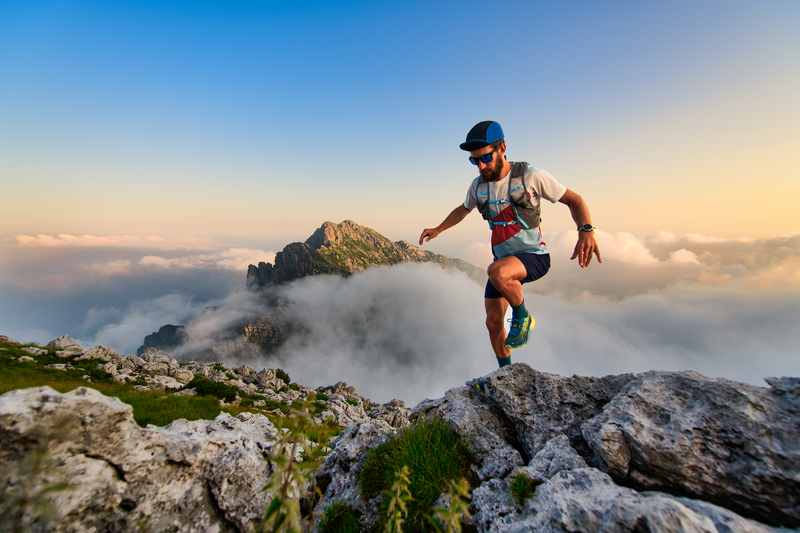
95% of researchers rate our articles as excellent or good
Learn more about the work of our research integrity team to safeguard the quality of each article we publish.
Find out more
REVIEW article
Front. Ecol. Evol. , 05 December 2023
Sec. Conservation and Restoration Ecology
Volume 11 - 2023 | https://doi.org/10.3389/fevo.2023.1268969
This article is part of the Research Topic Large-Scale Dam Removal and Ecosystem Restoration View all 25 articles
Large dam removals are increasing in frequency and the response of natural and managed revegetation is a critical consideration for managed restoration of dewatered reservoir landscapes post dam removal. The removal of two large dams on the Elwha River in 2011-2014 provides insight into reservoir revegetation. We review literature and datasets from 2012 through 2018, 1-6 years since reservoir dewatering, to compare pre-dam removal predictions on the Elwha to post-dam removal of natural revegetation, managed revegetation effects and invasive non-native vegetation response. Pre-dam removal hypotheses about natural revegetation did not predict species performance on reservoir sediments, seed rain patterns, or seed bank response. Sediment texture and landform affected multiple aspects of revegetation, including vegetation cover, species richness, woody stem densities and species composition. Reservoir drawdown timing influenced species composition and seedling densities. Predictions about managed revegetation effects were mixed. Planting trees and shrubs did not accelerate woody cover but did increase species richness. Seeding reduced non-native vegetation frequency and species richness, had no effect on vegetation cover on fine sediments, but increased vegetation cover on coarse sediments. Planting trees and shrubs during drawdown appeared to result in higher survival rates compared to plantings installed 1+ years post drawdown. Seeding Lupinus rivularis (riverbank lupine) on coarse sediments was successful and increased foliar nitrogen in planted conifers. Invasive non-native vegetation was correctly predicted to be more abundant in the Aldwell reservoir but did not preclude native species establishment in either reservoir, likely due to rapid establishment of native species and robust management that occurred before, during and after dam removal.
As more dams are being removed worldwide (O'Connor et al., 2015), more information is becoming available about the ecological consequences of dam removal and the outcomes of post-dam removal management strategies. Revegetation (natural or managed) of drained reservoirs is an important component of post-dam removal restoration, as the reservoir is often the part of the river most dramatically affected by dam emplacement and removal (Bellmore et al., 2019). Yet few data are available to describe vegetation responses in dewatered reservoirs, particularly following removal of large dams (O'Connor et al., 2015; Foley et al., 2017).
Revegetation of dewatered reservoirs is considered critical to successful restoration and is characterized by various biophysical interactions (Bellmore et al., 2019). Vegetation in former reservoirs provides habitat for upland and riparian fauna (Kelsey and West, 1998), and moderates erosion and stabilizes slopes (Riis et al., 2020), which is critical when lacustrine sediments are prevalent. Initial plant colonization after reservoir dewatering can be rapid, but it can take decades for mature forest vegetation to become sufficiently established to stabilize banks and terraces and fulfill other functions that enhance stream habitat (Shafroth et al., 2002; Orr and Stanley, 2006). Invasive plants represent a common management concern, as the initially bare, open nature of dewatered reservoirs makes them suitable for opportunistic pioneer species, many of which are non-native (Tullos et al., 2016). At some sites, non-native plants can colonize quickly and have an adverse effect on biodiversity and ecological condition (Orr and Stanley, 2006; Bellmore et al., 2019), but at many sites, natural (i.e., passive) revegetation results in a proportion of non-native species that is no greater than that found in typical riparian communities (Tullos et al., 2016). Diverse communities may develop (Ravot et al., 2020), but vegetation recovery might not result in a return to pre-dam conditions (Foley et al., 2017; Bellmore et al., 2019).
The objectives of this synthesis are to characterize revegetation trajectories in former reservoirs following the removal of two large dams on the Elwha River, and to consider how responses compare to hypotheses and predictions about revegetation generated prior to dam removal. Our synthesis is based on a review of literature and datasets. The main revegetation drivers were 1) biotic – the availability of species for colonization (e.g., distance from seed sources, seed banks, native species planting and seeding, invasive species), and 2) abiotic– the physical factors that affect plant growth (e.g., reservoir drawdown timing, sediment texture, geomorphic landforms, large woody debris placement). Finally, we discuss key lessons that may apply to other dam removal revegetation projects.
The Elwha River drains 833 km2 of forested mountain terrain, primarily in Olympic National Park in Washington state, USA (Figure 1; from Chenoweth et al., 2022). Two large dams were removed on the Elwha River between 2011 and 2014, exposing two former reservoirs. Elwha Dam, completed in 1912, was 33-m tall and impounded Aldwell Reservoir, which extended 4.8 km upstream from the dam (river km 7.9-12.7). Glines Canyon Dam, completed in 1927, was 64-m tall and impounded Lake Mills reservoir, which extended 3.8 km upstream from the dam (river km 21-24.8).
Figure 1 Map of the Elwha watershed, located west of Port Angeles, Washington, USA. The headwaters of the Elwha River reside 72 km within the Olympic Mountains where it flows south to north into the Strait of Juan de Fuca. Map illustrates the placement of the two former dams (in red); Elwha dam and Glines Canyon with former Lake Aldwell (river km 7.9-12.7) and Lake Mills (river km 21-24.8) labeled in purple.
The upland vegetation surrounding the reservoirs are dense low-elevation coastal forests common in Western Washington, dominated by 100-300-year-old conifer species such as Pseudotsuga menziesii (Douglas-fir), Abies grandis (grand fir) and Tsuga heterophylla (western hemlock). Species characteristic of the understory are mid-to-late seral species such as Polystichum munitum (sword fern), Gaultheria shallon (salal), Berberis nervosa (dull Oregon-grape), Linnaea borealis (twinflower) and Rubus spectabilis (salmonberry). Common riparian vegetation that also was found in thin bands along the reservoir shorelines include Alnus rubra (red alder), Acer macrophyllum (bigleaf maple), Populus balsamifera ssp. trichocarpa (black cottonwood), and Salix species (Prach et al., 2019). Non-native, invasive species are present but are not common in the surrounding forest matrix (Woodward et al., 2011).
The two reservoirs contained an estimated 21 million m3 of stored sediments at the start of dam removal (Randle et al., 2015; Warrick et al., 2015). In Lake Mills, where an estimated 16.1 million m3 of sediment were stored, over half (56%) of the sediments were coarse-grained (sands, gravels, cobbles) and confined to the Lake Mills delta in the upper reservoir that was 24-27 m thick and 1.5 km long (Randle et al., 2015). Fine sediments comprised 44% of the total and were mainly composed of silt and clay with some fine sands (Mussman et al., 2008; Cavaliere and Homann, 2012). Fine sediments covered the valley bottom (2-14 m deep) and the valley walls (2 m deep). In Lake Aldwell an estimated 4.9 million m3 of sediment were stored; 54% were fine-grained (silt and clay) and 46% were coarse (sands, gravel, cobbles; Randle et al., 2015). The Aldwell delta was estimated to be 6-8 m thick and 2.2 km long.
Initial drawdown of both reservoirs began in late May 2011 in preparation for dam removal. Power production ceased and the reservoirs dropped to the base of the spillway gates, a decrease of 4.5 m in water surface elevation, exposing a narrow band of uplands over a two-week period. Removal of the two dams and full reservoir dewatering began simultaneously in September 2011. Dam removal and reservoir drawdown was relatively rapid for the Elwha Dam, ending in March 2012. The larger dam, Glines Canyon Dam, was designed to be removed more slowly to allow for the Elwha River to gradually erode the Mills reservoir delta. The reservoir pool was drained slowly over a period of 13 months, September 2011-October 2012 (Randle et al., 2015). Glines Canyon Dam removal was completed in August 2014. The newly exposed valleys included channels, floodplains, terraces, and valley wall landforms (Figure 2; from Chenoweth et al., 2022). Because the channel and floodplain areas were especially dynamic following dam removal, most studies and management actions focused on the terraces and valley walls.
Figure 2 Stylized profile (not to scale) of the dewatered reservoir from forest edge to river. Fine sediments accumulated throughout the reservoir while the dam was in place. During dam removal, coarse sediments from the delta eroded and were redeposited as terraces on top of fine sediments. In the floodplain, the river eroded both fine sediments and terraces (Chenoweth et al., 2022).
The phased removal of Glines Canyon Dam successfully eroded the Mills reservoir delta, re-depositing sediments into new terraces 2-10 m thick covering the valley bottom (Randle et al., 2015). These new deposits overtopped fine sediments, resulting in novel terrace landforms 6-18 m thick (Figure 2). Many of the terraces eroded away after dam removal was completed (Ritchie et al., 2018), but approximately 69 ha of terraces remained in 2016. Most of the terrace erosion occurred during high flow events in the first few years after dam removal. Erosion has continued since 2016 but with only minor loss of terraces (A. Ritchie, U.S. Geological Survey, oral personal communication, 2023).
Revegetation began simultaneously with dam removal in September 2011 and the initial 7-year revegetation effort ended in the fall of 2018 (Chenoweth et al., 2011). Revegetation approaches included planting containerized plants and bare root plants as well as manual seeding. During the first year (November 2011-March 2012) only container plants were planted; no seed was sown except for a small seeding trial at Aldwell reservoir (Baker, 2013). Seeding the reservoirs began in the fall of 2012, one year after the start of dam removal. In the former Mills reservoir, 218,116 plants were planted, and 2,193 kilograms of seed were sown on 14.1 ha of the valley wall and 44.0 ha of terraces. A substantial area was left to naturally revegetate; 25.6 ha of valley wall landforms and 18.7 ha of terraces. In the Aldwell reservoir, 85,764 rooted plants were installed, and 716 kg of seed were sown on 16.7 ha leaving 103.7 hectares to revegetate naturally. Most of the plantings were trees and shrubs representing 60 species (Supplementary Table 1). The seed mixes varied but were composed primarily of nine native species (Supplementary Table 2). Average seeding rate was 183 seeds/m2 on fine sediments and 488 seeds/m2 on coarse sediments (Chenoweth et al., 2022). Species composition and planting density varied by site and by year.
A coordinated effort between Olympic National Park and the Lower Elwha Klallam Tribe was implemented to control invasive plants in the watershed several years prior to dam removal, continuing through 2018. Invasive species management occurred annually from spring through fall with species-specific control methods targeting the species of concern lists outlined in the Elwha Revegetation Plan (Chenoweth et al., 2011). As soon as the reservoirs were drained, the new lands were systematically mapped annually and treated within the same year, following the methods in Woodward et al. (2011). Treatments included the use of herbicides and mechanical removal. All areas were treated in both reservoirs including unplanted and unseeded areas.
The Lower Elwha Klallam Tribe and the Olympic National Park in 2012 and 2014 translocated 835 logs (log boles and logs with attached root-wads) by helicopter into the former Mills reservoir onto the coarse-textured terraces formed during dam removal. The logs were arranged in single, parallel, and overlapping configurations (Colton, 2018). Roughly two-thirds of the translocation area was planted and seeded.
The Elwha River reservoirs were large, with many newly exposed surfaces expected to be far from native seed sources, limiting revegetation (Chenoweth et al., 2011). Seed banks, an important source of new vegetation after disturbance, were predicted to be limited in density and species (Brown and Chenoweth, 2008; Michel et al., 2011).
Prach et al. (2019) and Chenoweth et al. (2022) found distance from seed sources in the former Mills reservoir was a significant explanatory revegetation variable. Prach et al. (2019) determined that distance from the forest edge affected species composition in 2015, 2-3 years after reservoir drawdown. Chenoweth et al. (2022) further documented that distance from forest edge affected bare ground, species composition and species richness in 2016. However, both articles note that coarse-textured sediment, exclusive to terrace landforms, were predominantly located in the middle of the dewatered reservoir and, on average, farther from seed sources compared to the fine sediments covering the valley wall landforms. The interaction between landform, sediment texture and distance from forests was likely a contributing factor. On the coarse-textured terraces, Cendejas-Zarelli (2021) reported that as distance from the forest edge increased, diversity, species richness, and percent non-native species declined. However, plant abundance did not change significantly with distance. Instead, further distances favored wind-dispersed species and excluded plants with gravity and ballistic dispersal mechanisms.
The seed bank may have played a significant role in revegetation (Figure 3). Vegetation cover in the early years after dam removal was dominated by species commonly found in soil seed banks. The six most abundant species detected in pre-dam removal seed bank studies, including Juncus species (rushes), the most abundant seed bank species), Carex deweyana var. deweyana (Dewey’s sedge), A. rubra, Epilobium ciliatum ssp. ciliatum (fringed willowherb), Equisetum species (horsetail), and the non-native Mycelis muralis (wall lettuce; Brown and Chenoweth, 2008), were common in the drained reservoirs.
Figure 3 Juncus species, Carex species and other wetland plants quickly germinated from a seed bank in 2012. Photograph is from the east side of the former Mills reservoir, July 24, 2012. Photograph provided by Joshua Chenoweth.
There were several hypotheses and predictions associated with managed revegetation. Chenoweth et al. (2011) predicted that planting and seeding native species would 1) minimize the abundance of non-native, invasive species, 2) accelerate vegetation growth (cover) relative to unplanted and unseeded areas, 3) increase native species diversity and richness, and 4) alter plant community composition.
The four studies looking at the effects of seeding and planting in the former Mills reservoir (Morgan, 2018, Prach et al. 2019, Cendejas-Zarelli, 2021; Chenoweth et al., 2022) did not always have consistent results. However, most showed that seeding reduced non-native species relative frequency, species richness, or abundance on coarse sediments (Morgan, 2018; Cendejas-Zarelli, 2021; Chenoweth et al., 2022). Half of the studies showed that seeding and planting altered species composition (Morgan, 2018; Chenoweth et al., 2022), and one each showed that seeding or planting increased species richness (Cendejas-Zarelli, 2021; Chenoweth et al., 2022) as described below.
Chenoweth et al. (2022) assessed the effect of planting and seeding on species richness, relative frequency of non-native species, and species composition in 2016, using permutational multivariate analysis of variance and nonmetric multidimensional scaling (NMDS) ordinations. They found that planting increased native species richness while seeding significantly reduced the relative frequency of non-native species on both fine and coarse-textured sediments. Planting and seeding both altered species composition. They used the same analysis to assess the effects of seeding and planting on percent bare ground from 2013 to 2017. They found that seeding reduced bare ground in all years on coarse sediments with substantial reductions by 2016, but had no effect on fine sediments.
Using 60 100-m2 plots on Lake Mills, including some sampled at the same locations as the Chenoweth et al. (2022) plots, with three treatments (seeding, planting + seeding, control) stratified across valley wall and terrace landforms, Morgan (2018) found that both seeded and seeded + planted plots had lower non-native species richness on terrace landforms with coarse sediments in 2017. Surprisingly, seeding (only) also reduced native species richness on coarse-textured landforms. Neither planting nor seeding affected percent cover. Using PERMANOVA analysis and NMDS, Morgan (2018) also found that treatment interacted with landform to significantly affect plant community composition. On valley walls, A. rubra was the most abundant species across all treatments; however, Thuja plicata (western redcedar), a planted species, was an indicator species for planted plots.
Cendejas-Zarelli (2021) surveyed plants in seeded and unseeded plots on coarse sediment terraces with varying degrees of large woody debris (LWD) cover in Lake Mills in 2016. Each plot consisted of six 1-m2 quadrats clustered around LWD or a 2 x 3-m plot without wood. She found that, in seeded areas, species richness and plant abundance increased and percent non-native species declined, results consistent with Chenoweth et al. (2022). She also found that large woody debris and seeding interacted to affect plant abundance, with greater abundance associated with single logs, and no difference where there was no large woody debris or clusters of logs.
Finally, Prach et al. (2019) assessed revegetation effects in 2015. This study measured species cover in 50-m2 plots in the same locations sampled by Chenoweth et al. (2022) and analyzed the data using detrended correspondence analysis (DCA) and canonical correspondence analysis. The results of this work indicated that seeding and planting did not significantly affect the plant community in terms of overall species richness, non-native species richness or wetland species richness.
The most common species on seeded and planted + seeded plots on the coarse-textured terraces, where it thrived, was Lupinus rivularis (riverbank lupine), a seeded species (Morgan, 2018). Lupinus rivularis is a 30 to 150 cm tall pioneer species adapted to well drained sandy or gravelly soils and may grow as an annual, biennial, or short-lived perennial (Darris and Young-Mathews, 2012). As a legume, L. rivularis assimilates nitrogen (N) through N-fixing Rhizobia bacteria that form an endosymbiotic association in root nodules (Staniewski, 1970). Nitrogen fixing species such as Lupinus spp. can enhance N availability in nutrient-limited soils (Myrold and Huss-Danell, 2003). However, the dense herbaceous canopy created by L. rivularis may hinder facilitation through competition and negatively affect native seedling survival (Morris and Wood, 1989; Walker and del Moral, 2003), or alternatively, L rivularis may facilitate soil development and promote plant community recovery (Bishop, 2002; del Moral, 2007).
Kardouni et al. (2023) examined the effects of L. rivularis density on growth of planted conifers, specifically P. menziesii, A. grandis, and Pinus monticola (western white pine), and on neighboring plant species composition and ectomycorrhizal (ECM) root tip colonization, three years after planting on the Mills reservoir terraces. Dense L. rivularis increased foliar nitrogen in planted conifers without reducing growth, indicating nitrogen provided by lupine detritus is facilitating conifer growth. Dense L. rivularis cover also reduced herbaceous species richness compared to sparse stands of L. rivularis, which included a reduction in the non-native grasses Holcus lanatus (common velvet grass) and Vulpia bromoides (brome fescue). ECM root colonization increased in sparse plots and was lower on conifer roots in the medium and dense lupine plots.
Non-native, invasive plant species were expected to dominate new landforms after reservoir drawdown with a more pervasive presence at the Aldwell reservoir due to its proximity to more developed areas with non-native plant populations (Woodward et al., 2011). Prior to dam removal non-native plant species were prioritized for control into three categories: primary species of concern, secondary species of concern, and species of concern that were not locally present but potentially could appear (“watch list”; Chenoweth et al., 2011).
Invasive, non-native species of concern were not prominent in the Chenoweth et al. (2022) plots in any year. One species considered to be a secondary species of concern for management (Chenoweth et al., 2011) was significant in the plot data: H. lanatus. This species increased in frequency each year and was particularly abundant on fine sediments on the valley wall. It was never treated, as treatments were focused on primary species of concern (Chenoweth et al., 2011). Indicator species analysis by Morgan (2018) revealed that the Aldwell reservoir had relatively more non-native species as indicators than the Mills reservoir, such as Lapsana communis (nipplewort), Leucanthemum vulgare (ox-eye daisy), and Agrostis stolonifera (creeping bentgrass), consistent with pre-dam removal predictions (Woodward et al., 2011). None of these species were primary species of concern and consequently were not treated.
Targeted invasive species mapping surveys were conducted annually across both reservoirs following the methods outlined in Woodward et al. (2011). The Aldwell reservoir contained many more observations in all years compared to the Mills reservoir, as expected (Supplementary Figure 1). Plot surveys of non-native species confirmed these patterns: In 2013, Schuster (2015) found that the Aldwell reservoir had over four times greater non-native species richness (9 vs. 2 species per 100 m2) and nine times greater non-native percent cover (9% vs. 1% in 100-m2 plots) than the Mills reservoir. However, these differences were no longer statistically significant by 2014 due to an increase in non-native species richness and cover in Lake Mills and high variability in Lake Aldwell (Schuster, 2015). In the Aldwell reservoir there was a spike in observations of targeted invasive species in 2014 outside of the randomized plot surveys (Supplementary Figure 2). Management of targeted invasive species contributed to a rapid decline in observations from 2015-2017 (Supplementary Figure 2). Targeted invasive species remained rare enough to not be prevalent in any of the randomized plot studies in any year. In the Schuster plots, non-native cover gradually increased with time after dam removal on both reservoirs, and by 2016 was slightly higher on the Aldwell reservoir, and highest on valley walls compared to other landforms (Mills: 17% valley walls, 6% terraces, 2% riparian, Aldwell: 22% valley walls, 15% terraces, 3% riparian; Morgan, 2018). The proportion of total species that were non-native in 100-m2 plots ranged from 21% on Aldwell valley walls to 38% on Aldwell terraces. In the Mills reservoir, non-native species cover was around 25% for all landforms. Hence, while non-native species were not dominant, they were certainly present, and warranted careful observation.
Phalaris arundinacea (reed canary grass) was particularly abundant in the Aldwell reservoir and its initial distribution suggests a seed bank may have been prevalent or seed was water-transported during reservoir drawdown. Active removal efforts likely limited the effect of primary species of concern and minimized their abundance, preventing direct competition effects on establishing native species.
Reservoir drawdown timing was predicted to be an important variable for plant community succession (Shafroth et al., 2002). Spring drawdown was expected to recruit riparian species such as Salicaceae species as moist, bare soil availability would coincide with germination windows (Mahoney and Rood, 1998; Auble et al., 2007), while summer drawdown was expected to result in few naturally occurring species, particularly in areas far from seed sources (Michel et al., 2011). Fall and winter drawdown was expected to benefit natural recruitment across groups as it occurs during peak seed rain of important native species such as T. heterophylla, P. menziesii, T. plicata and A. rubra (Burns and Honkala, 1990). Reservoir drawdown variability spanning several seasons was consequently expected to provide opportunities for diverse species to colonize the newly exposed sediments.
Reservoir drawdown timing affected several vegetation metrics. Naturally regenerating communities differed significantly with sediment exposure timing and among the two reservoirs in 2013; these differences persisted in 2015 (Werner, 2014). When combined with functional trait metrics from the TRY Plant Trait database (TRY Plant Trait Database (try-db.org); Kleyer et al., 2008; Kattge et al., 2011), drawdown timing-based differences in species establishment also translated to a difference in community-weighted functional trait metrics, with higher values of specific leaf area, seed mass, and maximum canopy height in the earlier-exposed soils.
As predicted, areas exposed during late spring were dominated by Salicaceae species. In the former Aldwell reservoir, areas exposed during the spring 2011 partial drawdown had a mean stem density of 71,200/ha in 2014, five times higher than areas exposed in fall 2011 through winter 2012 (13,600/ha in 2014). The dominant species in these sites were P. balsamifera ssp. trichocarpa (47,200/ha) and Salix species, largely S. sitchensis (Sitka willow; 20,020/ha). Fine sediment sites exposed in the fall and late winter/early spring in the Mills reservoir were dominated by A. rubra, constituting more than 50% of all stems by 2016 (Chenoweth et al., 2022).
Drawdown timing also affected species colonization of coarse sediments. Coarse sediment terraces exposed in late fall through spring were not readily colonized by A. rubra, even at sites directly adjacent to mature stands. In late spring of 2012, the erosion and re-deposition of the reservoir delta sediments occurred during the seed dispersal time of Salicaceae species while reservoir drawdown was on hold for 10 weeks beginning May 1st (Bountry et al., 2015). During this hold period, the newly formed terraces were inundated by multiple braided channels, allowing P. balsamifera (referred to without the subspecies name in all subsequent references) and Salix species to establish.
When reservoir drawdown resumed, over a 12-18-month period the river eroded down to the original river bed elevation, leaving a 12-ha terrace perched 16 m above the floodplain. Seedlings of P. balsamifera persisted despite the relatively fast, steep drop in the water table, and by 2017 the stem density was 9,600/ha with no mortality noted in the permanently marked sampling plots used in the Chenoweth et al. (2022) study. In contrast, in the 12 plots with coarse sediments located on terraces that did not form in late spring, mean stem density was only 300/ha, and nine of those plots had no woody stems or seedlings.
Sediment texture was predicted to vary among landforms and reservoirs, with more coarse sediment expected to be trapped in the upstream reservoir (Mills). Coarse sediments (coarse sands, gravels, and cobbles) were predicted to be deposited during drawdown into high terrace landforms several meters above the water table, which was a concern for project managers, as they were predicted to create a water-stressed environment that would negatively affect revegetation (Auble et al., 2007; Chenoweth et al., 2011). Woody species were expected to colonize coarse-textured sediments, whereas fine-textured (silt and clay) lacustrine sediments were predicted to favor fine-rooted plants such as grasses and forbs (Grubb, 1986) and preclude the establishment of woody species, including a common early colonizer, A. rubra (Shafroth et al., 2002; Chenoweth, 2007; Chenoweth et al., 2011). Plant-growing trials using fine sediments dredged from Lake Mills indicated that fine lacustrine soils favored germination and establishment of native grasses over native forbs and A. rubra (Chenoweth, 2007; Chenoweth et al., 2011) and hampered germination of native shrubs and forbs as well as the potentially invasive non-native species Cirsium arvense (Canada thistle) and Rubus discolor (Himalayan blackberry; Michel et al., 2011).
Different landforms developed within the reservoirs, distinguished based on elevation above river channel, distance from river channel and forest edge, and average coarse particle size (Table 1). The main landform types include valley walls, where the original pre-dam geomorphology is still present, as well as terraces formed from reservoir sediment redeposited during dam removal, and the low elevation, dynamic channels, bars, and floodplains (hereafter ‘riparian’), where fluvial process continue to shape channel morphology. Observations during and immediately after dam removal confirmed predictions that some landforms would be dominated by fine sediments and others by coarse sediments (Schuster, 2015; Morgan, 2018; Prach et al., 2019; Chenoweth et al., 2022). Generally finer sediments composed of silt and clay were found on valley walls, and coarser sediments composed of sand and cobbles were found on terraces and riparian landforms (Table 1, Schuster, 2015; Morgan, 2018). As predicted, the grain sizes were generally larger on the Mills reservoir than Aldwell reservoir (Figure 4). The finer sediments of valley walls and in Lake Aldwell had more organic matter and higher cation exchange capacity than the coarse sediments, and were associated with greater plant cover (Werner, 2014; Schuster, 2015; Morgan, 2018).
Table 1 Differences in elevation above river channel, distance from river channel and forest edge, and average coarse particle size among landforms and former reservoir beds observed in 2013 on the Elwha River, Washington, USA.
Figure 4 Log-transformed cumulative frequency curves comparing coarse sediment distribution between the Aldwell and Mills reservoirs along the Elwha River, WA. Sediment grain sizes were averaged between 2013 and 2014 for each reservoir. Grain sizes (x-axis) are plotted against the frequency in which they occur within each reservoir (y-axis). Squares, triangles, and rhombuses represent 2013-2014 landform average D50s (D50 - median grain size based on Wolman Pebble Counts). Asterisk denotes significance between the Mills valley walls and riparian landforms (p=0.001); number signs denote significance between the Mills and Aldwell terraces (p=0.003) (Schuster, 2015).
Predictions about which groups of species would colonize fine vs. coarse sediments were not consistently borne out. On fine sediments, plant performance exceeded expectations. Within a year, herbaceous and woody plants covered fine sediments on valley walls and some terraces of Lake Aldwell. One species in particular, A. rubra, rapidly colonized fine sediments (Prach et al., 2019; Chenoweth et al., 2022), defying predictions. In 2012, nine months after the start of dam removal, mean density of A. rubra seedlings was 0.43 per m2 (4,300 per ha) in unplanted plots. Seedlings successfully established, with the number of woody stems (>30 cm in height) increasing each year, reaching a peak of 1.4 per m2 in 2015 before slowly declining to 1.1 per m2 by 2017. Populus balsamifera and S. sitchensis also thrived, with mean densities of 0.7 per m2 and 0.3 per m2 by 2017. Although overall richness of woody species started low, with a mean of 3.5 species/50 m2 in 2012, richness increased annually to a peak mean of 6.2 species/50 m2 in 2017 (Chenoweth et al., 2022).
Woody species did not readily colonize coarse sediments except where draw-down timing matched establishment needs (Chenoweth et al., 2022). Alnus rubra was rare on coarse sediments, while P. balsamifera and S. sitchensis were present predominantly on one large terrace where the drawdown timing coincided with Salicaceae seed dispersal in May and June (Chenoweth et al., 2022). By 2016, mean densities of woody species on unplanted coarse sediments was 2,488 per ha compared to 26,882 per ha on unplanted fine sediments (Chenoweth et al., 2022). However, most of the stems in the unplanted terrace plots were counted in four plots located on the only terrace landform exposed during Salicaceae seed dispersal (Chenoweth et al., 2022). The remaining 10 untreated plots located on terraces that did not form during the May-June period had very few woody stems, with a mean of 360 stems/ha in 2016. Of those 10 plots, seven contained no woody stems at all.
Several studies found that plant species composition and richness were affected by sediment texture and landform (Schuster, 2015; Morgan, 2018; Prach et al., 2019; Chenoweth et al., 2022). Chenoweth et al. (2022) found that species richness was significantly higher on fine sediment landforms, with a mean of 23.4 species/50 m2 compared to 15.4 species on coarse sediments. This is consistent with findings of Schuster (2015), who found that in the first year following dam removal, native plant species richness and percent cover were greatest on valley wall landforms. On valley walls, terraces, and riparian landforms respectively in 2013, the Aldwell reservoir had 28, 19, and 9 species and 102%, 48%, and 18% cover, while the Mills reservoir had 20, 4, and 4 species and 42%, 4%, and 3% cover in 100-m2 plots; note that percent cover values can sum to more than 100% due to different layers of vegetation (Schuster, 2015).
The differences in plant diversity and cover among the two reservoirs was likely influenced by sediment texture. In 2013, the Aldwell reservoir had nearly twice as many native species per 100 m2 as the Mills reservoir (21 vs. 11), and had four times greater native percent cover (62.5% vs. 14.6%) across all landforms. In the second year (2014), species richness and cover increased in the Mills reservoir and there was no longer a significant difference between the two reservoirs. By year four (2016), species richness had increased on the Mills reservoir terrace and riparian landforms, and cover had increased on both reservoirs, though more on the Mills reservoir, making the two reservoirs more similar. In 2016, on valley wall, terrace, and riparian landforms respectively, the Aldwell reservoir had 22, 13, and 19 species and 185%, 82%, and 14% cover, while the Mills reservoir had 21, 13, and 14 species and 146%, 24%, and 6% cover (Morgan, 2018). Because valley walls had finer sediment (e.g., more silt/clay and smaller cobbles) compared to other landforms, and the Aldwell reservoir had finer sediments than the Mills reservoir, Schuster (2015) and Morgan (2018) concluded that finer sediments were more favorable for plant establishment and growth, consistent with the findings of Prach et al. (2019) and Chenoweth et al. (2022).
Texture and landform also influenced planted material. Whisman (2013) and Calimpong (2014) tagged planted species in the former Mills reservoir to determine sediment texture effects on short and long-term rates of survival and growth. Whisman tagged 860 plants (5 species) installed on fine and coarse sediments in 2011-2012 during the first year of reservoir drawdown. Calimpong tagged 675 plants (5 species) installed on fine and coarse sediments in 2012-2013 during the second year of dam removal on landforms exposed for over one year prior to planting. In both studies, survival after the first growing season was high (>80%). In the Whisman study, mortality was higher on coarse sediments, with a statistically significant difference in survival rates of 88% compared to 92% on fine sediments. Calimpong also found survival on fine sediments and associated higher soil moisture was significantly higher (96%) compared to coarse sediment sites (92% and 88%). Olympic National Park continued to monitor a subset of the tagged plants through 2017 to monitor long-term survival rates (5-6 years). This included 688 of the Whisman plants, of which 180 were on fine sediments and 508 were on coarse sediments, and 456 of the Calimpong plants, all located on the coarse sediment terraces. Survivorship of the Whisman plants, planted while the reservoir was being drained, remained high, with a mean of 82% surviving past the 2017 summer, substantially higher than plants installed in 2012-2013, which had a mean survival of only 33% in 2017 (Supplementary Figure 2).
Placement of large woody debris LWD was predicted to enhance managed and unmanaged revegetation by creating ‘safe sites’ for vegetation. LWD is used as a restoration tool to improve plant establishment by creating microenvironments that buffer temperature and wind velocities, provide shade, and enhance moisture retention in soils (Gray and Spies, 1997; Heinemann and Kitzberger, 2006; Calimpong, 2014; Colton, 2018). LWD adds perching sites for seed dispersers and may also protect vegetation by acting as a barrier against browsing ungulates (Harmon et al., 1986; Schreiner et al., 1996). Project managers predicted that LWD placement would result in higher plant abundance, diversity, and species richness.
Colton (2018) investigated LWD with east-west orientation and reported reduced wind speed; high wind speeds increase heat and evaporative stress (Wahid et al., 2007). In addition to direct stress to the plant, wind speeds can also contribute to soil erosion. Colton (2018) also demonstrated a reduction in sediment temperatures, which can inhibit or slow germination as well as contribute to overall plant mortality (Covell et al., 1986; MaChado and Paulsen, 2001) but may prove beneficial in extreme environments where high temperatures can hinder plant establishment.
Cendejas-Zarelli (2021) examined the effects of the translocated LWD and direct seeding on species composition. Counter to predictions, there were no differences in mean plant abundance between plots with and without LWD. However, all plots containing LWD did have higher plant diversity and species richness when compared to open areas without LWD. Plant species richness increased most when seeding was paired with LWD treatments. A diverse array of wind-dispersed forbs and grasses contributed to increased Shannon-Weiner diversity and species richness in plots. Because LWD acts as an effective trap for wind-blown seeds, it led to an increase in windblown non-native species, particularly when unseeded. In addition, all woody plants dispersed by birds were found in plots containing wood, with the majority around elevated root-wads, which likely attracted avian species that deposit scat at LWD perch sites. Counter to our predictions, there were no differences in mean plant abundance between plots with and without LWD (Cendejas-Zarelli, 2021).
Revegetation of the Elwha reservoirs after dam removal was rapid (Figure 5). On fine sediments (valley wall landforms), a rich assemblage of native species naturally colonized the reservoirs over a five-year period, often overwhelming planted sites. The rapid arrival and growth of predominantly riparian species such as A. rubra, P. balsamifera and Salix species exceeded expectations and resulted in a dense deciduous forest on valley walls. In contrast, revegetation was slower on coarse sediments (terrace landforms), likely due to moisture and nutrient limitations, although millions of slow growing P. balsamifera and Salix seedlings also colonized these landforms where reservoir drawdown matched moisture needs for germination (Mahoney and Rood, 1998). Results from the Elwha River corroborate previous work suggesting that drawdown timing can influence plant growth and survival as well as species composition and even community functional trait composition (Shafroth et al., 2002; Fukami et al., 2005; Auble et al., 2007). Timing the planting during or immediately after reservoir drawdown may also boost survival rates for many years compared to planting 1+ years post drawdown. It is unclear why survival rates were so much higher relative to later plantings but it may be due to the residual moisture that remained in the sediments as the reservoirs drained, creating favorable conditions for root growth in that critical first year of growth.
Figure 5 Photographs of the former Aldwell and Mills reservoirs on the Elwha River, Washington, USA. The Aldwell reservoir valley wall (A) in May, 2012, 2 months after full reservoir drawdown, (B) August 2013, 17 months after reservoir drawdown and (C) September 2016, 4 years post-drawdown. The Mills reservoir full valley showing floodplain, terraces and valley wall (D) April 2013, (E) August 2014 and (F) July 2017. Valley wall revegetation (upper margins in photograph) was rapid but significantly slower on terrace and floodplain landforms. Photographs provided by the National Park Service, available online at https://video-monitoring.com/construction/olympic/js.htm.
Contrary to expectations, invasive species did not dominate exposed sediments after dam removal, as has occurred in other dam removal studies (e.g., Lenhart, 2000; Orr and Koenig, 2006). This may have been due to active weed management of primary species of concern, rapid revegetation of areas with fine sediments by competitive native species such as A. rubra, and relatively harsh conditions on terraces with coarse sediments that slowed overall revegetation. The percentage of non-native species was similar to that of other dam removals (average 31% of species), while the relative abundance of non-natives (the highest of which was 22%) was slightly lower than in other dam removals (average 32%; Tullos et al., 2016). Over time, cover of non-native species gradually increased, largely comprising species that were not targets for removal. As expected, the Aldwell reservoir, which has larger amounts of nearby development and finer sediments, had relatively more non-native species than the more isolated Mills reservoir, consistent with studies showing the effect of human disturbance on non-native species invasion (Lázaro-Lobo and Ervin, 2021). One of the most problematic nonnative species in the Aldwell reservoir, P. arundinacea, is one of the most frequent management problems across many dam removal locations (Tullos et al., 2016). These results suggest that active management of species such as P. arundinacea can be effective after dam removal. However, monitoring the gradual increase of non-target nonnative species on the Elwha would contribute to understanding longer term invasive species dynamics following dam removal.
While the effects of seeding and planting were reduced on fine sediments due to the high cover of trees and shrubs, seeding and planting had a greater effect in coarse sediments, likely because natural recruitment was slower, with species richness and plant abundance metrics consistently lower compared to fine sediment landforms in all studies. Chenoweth et al. (2022); Cendejas-Zarelli (2021), and Morgan (2018) found significant reductions of non-native species in seeded sites, particularly on terraces with coarse sediments, but effects were weaker on valley walls with dense woody species.
Seeding L. rivularis was particularly successful on coarse sediments. Several studies in other environments have demonstrated the benefits of seeded Lupinus spp. in seral habitats, which include trapping seeds and leaf litter, creating microsites, reducing wind and water erosion, and increasing the establishment and survival rates of later successional woody species (Niederfriniger-Schlag and Erschbamer, 2000; Halvorson et al., 2005). Wind events alone can reduce leaf biomass by 36% in conifer forests (Kalma and Kuiper, 1966) and the presence of grass clumps and established shrubs have been demonstrated to decrease wind velocity by 70% and 40%, respectively (Mayaud et al., 2016). In the Elwha, moderate and dense stands of L. rivularis increased foliar N concentration in neighboring conifers. Increased foliar N levels can improve photosynthetic efficiency, increasing tree growth (Smethurst et al., 1986; Prietzel et al., 2008). As Lupinus spp. densities gradually decrease, their legacy facilitates soil development to promote plant community recovery (Bishop, 2002; del Moral, 2007). Though L. rivularis was associated with reduced species richness in the Elwha, non-native grasses were among the taxa suppressed. This may be important on coarse sediments that were not readily colonized by native species, leaving the site open and vulnerable to non-native species suited to open, dry conditions.
Comparisons of results among the different plot-based studies reviewed in this paper (Schuster, 2015; Morgan, 2018; Prach et al., 2019; Cendejas-Zarelli, 2021; Chenoweth et al., 2022) are complicated by inconsistencies in field methods and analytical approaches across the studies. For example, plot sizes varied among studies, which makes comparisons of some variables (e.g., species richness) challenging given the non-linear shape of species-area relationships (Dengler, 2009). Abundance measures were not consistent across studies, as some used percent cover (e.g., Prach et al., 2019) while others used other metrics such as relative frequency (Chenoweth et al., 2022). The extent to which the different landforms were sampled and the extent to which landform was incorporated into analyses also varied. Different years were sampled in the different studies, which makes it challenging to separate the possible effects of inter-annual variability associated with factors other than time since dam removal. In some instances, plots intended to monitor planting effects may not have been entirely located in a planted site. Planted sites were patchy, with planting staff instructed to plant clusters of vegetation in areas without obvious natural regeneration. As a result, a planted area may have only been partially planted with clusters surrounded by a matrix of naturally occurring vegetation. Randomized plots installed many years post-planting that are intended to sample a planted area could inadvertently sample a partially or completely unplanted site. This would result in species richness and diversity metrics with no relationship to planting effects. With more consistent sampling, results from the different studies would likely have been more consistent, however, our general conclusions would likely remain the same.
The placement of LWD appeared to increase richness and diversity, complementing the idea that structural diversity leads to biological complexity (Brown and Naeth, 2014). Using larger irregular surface topography such as cracks, rills, rocks, mounds, and depressions, studies have shown that these structures trap wind-blown seeds in early successional habitats, leading to increased seed deposition and ultimately increased germination of greater species (del Moral and Wood, 1993; Walker and del Moral, 2003; Jones and del Moral, 2005). However, contrary to predictions, plant abundance did not increase around LWD which is consistent with findings following the eruption of Mount St. Helens, where logs and boulders had negligible effects on plant establishment in seral post-volcanic habitats (Halpern and Harmon, 1983; Halpern et al., 1990). Since plant abundance did not differ significantly among wood and non-wood plots, it may indicate that seeds, albeit from fewer successful species, are dispersing to and germinating in open, coarse sediments. Studies in post-glacial habitats found that coarse surface sediments, similar to those that occur in the Mills reservoir, created favorable micro-sites for plant germination and establishment to occur (Jumpponen et al., 1999; Niederfriniger-Schlag and Erschbamer, 2000). Another factor was distance from the forest edge: As this increased, Shannon-Weiner diversity, species richness, and percent non-native species on unseeded plots declined. Seedlings of P. balsamifera and S. sitchensis were particularly abundant on terraces attributed to reservoir drawdown timing, which coincided with the height of both species’ seed production (Bountry et al., 2015; Prach et al., 2019; Chenoweth et al., 2022).
Ungulate and other mammal browsing pressures are often a concern to revegetation efforts. Light browsing (uninterrupted growth types – see Keigley, 1997) was common, mostly from Odocoileus hemionus columbianus (Columbia black-tailed deer), but was never significant (growth interrupting) in any of the 6 years of data collection. Chenoweth et al. (2011) hypothesized that woody seedlings planted or naturally occurring immediately during reservoir drawdown would have time to establish before browsing pressures were high. Ungulate foraging patterns typically take time to shift from historic movements to new, previously unavailable landscapes (Nolte, 2003). Nearby herds of Cervus canadensis roosevelti (Roosevelt elk) did not begin regular movements into the reservoirs until 5-6 years after reservoir dewatering. During the first 5 years of light browsing impacts, plantings and naturally occurring vegetation established successfully, reaching average canopy heights above browse levels prior to the regular presence of C. canadnsis roosevelti herds (J. Chenoweth, National Park Service, personal observation). Johnson et al. (2023) found that plants on the Elwha that were fully surrounded by large woody debris (LWD) clusters experienced significantly less browse intensity than plants growing in the open or with LWD on one or two sides.
Overall, findings from the studies we reviewed suggest that rapid regeneration of native vegetation is possible within five years after dam removal under careful drawdown and weed management regimes, even in the absence of seeding and planting. However, seeding and planting lead to important benefits, such as increased woody species diversity (from planting) and reduced non-native species richness (from seeding), especially in relatively harsh environments where plant growth is slow. Findings from these studies also suggest that with weed control, it is possible to prevent invasive species from dominating newly exposed reservoirs. Forest succession can take decades to centuries to proceed (Van Pelt et al., 2006), and over the long-term, planting and seeding and non-native species composition may have increasingly important effects, so it will be important to continue monitoring to gain a full understanding of the long-term patterns of revegetation after dam removal.
● Fine (silt and clay-sized particles) sediments do not inhibit revegetation and species not typically considered viable on silt and clay soils may establish. Planting trials using fine sediments in pots and planter boxes did not successfully predict species performance (Chenoweth et al., 2011; Michel et al., 2011), likely due to the effects of the containers on fine sediment performance (Poorter et al., 2012; Kawaletz et al., 2014). Plant trials in pots or raised beds create a unique environment that likely alters fine sediment characteristics and does not successfully mimic field conditions.
● Timing of reservoir drawdown can influence the trajectory of revegetation. If possible, reservoir drawdown timing could be scheduled for specific species desirable to revegetation goals. The natural recruitment of riparian species will enhance riparian habitats, a common goal for large dam removals. Timing the drawdown during the seed rain of Salicaceae species may result in a robust recruitment of Populus spp. and Salix spp. Alternatively, if the reservoirs are surrounded by undesirable non-native species of concern with wind-dispersed seed adaptations, reservoir drawdown timing can be scheduled when these species are not setting seed. A slow drawdown over many months can open the newly exposed lands during many different seed dispersal periods, enhancing the diversity of seed available to colonize the new landforms. Slow drawdowns will also provide more moisture to newly germinated seedlings to boost survival.
● Planting timing matters. Planting during reservoir drawdown may provide new plantings residual moisture beneficial to short-term and long-term establishment and performance. Early planting also provides an establishment window for trees and shrubs before ungulate browsing patterns divert into the new landscapes.
● Seeding is an effective tool for minimizing non-native species abundance; it is more important in coarsely textured, high-stress environments or sites far from natural seed sources.
● Plot locations, long-term monitoring methods, and analysis approaches are important considerations. It is crucial to establish plots immediately after planting to ensure the plots encompass planted and/or seeded material. Locating and establishing control plots immediately after seeding and planting is also important to ensure the site was, in fact, not seeded or planted. Ensure plots are well monumented for easy relocation and use consistent monitoring approaches to ensure sampling can be repeated long-term. Use similar analytical approaches to enable straightforward comparisons across different studies.
● Plot-based monitoring combined with qualitative monitoring are essential for adaptively managing revegetation of dewatered reservoirs after large dam removal. A multi-year planting plan, invasive species management plan and monitoring effort that spans at least 5-year post dam removal would help ensure that vegetation establishment and early seral conditions are on a desirable trajectory driven predominantly by native species interactions that are not impaired by dense populations of invasive, non-native species.
JC: Conceptualization, Project administration, Writing – original draft, Writing – review & editing. PS: Conceptualization, Writing – review & editing. RB: Visualization, Writing – original draft, Writing – review & editing. JH: Project administration, Writing – original draft, Writing – review & editing. JB: Writing – original draft. SC-Z: Data curation, Writing – original draft. CW: Writing – original draft. JS: Writing – review & editing. OM: Writing – review & editing.
The author(s) declare financial support was received for the research, authorship, and/or publication of this article. This work was supported by a Manuscript Preparation Grant from Western Washington University’s Office of Research and Sponsored Programs (Award #MS0148).
We would like to acknowledge the Lower Elwha Klallam Tribe and Olympic National Park for their restoration project leadership, support for this manuscript and research cooperation. We would also like to acknowledge Washington Conservation Corp and the dozens of technicians and volunteers that conducted all the planting, seeding, and monitoring for the Elwha Revegetation Project. Any use of trade, firm, or product names is for descriptive purposes only and does not imply endorsement by the U.S. Government.
The authors declare that the research was conducted in the absence of any commercial or financial relationships that could be construed as a potential conflict of interest.
All claims expressed in this article are solely those of the authors and do not necessarily represent those of their affiliated organizations, or those of the publisher, the editors and the reviewers. Any product that may be evaluated in this article, or claim that may be made by its manufacturer, is not guaranteed or endorsed by the publisher.
The Supplementary Material for this article can be found online at: https://www.frontiersin.org/articles/10.3389/fevo.2023.1268969/full#supplementary-material
Auble G. T., Shafroth P. B., Scott M. L., Roelle J. E. (2007). Early vegetation development on an exposed reservoir: implications for dam removal. Environ. Manage. 39, 806–818. doi: 10.1007/s00267-006-0018-z
Baker R. (2013). Elwha River Revegetation Project: 2012 Lake Aldwell Seeding Trials (Seattle (WA: University of Washington). master’s. thesis.
Bellmore J. R., Pess G. R., Duda J. J., O’Connor J. E., East A. E., Foley M. M., et al. (2019). Conceptualizing ecological responses to dam removal: if you remove it, what's to come? BioScience 69, 26–39. doi: 10.1093/biosci/biy152
Bishop J. (2002). Early primary succession on Mount St. Helens: impact of insect herbivores on colonizing lupines. Ecology 83, 191–202. doi: 10.1890/0012-9658(2002)083[0191:EPSOMS]2.0.CO;2
Bountry J., Crain P., Chenoweth J., Randle T., Ritchie A. (2015). Role of adaptive sediment management in Elwha dam removal. Proceedings of the 5th Federal Interagency Hydrologic Modeling Conference and the 10th Federal Interagency Sedimentation Conference, Reno, Nevada. Available at: https://acwi.gov/sos/pubs/3rdJFIC/Contents/10B-Bountry.pdf
Brown R. L., Chenoweth J. (2008). The effect of Glines Canyon Dam on hydrochorous seed dispersal in the Elwha River. Northwest Sci. 82 (sp1), 197–209. doi: 10.3955/0029-344X-82.S.I.197
Brown R. L., Naeth M. A. (2014). Woody debris amendment enhances reclamation after oil sands mining in Alberta, Canada. Restor. Ecol. 22, 40–48. doi: 10.1111/rec.12029
Burns R. M., Honkala B. H. (1990). “Silvics of north america: 1. Conifers; 2. Hardwoods,” in Agriculture Handbook 654, vol. 2. (Washington, DC: U.S. Department of Agriculture, Forest Service), 877. tech. coords.
Calimpong C. (2014). Elwha River Revegetation 2013: A plant Performance Study (Seattle, WA: University of Washington). master’s thesis.
Cavaliere E., Homann P. (2012). Elwha river sediments: phosphorus characterization and dynamics under diverse environmental conditions. Northwest Sci. 86, 95–107. doi: 10.3955/046.086.0202
Cendejas-Zarelli S. (2021). The effect of large woody debris, direct seeding, and distance from the forest edge on species composition on novel terraces following dam removal on the Elwha River, WA (Bellingham, WA: Western Washington University). Available at: https://cedar.wwu.edu/wwuet/1003. master’s thesis.
Chenoweth J. (2007). Predicting Seed Germination In The Sediments of Lake Mills After The Removal of The Glines Canyon Dam on the Elwha River (Seattle (WA: University of Washington). master’s thesis.
Chenoweth J., Acker S. A., McHenry M. L. (2011). Revegetation and Restoration Plan for Lake Mills and Lake Aldwell (Port Angeles, WA: Olympic National Park and the Lower Elwha Klallam Tribe).
Chenoweth J., Bakker J. D., Acker S. A. (2022). Planting, seeding, and sediment impact restoration success following dam removal. Restor. Ecol. 30, 1–13. doi: 10.1111/rec.13506
Colton M. (2018). Daytime summer microclimate influence of large woody debris on dewatered sediments in Lake Mills, WA. following dam removal (Bellingham (WA: Western Washington University). Available at: https://cedar.wwu.edu/wwuet/712/. master’s thesis.
Covell S., Ellis R., Roberts E., Summerfield R. (1986). The influence of temperature on seed germination rate in grain legumes in a comparison of chickpea, lentil, soyabean and cowpea at constant temperatures. J. Exp. Bot. 37, 705–715. doi: 10.1093/jxb/37.5.705
Darris D., Young-Mathews A. (2012). “Plant fact sheet for riverbank lupine (Lupinus rivularis),” in USDA-Natural Resources Conservation Service (Corvallis, OR: Corvallis Plant Materials Center).
del Moral R. (2007). Limits to convergence of vegetation during early primary succession. J. Vegetation Sci. 18, 479–488. doi: 10.1111/j.1654-1103.2007.tb02562.x
del Moral R., Wood D. M. (1993). Early primary succession on the volcano Mount St. Helens. J. Vegetation Sci. 4, 223–234. doi: 10.2307/3236108
Dengler J. (2009). Which function describes the species-area relationship best? A review and empirical evaluation. J. Biogeography 36, 728–744. doi: 10.1111/j.1365-2699.2008.02038.x
Foley M. M., Bellmore J. R., O’Connor J. E., Duda J. J., East A. E., Grant G. E., et al. (2017). Dam removal: listening in. Water Resour. Res. 5, 5229–5246. doi: 10.1002/2017WR020457
Fukami T., Bezemer T. M., Mortimer S. R., van der Putten W. H. (2005). Species divergence and trait convergence in experimental plant community assembly. Ecol. Lett. 8, 1283–1290. doi: 10.1111/j.1461-0248.2005.00829.x
Gray A. N., Spies T. A. (1997). Microsite controls on tree seedling establishment in conifer forest canopy gaps. Ecology 78, 2458–2473. doi: 10.1890/0012-9658(1997)078[2458:MCOTSE]2.0.CO;2
Grubb P. J. (1986). “The ecology of establishment,” in Ecology and Design in Landscape. Eds. Bradshaw A. D., Goode D. A., Thorp E. (Oxford, United Kingdom: Blackwell Scientific), 83–98.
Halpern C. B., Frenzen P. M., Means J. E., Franklin J. F. (1990). Plant succession in areas of scorched and blown-down forest after the 1980 eruption of Mount St. Helens, Washington. J. Vegetation Sci. 1, 181–194. doi: 10.2307/3235657
Halpern C. B., Harmon M. E. (1983). Early plant succession on the muddy river mudflow, Mount St. Helens, Washington. Am. Midland Nat. 110, 97–106. doi: 10.2307/2425215
Halvorson J. J., Smith J. L., Kennedy A. C. (2005). “Lupine effects on soil development and function during early primary succession,” in Mount St. Helens ecological research: ecological recovery of Mount St. Helens after the 1980 eruption. Eds. Dale V. H., Swanson F. J., Crisafulli C. M. (New York, N.Y.: Springer Science + Business Media, Inc), 243–254.
Harmon M. E., Franklin J. F., Swanson F. J., Sollins P., Gregory S. V., Lattin J. D., et al. (1986). Ecology of coarse woody debris in temperate ecosystems. Adv. Ecol. Res. 15, 133–302. doi: 10.1016/S0065-2504(08)60121-X
Heinemann K., Kitzberger T. (2006). Effects of position, understory vegetation and coarse woody debris on tree regeneration in two environmentally contrasting forests of north-western Patagonia: A manipulative approach. J. Biogeography 33, 1357–1367. doi: 10.1111/j.1365-2699.2006.01511.x
Johnson C., Douglas C., Mansmith T., McLaughlin J. (2023). Large wood supports Elwha revegetation by reducing ungulate browsing. Front. Ecol. Evol. 11, 1215144. doi: 10.3389/fevo.2023.1215144
Jones C. C., del Moral R. (2005). Effects of microsite conditions on seedling establishment on the foreland of Coleman Glacier, Washington. J. Vegetation Sci. 16, 293–300. doi: 10.1111/j.1654-1103.2005.tb02367.x
Jumpponen A., Varej H., Mattson K., Ohtonen R., Trappe J. M. (1999). Characterization of ‘safe sites’ for pioneers in primary succession on recently deglaciated terrain. J. Ecol. 87, 98–105. doi: 10.1046/j.1365-2745.1999.00328.x
Kalma J. D., Kuiper F. (1966). Transpiration and growth of Phaseolus vulgaris L. as affected by wind speed (Wegeningen, Nederland: Mededelingen Landbouwhoge school).
Kardouni J., Danilchik Lindsay M., Labay A., Bauman J. M. (2023). Riverbank lupine’s (Lupinus rivularis) influence on conifer growth, ectomycorrhizal colonization, and neighboring vegetation in coarse sediments left behind after dam removal. Front. Ecol. Evol 11. doi: 10.3389/fevo.2023.1214117
Kattge J., Diaz S., Lavorel S., Prentice I. C., Leadley P., Bönisch G., et al. (2011). TRY–a global database of plant traits. Global Change Biol. 17, 2905–2935. doi: 10.1111/j.1365-2486.2011.02451.x
Kawaletz H., Molder I., Annighofer P., Terwei A., Zerbe S., Ammer C. (2014). Pot experiments with woody species – a review. Forestry 87, 482–491. doi: 10.1093/forestry/cpu017
Kelsey K. A., West S. D. (1998). “Riparian wildlife,” in River Ecology and Management: Lessons from the Pacific Coastal Ecoregion. Eds. Naiman R. J., Bilby R. E. (New York: Springer-Verlag), 235–258.
Kleyer M., Bekker R. M., Knevel I. C., Bakker J. P., Thompson K., Sonnenschein M., et al. (2008). The LEDA Traitbase: a database of life-history traits of the Northwest European flora. J. Ecol. 96, 1266–1274. doi: 10.1111/j.1365-2745.2008.01430.x
Lázaro-Lobo A., Ervin G. N. (2021). Native and exotic plant species respond differently to ecosystem characteristics at both local and landscape scales. Biol. Invasions 23, 143–156. doi: 10.1007/s10530-020-02361-y
Lenhart C. (2000). The vegetation and hydrology of impoundments after dam removal in southern Wisconsin (Madison (WI: University of Wisconsin). master’s thesis.
MaChado S., Paulsen G. M. (2001). Combined effects of drought and high temperature on water relations of wheat and sorghum. Plant Soil 233, 179–187. doi: 10.1023/A:1010346601643
Mahoney J. M., Rood S. B. (1998). Streamflow requirements for cottonwood seedling recruitment – an integrative model. Wetlands 18, 634–645. doi: 10.1007/BF03161678
Mayaud J. R., Wiggs G. F., Bailey R. M. (2016). Characterizing turbulent wind flow around dryland vegetation. Earth Surface Processes Landforms 41, 1421–1436. doi: 10.1002/esp.3934
Michel J. T., Helfield J. M., Hooper D. U. (2011). Seed rain and revegetation of exposed substrates following dam removal on the Elwha River. Northwest Sci. 85, 15–29. doi: 10.3955/046.085.0102
Morgan O. A. (2018). Vegetation community development after dam removal on the Elwha River (Cheney (WA: Eastern Washington University). Available at: https://dc.ewu.edu/theses/523. master’s thesis.
Morris W. F., Wood D. M. (1989). The role of Lupinus lepidus in succession on Mount St. Helens: facilitation or inhibition? Ecology 70, 697–703.
Mussman E. K., Zabowski D., Acker S. A. (2008). Predicting secondary reservoir sediment erosion and stabilization following dam removal. Northwest Sci. 82 (sp1), 236–245. doi: 10.3955/0029-344X-82.S.I.236
Myrold D., Huss-Danell K. (2003). Alder and lupine enhance nitrogen cycling in a degraded forest soil in Northern Sweden. Plant Soil 254, 47–56. doi: 10.1023/A:1024951115548
Niederfriniger-Schlag R., Erschbamer B. (2000). Germination and establishment of seedlings on a glacier foreland in the central alps, Austria. Arctic Antarctic Alpine Res. 32, 270–277. doi: 10.1080/15230430.2000.12003364
O'Connor J. E., Duda J. J., Grant G. E. (2015). 1000 dams down and counting. Science 348, 496–497. doi: 10.1126/science.aaa9204
Orr C. H., Koenig S. (2006). Planting and vegetation recovery on exposed mud flats following two dam removals in Wisconsin. Ecol. Restor. 24, 79–86. doi: 10.3368/er.24.2.79
Orr C. H., Stanley E. H. (2006). Vegetation development and restoration potential of drained reservoirs following dam removal in Wisconsin. River Res. Appl. 22, 281–295. doi: 10.1002/rra.891
Poorter H., Hler J. H., van Dusschoten D., Climent J., Postma J. A. (2012). Pot size matters: a meta-analysis of the effects of rooting volume on plant growth. Funct. Plant Biol. 39, 839–850. doi: 10.1071/FP12049
Prach K., Chenoweth J., del Moral R. (2019). Spontaneous and assisted restoration of vegetation on the bottom of a former water reservoir, the Elwha River, Olympic National Park, WA, U.S.A. Restor. Ecol. 27, 592–599. doi: 10.1111/rec.12915
Prietzel J., Rehfuess K. E., Stetter U., Pretzsch H. (2008). Changes of soil chemistry, stand nutrition, and stand growth at two Scots pine (Pinus sylvestris L.) sites in Central Europe during 40 years after fertilization, liming, and lupine introduction. Eur. J. For. Res. 127, 43–61. doi: 10.1007/s10342-007-0181-7
Randle T. J., Bountry J. A., Ritchie A., Wille K. (2015). Large-scale dam removal on the Elwha River, Washington, USA: Erosion of reservoir sediment. Geomorphology 246, 709–728. doi: 10.1016/j.geomorph.2014.12.045
Ravot C., Laslier M., Hubert-Moy L., Dufour S., Le Coeur D., Bernez I. (2020). Large dam removal and early spontaneous riparian vegetation recruitment on alluvium in a former reservoir: lessons learned from the preremoval phase of the Sélune River project (France). River Res. Appl. 36, 894–906. doi: 10.1002/rra.3535
Riis T., Kelly-Quinn M., Aguiar F. C., Manolaki P., Bruno D., Bejarano M. D. (2020). Global overview of ecosystem services provided by riparian vegetation. BioScience 70, 501–514. doi: 10.1093/biosci/biaa041
Ritchie A. C., Warrick J. A., East A. E., Magirl C. S., Stevens A. W., Bountry J. A., et al. (2018). Morphodynamic evolution following sediment release from the world’s largest dam removal. Sci. Rep. 8, 13279. doi: 10.1038/s41598-018-30817-8
Schreiner E. G., Krueger K. A., Happe P. J., Houston D. B. (1996). Understory patch-dynamics and ungulate herbivory in old-growth forests of Olympic National Park, Washington. Can. J. For. Res. 26, 255–265. doi: 10.1139/x26-029
Schuster J. L. (2015). Vegetation colonization within exposed reservoirs following dam removal on the Elwha River, Washington (Cheney (WA: Eastern Washington University). Available at: https://dc.ewu.edu/theses/310. master’s thesis.
Shafroth P. B., Friedman J. M., Auble G. T., Scott M. L., Braatne J. H. (2002). Potential responses of riparian vegetation to dam removal. Bioscience 52, 703–712. doi: 10.1641/0006-3568(2002)052[0703:PRORVT]2.0.CO;2
Smethurst P. J., Turvey N. D., Attiwill P. M. (1986). Effect of Lupinus spp. on soil nutrient availability and the growth of Pinta radiata D. Don seedlings on a sandy podzol in Victoria, Australia. Plant Soil 95, 183–190. doi: 10.1007/BF02375070
Staniewski R. (1970). Typing of rhizobium by phages. Can. J. Microbiol. 16, 1003–1009. doi: 10.1139/m70-170
Tullos D. D., Collins M. J., Bellmore J. R., Bountry J. A., Connolly P. J., Shafroth P. B., et al. (2016). Synthesis of common management concerns associated with dam removal. J. Am. Water Resour. Assoc. 52, 1179–1206. doi: 10.1111/1752-1688.12450
Van Pelt R., O’Keefe T. C., Latterell J. J., Naiman R. J. (2006). Riparian forest stand development along the Queets River in Olympic National Park. Washington. Ecol. Monogr. 76, 277–298. doi: 10.1890/05-0753
Wahid A., Gelani S., Ashraf M., Foolad M. R. (2007). Heat tolerance in plants: an overview. Environ. Exp. Bot. 61, 199–223. doi: 10.1016/j.envexpbot.2007.05.011
Walker L. R., del Moral R. (2003). Primary succession and ecosystem rehabilitation (Cambridge, UK: Cambridge University Press).
Warrick J. A., Bountry J. A., East A. E., Magirl C. S., Randle T. J., Gelfenbaum G., et al. (2015). Large-scale dam removal on the Elwha River, Washington, USA: Source-to-sink sediment budget and synthesis. Geomorphology 246, 729–750. doi: 10.1016/j.geomorph.2015.01.010
Werner C. M. (2014). Early succession in plant communities: Regrowth and invasion in the drained Elwha Reservoirs (Princeton (NJ: Princeton University). undergraduate senior thesis.
Whisman M. (2013). Revegetation of Post-Dam Removal Riparian Sediments in the Lower Elwha River, WA (Olympia (WA: The Evergreen State College). master’s thesis.
Woodward A., Torgersen C., Chenoweth J., Beirne K., Acker S. A. (2011). Predicting spread of invasive exotic plants into dewatered reservoirs after dam removal on the Elwha River Olympic National Park, Washington (Reston, Virginia: U.S. Geological Survey). Available at: https://pubs.usgs.gov/of/2011/1048/pdf/ofr20111048.pdf. Open-File Report 2011-1048.
Keywords: succession, invasive species, sediment texture, ecological restoration, riparian
Citation: Chenoweth J, Shafroth PB, Brown RL, Helfield JM, Bauman JM, Cendejas-Zarelli S, Werner CM, Schuster JL and Morgan OA (2023) A review of natural and managed revegetation responses in two de-watered reservoirs after large dam removals on the Elwha River, Washington, USA. Front. Ecol. Evol. 11:1268969. doi: 10.3389/fevo.2023.1268969
Received: 28 July 2023; Accepted: 14 November 2023;
Published: 05 December 2023.
Edited by:
Rebecca McCaffery, United States Department of the Interior, United StatesReviewed by:
Diane Lynn Larson, United States Department of the Interior, United StatesCopyright © 2023 Chenoweth, Shafroth, Brown, Helfield, Bauman, Cendejas-Zarelli, Werner, Schuster and Morgan. This is an open-access article distributed under the terms of the Creative Commons Attribution License (CC BY). The use, distribution or reproduction in other forums is permitted, provided the original author(s) and the copyright owner(s) are credited and that the original publication in this journal is cited, in accordance with accepted academic practice. No use, distribution or reproduction is permitted which does not comply with these terms.
*Correspondence: Joshua Chenoweth, amNoZW5vd2V0aEB5dXJva3RyaWJlLm5zbi51cw==
†ORCID: Rebecca L. Brown, orcid.org/0000-0001-5726-809X
Chhaya M. Werner, orcid.org/0000-0002-2967-8603
Disclaimer: All claims expressed in this article are solely those of the authors and do not necessarily represent those of their affiliated organizations, or those of the publisher, the editors and the reviewers. Any product that may be evaluated in this article or claim that may be made by its manufacturer is not guaranteed or endorsed by the publisher.
Research integrity at Frontiers
Learn more about the work of our research integrity team to safeguard the quality of each article we publish.