- 1Department of Ecology and Evolutionary Biology, Princeton University, Princeton, NJ, United States
- 2Santa Fe Institute, Santa Fe, NM, United States
- 3Smithsonian Tropical Research Institute, Panama City, Panama
- 4Department of Life and Environmental Sciences, University of California, Merced, Merced, CA, United States
- 5Biology Department, Leeds University, Leeds, United Kingdom
- 6Cary Institute of Ecosystem Studies, Millbrook, NY, United States
Recent theoretical work has provided major new insights into the ways that species interactions in food webs are organized in ways that permit the coexistence of significant numbers of species. But, we seem to have forgotten about trees! Not the phylogenetic ones that are increasingly important for dissecting the evolutionary structure of food webs, but the trees, shrubs and grasses that are the basal species in all terrestrial ecosystems. Many of the food webs available for analysis over the last 30 years were based on freshwater or marine systems where algae were the main plants. Trees are very different from algae; they can live for centuries, while annually producing leaves, fruits and seeds that provide nutrients for a diversity of species on higher trophic levels. In sharp contrast to algae, they are only partly consumed by herbivores and usually compensate or recover from herbivory. Most of the biomass in terrestrial systems is in the plants, this again contrasts with aquatic systems, where most of the biomass is in primary and secondary consumers. Moreover, each individual tree supports its own food web of species that are only partially coupled to those of surrounding trees. If we are going to apply our theoretical understanding of food-web structure to species-rich terrestrial ecosystems in ways that are insightful for conservation, then we need a deeper examination of the role that higher plants play in food webs. While community ecology has developed an increasingly detailed understanding of the ways plant communities are organized, this seems to have evolved almost independently of the food-web literature. In this article, we make a plea to more sharply consider higher plants in food webs and to do this by combining recent theoretical work on food webs, with recent empirical and theoretical work on plant communities. Ultimately, we argue for a deeper integration of plant community ecology into studies of food webs.
Neutral, omniscient and basal
If you walk around Windsor Great Park in the south of England, you are surrounded by some of Britain’s oldest inhabitants. Not the British Royal Family, who live on the Estate, but the oak trees that have lived in the park since Henry VIII wooed his multiple wives here, trees that were already established when William the Conqueror and the Normans arrived in England in 1066 (Figure 1 - Green, pers comm). Trees are some of the oldest organisms on the planet, if we go to the western United States, we can find Bristlecone pines that are almost 5,000 years old (Brown, 1996); not far away in Sierra are Giant Sequoia’s that are several thousand years old and the largest organisms on the planet. In the Namibian desert, Welwitschia plants can be thousands of years old with same two leaves they first sprouted (Herre, 1961); Figure 1); in Sri Lanka, Jaya Sri Maha Bodhi—a sacred fig (Ficus religiosa) and the oldest planted tree—has stood since 288 BC (Ram, 2016). Simply seeing trees as perches, dens, or nest sites for the mammals and birds that live on, and in the tree, massively underestimates the foundational role that individual trees play for the multiple generations of species that they support. All ancient trees, and indeed, all higher plants are host to a community of fungi, bacteria, insects and nematodes that form a network of organisms that feeds on, or exchanges nutrients with the tree (Hardoim et al., 2015) (Parihar et al., 2020; Hawkins et al., 2023). Most trees sustain their own food web of diverse organisms that feed on, or in, them (Price, 2002). Ultimately, the food webs of forests are meta-webs, where each individual tree is a node that hosts its own sub-web of multi-species interactions. The long life of trees and the relatively short life of species that use them as resources, and the even shorter lives of the fungi and bacteria that form their microbiome, makes it unlikely that two trees in the same forest support identical food webs. Furthermore, the mature stages of most individual trees live longer than the careers of the researchers who study them! Indeed, some of the trees mentioned above are older than most religions and the evolutionary origin of conifers predates most terrestrial vertebrates! (Farjon, 2008). Does this longevity provide an underappreciated level of stability to terrestrial ecosystems? And does this ineluctably constrain the persistence of terrestrial animal and microbial communities to the survival of the trees and grasses that contain most of the biomass in terrestrial ecosystems?
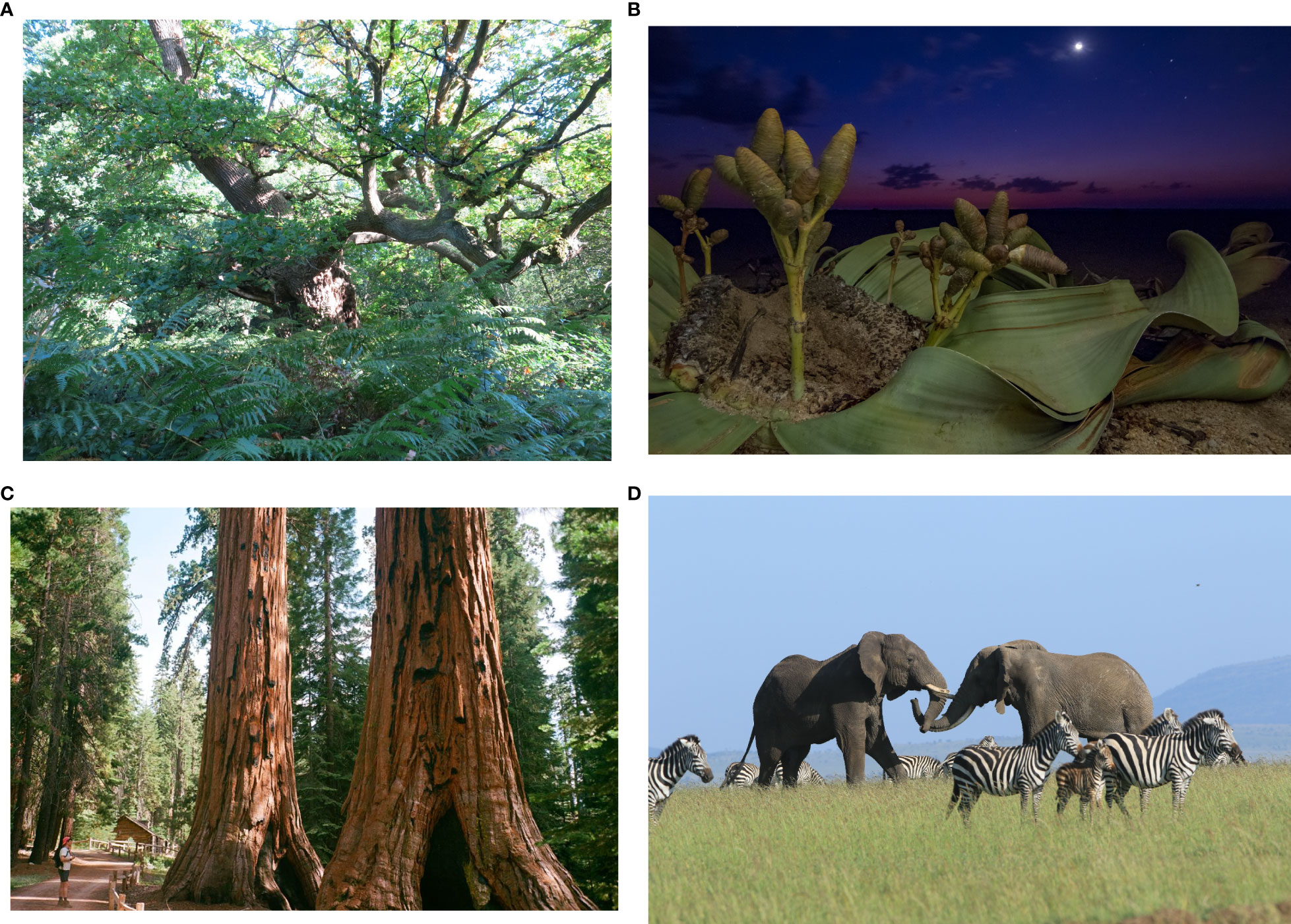
Figure 1 Three long-lived trees. (A) Oak tree in Windsor Great Park (Photo by Andy Dobson). (B) Welwitschia in the Namibian desert (Photo by Jennifer Guyton). (C) Giant Sequoia in the Mariposa Grove of Giant Sequoias, California (Photo Allegra Dobson). (D) Elephants and Zebra in Serengeti National Park surrounded by grasses that annually regrow from deep roots to support a large diversity and biomass of ungulates (Photo Andy Dobson).
Trees are some of the most ubiquitous organisms on Earth; a recent study estimates there are around 3.04 trillion trees on the planet (Crowther et al., 2015), just under half of these (1.39 Trillion) are in tropical and subtropical forests, the rest are split almost equally between boreal (0.74T) and temperate regions (0.61T); each tree contains a food web of interacting fungal, bacterial, insect and nematode species, and acts as a discrete patch in the habitats and territories of many bird and mammal species. Most individual plants have a web of mycorrhizal fungi associated with them and this may contain a significant proportion of the carbon dioxide scrubbed from the atmosphere by the tree A recent review estimates that as much as 30% of the carbon captured by trees from the atmosphere is stored in these fungal mutualists (Hawkins et al., 2023). Although individual trees can be incredibly long-lived, recent studies of data from long-term tropical forest plots show significant turnover in the populations of individual trees on multi-decadal timescales (Chisholm et al., 2014). A beguiling pattern underlies these data: variation in the population size of abundant trees tends to be driven by environmental variation (most likely climate and natural enemies), while variation in the abundance of rarer trees is dominated by demographic stochasticity. Presumably the food web associated with more abundant trees can persist as meta-communities whose species continuously recruit into the adult populations from the population of younger trees waiting to develop in the understory, or as seeds in the soil seed bank. These age-structured effects could also operate in rarer trees where higher levels of variability in abundance may compensate for the reduced probability of specific fungal, insect, and nematode species locating their host.
At a macro-ecological scale, it is also intriguing to ask how these patterns change from species-rich rainforests to species-poor boreal forests where the biomes associated with each tree are likely less dissimilar, than those associated with individual tropical trees. Likewise, the food webs associated with individuals trees in closed canopy forests may be more similar than the food webs associated to widely spaced savanna trees. What are the dynamic and structural consequences for food webs of switching our botanical perspective from algae in ponds, to oaks, yews, figs, Bristlecone pines in forests, and grasses in savannas? Recent work on forces that determine the stability of food webs has emphasized the role of long-lived species that create slow dynamics where changes in abundance occur on timescales that are orders of magnitude slower than for consumer species in the web (McCann et al., 2005; Rooney et al., 2006; McCann, 2011). Trees canonically fill this role in the food web dynamics of tropical, temperate and boreal forests; perennial grasses play an analogous role in savannas. While there is a rapid turnover and variance in the abundance of early life stages—fruits, seeds and seedlings are produced on a regular annual basis, or occasionally at longer time intervals in masting species.—The longevity of adult mature trees consistently and predictably generates, on a time scale of decades to centuries, spatially local resources for species on higher tropic levels that feed on fruits, seeds, and leaves, as well as for the decomposer community that feeds on fallen leaves and branches (Table 1). Variation in annual levels of productivity is partially offset by interactions between plants competing for space, nutrients, water, and sunlight (Tilman, 1982; Tilman, 1988). While this initially creates problems for stability from a theoretical food web perspective, as explicit competition is destabilizing (Loreau and De Mazancourt, 2013), these problems could be surmounted if light, water and nutrients were included as additional ‘limiting factors’ in the web, competition between plants would then be explicitly resource based (Allesina and Tang, 2012; Tang et al., 2014; Allesina and Tang, 2015).
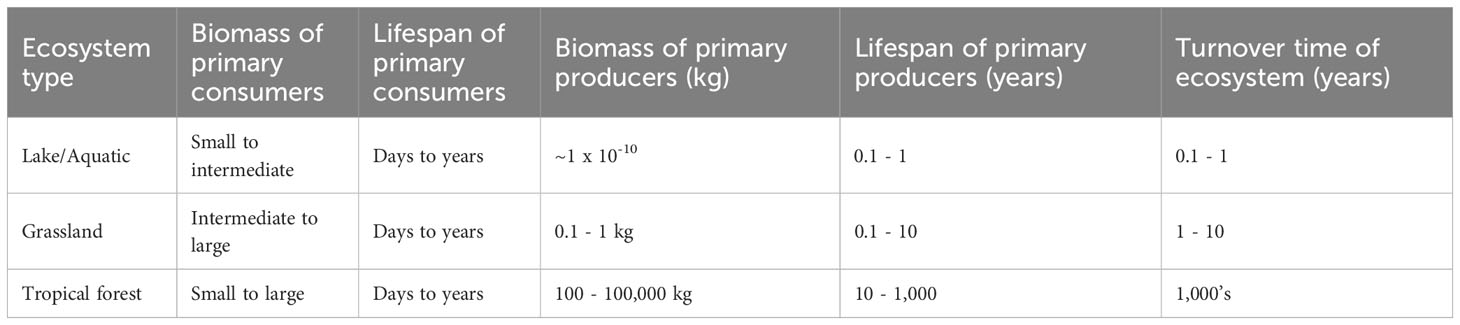
Table 1 Table comparing the biomass of plants in different ecosystems and relative timescales at which plant demography and ecosystem succession operate.
Food web models
Are there more explicit ways of dealing with the longevity of mature plants in food web models? Could this be captured by age- or stage-structured models that allow long-lived mature trees to compete slowly for light, water and nutrients, while supplying a variable annual supply of leaves, sap, fruits, pollen and seed stages to the consumers that form the rest of the web? Ultimately, this suggests we should use models of plant communities to provide a basal layer to models of terrestrial food webs. We could then add on top of these the faster and more ephemeral interactions between plant reproduction and animal, bacterial and fungal consumer species? Although some site-specific computer food-web models take this approach, it is missing from the more analytical models based on random networks (Box 1). Ultimately what happens at the base of the web determines the dynamics of what happens in higher trophic levels; so one major route that food web studies need to develop is to integrate the consumer-resource parts of food-web models with models for dynamics of plant communities (Tilman, 1982; Tilman, 1988; Hubbell, 2001).
The species composition and spatial distribution of the underlying plant community is the primary determinant of the foraging/grouping structure of the herbivore community, whose feeding activities interact with local soil and climate conditions to shape the local plant community. Most insects, birds and mammals display strong preferences for the plants that they feed upon (Fine et al., 2004; Hutchinson et al., 2022). In savanna ecosystems, vertebrate herbivores can quickly be divided into browsers and grazers, these will in turn divide the habitat into open areas of grassland and bush with tree density increasing in the occasional riparian areas. For example, the Serengeti grasslands are divided into different plant communities of short and long grasslands, woodlands and kopjes that have their own fairly well-delineated groups of herbivores that feed on them (Baskerville et al., 2011). These compete seasonally with the larger migratory herbivores (wildebeest, zebra, and elephants) that only use each of these communities for a few months each year (Figure 2). In turn, the herbivore communities in any location have both specialized local and generalized more widely spread carnivores that feed on them. Spatial patterns in tropical forests will be more complex, individual trees will have sharply defined insect webs closely associated with them that are coupled to the webs of other trees by the generalist birds, insects, and mammals that feed on fruit and leaves of different tree species and by the predators that then feed upon these primary consumers. This contrasts with aquatic systems where algae that are the dominant plant species, in general, these are more homogeneously distributed and do not generate the distinct patterns in the distribution of vegetation that characterize forests and savannas. Although vertical and horizontal stratification in the abundance of different algae do create subtle groupings in the web that only emerges when the full details of the feeding relationships are delineated (Hutchinson, 1961; D’Alelio et al., 2016). Nevertheless, algal-plankton driven, aquatic food webs will tend to be relatively spatially homogenous and generate an inverse biomass pyramid: most of the rapidly generated primary productivity in algal communities is consumed and turned into relatively short-lived fish and invertebrate species at higher trophic levels. This may make them intrinsically more unstable than spatially complex terrestrial systems where most of the biomass is in the long-lived plants at the base of the food web (Allesina et al., 2015).
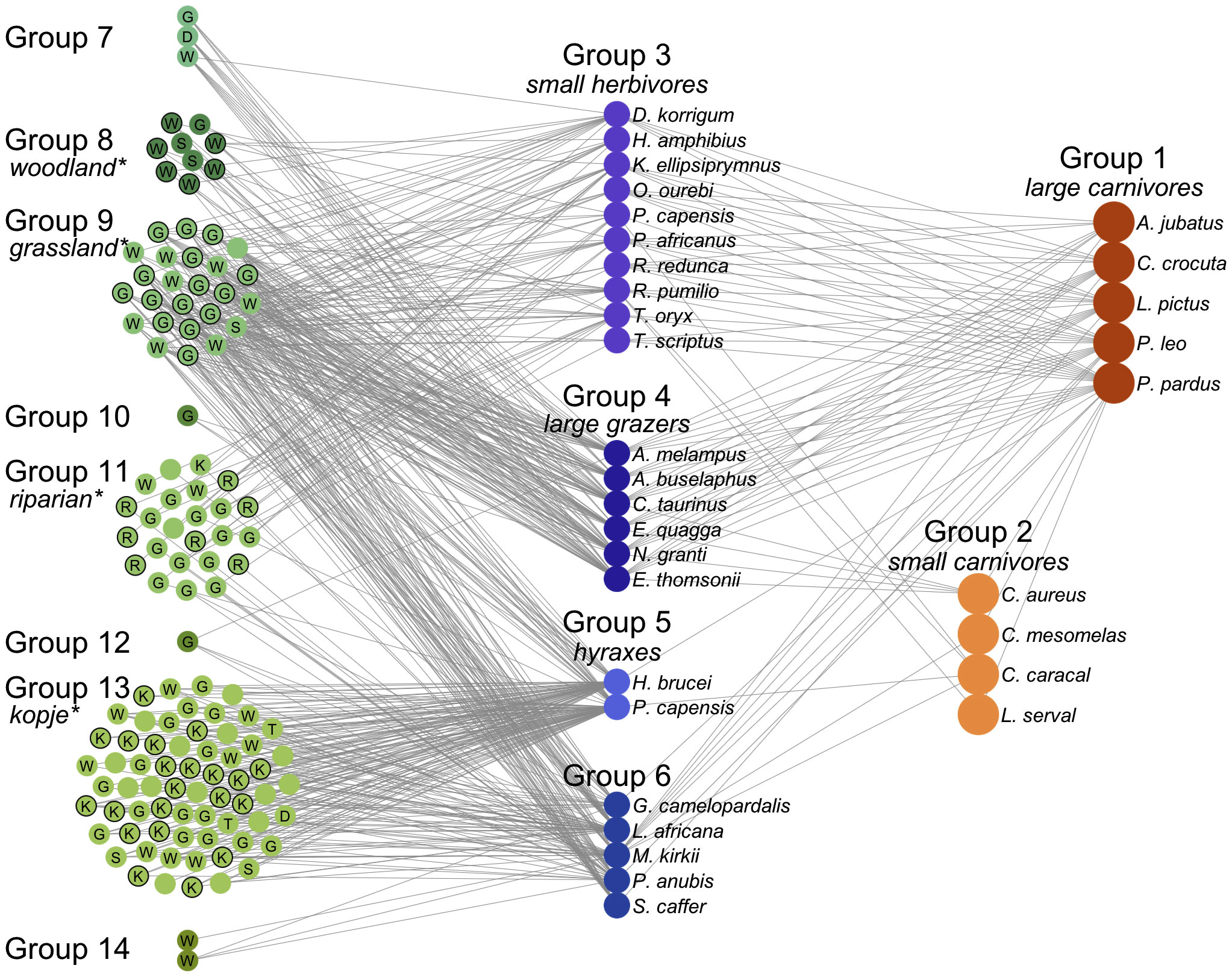
Figure 2 The Serengeti food web. The network is shown organized and colored by group according to the consensus partition method and arranged by trophic level from left (plants) to right (carnivores). Plants are identified by the first letter of identified habitat type, if available: (G)rassland, (W)oodland, (R)iparian, (K)opje, (S)hrubland, (T)hicket, and (D)isturbed. Plant groups are labeled by significantly overrepresented habitat types, and species assigned to the overrepresented type are labeled with black borders. An interactive version of this figure is available at http://edbaskerville.com/research/serengeti-food-web/. (Baskerville et al., 2011).
An overenthusiastic emphasis on algae as ‘canonical plants’ is not restricted to studies of food webs, in a survey and meta-analysis of experimental consumer and fertilization manipulation experiments researchers found that only 7% and 14% of the studies were of terrestrial systems (Hillebrand et al., 2007; Gruner et al., 2008). The international NutNet system has begun to address this balance, a review of the first decade of data from grassland communities at the core of NutNet (Borer et al., 2017) suggests that the stability of these grassland webs reflects a balance between competition among plants for nutrients and light, that is constantly redressed by the grazing of herbivores. Artificial fertilization or reduction in herbivores always leads to reductions in plant diversity due to intensification of competition between plants, disconcertingly echoing the mass uncontrolled experiments that humans are undertaking in many natural ecosystems. If we are ever going to use food-web models to understand these trophic-cascade effects, we will need to develop models that realistically capture the dynamics of the plant community.
The last ten years have seen considerable advances in our understanding of the forces that structure theoretical food webs; the advances developed by Allesina and colleagues almost match the increases of understanding gained in the previous four decades and take us a long way towards resolving the initial ‘complexity versus stability’ conundrum first described by May (see Box 1). The ‘devious strategies which make for stability in enduring natural systems’ that allow coexistence have now been considerably untangled. A major next step will be to develop new hybrid methods that combine insights from plant community ecology into food web theory. This will then provide a new framework for examining the structure of the temperate woodlands, tropical forests and savannas that contain most of the terrestrial biodiversity on the planet.
Box 1. field guide to random matrix food web models.
The original ‘random-graph’ food web models developed from “spin-glass theory” by Gardner and Ashby (1970) were refined by Robert May (1973) to produce a result that has had ecologists scratching their heads for forty years. The result showed that if a food web was characterized by a matrix of random positive and negative interactions between species (of magnitude 0<->1) then stability would always decrease with increasing species diversity. So how could complex ecological communities persist? May’s result showed that stability requires all the eigenvalues of the community interaction matrix to be negative, this requires , where s is number of species, c is connectance, or number of links in the web and i is average interaction strength. May suggested that ‘modularity’, organizing species into sub-webs of species that interacted more with each other than with other species might help enhance stability, although stability would still always decline with increased species diversity.
Allesina et al. (2008) helped formalize this conjecture when they showed that that subdividing webs into groups of highly interactive species could enhance stability. They then made a major breakthrough (Allesina and Pascual, 2009) when they realized May’s original formulation allocated each interaction strength a random number between -1 and 1. This meant that 1/4 of web interactions would be mutualisms (+/+), 1/4 would be competition (-/-) and half would be consumer-resource or predator-prey (+/-) relationships. This makes it very hard to have negative eigenvalues as the product of all the negative interactions will be balanced by the product of all the positive interactions (remembering -/- is always positive)!. They showed that if competitive and mutualistic interactions are more accurately characterized by the consumer-resource relationships that underlie them then it was much more likely that the community matrix would have negative eigenvalues and the community would be stable. Allesina and Tang (2012) extended this result to show that stability was possible with much larger community matrices when the vast majority of links were consumer resource (+/-) links, although May’s central conjecture still held, stability will still decline with increased species diversity, but the intercept of the relationship will be much higher. Some of these results were partly discovered by a paper written in direct response to May’s (1973) paper by (Roberts, 1974). He suggested that if the subset of random webs with feasible properties are considered (where feasible implies interaction strengths that permit all species to persist), then webs with more species are more stable. However, Roberts set up his arrays in a way that generates very strong within species regulation, which would inherently increase the stability of the web as more species are added.
Allesina and Levine (2011) then expanded the work to examine interactions between species coexisting on the same trophic level, essentially plant communities. This work showed that framing this debate within the traditional and algebraically tractable framework of interactions between two species is misleading, particularly when the traditional framework is expanded to include multiple species all of which are assumed to have similar demographic properties and interact by competing for the gaps that appear when an individual of one species dies and is replaced by any other species (“neutral” models that make a large bow to Peter Grubb’s “regeneration niche” Grubb, 1977). They showed that if species have higher-order interactions with each other such that A outcompetes B, but the presence of C allows B to outcompete A, then it is possible for many species to coexist on the same trophic level in ways that produce patterns that are closer to those observed empirically than occur in “neutral’ communities.
Grilli et al. (2017), expanded this framework to consider indirect interactions in whole food webs; this work showed that indirect interactions can considerably enhance the stability of multi trophic webs. Several ecologists have long argued for the importance of indirect interactions and this work confirms these interactions are important for web stability (Gibbs et al., 2022).
Allesina et al. (2015) also developed elegant methods for adding structure to webs that built upon Williams and Martinez ‘niche model’ which assumes that species can be arranged by body size such that larger species tend to feed on species within a range of smaller body sizes. This means that empirical webs tend to be ‘interval’ and can be arranged as sequence of species along an axis where species to the right can only feed on species to the left (an idea originally suggested by Joel Cohen in 1978). Webs of this type are more similar to empirical webs than ‘random graph’ webs which are not interval. This work further suggested that incorporating modularity considerably reduces the importance of interaction strength in determining stability. Modularity is the presence of substructure within the web such that species tend to form groups that interact much more frequently with each other than with other species. This again significantly increased the stability of the model webs. However, results from these analyses suggest modularity is only important under restrictive conditions and observed patterns of nestedness may simply be an epiphenomenon of intervality and is more likely to observed when food webs are assembled using binary (presence-absence) diet data, than when using quantitative diets (Staniczenko et al., 2013).
A key result to emerge from recent work on food webs described in Box 1 emphasizes the powerful stabilizing influence of indirect interactions between species that are mediated by a third or fourth species (Allesina et al., 2015; Grilli et al., 2017; Mayfield and Stouffer, 2017). Such interactions are central to the recruitment and survival of many tropical and temperate plant species; particularly the presence of fungal pathogens and mutualists. Ecologists have increasingly realized that Janzen-Connell effects that minimize recruitment of seedlings in sites adjacent to their parent tree are driven by fungal pathogens (Augspurger, 1983; Gilbert and Hubbell, 1996; Packer and Clay, 2000; Bagchi et al., 2010; Mangan et al., 2010). This in turn ensures that competition for light and soil resources between plants in tropical forests is mediated at the recruitment stage by indirect inter-specific interactions largely driven by fungal pathogens. The impact of fungal, nematode, insect, and bacterial pathogens that attack seedlings and older plants is considerably modified and ameliorated by the presence of a diversity of symbiotic fungi that organize themselves into a “symbiotic immune system” that helps protect older plants against further attacks (Jones and Dang, 2006; Lo Presti et al., 2015). The key thing about these fungal associations from a food-web perspective is that they generate a diversity of indirect effects that modulate more direct impacts on the plant’s fitness. They also create blocks of interactions that are specific to each tree species, and often to individual trees, thus creating patchy block motifs within the overall matrix of food web interactions (Grilli et al., 2016). These effects, and the modular compartmentalized structure they promote, could have a stabilizing influence on the overall structure of the web, a result that would not have been apparent using the earlier models for food web structure. Fungal interactions are considerably less diverse in marine and freshwater systems, perhaps because algae produce classes of toxins that are particularly detrimental to fungi and bacteria (Kini et al., 2020).
The community of host-specific fungal pathogens and insects combines with those generated by less specific seed-dispersers and pollinators to create considerable modularity the overall matrix of interactions between species in the food web for the ecosystem. Each modular group reflects the large number of interactions that occur between fungi, bacteria, nematodes and insects that are specialists on each plant species, or perhaps even each individual trees. In contrast, the overall matrix of species interactions will be shadowed by the more diffuse connections of generalist pollinators, seed-dispersers and herbivores species that utilize multiple species of trees. Theoretical studies show that the heterogeneities generated by specialists are likely to be more stabilizing than when generalists dominate these components of food web structure (Grilli et al., 2016). Similar patterns occur in the communities of parasites and pathogens that feed in and upon all the species that feed in the herbivorous and carnivorous trophic levels of the web (Lafferty et al., 2008).
Seasonal fluctuations and microbiomes
Deciduous forests are characterized by a huge annual fluctuation in basal resources when the leaves and fruit produced in the spring and consumed throughout the summer; concomitantly their leaves convert atmospheric CO2 into carbohydrates and add new structure to the canopy and roots as well as nutrients for consumers. Recent studies illustrate that in many temperate, tropical and boreal forests, a high proportion of carbon uptake is stored in fungi associated with the roots systems (Hawkins et al., 2023). In the autumn, leaf fall moves a huge volume of nutrients back to the ground and into the soil, sequentially (but asymmetrically) connecting the canopy food webs with those of the understory and the soil. These nutrients are consumed by a large community of fungi, worms and bacteria, many of which have symbiotic relationships with the roots of the parent tree (Cornwell et al., 2009; Hardoim et al., 2015). Other fungi are less altruistic and drive the ‘Janzen-Connell’ effect described above that provide an important driver of tropical forest diversity by preventing offspring of the parent tree establishing in their near vicinity (Bagchi et al., 2010; Mangan et al., 2010). These mechanisms most likely underlie the strong conspecific negative density-dependence, recently quantified in the 50 hectare forest plot on Barro Colorado Island in Panama (Kalyuzhny et al., 2023).
Similar plant-microbial couplings also operate in savannas and grassland (Petermann et al., 2008): the rainy season converts an almost barren and inedible landscape into high quality pasture that can feed a huge abundance of herbivores. In the Serengeti, when the rains end, and the long dry season begins, larger herbivores such as wildebeest and zebra migrate away from nutrient rich soils where they’ve fed during lactation. Their dung, urine, and the death of young calves and adults slowly disperse these nutrients to the soil in nutrient poor regions of the ecosystem. Recent studies of the Serengeti food web illustrate how the long-lived perennial grasses create an annual pulse of nutrients that drives the fast dynamics of the wildebeest and their predators, while the woodlands and the kopjes (rocky outcrops or inselbergs) support sub-communities of less abundant vertebrates that operate on a much slower timescale (Dobson, 2009). We are only just beginning to glimpse how the above ground heterogeneity created by different plant communities is matched by the mycorrhizal and invertebrate communities feeding on the roots beneath the soil surface (Wardle et al., 2004; Crowther et al., 2013). We suspect that it is similar, but soil organisms and other microbes are all too rarely considered in savanna food web studies.
Our hopes that food web studies will help us understand how ecosystems will respond to climate change and other anthropogenic insults founder on the paucity of food web-studies that include trees and their mycorrhizal associations. Acid rain is devastating for fungi, so many temperate plant communities have only been observed in the absence of the mycorrhizae they co-evolved with; similarly fires destroy mycorrhizae. If you visit Yellowstone National Park there are still bare areas of ground with essentially no tree recruitment since the fires of 1988 (Turner et al., 1997; Turner et al., 2003). These are areas where the hottest fires destroyed the mycorrhizal community and pine seedlings are denied the symbiotic mechanism that allows them access to vital soil nutrients (Franke, 2000). The really bad news here is that the last two years have seen extensive fires across the boreal forests and tundra of northern Canada; this has destroyed huge areas of habitat, if the mycorrhizae are lost, these areas may take even longer to recover.
Fire is an important component of many savanna and forest ecosystems; plants are the species that supply fuel for fires and fires return nutrients to the soil; but few food-web models consider fire as a component of the web (although see (Bowman et al., 2016). Some authors have suggested fire appears as a super-herbivore that profoundly effects the dynamics of tree-grass interaction in savannas (McNaughton, 1985; Bond and van Wilgren, 1996; Bond and Keeley, 2005). We tend to see it as more closely resembling an “uber-virus”; it has essentially zero mass and requires a threshold level of host abundance to establish. Like true viruses, the impact of fire on ecosystem structure can be subtle and profound (Holdo et al., 2009; Staver et al., 2011; Beale et al., 2018), to date no food-web models include fire in any form, yet it is essential to the structure and diversity of vegetation in savannas, fynbos, and drier forests (Bond and van Wilgren, 1996; Higgins et al., 2000). Our major concern here is that when fire removes trees and burns at sufficient heat to destroy the soil mycorrhizal community, then the most important stabilizing component of the food web may have been lost and may take the time of many generations of shorter-lived, tree-dependent taxa to recover.
Reproduction and the other webs
While mycorrhizal associations are the most well-known examples of plant-microbe interactions, there are a suite of additional ways in which plants are foundational to microbial ecosystems. Among these, floral nectaries play host to suites of microbial organisms that disperse and establish through flower-visiting animal vectors (Herrera et al., 2009; Belisle et al., 2012). Similarly, carnivorous pitcher plants support a diverse microbial ecosystem within the aqueous solution that decomposes their prey (Baiser et al., 2012). Among plants that do not ‘eat’ animals, diverse microbial communities are found on the surface (Vorholt, 2012) and inside of their leaves (Arnold, 2007). What is becoming clear is that the trophic relationships within the microbial communities of many individual plants may be as complex as even the most diverse of currently resolved macroscopic food webs!
Integrating higher plants into food web theory requires a shift from these species being the basal fodder of grazers and browsers to the central assemblage in terrestrial ecosystems upon which the vast majority of animal and microbial communities and their direct, indirect, and higher-order interactions are assembled (Figure 3). The historical dominance of aquatic ecosystems in food-web ecology has meant that the non-folivorous webs that higher plants host are often not explicitly accounted for in food-web theory. An increasingly large literature on the associations between plants and their pollinators and seed-dispersers, who are both mutualists and consumers of higher plants, coupled with their importance for plant reproduction primes these interactions for inclusion in broader food-web models. In short, to adequately incorporate higher plants into food-web theory we must not forget the birds and the bees!
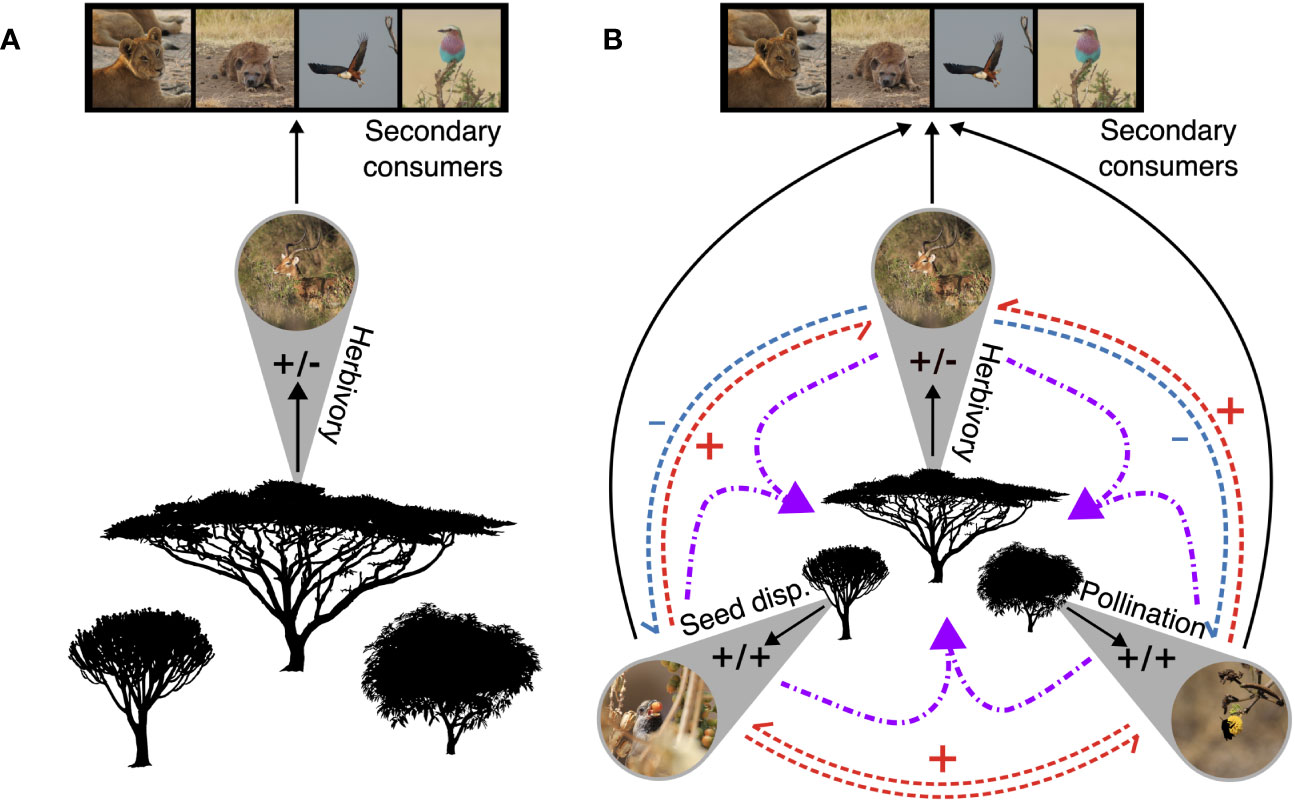
Figure 3 A comparison of two food webs. The first, (A), is a three-trophic-level food web of East African primary producers and consumers. Herbivory, as represented by the browsing impala, is the only form of primary consumption. This linear food web represents the classic mental model of feeding relationships and energetic pathways in an ecosystem. (B) Increasing the resolution of primary consumer guilds in the this food-web changes its complexity, the potential for indirect (blue and red) and higher-order (purple) interactions among primary consumer guilds, and illustrates how including a wider range of interactions and taxa into food webs can lead to a new mental model of feeding relationships, where plant population and community dynamics are central, primary consumer guilds are arrayed around them, and secondary consumers attach at the outer edges. All photos courtesy of MCH.
Animal pollinators facilitate reproduction for around 88% of all flowering plants (Ollerton et al., 2011), a proportion that can reach 98-99% in tropical forests (Bawa, 1990). Similarly, up to 90% of woody plants rely on vertebrates for seed-dispersal (Jordano, 2000), while a substantial proportion of herbaceous seeds are dispersed by ants (Howe and Smallwood, 1982). More specifically, endozoochorous seed-dispersal (i.e., where the disperser receives a meal and the association is often mutually beneficial) ranges from 30–40% of woody species in temperate forests, to 70–94% in neotropical rainforests (Jordano, 2000). Given the nature and ubiquity of these associations, this means that many of the primary consumers in any real food web rely on something other than a plant’s leaves (Figure 4).
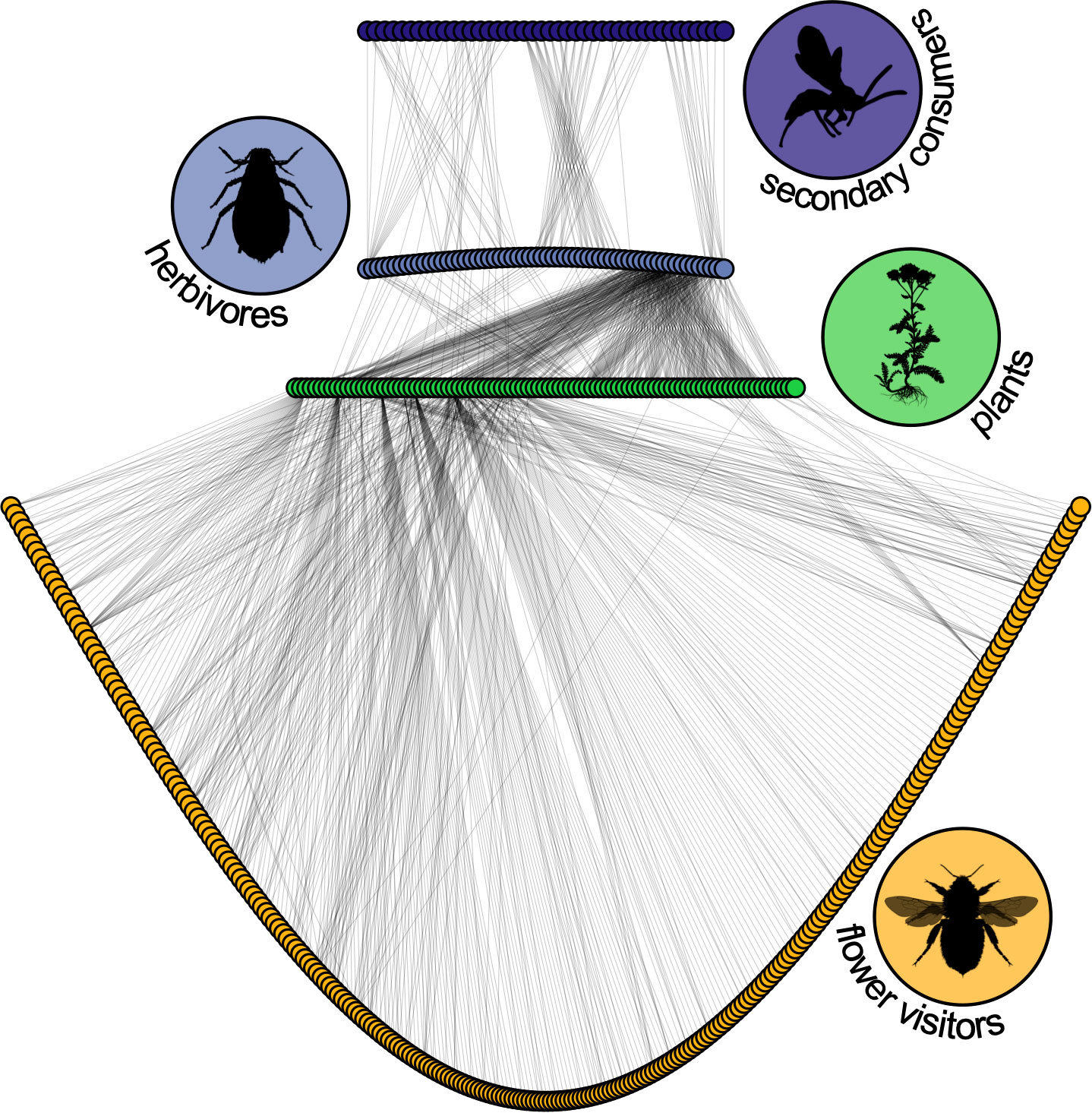
Figure 4 The food-web of Norwood Farm (Somerset, UK) collected by and recreated from the data of Pocock, Evans and Memmott (Pocock et al., 2012). Green circles represent plant species, gray-blue circles are antagonistic primary consumers (aphids and seed-feeders), dark purple circles are parasites and parasitoids, and yellow circles potential pollinators (flower visitors and butterflies). Out of the more than 1200 interactions, 45% are between plants and flower visitors. Likewise, 56% of taxa (252/451) are flower visitors. Their inclusion dramatically alters this food-web. Plant interactions with leaf-miner parasitoids were excluded from the original dataset. All silhouettes are from phylopic.org and the bee image was contributed by Melissa Broussard.
While ‘consumers of fruits and nectar’ are occasionally included in empirical food webs (Polis, 1991; de Visser et al., 2011), pollinators and seed-dispersers are generally beneficial to plants, in contrast to folivores that suppress plants (Pringle et al., 2023). When pollinators and seed-dispersers are lost, plant recruitment can be significantly reduced (Robertson et al., 1999; Cordeiro and Howe, 2001; Clark et al., 2007). Similarly, the importance of these species is highlighted by the fact that their loss can drive rapid directional evolutionary change in plant reproductive morphology (Galetti et al., 2013; Gervasi and Schiestl, 2017), and the long-term dynamics of plant communities are intimately bound to these mutualistic primary consumers (Jordano, 2000). The reproduction of higher plants also represents an under-appreciated avenue through which plants drive food web dynamics. While the annual loss and growth of foliage by deciduous trees is a temporally consistent resource pulse in food webs, the phenology of plant reproduction can be more variable and suggests how animal populations may be stabilized by a trickle of resources as opposed to a pulse. For example, the reproduction of Ficus species in the tropics is often aseasonal both at the scale of individual trees (Bronstein and Patel, 1992) and among species (Lambert and Marshall, 1991). The result of this asynchrony is a steady supply of fruit and floral resources for mutualistic primary consumers. Crucially, figs are the major source of calcium for many frugivorous birds and mammals (O'Brien et al., 1998). Even in climatically harsh boreal and tundra biomes, some plants retain fruits into the autumn and winter (Mulder et al., 2021), representing a crucial resource of frugivores outside of the growing season. Shifting availability of floral and fruit resources can also have strong effects on the spatial and temporal distribution of pollinators and frugivorous seed-disperser populations (Levey, 1988a; Levey, 1988b; Kinnaird et al., 1996; Olesen et al., 2011). Over the past 30 years, a rapidly growing literature on the structure and function of bipartite plant-mutualist networks has developed (Jordano, 1987; Bascompte and Jordano, 2013; Valdovinos, 2019; Valdovinos and III, 2021), yet it has remained largely distinct from classic food web studies. The incorporation of reproductive mutualisms into food web theory would represent the conceptual coupling of two dominant fields in community ecology. Food-web models that consider higher-plant population dynamics and the mutualists that drive those dynamics will also benefit from the conceptual advances in mutualistic-network ecology on the phenology of species interactions (CaraDonna et al., 2014; Ponisio et al., 2017), species-species interactions that do not occur (Olesen et al., 2011), and structure-stability relationships in different ecological networks (Thébault and Fontaine, 2010; Sauve et al., 2014).
The integration of pollination and seed-dispersal associations into food web theory has the potential to alter the image of complexity and stability of currently resolved food webs while also improving the biological realism of plant-population models (Figure 4). May’s stability criterion (Box 1) relies on species richness, interaction strength, and connectance (i.e. the proportion of trophic links observed out of the total possible links) (May, 1973; van Altena et al., 2016). Pollinators are a mega-diverse guild; the addition of these species to food webs will increase species richness and almost certainly alter connectance and network macrostructures (Figure 4). Furthermore, the links between pollinators, seed-dispersers, and their respective predators may produce more accurate food web representations by filling in some of the ‘missing links’ (Dobson et al., 2008; Lafferty et al., 2008). For precedence, the incorporation of parasites into food webs this has had startling implications for studies of food web complexity (Lafferty et al., 2006). Incorporating the population and community dynamics of higher plants to food web theory will not only improve the resolution of the basal resource level but also necessitate the inclusion of entire guilds of animal species not typically represented in classic predator-prey food webs, such as pollinators and frugivorous seed-dispersers.
Incorporating reproductive mutualisms into food-web theory involves more than tacking on a plant-pollinator or plant-frugivore module to existing food-webs and assuming that all interactions in these mutualistic modules are always mutually beneficial. Mutualisms are prone to cheating behaviors, which can shift these interactions, at least temporarily, towards parasitism (Jones et al., 2015). For example, endozoochorus dispersal—by virtue of fruits and seeds being ingested—tends to involve some kind of nutritional reward to the consumer, but the effect on the plant can range from seeds requiring gut passage for germination to seed predation. Epizoochorus dispersal, where seeds are dispersed without ingestion, such as by hooking onto fur, offer no cost to the disperser, but also no benefit. Furthermore, obligate frugivores are few and far between (Jordano, 2000), seed-predators, such as ants and small mammals, can still be important seed dispersers)(Janzen, 1971), and seed-dispersal by large folivores may be negated by their leaf and stem consumption. Browsing ungulates can be important seed-dispersers (Rodríguez-Pérez et al., 2011; Pringle et al., 2014) despite their largely folivorous diets and negative effect on plants (Pringle et al., 2023). Therefore, the devil of integrating plant reproduction into food webs almost certainly lies in the details of how reproductive mutualisms function and are quantified.
Seeing the web with the trees
In conclusion, we argue that a deeper consideration of the role of higher plants in food webs is needed to create the “next generation” of food web models. The simplest way to do this would be to include trees and plants as basal species in food webs where spatial competition for light, water, and nutrients creates a community of species that live for a very long time and thus generate significant underlying stability that trickles up to the species that feed in and upon them. These primary consumers can be divided into two broad classes of species: (1) those that feed on the regular production of fruits, leaves, sap and bark of the plant; and (2) the large community of fungi, bacteria and parasitic plants that drive interactions with the plant and its surrounding environment and through time modify the structure of the individual tree, in ways that usually enhance the persistence of both the tree and its ‘symbiotic’ community. The inclusion of this huge diversity of parasitic and mutualistic species echoes recent pleas to include parasites and pathogens into models for food webs (Lafferty et al., 2006; Dobson et al., 2008; Lafferty et al., 2008). Each of these groups of species generate fuzzy but modular blocks within the matrix of interactions between species that form the overall structure of any food web matrix.
The theoretical and technical advances required to bring higher plants into food web studies is beginning to emerge. Multilayer and multiplex ecological networks are an exciting development that allow several webs (be they the webs associated to individual trees in a forest or webs describing different types of ecological interactions) to be described as single mathematical object (Pilosof et al., 2015; García-Callejas et al., 2018; Guimaraes, 2020). An important recent study has plotted a potential course for the integration of niche theory with food web theory (Godoy et al., 2018). Yet others have described how bioenergetic food-web models may be extended to include terrestrial plant-herbivore interactions. (Valdovinos et al., 2022). These approaches will generate the food-web descriptions that will be used to explore the organization of terrestrial species interactions at the broadest community level and can quickly become the grist for the next generation of food-web models that needed to help understand the consequences resource exploitation and deforestation in all the world’s forests. Hopefully, they can also provide important guides for pathways to forest restoration.
We conclude on a final note of urgency. We are losing trees, forest and grasslands at a rapid rate. If the stability and persistence of terrestrial ecosystems is dependent upon long-lived plant species we need to considerably upgrade attempts to protect them. There are roughly 400 trees for every human on the planet, this number is decreasing for two reasons: increasing human population and the loss of 15 billion trees each year (Crowther et al., 2015); land-use conversion since the dawn of agriculture has led to the loss of around 46% of global tree abundance. Slowing this loss of trees, forests, and perennial grasslands is arguably the most efficient way to reduce the net rate of species extinction (Schleuning et al., 2016). Restoring degraded forests, savannas, and grasslands is arguably the most cost-effective and efficient way to reverse global climate heating. As most of the freshwater used by humans is supplied by rivers and streams that have their origins in forests and water sheds, then human dependence on freshwater should form the basis of any cogent argument about the importance of protecting forests (Garrick et al., 2017). This should protect both the forests and the trees that are arguably the key components of most terrestrial food webs.
Data availability statement
The original contributions presented in the study are included in the article/supplementary material. Further inquiries can be directed to the corresponding authors.
Author contributions
All authors listed have made a substantial, direct, and intellectual contribution to the work and approved it for publication.
Acknowledgments
The original genesis for this article came from a day wandering around Windsor Great Park with Ted Greene and Mick Crawley, we thank them for many inspiring conversations about plants. This paper is dedicated to them as lifetime sources of inspiration. Many thanks to Jen Guyton for the photograph of Welwitschia in Figure 1. We have also benefited hugely from conversations with Bob May, Mercedes Pascual, Jennifer Dunne, Jonathan Levine and Stefano Allesina about food webs and annual conversations at STRI in Panama with Allan Herre, Stefan Schnitzer, Scott Mangon, John Schroeder, Dan Petticord, and Luis Mejía about tropical plants and their pathogens.
Conflict of interest
The authors declare that the research was conducted in the absence of any commercial or financial relationships that could be construed as a potential conflict of interest.
Publisher’s note
All claims expressed in this article are solely those of the authors and do not necessarily represent those of their affiliated organizations, or those of the publisher, the editors and the reviewers. Any product that may be evaluated in this article, or claim that may be made by its manufacturer, is not guaranteed or endorsed by the publisher.
References
Allesina S., Grilli J., Barabás G., Tang S., Aljadeff J., Maritan A. (2015). Predicting the stability of large structured food webs. Nat. Commun. 6, 7842. doi: 10.1038/ncomms8842
Allesina S., Pascual M. (2009). Food web models: a plea for groups. Ecology letters 12 (7), 652–662.
Allesina S., Levine J. M. (2011). A competitive network theory of species diversity. Proc. Natl. Acad. Sci. 108 (14), 5638–5642.
Allesina S., Tang S. (2012). Stability criteria for complex ecosystems. Nature 483, 205–208. doi: 10.1038/nature10832
Allesina S., Tang S. (2015). The stability–complexity relationship at age 40: a random matrix perspective. Population Ecol. 57, 63–75. doi: 10.1007/s10144-014-0471-0
Allesina S., Alonso D., Pascual M. (2008). A general model for food web structure. Science 320 (5876), 658–661.
Arnold A. E. (2007). Understanding the diversity of foliar endophytic fungi: progress, challenges, and frontiers. Fungal biology reviews 21 (2-3), 51–66.
Augspurger C. K. (1983). Seed dispersal by the tropical tree, Platypodium elegans, and the escape of its seedlings from fungal pathogens. J. Of Ecol. 71, 759–771. doi: 10.2307/2259591
Bagchi R., Swinfield T., Gallery R. E., Lewis O. T., Gripenberg S., Narayan L., et al. (2010). Testing the Janzen-Connell mechanism: pathogens cause overcompensating density dependence in a tropical tree. Ecol. Lett. 13, 1262–1269. doi: 10.1111/j.1461-0248.2010.01520.x
Baiser B., Gotelli N. J., Buckley H. L., Miller T. E., Ellison A. M. (2012). Geographic variation in network structure of a nearctic aquatic food web. Global Ecology and Biogeography 21 (5), 579–591.
Baskerville E. B., Dobson A. P., Bedford T., Allesina S., Anderson T. M., Pascual M. (2011). Spatial guilds in the serengeti food web revealed by a Bayesian group model. PLoS Comput. Biol. 7, e1002321. doi: 10.1371/journal.pcbi.1002321
Bawa K. (1990). Plant-pollinator interactions in tropical rain forests. Annu. Rev. Of Ecol. And Systematics 21, 399–422. doi: 10.1146/annurev.es.21.110190.002151
Beale C. M., Courtney Mustaphi C. J., Morrison T. A., Archibald S., Anderson T. M., Dobson A. P., et al. (2018). Pyrodiversity interacts with rainfall to increase bird and mammal richness in African savannas. Ecol. Lett. 21, 557–567. doi: 10.1111/ele.12921
Belisle M., Peay K. G., Fukami T. (2012). Flowers as islands: spatial distribution of nectar-inhabiting microfungi among plants of Mimulus aurantiacus, a hummingbird-pollinated shrub. Microbial ecology 63 (7), 711–718.
Bond W. J., Keeley J. E. (2005). Fire as a global “herbivore”: the ecology and evolution of flammable ecosystems. Trends In Ecol. Evol. 20, 387–394. doi: 10.1016/j.tree.2005.04.025
Borer E. T., Grace J. B., Harpole W. S., MacDougall A. S., Seabloom E. W. (2017). A decade of insights into grassland ecosystem responses to global environmental change. Nat. Ecol. Evol. 1, 0118. doi: 10.1038/s41559-017-0118
Bowman D. M., Perry G. L., Higgins S. I., Johnson C. N., Fuhlendorf S. D., Murphy B. P. (2016). Pyrodiversity is the coupling of biodiversity and fire regimes in food webs. Philos. Trans. R. Soc. B: Biol. Sci. 371, 20150169.
Bronstein J. L., Patel A. (1992). Causes and consequences of within-tree phenological patterns in the Florida strangling fig, Ficus aurea (Moraceae). Am. J. Of Bot. 79, 41–48. doi: 10.1002/j.1537-2197.1992.tb12621.x
Brown P. M. (1996). “OldList: A Database of Maximum Tree Ages” Radiocarbon. In “Tree Rings, Environment and Humanity” eds. J. S. Dean, D. M. Meko, T. W. Swetnam. pp. 727–731.
CaraDonna P. J., Iler A. M., Inouye D. W. (2014). Shifts in flowering phenology reshape a subalpine plant community. Proc. Natl. Acad. Sci. 111, 4916–4921. doi: 10.1073/pnas.1323073111
Chisholm R. A., Condit R., Rahman K. A., Baker P. J., Bunyavejchewin S., Chen Y. Y., et al. (2014). Temporal variability of forest communities: empirical estimates of population change in 4000 tree species. Ecol. Lett. 17, 855–865. doi: 10.1111/ele.12296
Clark C., Poulsen J., Levey D., Osenberg C. (2007). Are plant populations seed limited? A critique and meta-analysis of seed addition experiments. Am. Nat. 170, 128–142. doi: 10.1086/518565
Cordeiro N. J., Howe H. F. (2001). Low recruitment of trees dispersed by animals in African forest fragments. Conserv. Biol. 15, 1733–1741. doi: 10.1046/j.1523-1739.2001.99579.x
Cornwell W. K., Cornelissen J. H., Allison S. D., Bauhus J., Eggleton P., Preston C. M., et al. (2009). Plant traits and wood fates across the globe: rotted, burned, or consumed? Global Change Biol. 15, 2431–2449.
Crowther T. W., Glick H. B., Covey K. R., Bettigole C., Maynard D. S., Thomas S. M., et al. (2015). Mapping tree density at a global scale. Nature 525, 201–205. doi: 10.1038/nature14967
Crowther T. W., Stanton D. W., Thomas S. M., A'Bear A. D., Hiscox J., Jones T. H., et al. (2013). Top-down control of soil fungal community composition by a globally distributed keystone consumer. Ecology 94, 2518–2528. doi: 10.1890/13-0197.1
D’Alelio D., Libralato S., Wyatt T., d’Alcalà M. R. (2016). Ecological-network models link diversity, structure and function in the plankton food-web. Sci. Rep. 6, 21806. doi: 10.1038/srep21806
de Visser S. N., Freymann B. P., Olff H. (2011). The Serengeti food web: empirical quantification and analysis of topological changes under increasing human impact. J. Of Anim. Ecol. 80, 484–494. doi: 10.1111/j.1365-2656.2010.01787.x
Dobson A. P. (2009). Food-web structure and ecosystem services: insights from the Serengeti. Philos. Trans. R. Soc. London Ser. B 364, 1665–1682. doi: 10.1098/rstb.2008.0287
Dobson A. P., Lafferty K. D., Kuris A. M., Hechinger R. F., Jetz W. (2008). Homage to Linnaeus: How many parasites? How many hosts? PNAS 105, 11482–11489. doi: 10.1073/pnas.0803232105
Fine P. V., Mesones I., Coley P. D. (2004). Herbivores promote habitat specialization by trees in Amazonian forests. Science 305, 663–665. doi: 10.1126/science.1098982
Franke M. A. (2000). Yellowstone in the Afterglow (Mammoth Hot Springs, Wyoming: Yellowstone National Park).
Galetti M., Guevara R., Côrtes M. C., Fadini R., Von Matter S., Leite A. B., et al. (2013). Functional extinction of birds drives rapid evolutionary changes in seed size. Science 340, 1086–1090. doi: 10.1126/science.1233774
García-Callejas D., Molowny-Horas R., Araújo M. B. (2018). Multiple interactions networks: towards more realistic descriptions of the web of life. OIKOS 127, 5–22. doi: 10.1111/oik.04428
Gardner M. R., Ashby W. R. (1970). Connectance of large dynamic (cybernetic) systems: critical values for stability. Nature 228 (5273), 784.
Garrick D. E., Hall J. W., Dobson A., Damania R., Grafton R. Q., Hope R., et al. (2017). Valuing water for sustainable development. Science 358, 1003–1005. doi: 10.1126/science.aao4942
Gervasi D. D., Schiestl F. P. (2017). Real-time divergent evolution in plants driven by pollinators. Nat. Commun. 8, 14691. doi: 10.1038/ncomms14691
Gibbs T., Levin S. A., Levine J. M. (2022). Coexistence in diverse communities with higher-order interactions. Proc. Natl. Acad. Sci. 119, e2205063119. doi: 10.1073/pnas.2205063119
Gilbert G. S., Hubbell S. P. (1996). Plant diseases and the conservation of tropical forests. BioSCience 46, 98–106. doi: 10.2307/1312812
Godoy O., Bartomeus I., Rohr R. P., Saavedra S. (2018). Towards the integration of niche and network theories. Trends In Ecol. Evol. 33, 287–300. doi: 10.1016/j.tree.2018.01.007
Grilli J., Barabás G., Michalska-Smith M. J., Allesina S. (2017). Higher-order interactions stabilize dynamics in competitive network models. Nature 548, 210–213. doi: 10.1038/nature23273
Grilli J., Rogers T., Allesina S. (2016). Modularity and stability in ecological communitiesNature Communications Vol. 7 (1), 12031.
Grubb P. J. (1977). The maintenance of species-richness in plant communities: the importance of the regeneration niche. Biol. Rev. 52, 107–145. doi: 10.1111/j.1469-185X.1977.tb01347.x
Gruner D. S., Smith J. E., Seabloom E. W., Sandin S. A., Ngai J. T., Hillebrand H., et al. (2008). A cross-system synthesis of consumer and nutrient resource control on producer biomass. Ecol. Lett. 11, 740–755. doi: 10.1111/j.1461-0248.2008.01192.x
Guimaraes J. P.R. (2020). The structure of ecological networks across levels of organization. Annu. Rev. Ecology Evolution Systematics 51, 433–460. doi: 10.1146/annurev-ecolsys-012220-120819
Hardoim P. R., Van Overbeek L. S., Berg G., Pirttilä A. M., Compant S., Campisano A., et al. (2015). The hidden world within plants: ecological and evolutionary considerations for defining functioning of microbial endophytes. Microbiol. Mol. Biol. Rev. 79, 293–320. doi: 10.1128/MMBR.00050-14
Hawkins H.-J., Cargill R. I., Van Nuland M. E., Hagen S. C., Field K. J., Sheldrake M., et al. (2023). Mycorrhizal mycelium as a global carbon pool. Curr. Biol. 33, R560–R573. doi: 10.1016/j.cub.2023.02.027
Herrera C. M., De Vega C., Canto A., Pozo M. I. (2009). Yeasts in floral nectar: a quantitative survey. Annals of botany 103 (9), 1415–1423.
Higgins S., Bond W.-J., Trollope W.-S.-W. (2000). Fire, resprouting and variability: A recipe for grass-tree coexistence in savanna. J. Of Ecol. 88, 213–229. doi: 10.1046/j.1365-2745.2000.00435.x
Hillebrand H., Gruner D. S., Borer E. T., Bracken M. E., Cleland E. E., Elser J. J., et al. (2007). Consumer versus resource control of producer diversity depends on ecosystem type and producer community structure. Proc. Natl. Acad. Sci. 104, 10904–10909. doi: 10.1073/pnas.0701918104
Holdo R. M., Sinclair A. R. E., Dobson A. P., Metzger K. L., Bolker B., Holt R. D. (2009). A disease-mediated trophic cascade in the Serengeti and its implications for ecosystem C. PloS Biol. 7 (9), 1-12. e1000210. doi: 10.1371/journal.pbio.1000210
Howe H. F., Smallwood J. (1982). Ecology of seed dispersal. Annu. Rev. Of Ecol. And Systematics 13, 201–228. doi: 10.1146/annurev.es.13.110182.001221
Hubbell S. (2001). The Unified Theory of Biodiversity and Biogeography (Princeton: Princeton University Press).
Hutchinson M. C., Dobson A. P., Pringle R. M. (2022). Dietary abundance distributions: Dominance and diversity in vertebrate diets. Ecol. Lett. 25, 992–1008. doi: 10.1111/ele.13948
Janzen D. H. (1971). Seed predation by animals. Annu. Rev. Of Ecol. And Systematics 2, 465–492. doi: 10.1146/annurev.es.02.110171.002341
Jones E. I., Afkhami M. E., Akçay E., Bronstein J. L., Bshary R., Frederickson M. E., et al. (2015). Cheaters must prosper: reconciling theoretical and empirical perspectives on cheating in mutualism. Ecology letters 18 (11), 1270–1284.
Jordano P. (1987). Patterns of mutualistic interactions in pollination and seed dispersal: connectance, dependence asymmetries, and coevolution. Am. Nat. 129, 657–677. doi: 10.1086/284665
Jordano P. (2000). “Fruits and frugivory,” in Seeds-the ecology of regeneration of plant communities. Ed. Fenner M. (Wallingford: CABI), 125–166.
Kalyuzhny M., Lake J. K., Wright S. J., Ostling A. M. (2023). Pervasive within-species spatial repulsion among adult tropical trees. Science 381, 563–568. doi: 10.1126/science.adg7021
Kini S., Divyashree M., Mani M. K., Mamatha B. S. (2020). “Algae and cyanobacteria as a source of novel bioactive compounds for biomedical applications,” in Advances in cyanobacterial biology (Academic Press), 173–194.
Kinnaird M. F., O'Brien T. G., Suryadi S. (1996). Population fluctuation in Sulawesi Red-knobbed Hornbills: tracking figs in space and time. Auk 113, 431–440. doi: 10.2307/4088909
Lafferty K. D., Allesina S., Arim M., Briggs C. J., De Leo G., Dobson A. P., et al. (2008). Parasites in food webs: the ultimate missing links. Ecol. Lett. 11, 533–546. doi: 10.1111/j.1461-0248.2008.01174.x
Lafferty K. D., Dobson A. P., Kuris A. M. (2006). Parasites dominate food web links. PNAS 103, 11211–11216. doi: 10.1073/pnas.0604755103
Lambert F. R., Marshall A. G. (1991). Keystone characteristics of bird-dispersed Ficus in a Malaysian lowland rain forest. J. Of Ecol. 79, 793–809. doi: 10.2307/2260668
Levey D. J. (1988a). Spatial and temporal variation in Costa RIcan fruit and fruit-eating bird abundance. Ecol. Monogr. 58, 251–269. doi: 10.2307/1942539
Levey D. J. (1988b). Tropcal wet forest treefall gaps and distribution of understory birds and plants. Ecology 69, 1076–1089. doi: 10.2307/1941263
Lo Presti L., Lanver D., Schweizer G., Tanaka S., Liang L., Tollot M., et al. (2015). Fungal effectors and plant susceptibility. Annu. Rev. Plant Biol. 66, 513–545. doi: 10.1146/annurev-arplant-043014-114623
Loreau M., De Mazancourt C. (2013). Biodiversity and ecosystem stability: a synthesis of underlying mechanisms. Ecol. Lett. 16, 106–115. doi: 10.1111/ele.12073
Mangan S. A., Schnitzer S. A., Herre E. A., Mack K. M., Valencia M. C., Sanchez E. I., et al. (2010). Negative plant-soil feedback predicts tree-species relative abundance in a tropical forest. Nature 466, 752. doi: 10.1038/nature09273
Mayfield M. M., Stouffer D. B. (2017). Higher-order interactions capture unexplained complexity in diverse communities. Nat. Ecol. Evol. 1, 0062. doi: 10.1038/s41559-016-0062
McCann K., Rasmussen J. B., Umbanhowar J. (2005). The dynamics of spatially coupled food webs. Ecol. Lett. 8, 513–523. doi: 10.1111/j.1461-0248.2005.00742.x
McNaughton S. J. (1985). Ecology of a grazing ecosystem: The Serengeti. Ecol. Monogr. 55, 259–294. doi: 10.2307/1942578
Mulder C. P., Spellman K. V., Shaw J. (2021). Berries in winter: A natural history of fruit retention in four species across Alaska. Madrono 68, 487–510. doi: 10.3120/0024-9637-68.4.487
O'Brien T. G., Kinnaird M. F., Dierenfeld E. S., Conklin-Brittain N. L., Silver S. C. (1998). What's so special about figs: A pantropical mineral analysis. Nature 392, 668. doi: 10.1038/33580
Olesen J. M., Bascompte J., Dupont Y. L., Elberling H., Rasmussen C., Jordano P. (2011). Missing and forbidden links in mutualistic networks. Proc. R. Soc. London B: Biol. Sci. 278, 725–732. doi: 10.1098/rspb.2010.1371
Ollerton J., Winfree R., Tarrant S. (2011). How many flowering plants are pollinated by animals? OIKOS 120, 321–326. doi: 10.1111/j.1600-0706.2010.18644.x
Packer A., Clay K. (2000). Soil pathogens and spatial patterns of seedling mortality in a temperate tree. Nature 404, 278–281. doi: 10.1038/35005072
Parihar M., Rakshit A., Meena V. S., Gupta V. K., Rana K., Choudhary M., et al. (2020). The potential of arbuscular mycorrhizal fungi in C cycling: a review. Arch. Microbiol. 202, 1581–1596. doi: 10.1007/s00203-020-01915-x
Petermann J. S., Fergus A. J., Turnbull L. A., Schmid B. (2008). Janzen-Connell effects are widespread and strong enough to maintain diversity in grasslands. Ecology 89, 2399–2406. doi: 10.1890/07-2056.1
Pilosof S., Porter M. A., Pascual M., Kéfi S. (2015). The multilayer nature of ecological networks. arXiv preprint arXiv 1511, 04453.
Pocock M. J., Evans D. M., Memmott J. J. S. (2012). The robustness and restoration of a network of ecological networks. Science 335, 973–977. doi: 10.1126/science.1214915
Polis G. A. (1991). Complex trophic interactions in deserts: an empirical critique of food web theory. Am. Nat. 138, 123–155. doi: 10.1086/285208
Ponisio L. C., Gaiarsa M. P., Kremen C. (2017). Opportunistic attachment assembles plant–pollinator networks. Ecol. Lett. 20, 1261–1272. doi: 10.1111/ele.12821
Price P. W. (2002). Resource-driven terrestrial interaction webs. Ecol. Res. 17, 241–247. doi: 10.1046/j.1440-1703.2002.00483.x
Pringle R. M., Goheen J. R., Palmer T. M., Charles G. K., DeFranco E., Hohbein R., et al. (2014). Low functional redundancy among mammalian browsers in regulating an encroaching shrub (Solanum campylacanthum) in African savannah. Proc. R. Soc. London B: Biol. Sci. 281, 20140390.
Pringle R. M., Abraham J. O., Anderson T. M., Coverdale T. C., Davies A. B., Dutton C. L., et al. (2023). Impacts of large herbivores on terrestrial ecosystems. Fungal biology reviews 21 (11), R584–R610.
Ram H. M. (2016). Iconic flora of heritage significance in India. Indian J. History Sci. 51, 312–342.
Roberts A. (1974). The stability of a feasible random ecosystem. Nature 251, 607–608. doi: 10.1038/251607a0
Robertson A. W., Kelly D., Ladley J. J., Sparrow A. D. (1999). Effects of pollinator loss on endemic New Zealand mistletoes (Loranthaceae). Conserv. Biol. 13, 499–508. doi: 10.1046/j.1523-1739.1999.97471.x
Rodríguez-Pérez J., Wiegand K., Ward D. (2011). Interaction between ungulates and bruchid beetles and its effect on Acacia trees: modeling the costs and benefits of seed dispersal to plant demography. Oecologia 167, 97–105. doi: 10.1007/s00442-011-1964-6
Rooney N., McCann K., Gellner G., Moore J. C. (2006). Structural asymmetry and the stability of diverse food webs. Nature 442, 265–269. doi: 10.1038/nature04887
Sauve A. M., Fontaine C., Thébault E. (2014). Structure–stability relationships in networks combining mutualistic and antagonistic interactions. OIKOS 123, 378–384. doi: 10.1111/j.1600-0706.2013.00743.x
Schleuning M., Fründ J., Schweiger O., Welk E., Albrecht J., Albrecht M., et al. (2016). Ecological networks are more sensitive to plant than to animal extinction under climate change. Nat. Commun. 7, 13965. doi: 10.1038/ncomms13965
Staniczenko P. P., Kopp J. C., Allesina S. (2013). The ghost of nestedness in ecological networks. Nat. Commun. 4, 1391. doi: 10.1038/ncomms2422
Staver A. C., Archibald S., Levin S. (2011). Tree cover in sub-Saharan Africa: rainfall and fire constrain forest and savanna as alternative stable states. Ecology 92, 1063–1072. doi: 10.1890/10-1684.1
Tang S., Pawar S., Allesina S. (2014). Correlation between interaction strengths drives stability in large ecological networks. Ecol. Lett. 17, 1094–1100. doi: 10.1111/ele.12312
Thébault E., Fontaine C. (2010). Stability of ecological communities and the architecture of mutualistic and trophic networks. Science 329, 853–856. doi: 10.1126/science.1188321
Tilman D. (1982). Resource Competition and Community Structure (Monographs in Population Biology – 17) (Princeton University Press).
Tilman D. (1988). Plant Strategies and the Dynamics and Structure of Plant Communities (Monographs in Population Biology-26). (Princeton University Press).
Turner M. G., Romme W. H., Gardner R. H., Hargrove W. W. (1997). Effects of fire size and pattern on early succession in Yellowstone National Park. Ecol. Monogr. 67, 411–433. doi: 10.1890/0012-9615(1997)067[0411:EOFSAP]2.0.CO;2
Turner M. G., Romme W. H., Tinker D. B. (2003). Surprises and lessons from the 1988 Yellowstone fires. Front. In Ecol. And Environ. 1, 351–358. doi: 10.1890/1540-9295(2003)001[0351:SALFTY]2.0.CO;2
Valdovinos F. S. (2019). Mutualistic networks: moving closer to a predictive theory. Ecol. Lett. 22, 1517–1534. doi: 10.1111/ele.13279
Valdovinos F. S., Hale K. R., Dritz S., Glaum P. R., McCann K. S., Simon S. M., et al. (2022). A bioenergetic framework for aboveground terrestrial food webs. Trends In Ecol. Evol.
Valdovinos F. S., III R. M. (2021). Niche theory for mutualism: A graphical approach to plant-pollinator network dynamics. Am. Nat. 197, 393–404. doi: 10.1086/712831
van Altena C., Hemerik L., de Ruiter P. C. (2016). Food web stability and weighted connectance: the complexity-stability debate revisited. Theor. Ecol. 9, 49–58. doi: 10.1007/s12080-015-0291-7
Vorholt J. A. (2012). Microbial life in the phyllosphere. Nature Reviews Microbiology 10 (12), 828–840.
Keywords: food webs, trees, stability, plant communities, pollination, mutualism
Citation: Dobson A, Hutchinson MC and Batterman S (2023) Plant communities and food webs. Front. Ecol. Evol. 11:1253084. doi: 10.3389/fevo.2023.1253084
Received: 04 July 2023; Accepted: 28 September 2023;
Published: 30 October 2023.
Edited by:
Jurek Kolasa, McMaster University, CanadaReviewed by:
Blai Vidiella, Spanish National Research Council (CSIC), SpainCopyright © 2023 Dobson, Hutchinson and Batterman. This is an open-access article distributed under the terms of the Creative Commons Attribution License (CC BY). The use, distribution or reproduction in other forums is permitted, provided the original author(s) and the copyright owner(s) are credited and that the original publication in this journal is cited, in accordance with accepted academic practice. No use, distribution or reproduction is permitted which does not comply with these terms.
*Correspondence: Andy Dobson, ZG9ic29uQHByaW5jZXRvbi5lZHU=; Matthew C. Hutchinson, bWh1dGNoaW5zb242QHVjbWVyY2VkLmVkdQ==; Sarah Batterman, c2FiYXR0ZXJtYW5AZ29vZ2xlbWFpbC5jb20=