- 1Neuroscience and Behavior Program, Vassar College, Poughkeepsie, NY, United States
- 2Departments of Otolaryngology, Biomedical Engineering, and Neuroscience, University of Rochester, Rochester, NY, United States
- 3Biology Department, Vassar College, Poughkeepsie, NY, United States
Anthropogenic noise and its impact on wildlife has recently received considerable attention. Research interest began to increase at the turn of the century and the number of publications investigating the effects of anthropogenic noise has been growing steadily ever since. Songbirds have been a major focus in the study of anthropogenic noise effects, with a significant portion of the literature focusing on the changes in singing behavior in noise. Many of these studies have found increases in the amplitude or frequency of song, or changes in the temporal patterning of song production, putatively due to the masking effects of noise. Implicit in the masking hypothesis is the assumption that all species process sounds in noise similarly and will therefore be subject to similar masking effects. However, the emerging comparative literature on auditory processing in birds suggests that there may be significant differences in how different species process sound, both in quiet and in noise. In this paper we will (1) briefly review the literature on anthropogenic noise and birds, (2) provide a mechanistic overview of how noise impacts auditory processing, (3) review what is known about the comparative avian auditory processing in noise, and (4) discuss the implications of species level differences in auditory processing for behavioral and physiological responses to anthropogenic noise.
The effects of anthropogenic noise on birds
With increasing urbanization across the globe, the threat of anthropogenic noise pollution to animals will increase over time (Brumm and Horn, 2019). Commonplace sounds found in areas of human settlement, including traffic and industrial noises, can have adverse effects on the behavior and health of wildlife (Barber et al., 2010). In accordance with the growing threat, research interest on the topic has steadily risen since the turn of the century, with publications including keywords such as “traffic noise” having increased at a greater rate than the total output of scientific papers.
Anthropogenic noise produces a wide array of negative effects in animals. Across species, anthropogenic noise leads to reduced rates of survival (Simpson et al., 2016), greater physiological stress responses (Kleist et al., 2018), poorer offspring success (Nedelec et al., 2017; Zollinger et al., 2019), and decreased cognitive performance (Maes and De Groot, 2003; Stansfeld and Matheson, 2003; Osbrink et al., 2021). Additionally, noise has been shown to have a number of adverse effects on acoustically-mediated behaviors. For instance, noise can lead to decreased anti-predator responses (Jung et al., 2020), lower foraging success (Mason et al., 2016; Halfwerk and van Oers, 2020), and increased time spent on vigilance (Meillère et al., 2015), presumably to compensate for diminished acoustic detection of threats. Perhaps the best studied effects of noise on acoustically-mediated behaviors are the impacts of noise on songbird communication.
Songbirds have long been a model for acoustic communication and therefore became a natural model for understanding how communication is affected by noise, both natural and anthropogenic (Brumm and Naguib, 2009; Naguib, 2013; Wiley, 2017). Anthropogenic noise can overlap signals produced by songbirds, which results in masking of these signals (Slabbekoorn and Peet, 2003). This masking is a problem both from a sender and a receiver perspective, although most research has focused on the impacts of noise on the production of the signal by the sender. Senders may benefit from a variety of strategies to enhance signal detectability including moving closer to the intended receiver or relocating to a higher perch (Dabelsteen et al., 1993; Mathevon et al., 1996; Mathevon et al., 2005), producing louder vocalizations (Brumm and Todt, 2002; Brumm, 2004), or producing signals during times when noise levels are lower (Fuller et al., 2007; Dominoni et al., 2016). Additionally, anthropogenic masking noise can result in frequency shifts in vocal signals (Brumm and Slabbekoorn, 2005; LaZerte et al., 2016), although there is some evidence to suggest that these pitch-shifts are a byproduct of singing at a higher amplitude which may be more effective in enhancing signal transmission (Nemeth and Brumm, 2010; Brumm and Zollinger, 2011). Receivers can employ similar strategies to senders and have additional options, such as changing the orientation of the head (Dent et al., 1997; Dooling and Leek, 2018). However, not all strategies are available to all species (Zollinger et al., 2017).
Some tactics to reduce the impact of noise seem to be relatively conserved across species, while others vary considerably in their employment. For instance, moving to a higher song post to enhance signal detectability is found in many species (see Mathevon et al., 2005; Barker and Mennill, 2009; Sprau et al., 2012), implying that this tactic may be effective for enhancing signal detection in noise regardless of the sensory biases of a particular species (Polak, 2014; Halfwerk et al., 2018). Pitch shifting also appears to be a common, (Bermúdez-Cuamatzin et al., 2011; Seger-Fullam et al., 2011; LaZerte et al., 2016; Roca et al., 2016; Halfwerk et al., 2018), but not universally employed tactic (Roca et al., 2016; Zollinger et al., 2017) and there appear to be constraints on the degree of pitch shifting that can occur. There is substantially more variability in whether species are able to switch to a new song type to reduce the effects of noise (Slabbekoorn and den Boer-Visser, 2006; Dowling et al., 2012). Some species appear to increase song switching in noise, while others reduce switching between song types, perhaps to increase redundancy of information (Brumm and Slater, 2006). Some species are known to alter the timing of song production on both relatively short (e.g., seconds to minutes; Brumm et al., 2009; Francis et al., 2011) or long-term (e.g., time of day; Fuller et al., 2007; Dominoni et al., 2016; Dorado-Correa et al., 2016) times scales. It is not yet clear how universally these time shifting tactics are employed, although they appear to be inaccessible to at least some species (Yang and Slabbekoorn, 2014).
Many of the behavioral responses to noise are thought to be a result of the masking effects of noise, although noise may also be aversive or distracting (Chan et al., 2010; Morris-Drake et al., 2016; Zhou et al., 2019). In all cases, the response to noise is mediated through the detection of noise by the auditory system and the effect of noise on the processing of signals of interested. For instance, signal parameters including frequency and duration have been shown to enhance auditory perception of signals by great tits (Parus major) in anthropogenic noise (Pohl et al., 2009; Pohl et al., 2012; Pohl et al., 2013). Yet, despite an understanding of how senders respond to noise and a growing understanding of how receivers behaviorally respond to signals in noise, there is surprisingly little comparative work on auditory processing of signals in noise for birds, songbirds in particular. Indeed, many papers on signal production and/or communication in anthropogenic noise that have invoked the masking hypothesis focus only on the relative overlap of the signal and noise in frequency, either incorrectly assuming that all species are processing signals in noise in an identical manner or ignoring the issue of auditory processing entirely. Notably, though, species can vary significantly in their auditory processing abilities in quiet and, although less explored, in noise. Therefore, differences in behavioral response to noise may be mediated by species-specific variation in the ability of the auditory system to process signals in noise. These variations, however, are rarely considered at the comparative level. Here we review the comparative literature on avian auditory processing in noise, focusing primarily on masked thresholds and critical ratios, as the greatest comparative literature exists for these metrics. However, these are relatively simplistic metrics and future work on more complex metrics of auditory processing in noise, including comodulation and spatial masking release, dip-listening, and the impact of signal features on detection and discrimination in noise, in a comparative framework would be particularly valuable (see Klump and Langemann, 1995; Dent et al., 1997; Dent et al., 2009; Pohl et al., 2009; Pohl et al., 2012; Pohl et al., 2013).
How the auditory system deals with noise
The cochlea in birds responds to sound based on many of the same general peripheral neural encoding principles found in mammals (reviewed in Gleich and Manley, 2000). Briefly, the avian cochlea in birds is tonotopically organized, with each location along the length of the hair-cell epithelium (basilar papilla in birds) tuned to a different characteristic frequency (CF, Table 1). Spectral analysis of sound, due to this tonotopic response, allows separation of competing sounds based on differences in frequency content, and therefore provides an important foundation for processing signals in noise as discussed below. In contrast to basic similarities in peripheral neural processing, the anatomy of the avian cochlea shows only a slight curvature along its longitudinal axis, while the mammalian cochlea has a prominent coiled structure, and the avian hair-cell epithelium is comparatively short and broad with up to thirty hair cells across the width in low-CF apical regions (Takasaka and Smith, 1971). Moreover, rather than distinct populations of specialized inner and outer hair cells as found in mammals, birds show a hair-cell gradient across the width of the epithelium from “tall” to “short” morphology. Tall hair cells are taller than they are wide, are located closer to the epithelial edge overlying the auditory-nerve ganglion, and are innervated by peripheral axons of afferent myelinated auditory-nerve fibers via ribbon synapses (Fischer, 1994), similar to mammalian inner hair cells. Short hair cells receive primarily unmyelinated efferent innervation and are implicated in cochlear nonlinearity and amplification like mammalian outer hair cells (Manley et al., 1989). Several aspects of tall/inner hair-cell innervation by afferent nerve fibers are shared between birds and mammals: each afferent neuron makes synaptic contact with a single tall hair cell through a peripheral axon terminating in a ribbon synapse, and has its soma located in the auditory-nerve ganglion (called spiral ganglion in mammals) at short distance from the hair cells. Central axons of auditory-nerve fibers bundle together to exit the cochlea and provide the primary input to the ascending auditory pathway.
Perhaps surprisingly, in light of peripheral anatomical differences, auditory-nerve fiber response properties in birds are similar in most respects to those reported in mammals (Sachs et al., 1974; Manley et al., 1985). Avian auditory-nerve fibers show irregular spontaneous discharge activity in the absence of sound, of variable rate (typically up to 100 spikes per second) across fibers and perhaps with an inverse relationship to the threshold at CF (Salvi et al., 1992). Average discharge rate increases with increasing sound level over a limited dynamic range before saturating at the maximum discharge rate. In response to tones of varying frequency and level, auditory-nerve fibers in birds show V-shaped threshold tuning curves with CFs (i.e., the frequency of the tuning curve tip) distributed across the frequency range of hearing sensitivity. Notably, measures of frequency selectivity such as Q10, defined as the CF divided by the bandwidth of the tuning curve 10 dB above the threshold at CF, are similar to if not slightly sharper than observed in typical mammalian species (reviewed in Gleich and Manley, 2000). These measures of frequency selectivity are of significance because this spectral analysis in the peripheral auditory system is a key mechanism facilitating detection and processing of signals in noise, and is the foundation of auditory filtering models (see below). Regarding temporal response properties, auditory-nerve fibers in birds show prominent phase locking to tone frequencies up to several kHz (Sachs et al., 1974; Manley et al., 1985; Gleich and Narins, 1988; Köppl, 1997), as well as to amplitude fluctuations in the envelopes of complex sounds (Gleich and Klump, 1995). All of the patterns described above are commonly seen in mammals. Indeed, the main differences in avian peripheral physiology compared to mammals appear to be tuning curves that are constrained to lower CFs (due to filtering properties of the avian middle ear system), slightly higher spontaneous and maximum discharge rates (perhaps associated with higher average body temperature in birds; Sachs et al., 1974), and higher thresholds for tone frequencies presented below CF (i.e., no tuning curve tails as commonly observed in mammalian auditory-nerve-fiber responses; Manley et al., 1985).
The frequency tuning of auditory neurons arises from tonotopy of the hair-cell epithelium within the cochlea and is the physiological manifestation of now classic band-pass auditory filtering models for hearing in noise (Fletcher, 1940; Glasberg and Moore, 1990). In this model framework, a narrowband signal such as a tone is detected in a competing noise “masker” based on the response of a single auditory filter centered on the tone frequency. For the classic critical-band model, which we considered first, detection of a tone in noise is based on an increase in the output level of the filter compared to a reference condition for which only the noise is presented. Detection is possible because for a tone of sufficiently high signal-to-noise ratio, addition of the tone to fixed-level noise increases the overall energy level at the output of the filter. Importantly, the sensitivity of the system for detecting the tone in noise depends crucially on the bandwidth of the auditory filter. These basic principles are illustrated in Figure 1, which in panel A shows input–output functions of auditory-filter models with bandwidths ranging from 100 to 1600 Hz. The threshold “critical ratio” occurs at the signal-to-noise ratio (SNR), expressed relative to the noise spectrum level, above which the distribution of energy-level values observed across the tone-plus-noise stimulus trials (vertical error bars) sufficiently exceeds the distribution observed across the noise-alone trials (horizontal band height). Crucially, auditory filters of greater bandwidth passes more total noise energy than narrow filters, which consequently increases the threshold critical ratio above which addition of the tone to the noise masker appreciably increases filter output. The resulting dependence between filter bandwidth and critical ratio is illustrated in Figure 1B. It is based on the above relationships between auditory filter bandwidth and masked threshold that critical ratios have been used for decades now as a first approximation for peripheral tuning bandwidths. Behavioral critical ratios in humans and many nonhuman animal species increase with increasing tone frequency (see further discussion below), in general agreement with typical increases in neural tuning bandwidth for higher CFs.
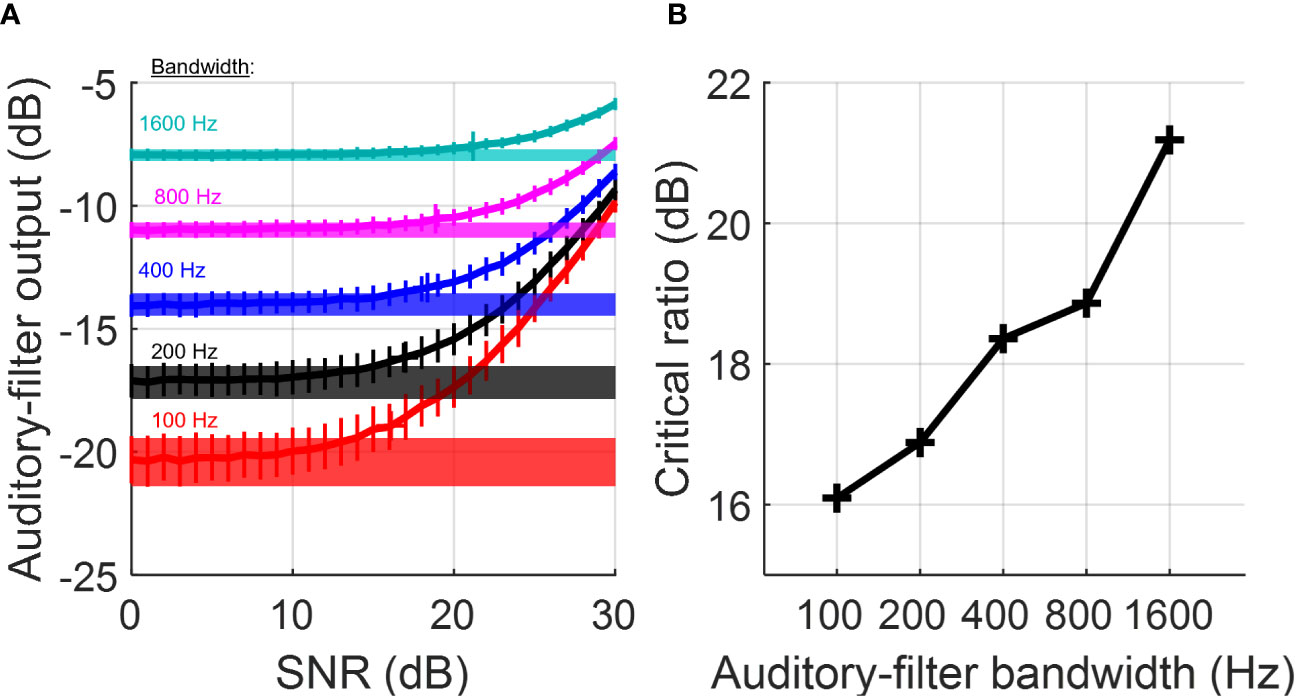
Figure 1 Dependence of tone-in-noise sensitivity on auditory-filter bandwidth. Input–output relationships of auditory-filter simulations (A) showing filter output (mean ± SD) as a function of stimulus signal-to-noise ratio (SNR) for filter bandwidths ranging from 100–1600 Hz (color-keyed text). Thick horizontal bands indicate filter output for noise alone (mean ± SD). The threshold “critical ratio” is defined as the SNR (re. noise spectrum level) above which the distribution of filter output between tone-plus-noise trials and noise alone differ by ≥1.5 SD. Critical ratios for tone-in-noise detection (B) increase for greater auditory filter bandwidths. Simulations were conducted in MATLAB using 12th order Butterworth filters, stimulus duration of 0.2 s, tone frequency of 2000 Hz, and 200 randomly generated stimuli per SNR.
The critical-band model discussed above provides only a starting point for understanding auditory processing in noise, as organisms clearly have access to other neural cues than those related to output energy from single auditory filters. Adding a tone to noise introduces temporal fine structure at the output of auditory filters, which can be encoded through neural phase locking (Costalupes, 1985; Henry and Heinz, 2012), and alters the statistics of the amplitude envelope at the filter output as well (Kohlrausch et al., 1997; Wang et al., 2021). Furthermore, neurons along the central auditory pathway can combine information across peripheral auditory-filter channels to perform signal-processing tasks in noise. For example, European starlings (Sturnus vulgaris) show improved detection of tones in noise when amplitude fluctuations are coherent across frequency bands of the masker rather than independent (Klump and Langemann, 1995). This phenomenon, known as comodulation masking release, has been widely studied in humans and is not explainable by the critical-band model. Consistent with limitations of the basic critical-band model, auditory behavioral studies show that birds perceive sound in ways inconsistent with its predictions, as also found in humans. For the basic task of tone-in-noise detection for which overall stimulus is randomly varied (“roved”) across trials, budgerigars (Melopsittacus undulatus) show essentially no change in sensitivity compared to fixed-level listening conditions (Henry et al., 2020; Henry and Abrams, 2021). This result echoes findings of human studies (Richards, 1992; Leong et al., 2020) but not the critical-band model, which in its basic form predicts large threshold shifts (impaired performance) for the roving-level condition. Follow-up analyses of trial-by-trial error patterns in budgerigars using decision-variable correlations showed that while behavioral responses were related to some extent with trial-by-trial variation in stimulus energy level, behavioral choices were also strongly associated with envelope cues (Henry et al., 2020; Henry and Abrams, 2021), also echoing human findings (Mao et al., 2015). For tone-in-noise detection, the envelope cue is a flattening of the envelope at the output of the auditory filter that occurs when a tone is added to the noise masker (Kohlrausch et al., 1997).
Another important limitation of the critical-band model is the inability to explain masking in the modulation domain, which is masking of amplitude-modulated signals by envelope fluctuations of similar frequency that occur in the noise background (Bacon and Grantham, 1989; Houtgast, 1989). Human listeners show band-pass modulation masking patterns consistent with a modulation filterbank processing strategy (Hose et al., 1987; Dau et al., 1997a; Dau et al., 1997b; Ewert et al., 2002) thought to occur in the central nervous system. Similar “modulation tuning” of neural responses has also been observed at the forebrain level in the mynah bird (Hose et al., 1987). Neural recordings at the midbrain processing level in zebra finches and budgerigars show band-pass modulation transfer functions in response to sinusoidally amplitude-modulated stimuli (Woolley and Casseday, 2005; Henry et al., 2016) that could potentially support perceptual modulation filtering. This aspect of hearing in noise could be especially important for avian species that communicate with amplitude and/or frequency modulated signals in environments with rapid envelope fluctuations. Studying masking in the modulation domain, temporal integration, and spectro-temporal masking effects such as comodulation masking release in a broad comparative context should be an important priority for future research, because it can provide much needed clarity into the evolution of auditory processing mechanisms for hearing in noise.
Comparative avian auditory processing in noise
Although we have a detailed understanding of how noise affects auditory processing in a few model organisms (see above) and a growing body of work on comparative avian auditory processing, there is still substantially less known about avian auditory processing in noise in a comparative framework. There have generally been two methodological approaches taken for comparative auditory processing work: psychophysics and gross electrophysiology, specifically auditory evoked potentials. Other techniques that have been employed to study auditory processing, such as single unit electrophysiology in the auditory nerve or auditory forebrain have been employed almost exclusively in model organisms, with a few exceptions, and we will not cover those here.
Psychophysical experiments provide a comprehensive, whole-organism response to acoustic stimuli and the results of these types of experiments may most closely mirror the responses of free-living birds to sound. However, the approach has limitations as well. Psychophysical experiments are sensitive to the motivation and learning abilities of individual subjects, are limited to subjects that can be sufficiently rewarded with access to food, and require prolonged housing in a laboratory setting. Historically, the results of such studies were based on a relatively limited number of subjects (typically 1–4 in critical ratio experiments, see Supplementary Table S1) and the same subjects were often used in several experiments. Additionally, repeated training on detection and discrimination tasks may result in an overestimate of detection and discrimination abilities in free-living birds that may have more demands on their attention. The training paradigm may also select for individuals that are not representative of the species.
Auditory evoked potentials can be employed for a diverse range of species, as only temporary laboratory housing is required, effectively increasing the breadth of comparative work. However, this technique represents only the subcortical processing of auditory information and is the summed response of all neural units responding to sound (Henry et al., 2017). Some aspects of audition, such as auditory thresholds, measured via auditory evoked potentials (AEPs) have been shown to correlate well with single unit and psychophysical measurements in a variety of avian taxa (Brittan-Powell et al., 2002; Gall et al., 2011; McGrew et al., 2022). However, AEP estimates of auditory thresholds are generally elevated relative to psychophysical thresholds and correlations are strongest in the frequency range of greatest sensitivity. For other aspects of auditory processing, such as temporal resolution, frequency resolution, and auditory processing in noise, there are very few species for which multiple methodological approaches have been taken and therefore the relationship between the peripheral processing and whole organism response is largely unknown. AEP estimates of auditory processing, therefore, are best used as relative comparisons among species, rather than absolute estimates of audition.
Noise can affect the detection, discrimination and recognition of acoustic information and these effects are a product of both peripheral processing and higher order cognitive functions (Dooling and Leek, 2018). However, most of our comparative understanding of avian audition comes from estimates of absolute sensitivities in quiet anechoic chambers. Although these absolute sensitivities are not fully sufficient to understand auditory processing in noise, they may provide some guidance on which species may be most affected by noise. Anthropogenic noise is largely concentrated at lower frequencies, although as the amplitude of noise increases there are concomitant changes in the upward spread of excitation (Slabbekoorn et al., 2018). This suggests that in moderate levels of noise species with lower peak frequencies of sensitivity or with greater sensitivity overall may be more subject to the effects of masking, as noise is more likely to overlap with their regions of best sensitivity and exceed their thresholds.
Absolute auditory thresholds have been estimated for many dozens of species spanning most of the major taxonomic clades using both psychoacoustic techniques (see Dooling et al., 2000; Crowell et al., 2016; Dooling and Blumenrath, 2016) and auditory evoked potentials (Pytte et al., 2004; Brittan-Powell et al., 2005; Lohr et al., 2013; Henry et al., 2017; Crowell et al., 2015; Beatini et al., 2018; McGee et al., 2019; Larsen et al., 2020). The shape of the audibility curve (i.e., audiogram) is broadly similar across birds. Peak sensitivity lies somewhere between 1 and 6 kHz for most avian species, with a steep increase in thresholds at frequencies above and below the range of peak sensitivity. However, differences do emerge both across and within clades that may influence the relative sensitivity to noise. Very broadly, the audibility curves for larger-bodied bird species, including members of the Anseriformes, Galliformes, and some Accipitriformes, are typically shifted away from higher frequencies relative to the audibility curves of smaller-bodied species, such as those of the order Passeriformes (reviewed in Dooling et al., 2000; Crowell et al., 2015; Crowell et al., 2016; McGee, 2019). However, there are also substantial differences among species that are likely a product of behavior and ecology. For instance, Northern gannets (Morus bassanus) have among the highest thresholds (i.e., low sensitivity) of any species measured with the AEP method and a peak sensitivity of only 1.7 kHz, lower than any other seabird tested (Crowell et al., 2015). This poor auditory sensitivity may be a result of their plunge diving foraging methods, high natural levels of noise in their environments, and relatively short distances over which they typically communicate. Northern gannets also have a small and thickened tympanic membrane, which may reduce damage during plunge diving at the expense of auditory sensitivity (Crowell et al., 2015). Masking noise, therefore, may have relatively little effect on this species except at very high amplitudes. On the other hand, barn owls (Tyto alba; psychophysical method Konishi, 1973; Dyson et al., 1998; AEP method Thiele and Koppl, 2018) and Northern saw-whet owls (Aegolius acadicus; AEP method Beatini et al., 2018) have the among the lowest thresholds for birds, as well as an extended range of peak sensitivity from 1.6 to 7.1 kHz. This again, is likely a reflection of the behavior and ecology of the animals. Barn owls and Northern saw-whet owls can hunt in total darkness, localizing prey by sound, which requires enhanced auditory sensitivity relative to other species (Payne, 1971; Mason et al., 2016). Barn owls and Northern saw-whet owls both have asymmetric ears and complete facial ruffs that likely enhance sound detection and localization (Norberg, 1977; Knudsen and Konishi, 1979; Moiseff, 1989). Although thresholds have not been determined psychophysically for Northern saw-whet owls, they have been shown behaviorally to have excellent sound localization abilities (Frost et al., 1989). The extremely low thresholds suggest that even very low amplitude masking noises may have an effect on the detection abilities of owls (Mason et al., 2016).
Although the differences in audibility curves are more subtle between species in the same order, differences in the frequency range of best sensitivity have been shown in free-living passerines (reviewed in Henry et al., 2017). These differences are often, but not always perfectly, aligned with the peak energy in their vocalizations. For example, Velez et al. (2015) found that song complexity, rather than habitat or peak frequency of vocalizations, best predicted the high frequency sensitivity of nine songbird species. Therefore, estimates of sensitivity to masking that rely solely on vocal analysis may be misleading, as auditory sensitivity and vocal energy are not always perfectly correlated.
The comparative information available for auditory processing in noise is more limited than for absolute sensitivity. There are several ways in which we can assess the effects on noise on auditory processing, but here we will focus primarily on the effect of noise on detection limits (i.e., thresholds), as we have the most information for this metric. This is a relatively simplistic way of approaching auditory processing in noise. To fully understand the effects of auditory processing on communication in noise requires a comparative understanding of masking in the modulation domain, temporal integration, spectro-temporal masking effects such as comodulation masking release, dip-listening in modulated maskers and spatial masking release. Currently, however, this information is available for only a small number of avian species (e.g., Klump and Langemann, 1995). Thus, we have largely limited our review to metrics available for a larger number of species, although we also briefly discuss several types of masking release and encourage comparative work that extends to these more nuanced aspects of auditory processing in noise.
We can assess the effects of noise on detection limits with masked thresholds and critical ratios. Masked thresholds are produced using procedures identical to those for producing audiograms, with the addition of a noise masker (typically white noise). When the masker is wideband white noise and the signal is a pure tone, the detection threshold for the tone expressed in dB relative to the spectrum level of the noise is known as the critical ratio. On average, critical ratios for avian species are approximately 22 dB at 0.5 kHz and 29 dB at 4 kHz. However, there is also a fair amount of variation across species in both the magnitude of their critical ratios and the shape of the critical ratio by frequency function. For instance, at 1 kHz critical ratios range from 21 dB in wild-caught hooded crows (Corvus corone cornix; Jensen and Klokker, 2006) to 42 dB in red-winged blackbirds (Agelaius phoeniceus) and brown-headed cowbirds (Molothrus ater; Hienz and Sachs, 1987). Many of the other songbird species have critical ratios in the 24–28 dB range at 1 kHz (Supplementary Table S1; Okanoya and Dooling, 1987; Langemann et al., 1998; Okanoya and Dooling 1988; Lohr et al., 2003; Noirot et al., 2011). This suggests that at 1 kHz, a frequency at which there is often substantial anthropogenic noise, blackbirds and cowbirds might be much more susceptible to the effects of noise masking than other species.
Different patterns emerge at 4 kHz, a frequency generally considered less susceptible to masking at all but the highest anthropogenic noise levels. Critical ratios range from 20.5 dB in budgerigars (Dooling and Saunders, 1975) on the low end and 34–37 dB for canaries (Serinus canaria; Okanoya and Dooling, 1987), blackbirds and cowbirds on the high end. Many of the songbirds lie in between, for instance a critical ratio of 29 dB for song (Melospiza melodia) and swamp sparrows (Melospiza georgiana) (Figure 2, Supplementary Table S1). European barns owls had critical ratios of 23.5 dB at 4 kHz and 27.5 dB at 6.3 kHz (Dyson et al., 1998). While these frequencies are not usually considered as susceptible to masking as lower frequencies, barn owls have an absolute threshold of −14.2 dB SPL at 6.3 kHz (Dyson et al., 1998), thus masking of low amplitude prey noise at 6.3 kHz could occur even with relatively small amounts of masking noise.
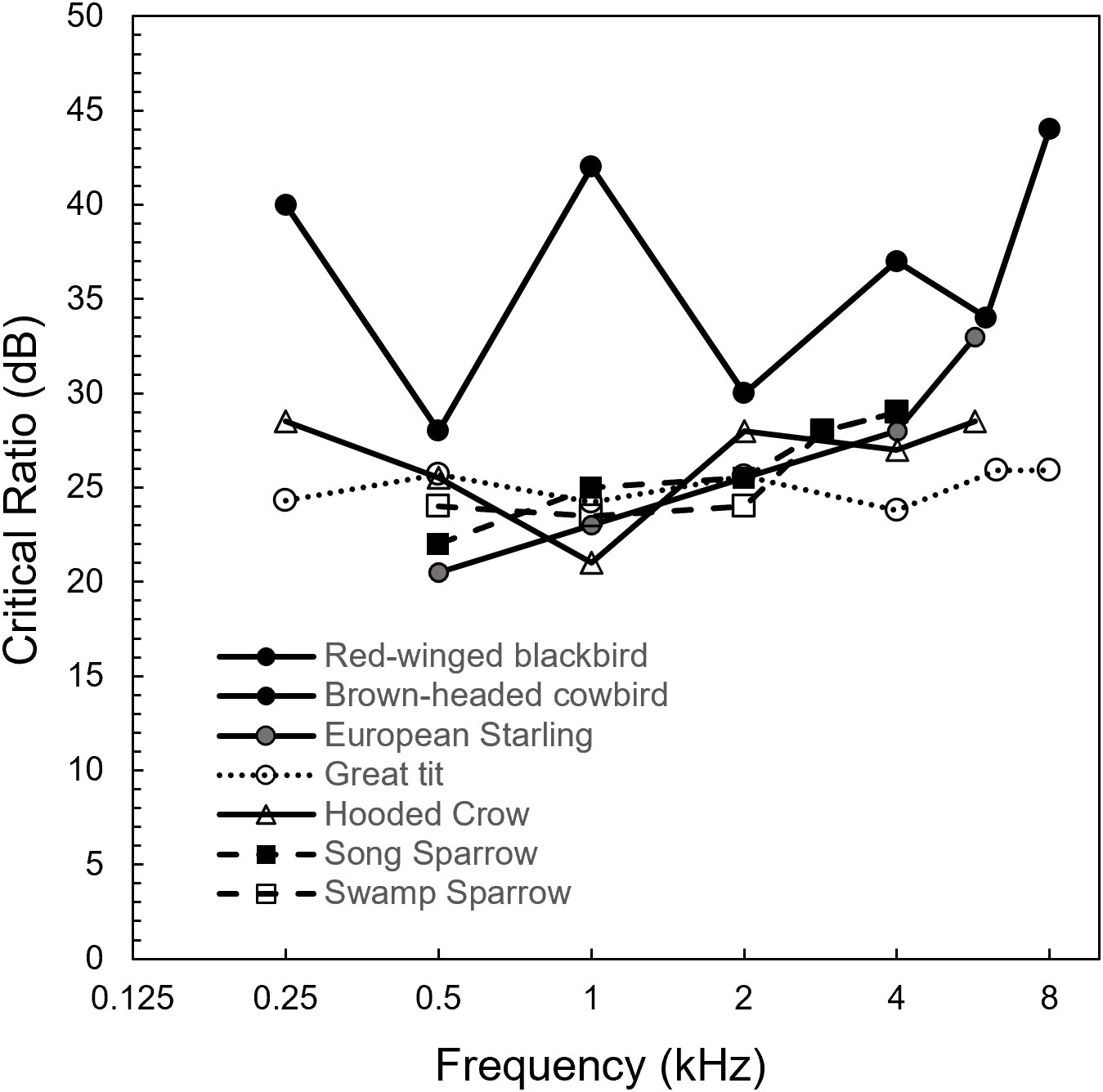
Figure 2 Critical ratio by frequency functions for all wild-caught passerine songbirds which have been studied to date. Not included are critical ratios of lab-bred or purchased songbirds. Data from Hienz and Sachs, 1987; Okanoya and Dooling, 1988; Langemann et al., 1998; Jensen and Klokker, 2006. Data were taken from the texts or table when available, or extrapolated to the nearest 0.5 dB from figures.
On average, the critical ratios of birds appear to increase at a rate of 2–3 dB per octave. However, there is substantial variation in the shape of the critical ratio by frequency function. Generally, these functions fall into one of three categories: (1) The standard increase of 2–3 (and up to 5.1) dB per octave, (2) relatively flat functions and (3) relative flat functions with sharp increases at high frequencies. In some cases (functions 2 and 3) the critical ratio initially appears to decrease as the function moves towards the best frequency for each species, before increasing rapidly at higher frequencies. Species that appear to follow the 2–3 dB increase per octave pattern include canaries, zebra finches (Taeniopygia guttata), European starlings (Langemann et al., 1995), song sparrows, swamp sparrows (Okanoya and Dooling, 1987), and domestic pigeons (Columba livia domestica; Hienz and Sachs, 1987). Species with relatively flat critical ratio by frequency functions include hooded crows (Jensen and Klokker, 2006), red-winged blackbirds, brown-headed cowbirds (Hienz and Sachs, 1987), and great tits (Langemann et al., 1998). Orange-fronted conures (Aratinga canicularis; Wright et al., 2003) and parakeets (a.k.a. budgerigars; Dooling and Saunders, 1975; Saunders et al., 1979; Okanoya and Dooling, 1987; Hashino et al., 1988) show relatively flat or slightly decreasing critical ratios as frequency increases towards best frequency, with a rapid increase in critical ratio at or above 5 kHz. Note that for all these psychoacoustic measurements of critical ratios, the sample sizes are quite small, so we don’t have a good estimate of the variation among individuals in critical ratios, nor the ability to statistically compare them.
Critical ratios have also been estimated for three species (budgerigars, canaries, and zebra finches) using auditory evoked potentials (Noirot et al., 2011). Generally, the AEP method results in critical ratios that are 18–23 dB higher than those measured psychophysically. Additionally, the shape of the critical ratio by frequency function appears to largely reflect the functions determined psychophysically, although the magnitude of differences between the two methods seems to be larger outside of the region of best sensitivity. The critical ratios measured via the AEP method appear to initially decrease or remain fairly flat as the frequency increases towards best frequency and then critical ratios increase as the stimulus frequency increases past best frequency. We have found similar critical ratio by frequency functions in three additional passerine species: black-capped chickadees, tufted titmice, and white-breasted nuthatches (Chou et al., unpublished). Additionally, in Northern saw-whet owls we have found a relatively flat critical ratio by frequency function, with a rapid increase in critical ratio at higher frequencies (Desai et al., submitted), a shape that largely mirrors the critical ratio function in barn owls (Dyson et al., 1998).
Attempts to measure the auditory processing of more complex signals in noise and conversely the processing of signals in more complex noise have covered a much narrower range of species, typically limited to traditional avian model species (e.g., zebra finch, canaries, budgerigars, and starlings). For instance, Lohr et al. (2003) measured detection and discrimination thresholds for natural contact call stimuli of zebra finches and canaries in flat and traffic shaped noise. Generally, they found that as the bandwidth of the calls increased and as the level of masking noise increased, so did the thresholds. Thresholds for discrimination were also higher than thresholds for detection, suggesting the active space of signals may be much smaller than previously estimated in communication scenarios where the signals need to be discriminated. Pohl et al. (2009) similarly found that great tit detection thresholds were impacted by the features of both signal elements and noise. Thresholds were lowest in quiet and increased in woodland and urban noise, with the highest thresholds in dawn chorus noise. Detection thresholds were lower for signal elements with narrower bandwidths (Pohl et al., 2009) and longer durations (Pohl et al., 2013). Additionally, higher frequency songs increased detection and discrimination of songs in urban noise for great tits (Pohl et al., 2012). Dooling and Blumenrath (2016) found that the critical ratios of budgerigars and canaries for simple stimuli in constant flat noise were similar to those in temporally fluctuating anthropogenic noise, suggesting that standard critical ratios could be reasonably applied to models of signal active spaces.
Birds may benefit also from maskers that are spatially segregated from the signal of interest or are modulated in amplitude (Klump and Langemann, 1995; Dent et al., 1997; Langemann and Klump, 2001; Dent et al., 2009). Moreover, there is reason to believe that species may differ in the degree of unmasking that is experienced for different signals and maskers. For instance, spatially separating a pure tone signals from white noise maskers by 90° or 180° can result in 8–9 dB of masking release in budgerigars (Dent et al., 1997). However, on a more naturalistic task – detecting a zebra finch vocalization in short duration maskers resembling songs or choruses – budgerigars did not experience a significant release from masking (an average of 1.6 dB) when signal and masker were segregated by 90°. Zebra finch performance improved (an average of 6.8 dB of masking released) at 90°, but showed no improvement when signal and masker were segregated by 180° (Dent et al., 2009). When maskers were presented continuously zebra finches experienced greater spatial release from masking (average 29 dB) than budgerigars (average of 20 dB), suggesting that species may differ significantly in the amount of spatial release from masking they experience for a given signal. Modulation of masker amplitude may also impact signal detection. Starlings experience an average of 11.8 dB of comodulation masking release when signals are presented in coherently modulated noise compared to unmodulated noise, with the greatest masking release in broader band maskers (Klump and Langemann, 1995). Additionally, starlings have been shown to experience up to 28 dB of masking releasing when target stimuli are presented during the troughs of coherently modulated maskers compared to targets presented during the peaks or in incoherently modulated maskers (Langemann and Klump, 2001). The greatest masking release occurred when the masker was spread across multiple auditory filters, rather than confined to the one auditory filter. Although the temporal modulation of noise is likely to be important in signal detection, it is not yet clear how much variation exists across species in the degree of masking release experienced in modulated maskers.
There have been some attempts to investigate the effects of noise on the processing of more complex signals using the AEP method. For instance, Goller et al. (2022) presented tones, amplitude modulated, and frequency modulated signals to bald eagles (Haliaeetus leucocephalus) and golden eagles (Aquila chrysaetos) in either white or pink noise. They found that noise had a substantial effect on less dynamic signals (i.e., those with less modulation) but that processing of more modulated signals was less effected by the addition of noise. This suggests that species with dynamic signals may experience less masking than those with more static signals, although this is somewhat at odds with the findings of Lohr et al. (2003) and Pohl et al. (2009) that broader bandwidth signals were more susceptible to masking.
Finally, some features of auditory processing (i.e., critical bands and auditory filter bandwidth) that can be determined directly with single unit techniques cannot be directly measured with psychophysical or auditory evoked potential techniques. Here the effects of noise masking are not the primary interest, rather noise-masked signals are used to suppress responses from adjacent cochlear partitions. The psychophysical estimates of critical bands appear to correspond well to the (4dB) bandwidth of tuning curves (i.e., auditory filter bandwidths) determined from single units in starlings (Langemann et al., 1995) and frequency representation on the basilar papilla (octaves mm−1) is correlated with critical ratios across several species (Gleich and Langemann, 2011), suggesting that masking effects of noise may be related to the frequency selectivity of the auditory periphery. Additionally, the output of an auditory filter model correlates well with perceptual distances between song exemplars in two noise conditions in great tits (Pohl et al., 2012), further suggesting that auditory filter widths may provide some insight into signal processing in noise.
Auditory filter bandwidths have also been determined for several species from both psychophysical and AEP tone thresholds in notched-noise maskers. Psychophysical estimates of auditory filter bandwidths in starlings increase in absolute width with increasing center frequency and correspond well with other methods of determining filter width (Marean et al., 1998). Auditory filter bandwidths have been estimated via AEPs for wild-caught Carolina chickadees, tufted titmice, white-breasted nuthatches, dark-eyed juncos (Junco hyemalis), brown-headed cowbirds, red-winged blackbirds, and house sparrows (Passer domesticus) (Gall and Lucas, 2010; Henry and Lucas, 2010a; Henry and Lucas, 2010b; Henry et al., 2011; Gall et al., 2013). In nearly all of these species filter bandwidth increases with increasing center frequency, with the exception of dark-eyed juncos, in which auditory filter bandwidth decreased slightly with increasing center frequency. Generally, open habitat species tended to have broader filters than woodland species (Henry and Lucas, 2010b). There is some evidence that there is sex-specific seasonal variation in auditory filter width in house sparrows, with females having narrower auditory filters than males during the breeding season, with no sex difference during the non-breeding season (Gall et al., 2013). Narrower filter bandwidth may increase neural synchrony to temporal fine structure in noise, based on studies of sensorineural hearing loss in the mammalian auditory system (Henry and Heinz, 2012).
As discussed above, filter bandwidth might affect the processing of signals in noise, with wider filters generally assumed to result in poor signal in noise processing, although this is likely an oversimplistic view. The notched-noise method also allows for the estimation of the efficiency or signal-to-noise ratios of the filters (K’), which do not always directly correspond to filter bandwidth. For instance, although white-breasted nuthatches have fairly narrow filters, they had a higher K’ than other woodland and open habitat species, suggesting that they may be more susceptible to masking than other species. Additionally, female chickadees had narrower auditory filters than males, but a higher K’ suggesting less efficient extraction of signals from noise (Henry and Lucas, 2010a). On the other hand, brown-headed cowbird females had both narrower auditory filters and lower K’ than males, suggesting greater frequency selectivity and improved signal-in-noise detection (Gall and Lucas, 2010). Together, the comparative literature suggests that different species, and perhaps even different individuals within the same species, may differ in their ability to detect and discriminate signals in noisy backgrounds, although there is much we have left to learn. Therefore, we must consider not only the signals and the noise spectrum when attempting to understand the effects of anthropogenic noise on communication and other acoustically-mediated behaviors, but also the relative auditory sensitivity of different species and their ability to extract signals in environmental noise. In the next section, we speculate on the effects that variation in auditory processing abilities might have on the behavioral responses of different species to noise.
Linking auditory processing to behavior in noise
The evolution of communication systems has been shaped by the natural noise of the environment (Klump, 1996; Wiley, 2017). Natural noise differs in amplitude and spectral and temporal properties across habitats, which can influence both the evolution of the signals and the properties of the auditory system of different species (Brumm and Naguib, 2009). The perceptual properties and signal structure of a given species may evolve to optimize signal detection under natural noise conditions (Wiley, 2017). The amplitude, spectrum, and timing of anthropogenic noise can vary significantly from natural noise and with sound source, so the relative impact of anthropogenic noise on each species will depend on particulars of the environment. However, if we consider a single case, we can see the effect that even moderate noise might have on signal detection. Nemeth and Brumm (2010) found city noise to have a spectrum level of approximately 23 ± 7.5 dB/Hz at 1 kHz, while forest noise had a spectrum level of only 6 dB/Hz. The critical ratio represents the signal level relative to this noise level that is needed for detection. Therefore, we can determine the signal spectrum level needed for detection by adding the critical ratio to the spectrum level of the noise for a given frequency. Under this noise paradigm, the introductory notes in the songs of red-winged blackbirds and brown-headed cowbirds would need to reach an amplitude of 65 dB at 1 kHz for detection at even a relatively short range in anthropogenic noise, while song sparrows, swamp sparrows, and great tits would need a signal of only 45.5–48 dB.
However, even this doesn’t tell the full story. While red-winged blackbirds and brown-headed cowbirds have very similar auditory processing (Hienz and Sachs, 1987; Gall et al., 2011; Figure 2) they differ dramatically in the spectral content of their songs and their reproductive strategies. Red-winged blackbirds have vocalizations with peak energy concentrated between 2 and 4 kHz, corresponding to their best sensitivity. Brown-headed cowbirds, have songs with a similar structure, but with much lower and higher frequency components in their songs (Lowther, 2020). These low amplitude elements are likely to be masked by anthropogenic noise, while the higher frequency components may largely evade anthropogenic noise masking. Red-winged blackbirds have high amplitude vocalizations that they use for advertisement and territory defense. The high amplitude of their vocalizations may be sufficient to overcome relatively high levels of masking, particularly at short distance, although the active space of signals is likely to be reduced. Brown-headed cowbirds, on the other hand, are brood parasites and males typically vocalize at a short distance from females. Even so, the high critical ratios and low frequency spectral content of brown-headed cowbirds may lead to significant masking of some components of song, even in low level noise. Both species typically pair vocalizations with a visual display, which may compensate for or enhance the detection of vocal components in noise (Peek, 1972; Cooper and Goller, 2004; Rı́os-Chelén et al., 2015; Magdaleno et al., 2022).
Red-winged blackbirds typically nest in freshwater marshes and prairies, which can be subject to low frequency masking by wind noise, although they are adaptable and can also be found nesting near roadside ditches and in urban parks (Yasukawa and Searcy, 2020). Song sparrows (Arcese et al., 2020) and swamp sparrows (Herbert and Mowbray, 2020) can be found in similar habitats to red-winged blackbirds, but have much lower critical ratios. Song sparrows have greater sensitivity and lower critical ratios than swamp sparrows at frequencies above 1 kHz and below 4 kHz and also have relatively greater spectral energy in their songs at these frequencies (Okanoya and Dooling, 1988). Both species have peak spectral energy in their songs at 4 kHz, with swamp sparrow broadcast song containing relatively greater spectral energy above 4 kHz. This suggests that song sparrow song is more likely to be masked by anthropogenic noise, which contains greater energy at lower frequencies. Moreover, low thresholds at these frequencies can enhance detection under quiet conditions, but may also result in masking of signals with lower amplitude noise. However, song sparrows also have lower critical ratios between 1 and 4 kHz, as well as more complex signals, which may enhance signal detection in noise. Song sparrows have also been shown to increase visual displays (Akçay and Beecher, 2019) and pitch shift songs in anthropogenic noise (Wood and Yezerinac, 2006), which could also enhance communication.
The SNR required for discrimination has been shown to be approximately 3dB greater than the SNR required for detection (Brenowitz, 1982; Lohr et al., 2003). Song sparrows have more complex songs and less redundancy compared to swamp sparrows, which may favor lower critical ratios. Additionally, song sparrow song is largely composed of tonal elements (i.e., spectral; Herbert and Mowbray, 2020), which may be better processed in narrower auditory filters (Henry and Lucas, 2010b) and therefore produce lower critical ratios. However, swamp sparrow song is trilled, with repeated frequency modulated elements (i.e temporal characteristics; Yasukawa and Searcy, 2020). These temporal qualities may be better processed in broader auditory filters (Henry et al., 2011), which in turn could result in elevated critical ratios. Further complicating the issue are the soft or warbled songs of the two species. The warbled or soft songs of song sparrows are more complex and have a greater spectral range than broadcast songs (Anderson et al., 2008). Swamp sparrow soft songs, on the other hand have less complexity and more repeated components (Ballentine et al., 2008). Here, again, the swamp sparrow songs are likely more detectable in noise than the song sparrow songs, given only the characteristics of the songs, but the lower thresholds and critical ratios of the song sparrow may compensate for these differences. Taken together, this suggests that there is significant complexity in determining whether species level differences in auditory processing in noise are likely to affect communication, due to significant differences in the spectral, temporal, and amplitude characteristics of vocalizations and background masking noise.
There are other contexts, however, in which auditory processing in noise may play an important role in determining whether some species are disproportionately affected by anthropogenic noise. For instance, red-winged blackbirds, song sparrows, and swamp sparrows are often found nesting in similar habitats and may be subject to similar nest predators (Arcese et al., 2020; Herbert and Mowbray, 2020; Yasukawa and Searcy, 2020). Acoustic detection of approaching nest or adult predators may be best achieved by species with the lowest critical ratio, favoring song sparrows over swamp sparrows in habitats with greater noise. Indeed, elevated levels of noise have been shown to increase vigilance and decrease foraging, presumably due to a diminished capacity to detect acoustic cues of approaching predators (Sweet et al., 2002). Differences in critical ratios might also be important in the detection of signals from heterospecifics, either through eavesdropping on anti-predator communication during the breeding season, or for interspecific communication in mixed species flocks. Differences in critical ratios may also be important for species that rely on acoustic information for prey detection. For instance, Northern saw-whet owls, short-eared owls (Asio flammeus) and long-eared owls (Asio otus, Senzaki et al., 2016) have impaired prey detection under even relatively low levels of anthropogenic noise, which may be due to elevated critical ratios in owls at frequencies corresponding to prey rustling sounds (Dyson et al., 1998).
The role of auditory processing in species level response to anthropogenic noise is still largely unclear. Although only one component of a complex set of interacting factors, there is reason to believe that species level differences may result in differential capacities of species to thrive in environments with anthropogenic noise. We recommend that future work expand the number of species for which we have information on auditory processing in noise, expand the scope of the stimuli and approaches taken in a comparative context, and explicitly consider the auditory processing abilities of species when investigating their behavioral responses to noise.
Author contributions
MG conceptualized the review. MF, TC, MG, and KH contributed to the writing, editing, and preparation of the manuscript. All authors contributed to the article and approved the submitted version.
Funding
Funding came from the Vassar College Biology Department Jipson Fund and the Vassar College Research Committee.
Conflict of interest
The authors declare that the research was conducted in the absence of any commercial or financial relationships that could be construed as a potential conflict of interest.
Publisher’s note
All claims expressed in this article are solely those of the authors and do not necessarily represent those of their affiliated organizations, or those of the publisher, the editors and the reviewers. Any product that may be evaluated in this article, or claim that may be made by its manufacturer, is not guaranteed or endorsed by the publisher.
Supplementary material
The Supplementary Material for this article can be found online at: https://www.frontiersin.org/articles/10.3389/fevo.2023.1233911/full#supplementary-material
References
Akçay Ç., Beecher M. D. (2019). Multi-modal communication: song sparrows increase signal redundancy in noise. Biol Lett. 15, 2019051. doi: 10.1098/rsbl.2019.0513
Anderson R. C., Searcy W. A., Peters S., Nowicki S. (2008). Soft song in song sparrows: acoustic structure and implications for signal function. Ethol 114, 662–676. doi: 10.1111/j.1439-0310.2008.01518.x
Arcese P., Sogge M. K., Marr A. B., Patten M. A. (2020). “Song sparrow (Melospiza melodia),” in Birds of the world. Eds. Poole A. F., Gill F. B. (Ithaca, NY: Cornell Lab of Ornithology). doi: 10.2173/bow.sonspa.01
Bacon S. P., Grantham D. W. (1989). Modulation masking: effects of modulation frequency, depth, and phase. J. Acoust. Soc Am. 85, 2575–2580. doi: 10.1121/1.397751
Ballentine B., Searcy W. A., Nowicki S. (2008). Reliable aggressive signalling in swamp sparrows. Anim. Behav. 75, 693–703. doi: 10.1016/j.anbehav.2007.07.025
Barber J. R., Crooks K. R., Fristrup K. M. (2010). The costs of chronic noise exposure for terrestrial organisms. Trends Ecol. Evol. 25, 180–189. doi: 10.1016/j.tree.2009.08.002
Barker N. K., Mennill D. J. (2009). Song perch height in rufous-and-white wrens: does behaviour enhance effective communication in a tropical forest? Ethol 115, 897–904. doi: 10.1111/j.1439-0310.2009.01674.x
Beatini J. R., Proudfoot G. A., Gall M. D. (2018). Frequency sensitivity in Northern saw whet owls (Aegolius acadicus). J. Comp. Physiol. A 204, 145–154. doi: 10.1007/s00359-017-1216-2
Bermúdez-Cuamatzin E., Ríos-Chelén A. A., Gil D., Garcia C. M. (2011). Experimental evidence for real-time song frequency shift in response to urban noise in a passerine bird. Biol. Lett. 7, 36–38. doi: 10.1098/rsbl.2010.0437
Brenowitz E. A. (1982). The active space of red-winged blackbird song. J. Comp. Physiol. 147, 511–522. doi: 10.1007/BF00612017
Brittan-Powell E., Dooling R. J., Gleich O. (2002). Auditory brainstem responses (ABR) in adult budgerigars (Melopsittacus undulatus). J. Acoust. Soc Am. 112, 999–1008. doi: 10.1121/1.1494807
Brittan-Powell E. F., Lohr B., Hahn D. C., Dooling R. J. (2005). Auditory brainstem responses in the eastern screech owl: an estimate of auditory thresholds. J. Acoust. Soc Am. 118, 314–321. doi: 10.1121/1.1928767
Brumm H. (2004). The impact of environmental noise on song amplitude in a territorial bird. J. Anim. Ecol. 73, 434–440. doi: 10.1111/j.0021-8790.2004.00814.x
Brumm H., Horn A. (2019). “Noise pollution and conservation,” in Encyclopedia of Animal Behavior. Ed. Choe J. C. (San Diego, CA: Elsevier), 254–259.
Brumm H., Naguib M. (2009). Environmental acoustics and the evolution of bird song. Adv. Study Behav. 40, 1–33. doi: 10.1016/S0065-3454(09)40001-9
Brumm H., Schmidt R., Schrader L. (2009). Noise-dependent vocal plasticity in domestic fowl. Anim. Behav. 78, 741–746. doi: 10.1016/j.anbehav.2009.07.004
Brumm H., Slabbekoorn H. (2005). “Acoustic communication in noise,” in Advances in the Study of Behavior. Eds. Slater P. J. B., Snowdon C. T., Roper T. J., Brockmann H. J., Naguib M. (Academic Press), 151–209. doi: 10.1016/S0065-3454(05)35004-2
Brumm H., Slater P. J. (2006). Ambient noise, motor fatigue, and serial redundancy in chaffinch song. Behav. Ecol. Sociobiol. 60, 475–481. doi: 10.1007/s00265-006-0188-y
Brumm H., Todt D. (2002). Noise-dependent song amplitude regulation in a territorial songbird. Anim. Behav. 63, 891–897. doi: 10.1006/anbe.2001.1968
Brumm H., Zollinger S. A. (2011). The evolution of the Lombard effect: 100 years of psychoacoustic research. Behaviour 148 (11-13), 1173–1198. doi: 10.1163/000579511X605759
Chan A. A. Y. H., Giraldo-Perez P., Smith S., Blumstein D. T. (2010). Anthropogenic noise affects risk assessment and attention: the distracted prey hypothesis. Biol. Lett. 6, 458–461. doi: 10.1098/rsbl.2009.1081
Cooper B. G., Goller F. (2004). Multimodal signals: enhancement and constraint of song motor patterns by visual display. Sci 303, 544–546. doi: 10.1126/science.1091099
Costalupes J. A. (1985). Representation of tones in noise in the responses of auditory nerve fibers in cats. I. Comparison with detection thresholds. J. Neurosci. 5, 3261–3269. doi: 10.1523/JNEUROSCI.05-12-03261.1985
Crowell S. E., Wells-Berlin A. M., Carr C. E., Olsen G. H., Therrien R. E., Yannuzzi S. E., et al. (2015). A comparison of auditory brainstem responses across diving bird species. J. Comp. Physiol. A 201, 803–815. doi: 10.1007/s00359-015-1024-5
Crowell S. E., Wells-Berlin A. M., Therrien R. E., Yannuzzi S. E., Carr C. E. (2016). In-air hearing of a diving duck: A comparison of psychoacoustic and auditory brainstem response thresholds. J. Acoust. Soc Am. 139, 3001–3008. doi: 10.1121/1.4948574
Dabelsteen T., Larsen O. N., Pedersen S. B. (1993). Habitat-induced degradation of sound signals: Quantifying the effects of communication sounds and bird location on blur ratio, excess attenuation, and signal-to-noise ratio in blackbird song. J. Acoust. Soc Am. 93, 2206–2220. doi: 10.1121/1.406682
Dau T., Kollmeier B., Kohlrausch A. (1997a). Modeling auditory processing of amplitude modulation. I. Detection and masking with narrow-band carriers. J. Acoust. Soc Am. 102, 2892–2905. doi: 10.1121/1.420345
Dau T., Kollmeier B., Kohlrausch A. (1997b). Modeling auditory processing of amplitude modulation. II. Spectral and temporal integration. J. Acoust. Soc Am. 102, 2906–2919. doi: 10.1121/1.420345
Dent M. L., Larsen O. N., Dooling R. J. (1997). Free-field binaural unmasking in budgerigars (Melopsittacus undulatus). Behav. Neurosci. 111, 590–598. doi: 10.1037/0735-7044.111.3.590
Dent M. L., McClaine E. M., Best V., Ozmeral E., Narayan R., Gallun F. J., et al. (2009). Spatial unmasking of birdsong in zebra finches (Taeniopygia guttata) and budgerigars (Melopsittacus undulatus). J. Comp. Psychol. 123, 357–367. doi: 10.1037/a0016898
Dominoni D. M., Greif S., Nemeth E., Brumm H. (2016). Airport noise predicts song timing of European birds. Ecol. Evol. 6, 6151–6159. doi: 10.1002/ece3.2357
Dooling R. J., Blumenrath S. H. (2016). Masking experiments in humans and birds using anthropogenic noises. Adv. Exp. Biol. Med. 875, 239–243. doi: 10.1007/978-1-4939-2981-8_28
Dooling R. J., Leek M. R. (2018). “Communication masking by man-made noise,” in Effects of Anthropogenic Noise on Animals. Eds. Slabbekoorn H., Dooling R. J., Popper A. N., Fay R. R. (New York, NY: Springer-Verlag), 23–46.
Dooling R. J., Lohr B., Dent M. L. (2000). “Hearing in birds and reptiles,” in Comparative Hearing: Birds and Reptiles. Eds. Dooling R. J., Fay R. R., Popper A. N. (New York: Springer-Verlag), 308–359.
Dooling R. J., Saunders J. C. (1975). Hearing in the parakeet (Melopsittacus undulatus): absolute thresholds, critical ratios, frequency difference limens, and vocalizations. J. Comp. Physiol. Psychol. 88, 1–20. doi: 10.1037/h0076226
Dorado-Correa A. M., Rodríguez-Rocha M., Brumm H. (2016). Anthropogenic noise, but not artificial light levels predicts song behaviour in an equatorial bird. R. Soc Op. Sci. 3, 160231. doi: 10.1098/rsos.160231
Dowling J. L., Luther D. A., Marra P. P. (2012). Comparative effects of urban development and anthropogenic noise on bird songs. Behav. Ecol. 23, 201–209. doi: 10.1093/beheco/arr176
Dyson M. L., Klump G. M., Gauger B. (1998). Absolute hearing thresholds and critical masking ratios in the European barn owl: a comparison with other owls. J. Comp. Physiol. A 182, 695–702. doi: 10.1007/s003590050214
Ewert S. D., Verhey J. L., Dau T. (2002). Spectro-temporal processing in the envelope- frequency domain. J. Acoust. Soc Am. 112, 2921–2931. doi: 10.1121/1.1515735
Fischer F. P. (1994). General pattern and morphological specializations of the avian cochlea. Scanning Microsc. 8, 351–364.
Francis C. D., Ortega C. P., Cruz A. (2011). Different behavioural responses to anthropogenic noise by two closely related passerine birds. Biol. Lett. 7, 850–852. doi: 10.1098/rsbl.2011.0359
Frost B. J., Baldwin P. J., Csizy M. (1989). Auditory localization in the northern saw-whet owl, Aegolius acadicus. Can. J. Zool. 67, 1955–1959. doi: 10.1139/z89-279
Fuller R. A., Warren P. H., Gaston K. J. (2007). Daytime noise predicts nocturnal singing in urban robins. Biol. Lett. 3, 368–370. doi: 10.1098/rsbl.2007.0134
Gall M. D., Brierley L. B., Lucas J. R. (2011). Species and sex effects on auditory processing in brown-headed cowbirds and red-winged blackbirds. Anim. Behav. 81, 973–982. doi: 10.1016/j.anbehav.2011.01.032
Gall M. D., Lucas J. R. (2010). Sex differences in auditory filters of brown-headed cowbirds (Molothrus ater). J. Comp. Physiol. A 196, 559–567. doi: 10.1007/s00359-010-0543-3
Gall M. D., Salameh T. S., Lucas J. R. (2013). Songbird frequency selectivity and temporal resolution vary with sex and season. Proc. R. Soc B 280, 20122296. doi: 10.1098/rspb.2012.2296
Glasberg B. R., Moore B. C. (1990). Derivation of auditory filter shapes from notched-noise data. Hear. Res. 47, 103–138. doi: 10.1016/0378-5955(90)90170-t
Gleich O., Klump G. M. (1995). Temporal modulation transfer functions in the European starling (Sturnus vulgaris): II. Responses of auditory-nerve fibers. Hear. Res. 82, 81–92. doi: 10.1016/0378-5955(94)00168-P
Gleich O., Langemann U. (2011). Auditory capabilities of birds in relation to the structural diversity of the basilar papilla. Hear. Res. 273, 80–88. doi: 10.1016/j.heares.2010.01.009
Gleich O., Manley G. (2000). “The hearing organ of birds and crocodilia,” in Comparative Hearing: Birds and Reptiles. Eds. Dooling R. J., Fay R. R., Popper A. N. (New York, NY: Springer-Verlag), 71–138.
Gleich O., Narins P. M. (1988). The phase response of primary auditory afferents in a songbird (Sturnus vulgaris L.). Hear. Res. 32, 81–91. doi: 10.1016/03785955(88)90148-7
Goller B., Baumhardt P., Dominguez-Villegas E., Katzner T., Fernández-Juricic E., Lucas J. R. (2022). Selecting auditory alerting stimuli for eagles on the basis of auditory evoked potentials. Conserv. Physiol. 10, coac059. doi: 10.1093/conphys/coac059
Halfwerk W., Lohr B., Slabbekoorn H. (2018). “Impact of man-made sound on birds and their songs,” in Effects of Anthropogenic Noise on Animals. Eds. Slabbekoorn H., Dooling R. J., Popper A. N., Fay R. R. (New York, NY: Springer-Verlag), 209–241.
Halfwerk W., van Oers K. (2020). Anthropogenic noise impairs foraging for cryptic prey via cross-sensory interference. Proc. R. Soc B 287, 20192951. doi: 10.1098/rspb.2019.2951
Hashino E., Sokabe M., Miyamoto K. (1988). Frequency specific susceptibility to acoustic trauma in the budgerigar (Melopsittacus undulatus). J. Acoust. Soc Am. 83, 2450–2453. doi: 10.1121/1.396325
Henry K. S., Abrams K. S. (2021). Normal tone-in-noise sensitivity in trained budgerigars despite substantial auditory-nerve injury: No evidence of hidden hearing loss. J. Neurosci. 41, 118–129. doi: 10.1523/JNEUROSCI.2104-20.2020
Henry K. S., Amburgey K. N., Abrams K. S., Carney L. H. (2020). Identifying cues for tone-in- noise detection using decision variable correlation in the budgerigar (Melopsittacus undulatus). J. Acoust. Soc Am. 147, 984–997. doi: 10.1121/10.0000621
Henry K. S., Gall M. D., Bidelman, Lucas J. R. (2011). Songbirds tradeoff auditory frequency resolution and temporal resolution. J. Comp. Physiol. A 197, 351–359. doi: 10.1007/s00359-010-0619-0
Henry K. S., Gall M. D., Velez A., Lucas J. R. (2017). “Avian auditory processing at four different scales: variation among species, seasons, sexes and individuals,” in Psychological Mechanisms in Animal Communication. Eds. Bee M. A., Miller C. T. (New York NY: Springer-Verlag), 17–55.
Henry K. S., Heinz M. G. (2012). Diminished temporal coding with sensorineural hearing loss emerges in background noise. Nat. Neurosci. 15, 1362–1364. doi: 10.1038/nn.3216
Henry K. S., Lucas J. R. (2010a). Auditory sensitivity and the frequency selectivity of auditory filters in the Carolina chickadee, Poecile carolinensis. Anim. Behav. 80, 497–507. doi: 10.1016/j.anbehav.2010.06.012
Henry K. S., Lucas J. R. (2010b). Habitat-related differences in the frequency selectivity of auditory filters in songbirds. Funct. Ecol. 24, 614–624. doi: 10.1111/j.1365-2435.2009.01674.x
Henry K. S., Neilans E. G., Abrams K. S., Idrobo F., Carney L. H. (2016). Neural correlates of behavioral amplitude modulation sensitivity in the budgerigar midbrain. J. Neurophysiol. 115, 1905–1916. doi: 10.1152/jn.01003.2015
Herbert J. A., Mowbray T. B. (2020). “Swamp Sparrow (Melospiza Georgiana),” in Birds of the World. Ed. Rodewald P. G. (Ithaca, NY: Cornell Lab of Ornithology). doi: 10.2173/bow.swaspa.01
Hienz R. D., Sachs M. B. (1987). Effects of noise on pure-tone thresholds in blackbirds (Agelaius phoeniceus and Molothrus ater) and pigeons (Columba livia). J. Comp. Psychol. 101, 16–24. doi: 10.1037/0735-7036.101.1.16
Hose B., Langner G., Scheich H. (1987). Brain Res Topographic representation of periodicities in the forebrain of the mynah bird: one map for pitch and rhythm? Brain. Res. 422, 367–373. doi: 10.1016/0006-8993(87)90946-2
Houtgast T. (1989). Frequency selectivity in amplitude-modulation detection. J. Acoust. Soc Am. 85, 1676–1680. doi: 10.1121/1.397956
Jensen K. K., Klokker S. (2006). Hearing sensitivity and critical ratios of hooded crows (Corvus corone cornix). J. Acoust. Soc Am. 119, 1269–1276. doi: 10.1121/1.2159431
Jung H., Sherrod A., LeBreux S., Price J. M., Freeberg T. M. (2020). Traffic noise and responses to a simulated approaching avian predator in mixed-species flocks of chickadees, titmice, and nuthatches. Ethol 126, 620–629. doi: 10.1111/eth.13013
Kleist N. J., Guralnick R. P., Cruz A., Lowry C. A., Francis C. D. (2018). Chronic anthropogenic noise disrupts glucocorticoid signaling and has multiple effects on fitness in an avian community. Proc. Nat. Acad. Sci. 115, E648–E657. doi: 10.1073/pnas.1709200115
Klump G. (1996). “Bird communication in the noisy world,” in Ecology and Evolution of Acoustic Communication in Birds. Eds. Kroodsma D. E., Miller. E. H. (Ithaca, NY: Cornell University Press), 321–338.
Klump G. M., Langemann U. (1995). Comodulation masking release in a songbird. Hear Res. 87, 157–164. doi: 10.1016/0378-5955(95)00087-k
Knudsen E. I., Konishi M. (1979). Mechanisms of sound localization in the barn owl (Tyto alba). J. Comp. Physiol. A. 133, 13–21. doi: 10.1007/BF00663106
Kohlrausch A., Fassel R., van der Heijden M., Kortekaas R., Van De Par S., Oxenham A. J., et al. (1997). Detection of tones in low-noise noise: further evidence for the role of envelope fluctuations. Acustica 83, 659–669.
Konishi M. (1973). How the owl tracks its prey: experiments with trained barn owls reveal how their acute sense of hearing enables them to catch prey in the dark. Am. Sci. 61, 414–424.
Köppl C. (1997). Phase locking to high frequencies in the auditory nerve and cochlear nucleus magnocellularis of the barn owl, Tyto alba. J. Neurosci 17, 3312–3321. doi: 10.1523/JNEUROSCI.17-09-03312.1997
Langemann U., Gauger B., Klump G. M. (1998). Auditory sensitivity in the great tit: perception of signals in the presence and absence of noise. Anim. Behav. 56, 763–769. doi: 10.1006/anbe.1998.0879
Langemann U., Klump G. M. (2001). Signal detection in amplitude-modulated maskers. I. Behavioural auditory thresholds in a songbird. Eur. J. Neurosci. 13, 1025–1032. doi: 10.1046/j.0953-816x.2001.01464.x
Langemann U., Klump G. M., Dooling R. J. (1995). Critical bands and critical-ratio bandwidth in the European starling. Hear. Res. 84, 167–176. doi: 10.1016/0378-5955(95)00023-W
Larsen O. N., Wahlbreg M., Christensen-Dalsgaard J. (2020). Amphibious hearing in a diving bird, the great cormorant (Phalacrocorax carbo sinensis). J. Exp. Biol. 223, 1–12. doi: 10.1242/jeb.217265
LaZerte S. E., Slabbekoorn H., Otter K. A. (2016). Learning to cope: vocal adjustment to urban noise is correlated with prior experience in black-capped chickadees. Proc. Roy Soc. B 283, 20161058. doi: 10.1098/rspb.2016.1058
Leong U. C., Schwarz D. M., Henry K. S., Carney L. H. (2020). Sensorineural hearing loss diminishes use of temporal envelope cues: evidence from roving-level tone-in-noise detection. Ear Hear. 41, 1009–1019. doi: 10.1097/aud.0000000000000822
Lohr B., Brittan-Powell E. F., Dooling R. J. (2013). Auditory brainstem responses and auditory thresholds in woodpeckers. J. Acoust. Soc Am. 133, 337–342. doi: 10.1121/1.4770255
Lohr B., Wright T. F., Dooling R. J. (2003). Detection and discrimination of natural calls in masking noise by birds: estimating the active space signal. Anim. Behav. 65, 763–777. doi: 10.1006/anbe.2003.2093
Lowther P. E. (2020). “Brown-headed Cowbird (Molothrus ater),” in Birds of the World. Eds. Poole A. F., Gill F. B. (Ithaca, NY: Cornell Lab of Ornithology). doi: 10.2173/bow.bnhcow.01
Maes J. H. R., De Groot G. (2003). Effects of noise on the performance of rats in an operant discrimination task. Behav. Processes 61, 57–68. doi: 10.1016/S0376-6357(02)00163-8
Magdaleno F. R., O'Loghlen A. L., Rothstein S. I. (2022). An agonistic visual signal during birdsong: Bill wiping in multimodal song displays by the male brown-headed cowbird (Molothrus ater). Wilson J. Ornithol 134, 215–226. doi: 10.1676/21-00004
Manley G. A., Gleich O., Kaiser A., Brix J. (1989). Functional differentiation of sensory cells in the avian auditory periphery. J. Comp. Physiol. A 164, 289–296. doi: 10.1007/BF00612989
Manley G. A., Gleich O., Leppelsack H. J., Oeckinghaus H. (1985). Activity patterns of cochlear ganglion neurones in the starling. J. Comp. Physiol. A 157, 161–181. doi: 10.1007/BF01350025
Mao J., Koch K. J., Doherty K. A., Carney L. H. (2015). Cues for diotic and dichotic detection of a 500-hz tone in noise vary with hearing loss. J. Assoc. Res. Otolaryngol. 16, 507–521. doi: 10.1007/s10162-015-0518-8
Marean G. C., Burt J. M., Beecher M. D., Rubel E. W. (1998). Auditory perception following hair cell regeneration in European starling (Sturnus vulgaris): frequency and temporal resolution. J. Acoust. Soc Am. 103, 3567–3580. doi: 10.1121/1.423085
Mason J. T., McClure C. J., Barber J. R. (2016). Anthropogenic noise impairs owl hunting behavior. Biol. Conserv. 199, 29–32. doi: 10.1016/j.biocon.2016.04.009
Mathevon N., Aubin T., Dabelsteen T. (1996). Song degradation during propagation: importance of song post for the wren Troglodytes troglodytes. Ethol 102, 397–412. doi: 10.1111/j.1439-0310.1996.tb01135.x
Mathevon N., Dabelsteen T., Blumenrath S. H. (2005). Are high perches in the blackcap Sylvia atricapilla song or listening posts? A sound transmission study. J. Acoust. Soc Am. 117, 442–449. doi: 10.1121/1.1828805
McGee J., Nelson P. B., Ponder J. B., Marr J., Redig P., Walsh E. J. (2019). Auditory performance in bald eagles and red-tailed hawks: a comparative study of hearing in diurnal raptors. J. Comp. Physiol. A 205, 793–811. doi: 10.1007/s00359-019-01367-9
McGrew K. A., Crowell S. E., Fiely J. L., Berlin A. M., Olsen G. H., James J., et al. (2022). Underwater hearing in sea ducks with applications for reducing gillnet bycatch through acoustic deterrence. J. Exp. Biol. 225, jeb243953. doi: 10.1242/jeb.243953
Meillère A., Brischoux F., Angelier F. (2015). Impact of chronic noise exposure on antipredator behavior: an experiment in breeding house sparrows. Behav. Ecol. 26, 569–577. doi: 10.1093/beheco/aru232
Moiseff A. (1989). Bi-coordinate sound localization by the barn owl. J. Comp. Physiol. A 164, 637–644. doi: 10.1007/BF00614506
Morris-Drake A., Kern J. M., Radford A. N. (2016). Cross-modal impacts of anthropogenic noise on information use. Curr. Biol. 26, R911–R912. doi: 10.1016/j.cub.2016.08.064
Naguib M. (2013). Living in a noisy world: indirect effects of noise on animal communication. Behav 150, 1069–1084. doi: 10.1163/1568539X-00003058
Nedelec S. L., Radford A. N., Pearl L., Nedelec B., McCormick M. I., Meekan M. G., et al. (2017). Motorboat noise impacts parental behaviour and offspring survival in a reef fish. Proc. R. Soc B. 284, 20170143. doi: 10.1098/rspb.2017.0143
Nemeth E., Brumm H. (2010). Birds and anthropogenic noise: are urban songs adaptive? Am. Nat. 176, 465–475. doi: 10.1086/656275
Noirot I. C., Brittan-Powell E. F., Dooling R. J. (2011). Masked and unmasked auditory thresholds in three species of birds as measured by the auditory brainstem response. J. Acoust. Soc Am. 129, 3445–3448. doi: 10.1121/1.3578452
Norberg R.Å. (1977). Occurrence and independent evolution of bilateral ear asymmetry in owls and implications on owl taxonomy. Phil. Trans. R. Soc B. 280, 375–408.
Okanoya K., Dooling R. J. (1987). Hearing in passerine and psittacine birds: a comparative study of absolute and masked auditory thresholds. J. Comp. Psychol. 101, 7–15. doi: 10.1037/0735-7036.101.1.7
Okanoya K., Dooling R. J. (1988). Hearing in the swamp sparrow, Melospiza Georgiana, and the song sparrow, Melospiza melodia. Anim. Behav. 36, 726–732. doi: 10.1016/S0003-3472(88)80155-6
Osbrink A., Meatte M. A., Tran A., Herranen K. K., Meek L., Murakami-Smith M., et al. (2021). Traffic noise inhibits cognitive performance in a songbird. Proc. R. Soc B. 288, 20202851. doi: 10.1098/rspb.2020.2851
Payne R. S. (1971). Acoustic location of prey by barn owls (Tyto alba). J. Exp. Biol. 54, 535–573. doi: 10.1242/jeb.54.3.535
Peek F. W. (1972). An experimental study of the territorial function of vocal and visual display in the male red-winged blackbird (Agelaius phoeniceus). Anim. Behav. 20, 112–118. doi: 10.1016/S0003-3472(72)80180-5
Pohl N. U., Leadbeater E., Slabbekoorn H., Klump G. M., Langemann U. (2012). Great tits in urban noise benefit from high frequencies song detection and discrimination. Anim. Behav. 83, 711–721. doi: 10.1016/j.anbehav.2011.12.019
Pohl N. U., Slabbekoorn H., Klump G. M., Langemann U. (2009). Effects of signal features and environmental noise on signal detection in the great tit, Parus major. Anim. Behav. 78, 1293–1300. doi: 10.1016/j.anbehav.2009.09.005
Pohl N. U., Slabbekoorn H., Neubauer H., Klump G. M., Langemann U. (2013). Why longer song elements are easier to detect: threshold level-duration functions in the great tit and comparison with human data. J. Comp. Physiol. A. 199, 239–252. doi: 10.1007/s00359-012-0789-z
Polak M. (2014). Relationship between traffic noise levels and song perch height in a common passerine bird. Trans. Res. D 30, 72–75. doi: 10.1016/j.trd.2014.05.004
Pytte C. L., Ficken M. S., Moiseff A. (2004). Ultrasonic singing by the blue-throated hummingbird: a comparison between production and perception. J. Comp. Physiol. A 190, 665–673. doi: 10.1007/s00359-004-0525-4
Richards V. M. (1992). The detectability of a tone added to narrow bands of equal-energy noise. J. Acoust. Soc Am. 91, 3424–3435. doi: 10.1121/1.402831
Ríos-Chelén A. A., Lee G. C., Patricelli G. L. (2015). Anthropogenic noise is associated with changes in acoustic but not visual signals in red-winged blackbirds. Behav. Ecol. Sociobiol. 69, 1139–1151. doi: 10.1007/s00265-015-1928-7
Roca I. T., Desrochers L., Giacomazzo M., Bertolo A., Bolduc P., Deschesnes R., et al. (2016). Shifting song frequencies in response to anthropogenic noise: a meta-analysis on birds and anurans. Behav. Ecol. 27, 1269–1274. doi: 10.1093/beheco/arw060
Sachs M. B., Young E. D., Lewis R. H. (1974). Discharge patterns of single fibers in the pigeon auditory nerve. Brain. Res. 70, 431–447. doi: 10.1016/0006-8993(74)90253-4
Salvi R. J., Saunders S. S., Powers N. L., Boettcher F. A. (1992). Discharge patterns of cochlear ganglion neurons in the chicken. J. Comp. Physiol. A 170, 227–241. doi: 10.1007/BF00196905
Saunders J. C., Rintelmann W. F., Bock G. R. (1979). Frequency selectivity in bird and man: a comparison among critical ratios, critical bands, and psychophysical tuning curves. Hear. Res. 1, 303–323. doi: 10.1016/0378-5955(79)90003-0
Seger-Fullam K. D., Rodewald A. D., Soha J. A. (2011). Urban noise predicts song frequency in northern cardinals and american robins. Bioacoust 20, 267–276. doi: 10.1080/09524622.2011.9753650
Senzaki M., Yamaura Y., Francis C. D., Nakamura F. (2016). Traffic noise reduces foraging efficiency in wild owls. Sci. Rep. 6, 1–7. doi: 10.1038/srep30602
Simpson S. D., Radford A. N., Nedelec S. L., Ferrari M. C., Chivers D. P., McCormick M. I., et al. (2016). Anthropogenic noise increases fish mortality by predation. Nat. Commun. 7, 1–7. doi: 10.1038/ncomms10544
Slabbekoorn H., den Boer-Visser A. (2006). Cities change the songs of birds. Curr. Biol. 16, 2326–2331. doi: 10.1016/j.cub.2006.10.008
Slabbekoorn H., Dooling R. J., Popper A. N. (2018). “Man-made sounds and animals,” in Effects of Anthropogenic Noise on Animals. Eds. Slabbekoorn H., Dooling R. J., Popper A. N., Fay R. R. (New York, NY: Springer-Verlag), 23–46.
Slabbekoorn H., Peet M. (2003). Birds sing at a higher pitch in urban noise. Nat 424, 267–267. doi: 10.1038/424267a
Sprau P., Roth T., Naguib M., Amrhein V. (2012). Communication in the third dimension: song perch height of rivals affects singing response in nightingales. PloS One 7, e32194. doi: 10.1371/journal.pone.0032194
Stansfeld S. A., Matheson M. P. (2003). Noise pollution: non-auditory effects on health. Brit. Med. Bull. 68, 243–257. doi: 10.1093/bmb/ldg033
Sweet K. A., Sweet B. P., Gomes D. G. E., Francis C. D., Barber J. R. (2002). Natural and anthropogenic noise increase vigilance and decrease foraging behaviors in song sparrows. Behav. Ecol. 33, 288–297. doi: 10.1093/beheco/arab141
Takasaka T., Smith C. A. (1971). The structure and innervation of the pigeon’s basilar papilla. J. Ultrastruct. Res. 35, 20–65. doi: 10.1016/S0022-5320(71)80141-7
Thiele N., Köppl C. (2018). Gas anesthesia impairs peripheral auditory sensitivity in barn owls (Tyto alba). Eneuro 5, eneuro.0140–18. doi: 10.1523/ENEURO.0140-18.2018
Velez A., Gall M. D., Fu J., Lucas J. R. (2015). Song structure, not high-frequency song content, determines high-frequency auditory sensitivity in nine species of New World sparrows (Passeriformes: Emberizidae). Funct. Ecol. 29, 487–497. doi: 10.1111/1365-2435.12352
Wang Y., Abrams K. S., Carney L. H., Henry K. S. (2021). Midbrain-level neural correlates of behavioral tone-in-noise detection: dependence on energy and envelope cues. J. Neurosci. 41, 7206–7223. doi: 10.1523/jneurosci.3103-20.2021
Wiley H. (2017). Noise matters: the evolution of communication (Boston, MA: Harvard University Press).
Wood W. E., Yezerinac S. M. (2006). Song sparrow (melospiza melodia) song varies with urban noise. . Auk 123, 650–659. doi: 10.1642/0004-8038(2006)123[650:SSMMSV]2.0.CO;2
Woolley S. M. N., Casseday J. H. (2005). Processing of modulated sounds in the zebra finch auditory midbrain: responses to noise, frequency sweeps, and sinusoidal amplitude modulations. J. Neurophysiol. 94, 1143–1157. doi: 10.1152/jn.01064.2004
Wright T. F., Cortopassi K. A., Bradbury J. W., Dooling R. J. (2003). Hearing and vocalizations in the orange-fronted conure (Aratinga canicularis). J. Comp. Psychol. 117, 87–95. doi: 10.1037/0735-7036.117.1.87
Yang X. J., Slabbekoorn H. (2014). Timing vocal behavior: lack of temporal overlap avoidance to fluctuating noise levels in singing Eurasian wrens. Behav. Processes. 108, 131–137. doi: 10.1016/j.beproc.2014.10.002
Yasukawa K., Searcy W. A. (2020). “Red-winged Blackbird (Agelaius phoeniceus),” in Birds of the World. Ed. Rodewald P. G. (Ithaca, NY: Cornell Lab of Ornithology). doi: 10.2173/bow.rewbla.01
Zhou Y., Radford A. N., Magrath R. D. (2019). Why does noise reduce response to alarm calls? Experimental assessment of masking, distraction and greater vigilance in wild birds. Funct. Ecol. 33, 1280–1289. doi: 10.1111/1365-2435.13333
Zollinger S. A., Dorado-Correa A., Goymann W., Forstmeier W., Knief U., BastidasUrrutia A. M., et al. (2019). Traffic noise exposure depresses plasma corticosterone and delays offspring growth in breeding zebra finches. Conserv. Physiol. 7, coz056. doi: 10.1093/conphys/coz056
Keywords: critical ratios, masked thresholds, birds, traffic noise, hearing
Citation: Fossesca M, Henry KS, Chou TL and Gall MD (2023) The silent assumption of the masking hypothesis: avian auditory processing and implications for behavioral responses to anthropogenic noise. Front. Ecol. Evol. 11:1233911. doi: 10.3389/fevo.2023.1233911
Received: 05 June 2023; Accepted: 25 August 2023;
Published: 13 September 2023.
Edited by:
Eve Schneider, University of Kentucky, United StatesReviewed by:
Georg Klump, University of Oldenburg, GermanyFelipe N Moreno-Gómez, Universidad Católica del Maule, Chile
Copyright © 2023 Fossesca, Henry, Chou and Gall. This is an open-access article distributed under the terms of the Creative Commons Attribution License (CC BY). The use, distribution or reproduction in other forums is permitted, provided the original author(s) and the copyright owner(s) are credited and that the original publication in this journal is cited, in accordance with accepted academic practice. No use, distribution or reproduction is permitted which does not comply with these terms.
*Correspondence: Megan D. Gall, bWVnYWxsQHZhc3Nhci5lZHU=