch expansion as individual mussels grow; e.g., Penchaszadeh, 1973; Arribas et al., 2015).
Species in the P/P clade could also play an important role in enhancing the resistance and resilience of mussel bed communities to heat waves outside our study range. As earlier mentioned, many P/P species have been regarded as “stress tolerant”, given their ability to withstand environmental conditions that can be detrimental to other seaweeds (Blouin et al., 2011). These species often thrive at shore levels dominated by mussels, even during the late spring and summer when temperature and solar irradiation reach their maximum. The occurrence of P/P species as mussel epibionts during the spring-summer at globally dispersed sites (Table S5) suggests a potentially widespread role of P/P as thermal refugia for mussels under globally warming climates. The tolerance of several P/P species to high temperatures (e.g., close or above 30°C in laboratory cultures; see Notoya and Nagaura, 1998; Sahoo et al., 2007) and desiccation (e.g., via the activation of antioxidant mechanisms; Contreras-Porcia et al., 2017; Contreras-Porcia et al., 2022), and their ability to accumulate UV-absorbing compounds (mostly mycosporine-like amino acids) to prevent UV damage (Korbee et al., 2004; Navarro et al., 2014) also points to a potentially widespread role of P/P species as climate rescuers in rocky shore communities.
This study adds to mounting evidence on the importance of mutualisms for the persistence of marine habitat-forming species (e.g., Hay et al., 2004; Bozec et al., 2013; Angelini et al., 2016; de Fouw et al., 2018). Mutualisms, however, occur under a restricted set of environmental conditions (Six, 2009). In a context of global climatic change, different environmental tolerances between mutualistic partners can lead to mutualism disruption (e.g., Hoegh-Guldberg et al., 2007; Wernegreen, 2012). Yet, as this study and Angelini et al. (2016) illustrate, habitat forming species can be associated with mutualistic, secondary habitat formers that protect them from the impact of climatic stressors that are projected to be stronger and more prevalent in the future (e.g., extreme temperatures, drought). In such cases, the increased environmental tolerance of secondary habitat formers can contribute to the persistence of their basal habitat formers, and the stability rather than the disruption of the mutualism.
Mutualisms between habitat-forming species and stress-alleviating, secondary habitat formers could potentially increase in strength under future climate scenarios, with the basal habitat-forming species increasingly depending on their secondary habitat-forming partners to resist to and recover from climate impacts. However, it is still unknown whether these mutualisms are widespread, nor whether they would remain stable under future climates (i.e., if secondary habitat-forming partners will be able to maintain comparable abundances and stress-alleviating functionality). Further research on these mutualisms and their stability against climate impacts appear as worthwhile, given the importance of habitat-forming species for the biodiversity and functioning of ecosystems (Ellison et al., 2005; Gutiérrez et al., 2011; Rossi et al., 2017) and the potential for co-management of mutualistic habitat formers to increase ecosystem resistance and resilience to climate change (e.g., Derksen-Hooijberg et al., 2018).
The original contributions presented in the study are included in the article/Supplementary Material. Further inquiries can be directed to the corresponding author.
Ethical approval was not required for the study involving animals in accordance with the local legislation and institutional requirements, because there is no local legislation in this regard.
JG: Conceptualization, Formal Analysis, Funding acquisition, Investigation, Methodology, Resources, Visualization, Writing – original draft. MB: Investigation, Methodology, Writing – review & editing. RL: Investigation, Methodology, Writing – review & editing. MP: Conceptualization, Funding acquisition, Investigation, Resources, Writing – review & editing.
The author(s) declare financial support was received for the research, authorship, and/or publication of this article. This study was financially supported by Agencia Nacional de Promoción de la Investigación, el Desarrollo Tecnológico y la Innovación (Agencia I+D+I; PICT 2015-2468 to MP, and PICT-2019-03904 to JG and MP) and Consejo Nacional de Investigaciones Científicas y Técnicas (CONICET; PIP 11220110100024).
We thank the Servicio de Hidrografía Naval – Sección Mareas (Ministerio de Defensa de la Nación) for providing tidal measurements from Mar del Plata port.
The authors declare that the research was conducted in the absence of any commercial or financial relationships that could be construed as a potential conflict of interest.
The author(s) declared that they were an editorial board member of Frontiers, at the time of submission. This had no impact on the peer review process and the final decision.
All claims expressed in this article are solely those of the authors and do not necessarily represent those of their affiliated organizations, or those of the publisher, the editors and the reviewers. Any product that may be evaluated in this article, or claim that may be made by its manufacturer, is not guaranteed or endorsed by the publisher.
The Supplementary Material for this article can be found online at: https://www.frontiersin.org/articles/10.3389/fevo.2023.1278762/full#supplementary-material
Aldeco L., Dominguez D., Garay N., Herrera N., Skansi M., Stella J. L., et al. (2016). Breves de clima -Diciembre 2015 (Buenos Aires, Argentina: Servicio Meteorológico Nacional, Doc. Number 0003CL2016).
Alvarado J. L., Pinto R., Marquet P., Pacheco C., Guiñez R., Castilla J. C. (2001). Patch recolonization by the tunicate Pyura praeputialis in the rocky intertidal of the Bay of Antofagasta, Chile: evidence for self-facilitation mechanisms. Mar. Ecol. Prog. Ser. 224, 93–101. doi: 10.3354/meps224093
Anderson R. J., Carrick P., Levitt G. J., Share A. (1997). Holdfasts of adult kelp Ecklonia maxima provide refuges from grazing for recruitment of juvenile kelps. Mar. Ecol. Prog. Ser. 159, 265–273. doi: 10.3354/meps159265
Angelini C., Griffin J. N., van de Koppel J., Lamers L. P., Smolders A. J., Derksen-Hooijberg M., et al. (2016). A keystone mutualism underpins resilience of a coastal ecosystem to drought. Nat. Commun. 7, 12473. doi: 10.1038/ncomms12473
Aquilino K. M., Bracken M. E., Faubel M. N., Stachowicz J. J. (2009). Local-scale nutrient regeneration facilitates seaweed growth on wave-exposed rocky shores in an upwelling system. Limnol. Oceanogr. 54, 309–317. doi: 10.4319/lo.2009.54.1.0309
Arribas L. P., Bagur M., Gutiérrez J. L., Palomo M. G. (2015). Matching spatial scales of variation in mussel recruitment and adult densities across southwestern Atlantic rocky shores. J. Sea Res. 95, 16–21. doi: 10.1016/j.seares.2014.10.015
Arribas L. P., Bagur M., Klein E., Penchaszadeh P., Palomo M. G. (2013). Geographic distribution of two mussel species and associated assemblages along the northern Argentinean coast. Aquat. Biol. 18, 91–03. doi: 10.3354/ab00495
Atanasoska K. (2021). Caracterización bioclimática de Mar del Plata: recomendaciones para el diseño arquitectónico. Investigación + Acción 24, 13–34.
Bagur M., Gutiérrez J. L., Arribas L. P., Palomo M. G. (2016). Complementary influences of co-occurring physical ecosystem engineers on species richness: insights from a Patagonian rocky shore. Biodivers. Conserv. 25, 2787–2802. doi: 10.1007/s10531-016-1203-x
Barros V. R., Boninsegna J. A., Camilloni I. A., Chidiak M., Magrín G. O., Rusticucci M. (2015). Climate change in Argentina: trends, projections, impacts and adaptation. Wiley Interdisciplin. Rev. Clim. Change 6, 151–169. doi: 10.1002/wcc.316
Becherucci M. E., Benavides H., Vallarino E. A. (2014). Effect of taxonomic aggregation in macroalgae assemblages in a rocky shore of Mar del Plata, Argentina, Southwest Atlantic Ocean. Thalassas 30, 9–20.
Bers A. V., D'Souza F., Klijnstra J. W., Willemsen P. R., Wahl M. (2006). Chemical defence in mussels, antifouling effect of crude extracts of the periostracum of the blue mussel Mytilus edulis. Biofouling 22, 251–259. doi: 10.1080/08927010600901112
Blouin N. A., Brodie J. A., Grossman A. C., Xu P., Brawley S. H. (2011). Porphyra: a marine crop shaped by stress. Trends Plant Sci. 16, 29–37. doi: 10.1016/j.tplants.2010.10.004
Boraso A., Zaixso J. M. (2011). “Algas marinas bentónicas,” in Atlas de Sensibilidad Ambiental de la Costa y el Mar Argentino. Ed. Boltovskoy D. (Buenos Aires, Argentina: Secretaría de Ambiente y Desarrollo Sustentable), 1–28.
Borthagaray A. I., Carranza A. (2007). Mussels as ecosystem engineers: their contribution to species richness in a rocky littoral community. Acta Oecol. 31, 243–250. doi: 10.1016/j.actao.2006.10.008
Bozec Y. M., Yakob L., Bejarano S., Mumby P. J. (2013). Reciprocal facilitation and non-linearity maintain habitat engineering on coral reefs. Oikos 122, 428–440. doi: 10.1111/j.1600-0706.2012.20576.x
Bulleri F., Eriksson B. K., Queirós A., Airoldi L., Arenas F., Arvanitidis C., et al. (2018). Harnessing positive species interactions as a tool against climate-driven loss of coastal biodiversity. PloS Biol. 16, e2006852. doi: 10.1371/journal.pbio.2006852
Callow M. E., Fletcher R. L. (1994). The influence of low surface energy materials on bioadhesion—a review. Int. Biodeterior. Biodegrad. 34, 333–348. doi: 10.1016/0964-8305(94)90092-2
Clausing R. J., de la Fuente G., Falace A., Chiantore M. (2023). Accounting for environmental stress in restoration of intertidal foundation species. J. Appl. Ecol. 60, 305–318. doi: 10.1111/1365-2664.14334
Contreras-Porcia L., López-Cristoffanini C., Meynard A., Kumar M. (2017). “Tolerance pathways to desiccation stress in seaweeds,” in Systems Biology of Marine Ecosystems. Eds. Kumar M., Ralph P. (Cham: Springer), 13–33.
Contreras-Porcia L., Meynard A., Piña F., Kumar M., Lovazzano C., Núñez A., et al. (2022). Desiccation stress tolerance in Porphyra and Pyropia species: a latitudinal analysis along the Chilean coast. Plants 12, 12. doi: 10.3390/plants12010012
de Fouw J., van der Heide T., van Belzen J., Govers L. L., Cheikh M. A. S., Olff H., et al. (2018). A facultative mutualistic feedback enhances the stability of tropical intertidal seagrass beds. Sci. Rep. 8, 12988. doi: 10.1038/s41598-018-31060-x
Denny M. W., Dowd W. W., Bilir L., Mach K. J. (2011). Spreading the risk: small-scale body temperature variation among intertidal organisms and its implications for species persistence. J. Exp. Mar. Biol. Ecol. 400, 175–190. doi: 10.1016/j.jembe.2011.02.006
Derksen-Hooijberg M., Angelini C., Lamers L. P., Borst A., Smolders A., Hoogveld J. R., et al. (2018). Mutualistic interactions amplify saltmarsh restoration success. J. Appl. Ecol. 55, 405–414. doi: 10.1111/1365-2664.12960
Dittman D., Robles C. (1991). Effect of algal epiphytes on the mussel Mytilus californianus. Ecology 72, 286–296. doi: 10.2307/1938922
Ellison A. M., Bank M. S., Clinton B. D., Colburn E. A., Elliott K., Ford C. R., et al. (2005). Loss of foundation species: consequences for the structure and dynamics of forested ecosystems. Front. Ecol. Environ. 3, 479–486. doi: 10.1890/1540-9295(2005)003[0479:LOFSCF]2.0.CO;2
Fields P. A., Graham J. B., Rosenblatt R. H., Somero G. N. (1993). Effects of expected global climate change on marine faunas. Trends Ecol. Evol. 8, 361–367. doi: 10.1016/0169-5347(93)90220-J
Fiore M. M., D’Onofrio E. E., Pousa J. L., Schnack E. J., Bertola G. R. (2009). Storm surges and coastal impacts at Mar del Plata, Argentina. Cont. Shelf Res. 29, 1643–1649. doi: 10.1016/j.csr.2009.05.004
Fitzgerald-Dehoog L., Browning J., Allen B. J. (2012). Food and heat stress in the California mussel: evidence for an energetic trade-off between survival and growth. Biol. Bull. 223, 205–216. doi: 10.1086/BBLv223n2p205
Fletcher R. L., Callow M. E. (1992). The settlement, attachment and establishment of marine algal spores. Br. Phycol. J. 27, 303–329. doi: 10.1080/00071619200650281
Flores A. A., Christofoletti R. A., Peres A. L. F., Ciotti A. M., Navarrete S. A. (2015). Interactive effects of grazing and environmental stress on macroalgal biomass in subtropical rocky shores: modulation of bottom-up inputs by wave action. J. Exp. Mar. Biol. Ecol. 463, 39–48. doi: 10.1016/j.jembe.2014.11.001
Galil B. S., Mienis H. K., Mendelson M., Gayer K., Goren M. (2022). Here today, gone tomorrow-the Levantine population of the Brown mussel Perna perna obliterated by unprecedented heatwave. Aquat. Invasions 17, 174–185. doi: 10.3391/ai.2022.17.2.03
Garrabou J., Coma R., Bensoussan N., Bally M., Chevaldonné P., Cigliano M., et al. (2009). Mass mortality in Northwestern Mediterranean rocky benthic communities: effects of the 2003 heat wave. Global Change Biol. 15, 1090–1103. doi: 10.1111/j.1365-2486.2008.01823.x
Giampietri L., Piccolo M. C. (2000). Diferencias climáticas en el área costera de la ciudad de Mar del Plata. Geoacta 25, 65–74.
Gowell M. R., Coombes M. A., Viles H. A. (2015). Rock-protecting seaweed? Experimental evidence of bioprotection in the intertidal zone. Earth Surf. Process. Landf. 40, 1364–1370. doi: 10.1002/esp.3736
Green T. J., Siboni N., King W. L., Labbate M., Seymour J. R., Raftos D. (2019). Simulated marine heat wave alters abundance and structure of Vibrio populations associated with the Pacific Oyster resulting in a mass mortality event. Microb. Ecol. 77, 736–747. doi: 10.1007/s00248-018-1242-9
Gutiérrez J. L., Bagur M., Arribas L. P., Palomo M. G. (2018). Does rock type account for variation in mussel attachment strength? A test with Brachidontes rodriguezii in the southwestern Atlantic. Helgol. Mar. Res. 72, 10. doi: 10.1186/s10152-018-0514-6
Gutiérrez J. L., Bagur M., Palomo M. G. (2019). Algal epibionts as co-engineers in mussel beds: effects on abiotic conditions and mobile interstitial invertebrates. Diversity 11, 17. doi: 10.3390/d11020017
Gutiérrez J. L., Hacker S. D., Coombes M. A., Wild C., Pereira-Filho G. H., Palomo M. G. (2022). “Marine hard bottom communities,” in Marine Biology: A Functional Approach to the Oceans and their Organisms. Eds. Pan J., Pratolongo P. (Boca Raton, FL: CRC Press), 226–267.
Gutiérrez J. L., Jones C. G., Byers J. E., Arkema K. K., Berkenbusch K., Committo J. A., et al. (2011). “Physical ecosystem engineers and the functioning of estuaries and coasts,” in Treatise on Estuarine and Coastal Science, vol. 7 . Eds. Wolanski E., McLusky D. (Amsterdam: Elsevier), 53–81. Functioning of Estuaries and Coastal Ecosystems, eds. C. H. R Heip, C. J. M. Philippart, and XXXJ. J. Middelburg.
Gutiérrez J. L., Jones C. G., Strayer D. L., Iribarne O. O. (2003). Mollusks as ecosystem engineers: the role of shell production in aquatic habitats. Oikos 101, 79–90. doi: 10.1034/j.1600-0706.2003.12322.x
Gutiérrez J. L., Palomo M. G. (2016). Increased algal fouling on mussels with barnacle epibionts: a fouling cascade. J. Sea Res. 112, 49–54. doi: 10.1016/j.seares.2016.04.002
Gutiérrez J. L., Palomo M. G., Bagur M., Arribas L. P., Soria S. A. (2015). Wave action limits crowding in an intertidal mussel. Mar. Ecol. Prog. Ser. 518, 153–163. doi: 10.3354/meps11086
Hanekom N. (2013). Environmental conditions during mass mortalities of the ascidian Pyura stolonifera (Heller) in the Tsitsikamma marine protected area. Afr. Zool. 48, 167–172.
Harley C. D.G. (2008). Tidal dynamics, topographic orientation, and temperature-mediated mass mortalities on rocky shores. Mar. Ecol. Prog. Ser. 371, 37-46. doi: 10.1890/0012-9615(2006)076[0461:MPOTSI]2.0.CO;2
Hay M. E., Parker J. D., Burkepile D. E., Caudill C. C., Wilson A. E., Hallinan Z. P., et al. (2004). Mutualisms and aquatic community structure: the enemy of my enemy is my friend. Annu. Rev. Ecol. Evol. Syst. 35, 175–197. doi: 10.1146/annurev.ecolsys.34.011802.132357
Helmuth B. S. (1998). Intertidal mussel microclimates: predicting the body temperature of a sessile invertebrate. Ecol. Monogr. 68, 51–74. doi: 10.1890/0012-9615(1998)068[0051:IMMPTB]2.0.CO;2
Helmuth B., Harley C. D., Halpin P. M., O’Donnell M., Hofmann G. E., Blanchette C. A. (2002). Climate change and latitudinal patterns of intertidal thermal stress. Science 298, 1015–1017. doi: 10.1126/science.107681
Helmuth B. S., Hofmann G. E. (2001). Microhabitats, thermal heterogeneity, and patterns of physiological stress in the rocky intertidal zone. Biol. Bull. 201, 374–384. doi: 10.2307/1543615
Helmuth B., Broitman B. R., Blanchette C. A., Gilman S., Halpin P., Harley C. D., et al. (2006). Mosaic patterns of thermal stress in the rocky intertidal zone: implications for climate change. Ecol. Monogr. 76, 461-479. doi: 10.1890/0012-9615(2006)076[0461:MPOTSI]2.0.CO;2
Hesketh A. V., Harley C. D. (2023). Extreme heatwave drives topography-dependent patterns of mortality in a bed-forming intertidal barnacle, with implications for associated community structure. Global Change Biol. 29, 165–178. doi: 10.1111/gcb.16390
Hesketh A. V., Schwindt E., Harley C. D. (2021). Ecological and environmental context shape the differential effects of a facilitator in its native and invaded ranges. Ecology 102, e03478. doi: 10.1002/ecy.3478
Hiebenthal C., Philipp E. E. R., Eisenhauer A., Wahl M. (2012). Interactive effects of temperature and salinity on shell formation and general condition in Baltic Sea Mytilus edulis and Arctica islandica. Aquat. Biol. 14, 289–298. doi: 10.3354/ab00405
Hoegh-Guldberg O., Mumby P. J., Hooten A. J., Steneck R. S., Greenfield P., Gomez E., et al. (2007). Coral reefs under rapid climate change and ocean acidification. Science 318, 1737–1742. doi: 10.1126/science.1152509
Hofmann G. E., Somero G. N. (1995). Evidence for protein damage at environmental temperatures: seasonal changes in levels of ubiquitin conjugates and hsp70 in the intertidal mussel Mytilus trossulus. J. Exp. Biol. 198, 1509–1518. doi: 10.1242/jeb.198.7.1509
Isla F. I., Taglioretti M., Dondas A. (2015). Revisión y nuevos aportes sobre la estratigrafía y sedimentología de los acantilados entre Mar de Cobo y Miramar, provincia de Buenos Aires. Rev. Asoc. Geol. Argent. 72, 235–250.
Jones C. G., Lawton J. H., Shachak M. (1994). Organisms as ecosystem engineers. Oikos 69, 373–386. doi: 10.2307/3545850
Jurgens L. J., Ashlock L. W., Gaylord B. (2022). Facilitation alters climate change risk on rocky shores. Ecology 103, e03596. doi: 10.1002/ecy.3596
Korbee N., Abdala Díaz R. T., Figueroa F. L., Helbling E. W. (2004). Ammonium and UV radiation stimulate the accumulation of mycosporine-like amino acids in Porphyra columbina (Rhodophyta) from Patagonia, Argentina. J. Phycol. 40, 248–259. doi: 10.1046/j.1529-8817.2004.03013.x
Kumar K. V., Bai R. K. (2008). Performance study on solar still with enhanced condensation. Desalination 230, 51–61. doi: 10.1016/j.desal.2007.11.015
Kordas R. L., Dudgeon S., Storey S., Harley C. D. (2015). Intertidal community responses to field‐based experimental warming. Oikos 124, 888-898. doi: 10.1111/oik.00806
Laudien J., Wahl M. (1999). Indirect effects of epibiosis on host mortality: seastar predation on differently fouled mussels. Mar. Ecol. 20, 35–47. doi: 10.1046/j.1439-0485.1999.00063.x
Leonard G. H. (2000). Latitudinal variation in species interactions: a test in the New England rocky intertidal zone. Ecology 81, 1015–1030. doi: 10.1890/0012-9658(2000)081[1015:LVISIA]2.0.CO;2
Lesser M. P. (2016). Climate change stressors cause metabolic depression in the blue mussel, Mytilus edulis, from the Gulf of Maine. Limnol. Oceanogr. 61, 1705–1717. doi: 10.1002/lno.10326
Li X. X., Tan Y., Sun Y. X., Wang J., Dong Y. W. (2021). Microhabitat temperature variation combines with physiological variation to enhance thermal resilience of the intertidal mussel Mytilisepta virgata. Funct. Ecol. 35, 2497–2507. doi: 10.1111/1365-2435.13885
Lima F. P., Gomes F., Seabra R., Wethey D. S., Seabra M. I., Cruz T., et al. (2016). Loss of thermal refugia near equatorial range limits. Global Change Biol. 22, 254-263. doi: 10.1111/gcb.13115
Lohse D. P. (1993). The importance of secondary substratum in a rocky intertidal community. J. Exp. Mar. Biol. Ecol. 166, 1–17. doi: 10.1016/0022-0981(93)90075-Y
López Gappa J. J., Tablado A., Magaldi N. H. (1990). Influence of sewage pollution on a rocky intertidal community dominated by the mytilid Brachidontes rodriguezii. Mar. Ecol. Prog. Ser. 63, 163–175. doi: 10.3354/meps063163
Micheli F., Heiman K. W., Kappel C. V., Martone R. G., Sethi S. A., Osio G. C., et al. (2016). Combined impacts of natural and human disturbances on rocky shore communities. Ocean Coast. Manage. 126, 42–50. doi: 10.1016/j.ocecoaman.2016.03.014
Mislan K. A. S., Wethey D. S. (2015). A biophysical basis for patchy mortality during heat waves. Ecology 96, 902–907. doi: 10.1890/14-1219.1
Miyamoto Y., Noda T. (2004). Effects of mussels on competitively inferior species: competitive exclusion to facilitation. Mar. Ecol. Progr. Ser. 276, 293–298. doi: 10.3354/meps276293
Navarro N. P., Mansilla A., Figueroa F. L., Korbee N., Jofre J., Plastino E. (2014). Short-term effects of solar UV radiation and NO3-supply on the accumulation of mycosporine-like amino acids in Pyropia columbina (Bangiales, Rhodophyta) under spring ozone depletion in the sub-Antarctic region, Chile. Bot. Mar. 57, 9–20. doi: 10.1515/bot-2013-0090
Notoya M., Nagaura K. (1998). Life history and growth of the epiphytic thallus of Porphyra lacerata (Bangiales, Rhodophyta) in culture. Algae 13, 207–211.
O’Connor N. E. (2010). Shore exposure affects mussel population structure and mediates the effect of epibiotic algae on mussel survival in SW Ireland. Estuar. Coast. Shelf Sci. 87, 83–91. doi: 10.1016/j.ecss.2009.12.011
O’Connor N. E., Crowe T. P., McGrath D. (2006). Effects of epibiotic algae on the survival, biomass and recruitment of mussels, Mytilus L. (Bivalvia: Mollusca). J. Exp. Mar. Biol. Ecol. 328, 265–276. doi: 10.1016/j.jembe.2005.07.013
Olabarria C., Gestoso I., Lima F. P., Vázquez E., Comeau L. A., Gomes F., et al. (2016). Response of two mytilids to a heatwave: the complex interplay of physiology, behaviour and ecological interactions. PLoS One 11, e0164330. doi: 10.1371/journal.pone.0164330
Palomo M. G., Bagur M., Calla S., Dalton M. C., Soria S. A., Hawkins S. J. (2019). “Biodiversity and interactions on the intertidal rocky shores of Argentina (South-West Atlantic),” in Interactions in the Marine Benthos: Global Patterns and Processes. Eds. Hawkins S. J., Bohn K., Firth L., Williams G. (Cambridge: Cambridge University Press), 164–189.
Penchaszadeh P. E. (1973). Ecología de la comunidad del mejillín (Brachidontes rodriguezii D'Orb.) en el mediolitoral rocoso de Mar del Plata, Argentina, el proceso de recolonización. Physis 32, 51–64.
Perkins S. E., Alexander L. V. (2013). On the measurement of heat waves. J. Clim. 26, 4500–4517. doi: 10.1175/JCLI-D-12-00383.1
Perkins S. E., Alexander L. V., Nairn J. R. (2012). Increasing frequency, intensity and duration of observed global heatwaves and warm spells. Geophys. Res. Lett. 39, L20714. doi: 10.1029/2012GL053361
Perkins-Kirkpatrick S. E., Gibson P. B. (2017). Changes in regional heatwave characteristics as a function of increasing global temperature. Sci. Rep. 7, 12256. doi: 10.1038/s41598-017-12520-2
Piatt J. F., Parrish J. K., Renner H. M., Schoen S. K., Jones T. T., Arimitsu M. L., et al. (2020). Extreme mortality and reproductive failure of common murres resulting from the northeast Pacific marine heatwave of 2014-2016. PLoS One 15, e0226087. doi: 10.1371/journal.pone.0226087
Raymond W. W., Barber J. S., Dethier M. N., Hayford H. A., Harley C. D., King T. L., et al. (2022). Assessment of the impacts of an unprecedented heatwave on intertidal shellfish of the Salish Sea. Ecology 103, e3798. doi: 10.1002/ecy.3798
Rico Y., Gómez Samus L., Bidegain J. C. (2020). Magnetoestratigrafía y parámetros magnéticos de los acantilados de San Eduardo del Mar. Buenos Aires, Argentina. Rev. Asoc. Geol. Argent. 77, 104–131.
Rossi S., Bramanti L., Gori A., Orejas C. (2017). Marine Animal Forests: The Ecology of Benthic Biodiversity Hotspots (Cham, Switzerland: Springer).
Rusticucci M., Kyselý J., Almeira G., Lhotka O. (2016). Long-term variability of heat waves in Argentina and recurrence probability of the severe 2008 heat wave in Buenos Aires. Theor. Appl. Climatol. 124, 679–689. doi: 10.1007/s00704-015-1445-7
Ruthrof K. X., Breshears D. D., Fontaine J. B., Froend R. H., Matusick G., Kala J., et al. (2018). Subcontinental heat wave triggers terrestrial and marine, multi-taxa responses. Sci. Rep. 8, 13094. doi: 10.1038/s41598-018-31236-5
Sahoo D., Baweja P., Kushwah N. (2007). Developmental studies in Porphyra Vietnamensis: a high-temperature resistant species from the Indian coast. J. Appl. Phycol. 18, 279–286. doi: 10.1007/s10811-006-9027-9
Santelices B., Correa J. (1985). Differential survival of macroalgae to digestion by intertidal herbivore molluscs. J. Exp. Mar. Biol. Ecol. 88, 183–191. doi: 10.1016/0022-0981(85)90037-1
Santelices B., Martínez E. (1988). Effects of filter-feeders and grazers on algal settlement and growth in mussel beds. J. Exp. Mar. Biol. Ecol. 118, 281–306. doi: 10.1016/0022-0981(88)90079-2
Scanes E., Parker L. M., O’Connor W. A., Dove M. C., Ross P. M. (2020). Heatwaves alter survival of the Sydney rock oyster, Saccostrea glomerata. Mar. pollut. Bull. 158, 111389. doi: 10.1016/j.marpolbul.2020.111389
Scardino A., de Nys R., Ison O., O’Connor W., Steinberg P. (2003). Microtopography and antifouling properties of the shell surface of the bivalve molluscs Mytilus galloprovincialis and Pinctada imbricata. Biofouling 19, 221–230. doi: 10.1080/0892701021000057882
Seuront L., Nicastro K. R., Zardi G. I., Goberville E. (2019). Decreased thermal tolerance under recurrent heat stress conditions explains summer mass mortality of the blue mussel Mytilus edulis. Sci. Rep. 9, 17498. doi: 10.1038/s41598-019-53580-w
Six D. L. (2009). Climate change and mutualism. Nat. Rev. Microbiol. 7, 686–686. doi: 10.1038/nrmicro2232
Soria S. A., Gutiérrez J. L., Gonzalez J. A., Callá S. L., Palomo M. G. (2022). Habitat properties and invertebrate composition in mussel-dominated rocky shores: a test of edge effects. Estuar. Coast. Shelf Sci. 277, 108035. doi: 10.1016/j.ecss.2022.108035
Soria S. A., Gutiérrez J. L., Palomo M. G. (2023). How climate-driven changes in disturbance frequency affect the recovery of intertidal mussel beds. J. Exp. Mar. Biol. Ecol. 562, 151885. doi: 10.1016/j.jembe.2023.151885
Sorte C. J., Bernatchez G., Mislan K. A. S., Pandori L. L., Silbiger N. J., Wallingford P. D. (2019). Thermal tolerance limits as indicators of current and future intertidal zonation patterns in a diverse mussel guild. Mar. Biol. 166, 1–13. doi: 10.1007/s00227-018-3452-6
Sousa R., Pilotto F., Aldridge D. C. (2011). Fouling of European freshwater bivalves (Unionidae) by the invasive zebra mussel (Dreissena polymorpha). Freshwat. Biol. 56, 867–876. doi: 10.1111/j.1365-2427.2010.02532.x
Stillman J. H. (2002). Causes and consequences of thermal tolerance limits in rocky intertidal porcelain crabs, genus Petrolisthes. Integr. Comp. Biol. 42, 790–796. doi: 10.1093/icb/42.4.790
Suchanek T. H. (1985). “Mussels and their role in structuring rocky shore communities,” in The Ecology of Rocky Coasts. Eds. Moore P. G., Seed R. (London: Hodder & Stoughton Press), 70–96.
Sutherland J. E., Lindstrom S. C., Nelson W. A., Brodie J., Lynch M. D., Hwang M. S., et al. (2011). A new look at an ancient order: generic revision of the Bangiales (Rhodophyta). J. Phycol. 47, 1131–1151. doi: 10.1111/j.1529-8817.2011.01052.x
Taylor A. M., Maher W. A., Ubrihien R. P. (2017). Mortality, condition index and cellular responses of Anadara trapezia to combined salinity and temperature stress. J. Exp. Mar. Biol. Ecol. 497, 172–179. doi: 10.1016/j.jembe.2017.09.023
Thomsen M. S., Mondardini L., Alestra T., Gerrity S., Tait L., South P. M., et al. (2019). Local extinction of bull kelp (Durvillaea spp.) due to a marine heatwave. Front. Mar. Sci. 6, 84. doi: 10.3389/fmars.2019.00084
Tokeshi M., Romero L. (1995). Filling a gap: dynamics of space occupancy on a mussel-dominated subtropical rocky shore. Mar. Ecol. Prog. Ser., 167–176. doi: 10.3354/meps119167
Trovant B., Orensanz J. L., Ruzzante D. E., Stotz W., Basso N. G. (2015). Scorched mussels (Bivalvia: Mytilidae: Brachidontinae) from the temperate coasts of South America: phylogenetic relationships, trans-Pacific connections and the footprints of Quaternary glaciations. Mol. Phylogenet. Evol. 82, 60–74. doi: 10.1016/j.ympev.2014.10.002
Tsuchiya M. (1983). Mass mortality in a population of the mussel Mytilus edulis L. caused by high temperature on rocky shores. J. Exp. Mar. Biol. Ecol. 66, 101–111. doi: 10.1016/0022-0981(83)90032-1
Wahl M. (2008). Ecological lever and interface ecology: epibiosis modulates the interactions between host and environment. Biofouling 24, 427–438. doi: 10.1080/08927010802339772
Wahl M., Kröger K., Lenz M. (1998). Non-toxic protection against epibiosis. Biofouling 12, 205–226. doi: 10.1080/08927019809378355
Wangkulangkul K., Hawkins S. J., Jenkins S. R. (2016). The influence of mussel-modified habitat on Fucus serratus L. @ a rocky intertidal canopy-forming macroalga. J. Exp. Mar. Biol. Ecol. 481, 63–70. doi: 10.1016/j.jembe.2016.04.007
Welbergen J. A., Klose S. M., Markus N., Eby P. (2008). Climate change and the effects of temperature extremes on Australian flying-foxes. Proc. R. Soc B Biol. Sci. 275, 419–425. doi: 10.1098/rspb.2007.1385
Wernegreen J. J. (2012). Mutualism meltdown in insects: bacteria constrain thermal adaptation. Curr. Opin. Microbiol. 15, 255–262. doi: 10.1016/j.mib.2012.02.001
Westley P. A. (2020). Documentation of en route mortality of summer chum salmon in the Koyukuk River, Alaska and its potential linkage to the heatwave of 2019. Ecol. Evol. 10, 10296–10304. doi: 10.1002/ece3.6751
Zárate M. A., Fasano J. L. (1989). The Plio-Pleistocene record of the central eastern Pampas, Buenos Aires province, Argentina: the Chapadmalal case study. Palaeogeogr. Palaeoclimatol. Palaeoecol. 72, 27–52. doi: 10.1016/0031-0182(89)90130-2
Keywords: climate change, foundation species, habitat-forming species, heat waves, mussels, mutualisms, physical ecosystem engineering, Porphyra/Pyropia
Citation: Gutiérrez JL, Bagur M, Lorenzo RA and Palomo MG (2023) A facultative mutualism between habitat-forming species enhances the resistance of rocky shore communities to heat waves. Front. Ecol. Evol. 11:1278762. doi: 10.3389/fevo.2023.1278762
Received: 16 August 2023; Accepted: 08 November 2023;
Published: 24 November 2023.
Edited by:
Guilherme O. Longo, Federal University of Rio Grande do Norte, BrazilReviewed by:
Henrique Borburema, Federal University of Rio Grande do Norte, BrazilCopyright © 2023 Gutiérrez, Bagur, Lorenzo and Palomo. This is an open-access article distributed under the terms of the Creative Commons Attribution License (CC BY). The use, distribution or reproduction in other forums is permitted, provided the original author(s) and the copyright owner(s) are credited and that the original publication in this journal is cited, in accordance with accepted academic practice. No use, distribution or reproduction is permitted which does not comply with these terms.
*Correspondence: Jorge L. Gutiérrez, amd1dGllcnJlekBncmlldGEub3JnLmFy
Disclaimer: All claims expressed in this article are solely those of the authors and do not necessarily represent those of their affiliated organizations, or those of the publisher, the editors and the reviewers. Any product that may be evaluated in this article or claim that may be made by its manufacturer is not guaranteed or endorsed by the publisher.
Research integrity at Frontiers
Learn more about the work of our research integrity team to safeguard the quality of each article we publish.