- 1Institute for Biological Sciences, Applied Ecology and Phycology, University Rostock, Rostock, Germany
- 2Soil Sciences, Faculty of Agriculture and Environmental Sciences, University Rostock, Rostock, Germany
- 3Botanical Garden, University Rostock, Rostock, Germany
- 4Institute for Natural Resource Conservation, Landscape Ecology, Kiel University, Kiel, Germany
- 5M.G. Kholodny Institute of Botany, National Academy of Sciences of Ukraine, Kyiv, Ukraine
Harsh environmental conditions form habitats colonized by specialized primary microbial colonizers, e.g., biological soil crusts (biocrusts). These cryptogamic communities are well studied in drylands but much less in temperate coastal dunes, where they play a crucial role in ecological functions. Following two dune chronosequences, this study highlights the successional development of the biocrust’s community composition on the Baltic Sea coast. A vegetation survey, followed by morphological species determination, was conducted. Sediment/soil cores of the different dune types were analyzed to uncover the potential impacts of the biocrust community on initial soil formation processes, with special emphasis on biogeochemical phosphorous (P) transformations. Biocrust succession was characterized by a dune type-specific community composition, shifting from thinner algae-dominated biocrusts in dynamic dunes to more stable moss-dominated biocrusts in mature dunes. The change in the biocrust community structure was accompanied by an increase in Chl a, water, and organic matter content. In total, 25 algal and cyanobacterial species, 16 mosses, and 26 lichens across all sampling sites were determined. The pedological characterization of these cores elucidated initial processes of soil genesis, such as decalcification, acidification, and the accumulation of organic matter with dune and biocrust development. Furthermore, the chemistry of iron (Fe)-containing compounds such as the Fedithionite/Fetotal ratios confirmed mineral weathering and the beginning of soil profile development. The biocrusts accumulated P over time, while the P content in the underlying sediment did not change. That implies that biocrusts take up P from the geological parent material in the dunes, thereby accumulating available P in the ecosystem, which gets transferred into subsoil horizons through leaching or redeposition. The relative proportion of the bioavailable P pool (56% to 74% of Pt) increased with dune succession. That happened at the expense of more stable bound P, which was transformed into labile P. Thus, the level of plant available P along the dune chronosequences increased due to the microbial activity of the biocrust organisms. It can be concluded that biocrusts of temperate coastal dunes play a crucial role in maintaining their habitat by accumulating nutrients and organic matter, supporting soil development and subsequent vegetation.
1 Introduction
The Baltic Sea is geologically relatively young, and so are its coasts. The recent geomorphology of the coastline originates from repetitive glaciations during the Pleistocene period (Niedermeyer and Janke, 2011). The ice shields of the last glaciation, the Weichselian, began to melt about 12,000 years ago, which was the starting point for the altering freshwater and saltwater history of the Baltic Sea. With the advancing melting of the ice shields, the sea level rose, which marked the beginning of the sea-level transgression (Lampe, 2002). The freshwater periods were repeatedly interrupted by saltwater intrusions from the North Sea, which started about 8,000 years ago due to sea level fluctuations and the uplift of land masses. Hereby, the brackish water-dominated Baltic Sea was formed (Lemke, 1998).
Due to today’s tideless conditions, erosion and accumulation processes dominate the coastline of the Baltic Sea (Schumacher and Bayerl, 1999; Tiepolt and Schumacher, 1999). Here, the constant impact of wind and waves on exposed coastal areas causes erosion. The eroded material gets transported by coastal-parallel currents and deposited in protected shallow bays. The result is a smoothened and relatively closed coastline, especially along the southern Baltic Sea coast (“graded or equilibrium coast”) (Müller et al., 2023), with characteristic geomorphological forms such as sand hooks and spits (Lampe et al., 2011). These coastal forms can be found and studied on the peninsula Fischland-Darss-Zingst.
This peninsula consists of a sequence of Late Weichselian period moraine island cores that are connected by sandy barriers (sand spits). The latter are under ongoing shaping forces causing coastal degradation, sand drift, and accumulation. The Darss is the northwestern part of the peninsula. Mainly due to the shifting of sediment by incoming and outgoing waves in shallow waters, the shoreline expanded in the northern direction of the initial island core. Thereby, an almost triangular foreland (“Neudarss”), showing a sequence of about 120 beach ridges, accumulated over time and today ends in a northeast turning sand hook (Darsser Ort). If no further sand is transported along the coastline to enlarge the hook, this coastline will be ablated again. The western coastline of the Darsser Ort already retreats (approximately 1 m/year) to the east by sediment erosion and is therefore called an abrasion coast. Cliff dunes are created in the process. On the other hand, new beach ridges form in the north and allow the hook at Darsser Ort to expand (Tiepolt and Schumacher, 1999; Lampe and Lorenz, 2010) (Supplementary Figure 1).
Eastwards of the peninsula, the coastline continues with an extensive sand accumulation in shallow water due to the transport of sediment parallel to the coast (Niedermeyer et al., 2011). This area is a wind flat of about 25 km². Depending on the wind strength and direction, shallow areas fall dry or are flooded (Karsten et al., 2012). Wind flats typically accumulate sediment particles and hence stabilize coastlines. As at the Darsser Ort, a coastline-parallel orientation of beach ridges can be observed here (Janke and Lampe, 1998; Reimann et al., 2010). Coastal sand dunes have formed on these ridges, resulting in a chronological series of this landform. The source material for these dunes originates from the Pleistocene island cores, which eroded during the sea level rise in the Holocene. After the sea level dropped about 6,000 years ago, this material became accessible again and contributed to the formation of spits, hooks, wind flat, and sand dunes (Schumacher, 2002). Like the coastline, dunes are mobile aeolian landforms that constantly change. The dune geomorphology is based on initial geneses as well as on the following abrasion and accumulation processes due to wind, waves, and storm events. Further inland, in areas where these extreme environmental conditions are declining, a change from mobile to more stable dune types can be observed (Martínez et al., 2004). The resulting sequence of dune types represents a chronological series and allows studies on their formation and development, soil genesis, and vegetation colonization over time (Ranwell, 1960; Isermann, 2011; Pollmann et al., 2020). The abiotic factors during the dune genesis hardly differ from each other. The only difference between the dune types is the time of their formation (Stevens and Walker, 1970; Walker et al., 2010). At the Baltic Sea coast, the successional dune development on a flat coast differs between four to five characteristically dune types, which can be divided into initial, growth, maturity, and decomposition stages (Niedermeyer et al., 2011). The foredune ridge adjoins the beach inland. It is the starting point of an idealistic dune chronosequence and is followed by mobile yellow dunes. Flooding and storms are causing frequent sand movement. In the subsequent grey dunes, phototrophic organisms are already progressively colonizing, thereby stabilizing the dune surface and promoting the formation of a humus layer on the loose sediment. The chronosequence ends in a mature dune area, which is likely to be forested with scrubs or smaller pine trees (Hesp, 1991; Leuschner and Ellenberg, 2017; Jurasinski and Buczko, 2023). The general trends along such a chronosequence include organic matter accumulation and increasing acidification, as well as decalcification, a decrease in salt stress, and sand transport and deposition (Salisbury, 1952; Hundt, 1985; Giani and Buhmann, 2004; Pollmann et al., 2020). In addition to the constant movement of the sand, extreme temperature fluctuations near the dune surface and drought make this area an extreme habitat (Martínez et al., 2004). On the one hand, living communities settling there have to cope with a low nutrient supply, flooding events, and salt stress (García Novo et al., 2004; Maun, 2009). On the other hand, biotic colonization can significantly influence these geomorphological processes during dune succession, promoting initial soil formation (Williams et al., 2012; Zaady et al., 2014). The colonization of a heterogeneous dune habitat strongly depends on the individual dune types. Driven by extrinsic (e.g., microclimate) and intrinsic (e.g., species competition) mechanisms, dune habitats develop their particular assemblage of microbial and vascular plant communities (Miller et al., 2010).
Cryptogamic vegetation (e.g., mosses, lichens, and fungi) are primary ground covers and characteristic growth forms on top of terrestrial bare soils (biological soil crusts = biocrusts) or rock surfaces (Elbert et al., 2012). They are characterized by an interaction of phototrophic and heterotrophic microorganisms, leading to a complex biotic network. Due to this organismic diversity, biocrusts are involved in ecosystem processes and linked to a wide range of ecological functions (Belnap et al., 2001). The initial habitat colonization of biocrusts is accompanied by an increase in organic matter content (Veste et al., 2011; Dümig et al., 2014) and in soil stability (Fischer et al., 2010; Felde et al., 2018). The latter can be seen as an essential function that biocrusts can provide to highly dynamic ecosystems like coastal sand dunes, which are constantly threatened by soil erosion and blowouts (Belnap and Büdel, 2016). Moreover, it has been shown that biocrusts are likely to influence local hydrological cycles, including absorptivity and water retention (Fischer et al., 2012; Chamizo et al., 2016). In addition, the organisms of the biocrusts are involved in various essential element nutrient cycles, such as carbon (Sancho et al., 2016) and nitrogen (Castillo-Monroy et al., 2010; Elbert et al., 2012; Barger et al., 2016), while the metabolic processes within the phosphorus cycle (P cycle) are far less studied but have progressively received attention in recent years (Richardson and Simpson, 2011; Schulz et al., 2015; Baumann et al., 2019; Kurth et al., 2021). Such complex functions depend on the respective biocrust type and, therefore, its structure, which varies with climate, underlying soil, and disturbance (Belnap, 2006; Sun et al., 2022).
The dominant biocrust-forming community, typical for a specific successional stage and biocrust type, plays a decisive role in the development and performance of its ecological functions (Li and Hu, 2021). Various environmental factors, characteristic of a geographical region, affect the natural successional process of the biocrust community. Prominent among these factors are the availability of water (Fischer et al., 2012), the temperature (Garcia-Pichel et al., 2013), radiation, topography, soil texture (Zaady et al., 2000; Fischer and Subbotina, 2014), microclimate (Veste and Littmann, 2006), and nutrient availability (Bu et al., 2018). The availability of these resources and disturbances impacts the succession (Pickett and McDonnell, 1989) of biocrusts and determines the rates of biocrust formation, which can range from 10 to 1,000 years (Langhans et al., 2010; Williams et al., 2012). The interactions of biocrust types with individual community compositions and morphologies influence the nutrient influx, soil chemistry, and surface microtopography. Therefore, biocrusts can be considered relevant promotors in pedogenesis and dune development (Williams et al., 2012).
Various studies focused on biocrust community development, functions, and changes in arid areas (Büdel et al., 2009; Samolov et al., 2020; Li and Hu, 2021), but less is known about biocrusts in coastal sand dunes of temperate regions (Kammann et al., 2023). Few studies on sand dunes along the Baltic Sea coast underlined the ecological importance of biocrusts in influencing soil properties, causing an increase in total phosphorus concentrations, and emphasized their high site-specific microorganism diversity (Schulz et al., 2015; Mikhailyuk et al., 2019; Kammann et al., 2023). Schaub et al. (2019) identified sand blasting and burial of the biocrust microorganisms as crucial factors in biocrust development on temperate sand dunes. Furthermore, the study showed gains in ecological soil functioning of the sand dunes by initial soil organic matter formation and an accumulation of nutrients, especially on the more protected landward dune slope. The results of these studies led to the first conclusions about the relevant ecosystem functions of biocrusts in dunes for nutrient cycling and further vegetation colonization. Information on community changes across biocrust successional stages along the natural dune development, however, is missing.
In this study, it is assumed that the natural coastal dynamics lead to a successive development of different types of biocrusts on the dunes. Therefore, the intention was to (1) define dominant biocrust types in the different dune successional stages and (2) survey the changes in the biodiversity of phototrophic organisms. In addition to vegetation surveys, (3) pedological investigations focus on initial soil formation processes and the chronosequential changes in dune soils. The P cycle is of particular importance in this context. Hence, a further objective was to (4) determine the P content and sequentially extracted P pools that reflect the availability of P to microorganisms and plants. Easily bioavailable P is expected to (5) accumulate with ongoing dune succession due to biocrusts’ impact on the biogeochemical P cycle. Thereby, the biocrust community is assumed to enhance labile P contents due to the conversion of more stable P into labile P.
2 Material and methods
2.1 Study site
Biocrust samples were collected in January 2021, along two dune successional sequences on the peninsula Fischland-Darss-Zingst (Mecklenburg Western Pomerania, Northern Germany; Figure 1A). Both sampling sites are located in the core zone of the national park Vorpommersche Boddenlandschaft, on a flat coast of the Baltic Sea, and differ in their morphology. While the sampling transect at Darsser Ort (Figure 1B) is located on a dune cliff (54.472167, 12.499556) endangered by abrasion, the flat coast at Pramort (Figure 1B) forms a sandspit (54.44475, 12.923). Both dune areas are under the strict protection of the national park. Trampling in the dunes is prohibited, but especially at the Darsser Ort, it cannot be excluded.
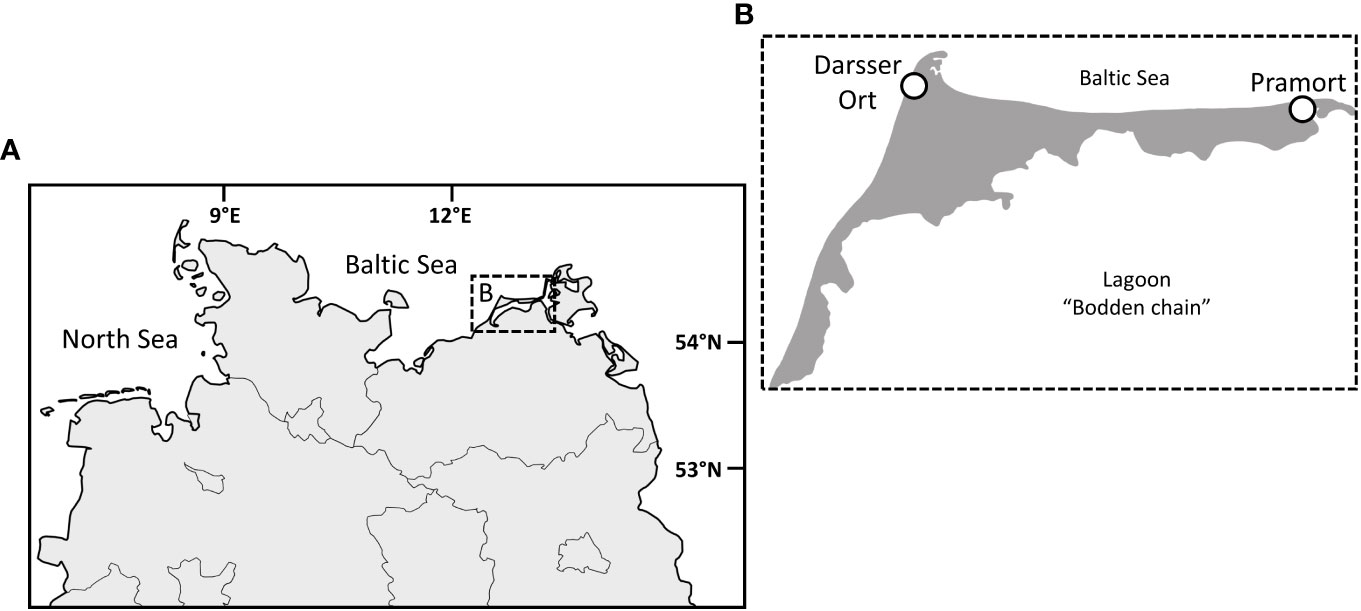
Figure 1 The peninsula Fischland-Darss-Zingst in the northern part of Germany (A). A close-up of the peninsula (B) and the investigated dune sampling sites, Darsser Ort and Pramort (circle). Copyright: GeoBasis-DE/M-V.
2.2 Sampling
2.2.1 Transects
Biocrust samples were taken along one transect at both study sites. Each transect followed the natural geomorphological succession (chronosequence) of the respective dune area. At Darsser Ort (Figure 2) it started at the edge of the dune cliff. The dune cliff represented a former grey dune identified visually in the field by visible slight accumulations of iron oxides and humus in the underlying soil horizon [Figure 2A (right)]. Due to erosion processes, this primary dune got eroded, which led to the formation of the dune cliff. As part of a secondary dune formation process, the dune cliff was continuously covered with a large amount of sand due to aeolian transport during storm events. Hence, the dune area today is characterized by a loose sand layer down to a depth of approximately 30 cm without any further visible zonation of the substrate. Due to this trait and the later described vegetational patters, this area of dunes is referred to below as the (secondary-formed) yellow dune (YD). The grey dune area following the geomorphological gradient was divided into two grey dune types. The grey dune area directly following the yellow dune was named the early grey dune area (eGD) since the dune surface was dominated by early microalgae biocrusts, some mosses, and bare sediment. Due to these characteristics, the dune type can be assigned partly to a yellow and partly to a grey dune and therefore reflects a transition zone. In addition, a decline in the marram grass stands (Ammophila arenaria (L.) Link) characteristic of the yellow dune was observed here. The part of the grey dune area further inland was defined as a late grey dune area (lGD). A significantly dominant moss cover and the frequent occurrence of lichens led to the differentiation of both grey dune subtypes within the large grey dune area. The transect followed the dune chronosequence further inland and ended in the mature dune area (MD), which was already overgrown with pine trees.
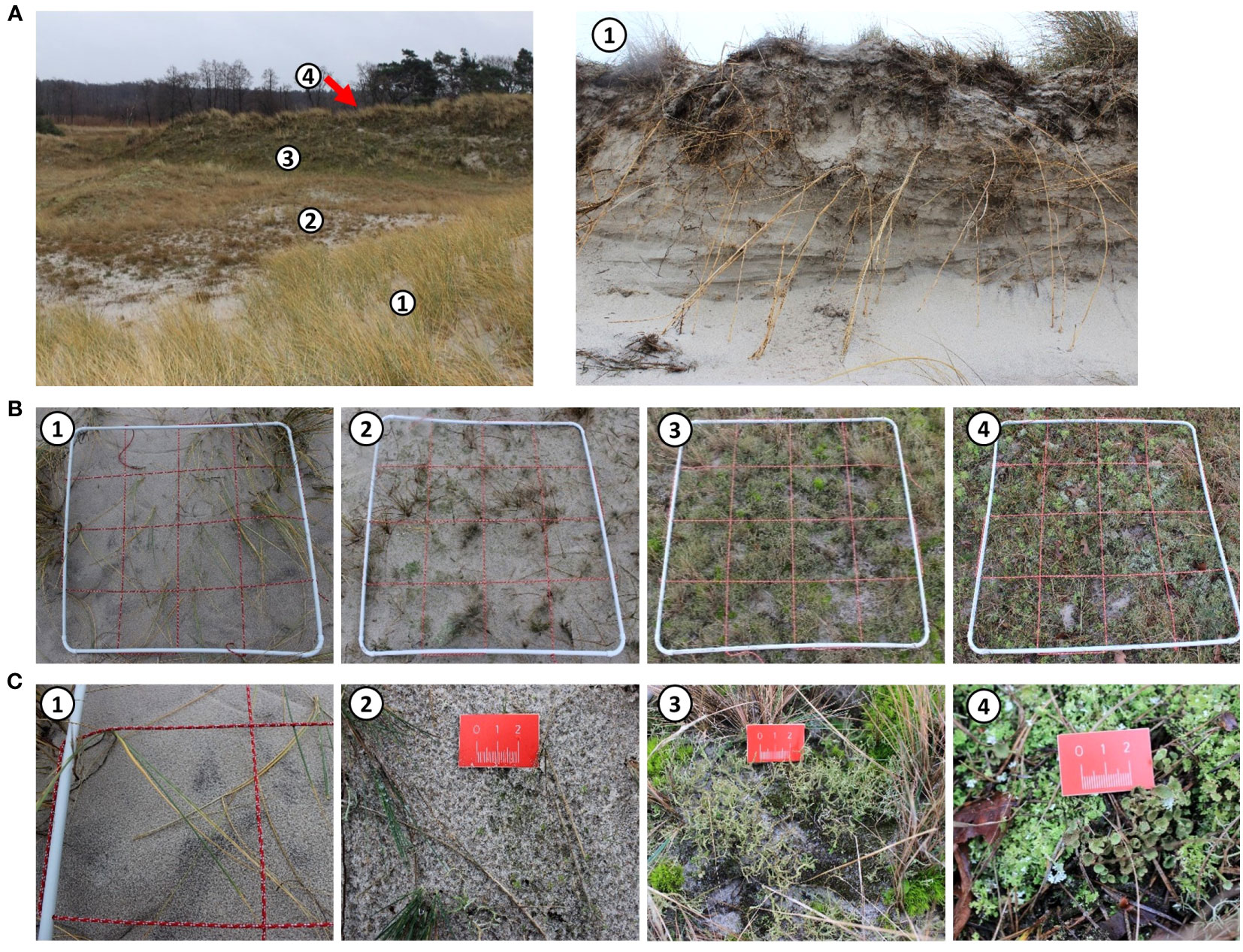
Figure 2 Overview of the transect at Darsser Ort; numbers indicate the sampling plots: 1, yellow dune (YD; secondary formed); 2, early grey dune (eGD); 3, late grey dune (lGD); and 4, mature dune (MD). Transect overview (left) and view at the dune cliff from the beach site (right) (A) close-ups of the sampling plots (B) and detail shots of the top sediment layer and dominating biocrust types in the respective plot (C).
The transect at Pramort started at the eastern accumulation coast of the peninsula, in a periodically submerged wind flat called windwatt (WW). Here, an initial formation of a spit could be seen on the basis of pioneer vegetation (Ammophila arenaria (L.) Link) and sand accumulation. After that, the transect crossed a yellow dune area (YD), where microbial and vegetational cover changed. Further inland, a grey dune (GD) area superseded the mobile and higher yellow dunes, finally reaching the mature dune area (MD) (Figure 3), similar to the transect progression at the Darsser Ort.
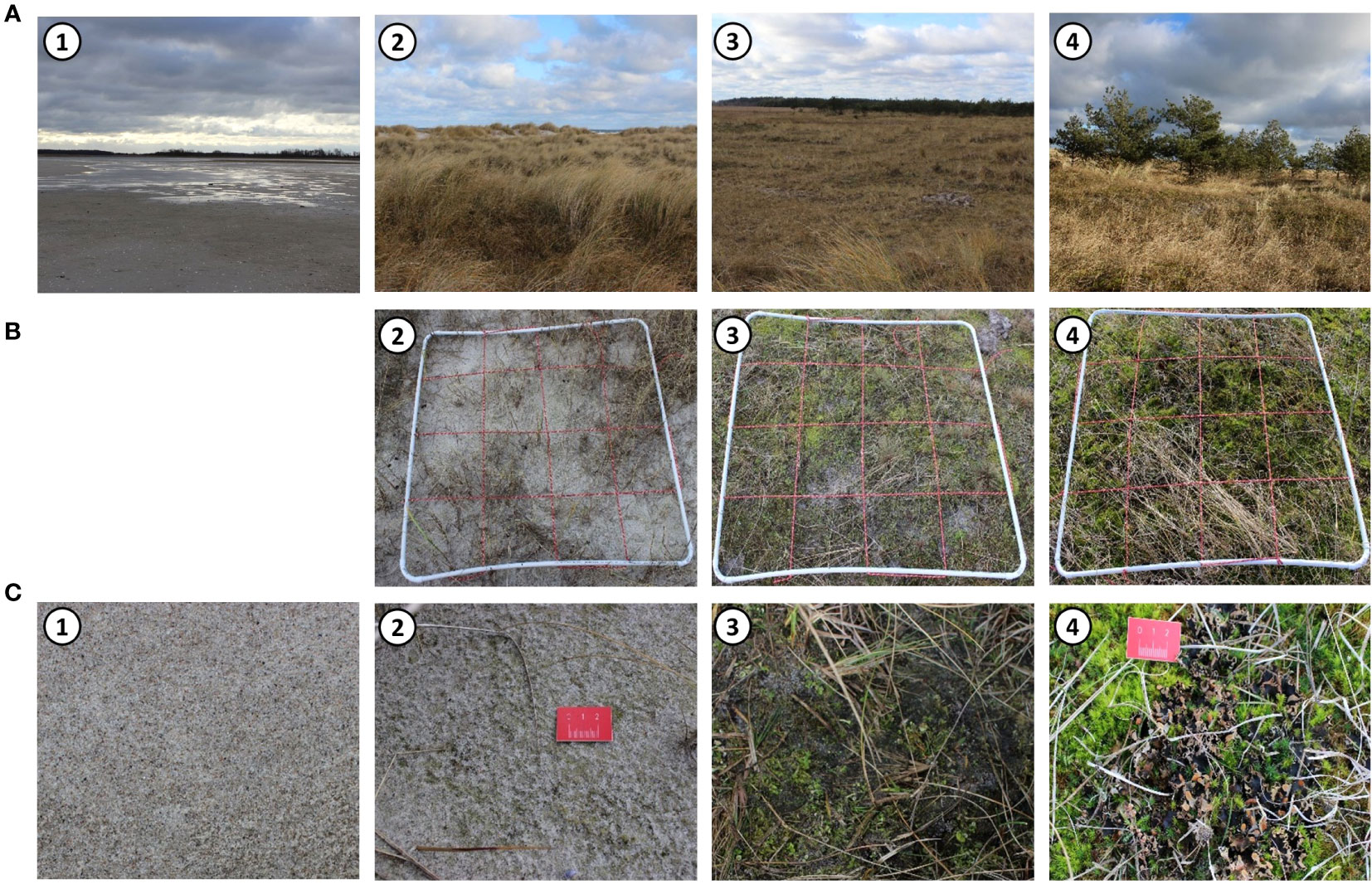
Figure 3 Overview of the transect at Pramort; numbers indicate the sampling plots: 1, windwatt (WW); 2, yellow dune (YD); 3, grey dune (GD); and 4, mature dune (MD) (A). Close ups of the biocrust-holding sampling plots (B) and detail shots of the top sediment layer and dominating biocrust type in the respective plot (C).
Along each transect, the described different successional dune stages were selected and further named dune subsites. At each subsite, a sampling plot of 1 m2 [maxi grid, cf. Figure 3B (2–4)] was established and used for further vegetation analyses, biocrust, and sediment sampling. In total, eight sampling plots at two study sites were under investigation in this study, four per site.
2.2.2 Sample collection and preparation
For sampling, Petri dishes (92 mm in diameter) were pushed gently into the respective biocrust. A metal spatula was then used to lift and further separate the biocrust from the underlying loose sediment. A maxi-grid (1 m × 1 m) divided each sampling plot into 16 equal subplots (25 cm × 25 cm) [cf. Figure 3B (2–4)]. One Petri dish was collected within each subplot.
The yellow dune at Darsser Ort and in the windwatt at Pramort had no biocrusts. To keep the sampling design uniform at each plot, samples were also taken from these two plots using Petri dishes, even when containing no visible biocrusts. At each sampling plot, a set of three samples (equals three Petri dishes) was collected for chlorophyll a analyses and for algae community cultivation, direct microscopy, and identification (Table 1). These samples were directly wrapped in aluminum foil and stored in a cooling box for transportation. Three further samples (second set) were taken in the same way, which were later used for nutrient element analyses (C, N, P) of biocrusts (Table 1). For the measurement of biocrust characteristics (water and organic matter content), one additional Petri dish was collected per plot (Table 1). All mosses and lichens detected in the sampling plots were collected by hand and stored in paper bags.
Furthermore, a hand auger (30 cm length, 5 cm diameter) was used for collecting the sediment/soil samples (Figure 4A). The auger was used three times, randomly within the sampling plot. The samples were already visually divided in the field for topsoil horizon and subsoil horizon/sediment layer (cf. Figure 4B, yellow box = topsoil horizon). The sediment/soil core samples were stored in plastic ziplock bags and transported to the laboratory for further chemical analyses. Since before detailed chemical analyses, it cannot be decided to what extent the sediment has already been transformed into soil, the exact naming of the upper and lower parts of the samples in the auger remains open (soil horizon or sediment layer) until the presentation of the results.
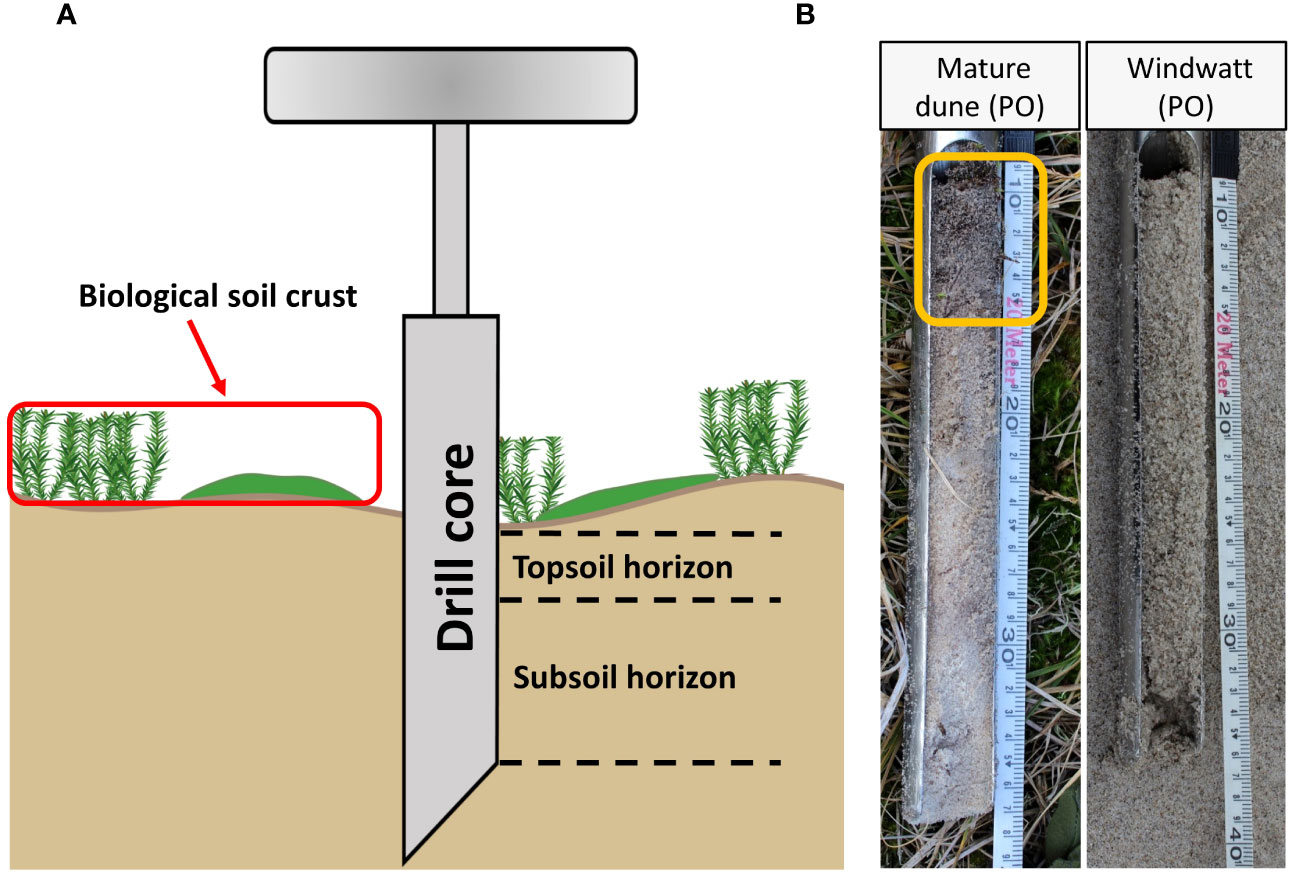
Figure 4 Schematic figure of the hand auger application in relation to biocrusts (A). Exemplary representation of two sediment/soil cores sampled at Pramort. Yellow box represents a subdivided topsoil horizon, based on visual inspection and confirmed by chemical analyses (B).
2.3 Analyses of biocrusts and sediment/soil characteristics
2.3.1 Biocrusts
In the laboratory, two subsamples were taken using a cork drill (Ø 1.5 cm) from each of the three Petri dishes (first set) planed for the chlorophyll a analysis. This tool allowed to punch out a defined biocrust surface area. This resulted in six chlorophyll a measurements per sampling plot, i.e., in total, 48 analysis (8 sampling plots × 3 petri dishes (replicates) × 2 subsamples per dish) were done along both transects. These individual samples were each stored in a 15 mL Falcon® tube and frozen at −20°C until chlorophyll a extraction. The remaining biocrust material in the Petri dishes was air-dried and further stored in the dark until the subsequent algae isolation and identification.
Chlorophyll a (Chl a) content was used as a proxy for the photosynthetic biomass (Chl a mg m−2) in biocrusts. Even though no biocrusts were visually recognizable in the windwatt (Pramort) and the yellow dune (Darsser Ort), the Chl a analysis was performed identically to the protocol for biocrusts. The Chl a extraction was conducted after a standard laboratory protocol, and the Chl a absorbance at 632 nm, 649 nm, 665 nm, and 696 nm wavelengths was measured using a spectrophotometer (Shimadzu UV-2401 PC, Kyoto, Japan). The Chl a content was calculated according to Ritchie (2008) and normalized to a square meter (m2). A detailed description can be found in Kammann et al. (2023). The biocrusts in the additional three Petri dishes (second set) were separated from the sediment with a razor blade to measure their nutrient element contents. The biocrust samples were sieved (< 2 mm) and subsequently milled to 10 to 20 µm particle size (mortar grinder “Pulverisette 2”, Idar-Oberstein Germany) for further homogenization. Total carbon and nitrogen content (Ct and Nt) were determined by dry combustion of about 25 to 30 mg of sample in an elemental analyzer (vario EL cube, Elementar Analysensysteme GmbH, Langenselbold, Germany). Total phosphorus (Pt) was extracted from 500 mg of air-dried material by microwave-assisted digestion using aqua regia solution (3:1 hydrochloric acid:nitric acid). The concentration in the extract was measured by inductively coupled plasma optical emission spectroscopy at a 214.914 nm wavelength (Perkin-Elmer Optima 8300 DV, Waltham, MA, USA). At each plot, each parameter was measured in triplicate.
For analyzing the biocrust characteristics (water and organic matter content), the entire biocrust of the remaining single Petri dish sample was used. After fresh weight (FW) determination, the samples were dried at 105°C for 24 h and weighed again to determine the dry weight (DW). The water content was calculated from these two measurements and expressed as a percentage of total fresh weight (% FW). The sample drying was followed by their combustion at 450°C for 5 h to determine the organic matter (OM) content. Therefore, the sample weight loss after combustion was determined, and the organic matter content was expressed as a percentage of total dry mass (% DW).
2.3.2 Sediment/soil cores
For further analytical determination of basic sediment/soil parameters such as pH, electrical conductivity, and calcium carbonate content (CaCO3), the core material was used. This material was air-dried in the laboratory and sieved to < 2 mm. These basic parameters were determined by routine methods described in Blume et al. (2010): pH electrometrically in 1:2.5 (w/v) suspension with 0.01 M CaCl2; electrical conductivity in saturation extract with de-ionized water by temperature-compensated conductivity measurement device (WTW Microprocessor Conductivity Meter LF196) and CaCO3 content by dissolution with 10% HCl and volumetric CO2 measurement in a Scheibler calcimeter. Al, Fe, Mn, and P from poorly crystalline pedogenic oxides were extracted from 0.5 g of soil (< 2 mm) using a 0.2 M NH4 oxalate solution (pH 3) in the dark (Schwertmann, 1964). The total amount of Fe, Al, Mn from pedogenic oxides (poorly and well crystallized oxides) was extracted by the dithionite–citrate–bicarbonate (DCB) method after Mehra and Jackson (1958) with slight modifications. In brief, 1 g of soil (< 2 mm) was incinerated at 550°C before being extracted twice with 25 mL citrate–bicarbonate solution and 1 g of Na dithionite at 80°C and washed with a 10 mL 0.1 M MgSO4 solution thereafter. Concentrations of elements in the oxalate extract (Feox, Alox, Mnox, Pox) and DCB extract (Fedit, Aldit, Mndit) were measured by ICP OES. The amount of pedogenic oxides with a higher degree of crystallinity was estimated by subtracting the amount of poorly crystalline oxides from the amount of total pedogenic oxides (Aldit-ox, Fedit-ox, Mndit-ox).
The determination of the Ct, Nt, and Pt concentrations followed the same protocol as for the biocrusts. In addition to the total carbon content, the organic carbon content of the sediment/soil core samples was also determined.
2.4 Sequential P fractionation
The method of sequential P fractionation was used to characterize P pools of different solubility in the sediment. Here, the inorganic and organic P fractions in the sediment were characterized according to extractability with increasingly stronger chemicals, which traditionally has been interpreted as an indication of bioavailability for microorganisms and plants. The method was adjusted by Hedley et al. (1982) and applied to the core samples. If these had already been separated into upper and lower layers or horizons in the field, only the upper sediment/soil core samples were examined for P fractions. Exemplarily, biocrusts from two sampling plots along each transect were additionally analyzed. This included 30 mL purified water in the presence of an anion exchange resin (membrane, 2 cm × 6 cm), NaHCO3, NaOH, and H2SO4, as described in detail in the Supplementary Material (Supplementary Figure 2; Table 1). The concentration of Pt in the extracts was determined by ICP OES. Concentrations of molybdate-reactive P, which is mostly considered inorganic P (Pi), in each extract was determined using the molybdenum blue method of Murphy and Riley (1962). The concentration of molybdate-unreactive P, here considered organically bound P (Po, e.g., ADP/ATP, phospholipids), was calculated as the difference between Pt and Pi of each fraction. Resin-P and NaHCO3-P are ascribed to labile P, whereas NaOH-P is considered moderately labile P, and H2SO4-P are relatively stable P compounds.
2.5 Vegetation survey
A category system of seven pre-defined functional vegetation groups was designed to describe the overall surface coverage of each sampling plot (Supplementary Table 2). The set of functional groups was based on previous work conducted by Büdel et al. (2009); Lan et al. (2012), and Williams et al. (2017), but was slightly modified. The early successional stages of biocrust development were characterized by thin (1–3 mm) microalgae-dominated biocrusts (MA). Slightly thicker (3–8 mm) microalgae biocrusts, which were additionally partly moss-covered, were defined as the intermediate successional biocrust stage (MA/M). The mature successional stages of biocrusts consisted of moss-dominated biocrusts (MD) and those associated with lichens (MD/L). Moreover, two additional biotic functional groups were established: vascular plants and litter (dead organic matter, e.g., pine needles, leaves, and branches). Bare sediment was the only abiotic functional group.
The point-intercept method of Levy and Madden (1933) was used to record the predefined functional groups. A maxi-grid (1 m × 1 m) divided each sampling plot into 16 equal subplots (25 cm × 25 cm). Furthermore, a smaller metal grid (25 cm × 25 cm) was randomly placed within four of these 16 subplots (Supplementary Figure 3). Within these four subplots, the functional groups were recorded by 25 point-intercept measurements according to the approach of Williams et al. (2017). At each intersection where two lines crossed, a metal pin was dropped, and the ground covering functional group, including bare sediment, was recorded. This made a total of 100 point measurements per sampling plot (1 m2).
2.6 Algae isolation, community cultivation, and identification
The air-dried biocrust material, stored in the dark, was further used for the identification of the most frequent and dominant species. Pieces of biocrusts were placed in Petri dishes with Bold basal (1 N BBM) agarized medium to obtain enrichment cultures (Bischoff and Bold, 1963). Cultures grew with a 12-h alteration of light and dark phases and irradiation of 25 μmol photons m−2 s−1 at a temperature of 20°C ± 5°С. After 3 weeks of cultivation, morphological investigations were performed using an Olympus BX53 light microscope with Nomarski DIC optics (Olympus Ltd, Hamburg, Germany) and a digital camera (Olympus LC30) attached to the microscope. The micrographs herewith recorded were processed by the Olympus software cellSens Entry. Parallel to cultivation, direct microscopy of rewetted samples was performed. Here, the dominant species of algae and cyanobacteria in the original samples were evaluated. Morphological identification of the biocrust organisms was based mainly on Ettl and Gärtner (2014) for green microalgae and on Komárek and Anagnostidis (2005) as well as Komárek (2013) for cyanobacteria. Furthermore, monographs and papers devoted to taxonomic revisions of the taxa of interest were used, such as Lokhorst (1996); Fučíková et al. (2014); Mikhailyuk et al. (2019), and others. Algae and cyanobacteria were presented according to the modern taxonomic interpretations (Guiry and Guiry, 2023). It was not feasible to assess the abundance of each identified taxa within each sample due to the method of enrichment cultivation. To still be able to make a statement about the diversity at each sampling point, the total number of identified algal species per sampling plot, referred to as species richness, was used.
2.7 Moss and lichen determination
Moss and lichen samples were air-dried in the laboratory. A microscope with a maximum magnification of ×400 was used for the determination. Morphological identification of mosses was based on Frahm and Frey (2004), with taxonomical reference to Hodgetts et al. (2020). Lichens were determined according to Wirth et al. (2013) and followed the nomenclature concept provided by Printzen et al. (2022). Some species of the genus Cladonia needed a deeper morphological treatment. Therefore, these samples were additionally chemo-taxonomically analyzed by thin-layer chromatography according to Culberson and Ammann (1979) in solvent system A.
2.8 Statistical analyses
The percentage of areal coverage by each functional group recorded in the vegetation survey obtained via the point-intercept method was visualized as a stacked bar graph. Significant differences in the measured biocrust and sediment/soil characteristics, including nutrient contents, were elucidated by performing analyses of similarities (“anosim” function in R) between the sampling sites and across the transect plots in both dune areas. To reflect differences in the overall biocrust community between the different sites and dune successional stages, nonmetric multidimensional scaling (nMDS) using the vegan R package, and the Bray–Curtis dissimilarity index implemented in R was applied. Furthermore, permutational multivariate analyses of variance (PerMANOVA) (“adonis” function in R) using the Bray–Curtis distance matrix were conducted to reveal potential correlations of community composition with biocrust and sediment plot characteristics and nutrient contents (Oksanen et al., 2018). If a significant correlation between the phototrophic community and the sediment/soil characteristics was detected, these parameters were added to the nMDS plot. All statistical analyses were conducted using R version 4.2.1 (R Core Team (2022) Vienna, Austria). The total species number of each phototroph group (algae, moss, and lichen) per sample was considered as alpha diversity. As a measure of beta diversity, the presence/absence data of the biocrust-inhabiting species were visualized within Venn diagrams using Microsoft Excel 2013 (Microsoft Corporation, Redmond, WA 98052-6399, USA).
3 Results
3.1 Analyses of biocrusts and sediment/soil characteristics
3.1.1 Biocrusts
Along both transects, the phototrophic biomass increased from algal biofilms towards more stable and moss-dominated biocrusts (Table 2). At Darsser Ort, the Chl a concentration was lowest in the yellow dune (YD = 1.2 mg m−2 ± 0.9 mg m−2) and reached a value of 100.3 mg m−2 ± 18.9 mg m−2 in the early grey dune area where the microalgae-dominated crusts and some mosses were present. In the following late grey dune area, the percentage coverage of biocrusts further increased, and so did the Chl a content in the biocrusts (327.1 mg m−2 ± 88.4 mg m−2). In the mature dunes, the Chl a content reached its highest value (453.8 mg m−2 ± 188.0 mg m−2). A similar pattern was observed along the transect at Pramort. The windwatt at the beginning of the transect had no Chl a in the bare sediment. In the following yellow dune, Chl a was detected as the first sign of early biocrust development (127.6 mg m−2 ± 4.2 mg m−2). The moss-dominated biocrusts in the grey and mature dunes had the highest Chl a values (GD = 453.2 mg m−2 ± 35.4 mg m−2, MD = 482.8 mg m−2 ± 125.9 mg m−2).
The gravimetric water content of the biocrusts at Darsser Ort ranged between 7.3% (w/w) in the early grey dune area and 25.3% (w/w) in the biocrusts growing in the mature dune area. At Pramort, the sediment water content along the successional dune gradient was slightly higher than at Darsser Ort. The water content measured in the windwatt was relatively high, at 7.9% (w/w), even though it represented an unvegetated area due to its periodic submersion. While the biocrusts in the yellow dune area exhibited 5.2% (w/w) of sediment water content, the grey and mature dune areas had 21.9% and 40.9% (w/w) moisture, respectively (Table 2).
The biocrust organic matter content (OM) increased along the transect at Darsser Ort. When starting in the early grey dune area, low contents (1.0% DW) were measured. With biocrust development, the OM content rose toward the mature dune, up to 11.0% DW in the biocrusts. The development of the OM content in biocrusts showed a similar pattern at Pramort. The biocrust-free and temporarily submerged sediment of the windwatt had an OM content of 0.2% DW. The OM contents along the successional gradient followed the order: yellow dune (0.3% DW) < grey dune (5.7% DW) < mature dune (18.6% DW) (Table 2).
The contents of nutrient elements (Ct, Nt, Pt) in the biocrusts increased along both transects towards older dune successional stages. The Ct values measured in the biocrusts at Pramort exceeded the values at Darsser Ort by a factor of 2 to 4. Furthermore, the Ct contents at the Darsser Ort increased less steeply (0.9 to 29.9 g kg−1 DW) than at Pramort (2.1 to 61.7 g kg−1 DW) (Table 3). In the biocrusts, a gradual increase in total nitrogen (Nt) content was measured along both transects. The Nt concentrations within the biocrusts along the transect at Darsser Ort increased 14-fold from the eGD (0.1 g kg−1 DW) to the MD (1.4 g kg−1 DW) area. The nitrogen concentrations in the GD (2.6 g kg−1 DW) and MD (2.0 g kg−1 DW) at Pramort exceed the highest concentration measured at the Darsser Ort MD (Table 3). Pt concentrations in the biocrusts indicated a slight accumulation of P along each transect toward the mature dune area. For biocrust at Darsser Ort, Pt ranged between 71.8 and 110.4 mg kg−1 DW). Total P along the Pramort transect ranged between 34.8 and 199.1 mg kg−1 DW (Table 3).
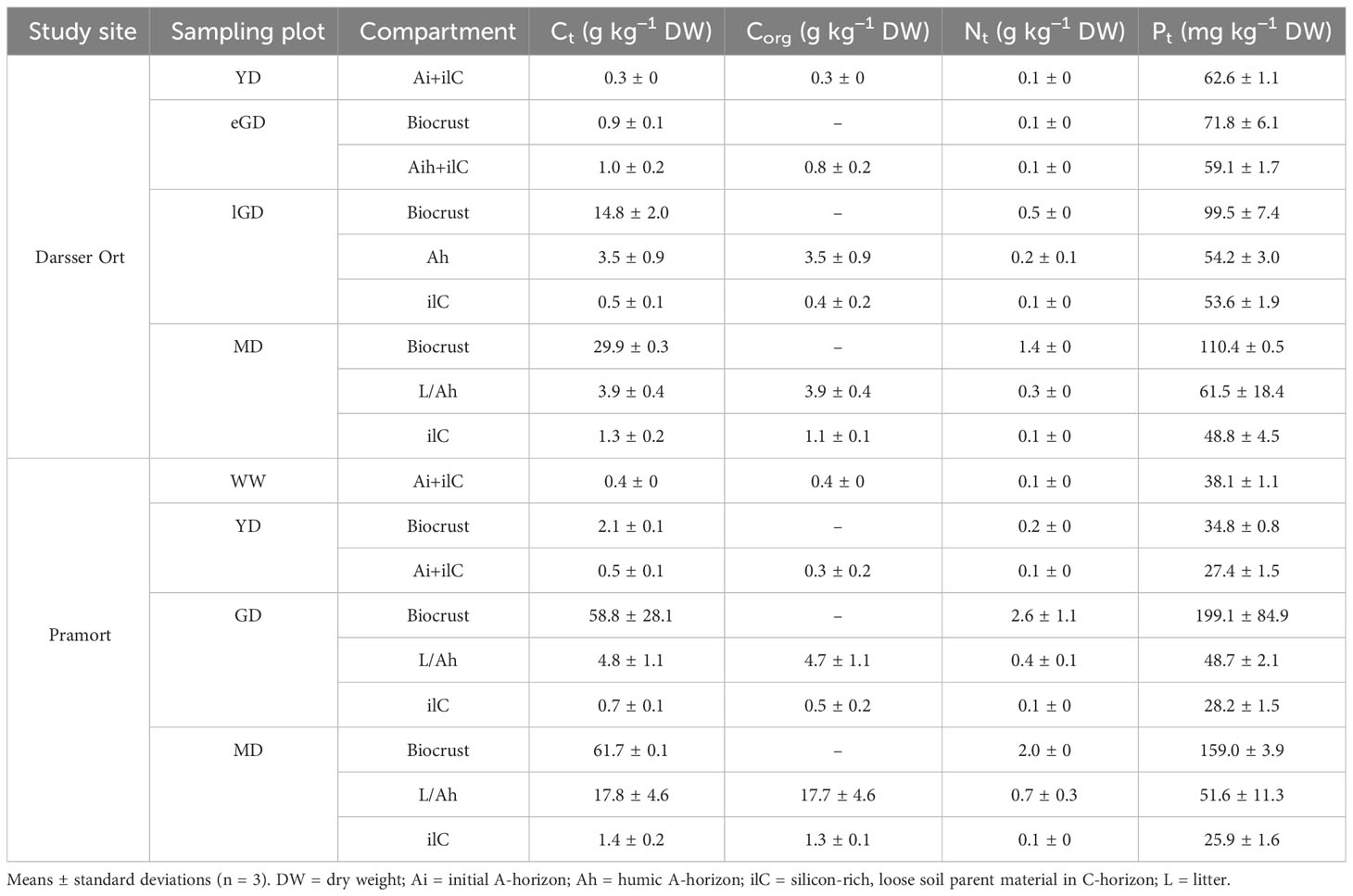
Table 3 Pedological description and nutrient element contents in biocrusts and sediment/soil cores of both sampling sites.
3.1.2 Sediment/soil cores
As a first step in pedological analyses, the sediment/soil cores were interpreted in terms of abundant soil horizons according to the German classification system KA5 (Ad-hoc Arbeitsgruppe Boden, 2005). Cores of the yellow and early grey dune at Darsser Ort, as well as the windwatt and yellow dune at Pramort, were initially assigned to parent material (C-horizon) from the sole visual inspection. However, the organic carbon of these samples indicated initial soil A-horizon forming processes, resulting in the description as “A + C”. A layer of undecomposed litter on top of the mineral material was indicated by the symbol “L”. The cores of the remaining dune plots were already visually divided into soil horizons in the field (Figure 4). Additional geogenic and pedogenic characteristics were added to qualify the main soil horizons.
The Ct and Nt concentrations of the top- and subsoil horizons increased along both transects toward older dune successional stages. It was noticeable that the topsoil horizon Ct concentrations at the Darsser Ort increased significantly less (0.3 to 3.9 g kg−1 DW) than at Pramort (0.4 to 17.8 g kg−1 DW) (Table 3). At the Darsser Ort, significant differences in the Ct concentration (p < 0.001) were detected between the younger dune successional stages (YD and eGD) and the older ones (lGD and MD). At Pramort, significant differences in the Ct concentrations (p < 0.001) between all dune stages, except for the windwatt and the yellow dune, were determined. Organic carbon (Corg) was detected in all samples and increased clearly in the topsoil horizons along both transects. Although both transects started with almost similar Corg concentrations (yellow dune (DO) = 0.3 g kg−1 DW; windwatt (PO) = 0.4 g kg−1 DW), the values then differed significantly from each other in the mature dune area at Darsser Ort (3.9 g kg−1 DW) compared to Pramort (17.7 g kg−1 DW). In general, an increase in the Ct and Corg carbon content was observed in the top- and subsoil horizons, however, in the latter to a much lesser extent (Table 3). The Nt concentrations of the cores showed a less steep increase along both transects compared to the Ct concentration. The Nt values at Darsser Ort were in the range of 0.1 to 0.3 g kg−1 DW, while for Pramort, the values increased from 0.1 g kg−1 in the windwatt to 0.7 g kg−1 DW in the mature dune area. The concentrations in the topsoil horizons were higher compared to the subsoil horizon, similar to the carbon contents (Table 3). For the significant differences, the pattern for nitrogen is identical to that for total carbon. The total P concentrations of the sediment/soil cores were below 0.1 g kg−1. The Pt concentrations were slightly higher in the topsoil than in the subsoil horizon. The differences between the top- and subsoil horizons were highest in the mature dune areas of both transects. While the Pt values of the windwatt and the yellow dune at the Pramort scarcely differed from each other, significant differences in the Pt content were found between the yellow dune and the grey dune, as well as the mature dune (p < 0.01). At the Darsser Ort, on the other hand, there were no significant differences in the Pt concentration between the dune types. The biocrusts generally showed higher Ct, Nt, and Pt contents than the respective soil parent materials in C-horizons (Table 3).
Overall, the iron (Fe) contents of all samples were low, averaging 790 mg kg-1 for total Fe (Fet), 210 mg kg-1 for dithionite-citrate-bicarbonate extracted Fe (Fedit), and 24 mg kg-1 for oxalate extracted Fe (Feox). The lowest concentrations of Fedit and Feox were detected in the windwatt and YD samples, and the highest in the mature dune samples. Slightly irregular increases in the Fedit contents toward the inlands were recognized, which confirmed an advanced degree of weathering of Fe-containing minerals and the formation of secondary pedogenic oxides in this direction. The ratios Fedit/Fet ranged between 0.14 and 0.4. The Feox/Fedit ratios ranged between 0 and 0.2 (Supplementary Table 3).
The topsoil horizons of the sample cores were low in calcium carbonate (CaCO3). However, if a distinction was made between the topsoil and C-horizons, the latter had significantly more carbonate, except for the mature dune at Pramort. The pH (CaCl2) was measured in the sediment/soil cores, if possible, in all divided soil horizons. At both transects, the pH decreased with increasing dune succession. All samples were slightly to strongly acidic, with pH values ranging from 3.6 to 6.2. At the Darsser Ort transect, no difference in the pH between different horizons was measured. However, at Pramort (grey dune and mature dune), the L/Ah horizon tended to be more alkaline than the underlining ilC horizon. The values for the electrical conductivity of the saturation extract in the sediment/soil cores were below 0.75 mS cm−1 in all samples, which is a threshold for description of a soil horizon as “saltish” according to Ad-hoc Arbeitsgruppe Boden (2005) (Supplementary Table 4).
3.2 Sequentially extracted P (P pools)
The Pt concentrations in the topsoil horizons were generally very low. The Pt concentrations of sequentially extracted fractions were below the detection limit of ICP OES (≤ 0.05 mg L−1). However, determination of molybdate reactive P was possible, which is considered to be inorganic P (Pi). Whenever the ICP OES values were above the detection limit, they were used to calculate the organic phosphorus (Po) content of the sample as well.
For the coastal dune area at Darsser Ort, the H2SO4 fraction had the highest P concentrations and proportions in the soil samples (27.6 to 35.4 mg kg−1, corresponding to 46.2% to 56.6% of Pt; Table 4). This was the only fraction where Pi and Po could be distinguished. On the other hand, at Pramort, the labile P fractions (resin-P and NaHCO3-P) exhibited the highest P concentrations and proportions (25 to 45.4 mg kg−1, 56.4% to 74% of Pt; Table 4). Proportions of NaOH-P (moderately labile Pi and Po, latter only at Pramort MD) ranged between 0% and 8.7% of Pt at Darsser Ort and 0% and 26% of Pt at Pramort. The distribution of the different P fractions showed the same trends with increasing age and soil development at both transects. An enrichment of more labile (easily bioavailable) P was observed at Darsser Ort. At Pramort, the enrichment became more obvious in the moderately labile P fraction. At the same time, the Ca-bound P concentrations and proportions (H2SO4-fraction) decreased. This trend was more pronounced at the Pramort dune chronosequence than at Darsser Ort. For all sediment/soil cores of the Darsser Ort and Pramort transect, the proportions of summed Pi-fractions ranged between 76.7% to 81.1% and 80.8% for 97.2% of Pt, respectively. The proportions of summed Po fractions were between 18.9% to 23.3% of Pt at Darsser Ort and 2.8% to 25.8% of Pt along the Pramort transect. In the biocrusts of coastal dunes, the P contents were larger by factor three than in the sediment/soil cores. Labile and easily soluble P was absolutely enriched at the expense of Ca-bound P (H2SO4-fraction) similar to the finding for sediment/soil cores (Supplementary Figure 4).
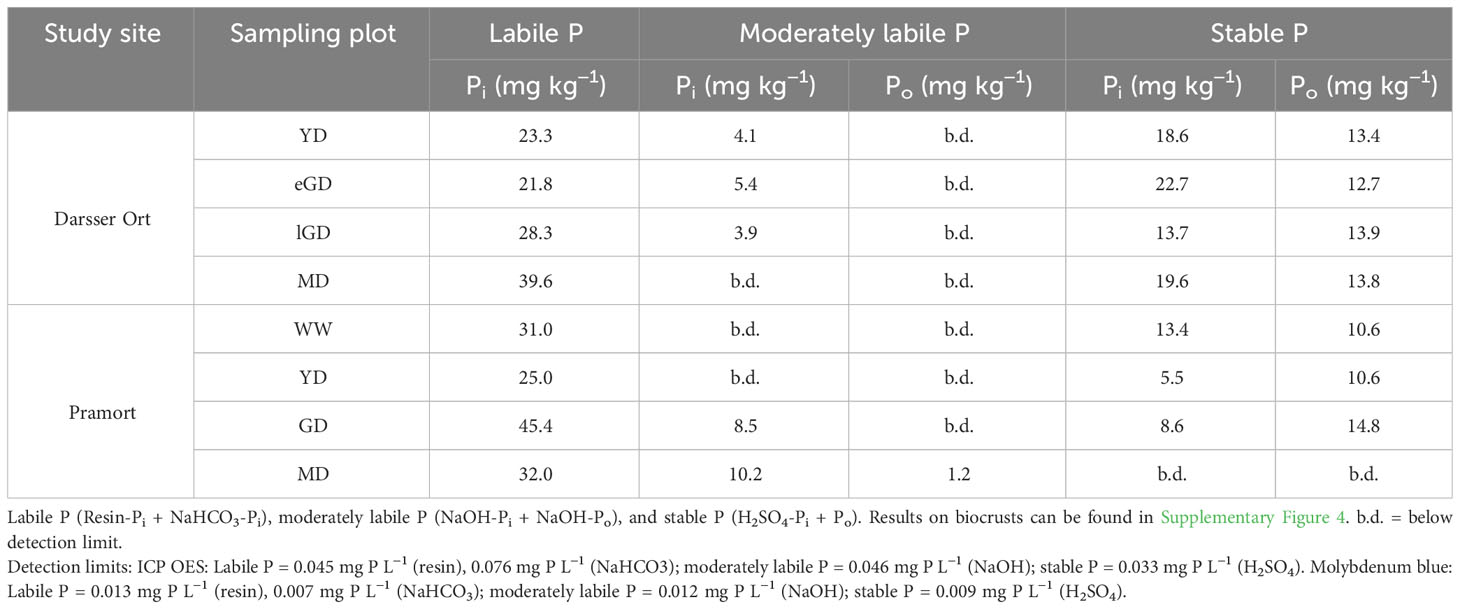
Table 4 Concentrations (mg kg−1) of sequentially extracted inorganic (Pi) and organically bound (Po) phosphorus fractions (mg kg−1) in the topsoil horizon of the sediment/soil cores (n = 3).
3.3 Vegetation survey
At both study sites, a general shift from bare sediment via microalgae-dominated biocrusts in younger dunes to moss- and lichen-dominated biocrusts covers in later dune stages was observed. At Darsser Ort, the biocrusts percentage coverage ranged between 0% and 72% in the different dune stages, while at Pramort it was 0% and 76%. The dominating biocrust cover showed high variability between the different successional dune stages at each site. The following simplified figure schematically illustrates the distribution of the dominant functional groups along an idealized dune succession gradient (Figure 5).
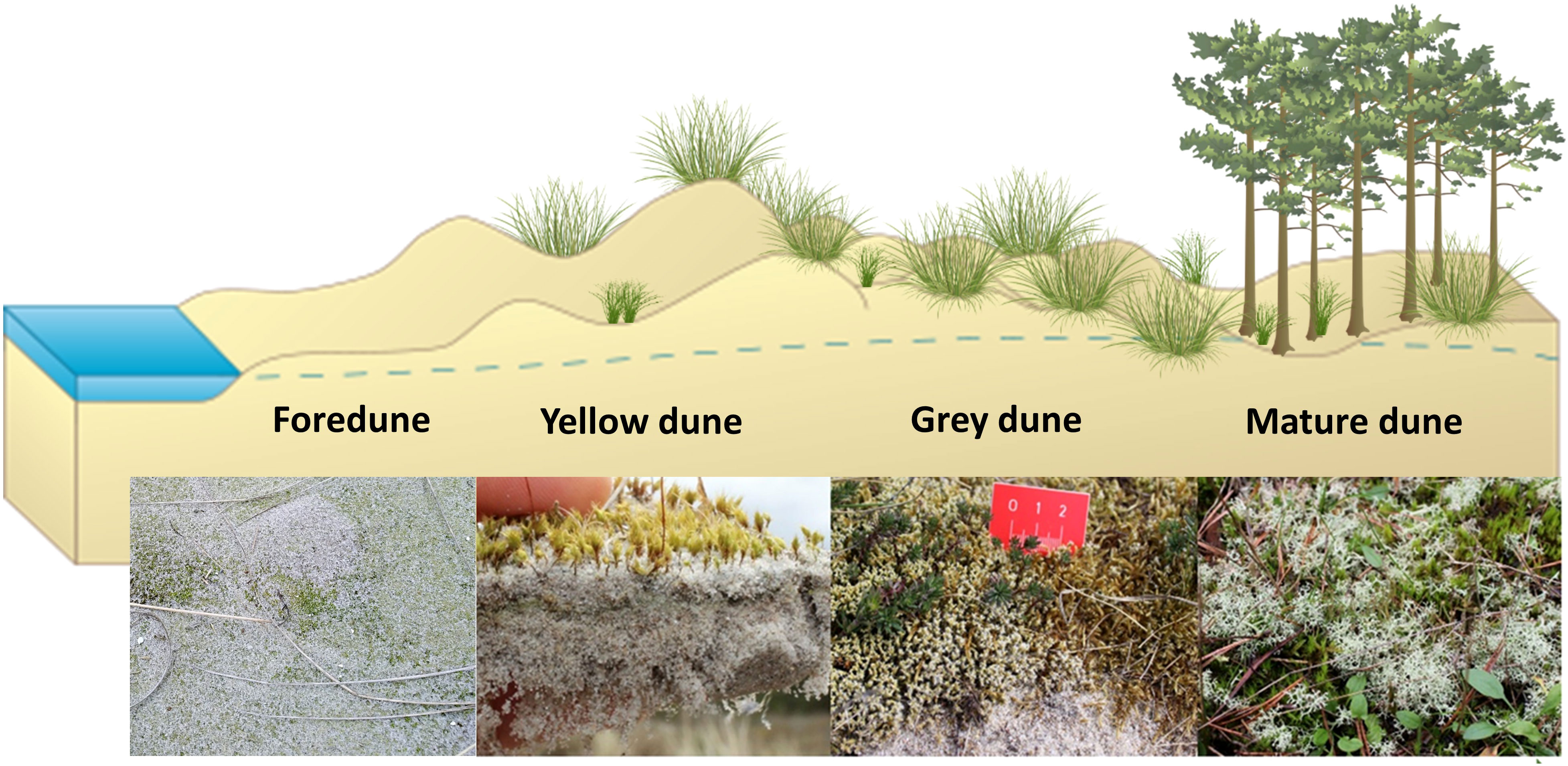
Figure 5 Schematic dune succession and pictures of the functional groups dominating the individual dune subsites. Primary dune formation (foredune) is assumed in the scheme. For the dune formation at Darsser Ort, a deviating secondary dune formation at the dune cliff applies. Created with BioRender.com.
At Darsser Ort, the first dune type under investigation was a yellow dune at a dune cliff. This had no cryptogamic cover (Figure 6). The surface was mainly bare sediment (68%) or covered by vascular plants (20%), identified as Ammophila arenaria (L.) Link. Organic litter occurred sporadically (12%). When following the transect inland into the grey dune area, the earliest biocrust covers were detected in this dune area. The grey dune area could be visually divided (by dune sand color and vegetation) into an early phase of grey dune succession (eGD) and a later, more stabilized grey dune area (lGD). In the eGD, first biocrust covers dominated by microalgae (44%), defined as initial biocrusts, were detected. Furthermore, smaller mosses and occasionally lichens grew in this early grey dune area as well (4%). Biocrusts were the dominant surface cover, with 60% in the eGD area, followed by patches of bare sediment (20%) and vascular plants (12%) (Figure 6). The late grey dune area showed a change in dominant biocrust functional groups. Moss-dominated biocrusts (36%) and those associated with lichens (28%) covered the lGD area (Figure 6). A thick moss and lichen carpet overgrew the dune surface with further dune succession. This shift in the biocrust functional groups denoted the transition into the mature dune area. The functional groups of moss-dominated (36%) and moss-lichen-dominated biocrusts (36%) were most present in this area (Figure 6). Moreover, patchy accumulations of mainly pine (Pinus sylvestris L.) needles, fallen leaves, and other dead plant material covered 12% of the investigated dune surface. Bare sand areas decreased along the dune chronosequence and became rare in the late grey and mature dunes.
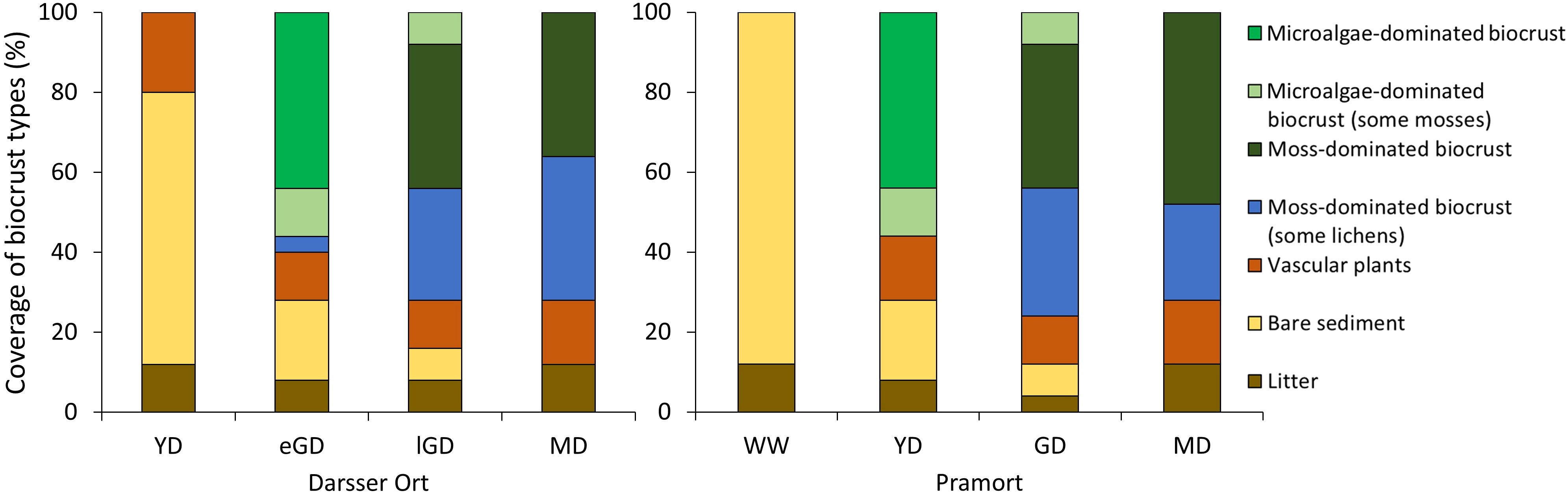
Figure 6 Summary of the vegetation survey. The percentage of the area covered by the different functional groups as determined with the point intercept method along the two transects. Darsser Ort; dune types: YD, yellow dune; eGD, early grey dune; lGD, later grey dune; and MD, mature dune. Site: Pramort; dune types: WW, windwatt; YD, yellow dune; GD, grey dune; and MD, mature dune.
As observed at Darsser Ort, the surface coverage of biological soil crusts increased along the succession gradient at Pramort, too. Bare sediment (88%) and organic litter (12%) characterized the windwatt (Figure 6). This wind flat becomes exposed when the wind drives water out of the lagoon. Detected litter was therefore mostly identified as flotsam, remains of reed material, and molluscan shells. The first biocrusts along the chronosequence were detected in the following yellow dune area. This sampling plot was dominated by early microalgae biocrusts (44%). Small mosses associated with microalgae (12%) occurred only near vascular plants (16%), identified as Ammophila arenaria. Further inland in the grey dune area, moss-dominated biocrusts became the prevailing biocrust functional group (36%). Moreover, this dune successional stage had the highest variety of biocrust functional groups found at all four subsites (three functional groups out of four) (Figure 6). The following mature dune area was partly forested with pine trees, like the mature dune area at Darsser Ort. Mosses were dominating and interspersed with lichens.
3.4 Biocrust phototrophic community composition
3.4.1 Algae and cyanobacteria
In total, 25 algal and cyanobacterial species have been detected at seven out of eight sampling plots. The richness of species was expressed as the total species number identified per plot. The only plot showing no species was the yellow dune at Darsser Ort. A total of 21 algal species (10 at Darsser Ort, 14 at Pramort) and four cyanobacterial species were detected using culture-dependent methods, followed by morphological identification (Table 5 and Figures 7A–R). Along the Darsser Ort transect, the early grey dune showed the highest algal species richness (six out of 11 species). The yellow dune plot was the most rich in species (seven out of 17 species) at the Pramort transect.
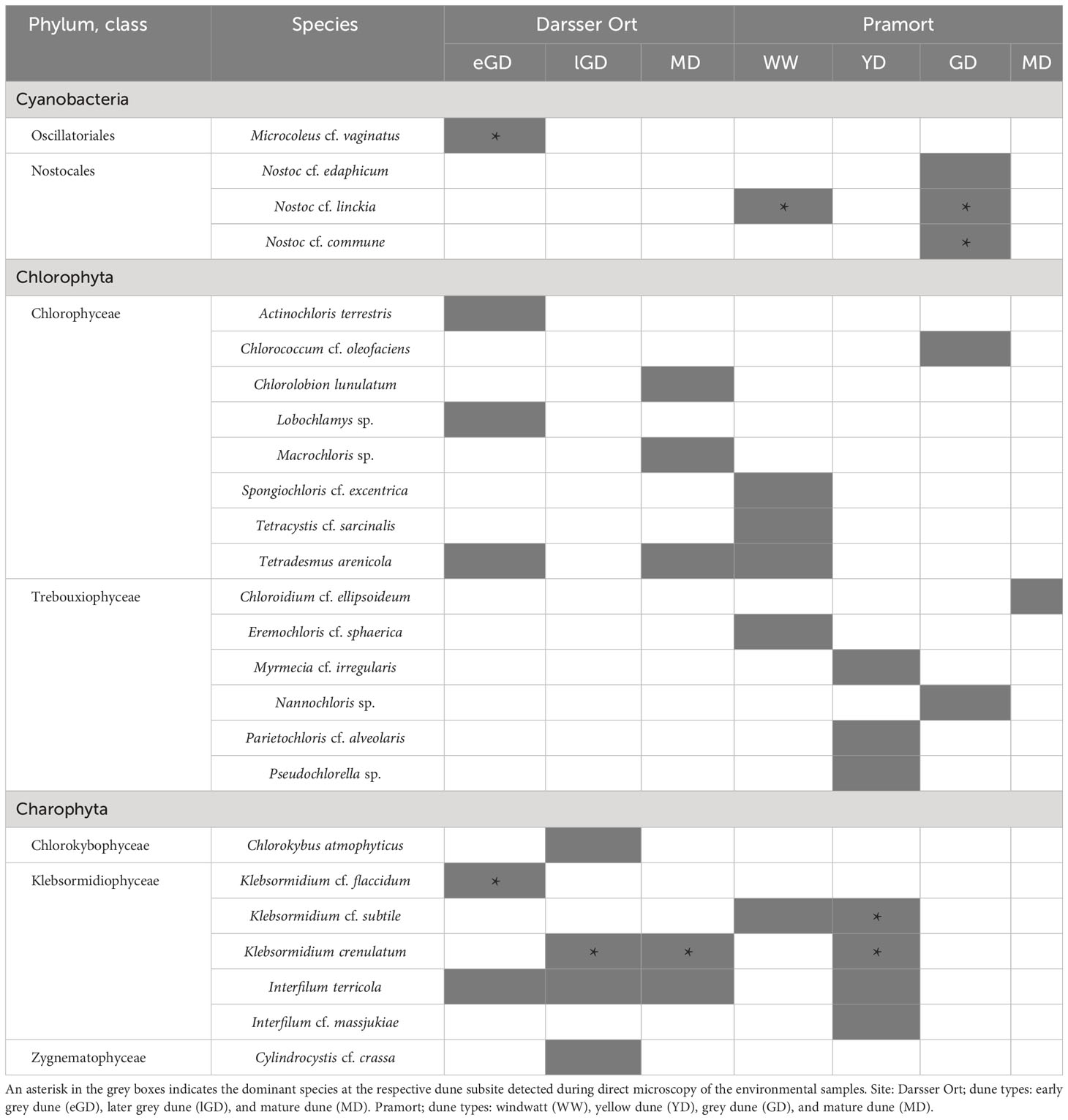
Table 5 Green algal and cyanobacterial species in biocrusts identified at seven different dune plots using a culture-dependent approach (present species are colored in grey shade).
Fourteen species of Chlorophyta dividing into two classes were determined (eight species of Chlorophyceae, six species of Trebouxiophyceae). The phylum of Charophyta was represented by three classes with the Klebsormidiophyceae being the most widespread (five out of seven Charophyta species). Four species of Cyanobacteria were found: representatives of Oscillatoriales and Nostocales. The most frequently detected species were Interfilum terricola (Figures 7E, F), Klebsormidium crenulatum (Figures 7A, B), and Tetradesmus arenicola (Figures 7G–I). Klebsormidium crenulatum was the dominant algal species in all plots, if present. If cyanobacteria occurred, these were almost always the dominant species in the respective plot (DO: eGD; PO: WW, GD). The remaining species dominated in two [Klebsormidium cf. subtile (Figure 7C) and Nostoc cf. linckia (Figure 7J)] or just one sampling plot. Both study sites had only 12% (three species) of the identified species in common (Supplementary Figure 5A). Biocrusts of different sampling sites are characterized as cyanobacterial (with dominance of Nostoc, windwatt, and grey dune of Pramort), green (with dominance of Klebsormidium, later grey and mature dunes of Darsser Ort as well as yellow dune of Pramort) or mixed nature (Microcoleus cf. vaginatus (Figures 7N, O) and Klebsormidium cf. flaccidum (Figures 7D), early grey dune of Darsser Ort). Mature dune crust of Pramort included only one occasionally occurring green algal species (Chloroidium cf. ellipsoideum).
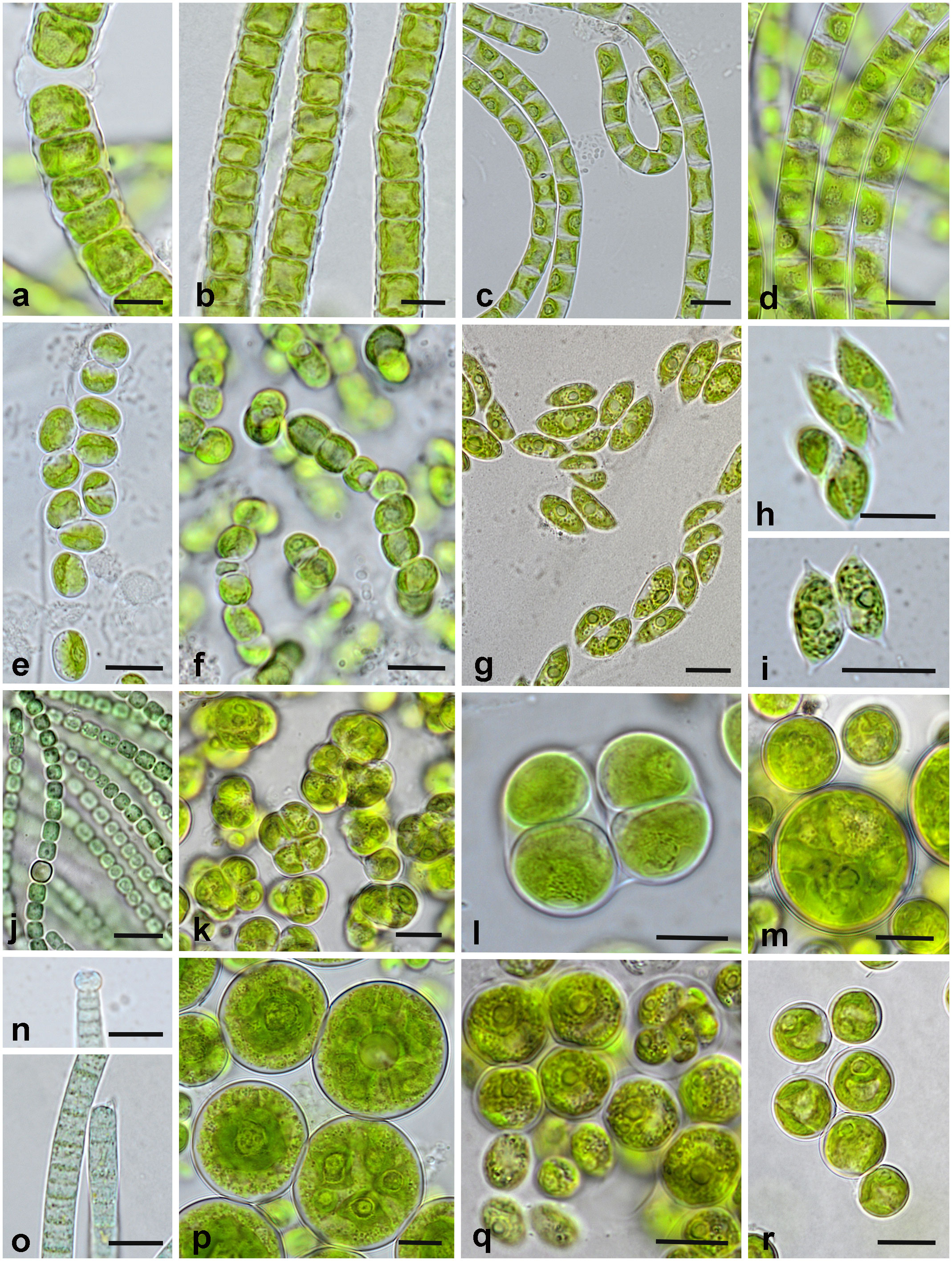
Figure 7 Representative algae and cyanobacteria from the biocrusts of Darsser Ort (DO) and Pramort (PO). (A, B) Klebsormidium crenulatum [(A) PO-YD; (B) DO-lGD]; (C) Klebsormidium cf. subtile (PO-YD); (D) Klebsormidium cf. flaccidum (DO-eGD); (E, F) Interfilum terricola [(E) DO-lGD; (F) PO-YD]; (G–I) Tetradesmus arenicola [(G) PO-WW; (H, I) DO-eGD]; (J) Nostoc cf. linckia (PO-WW); (K) Tetracystis cf. sarcinalis (PO-WW); (L) Chlorokybus atmophyticus (DO-lGD); (M) Spongiochloris cf. excentrica (PO-WW); (N, O) Microcoleus cf. vaginatus (DO-eGD); (P) Actinochloris terrestris (DO-eGD); (Q) Chlorococcum cf. oleofaciens (PO-GD); (R) Eremochloris cf. sphaerica (PO-WW). Scale bars = 10 µm.
3.4.2 Moss species
In total, 16 moss species (Bryophta) were detected at six of eight sampling plots in this study. The mature dune plot at Darsser Ort showed the highest species number of all investigated plots (six out of eight species). The species-richest plot along the Pramort transect was in the grey dune area (Table 6). With the exception of one species from the Polytrichopsida class (Polytrichum juniperinum), 14 species have been identified, all of which belong to the Bryopsida class. The remaining two species were assigned to the class of Jungermanniopsida (Marchantiophyta). Four out of all 16 species occurred in ≥50% of all moss-holding sampling plots. Two species (Cephaloziella divaricata and Ceratodon purpureus) were almost omnipresent; 10 of all detected species were found in one plot only. The two study sites showed 37.5% (six species) of the identified species in common (Supplementary Figure 5B).
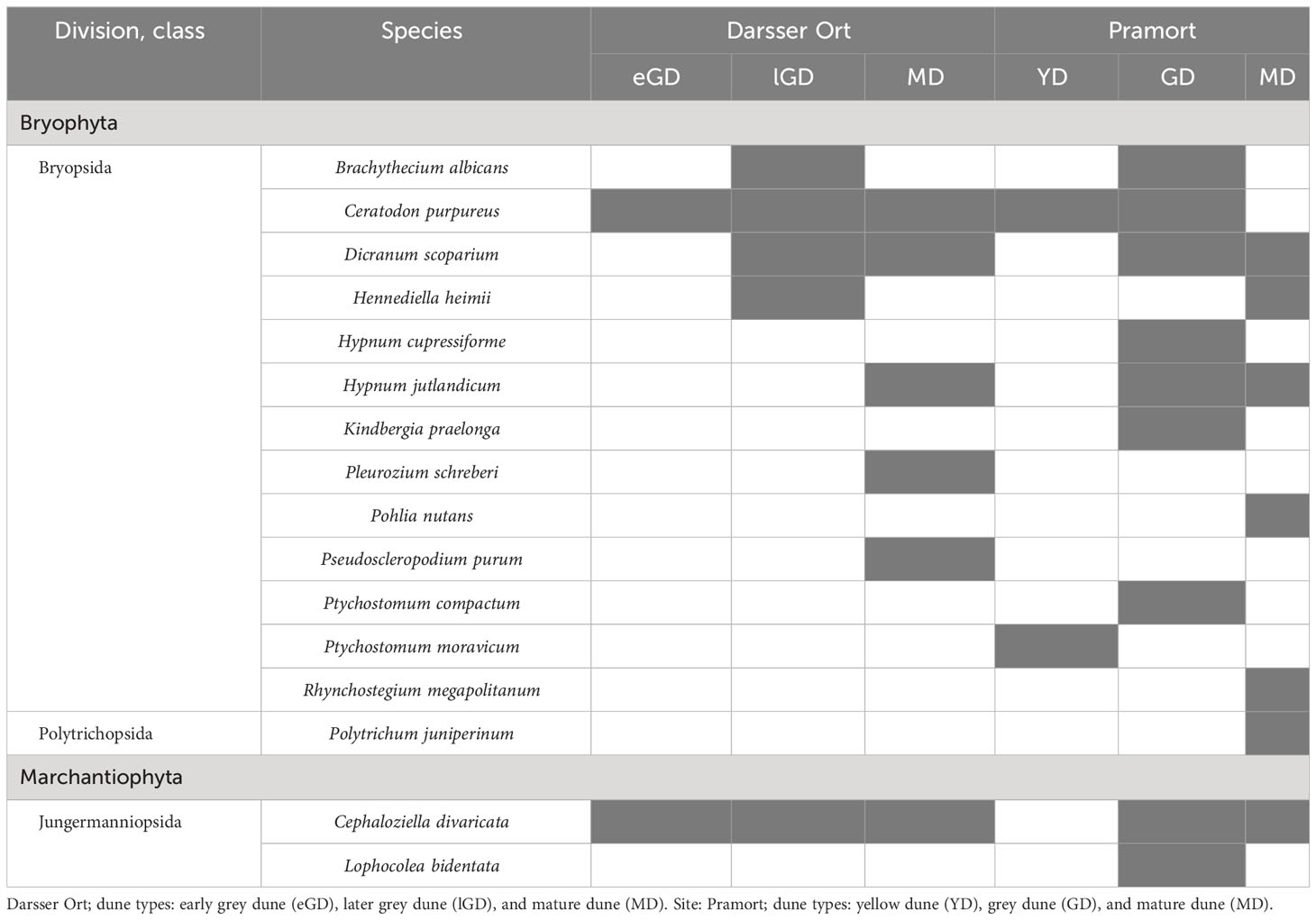
Table 6 Moss species in biocrusts detected at six different dune plots (present species are colored in grey shade).
3.4.3 Lichen species
In this study, 26 lichen species were found at five out of eight sampling plots (Table 7). The late grey dune and mature dune plots at Darsser Ort, as well as the grey dune and mature dune plots at Pramort, hardly differed in the number of lichen species. The early grey dune area at Darsser Ort was the species poorest lichen-holding sampling plot (three of 26 species). All determined lichen species belonged to families of the class Lecanoromycetes, and 24 out of 26 species were members of the order Lecanorales. Cladonia was the dominant genus across both study sites. Of the determined species, 19 belonged to this genus; 14 out of the overall detected species were found at both sampling sites (53.9%) (Supplementary Figure 5C). Lichen species that preferred to grow on sandy substrates predominated at both study sites. Only four out of 26 determined species are known to preferably grow on litter. A detailed table on the pH preference of the individual taxa is compiled in Supplementary Table 5. Those litter-preferring species were found in the lGD (DO) and respectively grey dune area (PO) and belong to the subneutrophytic and basidophytic lichen species. Strongly acidophytic species (e.g., Cladonia macilenta) were found especially in the mature dune area.
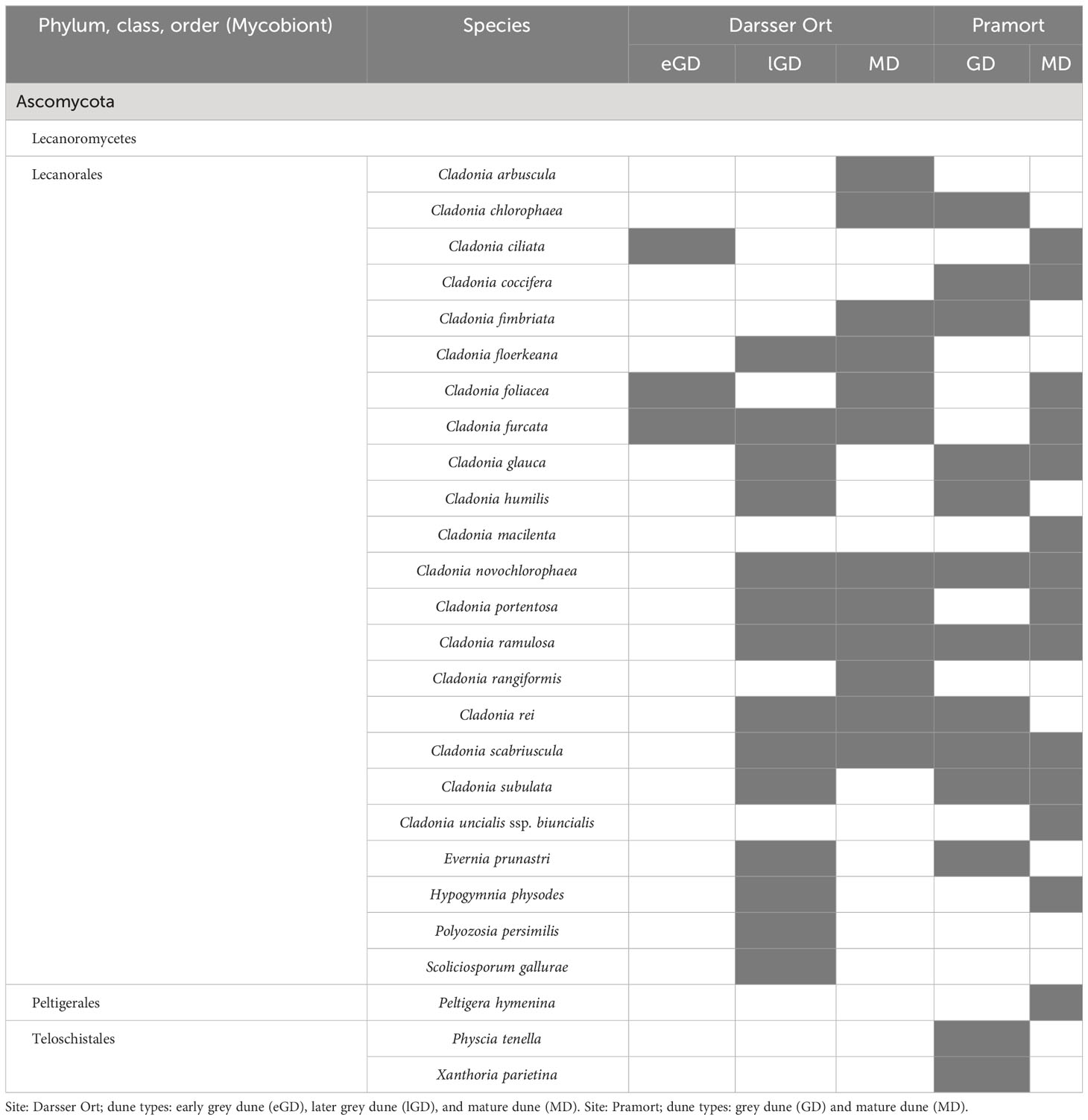
Table 7 Lichen species in biocrusts detected at five different dune plots (present species are colored in grey shade).
3.5 Relationship between chemical soil properties and biocrust communities
A relationship between nutrient element concentrations in the biocrusts (Ct, Nt, Pt) and their successional stage became obvious. The growth of moss-dominated biocrusts in later successional dune stages led to enhanced biomass production in these dune types. The parallel increase in the Chl a content confirmed this observation. Hence, a directly related nutrient element accumulation could be proven. The total species number of mosses and lichens (alpha diversity = α) in the biocrusts differed between the successional dune stages, as visualized in the nMDS plot (Figure 8). A grouping of the early dune successional stages (PO:WW and YD; DO:eGD) relative to the older ones was evident from the biocrust community (PO:GD and MD; DO:lGD and MD). The PerMANOVA showed significant correlations between the community composition of the biocrusts and enrichment with Ct (p = 0.038) and Pt (p < 0.01) in the topsoil horizons.
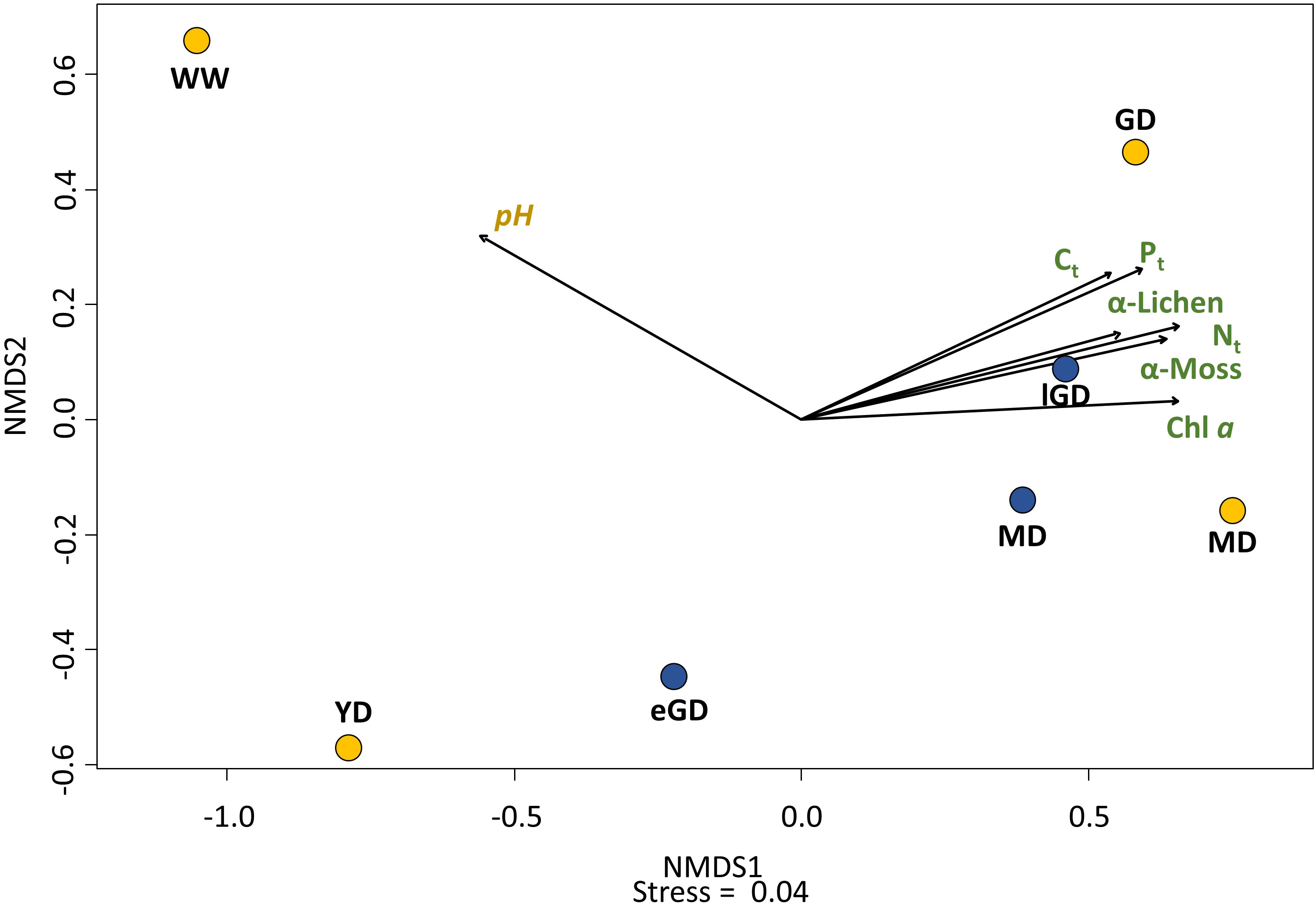
Figure 8 The nMDS plot based on the Bray–Curtis dissimilarity index visualized the differences and similarities in the community composition of grouped lichen, mosses, and algae (α-diversity) between the seven sampling sites. Yellow: accumulation coast (Pramort). Dune types: WW, windwatt; YD, yellow dune; GD, grey dune; and MD, mature dune. Blue: abrasion coast (Darsser Ort). Dune types: eGD, early grey dune; lGD, later grey dune; and MD, mature dune. The black arrows indicate the influence direction of the significantly correlated biocrust and sediment characteristics. Stress = 0.04.
4 Discussion
4.1 Analyses of nutrient and sediment/soil characteristics
In contrast to previous studies, which only examined Ct and Nt element concentrations in individual dune types, this study measured for the first time changes in these element concentrations along an entire dune succession. Thereby, the Ct and Nt contents increased along both successional dune gradients. The Ct concentrations of the biocrusts measured at Darsser Ort (0.9–29.9 g C kg−1) were similar to results from a former study (3.4–48 g C kg−1) conducted on the islands Rügen and Usedom, which are 70 to 100 km further east (Baumann et al., 2019). The Ct contents of biocrusts measured in the grey and mature dunes at Pramort (58.8 g C kg−1 and 61.7 g C kg−1, respectively) and Darsser Ort (14.8 g C kg−1 and 29.9 g C kg−1, respectively) were similar to biocrusts from temperate forests (23–56 g C kg−1) (Baumann et al., 2017). The biocrusts in the later successional dune stages at Pramort (PO) were more dominated by mosses than at Darsser Ort (DO), as reflected in their higher proportion of organic matter and larger Ct values (Table 3). Not surprisingly, the Ct concentrations in the biocrusts always significantly exceeded those of the underlying soil horizons because of the organism’s biomass and their released metabolites in the biocrust matrix. Furthermore, Ct in the soil samples was almost exclusively organically bound, which can be explained by low proportions of carbonate from marine organisms such as the shells of mussels. This indicates that organic molecules formed in the biocrusts may have been translocated downwards in the soil profiles, pointing to a strong influence of the biocrust community on the underlying soil horizons, which otherwise scarcely receive organic carbon from other sources. The importance of the biocrust community forming the first organic layer at the topsoil horizon becomes obvious. Moreover, fungal hyphae, bacterial communities, and moss rhizomes connect the biocrust with the underlying soil and sediment and support nutrient transfer to these less inhabited compartments (Eldridge and Greene, 1994). An increase in the Nt concentration in the biocrusts along the succession gradients was also observed (Table 3). Likewise, the Nt concentrations in the biocrusts were above those of the underlying soil and sediment samples. Zhao et al. (2010) showed that biocrust-fixed atmospheric N is an important N source for the ecosystem and its vegetation restoration in the Loess Plateau, China, by accumulating between 4 and 13 kg N ha−1, depending on the dominant biocrust type. Further studies confirmed this biologically mediated terrestrial N accumulation by biocrusts for temperate forests (Glaser et al., 2018) and the Arctic tundra (Pushkareva et al., 2022).
Only very low iron (Fet) contents were measured in the topsoil horizons at both study sites. The Fet values at all sampling points at Darsser Ort were higher than those at Pramort, suggesting a larger supply of Fe-containing minerals in the parent rock material at this site. The proportion of dithionite-soluble iron (Fedit) increased along the dune chronosequence, confirming an advanced degree of mineral weathering towards the inland. Cornell and Schwertmann (2003) described the Fedit/Fet ratio as a measure of soil development, and values between 0.2 and 0.3 indicate progressing soil development in the northern hemisphere. The Fedit/Fet ratios measured in this study (DO: 0.14–0.3, PO: 0.17–0.4, Supplementary Table 3) confirmed the beginning of soil development in the dunes. Initial silicate weathering is expected to lead to an increase in oxalate-soluble iron (Feox) contents (Cornell and Schwertmann, 2003) with dune succession, as the present study indicated for temperate coastal dunes at the Baltic Sea coast. However, both chronosequences did not show the expected decrease in Feox/Fedit ratio with soil development, according to Cornell and Schwertmann (2003). This result indicates that at least the investigated dunes of the Baltic Sea could be an exception here. Likely, such a development is possible if the supply from weatherable Fe-containing minerals in the parent rock material or the developing soil is sufficiently high for a continuous and subsequent Feox supply. If this replenishment stops, the expected development of pedogenic Fe oxides cannot happen over time. Most probably, that was the case in both studied dune areas since the Fet supply in the quartz-rich sand might have been quickly exhausted over time. In the case of the younger dune types, advanced weathering of Fe-bearing silicates cannot be expected (Fischer et al., 2010).
The various possible soil-forming processes in the Holocene sands of the dunes were related to decreases in sediment pH in parallel to the calcium carbonate weathering in the sediment/soil cores. The measured carbonate contents at all dune subsites were very low (0–2.1 g kg−1). They were clearly below the values measured on the coast of Rügen (14.6–127.8 g kg−1) and Usedom (6.6–7.5 g kg−1) (Baumann et al., 2019). Likely, the chalk cliffs on Rügen, mainly formed by calcium carbonate-containing limestone, positively influenced the carbonate concentration in the northeast part of the island. Slightly higher carbonate contents in the younger yellow dune stages (Supplementary Table 4) represented the original, almost unweathered parent material, which is shell-containing dune sand. A decrease in the carbonate content with dune succession suggested an ongoing decalcification of the sandy sediment. This observation is consistent with the studies on the Arenosols of dunes on the island Spiekeroog (Giani and Buhmann, 2004; Pollmann et al., 2018). In all previously mentioned studies, the top sediment layers had lower carbonate contents than the subsediment. This observation reflects the metabolic activity of the biocrusts and their impact on soil formation in sandy substrates. Due to CO2 and H+ produced during microbial soil respiration (Kuzyakov, 2006) and the excretion of organic acids, biocrusts releasing these substances could potentially promote a more rapid decalcification in the uppermost sediment layer (Fox, 1995; Belnap, 2011), thereby forming a soil horizon. Consequently, carbonate appeared enriched in the deeper horizons of the older dune types, which can be explained as an indication of the original sediment Ca-carbonate content, perhaps plus precipitates from leached Ca- and hydrogen-carbonate ions. Calcite weathering is a fundamental soil-forming process in all Ca-containing parent rocks, including Pleistocene glacial till and Holocene dune sands (Earle, 2019; Daumantas et al., 2022), and could be tracked in detail along a chronosequence in this study.
The decrease of the pH along the chronosequences is in line with results from the coastal dunes of the North Sea island Spiekeroog (Isermann, 2011; Pollmann et al., 2020). The drop in pH was more pronounced in the topsoil horizon along the chronosequence at Pramort (pH 6.2 to 3.6) than at Darsser Ort (pH 5.0 to 4.3). This indicates that the dunes at Darsser Ort are older than those at Pramort, assuming similar calcite contents and aboveground impacts on the acidity of the sediment, either from the atmosphere or the initial organism colonization. Isermann (2011) measured a pH of 4.6 in early and 3.4 in mature grey dunes. The same applies to a study by Giani and Buhmann (2004), who reported a pH of 4.0 in grey dunes. These analytical data underline the fact that the dunes at Darsser Ort were in a later developmental stage compared to Pramort. The area, initially described as a yellow dune at a dune cliff, is more likely to be an eroded grey dune based on the measured pH (pH 4.9). The dune cliff was continuously covered with a large amount of sand as part of a secondary dune formation process and was therefore designated as a yellow dune in the present study. Isermann (2005) detected that the species richness of vascular plants was greatest at intermediate levels of soil pH along the Baltic Sea coast. The present study was now able to establish such a connection for the algal community in coastal sand dunes of the Baltic Sea since the highest algal species richness was detected in the (early) grey dune stages.
4.2 Total phosphorus and P pools (fractionation)
The total P of the sediment did not decrease, neither in the top- nor the subsoil horizon, with the successional development of the dunes along both chronosequences. This disagrees with the Pt decrease during soil formation, as reported by Walker and Syers (1976). A reason could be that soil formation along both dune successional gradients has not yet progressed far. The Pt concentration of the biocrusts ranged between 35 and 199 mg kg−1, which is slightly less compared to a study by Schulz et al. (2015) (90–310 mg kg−1) along a weathering gradient at the Baltic Sea coast. This difference can be explained by the inhomogeneity of the dune sands with respect to P. The biocrusts accumulated P over time, but the P content in the underlying sediment remained rather constant along the gradient and increased only slightly toward the older dunes, as found at Pramort. This implies that the biocrust community is able to take up essential amounts of P, irrespective of the parent source material on the dunes. With this result, the present study highlights a well-adapted biocrust community that is able to acquire P from different sources in addition to those from the parent material to make P bioavailable. The trapping of nutrient-enriched aeolian dust could be such a potential P source (Reynolds et al., 2001; Chamizo et al., 2012) or P-containing precipitation, which is a quantitatively important nutrient source in the study region (Berthold et al., 2019). Furthermore, it was evident that the P concentration in the topsoil horizons exceeded that in the subsoil horizons. This indicates that some of the P biologically accumulated in the biocrusts has been transferred into the subsoil horizons through leaching or any other redeposition process. Thus, our data confirmed Young et al. (2022), who described this as one of the main mechanisms by which biocrust fertility is transferred downward in the soil.
In the early successional dune stages at Darsser Ort, stable P was identified as the major P pool. This included Ca-bound P, e.g., P in primary minerals such as apatite, P occluded within sesquioxides, as well as stable organic P compounds (Hedley et al., 1982; Sims and Pierzynski, 2005). Moderately labile P, likely originating from P bound to Fe-/Al-oxide surfaces (Hedley et al., 1982), constituted a minor proportion of the P pools at Darsser Ort (Table 4). This can be explained by the low contents of weatherable Fe-containing minerals and already-formed Fe oxides in the aeolian sands. The proportion of labile and bioavailable P compounds continued to increase with dune succession and became the major P pool in later dune types. Such a conversion took place at the expense of the stable P pool, which is in line with the results of Baumann et al. (2019) from a similar study area. In contrast, the labile P was identified as the major P pool in all dune types along the dune chronosequence at Pramort. The relative proportion of P in this pool continued to increase (56% to 74% of Pt) as dune succession progressed (Table 4). The proportion of stable-bound P played only a minor role in the later dune types and was no longer measurable in the mature dune area. This development indicated an accumulation of easily available P in the topsoil horizon, which indicated the microbial activity of the biocrust organisms as assumed in hypothesis (5). It remains to be clarified whether this labile P pool is a P source accessible to other organisms. Hence, it is possible that P was stored in the biocrust organisms and was thus involved in a more closed P cycle. The proportion of organically bound P (Po) in the biocrusts was higher than in the underlying sediment, reflecting the presence and biological activity of biocrust organisms as well as their organic decomposition products. The low to non-detectable proportion of Po (0%–1.2% of Pt) calculated for the younger dune types was likely due to the low amounts of organic matter found on these dune types.
4.3 Biocrust characteristics and vegetation survey
The sand dunes on the peninsula Fischland-Darss-Zingst are one example in which microbial diversity changed with soil development during natural succession, which so far has received little attention in this area. In younger dune habitats, which are more endangered by erosive wind forces leading to sand mobility (Martínez et al., 2001), algae-dominated biocrusts predominated. Moss-dominated biocrusts were found in older dune succession stages. The highly mobile dunes nearshore and the airborne salt spray shape environmental conditions where colonization is difficult (Hesp, 1991; García Novo et al., 2004). Consequently, only small to no biocrusts could be formed in mobile dunes. This explained the low chlorophyll a values and organic matter content measured in the windwatt (Pramort) and the yellow dune area at Darsser Ort. The Chl a likely changed with sampling site, season, and biocrust communities. As expected, and in agreement with Büdel et al. (2009), a significant increase in the biocrust Chl a content from the early successional stage to the later ones was observed. Langhans et al. (2009) measured Chl a content between 11 and 295 mg m−2 in a temperate sand ecosystem in the northern upper Rhine valley in south Hessen, Germany, which were similar to those of the present study (1 to 483 mg m−2). Whereas Langhans et al. (2009) could not prove an increase in Chl a content between initial and stable biocrusts, this was for the first time proven for the biocrust succession on the peninsula Fischland-Darss-Zingst. While the moss-dominated biocrusts grown under temperate climatic conditions reached Chl a values up to 483 mg m−2, Büdel et al. (2009) demonstrated significantly lower concentrations in bryophyte-dominated biocrusts in arid regions (120 mg m−2). Further enrichment of the organic matter content along a dune chronosequence was expected, based on the theory of Clements (1916) of habitat alteration and facilitation. The findings of the present study confirmed the assumption of Clements (1916). While in transitional dune areas, the establishment of mature biocrusts was rarely observed, moss-dominated biocrusts took over in the mature dune areas and covered the majority of the dune surface. This dune area is characterized by increased sand stabilization along with less sand accumulation (Martin, 1959) and represents the latest phase of dune succession. The accumulation of organic matter is an indicator of early soil formation (Ampe and Langohr, 2003; Dümig et al., 2014), which is assumed to be based on faster biomass formation and litter input than decomposition within the studied two dune areas. Furthermore, it was proven that biocrusts of mature successional stages were capable of promoting sediment moisture, as reflected by an increase in water-holding capacity and water availability of the thicker moss-dominated biocrusts (Gypser et al., 2016; Sun et al., 2022). Compared to algae-dominated biocrusts, mosses can absorb more water per biomass unit (Gypser et al., 2016) and transfer it via their rhizoids into deeper soil layers (Kidron et al., 2010). Thus, these mature biocrusts fulfill a relevant function for colonization with higher vegetation, which is essential for dune stabilization and finally coastal protection.
4.4 Community composition of the phototrophic species within the biocrusts
Within the present study, the algae species richness was lower compared to other studies conducted in temperate regions. Schulz et al. (2015), for example, detected 70 cyanobacterial and non-diatom algal taxa in association with biocrusts in the coastal dunes of the Baltic Sea islands Rügen and Usedom. Common to both studies was the fact that green algae showed the highest species richness in biocrusts, which is well known from humid terrestrial habitats (Hoffmann, 1989; Glaser et al., 2018). Moreover, 76% (19 species) of the identified algal species composition of the present study is in agreement with those algae from biocrusts of Rügen and Usedom (Schulz et al., 2015; Mikhailyuk et al., 2019). Several rare and taxonomically interesting green algal species common for both studies were found: Tetracystis cf. sarcinalis, Tetradesmus arenicola [described from sand dunes of Germany and Ukraine (Mikhailyuk et al., 2019)], Eremochloris cf. sphaerica, Chlorokybus atmophyticus, and Interfilum cf. massjukiae. Nevertheless, representatives of the Chlorophyta were never the dominant algal group in all types of dunes studied, for several reasons. Representatives of the genus Klebsormidium (Charophyta) as well as the cyanobacterial genera Nostoc and Microcoleus tend to be the dominant species along both chronosequences. Klebsormidium exhibits a wide tolerance to temperature, irradiance, and water availability (Karsten and Rindi, 2010; Holzinger and Karsten, 2013), and their sticky filaments provide optimal traits to glue sand particles together. With this broad ecological tolerance and properties, Klebsormidium can easily cope with the harsh conditions in the dunes and coexist in competition with other cryptogamic species, such as mosses and lichens. Klebsormidium crenulatum is a typical soil crust-forming alga widespread at coastal sand dunes of the Baltic (Schulz et al., 2015) as well as the Black Sea (Mikhailyuk et al., 2021). Nostoc and Microcoleus are commonly found in the biocrusts of drylands (Lange and Belnap, 2016). One of Nostoc’s many survival strategies includes the production of exopolysaccharides, which leads to enhanced soil particle aggregation and the prevention of dune erosion (Büdel et al., 2016). This functional trait makes this genus particularly interesting, such as for coast protection measures. Further, Roncero Ramos et al. (2019) mentioned the potential benefits of Nostoc for reclaiming degraded soils by enriching them with organic carbon and nitrogen. Cyanobacteria generally play a crucial role in the enrichment of sandy sediment with nutrients, especially in this nitrogen-poor habitat (Langhans et al., 2009; Zhang et al., 2009). Nevertheless, it must be taken into account that the morphologically determined richness of algae species in enrichment cultures is lower than in nature. Since enrichment cultivation stimulates the growth of only culturable algae, information about the identity of other, often rare species is underrepresented (Langhans et al., 2009). Using molecular biological methods such as eDNA barcoding, it is assumed that more, but still not all species could be identified (Rippin et al., 2018).
Moss- and lichen-dominated biocrusts covered large parts of the later successional dune stages (Tables 6, 7). Leuschner and Ellenberg (2017) described the development of a cryptogamic layer as an indicator of the development of grey dunes. Hence, the cryptogams are not in danger of being buried by sand anymore. Furthermore, these authors assumed cryptogams to stabilize drifting sand grains and to accumulate and transfer organic matter into the underlying soil layers. Martínez and Maun (1999) showed a high tolerance of Ceratodon purpureus against burial by up to a 7 cm thick sand layer. Comparable to this, Gypser et al. (2016) identified C. purpureus as a characteristic species growing in the initial soils of postmining sites in Lower Lusatia, NE Germany. This is consistent with the fact that C. purpureus was the dominant moss species, particularly in the later stages of dune succession at Pramort and Darsser Ort. Hennediella heimii was found in the late grey dune of Darsser Ort and the mature dunes at Pramort. The distribution of this halophytic moss species is linked to the natural salinity gradient in coastal habitats. A recent study by Eldridge et al. (2023) provides a detailed insight into the contribution of soil mosses to ecosystem services, highlighting their value for carbon sequestration and soil, as well as P in soils globally.
The lichen community of the biocrusts at both study sites was dominated by species of the genus Cladonia. Those species prefer to grow in sands with acidophilic conditions and were therefore found mainly in the later successional stages of the dune landscape, where pH < 5.5 was measured. It can be assumed that Cladonia and other lichen species fulfill important ecological functions in the dune ecosystem. For instance, the lichens within the biocrusts might be involved in the accumulation of available P since the present study points to an enrichment of the labile and easily soluble P fraction at the expense of Ca-bound P (H2SO4 fraction) (Table 4). Jung et al. (2020) demonstrated the biogeochemical function of lichens within so-called grit crusts in the bioweathering of P-containing minerals of the underlying soil, since they found a significantly higher total P concentration in the second sediment centimeter (643 mg kg−1 average P concentration) than in the first one (211 mg kg−1 average P concentration). They interpreted this as P transfer through fungal hyphae that penetrated the material. Another functional trait of lichen-dominated biocrusts might be maintaining resistance to ecosystem invasion (e.g., non-native species or higher shrubs), as shown by Deines et al. (2007) and Serpe et al. (2008) in two shrub-steppes (Northwestern and Southeastern USA, respectively). However, this trait can also be weakened by environmental influences like nutrient enrichment. Even low to medium nitrogen deposition impacts the vegetation in acid coastal dunes of the Baltic Sea, promoting the dominance of taller graminoids (Remke et al., 2009), and, thus, habitats for lichens may decline. Further development of the lichen community on the dunes remains an interesting research topic for the future.
5 Synthesis
This study highlights the successional development of a diverse and changing cryptogamic community along two temperate dune chronosequences of the Baltic Sea coast in conjunction with initial soil formation processes. The results clearly point to a dune-type-dependent, specific phototrophic community composition. While mobile dune types, which initially only provide a small amount of nutrients, are dominated by thin microalgae-dominated biocrusts, older dunes are dominated by mosses and lichens. The processes of earliest soil development on Holocene deposits along the Baltic Sea coast include acidification and decalcification of the sediment. In addition, pedogenic Fe compounds and organic matter are accumulated, along with enrichments in bioavailable plant nutrients such as phosphates. More mature biocrusts accumulated more carbon and P than thinner microalgae-dominated biocrusts due to a higher biomass formation. With this initial phase of soil development, the assumed enrichment of easily soluble and therefore bioavailable P compounds, especially in the mature biocrusts and the adjacent underlying topsoil horizons, could be approved. In conclusion, all these data emphasize the important ecological functions of biocrust communities for dune habitats. Subsequent studies could build on this knowledge and examine, for example, their application in dune stabilization and coastal protection.
Data availability statement
The original contributions presented in the study are included in the article/Supplementary Material. Further inquiries can be directed to the corresponding author.
Author contributions
SK: Conceptualization, Data curation, Formal analysis, Investigation, Methodology, Visualization, Writing – original draft. PL: Conceptualization, Formal analysis, Methodology, Resources, Writing – review & editing. KG: Data curation, Writing – review & editing. US: Formal analysis, Investigation, Writing – review & editing. CD: Formal analysis, Writing – review & editing. TM: Formal analysis, Visualization, Writing – review & editing. ED: Formal analysis, Writing – review & editing. EH: Data curation, Formal analysis, Writing – review & editing. UK: Funding acquisition, Supervision, Writing – review & editing.
Funding
The author(s) declare financial support was received for the research, authorship, and/or publication of this article. This research was financially supported by the Leibniz Association within the scope of the Leibniz ScienceCampus Phosphorus Research Rostock (grant number W19/2018) and the University Rostock. We acknowledge financial support from the University of Rostock within the funding program Open Access Publishing.
Acknowledgments
The authors thank Britta Balz (University of Rostock, Soil Science) for her valuable technical assistance. Fieldwork permits were granted by the National Park Office, Vorpommersche Boddenlandschaft. The samples were collected under the research permit 32-5303.3. We thank Stephanie Puffpaff from the National Park Office, Department 32 - Research, Monitoring for good cooperation.
Conflict of interest
The authors declare that the research was conducted in the absence of any commercial or financial relationships that could be construed as a potential conflict of interest.
The reviewer ML declared a past co-authorship with the author UK to the handling editor.
Publisher’s note
All claims expressed in this article are solely those of the authors and do not necessarily represent those of their affiliated organizations, or those of the publisher, the editors and the reviewers. Any product that may be evaluated in this article, or claim that may be made by its manufacturer, is not guaranteed or endorsed by the publisher.
Supplementary material
The Supplementary Material for this article can be found online at: https://www.frontiersin.org/articles/10.3389/fevo.2023.1266209/full#supplementary-material
References
Ad-hoc Arbeitsgruppe Boden (2005). Bodenkundliche Kartieranleitung. 5th (Stuttgart: E. Schweitzerbart’sche Verlagsbuchhandlung).
Ampe C., Langohr R. (2003). Morphological characterisation of humus forms in recent coastal dune ecosystems in Belgium and northern France. CATENA 54 (3), 363–383. doi: 10.1016/S0341-8162(03)00128-0
Barger N. N., Weber B., Garcia-Pichel F., Zaady E., Belnap J. (2016). “Patterns and controls on nitrogen cycling of biological soil crust,” in Biological soil crusts: An organizing principle in drylands. Eds. Weber B., Büdel B., Belnap J. (Cham (ZG): Springer International Publishing), 257–285.
Baumann K., Glaser K., Mutz J. E., Karsten U., MacLennan A., Hu Y., et al. (2017). Biological soil crusts of temperate forests: Their role in P cycling. Soil Biol. Biochem. 109, 156–166. doi: 10.1016/j.soilbio.2017.02.011
Baumann K., Siebers M., Kruse J., Eckhardt K. U., Hu Y., Michalik D., et al. (2019). Biological soil crusts as key player in biogeochemical P cycling during pedogenesis of sandy substrate. Geoderma. 338, 145–158. doi: 10.1016/j.geoderma.2018.11.034
Belnap J. (2006). The potential roles of biological soil crusts in dryland hydrologic cycles. Hydrological Processes. 20 (15), 3159–3178. doi: 10.1002/hyp.6325
Belnap J. (2011). “Biological phosphorus cycling in dryland regions,” in Phosphorus in Action. Eds. Bünemann E. K., Oberson A., Frossard E. (Berlin, Heidelberg: Springer-Verlag), 371–406.
Belnap J., Büdel B. (2016). “Biological soil crusts as soil stabilizers,” in Biological soil crusts: an organizing principle in drylands. Eds. Weber B., Büdel B., Belnap J. (Cham (ZG): Springer International Publishing), 305–320.
Belnap J., Büdel B., Lange O. L. (2001). “Biological soil crusts: characteristics and distribution,” in Biological soil crusts: structure, function, and management. Eds. Belnap J., Lange O. L. (Berlin, Heidelberg: Springer-Verlag), 3–30. doi: 10.1007/978-3-642-56475-8_1
Berthold M., Wulff R., Reiff V., Karsten U., Nausch G., Schumann R. (2019). Magnitude and influence of atmospheric phosphorus deposition on the southern Baltic Sea coast over 23 years: implications for coastal waters. Environ. Sci. Europe. 31 (27):1–11. doi: 10.1186/s12302-019-0208-y
Bischoff H. W., Bold H. C. (1963). Some soil algae from Enchanted Rock and related algal species. (Austin, Texas: University of Texas).
Blume H.-P., Stahr K., Leinweber P. (2010). “Laboruntersuchungen,” in Bodenkundliches Praktikum. (Heidelberg: Spektrum Akademischer Verlag), 77–154. doi: 10.1007/978-3-8274-2733-5_5
Bu C., Li R., Wang C., Bowker M. A. (2018). Successful field cultivation of moss biocrusts on disturbed soil surfaces in the short term. Plant Soil 429 (1–2), 227–240. doi: 10.1007/s11104-017-3453-0
Büdel B., Darienko T., Deutschewitz K., Dojani S., Friedl T., Mohr K. I., et al. (2009). Southern african biological soil crusts are ubiquitous and highly diverse in drylands, being restricted by rainfall frequency. Microbial Ecol. 57 (2), 229–247. doi: 10.1007/s00248-008-9449-9
Büdel B., Dulic T., Darienko T., Rybalka N., Friedl T. (2016). “Cyanobacteria and algae of biological soil crusts,” in Biological soil crusts: an organizing principle in drylands. Eds. Weber B., Büdel B., Belnap J. (Cham (ZG): Springer International Publishing), 55–80.
Castillo-Monroy A. P., Maestre F. T., Delgado-Baquerizo M., Gallardo A. (2010). Biological soil crusts modulate nitrogen availability in semi-arid ecosystems: Insights from a Mediterranean grassland. Plant Soil 333 (1), 21–34. doi: 10.1007/s11104-009-0276-7
Chamizo S., Cantón Y., Miralles I., Domingo F. (2012). Biological soil crust development affects physicochemical characteristics of soil surface in semiarid ecosystems. Soil Biol. Biochem. 49, 96–105. doi: 10.1016/j.soilbio.2012.02.017
Chamizo S., Cantón Y., Rodríguez-Caballero E., Domingo F. (2016). Biocrusts positively affect the soil water balance in semiarid ecosystems. Ecohydrology. 9 (7), 1208–1221. doi: 10.1002/eco.1719
Clements F. E. (1916). Plant Succession: An analysis of the development of vegetation. (Washington: Carnegie Institution of Washington). doi: 10.5962/bhl.title.56234
Cornell R. M., Schwertmann U. (2003). The iron oxides: structure, properties, reactions, occurences and uses. 2nd (Weinheim: Wiley-VCH). doi: 10.1002/3527602097
Culberson C. F., Ammann K. (1979). Standardmethode zur Dünnschichtchromatographie von Flechtensubstanzen. Herzogia 5, 1–24. doi: 10.1127/herzogia/5/1979/1
Daumantas L., Šinkūnas P., Rudnickaitė E., Dobrotin N., Kisieliene D., Spiridonov A. (2022). Late Pleistocene to Middle Holocene record of sedimentation and carbonate content in the Zervynos paleolake-dune complex, Lithuania. Estonian J. Earth Sci. 71, 214. doi: 10.3176/earth.2022.15
Deines L., Rosentreter R., Eldridge D. J., Serpe M. D. (2007). Germination and seedling establishment of two annual grasses on lichen-dominated biological soil crusts. Plant Soil 295 (1), 23–35. doi: 10.1007/s11104-007-9256-y
Dümig A., Veste M., Hagedorn F., Fischer T., Lange P., Spröte R., et al. (2014). Organic matter from biological soil crusts induces the initial formation of sandy temperate soils. Catena. 122, 196–208. doi: 10.1016/j.catena.2014.06.011
Earle S. (2019). “Weathering and the formation of soil,” in Physical geology, 2nd (Victoria, B.C: BCcampus). Available at: https://opentextbc.ca/geology/.
Elbert W., Weber B., Burrows S., Steinkamp J., Büdel B., Andreae M. O., et al. (2012). Contribution of cryptogamic covers to the global cycles of carbon and nitrogen. Nat. Geosci. 5 (7), 459–462. doi: 10.1038/ngeo1486
Eldridge D. J., Greene R. S. B. (1994). Microbiotic soil crusts: A review of their roles in soil and ecological processes in the rangelands of Australia. Aust. J. Soil Res. 32 (3), 389–415. doi: 10.1071/SR9940389
Eldridge D. J., Guirado E., Reich P. B., Ochoa-Hueso R., Berdugo M., Sáez-Sandino T., et al. (2023). The global contribution of soil mosses to ecosystem services. Nat. Geosci. 16, 430–438. doi: 10.1038/s41561-023-01170-x
Ettl H., Gärtner G. (2014). Syllabus der Boden-, Luft- und Flechtenalgen. 2nd (Berlin, Heidelberg: Springer Spektrum). doi: 10.1007/978-3-642-39462-1
Felde V. J. M. N. L., Chamizo S., Felix-Henningsen P., Drahorad S. L. (2018). What stabilizes biological soil crusts in the Negev Desert? Plant Soil. 429, 9–18. doi: 10.1007/s11104-017-3459-7
Fischer T., Veste M., Schaaf W., Dümig A., Kögel-Knabner I., Wiehe W., et al. (2010). Initial pedogenesis in a topsoil crust 3 years after construction of an artificial catchment in Brandenburg, NE Germany. Biogeochemistry 101 (1), 165–176. doi: 10.1007/s10533-010-9464-z
Fischer T., Subbotina M. (2014). Climatic and soil texture threshold values for cryptogamic cover development: a meta analysis. Biologia 69 (11), 1520–1530. doi: 10.2478/s11756-014-0464-7
Fischer T., Yair A., Veste M. (2012). Infiltration, water holding capacity and growth patterns of biological soil crusts on sand dunes under arid and temperate climates. Geophysical Res. Abstracts 14, 5034.
Fox T. R. (1995). “The influence of low-molecular-weight organic acids on properties and processes in forest soils,” in Carbon forms and functions in forest soils. Eds. McFee W. W., Kelly J. M. (Madison, WI: Soil Science of America), 43–61.
Fučíková K., Lewis P., Lewis L. (2014). Widespread desert affiliation of trebouxiophycean algae (Trebouxiophyceae, Chlorophyta) including discovery of three new desert genera. Phycological Res. 62 (4), 294–305. doi: 10.1111/pre.12062
García Novo F., Díaz Barradas M. C., Zunzunegui M., García Mora R., Gallego Fernández J. B. (2004). “Plant functional types in coastal dune habitats,” in Coastal dunes: ecology and conservation. Eds. Martínez M. L., Putsy N. P. (Berlin Heidelberg: Springer-Verlag), 155–169. doi: 10.1007/978-3-540-74002-5
Garcia-Pichel F., Loza V., Marusenko Y., Mateo P., Potrafka R. M. (2013). Temperature drives the continental-scale distribution of key microbes in topsoil communities. Science 340, 1574–1577. doi: 10.1126/science.1236404
Giani L., Buhmann S. (2004). Entwicklung, Eigenschaften und Klassifikation von Dünenböden der Ostfriesischen Inseln - Am Beispiel Spiekeroogs. J. Plant Nutr. Soil Sci. 167 (5), 645–648. doi: 10.1002/jpln.200421394
Glaser K., Baumann K., Leinweber P., Mikhailyuk T., Karsten U. (2018). Algal richness in BSCs in forests under different management intensity with some implications for P cycling. Biogeosciences. Copernicus GmbH 15 (13), 4181–4192. doi: 10.5194/bg-15-4181-2018
Guiry M. D., Guiry G. M. (2023). AlgaeBase. (Galway: World-Wide Electronic Publication, National University of Ireland).
Gypser S., Veste M., Fischer T., Lange P. (2016). Infiltration and water retention of biological soil crusts on soils of former open-cast lignite mining sites in Brandenburg, north-east Germany. J. Hydrol. Hydromech. 64 (1), 1–11. doi: 10.1515/johh-2016-0009
Hedley M. J., Stewart J. W. B., Chauhan B. S. (1982). Changes in inorganic and organic soil phosphorus fractions induced by cultivation practices and by laboratory incubations. Soil Sci. Soc. America J. 46 (5), 970–976. doi: 10.2136/sssaj1982.03615995004600050017x
Hesp P. A. (1991). Ecological processes and plant adaptations on coastal dunes. J. Arid Environ. 21, 165–191. doi: 10.1016/S0140-1963(18)30681-5
Hodgetts N. G., Söderström L., Blockeel T. L., Caspari S., Ignatov M. S., Konstantinova N. A., et al. (2020). An annotated checklist of bryophytes of Europe, Macaronesia and Cyprus. J. Bryology. 42 (1), 1–116. doi: 10.1080/03736687.2019.1694329
Hoffmann L. (1989). Algae of terrestrial habitats. Bot. Rev. 55 (2), 77–105. doi: 10.1007/BF02858529
Holzinger A., Karsten U. (2013). Desiccation stress and tolerance in green algae: Consequences for ultrastructure, physiological, and molecular mechanisms. Front. Plant Sci. 4. doi: 10.3389/fpls.2013.00327
Hundt R. (1985). Phytosociological and ecological aspects of the dunes on the isle of Rügen, Baltic Sea. Vegetatio 61 (1/3), 97–103. doi: 10.1007/BF00039814
Isermann M. (2005). Soil pH and species diversity in coastal dunes. Plant Ecol. 178 (1), 111–120. doi: 10.1007/s11258-004-2558-8
Isermann M. (2011). Patterns in species diversity during succession of coastal dunes. J. Coast. Res. 27 (4), 661–671. doi: 10.2112/JCOASTRES-D-09-00040.1
Janke W., Lampe R. (1998). Development of the Fischland-Darss-Zingst spit and its surrounding area since the Litorina transgression and the reconstruction of its subrecent dynamics using historical maps. Z. für Geomorphologie Supplementband 112, 177–194.
Jung P., Baumann K., Emrich D., Springer A., Felde V. J. M. N. L., Dultz S., et al. (2020). Lichens bite the dust – A bioweathering scenario in the Atacama desert. iScience. 23 (11):1–17. doi: 10.1016/j.isci.2020.101647
Jurasinski G., Buczko U. (2023). “Environmental conditions at the coast: shoreline ecosystems,” in Southern Baltic coastal systems analysis. Eds. Schubert H., Müller F. (Cham: Springer International Publishing), 71–80. doi: 10.1007/978-3-031-13682-5_6
Kammann S., Schiefelbein U., Dolnik C., Mikhailyuk T., Demchenko E., Karsten U., et al. (2023). Successional development of the phototrophic community in biological soil crusts on coastal and inland dunes. MDPI Biol. 12 (58):1–26. doi: 10.3390/biology12010058
Karsten U., Baudler H., Himmel B., Jaskulke R., Ewald H., Schumann R. (2012). Short-term measurements of exposure and inundation of sediment areas in a tide-less wind flat system at the Southern Baltic Sea coast. J. Mar. Syst. 105, 187–193. doi: 10.1016/j.jmarsys.2012.08.004
Karsten U., Rindi F. (2010). Ecophysiological performance of an urban strain of the aeroterrestrial green alga Klebsormidium sp. (Klebsormidiales, Klebsormidiophyceae). Eur. J. Phycology 45 (4), 426–435. doi: 10.1080/09670262.2010.498587
Kidron G., Vonshak A., Dor I., Barinova S., Abeliovich A. (2010). Properties and spatial distribution of microbiotic crusts in the Negev Desert, Israel. Catena 82, 92–101. doi: 10.1016/j.catena.2010.05.006
Komárek J. (2013). “Cyanoprokaryota. 3rd pt: Heterocytous genera,” in Süßwasserflora von Mitteleuropa, vol. 19/3 . Eds. Büdel B., Gärtner G., Krienitz L., Schagerl M. Berlin, Heidelberg: Elsevier. 1130 p.
Komárek J., Anagnostidis K. (2005). “Cyanoprokaryota. Oscillatoriales,” in Süsswasserflora von mitteleuropa. Bd, vol. 19 . Ed. Büdel B., Gärtner G., Krienitz L., Schagerl M. München: Elsevier Spectrum. 759 p.
Kurth J. K., Albrecht M., Karsten U., Glaser K., Schloter M., Schulz S. (2021). Correlation of the abundance of bacteria catalyzing phosphorus and nitrogen turnover in biological soil crusts of temperate forests of Germany. Biol. Fertility Soils 57 (2), 179–192. doi: 10.1007/s00374-020-01515-3
Kuzyakov Y. (2006). Sources of CO2 efflux from soil and review of partitioning methods. Soil Biol. Biochem. 38 (3), 425–448. doi: 10.1016/j.soilbio.2005.08.020
Lampe R. (2002). “Post-glacial water-level variability along the south Baltic coast – a short overview,” in Greifswalder geographische Arbeiten: Holocene evolution of the south-western Baltic coast - geologiacal, archaeological and palaeo-environmental aspects. Ed. Lampe R. Ernst-Moritz-Arndt-Univ. Greifswald, Volume 27, 224. doi: 10.23689/fidgeo-1957
Lampe R., Niedermeyer R.-O., Werner F., Schwarzer K. (2011). “Die heutige Ostsee,” in Die deutsche Ostseeküste, 2nd. Ed. Niedermeyer R.-O., et al. (Stuttgart, Germany: Schweizerbart Science Publishers), 370. Available at: http://www.schweizerbart.de//publications/detail/isbn/9783443150914/Bd%5C_105%5C_Sammlung%5C_geol%5C_Fuhrer%5C_Die%5C_deuts.
Lan S., Wu L., Zhang D., Hu C. (2012). Successional stages of biological soil crusts and their microstructure variability in Shapotou region (China). Environ. Earth Sci. 65 (1), 77–88. doi: 10.1007/s12665-011-1066-0
Lange O. L., Belnap J. (2016). “How biological soil crusts became recognized as a functional unit: a selective history,” in Biological soil crusts: An organizing principle in drylands. Eds. Weber B., Büdel B., Belnap J. (Cham (ZG): Springer International Publishing), 15–33.
Langhans T. M., Storm C., Schwabe A. (2009). Community assembly of biological soil crusts of different successional stages in a temperate sand ecosystem, as assessed by direct determination and enrichment techniques. Microbial Ecol. 58 (2), 394–407. doi: 10.1007/s00248-009-9532-x
Langhans T. M., Storm C., Schwabe A. (2010). Regeneration processes of biological soil crusts, macro-cryptogams and vascular plant species after fine-scale disturbance in a temperate region: Recolonization or successional replacement? Flora: Morphology, Distribution, Funct. Ecol. Plants. Elsevier 205 (1), 46–60. doi: 10.1016/j.flora.2008.12.001
Lemke W. (1998) Sedimentation und paläogeographische Entwicklung im westlichen Ostseeraum (Mecklenburger Bucht bis Arkonabecken) vom Ende der Weichselvereisung bis zur Litorinatransgression. Available at: https://www.io-warnemuende.de/tl_files/forschung/meereswissenschaftliche-berichte/mebe31_1998_lemke.pdf.
Leuschner C., Ellenberg H. (2017). “Sand dunes and their vegetation series,” in Ecology of central european non-forest vegetation: coastal to alpine, natural to man-made habitats: vegetation ecology of central Europe, vol. II. (Cham: Springer International Publishing), 63–115. doi: 10.1007/978-3-319-43048-5_2
Li Y., Hu C. (2021). Biogeographical patterns and mechanisms of microbial community assembly that underlie successional biocrusts across northern China. NPJ Biofilms Microbiomes. 7 (15):1–11. doi: 10.1038/s41522-021-00188-6
Lokhorst G. M. (1996). Comparative taxonomic studies on the genus Klebsormidium (Charophyceae) in Europe. 5th. Ed. Jülich W. (Stuttgart: Gustav Fischer).
Martin W. E. (1959). The vegetation of island beach state park, New Jersey. Ecol. Monogr. 29 (1), 1–46. doi: 10.2307/1948540
Martínez M. L., Maun M. A. (1999). Responses of dune mosses to experimental burial by sand under natural and greenhouse conditions. Plant Ecol. 145, 209–219. doi: 10.1023/A:1009850304137
Martínez M. L., Psuty N. N., Lubke R. A. (2004). “A perspective on coastal dunes,” in Coastal dunes: ecology and conservation., vol. 171 . Eds. Martínez M. L., Psuty N. P. (Berlin Heidelberg: Springer-Verlag), 3–10.
Martínez M. L., Vázquez G., Sánchez Colón S. (2001). Species composition and sand movement during primary succession on tropical dunes. J. Vegetation Sci. 12, 361–372. doi: 10.2307/3236850
Maun M. A. (2009). “The sand dune environment,” in The biology of coastal sand dunes. Ed. Maun M. A. (Oxford: Oxford University Press), 23–40.
Mehra O. P., Jackson M. L. (1958). Iron oxide removal from soils and clays by a dithionite-citrate system buffered with sodium bicarbonate. Clays Clay Minerals 7 (1), 317–327. doi: 10.1346/CCMN.1958.0070122
Mikhailyuk T., Glaser K., Tsarenko P., Demchenko E., Karsten U. (2019). Composition of biological soil crusts from sand dunes of the Baltic Sea coast in the context of an integrative approach to the taxonomy of microalgae and cyanobacteria. Eur. J. Phycology. Taylor Francis 54 (3), 1–28. doi: 10.1080/09670262.2018.1557257
Mikhailyuk T., Vinogradova O., Glaser K., Rybalka N., Demchenko E., Karsten U. (2021). Algae of biological soil crusts from sand dunes of the Danube delta biosphere reserve (Odesa region, Ukraine). Int. J. Algae 23 (1), 7–42. doi: 10.1615/InterJAlgae.v23.i1.20
Miller T. E., Gornish E. S., Buckley H. L. (2010). Climate and coastal dune vegetation: Disturbance, recovery, and succession. Plant Ecology. Springer Netherlands 206 (1), 97–104. doi: 10.1007/s11258-009-9626-z
Müller F., Bicking S., Ahrendt K., Sterr H. (2023). “Environmental conditions at the coast: the terrestrial ecosystems,” in Southern Baltic coastal systems analysis. Eds. Schubert H., Müller F. (Cham: Springer International Publishing), 49–69. doi: 10.1007/978-3-031-13682-5_5
Murphy J., Riley J. P. (1962). A modified single solution method for the determination of phosphate in natural waters. Analytica Chimica Acta 27, 31–36. doi: 10.1016/S0003-2670(00)88444-5
Niedermeyer R.-O., Lampe R., Kliewe H., Schwarzer K. (2011). “Die Küste der südwestlichen Ostsee,” in Die deutsche Ostseeküste., 2nd. Ed. Niedermeyer R.-O., Lampe R., Janke W., Schwarzer K., Duphorn K., Kliewe H., et al. (Stuttgart, Germany: Schweizerbart Science Publishers), 370. Available at: http://www.schweizerbart.de//publications/detail/isbn/9783443150914/Bd%5C_105%5C_Sammlung%5C_geol%5C_Fuhrer%5C_Die%5C_deuts.
Niedermeyer R.-O., Janke W. (2011). “Geologische Entwicklung im Pleistozän,” in Die deutsche Ostseeküste., 2nd. Ed. Niedermeyer R.-O., Lampe R., Janke W., Schwarzer K., Duphorn K., Kliewe H., et al. (Stuttgart, Germany: Schweizerbart Science Publishers), 370. Available at: http://www.schweizerbart.de//publications/detail/isbn/9783443150914/Bd%5C_105%5C_Sammlung%5C_geol%5C_Fuhrer%5C_Die%5C_deuts.
Oksanen J., Blanchet F. G., Friendly M., Kindt R., Legendre P., McGlinn D., et al. (2018). vegan: Community Ecology Package. Ordination methods, diversity analysis and other functions for community and vegetation ecologists. Version 2.5-1. (Comprehensive R Archive Network). Available at: https://CRAN.R-project.org/package=vegan.
Pickett S. T. A., McDonnell M. J. (1989). Changing perspectives in community dynamics: A theory of successional forces. Trends Ecol. Evol. 4 (8), 241–245. doi: 10.1016/0169-5347(89)90170-5
Pollmann T., Tsukamoto S., Frechen M., Giani L. (2020). Rate of soil formation in Arenosols of dunes on Spiekeroog Island (Germany). Geoderma Regional 20, e00246. doi: 10.1016/j.geodrs.2019.e00246
Pollmann T., Junge B., Giani L. (2018). Landscapes and soils of North Sea barrier islands: A comparative analysis of the old west and young east of Spiekeroog Island (Germany). Erdkunde 4, 273–286. doi: 10.3112/erdkunde.2018.04.02
Printzen C., von Brackel W., Bültmann H., Cezanne R., Dolnik C., Dornes P., et al. (2022). Die Flechten, flechtenbewohnenden und flechtenähnlichen Pilze Deutschlands – eine überarbeitete Checkliste. Herzogia 35 (1), 193–393. doi: 10.13158/heia.35.1.2022.193
Pushkareva E., Elster J., Holzinger A., Niedzwiedz S., Becker B. (2022). Biocrusts from Iceland and Svalbard: Does microbial community composition differ substantially? Front. Microbiol. 13. doi: 10.3389/fmicb.2022.1048522
R Core Team. (2022). R: A language and environment for statistical computing. R Foundation for Statistical Computing, Vienna, Austria. URL https://www.R-project.org/.
Ranwell D. (1960). Newborough Warren, Anglesey. II. Plant associes and succession cycles of the sand dune and dune slack vegetation. J. Ecol. 48, 117–142. doi: 10.2307/2257311
Reimann T., Naumann M., Tsukamoto S., Frechen M. (2010). Luminescence dating of coastal sediments from the Baltic Sea coastal barrier-spit Darss-Zingst, NE Germany. Geomorphology. 122 (3–4), 264–273. doi: 10.1016/j.geomorph.2010.03.001
Remke E., Brouwer E., Kooijman A., Blindow I., Esselink H., Roelofs J. G. M. (2009). Even low to medium nitrogen deposition impacts vegetation of dry, coastal dunes around the Baltic Sea. Environ. pollut. (Barking Essex : 1987) 157 (3), 792–800. doi: 10.1016/j.envpol.2008.11.020
Reynolds R., Belnap J., Reheis M., Lamothe P., Luiszer F. (2001). Aeolian dust in Colorado Plateau soils : Nutrient inputs and recent change in source. Proc. Natl. Acad. Sci. United States America 98 (July), 7123–7127. doi: 10.1073/pnas.121094298
Richardson A. E., Simpson R. J. (2011). Soil microorganisms mediating phosphorus availability. Plant Physiol. 156 (3), 989–996. doi: 10.1104/pp.111.175448
Rippin M., Borchhardt N., Williams L., Colesie C., Jung P., Büdel B., et al. (2018). Genus richness of microalgae and Cyanobacteria in biological soil crusts from Svalbard and Livingston Island: morphological versus molecular approaches. Polar Biol. 41 (5), 909–923. doi: 10.1007/s00300-018-2252-2
Ritchie R. J. (2008). Universal chlorophyll equations for estimating chlorophylls a, b, c, and d and total chlorophylls in natural assemblages of photosynthetic organisms using acetone, methanol, or ethanol solvents. PHOTOSYNTHETICA 46 (1), 115–126. doi: 10.1007/s11099-008-0019-7
Roncero Ramos B., Román R., Gómez-Serrano C., Cantón Y., Acien G. (2019). Production of a biocrust-cyanobacteria strain (Nostoc commune) for large-scale restoration of dryland soils. J. Appl. Phycology 31 (4), 2217–2230. doi: 10.1007/s10811-019-1749-6
Salisbury E. J. (1952). Downs & dunes : their plant life and its environment. G. Bell, London 1–328.
Samolov E., Baumann K., Büdel B., Jung P., Leinweber P., Mikhailyuk T., et al. (2020). Biodiversity of algae and cyanobacteria in biological soil crusts collected along a climatic gradient in Chile using an integrative approach. Microorganisms 8 (7), 1047. doi: 10.3390/microorganisms8071047
Sancho L. G., Belnap J., Colesie C., Raggio J., Weber B. (2016). “Carbon budgets of biological soil crusts at micro-, meso-, and global scales,” in Biological soil crusts: An organizing principle in drylands. Eds. Weber B., Büdel B., Belnap J. (Cham (ZG): Springer International Publishing), 287–304.
Schaub I., Baum C., Schumann R., Karsten U. (2019). Effects of an early successional biological soil crust from a temperate coastal sand dune (NE Germany) on soil elemental stoichiometry and phosphatase activity. Microbial Ecology. 77 (1), 217–229. doi: 10.1007/s00248-018-1220-2
Schulz K., Mikhailyuk T., Dreßler M., Leinweber P., Karsten U. (2015). Biological soil crusts from coastal dunes at the Baltic Sea: cyanobacterial and algal biodiversity and related soil properties. Microbial Ecology. 71 (1), 178–193. doi: 10.1007/s00248-015-0691-7
Schumacher W. (2002). “Coastal evoloution of the Darss peninsula,” in Greifswalder geographische Arbeiten: Holocene evolution of the south-western Baltic coast - geologiacal, archaeological and palaeo-environmental aspects. (Ernst-Moritz-Arndt-University, Greifswald) 27, 165–168.
Schumacher W., Bayerl K.-A. (1999). The shoreline displacement curve of Rügen Island (Southern Baltic Sea). Quaternary Int. 56 (1), 107–113. doi: 10.1016/S1040-6182(98)00027-5
Schwertmann U. (1964). Differenzierung der Eisenoxide des Bodens durch Extraktion mit Ammoniumoxalat-Lösung. Z. für Pflanzenernährung Düngung Bodenkunde 105 (3), 194–202. doi: 10.1002/jpln.3591050303
Serpe M. D., Zimmerman S. J., Deines L., Rosentreter R. (2008). Seed water status and root tip characteristics of two annual grasses on lichen-dominated biological soil crusts. Plant Soil 303 (1), 191–205. doi: 10.1007/s11104-007-9498-8
Sims J. T., Pierzynski G. M. (2005). “Chemistry of phosphorus in soils,” in Chemical processes in soils. SSSA book (Madison, WI, USA: Soil Science Society of America), 151–192.
Stevens P. R., Walker T. W. (1970). The Chronosequence Concept and soil formation. Q. Rev. Biol. 45 (4), 333–350. http://www.journals.uchicago.edu/t-and-c. doi: 10.1086/406646
Sun F., Xiao B., Kidron G. J. (2022). Towards the influences of three types of biocrusts on soil water in drylands: Insights from horizontal infiltration and soil water retention. Geoderma 428, 116136. doi: 10.1016/j.geoderma.2022.116136
Tiepolt L., Schumacher W. (1999). Historische bis rezente Küstenveranderungen im Raum Fischland-Darss-Zingst-Hiddensee anhand von Karten, Luft- und Satellitenbildern. Die Küste 61), 21–46.
Veste M., Breckle S.-W., Eggert K., Littmann T. (2011). Vegetation pattern in arid sand dunes controlled by biological soil crusts along a climatic gradient in the Northern Negev desert. Basic Appl. Dryland Res. 5 (1), 1–16. doi: 10.1127/badr/5/2011/1
Veste M., Littmann T. (2006). Dewfall and its geo-ecological implication for biological surface crusts in desert sand dunes (North-western Negev, Israel). J. Arid Land Stud. 16 (3), 139–147.
Walker L. R., Wardle D. A., Bardgett R. D., Clarkson B. D. (2010). The use of chronosequences in studies of ecological succession and soil development. J. Ecol. 98 (4), 725–736. doi: 10.1111/j.1365-2745.2010.01664.x
Walker T. W., Syers J. K. (1976). The fate of phosphorus during pedogenesis. Geoderma 15 (1), 1–19. doi: 10.1016/0016-7061(76)90066-5
Williams A. J., Buck B. J., Beyene M. A. (2012). Biological soil crusts in the Mojave desert, USA: micromorphology and pedogenesis. Soil Sci. Soc. America J. 76 (5), 1685–1695. doi: 10.2136/sssaj2012.0021
Williams L., Borchhardt N., Colesie C., Baum C., Komsic-Buchmann K., Rippin M., et al. (2017). Biological soil crusts of Arctic Svalbard and of Livingston Island, Antarctica. Polar Biol. 40 (2), 399–411. doi: 10.1007/s00300-016-1967-1
Young K. E., Ferrenberg S., Reibold R., Reed S. C., Swenson T., Northen T., et al. (2022). Vertical movement of soluble carbon and nutrients from biocrusts to subsurface mineral soils. Geoderma. 405 (115495). doi: 10.1016/j.geoderma.2021.115495
Zaady E., Kuhn U., Wilske B., Sandoval-Soto L., Kesselmeier J. (2000). Patterns of CO2 exchange in biological soil crusts of successional age. Soil Biol. Biochem. 32 (7), 959–966. doi: 10.1016/S0038-0717(00)00004-3
Zaady E., Katra I., Yizhaq H., Kinast S., Ashkenazy Y. (2014). Inferring the impact of rainfall gradient on biocrusts’ developmental stage and thus on soil physical structures in sand dunes. Aeolian Res. 13, 81–89. doi: 10.1016/j.aeolia.2014.04.002
Zhang B., Zhang Y., Zhao J., Wu N., Chen R., Zhang J. (2009). Microalgal species variation at different successional stages in biological soil crusts of the Gurbantunggut Desert, Northwestern China. Biol. Fertility Soils 45 (5), 539–547. doi: 10.1007/s00374-009-0364-0
Keywords: biocrusts, chronosequence, dunes, phosphorus, phototrophic diversity, soil development
Citation: Kammann S, Leinweber P, Glaser K, Schiefelbein U, Dolnik C, Mikhailyuk T, Demchenko E, Heilmann E and Karsten U (2024) Successional development of the phototrophic community in biological soil crusts, along with soil formation on Holocene deposits at the Baltic Sea coast. Front. Ecol. Evol. 11:1266209. doi: 10.3389/fevo.2023.1266209
Received: 01 August 2023; Accepted: 18 December 2023;
Published: 18 January 2024.
Edited by:
Lynne Beaty, Penn State Erie, The Behrend College, United StatesReviewed by:
Josef Elster, University of South Bohemia in České Budějovice, CzechiaMichael Lakatos, Hochschule Kaiserslautern University of Applied Sciences, Germany
Copyright © 2024 Kammann, Leinweber, Glaser, Schiefelbein, Dolnik, Mikhailyuk, Demchenko, Heilmann and Karsten. This is an open-access article distributed under the terms of the Creative Commons Attribution License (CC BY). The use, distribution or reproduction in other forums is permitted, provided the original author(s) and the copyright owner(s) are credited and that the original publication in this journal is cited, in accordance with accepted academic practice. No use, distribution or reproduction is permitted which does not comply with these terms.
*Correspondence: Sandra Kammann, c2FuZHJhLmthbW1hbm5AdW5pLXJvc3RvY2suZGU=
†ORCID: Sandra Kammann, orcid.org/0000-0002-8032-3648
Karin Glaser, orcid.org/0000-0002-5962-3603