- Laboratório de Embriologia Molecular e Câncer, Federal University of Santa Catarina, Florianópolis, SC, Brazil
The formation of the innate immune system of animals can only be envisioned after the development of the first metazoan embryo. The decisive role of Embryology in understanding the evolution of the immune system has been inexplicably disregarded in the history of science. Some characteristics of our holozoan ancestors, including macrophage-like movement and enteric phagocytosis, were suppressed by the formation of chains of physically attached cells in the context of embryo multicellularity. The formation of the archenteron during morphogenesis of the first embryo resulted in a meta-organism whose survival was dependent on the ability to perform enteric phagocytosis (nutrition on bacteria). By recognizing the neoplastic basis of embryo formation, it is possible to venture a glimpse at its other face, a process that becomes evident when the extracellular matrix and cadherin junctions are destroyed. What ensues is metastasis (in the case of cancer) or an alternative version controlled by cell differentiation (during embryogenesis). In the context of innate immunity, the development of mesogleal cells by epithelial–mesenchymal transition and differentiation into cells specialized in bacterial recognition allowed the newly formed animal to preserve homeostasis, an innovation that has been maintained throughout evolution. In this article, I will share my first reflections on the embryonic origin of innate immunity and its close relationship with cancer. Innate immunity arises naturally during embryogenesis, which explains why the immune system typically does not react against cancer cells. In its essence, the immune system was created from them. Here, I argue that the first embryo can be understood as a benign tumor nourished and protected by the innate immune system.
Introduction
The embryo conceived as a benign tumor nourished and protected by macrophages
Innate immunity is common to all multicellular organisms. The innate immune system acts by producing responses to microbes recognized as exogenous through the detection of highly conserved antigens (Haney et al., 2014; Cao and Kagan, 2023). The evolution of the innate immune system, and of macrophages in particular, has been thought of as a functional repertoire brought on from the unicellular ancestors of animals. The system is believed to have expanded with the evolution of multicellularity and the increasing complexity of tissues and organic systems (Bajgar and Krejčová, 2023). What is undeniable is the lack of any embryological basis in discussions of the origin and evolution of the immune system in a multicellular context.
Another neglected aspect when discussing multicellularity and metazoan emergence is that the multicellular organization of animals could not have evolved without the formation of the first embryo (Cofre and Saalfeld, 2023). In this context, the innate immune system emerges and evolves linked to embryonic development. In this article, I discuss the idea that some of the characteristics of holozoan precursors, including amoeboid movement (macrophage-like movement) and phagocytosis (enteric phagocyte), were initially repressed by the formation of chains of physically attached cells in the creation of the first multicellular embryo. However, these repressed characteristics reappear in the embryonic context when the bonds that connect cells during morphogenesis are broken in a process known as epithelial–mesenchymal transition (EMT). This moment of evolutionary history is of great importance, being considered as one of the points of convergence (commonplace) between clinical pathologists/oncologists and embryologists, as evidenced by the enormous similarity between gastrulation and metastasis (Thiery and Sleeman, 2006; Kalluri and Weinberg, 2009; Micalizzi et al., 2010; Nakaya and Sheng, 2013; Ribatti et al., 2020).
In this article, I also discuss the idea that the evolutionary engine of metazoans is a Neoplastic force (Cofre, 2024); therefore, I will try to demonstrate how cancer (also a biological process) is intertwined with the emergence of the innate immune system during embryonic development. With the destruction of the extracellular matrix (ECM) and separation of cadherin junctions during embryo morphogenesis, a controlled metastasis was generated. This allowed cells to be projected and differentiated into the mesoglea by EMT, with the new animal having specialized functions of bacterial recognition and homeostasis protection. This innovation was maintained and enriched throughout animal evolution.
Thus, I propose in this article that the first embryo can be regarded as a benign tumor protected and nourished by the innate immune system. Embryological and neoplastic perspectives on the emergence of the innate immune system may elucidate some of the behaviors of macrophages that seem counterintuitive, protecting tumor growth (da Fonseca et al., 2016). The immune system might not be able to react against cancer cells, because, in essence, it was created from such cells during embryogenesis.
Nutrition or defense: primordia of the innate immune system in unicellular holozoa
Phagocytosis, the central function of innate immune responses, first appeared in primitive unicellular eukaryotes. Unicellular holozoan genomes encode homologs of key components of the immune system, including Toll-like receptor (TLR) gene homologs (Suga et al., 2012; Richter et al., 2018; Ros-Rocher et al., 2021). Among the Protozoa, the amoeba Dictyostelium discoideum is often regarded as a primitive macrophage (Cosson and Soldati, 2008). Human phagocytic cells and D. discoideum share several unique functions, such as the ability to migrate over a substrate, the capacity to ingest microorganisms by phagocytosis, and a notably high degree of proteome conservation (Eichinger et al., 2005). Perhaps unsurprisingly, it was in D. discoideum that the first evidence of modifications in the phagocytic activity of bacteria for exclusively nutritional purposes (Clarke and Maddera, 2006), that is, for purposes other than defense, was found. Some types of bacteria can escape phagocytosis and infect D. discoideum (Skriwan et al., 2002; Cosson and Soldati, 2008). To counteract this threat and survive bacterial infections, D. discoideum developed different strategies that include specialized sentinel cells and expression of gene products involved in bacterial death (Eichinger et al., 2005; Benghezal et al., 2006).
Human macrophages must detect and recognize specific pathogen-associated antigens on the surface of foreign cells in order to effectively distinguish pathogens from the body’s own cells. Such antigens are identified by immune cell receptors called pattern recognition receptors (PRRs) (Akira et al., 2001). Dictyostelium is equipped with a broad array of surface receptors that exhibit substantial homology to mammalian PRRs, such as scavenger receptors (LIMP-2), TLRs (tirA, tirB), leucine-rich repeat receptors (LrrA), and C-type lectin receptors (Bajgar and Krejčová, 2023). Activation of such receptors triggers intracellular signaling cascades initiating phagocytosis, phagosome maturation, and bacterial death, as well as cascades related to stress and detoxification responses (Dunn et al., 2018). Metchnikoff’s seminal observations shed light on the intimate connection between Biology (physiology) and Pathology (defense) (Metchnikoff, 1893). I speculate that bacteria began to explore a “wide new universe” with the multicellular experiences of Protozoa, culminating in the emergence of the immune system. From then on, a metazoan embryo was all that was needed for the immune system to perpetuate itself throughout animal evolution.
I consider that a theory encompassing bacterial development (a process that allows bacteria to develop into different cellular states, directly or indirectly interfering with host–pathogen interactions) is essential in order to understand the intimate connection between Biology (physiology) and Defense. Bacteria specifically exploit adhesion molecules (i.e., cell surface proteins involved in cell–cell and cell–matrix adhesion) to endeavor to enter into the multicellular world of Protozoa and Metazoa (Sansonetti et al., 1994; Kerr, 1999; Boyle and Finlay, 2003; Bonazzi et al., 2009). Proteins such as cadherins, integrins, selectins, and immunoglobulin superfamily molecules are well-known targets of a large number of bacteria and other classes of pathogens. A possible reason for this selectivity appears to be the intracellular signaling cascades that such receptors activate, which, in most cases, leads to remodeling of the actin cytoskeleton (Boyle and Finlay, 2003). This argument is very important for the hypothesis raised in this article. Thus, for pathogenesis, bacteria “utilize” the primordial cellular basis of the construction of the first metazoan embryo (Cofre and Saalfeld, 2023). Given that the NFM is an evolutionary engine (Cofre, 2024) and that bacteria interact with its main components (cadherins, integrins, and actin remodeling), it will be very useful to understand the co-evolution of microbes and metazoans.
The first embryo as the first step in the evolution of the immune system
By comparing the genome of ctenophores, sponges, and other basal animal phyla with those of non-metazoan eukaryotes, it can be inferred that most components of signaling cascades, including innate immune system receptors, evolved after the appearance of metazoans. In most cases, these proteins are composed of specific domains (e.g., Pellino) or architectures (e.g., association of a death domain with a Toll/interleukin-1 receptor, TIR, domain in myeloid differentiation factor 88, MyD88) that are found exclusively in metazoans (Gauthier et al., 2010). Yet more relevant is the finding that the dynamic expression of TLRs during embryogenesis and larval development of Amphimedon is consistent with a role in both embryonic development and immunity (Gauthier et al., 2010). In other words, embryogenesis and immune system are closely linked.
Today, it is known that basal metazoans such as ctenophores did not have prototypical TLRs. It is hypothesized that prototypical TLRs arose from the fusion of TRR (a hydrophobic leucine-rich region ectodomain) and TIR (an intracellular Toll/interleukin-1 receptor domain), resulting in the so-called mccTLRs (TLRs with multiple cysteine clusters in their ectodomain) from Cnidaria to Nematoda (Brennan and Gilmore, 2018). With the expansion of TLRs in mollusks, sccTLRs (TLRs with a single cysteine cluster in their ectodomain) emerged. The developmental (convergence extension, planar polarity, and invagination) and immunity functions of Toll (Kuebler and Paré, 2023) were first identified in studies with Drosophila (Gerttula et al., 1988). These embryological functions were also demonstrated in more basal Arthropoda (Benton et al., 2016), indicating that they are highly evolutionarily conserved biological functions, being present in basal groups of animals. An example of such functions is seen with TLR knockdown, which results in abnormal embryonic development in Nematostella vectensis. The species has NF-κB-dependent innate immune system signaling (Brennan et al., 2017). On the other hand, the expression patterns of TLRs in Amphimedon (Gauthier et al., 2010), from an embryonic standpoint, are suggestive of a developmental function that, in my view, serves to facilitate morphogenetic movements. This function has been reported in D. melanogaster (Gerttula et al., 1988).
Surprisingly, the developmental functions of TLRs in vertebrates appear to have been taken over by Wnt/PCP (Roszko et al., 2009), and TLRs became mainly, but not exclusively, restricted to immunity (Leulier and Lemaitre, 2008). Regardless of the participation of Wnt/PCP or TLRs in gastrulation, the invagination process seems to reflect a highly evolutionarily conserved mechanism involving mechanotransduction (Brunet et al., 2013; Pukhlyakova et al., 2018). Such a mechanism was widely discussed in the proposal of NFM as a true evolutionary engine (Cofre, 2024). Therefore, the separation of the embryological and immune functions of TLRs could explain the differences between vertebrate Toll and Drosophila Toll (Hibino et al., 2006). Such a view seems to challenge the idea of a common ancestor in the origin of the innate immune system (Leulier and Lemaitre, 2008). Therefore, in the current hypothesis, there exists a common ancestral origin of TLRs. The decisive embryological events that explain TLR dissociation (gastrulation dissociation) are recent in metazoan evolution. I believe that the decoupling of TLRs and gastrulation in vertebrates has a developmental basis and was crucial for the emergence of the adaptive immune system, a topic that I will cover extensively in a separate article.
Animal evolution played a determinant role in refining the innate immune system. An efficient “digestive” cellular system for ingested material represents only the primary function (Gotthardt et al., 2002) through which phagocytosis extends its functional capacity throughout evolution (Desjardins et al., 2005). A previously formulated hypothesis proposes that many of the characteristics of pro-inflammatory macrophages evolved in the unicellular ancestors of animals, and that the functional repertoire of macrophage-like amebocytes was further expanded with the emergence of multicellularity and the increasing complexity of tissue and organ systems (Bajgar and Krejčová, 2023). As argued by Bajgar and Krejčová, there is a link between the emergence of embryonic morphogenesis and the expansion of possibilities and complexity of the innate immune system. Other authors also acknowledged that the history of the immune system has been shaped over billions of years of bacteria–metazoan co-evolution (Li and Wu, 2021) or by beneficial symbiotic associations with complex communities of microbes (Gerardo et al., 2020), shedding light on the relevant role that bacteria might have played in animal evolution (Hughes and Sperandio, 2008; Alegado et al., 2012). Some bacteria are even able to induce multicellular behavior in choanoflagellates, which appeared before the evolutionary radiation of animals (Alegado et al., 2012).
Capsaspora owczarzaki is a unicellular eukaryote—a protist. Phylogenetically, Capsaspora belongs to the clade Filasterea, one of the closest unicellular relatives of animals, together with choanoflagellates, ichthyosporeans, and corallochytreans (Ferrer-Bonet and Ruiz-Trillo, 2017). Based on this close evolutionary relationship, Capsaspora is becoming pivotal to understanding the origins of animal multicellularity. Recent investigations revealed that C. owczarzaki and some choanoflagellates contain genes encoding nuclear factor-kappa B (NF-κB)-like proteins (Sebé-Pedrós et al., 2011; Suga et al., 2013; Richter et al., 2018). NF-κB is a molecule extensively studied for its role in embryonic development and immunity, from the first animal to modern humans (Gilmore, 2006; Ghosh and Hayden, 2012; Williams and Gilmore, 2020). It should be noted, however, that functional NF-κB-like proteins (DNA binding and transcription activators) vary considerably in general structure, activity, and regulation mechanisms, both between protists and in comparison with animal NF-κB (Williams et al., 2021). The emergence of metazoans seems to have been essential for the definitive configuration of the NF-κB protein, further supporting the view that the emergence of the first embryo was a fundamental step in the development of the immune system.
Considerations on the trophic conditions of the first animal
Let us ponder on the trophic conditions of the first metazoan (multicellular embryo). What could this unique organism eat? It is important to point out that this primitive condition might have fostered the first parasite–host relationship. I can predict through my hypothesis that the preservation of phagocytic capabilities from unicellular holozoans in the archenteron of the first embryo would be a matter of survival (Figure 1A). Likewise, the differentiation of specialized macrophages can be seen as a matter of preservation of homeostasis and integrity in this new multicellular metazoan niche, as will be discussed later.
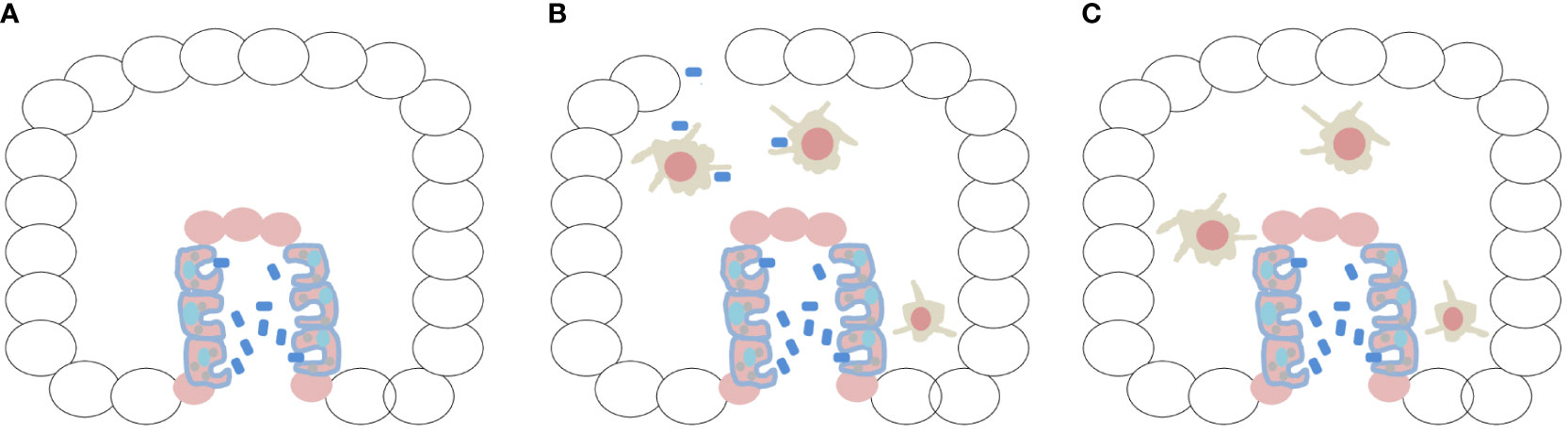
Figure 1 The first embryo would be a benign tumor, protected and fed by the innate immune system. (A) Creation of a meta-organism after invagination and archenteron formation, linked to the conservation of the ability to recognize bacteria, would have been essential for the nutrition of the first embryo, being a matter of survival. Ciliated digestive cells lining the archenteron were identified in ctenophores (Franc 1972; Hernandez-Nicaise 1991; Presnell et al. 2016; Vandepas et al. 2017). (B) Animals such as ctenophores are very fragile, and their rupture or mechanical injury might have allowed bacterial invasion of the embryo. During morphogenesis, macrophages differentiated and protruded into the mesoglea by epithelial–mesenchymal transition. This created a different phase of phagocytosis (and exaptation of TLRs) represented by professional macrophages, constituting protective immunity. (C) The embryo would be a benign tumor nourished and protected by the immune system, and the two phenomena, enteric phagocytosis and protective immunity by professional macrophages, were formed in association with physical phenomena (invagination/EMT/gastrulation) that occurred during morphogenesis of the first embryo.
Although body plan is not the focus of this article, it is possible to affirm that the first embryo likely subsisted on picoplankton (planktonic microbes measuring 0.2–2 µm in diameter) and dissolved organic matter (Mills and Canfield, 2017). At the time of their emergence, the first metazoans would be the only representatives of complex multicellular clades (without cell wall) with the ability to phagocytose particles and other cells for nutrition purposes. The first embryo, therefore, probably occupied similar trophic levels and niches as its unicellular flagellate ancestors (Richter and King, 2013). To clarify, the appearance of the first animal was not necessarily accompanied by major changes in prey preferences (Valentine and Marshall, 2015).
What food would be available to the first metazoans? Bacteria, flagellates, multicellular structures, and phytoplankton would likely have been their primary prey (Lenton et al., 2014; Leys, 2015). Without wide availability of animal life, primitive seas would unlikely be rich in bacteria and fecal aggregates, which contain high levels of dissolved organic carbon. Filtration as a feeding mechanism would have been energetically costly (Leys et al., 2011; Leys, 2015), so it is unlikely to have originated in oxygen-poor oceans. Even if the hypothesis of the emergence of the most basal animal taxon (Ctenophora) turns out to be correct (Ryan et al., 2013; Moroz et al., 2014; Arcila et al., 2017; Shen et al., 2017; Whelan et al., 2017), the first animals could not have been carnivores like modern ctenophores. Thus, the most likely feeding strategy during embryonic and early animal development is bacterial phagocytosis.
On the first animal and its immune system
Although the traditional view is that sponges form the first branch of the animal tree (Linden and King, 2021), several studies suggest the Ctenophora as the basal-most animal lineage (Ryan et al., 2013; Moroz et al., 2014; Arcila et al., 2017; Shen et al., 2017; Whelan et al., 2017). Also, there remains no doubt that ctenophores have a well-developed innate immune system (Traylor-Knowles et al., 2019; Arroyo Portilla et al., 2021). Two TLR homologs identified in Mnemiopsis leidyi and Hormiphora californiensis contain LRR domains that mediate interactions with microbial pathogens in humans (Ng et al., 2011). Furthermore, both species were found to express perforin-2 (MPEG-1) (Traylor-Knowles et al., 2019), suggesting an older role in attacking Gram-negative bacteria (McCormack and Podack, 2015). On the other hand, a single homolog of NOD-like receptor (NLR), Jun, and Fos was detected (Traylor-Knowles et al., 2019), potentially indicating a well-simplified cytosolic defense system against other metazoan lineages and at least suggesting that a heterodimerization interaction might have produced a functional AP-1 homolog at the base of the evolutionary radiation of animals.
An astounding finding was the identification of highly mobile cells traveling through the mesoglea of ctenophores, exhibiting amebocyte-like morphology and converging on experimentally damaged areas under the dermis (Traylor-Knowles et al., 2019). When challenged with live or heat-killed bacteria, in vivo and in vitro, these mobile amoebocyte-like cells exhibited pinocytic and phagocytic behavior. Bacteria were rapidly engulfed (within minutes) and transported to acidic compartments within the cell. Ciliated digestive cells lining the gastrovascular canal (archenteron) had already been observed in M. leidyi and other ctenophore taxa (Franc, 1972; Hernandez-Nicaise, 1991; Presnell et al., 2016; Vandepas et al., 2017), but ciliated digestive cells exhibiting phagocytic behavior toward bacteria were only recently reported (Traylor-Knowles et al., 2019). In my view, both observations, namely amoeboid cells in the mesoglea and phagocytic cells in the primitive gut, are a reflection of immunity (protection) and survival (nutrition) behaviors. (Hartenstein and Martinez, 2019). As will be discussed later, immunity (protection) arose from the epithelial–mesenchymal transition (EMT) of the primitive intestine (Figure 1B), constituting the neoplastic basis for the formation of the first embryo.
Considerations on ctenophores as meta-organisms and their influence on the formation of the immune system
All multicellular organisms are intimately associated with bacteria and, therefore, together constitute metaorganisms, a fact that has already attracted public and scientific attention worldwide. The scientific community has begun to elucidate the essential role of the microbiota in the functioning of humans (Rook et al., 2017) and in the life history of animals. This role becomes evident, for example, in basal marine metazoans such as corals and jellyfish. Bacteria and their exudates have a crucial impact on the induction of metamorphosis and settlement of the planula larva of Cassiopea andromeda (Neumann, 1979) and the coral Acropora millepora (Tebben et al., 2011). Bacterial exudates were also reported to induce sexual reproduction (Woznica et al., 2017) and the formation of multicellular structures (Alegado et al., 2012) in choanoflagellates.
Such discoveries enticed us to think deeper and allowed us to conclude that interactions with bacteria can moderate/modulate/mediate evolutionary processes (Reshef et al., 2006; Zilber-Rosenberg and Rosenberg, 2008; Rosenberg et al., 2009; Bosch and McFall-Ngai, 2011). There is a call for transdisciplinary collaboration in zoology (Bosch and McFall-Ngai, 2011) that should be well extended to evolution. Animals do not live in isolation but rather in complex communities that interact with environmental conditions—a highly relevant perspective when it comes to evolutionary phenomena. In the context of this article, ctenophores, as a basal metazoan phylum (Ryan et al., 2013; Moroz et al., 2014; Arcila et al., 2017; Shen et al., 2017; Whelan et al., 2017), are considered meta-organisms (Daniels and Breitbart, 2012; Jaspers et al., 2019).
A more detailed understanding of Ctenophora–bacteria interactions at the base of the metazoan tree may reveal important information about the evolution of meta-organisms. In this article, I will make predictions about the possible contributions of bacteria to the exaptation of the neoplastic functional module (NFM) and the differentiation of the innate immune system. The next section explores the environmental conditions that enabled the emergence of these phenomena.
Ediacaran ocean
The Ediacaran fossil record reveals an intriguing pattern: the first animals inhabited only deep-water environments and experienced frequent hypoxia (Boag et al., 2018). Therefore, it appears that hypoxia resistance was acquired at the beginning of animal life, and the Warburg effect (which will be discussed in the following section) may be a remnant of the evolutionary history of metazoans. The fossil record also suggests that the Ediacaran biota initially appeared around 571 Ma in deep ocean waters before appearing in shelf environments at approximately 555 Ma (Jablonski et al., 1983; Boag et al., 2018) Thus, from an evolutionary perspective, understanding the tolerance of marine animals to hypoxia is of utmost importance for my hypothesis.
Today, it is thought that metazoan evolution was facilitated by a series of dynamic and global changes in redox conditions and nutrient supply, which sustained multiple radiation phases in metazoans, one of them in the Ediacaran Period (Wood et al., 2019). Thus, it has been proposed that changes in Ca2+ concentration in the Ediacaran ocean were a crucial driving force behind major innovations in evolution, including multicellularity and the origin of metazoans (Brennan et al., 2004; Kazmierczak et al., 2013).
Also, it is postulated that the ancient ocean had high alkalinity and low concentrations of Ca2+ and Mg2+, similar to modern soda lakes, commonly associated with volcanic regions (Kempe and Degens, 1985). It is assumed that the first soda ocean gradually evolved into a sodium chloride-rich ocean through “titration,” emanating volatile acids such as HCl, thereby reducing the pH and finally reaching a titration point of Ca2+, allowing its relatively rapid accumulation at the end of the Precambrian (Kempe and Degens, 1985; Kazmierczak et al., 2013). In a time period of 3 billion years, the primitive concentration of calcium in the ocean increased by 1,000 to about 100,000 times (Kempe and Degens, 1985; Kazmierczak et al., 2013). A strong indicator of an early alkaline ocean is the predominance of alkaloid cyanobacteria (Cyanophycea) in Precambrian biota (Kempe and Degens, 1985).
There is evidence of alkali-tolerant and extremely alkalophilic bacteria (Padan et al., 2005) that could withstand the hostile environment of the Ediacaran ocean. Such organisms are important from the viewpoint of current medicine (Livermore, 2003; Levy and Marshall, 2004) because the emergence of resistance to novel antibiotics is linked to alkali tolerance (Krulwich et al., 2005). The answer to this problem may lie in our evolutionary history. Systematic studies have shown that many alkalophilic prokaryotes represent separate lineages within currently accepted taxa, whereas some have no strong relationship with other known prokaryotes (Jones et al., 1998). It appears that these alkalophilic communities evolved independently in an early period of Earth’s history. What is important to our hypothesis is that the first embryo and animal had access to alkalophilic bacteria as a source of nutrition.
The Warburg effect
There is still considerable debate as to whether or not oxygenation was the main factor in early metazoan evolution (Towe, 1970; Wood et al., 2019). Although all existing animals need oxygen, their demands are not always the same. The most tolerant organisms of extreme oxygen depletion on the seafloor include calcareous foraminiferans, nematodes, and annelids (Levin, 2021). A recently developed biogeochemical model revealed that atmospheric and oceanic oxygen levels in the Ediacaran Period were dissociated, indicating that the early increase in atmospheric oxygen levels in the Ediacaran might not have promoted generalized oxygenation of the deep ocean (Shi et al., 2022), which is also consistent with geochemical records (Lenton et al., 2014). Experimental results with basal groups of animal evolution demonstrated that the sea sponge Tethya wilhelma can withstand oxygen levels as low as 0.25% to 4% of current atmospheric levels (Mills et al., 2014).
Ctenophores also live in deep ocean waters (Harbison et al., 1978; Haddock et al., 2017) and some have been found at 7,200 m depth (Lindsay and Miyake, 2007), where there are extremely low oxygen levels. Genomic analyses of sponges and ctenophores showed that both lack important components of the hypoxia-inducible factor (HIF) pathways that maintain cellular oxygen homeostasis (Mills et al., 2018). Therefore, ctenophores might have performed aerobic metabolism under very low concentrations of oxygen.
As for copepods, which are highly resistant to hypoxia and important representatives of marine zooplankton, Calanoides carinatus carries out part of its embryonic development at a depth of 700 m in the ocean (Auel et al., 2005; Verheye et al., 2005; Auel and Verheye, 2007). The nauplius larvae of Labidocera aestiva and Acartia tonsa are highly resistant to extreme hypoxia conditions. Surprisingly, Tigriopus californicus, a resident of supralittoral rocky pools where dissolved oxygen levels can drop to ~0 mg/L (Truchot and Duhamel-Jouve, 1980), has become an excellent physiological model for the study of oxygen deprivation in animals without respiratory structures (Graham and Barreto, 2019). It is the first marine animal whose high hypoxia resistance (Goolish and Burton, 1989; Graham and Barreto, 2019) can be linked to the Warburg effect.
Surprisingly, studies on T. californicus, showed that these organisms can withstand prolonged exposure to extreme oxygen deprivation, despite having secondarily lost the main members of the HIF pathway, being similar to tumor systems (Graham and Barreto, 2019). Allie Graham and Felipe Barreto predicted a shift from oxidative phosphorylation to glycolysis under hypoxic conditions. Consistent with this prediction, the authors observed a significant increase in pyruvate dehydrogenase kinase (PDK) mRNA levels (Graham and Barreto, 2019). This is the Warburg effect (Warburg, 1956) occurring in highly hypoxic animals and thereby revealing, in my opinion, a vestige of our origin in deep ocean waters. Despite a large volume of papers researching this effect in cancer, the function of the Warburg effect has remained unclear (Liberti and Locasale, 2016).
Another expected prediction for the Warburg effect is that elevated glucose metabolism decreases the pH within the cell due to lactate formation (Estrella et al., 2013), thereby requiring greater activity of H+ extrusion mechanisms to prevent cell death (DeCoursey, 2015). Considering the animal formed in the Ediacaran ocean under hypoxic conditions (Shi et al., 2022) in an alkaline sea (Kempe and Degens, 1985), it is expected that it would show H+ efflux. Voltage-gated proton channels (Hv1) with ΔpH-dependent regulation occur in several organisms, from protists to humans (DeCoursey, 2015), and are responsible for bioluminescence in dinoflagellates (Rodriguez et al., 2017; Kigundu et al., 2018). These channels might have helped calcify (Taylor et al., 2011; Kottmeier et al., 2022) and acidify the Ediacaran ocean (external environment).
In line with these observations, acidification of the microenvironment in tumor models has always been considered an intriguing aspect of the disease (Schlappack et al., 1991; Rofstad et al., 2006; Moellering et al., 2008). The potential benefits of acidosis to cancer cells have been discussed. Some hypotheses of acid-mediated invasion suggest that H+ ions secreted by cancer cells diffuse into the surrounding environment and alter the stroma interface of the tumor, allowing for greater invasiveness (Gatenby and Gawlinski, 1996; Estrella et al., 2013). It is possible that the Warburg effect and acidification are a remnant of our evolutionary past linked to Neoplasia. Thus, in the first ctenophores, the Warburg effect might have promoted high proliferation and cell growth in a hypoxic and highly alkaline environment.
Unraveling the malevolent alliance of macrophages with tumor cells at its evolutionary origin
Most surprising in oncology and modern immunology is the recognition of the functional ambiguity of tumor-associated macrophages (TAMs), mainly because they seem to promote tumor evolution, which represents a challenging obstacle in cancer treatment (Ostuni et al., 2015). It is also remarkable how tumors seem to exploit the trophic activity of macrophages to survive and progress. Disrupting this “malevolent alliance” between cancer cells and macrophages might represent a crucial step toward cancer cure (Allavena et al., 2021).
Later in this article, I will present a summarized part of my hypothesis proposing that the first embryo was a benign tumor, with some cells performing digestive phagocytosis in the archenteron as a trophic survival activity. At more advanced stages of morphogenesis, macrophages are formed and, through EMT, move into the mesoglea (in a kind of controlled metastasis by specific exaptation of the NFM) and create a different function for phagocytosis (immunity conferred by professional macrophages) (Figure 1B). Therefore, the first embryo is a tumor with macrophages that feed and protect it (Figure 1C). From the point of view of my hypothesis, rather than a “malevolent alliance,” there is an intimate and common evolutionary origin with Neoplasia. The immune system cannot deny its origin.
Evolutionary remnants of the Warburg effect in the immune system and macrophages
Before describing my hypothesis on the emergence of the innate immune system, I would like to clarify the evidence of the Warburg effect in phagocytic cells. Phagocytosis uses acidification systems that nowadays involve NADPH oxidase and energy (ATP) (Lukacs et al., 1990; Nunes et al., 2013). This functioning makes sense in the context of the current environment, without alkalization and in the presence of oxygen. But the presence of voltage-gated proton channels (Hv1) with ΔpH-dependent regulation in neutrophil phagosomes (Okochi et al., 2009) is not plausible without recognizing the evolutionary history of the immune system in the first embryo. These observations point to remnants of the Warburg effect in the innate immune system. As for the adaptive immune system, I published a personal communication discussing evidence of the Warburg effect in vertebrate lymphocytes (Cofre, 2022).
The primary function of Hv1 channels, in an oxygen-free and highly alkaline environment (Shi et al., 2022), would have been to neutralize soda in the Ediacaran ocean (Kempe and Degens, 1985). Subsequently, in response to oxygenation of the Earth’s atmosphere, Hv1 channels would have been co-opted for the production of oxygen free radicals, coupled to NADPH oxidase (Okochi et al., 2009). Therefore, I propose that the presence of HV1 channels in myeloid-derived suppressor cells (MDSCs) (Cozzolino, 2022; Cozzolino et al., 2023) also points to the evolutionary origin of the immune system.
Consistent with my hypothesis, traces of the Warburg effect are also found in the response of macrophages to hypoxia. Multiple studies have shown that experimental hypoxia alters cell morphology, expression of cell surface markers, cell viability, phagocytosis, metabolic activity, and cytokine release in macrophages (Lewis et al., 1999). Surprisingly, exposure to hypoxia markedly reduced cytochrome oxidase activity and increased pyruvate kinase activity in alveolar macrophages (Butterick et al., 1981), revealing the occurrence of the Warburg effect in the innate immune system. These findings suggest that macrophages can adapt to a lack of oxygen by switching to anaerobic glycolytic pathways for ATP production, resembling malignant cells under hypoxic conditions (Lewis et al., 1999).
Looking at our evolutionary history and the conditions of the Ediacaran ocean, I find plenty of antecedents of the intimate relationship between embryogenesis, cancer, and immune function. The following section presents a description of my hypothesis.
Discussion
Nothing in biology makes sense except in the light of evolution
All metazoans (animals) are produced by fertilization, so the beginning of animal life only makes sense with the formation of an embryo (Cofre and Saalfeld, 2023). Developing an embryo requires cells to proliferate physically united under an initial mechanical phenomenon that I believe would be a multiflagellate fusion event (Cofre, 2022). The initial impact of multiflagellate fusion is a determinant physical event at the beginning of animal phylogeny that left its records imprinted on the first metazoan cells in the form of cytasters (Cofre, 2022). It also promoted intense reorganization of the actin cytoskeleton in the cytoplasm and nucleus of the first “zygote” and produced a form of genomic reorganization that produced the NFM (Cofre and Saalfeld, 2023). In the context of the genesis of the innate immune system, there are at least three basic elements of the NFM that are interconnected with the genome: (i) the proliferation process (cell cycle), (ii) the adhesion process (surface molecules, such as cadherins), and (iii) the bacterial recognition process (phagocytosis).
Two important discoveries guided the field of innate immunity. The Drosophila Toll protein, previously known only for its functions in embryonic development (Anderson et al., 1985), was characterized as necessary for flies to mount an effective immune response to the fungus Aspergillus fumigatus (Lemaitre et al., 1996). TLR4 was later identified as necessary for mice to mount effective responses to Gram-negative bacteria (Poltorak et al., 1998). Therefore, the innate immune system of insects and mammals has an evolutionary relationship; TLRs were proposed to have a central role in the primary recognition of infectious pathogens throughout evolution (Beutler, 2004). This evolutionary basis was consolidated because TLRs, as discussed previously in this article, were found in protists and ctenophores.
The formation of a multicellular embryo connected by a cytoskeleton producing mechanical tension forces during morphogenesis is an extremely energy-demanding process. Therefore, it seems logical to me to incorporate TLR elements as fundamental components of the first animal, participating in its survival, a real matter of subsistence. By co-opting TLRs, this first organization ensured that the organism would be able to phagocytose bacteria and supply the necessary resources for ATP production under anaerobic conditions during embryogenesis. The first resources of our first embryo likely stemmed from multiflagellate fusion and were therefore scarce until the formation of the primitive gut (Presnell et al., 2016). It is easy to understand why TLRs (PRRs in general) are so strongly related to embryology (dorsal/ventral patterning was their first function) (Lemaitre et al., 1996), cancer (immunotherapy) (Anderson, 2000; Chen et al., 2008; Rakoff-Nahoum and Medzhitov, 2009; So and Ouchi, 2010; Pradere et al., 2014; Shi et al., 2016; Braunstein et al., 2018), and the survival of the first animal (enteric phagocytosis) (Hartenstein and Martinez, 2019).
Animal genesis involving the co-option of the NFM led to unbridled and vertiginous proliferation limited by cadherins. This process, this initial expansive force, would be Neoplasia. Neoplasia is not simply cell proliferation but rather a force of transformation, a process that arose with the multiflagellate biophysical impact on animal genesis. Therefore, Neoplasia is an embryological process that represents the cancer side in all embryos (Cofre and Saalfeld, 2023). The disease cancer will change throughout animal phylogeny (as will be discussed in several separate articles), but, whenever cancer appears in any species, it reveals its embryonic evolutionary origin (Ben-Porath et al., 2008; Yu and Xu, 2020; Lee, 2022).
During the first growth phase of the embryo, cells learned to grow together and managed to migrate together to produce the first great revolution—epiboly—the outline of the first collective migration (Cofre, 2024). In this context, by growing together, cells held great potential for the creation of new animal forms. However, many of the characteristics of our unicellular holozoan ancestors were suppressed. For instance, in a mechanically united epithelium, amoeboid and migratory characteristics cannot manifest themselves freely. On the other hand, phagocytosis can still occur in restricted places of the plasma membrane. In primary cell cultures of ctenophores (Vandepas et al., 2017; Traylor-Knowles et al., 2019), despite the mechanical destruction of cells with trypsinization, centrifugation, and exposure to classic mammalian culture media, it is possible to observe the basic characteristics of the NFM in the first adult animal: (i) ectodermal and endodermal proliferative cells (Vandepas et al., 2017), (ii) some cellular associations (cell–cell interactions, cells that learn to grow together) (Vandepas et al., 2017), and (iii) amoeboid cells with the ability to phagocytose bacteria (Traylor-Knowles et al., 2019).
During embryo construction, the initial module undergoes exaptations, which are a representation of the physical changes (and their impact on genomic reorganization) that occur during morphogenesis of the first embryo. The second great revolution was the co-option of extracellular matrix (ECM) construction and remodeling to the Neoplastic module (Cofre, 2024). The latter has consequences on the formation of the two faces of the innate immune system (nutrition and defense); the innate immune system becomes linked to two sequential morphogenic processes that are important for embryo formation.
Constructing an ECM on a mechanically joined epithelium translates into the creation of textural gradients (Hartman et al., 2017; Zhu et al., 2023) and the onset of durotaxis (Sunyer et al., 2016) and topotaxis (Park et al., 2018), which will signal cell differentiation. But the relevant aspect for animal evolution is ECM remodeling, which will translate into shape changes, such as invagination and EMT movement. EMT is a fundamental embryonic process for embryo construction and reconstruction of the adult animal (Savagner, 2001; Arnoux et al., 2005). In the framework of my hypothesis, it represents the cancer (Neoplastic) side of the embryo revealing itself (Berx et al., 2007; Thiery et al., 2009; Cofre and Saalfeld, 2023), although always contained by ECM topology, leading EMT to cell differentiation (controlled metastasis).
Considering the first embryological event that was decisive for the formation of the innate immune system, with evidence in ctenophores (Martindale and Henry, 2015), I speculate that invagination by tensional forces began at the aboral pole (Cofre, 2024), which led to the production of a meta-organism (Daniels and Breitbart, 2012; Jaspers et al., 2019). This marked the occurrence of the first co-option of phagocytosis and TLRs to a mechanical embryonic event (which was registered in the NFM), linking the innate immune system of animals to archenteron differentiation (Figure 1A). I draw the attention of readers to the fact that phagocytosis and TLRs are of protist origin (Suga et al., 2012; Richter et al., 2018; Ros-Rocher et al., 2021) and incorporated into the NFM. Therefore, I speculate that they have an important link with cancer (Rakoff-Nahoum and Medzhitov, 2009; Pradere et al., 2014). TLRs were co-opted to the NFM during a morphogenic event, explaining their relevant role in embryogenesis throughout animal evolution (Brennan and Gilmore, 2018).
I underscore that TLRs were associated with enteric phagocytosis in the archenteron (Figure 1A). This unique moment allowed consolidating the embryonic structure by means of the energy support obtained by digestion of alkalophilic bacteria. TLR proteins changed throughout animal evolution (Patthy, 1999), and Neoplasia itself created innovations from these proteins (Cofre, 2024). Such processes will allow us to understand the emergence of the adaptive immune system at a different time point of animal history. Ctenophores have Transib transposons in their genome (Kapitonov and Jurka, 2005), which would enable the reordering and creation of new domains in TLRs, triggering exclusive innovations in animals (Gauthier et al., 2010), and bacteria in their archenteron, from which RAG recombinase is derived to mediate V(D)J recombination (Tao et al., 2022). The emergence of the adaptive immune system and its relationship with embryonic development will be discussed in a separate article.
The innate immune system rapidly underwent a second co-option associated with ECM remodeling processes. Matrix metalloproteinases (MMPs) were co-opted to the NFM, along with TLRs and phagocytosis. Some cells with amoeboid characteristics (capacity for phagocytosis and TLR expression) were able to project into the mesoglea and fulfill a completely different function for the embryo, that is, of defensive immunity (professional macrophages) (Figure 1B). Thus, phagocytosis and TLRs were co-opted to the NFM via two mechanical phenomena that occurred at different periods.
A good strategy to test the hypothesis of modular exaptation of phagocytosis and TLRs during embryogenesis is to analyze the other cells that were incorporated into the mesoglea. If the process is linked to EMT in ctenophore embryos, TLRs and phagocytosis should be found in other mesodermal cells. This behavior is observed in microglia of Beroe cucumis, and phagocytosis occurs in the mesoglea (Aronova and Alekseeva, 2004). In mammals, microglia (TLRs and phagocytosis) perform important functions in the context of the complexity of the nervous system (Xu et al., 2022). Are the microglia of B. cucumis and mammals equivalent? No. There is a long evolutionary history that explains how ctenophore microglia led to mammalian microglia. With this, it will be possible to understand the keys to phagocytosis and inflammatory responses in Alzheimer’s disease, multiple sclerosis, Parkinson’s disease, traumatic brain injury, ischemic, and other brain diseases (Fu et al., 2014; Gibson and Monje, 2021; Xu et al., 2022). Are microglia part of the innate immune system of ctenophores? Yes. From an embryological point of view, in my opinion, there is no doubt that microglia are at the origin of the first embryo and linked to Neoplastic phenomena, mainly because cells perform EMT to project to the mesoglea. Also, the same malevolent alliance can be found between microglia and glioblastomas (da Fonseca et al., 2016).
Co-option of TLRs (now alone, without phagocytosis) is also associated with cells of the enteric nervous system of humans (Vicentini et al., 2022). I do not yet intend to establish any structural relationship between the enteric nervous system and the mesogleal nervous network of ctenophores (origin of the neuromesoderm) (Jager et al., 2011). However, at present, all evidence points to the importance of microbiota in neural regulation and neurogenic functions (Belkind-Gerson et al., 2017; Aktar et al., 2020) and the key role of bacteria in colorectal (Candela et al., 2014; Sobhani et al., 2019), pancreatic (Zhang et al., 2020), and gastric (Ferreira et al., 2018) cancers. One of the current challenges of science is to understand to what extent bacteria in the meta-organism influenced the emergence of immunity. Today, evolutionary biologists know that bacteria influence the multicellular experiences of protists; therefore, it is interesting to think of the microbiota of the meta-organism as an inducer of morphogenesis.
This unique condition of modular exaptation to the NFM at two different times provides diversification possibilities for the embryo. Cells will be able to eat and react to bacteria invading the mesoglea. How do bacteria invade? Ctenophores are very fragile animals, and mechanical rupture of the animal body might have led to an exhaustive invasion and homeostasis interference. Moreover, their epithelial cell–cell bonds are more tenuous (transient adherens junctions) (Tamm and Tamm, 1991; Tamm and Tamm, 1993), and, thus, invasion of the mesodermal environment would be inevitable.
Thus, the initial embryo had at its disposal a mechanism of bacterial recognition rooted and inherited from holozoans (which used this mechanism for trophic purposes). The immune system emerged via modification of the NFM, which incorporated the system at different times and structures during embryo morphogenesis. It is important to emphasize that there is no competitive fitness here; this is the only animal in the immense Ediacaran ocean. This first animal was not competing with anyone, it simply manifested a potential inherent to its holozoan origin. The first animal is a representation of how embryos imbibed DNA through their physical and environmental contexts during early embryogenesis. Was the construction of the NFM successful? NFM has been conserved so far in humans and apical groups. It is also present and conserved in the evolution of the immune system, whose evolutionary records were made through the Warburg effect.
The first animal would not be able to compete with bacteria; it would be, from any point of view, an unfair competition for the first fragile representative of metazoans. I believe that it is not possible to compare the long successful history of the phylum Archaea on planet Earth to that of animals, mainly because the two have, so far, collaborated intensely and evolved together.
To conclude my proposal, I wish to quickly and briefly address the fundamentals of reconstruction in the next generation of the innate immune system created in this hypothetical embryo, as described by Cofre and Saalfeld (2023). How would it be possible to reestablish bodily coherence and integrity throughout evolution? Any embryonic event that brings mechanical and physical consequences for the embryo “as a whole” will be exapted to the NFM, conceptually denoting a mechanical topology in the genome (Zheng et al., 2009; Fedorchak et al., 2014; Cho et al., 2017; Miroshnikova et al., 2017; Uhler and Shivashankar, 2017a; Uhler and Shivashankar, 2017b; Wang et al., 2017). Maintaining the mechanical records of these events in the nucleus, or in a restricted set of embryonic cells, was a primordial step in animal phylogeny and is one of the conceptual bases of my hypothesis. The basis is in the stress waves generated by morphogenic processes (Pajic-Lijakovic and Milivojevic, 2022) and in the bionanowires of the nuclear actin cytoskeleton (Mori et al., 2014; Okuno et al., 2020; Wesolowska et al., 2020; Hunley and Marucho, 2022), which support an integrated embryonic structure influenced by galvanotaxis directed from the apical pole (Coonfield, 1936).
Experiments on ctenophores demonstrated their incredible ability to regenerate (Martindale and Henry, 1996; Tamm, 2012; Edgar et al., 2021). Cutting ctenophores in half results in the creation of two perfectly equal animals (Coonfield, 1936). These experiments provide insight into a construction network in which the parts can generate the whole. Nevertheless, a paradigm is evident from the conclusions of Coonfield: “Halves, thirds, and fourths of Mnemiopsis regenerated the lost parts while eighths of this animal failed to regenerate.” I will address this paradigm separately, because it is known that two cells (a tiny part of the whole), sperm and oocyte, can generate a whole animal.
Consistent with my proposal, basal animals in animal phylogeny belatedly separate the germline (Juliano et al., 2010; Fierro-Constaín et al., 2017; Edgar et al., 2021). This is completely expected and necessary for the beginning of animal life. In the first embryo, germ cells must first receive all mechanical and physical stimuli from embryogenesis to trigger the separation of the germ line. This brings to mind the philosophical question of what came first, the egg or a beroid-like ctenophore? The answer is unequivocal: the egg came first. But there is one caveat. It emerged only after it had been completely impregnated by its surroundings and had its physical trajectory defined inside the embryo (Cofre and Saalfeld, 2023). This first embryonic trajectory is fundamental, and I can represent it in the words of a Spanish poet, “Walker there is no path, you make the path by walking (when walking you make the path)” (MaChado, 2021). Therefore, the embryo modeled the genomic records of its construction during embryogenesis. This rule is absolute for the first embryo that will establish a topological map of Physics in the genetic material (Uhler and Shivashankar, 2017a; Uhler and Shivashankar, 2017b; Uhler and Shivashankar, 2018; Tsai and Crocker, 2022).
In line with these arguments, there is evidence of biophysical regulation of the chromatin architecture and of how the load and persistence of forces applied to the nucleus can trigger and define the trajectory of a given cell population (Heo et al., 2015; Dai et al., 2020). A long dynamic tensile loading resulted in a more robust chromatin condensation, not always related to actin cytoskeleton contractility. Scientists have avoided discussing biological (molecular/genetic) clocks as mechanical (Hubaud et al., 2017). But segmentation of somites, classically a biological clock (Dubrulle and Pourquié, 2002; Pourquié, 2003; Andrade et al., 2007; Klepstad and Marcon, 2023), is evidently subjected to tensile forces of elongation and folding (Mongera et al., 2019; Anand et al., 2023; Yaman and Ramanathan, 2023). Thus, in the context of this hypothesis, mechanical memory (like a clock) will explain how germ cells manage to rebuild all structural coherence in the next generation; these topics are discussed in a separate article (Cofre, 2022).
Finally, I have openly said that the embryo is a benign tumor, protected and nourished by macrophages. Therefore, the embryo and macrophages are inseparable (Figure 1C). Readers should have many doubts as to why an EMT process with rupture of the basal lamina did not turn into cancer. It actually is cancer, the cancer side of the embryo, but it differentiated and was regulated/controlled by the embryonic microenvironment (Figures 2A, B).
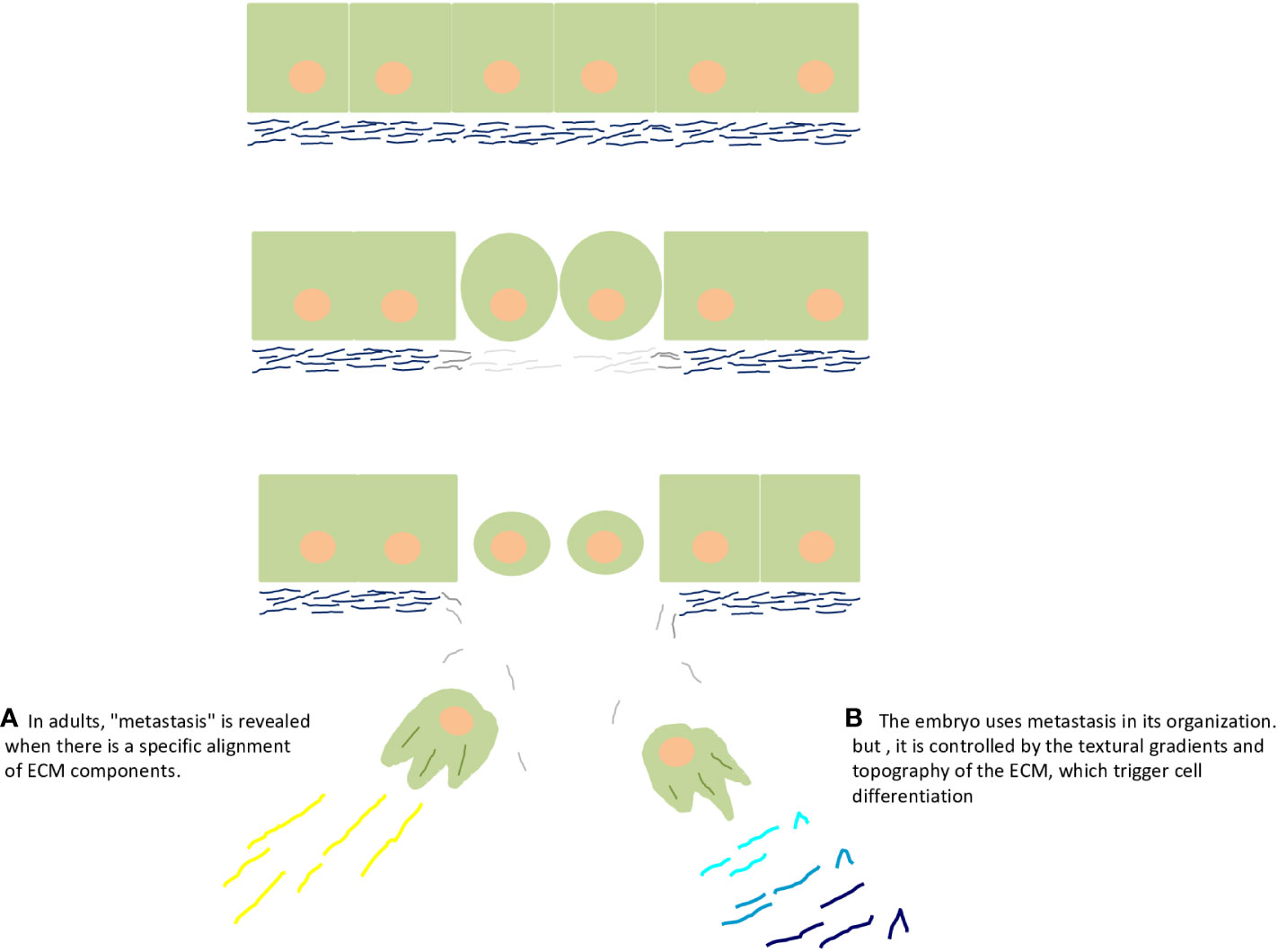
Figure 2 Embryo and cancer are two sides of the same coin, of the same process. The upper part of the figure depicts rupture of the basal lamina by matrix metalloproteinases during epithelial–mesenchymal transition. (A) Epithelial–mesenchymal transition reveals the cancer side of the embryo and adults. Particularly, in adults, "metastasis" is revealed when there is a specific alignment of extracellular matrix components, such as collagen and fibronectin. (B) The embryo uses metastasis in its organization (commonly called gastrulation), but it is controlled by the textural gradients and topography of the extracellular matrix, which trigger cell differentiation, as supported by the seminal ideas of G Barry Pierce on the control of cancer by the embryo.
To clarify this seemingly controversial point, I highlight my great inspiration in oncology and pay tribute again to the work of G. Barry Pierce.
I propose to discuss a concept of cancer that has been formulated upon our experiments on differentiation and cancer, outline the current experiments from our laboratory in regulation of cancer by the embryo, and indicate the potential importance of this approach for therapy (Pierce, 1983).
Thus, what is controlling the cancer side of the embryo? Why did the cells that protruded into the mesoglea not turn into cancer? In his articles, Pierce suggested that it would be the “differentiation of malignant to benign cells” (Pierce and Johnson, 1971; Pierce and Wallace, 1971; Pierce et al., 1982) happening inside the embryo (Pierce, 1983). All of us who work with cancer know that it is a disease of differentiation (Markert, 1968). So, what controls cancer in the embryo? Cell differentiation is stimulated by cadherins in epithelia (Cofre and Saalfeld, 2023) and by textural gradients in the ECM (Figures 2A, B) (Cofre, 2024).
There are strong scientific indications that alignment of ECM proteins such as collagen (linear collagen molecules) and fibronectin (linearly aligned structure) influences metastasis in several types of tumors (Conklin et al., 2011; Ao et al., 2015; Drifka et al., 2016; Yang and Friedl, 2016; Erdogan et al., 2017; Conklin et al., 2018; Dzobo and Dandara, 2023). Also, it is widely recognized that tumor progression coincides with mechanochemical changes in the ECM, providing a denser network of collagen-rich fibers (Nieponice et al., 2009; Nguyen et al., 2014; Barcus et al., 2015; Harisi and Jeney, 2015; Holle et al., 2016; McLane and Ligon, 2016; Mittal et al., 2016; Barcus et al., 2017). What the scientific community must recognize is that no one has investigated ECM and its textural gradient in embryonic models in the search for cancer cure. In my view, the best model to study gliomas, which developed within a mechanical microenvironment characterized by dense ECM and under conditions of hypoxia and HIF1α activation (Miroshnikova et al., 2016), is the mesoglea of ctenophores. The mesoglea would help to understand normal textural configuration, and this knowledge can be applied to develop new cancer therapy strategies (modeling ECM texture in cancer with physical foundations that make it look like mesoglea).
Concluding remarks and perspectives
Nothing in biology makes sense except in the light of evolution (Dobzhansky, 1964; Dobzhansky, 2013). In a narrower sense and in an organismic context, I can now state that nothing in animal developmental biology makes sense except in the light of Neoplasia (cancer). But what would be the relevance of publishing a study on the Neoplastic origin of the innate immune system? What would be the influence on immunotherapy strategies? What are the new prospects for curing cancer?
Biophysicists have resumed a mechanical and physical glimpse at tumor and immune system formation and its application in therapeutic strategies in adults (Paiva et al., 2013; Stromberg and Carlson, 2013; Yu et al., 2021; Pratavieira et al., 2022). Today, in my view, there are incredible methods to evaluate textural gradients in a non-invasive way using embryological models (Zhu et al., 2023). I envision their urgent application to understand the tumor and its relationship with macrophages, aiming at the cure of cancer.
For this to happen, evolutionary biologists must first understand cancer evolution and all its developmental transitions. Knowing the origin of the immune system—so deeply rooted in Neoplasia since the emergence of the first animal—is the first step. It is also crucial to understand how the immune system has been modified throughout metazoan history for the creation of novel therapeutic strategies that involve advances in Physics from an evolutionary perspective.
Everything indicates, from an embryological point of view, that the great modifications of the immune system throughout animal evolution are linked to the great modifications of cancer throughout evolutionary history. Here, I can only briefly mention what will be described in another separate article, namely the adaptive immune system. In other words, if Neoplasia is understood as an evolutionary engine (Cofre, 2024), it is at the heart of the evolution of acquired immunity (Personal communication). Therefore, there is no malevolent alliance or artifices of cancer to circumvent the immune system, as it could not deny its own origin.
Finally, I want to ask an important question: Why don’t biologists think like Newtonian physicists? There seems to be an explanation that is associated with the wrong idea of natural selection, that is, of a slow and gradual evolution (Saunders, 1994; Gaucherel and Jensen, 2012), and that biological phenomena need historical explanations (Quenette and Gerard, 1993). As if many of the historical explanations in embryology, evolution, and cancer had no physical foundation. There is no inherent antagonism between this “physicalist” perspective and evolution, for physics acts on matter (Newman and Linde-Medina, 2013), establishing a topological map on the genomic material (Uhler and Shivashankar, 2017a; Uhler and Shivashankar, 2017b; Uhler and Shivashankar, 2018; Tsai and Crocker, 2022). From my point of view, the empowerment of physicists (Newman et al., 2006; Tiezzi, 2006; Newman and Linde-Medina, 2013; Newman, 2016) to defend the important explanatory role of Physics in biological processes such as evolution and embryology in the context of an abrupt “emergence” of animals may quickly undo the tenuous line that divided these two areas of Natural Sciences (Murray, 1992) and our way of thinking (Rowbottom, 2009).
Data availability statement
The original contributions presented in the study are included in the article/supplementary material. Further inquiries can be directed to the corresponding author.
Ethics Statement
Ethics oversight was not required for this article as we look at theoretical animals. The study was conducted under strict ethical standards, respecting the scientific literature, different opinions, and citing at least two hundred authors.
Author contributions
JC: Conceptualization, Data curation, Investigation, Writing – original draft, Writing – review & editing.
Funding
The author(s) declare that no financial support was received for the research, authorship, and/or publication of this article.
Acknowledgments
This hypothesis was inspired during my work on cancer and differentiation, conducted at the National Institute of Cancer, INCA, Rio de Janeiro, coordinated by Prof. Dr. Eliana Abdelhay. I would like to deeply thank the Institute of Anatomopathology Diagnostics, Florianópolis, Brazil, directed by Dr. José Bastos, for its incessant collaboration with my intellectual and scientific work. I also wish to honor and thank Prof. Dr. G Barry Pierce (in memoriam), Oncologist, and Prof. Dr. Stuart Newman, Physicist, whose ideas were inspiring and fundamental to the construction of this article. I offer apologies to all researchers who were not mentioned in the article, given the need to establish priorities in the article’s construction.
Conflict of interest
The author declares that the research was conducted in the absence of any commercial or financial relationships that could be construed as a potential conflict of interest.
Publisher’s note
All claims expressed in this article are solely those of the authors and do not necessarily represent those of their affiliated organizations, or those of the publisher, the editors and the reviewers. Any product that may be evaluated in this article, or claim that may be made by its manufacturer, is not guaranteed or endorsed by the publisher.
References
Akira S., Takeda K., Kaisho T. (2001). Toll-like receptors: critical proteins linking innate and acquired immunity. Nat. Immunol. 2, 675–680. doi: 10.1038/90609
Aktar R., Parkar N., Stentz R., Baumard L., Parker A., Goldson A., et al. (2020). Human resident gut microbe Bacteroides thetaiotaomicron regulates colonic neuronal innervation and neurogenic function. Gut Microbes 11, 1745–1757. doi: 10.1080/19490976.2020.1766936
Alegado R. A., Brown L. W., Cao S., Dermenjian R. K., Zuzow R., Fairclough S. R., et al. (2012). A bacterial sulfonolipid triggers multicellular development in the closest living relatives of animals. Elife 1, e00013. doi: 10.7554/eLife.00013
Allavena P., Digifico E., Belgiovine C. (2021). Macrophages and cancer stem cells: a malevolent alliance. Mol. Med. 27, 121. doi: 10.1186/s10020-021-00383-3
Anand G. M., Megale H. C., Murphy S. H., Weis T., Lin Z., He Y., et al. (2023). Controlling organoid symmetry breaking uncovers an excitable system underlying human axial elongation. Cell 186, 497–512.e23. doi: 10.1016/j.cell.2022.12.043
Anderson K. V. (2000). Toll signaling pathways in the innate immune response. Curr. Opin. Immunol. 12, 13–19. doi: 10.1016/S0952-7915(99)00045-X
Anderson K. V., Jürgens G., Nüsslein-Volhard C. (1985). Establishment of dorsal-ventral polarity in the Drosophila embryo: Genetic studies on the role of the Toll gene product. Cell 42, 779–789. doi: 10.1016/0092-8674(85)90274-0
Andrade R. P., Palmeirim I., Bajanca F. (2007). Molecular clocks underlying vertebrate embryo segmentation: A 10-year-old hairy-go-round. Birth Defects Res. Part C Embryo Today Rev. 81, 65–83. doi: 10.1002/bdrc.20094
Ao M., Brewer B. M., Yang L., Franco Coronel O. E., Hayward S. W., Webb D. J., et al. (2015). Stretching fibroblasts remodels fibronectin and alters cancer cell migration. Sci. Rep. 5, 8334. doi: 10.1038/srep08334
Arcila D., Ortí G., Vari R., Armbruster J. W., Stiassny M. L. J., Ko K. D., et al. (2017). Genome-wide interrogation advances resolution of recalcitrant groups in the tree of life. Nat. Ecol. Evol. 1, 20. doi: 10.1038/s41559-016-0020
Arnoux V., Côme C., Kusewitt D. F., Hudson L. G., Savagner P. (2005). “Cutaneous wound reepithelialization: A partial and reversible EMT,” in Rise and Fall of Epithelial Phenotype: Concepts of Epithelial-Mesenchymal Transition. Ed. Savagner P. (Boston, MA: Springer US), pp 111–pp 134.
Aronova M. Z., Alekseeva T. M. (2004). Ultrastructural identification of glial cells in the oral area of the comb-bearer Beroë cucumis. J. Evol. Biochem. Physiol. 40, 710–720. doi: 10.1007/s10893-004-0011-1
Arroyo Portilla C., Tomas J., Gorvel J.-P., Lelouard H. (2021). From species to regional and local specialization of intestinal macrophages. Front. Cell Dev. Biol. 8. doi: 10.3389/fcell.2020.624213
Auel H., Hagen W., Ekau W., Verheye H. M. (2005). Metabolic adaptations and reduced respiration of the copepod Calanoides carinatus during diapause at depth in the Angola-Benguela Front and northern Benguela upwelling regions. Afr. J. Mar. Sci. 27, 653–657. doi: 10.2989/18142320509504125
Auel H., Verheye H. M. (2007). Hypoxia tolerance in the copepod Calanoides carinatus and the effect of an intermediate oxygen minimum layer on copepod vertical distribution in the northern Benguela Current upwelling system and the Angola–Benguela Front. J. Exp. Mar. Bio Ecol. 352, 234–243. doi: 10.1016/j.jembe.2007.07.020
Bajgar A., Krejčová G. (2023). On the origin of the functional versatility of macrophages. Front. Physiol. 14. doi: 10.3389/fphys.2023.1128984
Barcus C. E., Holt E. C., Keely P. J., Eliceiri K. W., Schuler L. A. (2015). Dense collagen-I matrices enhance pro-tumorigenic estrogen-prolactin crosstalk in MCF-7 and T47D breast cancer cells. PloS One 10, e0116891. doi: 10.1371/journal.pone.0116891
Barcus C. E., O’Leary K. A., Brockman J. L., Rugowski D. E., Liu Y., Garcia N., et al. (2017). Elevated collagen-I augments tumor progressive signals, intravasation and metastasis of prolactin-induced estrogen receptor alpha positive mammary tumor cells. Breast Cancer Res. 19, 9. doi: 10.1186/s13058-017-0801-1
Belkind-Gerson J., Graham H. K., Reynolds J., Hotta R., Nagy N., Cheng L., et al. (2017). Colitis promotes neuronal differentiation of Sox2+ and PLP1+ enteric cells. Sci. Rep. 7, 2525. doi: 10.1038/s41598-017-02890-y
Benghezal M., Fauvarque M.-O., Tournebize R., Froquet R., Marchetti A., Bergeret E., et al. (2006). Specific host genes required for the killing of Klebsiella bacteria by phagocytes. Cell Microbiol. 8, 139–148. doi: 10.1111/j.1462-5822.2005.00607.x
Ben-Porath I., Thomson M. W., Carey V. J., Ge R., Bell G. W., Regev A., et al. (2008). An embryonic stem cell–like gene expression signature in poorly differentiated aggressive human tumors. Nat. Genet. 40, 499–507. doi: 10.1038/ng.127
Benton M. A., Pechmann M., Frey N., Stappert D., Conrads K. H., Chen Y.-T., et al. (2016). Toll genes have an ancestral role in axis elongation. Curr. Biol. 26, 1609–1615. doi: 10.1016/j.cub.2016.04.055
Berx G., Raspé E., Christofori G., Thiery J. P., Sleeman J. P. (2007). Pre-EMTing metastasis? Recapitulation of morphogenetic processes in cancer. Clin. Exp. Metastasis 24, 587–597. doi: 10.1007/s10585-007-9114-6
Beutler B. (2004). Inferences, questions and possibilities in Toll-like receptor signalling. Nature 430, 257–263. doi: 10.1038/nature02761
Boag T. H., Stockey R. G., Elder L. E., Hull P. M., Sperling E. A. (2018). Oxygen, temperature and the deep-marine stenothermal cradle of Ediacaran evolution. Proc. R Soc. B Biol. Sci. 285, 20181724. doi: 10.1098/rspb.2018.1724
Bonazzi M., Lecuit M., Cossart P. (2009). Listeria monocytogenes internalin and E-cadherin: from bench to bedside. Cold Spring Harb. Perspect. Biol. 1, 693–702. doi: 10.1101/cshperspect.a003087
Bosch T. C. G., McFall-Ngai M. J. (2011). Metaorganisms as the new frontier. Zoology 114, 185–190. doi: 10.1016/j.zool.2011.04.001
Boyle E. C., Finlay B. B. (2003). Bacterial pathogenesis: Exploiting cellular adherence. Curr. Opin. Cell Biol. 15, 633–639. doi: 10.1016/S0955-0674(03)00099-1
Braunstein M. J., Kucharczyk J., Adams S. (2018). Targeting toll-like receptors for cancer therapy. Target Oncol. 13, 583–598. doi: 10.1007/s11523-018-0589-7
Brennan J. J., Gilmore T. D. (2018). Evolutionary origins of toll-like receptor signaling. Mol. Biol. Evol. 35, 1576–1587. doi: 10.1093/molbev/msy050
Brennan S. T., Lowenstein T. K., Horita J. (2004). Seawater chemistry and the advent of biocalcification. Geology 32, 473–476. doi: 10.1130/G20251.1
Brennan J. J., Messerschmidt J. L., Williams L. M., Matthews B. J., Reynoso M., Gilmore T. D. (2017). Sea anemone model has a single Toll-like receptor that can function in pathogen detection, NF-κB signal transduction, and development. Proc. Natl. Acad. Sci. 114, E10122–E10131. doi: 10.1073/pnas.1711530114
Brunet T., Bouclet A., Ahmadi P., Mitrossilis D., Driquez B., Brunet A.-C., et al. (2013). Evolutionary conservation of early mesoderm specification by mechanotransduction in Bilateria. Nat. Commun. 4, 2821. doi: 10.1038/ncomms3821
Butterick C. J., Williams D. A., Boxer L. A., Jersild R. A., Mantich J. N., Higgins C., et al. (1981). Changes in energy metabolism, structure and function in alveolar macrophages under anaerobic conditions. Br. J. Haematol. 48, 523–532. doi: 10.1111/j.1365-2141.1981.tb02749.x
Candela M., Turroni S., Biagi E., Carbonero F., Rampelli S., Fiorentini C., et al. (2014). Inflammation and colorectal cancer, when microbiota-host mutualism breaks. World J. Gastroenterol. 20, 908–922. doi: 10.3748/wjg.v20.i4.908
Cao L. L., Kagan J. C. (2023). Targeting innate immune pathways for cancer immunotherapy. Immunity 56, 2206–2217. doi: 10.1016/j.immuni.2023.07.018
Chen R., Alvero A. B., Silasi D. A., Steffensen K. D., Mor G. (2008). Cancers take their Toll - The function and regulation of Toll-like receptors in cancer cells. Oncogene 27, 225–233. doi: 10.1038/sj.onc.1210907
Cho S., Irianto J., Discher D. E. (2017). Mechanosensing by the nucleus: From pathways to scaling relationships. J. Cell Biol. 216, 305–315. doi: 10.1083/jcb.201610042
Clarke M., Maddera L. (2006). Phagocyte meets prey: Uptake, internalization, and killing of bacteria by Dictyostelium amoebae. Eur. J. Cell Biol. 85, 1001–1010. doi: 10.1016/j.ejcb.2006.05.004
Cofre J. (2022). The Neoplasia as embryological phenomenon and its implication in the animal evolution and the origin of cancer. III. The role of flagellated cell fusion in the formation of the first animal and evolutionary clues to the Warburg effect (arXiv Prepr arXiv221010911).
Cofre J. (2024). The first embryo, the origin of cancer and animal phylogeny. II. The neoplastic process as an evolutionary engine. Journal of Biosciences 49, 1–27. doi: 10.1007/s12038-023-00400-z
Cofre J., Saalfeld K. (2023). The first embryo, the origin of cancer and animal phylogeny. I. A presentation of the neoplastic process and its connection with cell fusion and germline formation. Front. Cell Dev. Biol. 10. doi: 10.3389/fcell.2022.1067248
Conklin M. W., Eickhoff J. C., Riching K. M., Pehlke C. A., Eliceiri K. W., Provenzano P. P., et al. (2011). Aligned collagen is a prognostic signature for survival in human breast carcinoma. Am. J. Pathol. 178, 1221–1232. doi: 10.1016/j.ajpath.2010.11.076
Conklin M. W., Gangnon R. E., Sprague B. L., Van Gemert L., Hampton J. M., Eliceiri K. W., et al. (2018). Collagen alignment as a predictor of recurrence after ductal carcinoma in situ. Cancer Epidemiol. Biomarkers Prev. 27, 138–145. doi: 10.1158/1055-9965.EPI-17-0720
Coonfield B. R. (1936). Regeneration in Mnemiopsis leidyi, agassiz. Biol. Bull. 71, 421–428. doi: 10.2307/1537369
Cosson P., Soldati T. (2008). Eat, kill or die: when amoeba meets bacteria. Curr. Opin. Microbiol. 11, 271–276. doi: 10.1016/j.mib.2008.05.005
Cozzolino M. (2022). Proton currents through Hv1 channels characterize myeloid-derived suppressor cells. Biophys. J. 121, 247a. doi: 10.1016/j.bpj.2021.11.1480
Cozzolino M., Gyöngyösi A., Korpos E., Gogolak P., Naseem M. U., Kállai J., et al. (2023). The voltage-gated Hv1 H+ Channel is expressed in tumor-infiltrating myeloid-derived suppressor cells. Int. J. Mol. Sci. 24. doi: 10.3390/ijms24076216
da Fonseca A. C. C., Amaral R., Garcia C., Geraldo L H., Matias D., Lima F. R. S. (2016). “Microglia in cancer: for good or for bad?,” in Glial Cells in Health and Disease of the CNS. Advances in Experimental Medicine and Biology. Ed. von Bernhardi R. (Cham: Springer International Publishing), pp 245–pp 261.
Dai E. N., Heo S.-J., Mauck R. L. (2020). “Looping in” Mechanics: mechanobiologic regulation of the nucleus and the epigenome. Adv. Healthc. Mater. 9, 2000030. doi: 10.1002/adhm.202000030
Daniels C., Breitbart M. (2012). Bacterial communities associated with the ctenophores Mnemiopsis leidyi and Beroe ovata. FEMS Microbiol. Ecol. 82, 90–101. doi: 10.1111/j.1574-6941.2012.01409.x
DeCoursey T. E. (2015). The voltage-gated proton channel: A riddle, wrapped in a mystery, inside an enigma. Biochemistry 54, 3250–3268. doi: 10.1021/acs.biochem.5b00353
Desjardins M., Houde M., Gagnon E. (2005). Phagocytosis: the convoluted way from nutrition to adaptive immunity. Immunol. Rev. 207, 158–165. doi: 10.1111/j.0105-2896.2005.00319.x
Dobzhansky T. (1964). Biology, molecular and organismic. Am. Zool. 4, 443–452. doi: 10.1093/icb/4.4.443
Dobzhansky T. (2013). Nothing in biology makes sense except in the light of evolution. Am. Biol. Teach. 75, 87–91. doi: 10.2307/4444260
Drifka C. R., Loeffler A. G., Mathewson K., Keikhosravi A., Eickhoff J. C., Liu Y., et al. (2016). Highly aligned stromal collagen is a negative prognostic factor following pancreatic ductal adenocarcinoma resection. Oncotarget 7 (46). doi: 10.18632/oncotarget.12772
Dubrulle J., Pourquié O. (2002). From head to tail: links between the segmentation clock and antero-posterior patterning of the embryo. Curr. Opin. Genet. Dev. 12, 519–523. doi: 10.1016/S0959-437X(02)00335-0
Dunn J. D., Bosmani C., Barisch C., Raykov L., Lefrancois L. H., Cardenal-Munoz E., et al. (2018). Eat prey, live: Dictyostelium discoideum as a model for cell-autonomous defenses. Front. Immunol. 8, 1906. doi: 10.3389/fimmu.2017.01906
Dzobo K., Dandara C. (2023). The extracellular matrix: its composition, function, remodeling, and role in tumorigenesis. Biomimetics 8, 146. doi: 10.3390/biomimetics8020146
Edgar A., Mitchell D. G., Martindale M. Q. (2021). Whole-body regeneration in the lobate ctenophore Mnemiopsis leidyi. Genes (Basel) 12, 867. doi: 10.3390/genes12060867
Eichinger L., Pachebat J. A., Glöckner G., Rajandream M.-A., Sucgang R., Berriman M., et al. (2005). The genome of the social amoeba Dictyostelium discoideum. Nature 435, 43–57. doi: 10.1038/nature03481
Erdogan B., Ao M., White L. M., Means A. L., Brewer B. M., Yang L., et al. (2017). Cancer-associated fibroblasts promote directional cancer cell migration by aligning fibronectin. J. Cell Biol. 216, 3799–3816. doi: 10.1083/jcb.201704053
Estrella V., Chen T., Lloyd M., Wojtkowiak J., Cornnell H. H., Ibrahim-Hashim A., et al. (2013). Acidity generated by the tumor microenvironment drives local invasion. Cancer Res. 73, 1524–1535. doi: 10.1158/0008-5472.CAN-12-2796
Fedorchak G. R., Kaminski A., Lammerding J. (2014). Cellular mechanosensing: Getting to the nucleus of it all. Prog. Biophys. Mol. Biol. 115, 76–92. doi: 10.1016/j.pbiomolbio.2014.06.009
Ferreira R. M., Pereira-Marques J., Pinto-Ribeiro I., Costa J. L., Carneiro F., MacHado J. C., et al. (2018). Gastric microbial community profiling reveals a dysbiotic cancer-associated microbiota. Gut 67, 226–236. doi: 10.1136/gutjnl-2017-314205
Ferrer-Bonet M., Ruiz-Trillo I. (2017). Capsaspora owczarzaki. Curr. Biol. 27, R829–R830. doi: 10.1016/j.cub.2017.05.074
Fierro-Constaín L., Schenkelaars Q., Gazave E., Haguenauer A., Rocher C., Ereskovsky A., et al. (2017). The conservation of the germline multipotency program, from sponges to vertebrates: A stepping stone to understanding the somatic and germline origins. Genome Biol. Evol. 9, evw289. doi: 10.1093/gbe/evw289
Franc J. M. (1972). Activity and ultrastructure of the ctenophore cell rosettes. Z Zellforsch Mikrosk Anat. 130, 527–544. doi: 10.1007/BF00307005
Fu R., Shen Q., Xu P., Luo J. J., Tang Y. (2014). Phagocytosis of microglia in the central nervous system diseases. Mol. Neurobiol. 49, 1422–1434. doi: 10.1007/s12035-013-8620-6
Gatenby R. A., Gawlinski E. T. (1996). A reaction-diffusion model of cancer invasion. Cancer Res. 56, 5745–5753.
Gaucherel C., Jensen H. J. (2012). Origins of evolution: Non-acquired characters dominates over acquired characters in changing environment. J. Theor. Biol. 304, 111–120. doi: 10.1016/j.jtbi.2012.02.028
Gauthier M. E. A., Du Pasquier L., Degnan B. M. (2010). The genome of the sponge Amphimedon queenslandica provides new perspectives into the origin of Toll-like and interleukin 1 receptor pathways. Evol. Dev. 12, 519–533. doi: 10.1111/j.1525-142X.2010.00436.x
Gerardo N. M., Hoang K. L., Stoy K. S. (2020). Evolution of animal immunity in the light of beneficial symbioses. Philos. Trans. R Soc. B Biol. Sci. 375, 20190601. doi: 10.1098/rstb.2019.0601
Gerttula S., Jin Y. S., Anderson K. V. (1988). Zygotic expression and activity of the Drosophila Toll gene, a gene required maternally for embryonic dorsal-ventral pattern formation. Genetics 119, 123–133. doi: 10.1093/genetics/119.1.123
Ghosh S., Hayden M. S. (2012). Celebrating 25 years of NF-κB research. Immunol. Rev. 246, 5–13. doi: 10.1111/j.1600-065X.2012.01111.x
Gibson E. M., Monje M. (2021). Microglia in cancer therapy-related cognitive impairment. Trends Neurosci. 44, 441–451. doi: 10.1016/j.tins.2021.02.003
Gilmore T. D. (2006). Introduction to NF-κB: players, pathways, perspectives. Oncogene 25, 6680–6684. doi: 10.1038/sj.onc.1209954
Goolish E. M., Burton R. S. (1989). Energetics of osmoregulation in an intertidal copepod: effects of anoxia and lipid reserves on the pattern of free amino accumulation. Funct. Ecol. 3, 81–89. doi: 10.2307/2389678
Gotthardt D., Warnatz H. J., Henschel O., Brückert F., Schleicher M., Soldati T. (2002). High-resolution dissection of phagosome maturation reveals distinct membrane trafficking phases. Mol. Biol. Cell 13, 3508–3520. doi: 10.1091/mbc.e02-04-0206
Graham A. M., Barreto F. S. (2019). Loss of the HIF pathway in a widely distributed intertidal crustacean, the copepod Tigriopus californicus. Proc. Natl. Acad. Sci. U.S.A. 116, 12913–12918. doi: 10.1073/pnas.1819874116
Haddock S., Christianson L., Francis W., Martini S., Powers M., Dunn C., et al. (2017). Insights into the biodiversity, behavior, and bioluminescence of deep-sea organisms using molecular and maritime technology. Oceanography 30, 38–47. doi: 10.5670/oceanog.2017.422
Haney C. H., Urbach J. M., Ausubel F. M. (2014). Differences and similarities: Innate immunity in plants and animals. Biochem. (Lond.) 36, 40–44. doi: 10.1042/bio03605040
Harbison G. R., Madin L. P., Swanberg N. R. (1978). On the natural history and distribution of oceanic ctenophores. Deep Sea Res. 25, 233–256. doi: 10.1016/0146-6291(78)90590-8
Harisi R., Jeney A. (2015). Extracellular matrix as target for antitumor therapy. Onco. Targets Ther. 8, 1387–1398. doi: 10.2147/OTT.S48883
Hartenstein V., Martinez P. (2019). Phagocytosis in cellular defense and nutrition: a food-centered approach to the evolution of macrophages. Cell Tissue Res. 377, 527–547. doi: 10.1007/s00441-019-03096-6
Hartman C. D., Isenberg B. C., Chua S. G., Wong J. Y. (2017). Extracellular matrix type modulates cell migration on mechanical gradients. Exp. Cell Res. 359, 361–366. doi: 10.1016/j.yexcr.2017.08.018
Heo S. J., Thorpe S. D., Driscoll T. P., Duncan R. L., Lee D. A., Mauck R. L. (2015). Biophysical regulation of chromatin architecture instills a mechanical memory in mesenchymal stem cells. Sci. Rep. 5, 16895. doi: 10.1038/srep16895
Hernandez-Nicaise M. L. (1991). Microscopic Anatomy of Invertebrates: Placozoa, Porifera, Cnidaria, and Ctenophora (New York: Wiley).
Hibino T., Loza-Coll M., Messier C., Majeske A. J., Cohen A. H., Terwilliger D. P., et al. (2006). The immune gene repertoire encoded in the purple sea urchin genome. Dev. Biol. 300, 349–365. doi: 10.1016/j.ydbio.2006.08.065
Holle A. W., Young J. L., Spatz J. P. (2016). In vitro cancer cell–ECM interactions inform in vivo cancer treatment. Adv. Drug Delivery Rev. 97, 270–279. doi: 10.1016/j.addr.2015.10.007
Hubaud A., Regev I., Mahadevan L., Pourquié O. (2017). Excitable dynamics and Yap-dependent mechanical cues drive the segmentation clock. Cell 171, 668–682.e11. doi: 10.1016/j.cell.2017.08.043
Hughes D. T., Sperandio V. (2008). Inter-kingdom signalling: Communication between bacteria and their hosts. Nat. Rev. Microbiol. 6, 111–120. doi: 10.1038/nrmicro1836
Hunley C., Marucho M. (2022). Electrical propagation of condensed and diffuse ions along actin filaments. J. Comput. Neurosci. 50, 91–107. doi: 10.1007/s10827-021-00795-4
Jablonski D., Sepkoski J. J., Bottjer D. J., Sheehan P. M. (1983). Onshore-offshore patterns in the evolution of phanerozoic shelf communities. Science (80-) 222, 1123–1125. doi: 10.1126/science.222.4628.1123
Jager M., Chiori R., Alié A., Dayraud C., Quéinnec E., Manuel M. (2011). New insights on ctenophore neural anatomy: Immunofluorescence study in Pleurobrachia pileus (Müller 1776). J. Exp. Zool. Part B Mol. Dev. Evol. 316B, 171–187. doi: 10.1002/jez.b.21386
Jaspers C., Weiland-Bräuer N., Fischer M. A., Künzel S., Schmitz R. A., Reusch T. B. H. (2019). Microbiota differences of the comb jelly Mnemiopsis leidyi in native and invasive sub-populations. Front. Mar. Sci. 6, 635. doi: 10.3389/fmars.2019.00635
Jones B. E., Grant W. D., Duckworth A. W., Owenson G. G. (1998). Microbial diversity of soda lakes. Extremophiles 2, 191–200. doi: 10.1007/s007920050060
Juliano C. E., Swartz S. Z., Wessel G. M. (2010). A conserved germline multipotency program. Development 137, 4113–4126. doi: 10.1242/dev.047969
Kalluri R., Weinberg R. A. (2009). The basics of epithelial-mesenchymal transition. J. Clin. Invest. 119, 1420–1428. doi: 10.1172/JCI39104
Kapitonov V. V., Jurka J. (2005). RAG1 core and V (D) J recombination signal sequences were derived from Transib transposons. PloS Biol. 3, e181. doi: 10.1371/journal.pbio.0030181
Kazmierczak J., Kempe S., Kremer B. (2013). Calcium in the early evolution of living systems: A biohistorical approach. Curr. Org. Chem. 17, 1738–1750. doi: 10.2174/13852728113179990081
Kempe S., Degens E. T. (1985). An early soda ocean? Chem. Geol. 53, 95–108. doi: 10.1016/0009-2541(85)90023-3
Kerr J. R. (1999). Cell adhesion molecules in the pathogenesis of and host defence against microbial infection. J. Clin. Pathol. Mol. Pathol. 52, 220–230. doi: 10.1136/mp.52.4.220
Kigundu G., Cooper J. L., Smith S. M. E. (2018). H v 1 proton channels in dinoflagellates: not just for bioluminescence? J. Eukaryot. Microbiol. 65, 928–933. doi: 10.1111/jeu.12627
Klepstad J., Marcon L. (2023). A Clock and Wavefront Self-Organizing model explains somitogenesis in vivo and in vitro. bioRxiv 2023.01.18.524516. doi: 10.1101/2023.01.18.524516
Kottmeier D. M., Chrachri A., Langer G., Helliwell K. E., Wheeler G. L., Brownlee C. (2022). Reduced H+ channel activity disrupts pH homeostasis and calcification in coccolithophores at low ocean pH. Proc. Natl. Acad. Sci. U.S.A. 119, e2118009119. doi: 10.1073/pnas.2118009119
Krulwich T. A., Lewinson O., Padan E., Bibi E. (2005). Do physiological roles foster persistence of drug/multidrug-efflux transporters? A case study. Nat. Rev. Microbiol. 3, 566–572. doi: 10.1038/nrmicro1181
Kuebler C. A., Paré A. C. (2023). Striped expression of leucine-rich repeat proteins coordinates cell intercalation and compartment boundary formation in the early Drosophila embryo. Symmetry (Basel) 15, 1490. doi: 10.3390/sym15081490
Lee M. Y. (2022). Embryonic programs in cancer and metastasis—Insights from the mammary gland. Front. Cell Dev. Biol. 10. doi: 10.3389/fcell.2022.938625
Lemaitre B., Nicolas E., Michaut L., Reichhart J. M., Hoffmann J. A. (1996). The dorsoventral regulatory gene cassette spatzle/Toll/Cactus controls the potent antifungal response in Drosophila adults. Cell 86, 973–983. doi: 10.1016/S0092-8674(00)80172-5
Lenton T. M., Boyle R. A., Poulton S. W., Shields-Zhou G. A., Butterfield N. J. (2014). Co-evolution of eukaryotes and ocean oxygenation in the Neoproterozoic era. Nat. Geosci. 7, 257–265. doi: 10.1038/ngeo2108
Leulier F., Lemaitre B. (2008). Toll-like receptors — taking an evolutionary approach. Nat. Rev. Genet. 9, 165–178. doi: 10.1038/nrg2303
Levin L. A. (2021). “Oxygen minimum zone benthos: adaptation and community response to hypoxia,” in Oceanography and Marine Biology, An Annual Review, vol. Volume 41 . Eds. Gibson R. N., Atkinson R. J. A. (London: CRC press), pp 9–pp 9.
Levy S. B., Marshall B. (2004). Antibacterial resistance worldwide: causes, challenges and responses. Nat. Med. 10, S122–S129. doi: 10.1038/nm1145
Lewis J. S., Lee J. A., Underwood J. C. E., Harris A. L., Lewis C. E. (1999). Macrophage responses to hypoxia: relevance to disease mechanisms. J. Leukoc. Biol. 66, 889–900. doi: 10.1002/jlb.66.6.889
Leys S. P. (2015). Elements of a ‘nervous system’ in sponges. J. Exp. Biol. 218, 581–591. doi: 10.1242/jeb.110817
Leys S. P., Yahel G., Reidenbach M. A., Tunnicliffe V., Shavit U., Reiswig H. M. (2011). The sponge pump: the role of current induced flow in the design of the sponge body plan. PloS One 6, e27787. doi: 10.1371/journal.pone.0027787
Li D., Wu M. (2021). Pattern recognition receptors in health and diseases. Signal Transduct. Target Ther. 6, 291. doi: 10.1038/s41392-021-00687-0
Liberti M. V., Locasale J. W. (2016). The warburg effect: how does it benefit cancer cells? Trends Biochem. Sci. 41, 211–218. doi: 10.1016/j.tibs.2015.12.001
Linden T. A., King N. (2021). Widespread distribution of collagens and collagen-associated domains in eukaryotes. bioRxiv 2021.10.08.463732. doi: 10.1101/2021.10.08.463732
Lindsay D. J., Miyake H. (2007). A novel benthopelagic ctenophore from 7,217m depth in the Ryukyu Trench, Japan, with notes on the taxonomy of deepsea cydippids. Plankt. Benthos. Res. 2, 98–102. doi: 10.3800/pbr.2.98
Livermore D. M. (2003). Bacterial resistance: origins, epidemiology, and impact. Clin. Infect. Dis. 36, S11–S23. doi: 10.1086/344654
Lukacs G. L., Rotstein O. D., Grinstein S. (1990). Phagosomal acidification is mediated by a vacuolar-type H(+)-ATPase in murine macrophages. J. Biol. Chem. 265, 21099–21107. doi: 10.1016/S0021-9258(17)45332-4
Markert C. L. (1968). Neoplasia: A disease of cell differentiation. Cancer Res. 28, 1908–1914. doi: 10.1158/0008-5472.CAN-21-2176
Martindale M. Q., Henry J. Q. (1996). Development and regeneration of comb plates in the Ctenophore Mnemiopsis leidyi. Biol. Bull. 191, 290–292. doi: 10.1086/BBLv191n2p290
Martindale M. Q., Henry J. Q. (2015). “Ctenophora,” in Evolutionary Developmental Biology of Invertebrates. Ed. Wanninger A. (Vienna: Springer Vienna), pp 179–pp 201.
McCormack R., Podack E. R. (2015). Perforin-2/Mpeg1 and other pore-forming proteins throughout evolution. J. Leukoc. Biol. 98, 761–768. doi: 10.1189/jlb.4MR1114-523RR
McLane J. S., Ligon L. A. (2016). Stiffened extracellular matrix and signaling from stromal fibroblasts via osteoprotegerin regulate tumor cell invasion in a 3-D tumor in situ model. Cancer Microenviron. 9, 127–139. doi: 10.1007/s12307-016-0188-z
Metchnikoff E. (1893). Lectures on the comparative pathology of inflammation (London: Kegan Paul, Trench, Trubner & Co.).
Micalizzi D. S., Farabaugh S. M., Ford H. L. (2010). Epithelial-mesenchymal transition in cancer: parallels between normal development and tumor progression. J. Mammary Gland Biol. Neoplasia 15, 117–134. doi: 10.1007/s10911-010-9178-9
Mills D. B., Canfield D. E. (2017). A trophic framework for animal origins. Geobiology 15, 197–210. doi: 10.1111/gbi.12216
Mills D. B., Francis W. R., Vargas S., Larsen M., Elemans C. P. H., Canfield D. E., et al. (2018). The last common ancestor of animals lacked the HIF pathway and respired in low-oxygen environments. Elife 7, e31176. doi: 10.7554/eLife.31176
Mills D. B., Ward L. M., Jones C. A., Sweeten B., Forth M., Treusch A. H., et al. (2014). Oxygen requirements of the earliest animals. Proc. Natl. Acad. Sci. U.S.A. 111, 4168–4172. doi: 10.1073/pnas.1400547111
Miroshnikova Y. A., Mouw J. K., Barnes J. M., Pickup M. W., Lakins J. N., Kim Y., et al. (2016). Tissue mechanics promote IDH1-dependent HIF1α–tenascin C feedback to regulate glioblastoma aggression. Nat. Cell Biol. 18, 1336–1345. doi: 10.1038/ncb3429
Miroshnikova Y. A., Nava M. M., Wickström S. A. (2017). Emerging roles of mechanical forces in chromatin regulation. J. Cell Sci. 130, 2243–2250. doi: 10.1242/jcs.202192
Mittal V., El Rayes T., Narula N., McGraw T. E., Altorki N. K., Barcellos-Hoff M. H., et al. (2016). “The microenvironment of lung cancer and therapeutic implications,” in Lung Cancer and Personalized Medicine: Novel Therapies and Clinical Management. Eds. Ahmad A., Gadgeel S. M. (Cham: Springer International Publishing), pp 75–pp110.
Moellering R. E., Black K. C., Krishnamurty C., Baggett B. K., Stafford P., Rain M., et al. (2008). Acid treatment of melanoma cells selects for invasive phenotypes. Clin. Exp. Metastasis 25, 411–425. doi: 10.1007/s10585-008-9145-7
Mongera A., Michaut A., Guillot C., Xiong F., Pourquié O. (2019). Mechanics of anteroposterior axis formation in vertebrates. Annu. Rev. Cell Dev. Biol. 35, 259–283. doi: 10.1146/annurev-cellbio-100818-125436
Mori M., Somogyi K., Kondo H., Monnier N., Falk H. J., Machado P., et al. (2014). An Arp2/3 nucleated F-actin shell fragments nuclear membranes at nuclear envelope breakdown in starfish oocytes. Curr. Biol. 24, 1421–1428. doi: 10.1016/j.cub.2014.05.019
Moroz L. L., Kocot K. M., Citarella M. R., Dosung S., Norekian T. P., Povolotskaya I. S., et al. (2014). The ctenophore genome and the evolutionary origins of neural systems. Nature 510, 109–114. doi: 10.1038/nature13400
Murray B. G. (1992). Research methods in physics and biology. Oikos 64, 594–596. doi: 10.2307/3545180
Nakaya Y., Sheng G. (2013). EMT in developmental morphogenesis. Cancer Lett. 341, 9–15. doi: 10.1016/j.canlet.2013.02.037
Neumann R. (1979). Bacterial induction of settlement and metamorphosis in the planula larvae of Cassiopea andromeda (Cnidaria: Scyphozoa, Rhizostomeae). Mar. Ecol. Prog. Ser. 1, 21–28. doi: 10.3354/meps001021
Newman S. A. (2016). “Multicellularity, the emergence of animal body plans, and the stabilizing role of the egg,” in Multicellularity: origins and evolution. Eds. Niklas K. J., Newman S. A. (Cambridge, Massachusetts: MIT Press), pp 225–pp 246.
Newman S. A., Forgacs G., Müller G. B. (2006). Before programs: The physical origination of multicellular forms. Int. J. Dev. Biol. 50, 289–299. doi: 10.1387/ijdb.052049sn
Newman S. A., Linde-Medina M. (2013). Physical determinants in the emergence and inheritance of multicellular form. Biol. Theory 8, 274–285. doi: 10.1007/s13752-013-0116-0
Ng A. C. Y., Eisenberg J. M., Heath R. J. W., Huett A., Robinson C. M., Nau G. J., et al. (2011). Human leucine-rich repeat proteins: a genome-wide bioinformatic categorization and functional analysis in innate immunity. Proc. Natl. Acad. Sci. 108, 4631–4638. doi: 10.1073/pnas.1000093107
Nguyen T. V., Sleiman M., Moriarty T., Nguyen T. V., Sleiman M., Moriarty T., et al. (2014). Sorafenib resistance and JNK signaling in carcinoma during extracellular matrix stiffening. Biomaterials 35, 5749–5759. doi: 10.1016/j.biomaterials.2014.03.058
Nieponice A., McGrath K., Qureshi I., Beckman E. J., Luketich J. D., Gilbert T. W., et al. (2009). An extracellular matrix scaffold for esophageal stricture prevention after circumferential EMR. Gastrointest. Endosc. 69, 289–296. doi: 10.1016/j.gie.2008.04.022
Nunes P., Demaurex N., Dinauer M. C. (2013). Regulation of the NADPH oxidase and associated ion fluxes during phagocytosis. Traffic 14, 1118–1131. doi: 10.1111/tra.12115
Okochi Y., Sasaki M., Iwasaki H., Okamura Y. (2009). Voltage-gated proton channel is expressed on phagosomes. Biochem. Biophys. Res. Commun. 382, 274–279. doi: 10.1016/j.bbrc.2009.03.036
Okuno T., Li W. Y., Hatano Y., Takasu A., Sakamoto Y., Yamamoto M., et al. (2020). Zygotic nuclear F-actin safeguards embryonic development. Cell Rep. 31, 107824. doi: 10.1016/j.celrep.2020.107824
Ostuni R., Kratochvill F., Murray P. J., Natoli G. (2015). Macrophages and cancer: from mechanisms to therapeutic implications. Trends Immunol. 36, 229–239. doi: 10.1016/j.it.2015.02.004
Padan E., Bibi E., Ito M., Krulwich T. A. (2005). Alkaline pH homeostasis in bacteria: New insights. Biochim. Biophys. Acta Biomembr. 1717, 67–88. doi: 10.1016/j.bbamem.2005.09.010
Paiva L. R., Silva H. S., Ferreira S. C., Martins M. L. (2013). Multiscale model for the effects of adaptive immunity suppression on the viral therapy of cancer. Phys. Biol. 10, 25005. doi: 10.1088/1478-3975/10/2/025005
Pajic-Lijakovic I., Milivojevic M. (2022). Mechanical waves caused by collective cell migration: generation. Eur. Biophys. J. 51, 1–13. doi: 10.1007/s00249-021-01581-x
Park J., Kim D.-H., Levchenko A. (2018). Topotaxis: A new mechanism of directed cell migration in topographic ECM gradients. Biophys. J. 114, 1257–1263. doi: 10.1016/j.bpj.2017.11.3813
Patthy L. (1999). Genome evolution and the evolution of exon-shuffling — a review. Gene 238, 103–114. doi: 10.1016/S0378-1119(99)00228-0
Pierce G. B. (1983). The cancer cell and its control by the embryo. Rous-Whipple Award lecture. Am. J. Pathol. 113, 117.
Pierce G. B., Pantazis C. G., Caldwell J. E., Wells R. S. (1982). Specificity of the control of tumor formation by the blastocyst. Cancer Res. 42, 1082–1087.
Pierce G. B., Wallace C. (1971). Differentiation of Malignant to benign cells. Cancer Res. 31, 127–134.
Poltorak A., He X., Smirnova I., Liu M.-Y., Huffel C., van Du X., et al. (1998). Defective LPS signaling in C3H/HeJ and C57BL/10ScCr mice: mutations in Tlr4 gene. Science (80-) 282, 2085–2088. doi: 10.1126/science.282.5396.2085
Pourquié O. (2003). The segmentation clock: converting embryonic time into spatial pattern. Science (80-) 301, 328–330. doi: 10.1126/science.1085887
Pradere J. P., Dapito D. H., Schwabe R. F. (2014). The Yin and Yang of Toll-like receptors in cancer. Oncogene 33, 3485–3495. doi: 10.1038/onc.2013.302
Pratavieira S., Requena M. B., Stringasci M. D., Ayala E. T. P., Bagnato V. S. (2022). The physics of light and sound in the fight against skin cancer. Braz. J. Phys. 52, 106. doi: 10.1007/s13538-022-01121-8
Presnell J. S., Vandepas L. E., Warren K. J., Swalla B. J., Amemiya C. T., Browne W. E., et al. (2016). The presence of a functionally tripartite through-gut in ctenophora has implications for metazoan character trait evolution. Curr. Biol. 26, 2814–2820. doi: 10.1016/j.cub.2016.08.019
Pukhlyakova E., Aman A. J., Elsayad K., Technau U. (2018). β-Catenin-dependent mechanotransduction dates back to the common ancestor of Cnidaria and Bilateria. Proc. Natl. Acad. Sci. U.S.A. 115, 6231–6236. doi: 10.1073/pnas.1713682115
Quenette P. Y., Gerard J. F. (1993). Why biologists do not think like Newtonian physicists. Oikos 68, 361. doi: 10.2307/3544852
Rakoff-Nahoum S., Medzhitov R. (2009). Toll-like receptors and cancer. Nat. Rev. Cancer 9, 57–63. doi: 10.1038/nrc2541
Reshef L., Koren O., Loya Y., Zilber-Rosenberg I., Rosenberg E.. (2006). The coral probiotic hypothesis. Environ. Microbiol. 8, 2068–2073. doi: 10.1111/j.1462-2920.2006.01148.x
Ribatti D., Tamma R., Annese T. (2020). Epithelial-mesenchymal transition in cancer: A historical overview. Transl. Oncol. 13, 100773. doi: 10.1016/j.tranon.2020.100773
Richter D. J., Fozouni P., Eisen M. B., King N. (2018). Gene family innovation, conservation and loss on the animal stem lineage. Elife 7, e34226. doi: 10.7554/eLife.34226
Richter D. J., King N. (2013). The genomic and cellular foundations of animal origins. Annu. Rev. Genet. 47, 509–537. doi: 10.1146/annurev-genet-111212-133456
Rodriguez J. D., Haq S., Bachvaroff T., Nowak K. F., Nowak S. J., Morgan D., et al. (2017). Identification of a vacuolar proton channel that triggers the bioluminescent flash in dinoflagellates. PloS One 12, e0171594. doi: 10.1371/journal.pone.0171594
Rofstad E. K., Mathiesen B., Kindem K., Galappathi K. (2006). Acidic extracellular pH promotes experimental metastasis of human melanoma cells in athymic nude mice. Cancer Res. 66, 6699–6707. doi: 10.1158/0008-5472.CAN-06-0983
Rook G., Bäckhed F., Levin B. R., McFall-Ngai M. J., McLean A. R. (2017). Evolution, human-microbe interactions, and life history plasticity. Lancet 390, 521–530. doi: 10.1016/S0140-6736(17)30566-4
Rosenberg E., Sharon G., Zilber-Rosenberg I. (2009). The hologenome theory of evolution contains Lamarckian aspects within a Darwinian framework. Environ. Microbiol. 11, 2959–2962. doi: 10.1111/j.1462-2920.2009.01995.x
Ros-Rocher N., Pérez-Posada A., Leger M. M., Ruiz-Trillo I. (2021). The origin of animals: an ancestral reconstruction of the unicellular-to-multicellular transition. Open Biol. 11, rsob.200359. doi: 10.1098/rsob.200359
Roszko I., Sawada A., Solnica-Krezel L. (2009). Regulation of convergence and extension movements during vertebrate gastrulation by the Wnt/PCP pathway. Semin. Cell Dev. Biol. 20, 986–997. doi: 10.1016/j.semcdb.2009.09.004
Rowbottom D. P. (2009). Models in biology and physics: What’s the difference? Found Sci. 14, 281–294. doi: 10.1007/s10699-009-9160-4
Ryan J. F., Pang K., Schnitzler C. E., Nguyen A-D., Moreland R. T., Simmons D. K., et al. (2013). The genome of the ctenophore Mnemiopsis leidyi and its implications for cell type evolution. Science (80-) 342, 1242592. doi: 10.1126/science.1242592
Sansonetti P. J., Mounier J., Prévost M. C., Mège R.-M. (1994). Cadherin expression is required for the spread of Shigella flexneri between epithelial cells. Cell 76, 829–839. doi: 10.1016/0092-8674(94)90358-1
Saunders P. T. (1994). Evolution without natural selection: further implications of the daisyworld parable. J. Theor. Biol. 166, 365–373. doi: 10.1006/jtbi.1994.1033
Savagner P. (2001). Leaving the neighborhood: molecular mechanisms involved during epithelial-mesenchymal transition. Bioessays 23, 912–923. doi: 10.1002/bies.1132
Schlappack O. K., Zimmermann A., Hill R. P. (1991). Glucose starvation and acidosis: effect on experimental metastatic potential, DNA content and MTX resistance of murine tumour cells. Br. J. Cancer 64, 663–670. doi: 10.1038/bjc.1991.378
Sebé-Pedrós A., de Mendoza A., Lang B. F., Degnan B. M., Ruiz-Trillo I. (2011). Unexpected repertoire of metazoan transcription factors in the unicellular holozoan capsaspora owczarzaki. Mol. Biol. Evol. 28, 1241–1254. doi: 10.1093/molbev/msq309
Shen X.-X., Hittinger C. T., Rokas A. (2017). Contentious relationships in phylogenomic studies can be driven by a handful of genes. Nat. Ecol. Evol. 1, 126. doi: 10.1038/s41559-017-0126
Shi M., Chen X., Ye K., Yao Y., Li Y. (2016). Application potential of toll-like receptors in cancer immunotherapy. Med. (United States) 95. doi: 10.1097/MD.0000000000003951
Shi W., Mills B. J. W., Li C., Poulton S. W., Krause A. J., He T., et al. (2022). Decoupled oxygenation of the Ediacaran ocean and atmosphere during the rise of early animals. Earth Planet Sci. Lett. 591, 117619. doi: 10.1016/j.epsl.2022.117619
Skriwan C., Fajardo M., Hägele S., Horn M., Wagner M., Michel R., et al. (2002). Various bacterial pathogens and symbionts infect the amoeba Dictyostelium discoideum. Int. J. Med. Microbiol. 291, 615–624. doi: 10.1078/1438-4221-00177
So E. Y., Ouchi T. (2010). The application of toll like receptors for cancer therapy. Int. J. Biol. Sci. 6, 675–681. doi: 10.7150/ijbs.6.675
Sobhani I., Bergsten E., Couffin S., Amiot A., Nebbad B., Barau C., et al. (2019). Colorectal cancer-associated microbiota contributes to oncogenic epigenetic signatures. Proc. Natl. Acad. Sci. U.S.A. 116, 24285–24295. doi: 10.1073/pnas.1912129116
Stromberg S. P., Carlson J. M. (2013). Diversity of T-cell responses. Phys. Biol. 10, 25002. doi: 10.1088/1478-3975/10/2/025002
Suga H., Chen Z., de Mendoza A., Sebé-Pedrós A., Brown M W., Kramer E., et al. (2013). The Capsaspora genome reveals a complex unicellular prehistory of animals. Nat. Commun. 4, 2325. doi: 10.1038/ncomms3325
Suga H., Dacre M., de Mendoza A., Shalchian-Tabrizi K., Manning G., Ruiz-Trillo I. (2012). Genomic survey of premetazoans shows deep conservation of cytoplasmic tyrosine kinases and multiple radiations of receptor tyrosine kinases. Sci. Signal 5, ra35–ra35. doi: 10.1126/scisignal.2002733
Sunyer R., Conte V., Escribano J., Elosegui-Artola A., Labernadie A., Valon L., et al. (2016). Collective cell durotaxis emerges from long-range intercellular force transmission. Science (80-) 353, 1157–1161. doi: 10.1126/science.aaf7119
Tamm S. L. (2012). Regeneration of ciliary comb plates in the ctenophore Mnemiopsis leidyi. i. Morphology. J. Morphol 273, 109–120. doi: 10.1002/jmor.11016
Tamm S. L., Tamm S. (1991). Reversible epithelial adhesion closes the mouth of beroe, a carnivorous marine jelly. Biol. Bull. 181, 463–473. doi: 10.2307/1542367
Tamm S. L., Tamm S. (1993). Dynamic control of cell-cell adhesion and membrane-associated actin during food-induced mouth opening in Beroe. J. Cell Sci. 106, 355–364. doi: 10.1242/jcs.106.1.355
Tao X., Huang Z., Chen F., Wang X., Zheng T., Yuan S., et al. (2022). The RAG key to vertebrate adaptive immunity descended directly from a bacterial ancestor. Natl. Sci. Rev. 9, nwac073. doi: 10.1093/nsr/nwac073
Taylor A. R., Chrachri A., Wheeler G., Goddard H., Brownlee C. (2011). A voltage-gated H+ Channel underlying pH homeostasis in calcifying coccolithophores. PloS Biol. 9, e1001085. doi: 10.1371/journal.pbio.1001085
Tebben J., Tapiolas D. M., Motti C. A., Goddard H., Brownlee C. (2011). Induction of larval metamorphosis of the coral Acropora millepora by tetrabromopyrrole isolated from a Pseudoalteromonas bacterium. PloS One 6, e19082. doi: 10.1371/journal.pone.0019082
Thiery J. P., Acloque H., Huang R. Y. J., Nieto M. A. (2009). Epithelial-mesenchymal transitions in development and disease. Cell 139, 871–890. doi: 10.1016/j.cell.2009.11.007
Thiery J. P., Sleeman J. P. (2006). Complex networks orchestrate epithelial–mesenchymal transitions. Nat. Rev. Mol. Cell Biol. 7, 131–142. doi: 10.1038/nrm1835
Towe K. M. (1970). Oxygen-collagen priority and the early metazoan fossil record. Proc. Natl. Acad. Sci. 65, 781–788. doi: 10.1073/pnas.65.4.781
Traylor-Knowles N., Vandepas L. E., Browne W. E. (2019). Still enigmatic: innate immunity in the ctenophore Mnemiopsis leidyi. Integr. Comp. Biol. 59, 811–818. doi: 10.1093/icb/icz116
Truchot J.-P., Duhamel-Jouve A. (1980). Oxygen and carbon dioxide in the marine intertidal environment: Diurnal and tidal changes in rockpools. Respir. Physiol. 39, 241–254. doi: 10.1016/0034-5687(80)90056-0
Tsai A., Crocker J. (2022). Nuclear morphogenesis: forming a heterogeneous nucleus during embryogenesis. Development 149, dev200266. doi: 10.1242/dev.200266
Uhler C., Shivashankar G. V. (2017a). Regulation of genome organization and gene expression by nuclear mechanotransduction. Nat. Rev. Mol. Cell Biol. 18, 717–727. doi: 10.1038/nrm.2017.101
Uhler C., Shivashankar G. V. (2017b). Chromosome intermingling: mechanical hotspots for genome regulation. Trends Cell Biol. 27, 810–819. doi: 10.1016/j.tcb.2017.06.005
Uhler C., Shivashankar G. V. (2018). Nuclear mechanopathology and cancer diagnosis. Trends Cancer 4, 320–331. doi: 10.1016/j.trecan.2018.02.009
Valentine J. W., Marshall C. R. (2015). Fossil and Transcriptomic Perspectives on the Origins and Success of Metazoan Multicellularity BT - Evolutionary Transitions to Multicellular Life: Principles and mechanisms. Eds. Ruiz-Trillo I., Nedelcu A. M. (Dordrecht: Springer Netherlands), pp 31–pp 46.
Vandepas L. E., Warren K. J., Amemiya C. T., Browne W. E. (2017). Establishing and maintaining primary cell cultures derived from the ctenophore Mnemiopsis leidyi. J. Exp. Biol. 220, 1197–1201. doi: 10.1242/jeb.152371
Verheye H. M., Hagen W., Auel H., Ekau W., Loick N., Rheenen I., et al. (2005). Life strategies, energetics and growth characteristics of Calanoides carinatus (Copepoda) in the Angola-Benguela frontal region. Afr. J. Mar. Sci. 27, 641–651. doi: 10.2989/18142320509504124
Vicentini F. A., Fahlman T., Raptis S. G., Wallace L. E., Hirota S. A., Sharkey K. A. (2022). “New concepts of the interplay between the gut microbiota and the enteric nervous system in the control of motility,” in The Enteric Nervous System II. Advances in Experimental Medicine and Biology. Eds. Spencer N. J., Costa M., Brierley S. M. (Cham: Springer International Publishing), pp 55–pp 69.
Wang Y., Nagarajan M., Uhler C., Shivashankar G. V. (2017). Orientation and repositioning of chromosomes correlate with cell geometry–dependent gene expression. Mol. Biol. Cell 28, 1997–2009. doi: 10.1091/mbc.e16-12-0825
Warburg O. (1956). On the origin of cancer cells. Science (80-) 123, 309–314. doi: 10.1126/science.123.3191.30
Wesolowska N., Avilov I., MaChado P., Geiss C., Kondo H., Mori M., et al. (2020). Actin assembly ruptures the nuclear envelope by prying the lamina away from nuclear pores and nuclear membranes in starfish oocytes. Elife 9, e49774. doi: 10.7554/eLife.49774
Whelan N. V., Kocot K. M., Moroz T. P., Mukherjee K., Williams P., Paulay G., et al. (2017). Ctenophore relationships and their placement as the sister group to all other animals. Nat. Ecol. Evol. 1, 1737–1746. doi: 10.1038/s41559-017-0331-3
Williams L. M., Gilmore T. D. (2020). Looking down on NF- κ B. Mol. Cell Biol. 40, e00104–e00120. doi: 10.1128/MCB.00104-20
Williams L. M., Sridhar S., Samaroo J., Peart J., Adindu E. K., Addanki A., et al. (2021). Comparison of NF-κB from the protists Capsaspora owczarzaki and Acanthoeca spectabilis reveals extensive evolutionary diversification of this transcription factor. Commun. Biol. 4, 1404. doi: 10.1038/s42003-021-02924-2
Wood R., Liu A. G., Bowyer F., Wilby P. R., Dunn F. S., Kenchington C. G., et al. (2019). Integrated records of environmental change and evolution challenge the Cambrian Explosion. Nat. Ecol. Evol. 3, 858–858. doi: 10.1038/s41559-019-0897-z
Woznica A., Gerdt J. P., Hulett R. E., Clardy J., King N. (2017). Mating in the closest living relatives of animals is induced by a bacterial chondroitinase. Cell 170, 1175–1183.e11. doi: 10.1016/j.cell.2017.08.005
Xu T., Liu C., Deng S., Gan L., Zhang Z., Yang G.-Y., et al. (2022). The roles of microglia and astrocytes in myelin phagocytosis in the central nervous system. J. Cereb Blood Flow Metab. 43, 325–340. doi: 10.1177/0271678X221137762
Yaman Y. I., Ramanathan S. (2023). Controlling human organoid symmetry breaking reveals signaling gradients drive segmentation clock waves. Cell 186, 513–527.e19. doi: 10.1016/j.cell.2022.12.042
Yang N., Friedl A. (2016). Syndecan-1-induced ECM fiber alignment requires integrin αvβ3 and syndecan-1 ectodomain and heparan sulfate chains. PloS One 11, e0150132. doi: 10.1371/journal.pone.0150132
Yu C. C., Wortman J. C., He T.-F., Solomon S., Zhang R. Z., Rosario A., et al. (2021). Physics approaches to the spatial distribution of immune cells in tumors. Rep. Prog. Phys. 84, 22601. doi: 10.1088/1361-6633/abcd7b
Yu X., Xu J. (2020). A ‘Goldmine’ for digging cancer-specific targets: The genes essential for embryo development but non-essential for adult life. J. Mol. Cell Biol. 12, 669–673. doi: 10.1093/jmcb/mjaa024
Zhang X., Liu Q., Liao Q., Zhao Y. (2020). Pancreatic cancer, gut microbiota, and therapeutic efficacy. J. Cancer 11, 2749. doi: 10.7150/jca.37445
Zheng B., Han M., Bernier M., Wen J. (2009). Nuclear actin and actin-binding proteins in the regulation of transcription and gene expression. FEBS J. 276, 2669–2685. doi: 10.1111/j.1742-4658.2009.06986.x
Zhu M., Gu B., Thomas E., Tao H., Yung T. M., Zhang K., et al. (2023). Durotaxis bridges phase transition as a function of tissue stiffness in vivo. bioRxiv 2023.01.05.522913. doi: 10.1101/2023.01.05.522913
Keywords: cancer, neoplasia, evolution, embryology, metazoa, unicellular holozoa, evolutionary radiation, co-option
Citation: Cofre J (2024) The first embryo, the origin of cancer and animal phylogeny. IV. The neoplastic basis for the formation of the innate immune system. Front. Ecol. Evol. 11:1260930. doi: 10.3389/fevo.2023.1260930
Received: 31 July 2023; Accepted: 20 December 2023;
Published: 24 January 2024.
Edited by:
Deirdre Lyons, University of California, San Diego, United StatesReviewed by:
Jonathan P. Rast, Emory University, United StatesAssunta Liberti, Anton Dohrn Zoological Station Naples, Italy
Copyright © 2024 Cofre. This is an open-access article distributed under the terms of the Creative Commons Attribution License (CC BY). The use, distribution or reproduction in other forums is permitted, provided the original author(s) and the copyright owner(s) are credited and that the original publication in this journal is cited, in accordance with accepted academic practice. No use, distribution or reproduction is permitted which does not comply with these terms.
*Correspondence: Jaime Cofre, amFpbWUuY29mcmVAdWZzYy5icg==