- 1Institute for Evolution & Biodiversity, University of Münster, Münster, Germany
- 2Laboratory of Genetics, Wageningen University & Research, Wageningen, Netherlands
Ecotypes, subpopulations or strains of a single species locally adapted to divergent ecological conditions within the same habitat are often considered to be the first steps in sympatric speciation. It has been suggested that two ecotypes are distinguishable in Nasonia vitripennis, a prominent model organism for parasitic Hymenoptera, with one ecotype parasitizing fly pupae in bird nests, and the other one parasitizing fly pupae on carrion. This differentiation into two ecotypes has been hypothesized to indicate incipient sympatric speciation in populations of this globally distributed species. In the present study, we investigated the differentiation into these two distinct ecotypes focusing on chemical profiles and the population genetic divergence in a wild N. vitripennis population from the Netherlands. Isofemale lines were obtained from bird nest boxes and from deer carrion, respectively, representing both microhabitats. To test for phenotypic differentiation, we determined the surface cuticular hydrocarbon (CHC) profiles from wasps of both host patches. Using a panel of 14 microsatellites, we concordantly determined the population genetic structure and tested for genetic differentiation between foundresses obtained from both microhabitats. Both the phenotypic as well as the genetic datasets show no evidence for any kind of separation based on the postulated two ecotypes, but rather suggest free interbreeding with no gene flow interruption between the two distinct host patches. Our findings challenge previous assumptions on clearly distinguishable ecotypes in N. vitripennis, and demonstrate how a chemical ecological assessment coupled with population genetics can be instrumental in re-evaluating the potential of ecological differentiation and incipient speciation mechanisms in parasitoid wasps.
1 Introduction
Niche differentiation can occur when co-existing populations split through local adaptation to divergent conditions based on biotic or abiotic factors (Hutchinson, 1957; Holt, 2009). Consequently, a population can become separated through adaptation to different microhabitats, e.g., by divergent dietary specializations (e.g. Bakovic et al., 2019), or through phenological separation (e.g. Lin and Hyyppä, 2019). The formation of such barriers to gene flow has the potential to gradually increase reproductive isolation of emerging subpopulations or ecotypes (Coyne and Orr, 2004). Ecotypes are defined as subpopulations for which the main barriers to gene flow are ecological (Turesson, 1922; Stronen et al., 2022). Correlated divergences in host or mate preferences can further increase pre-existing niche differentiation (Holt, 2009), which then can be reinforced by genetic incompatibilities (Butlin, 1987; Higgie et al., 2000). Persistent reproductive isolation is required for the maintenance of ecotypes (Yannic et al., 2018; Stronen et al., 2022). Eventually, the long-term coexistence of increasingly diverging ecotypes may lead to sympatric speciation (Coyne and Orr, 2004; Ortiz-Barrientos et al., 2009).
While differentiation between populations or strains from different microhabitats may be due to phenotypic plasticity (Yeh and Price, 2004; Pigliucci, 2005), genetic divergence between such strains constitutes potential evidence of reduced gene flow (Coyne and Orr, 2004; Sunamura et al., 2011). Ecotypes can coexist without clearly separated phenotypes (Menz et al., 2015; Taylor et al., 2020), but they cannot be maintained without some degree of reproductive isolation (Holt, 2009; Stronen et al., 2022). Unambiguous cases of ecotypes show evidence of phenotypic differentiation that correlates with genetic differentiation (e.g. Menz et al., 2015; Le Moan et al., 2016; Yannic et al., 2018).
However, empirical evidence for ecotypes is generally biased towards plants, fish and mammals, while other taxa, particularly invertebrates, remain largely understudied in this respect (Stronen et al., 2022). In light of the continuous and prominent decline in insect biodiversity, undermining their tremendous contributions to virtually all ecosystems on earth, this massive oversight appears particularly puzzling (Scudder, 2017; Crespo-Pérez et al., 2020; Raven and Wagner, 2021). Therefore, eligible insect model organisms where the discrimination between populations, ecotypes, subspecies and “true species” can be readily assessed are necessary to properly direct countermeasures against insect biodiversity decline (Bakovic et al., 2019; Stronen et al., 2022).
The parasitoid jewel wasp genus Nasonia (Hymenoptera: Pteromalidae) has been well-established as an insect model system to study ecological and evolutionary aspects of adaptation (e.g. Koppik et al., 2014; Shuker, 2023), reproductive isolation (e.g. Giesbers et al., 2013; Bell and Bordenstein, 2022) and evolutionary genetics (e.g. van de Zande et al., 2014; Pannebakker et al., 2020). Its most well-known and cosmopolitan species, Nasonia vitripennis, has been proposed to be differentiated into two ecotypes distinguishable by their respective microhabitats (Schröder, 2000; Malec et al., 2021; Figure 1). Females of this species parasitize a wide array of different fly pupae, most commonly from the genera Sarcophaga (flesh flies), Calliphora (blowflies) and Protocalliphora (bird blowflies). Depending on the microhabitat where these host genera mainly occur, the suggested N. vitripennis ecotypes are the “nest ecotype”, where the wasps primarily parasitize Protocalliphora fly pupae on nestlings in bird nest boxes (Darling and Werren, 1990; Peters and Abraham, 2010), and the “carrion ecotype”, where Calliphora and Sarcophaga fly pupae on vertebrate cadavers are mainly parasitized (Grassberger and Frank, 2004; Figure 1).
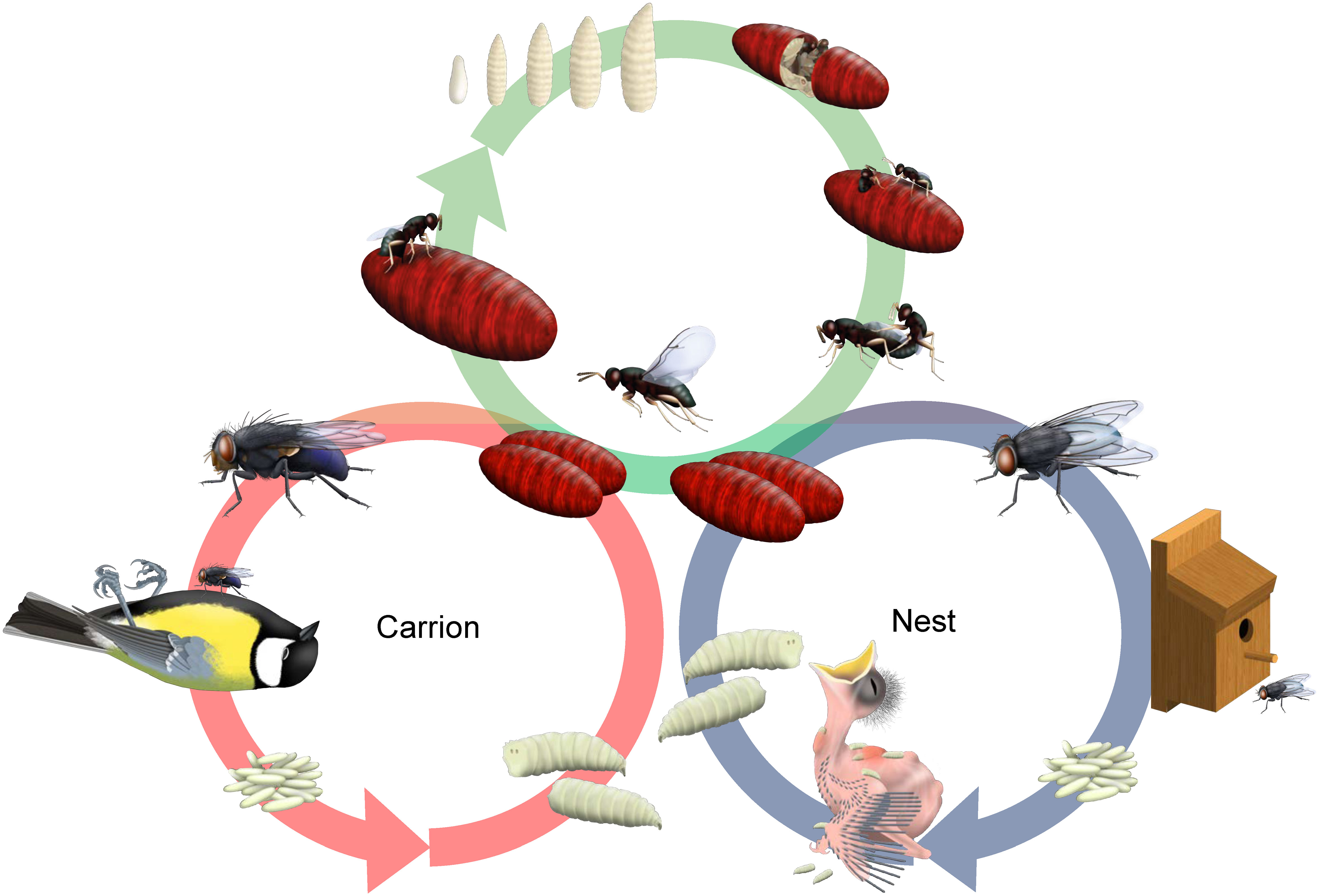
Figure 1 Schematic overview of the life cycle of Nasonia vitripennis. Female wasps locate fly pupae, which can occur in two microhabitats: bird nests or carrion. After localization of the hosts, female wasps inject venom into the host pupa and will oviposit typically 20–50 eggs. Larvae hatch from the eggs, and will normally pupate after 3 larval instars (unless an alternative diapause stage is entered under adverse conditions). Adults eclose from pupation within the host pupae. Males chew an exit hole into the host puparium wall and emerge first and will mate with females upon emergence from the host. Figure drawn by Marc Maas.
Ecotype formation in N. vitripennis may be reinforced by reduced gene flow due to high levels of natural inbreeding: males are flightless and thus mostly confined to their place of emergence from the host, where they compete for access to mate with the later emerging females (van den Assem et al., 1980). Due to their gregarious lifestyle, matings then generally occur between siblings of the same patch and are thus characterized by frequent local inbreeding (Grillenberger et al., 2008). However, females can disperse after being mated for distances of up to 2 km and locate new host patches (Grillenberger et al., 2009).
A recent study demonstrated very early reproductive barriers between the nest and carrion ecotypes in a sympatric N. vitripennis population from Southern Germany, hinting at premating and sexual isolation preceding ecological separation (Malec et al., 2021). Here, we focus on a sympatric N. vitripennis population from the Netherlands and test for potential degrees of separation between the different ecotypes on a phenotypic and population genetic level. We therefore assess the divergence of cuticular hydrocarbon (CHC) surface profiles as a phenotypic indicator for an incipient differentiation concordant with the studied microhabitats. CHC profiles have repeatedly been demonstrated to diverge rapidly in populations according to different ecological conditions (Menzel et al., 2017; Menzel et al., 2018; Hartke et al., 2019). Furthermore, CHC profiles have the potential to be utilized as chemotaxonomic traits to successfully discriminate otherwise indistinguishable sympatric populations of a single species (Takahashi et al., 2001; Everaerts et al., 2008) or recently diverged sister species (Finck et al., 2016; Sprenger et al., 2021). Notably, CHC profiles were the most apparent phenotypic traits clearly distinguishing the two most recently diverged species of the Nasonia genus, N. giraulti and N. oneida (Raychoudhury et al., 2010; Werren et al., 2010). In addition, female CHC profiles contain sexual cues for the males in most Nasonia species, triggering courtship and copulation behavior (Steiner et al., 2006; Buellesbach et al., 2013; Mair et al., 2017; Sun et al., 2023).
Furthermore, we screened the population genetic structure of representative foundresses from the two distinct host microhabitats with established Nasonia microsatellite markers for any degree of genetic differentiation. Interestingly, we could not confirm any separation between the ecotypes, neither on the phenotypic nor on the genetic level, hinting at unhindered gene flow between wasps from the carrion and nest microhabitats and strongly suggesting a re-evaluation of the potential of N. vitripennis to form truly separated ecotypes.
2 Materials and methods
2.1 Wasp collection and establishment of experimental strains
Wasps were collected from nest boxes and carrion in a 1.4 × 2.5 km field site in the Hoge Veluwe National Park, the Netherlands, in June 2018. Wasps originating from nest boxes were sampled by collecting the fly host pupae from 45 nest boxes after fledging of the birds (Parus major L.). The collected host pupae were incubated individually at room temperature, and the emerging wasps were identified as N. vitripennis based on morphology.
Wasps originating from carrion were sampled by laying out four deer legs (Cervus elaphus L.), baited with mesh bags containing fly pupae (Calliphora spp.) in individual containers on a layer of vermiculite. To prevent interference with non-insect carrion feeders, the containers were placed in a 1 m3 wire cage with a wide mesh, suspended at 1 m above the ground (Supplementary Figure S1). Two cages, each with two deer legs, were placed at two locations in the field site. In those, mesh bags with fly pupae were replaced every 6 days upon which the carrion was visually inspected for the presence of adult wasps. When adult wasps were present on the carrion (we did not observe alive adults in the nest boxes), individual females were isolated directly and were allowed to parasitize Calliphora spp. host pupae as foundresses of separate isofemale lines. From the bird nest boxes, fly host pupae were collected and isolated individually in glass vials (11 × 63 mm). Upon emergence, a single female from each host was isolated and allowed to parasitize Calliphora spp. host pupae for establishing an isofemale line similar to the carrion-derived females. To keep relatedness among isofemale lines low, only one single isofemale line was set up per nest box. After parasitization, individual foundresses of the isofemale lines from both carrions and the nest boxes were stored at −80°C for molecular analysis.
2.2 Chemical analysis and CHC profiling
After approximately 31 laboratory generations, we randomly selected males from five and females from four isofemales lines derived from carrion, as well as males from three and females from four isofemale lines derived from nest boxes, respectively. For each sex from each isofemale line, five respective individual wasps were each extracted in 30 µl of MS pure hexane (UniSolv, Darmstadt, Germany) in a GC-vial (Agilent, Santa Clara, California, USA) while being swirled on an orbital shaker (IKA KS 130 Basic, Staufen, Germany) for 10 minutes. Extracts were then transferred to a 250 µl conical insert (Agilent, Santa Clara, California, USA) and evaporated under a constant flow of CO2. The dried extracts were resuspended with 5 µl of an MS pure hexane solution containing 7.5 ng/μl of n-dodecane (EMD Millipore Corp., Billerica, Massachusetts, USA) as an internal standard. Three μl of the extract were injected into a GC-QQQ Triple Quad (GC: 7890B, Triple Quad: 7010B, Agilent, Waldbronn, Germany) with a PAL Autosampler system operating in electron impact ionization mode with 70 eV. The split/splitless injector was operated at 300°C in Pulsed splitless mode at 10.42 psi until 2 min with the Purge Flow to Split Vent set at 50 ml/min at 2 min. Separation of compounds was performed on a 30 m × 0.25 mm ID × 0.25 μm HP-1 Dimethylpolysiloxane column (Agilent J&W GC columns, Santa Clara, California, USA). The temperature program started from 60°C, held for 1 min, and increasing by 40°C per min to 200°C, followed by an increase of 5°C per min to 320°C, where it was held for 5 min. Helium served as carrier gas with a constant flow of 1.2 ml per min and a pressure of 10.42 psi. CHC peak detection, identification and quantification were performed using Quantitative Analysis MassHunter Workstation Software (Version B.09.00/Build 9.0.647.0, Agilent Technologies, Santa Clara, California, USA). Peaks were identified according to their diagnostic ions and retention indices calculated with a C21–C40 alkane standard solution (Merck, Darmstadt, Germany). The pre-defined integrator Agile 2 was used for the peak integration algorithm to allow for maximum flexibility. All peaks were then additionally checked for correct integration and quantification, and, where necessary, re-integrated manually. To standardize the peak areas, they were divided by total peak area sum per chromatogram, resulting in relative ratios. A Principal component analysis was performed based on the relative overall CHC divergence between the putative ecotypes and sexes with the program R, version 4.1.0 (R Core Team, 2020).
2.3 Microsatellite and population genetic analysis
DNA was isolated from ten adult isofemale line foundresses collected from carrion, and from 18 foundresses emerging from the fly hosts collected from the nest boxes (one per nest box) using a standard high-salt chloroform protocol (Maniatis et al., 1982). A set of 14 established microsatellite loci was used to determine genetic differentiation between all tested individuals from the Hoge Veluwe population (Beukeboom et al., 2010; Pannebakker et al., 2010; Koevoets et al., 2012). Microsatellite details are provided in Supplementary Table S1. Microsatellite markers were amplified using the Qiagen multiplex PCR kit (Qiagen, Hilden, Germany) according to the manufacturer’s recommendations. Amplification was done in 5 μl volumes, PCR conditions were as follows: Denaturation at 95°C for 15 min, followed by 30 cycles of 94°C for 30 s, 57°C for 1.5 min, and 72°C for 1 min, followed by a final extension at 72°C for 45 min. Fragments were diluted 250:1, separated on an Applied Biosystems 3730 DNA Analyzer (Applied Biosystems, Foster City, CA, USA) and analyzed using Geneious R11 (Biomatters Ltd., Auckland, New Zealand). Hardy-Weinberg equilibrium was calculated for all microsatellite markers using a heterozygosity-based estimator (GIS) (Nei, 1987) and P-values based on 9999 permutations. All marker pairs were tested for linkage disequilibrium (LD), using a Markov chain method (10,000 dememorization steps, 100 batches, 5,000 iterations) and Fisher’s exact test, followed by Bonferroni correction for multiple testing. Genetic diversity parameters, number of alleles (Na), effective number of alleles (Ne), observed heterozygosity (Ho), expected heterozygosity (He) and the fixation index (GIS) were calculated. To quantify the degree of differentiation between wasps collected from carrion and the nest box habitats, we calculated the fixation index G’ST, which is an FST equivalent that corrects for bias from sampling only a limited number of populations (Nei, 1987), and P-values based on 9999 permutations. Next, we tested for the existence of genetic clusters within the Hoge Veluwe population, using a Principal component analysis (PCA) based on the allele frequencies, and a Bayesian assignment as implemented in the program InStruct 1.0 (Gao et al., 2007), which is an extension of the STRUCTURE algorithm (Pritchard et al., 2000) that allows for deviations from the Hardy-Weinberg equilibrium through correction for inbreeding. Because N. vitripennis shows high levels of inbreeding (Luna and Hawkins, 2004; Grillenberger et al., 2008), it is relevant to account for this in our analysis. Using InStruct, we tested a range of 1–12 clusters (K), with a burn-in of 100,000 and 1,000,000 MCMC iterations and 20 iterations for each K, using the inference of population structure and the selfing rates for subpopulations mode (mode 2). The optimal K was inferred from the deviance information criterion (DIC: Gao et al., 2011). As an alternative method, we estimated the number of clusters using a K-means clustering method based on the analysis of molecular variance (AMOVA, Excoffier et al., 1992; Meirmans, 2012), for K=1–12 clusters, with the simulated annealing approach for 1,000,000 steps with 20 random starts. The optimal K was inferred from the Bayesian information criterion (BIC). All analyses were performed using GenoDive 3.06 (Meirmans, 2020), except for the pair-wise linkage analysis between the markers that was done using Genepop 4.7.5 (Raymond and Rousset, 1995; Rousset, 2008).
3 Results
3.1 Trapping success on carrion and in nest boxes
In June 2018, a total of 45 nest boxes in the Hoge Veluwe National Park were inspected for the presence or absence of fly pupae. Twenty-six nest boxes (57.7%) contained fly pupae, and of those fly-infested nest boxes, 19 (73%) contained N. vitripennis wasps. Sampling on the carrion yielded a total of ten adult N. vitripennis females (N=7 and N=3 on each deer leg, respectively).
3.2 Chemical analysis
We were able to detect 49 distinct CHC compounds in our sampled N. vitripennis isofemale lines representative of both postulated ecotypes. Their identifications or, in ambiguous cases, all potential configurations according to their diagnostic ions, as well as their mean relative quantities per postulated ecotype and each respective sex are given in Supplementary Table S2. A Principal component analysis only clearly discriminated the two sexes into two distinct clusters, with no recognizable divergence based on postulated ecotype. The first two principal components contributed to 42.6% and 19.2% to the total separation of the chemical profiles, respectively (Figure 2). A graphical overview of relative quantities for each individual CHC compound identified in our analysis separated by sex and postulated ecotype is given in Supplementary Figure S2.
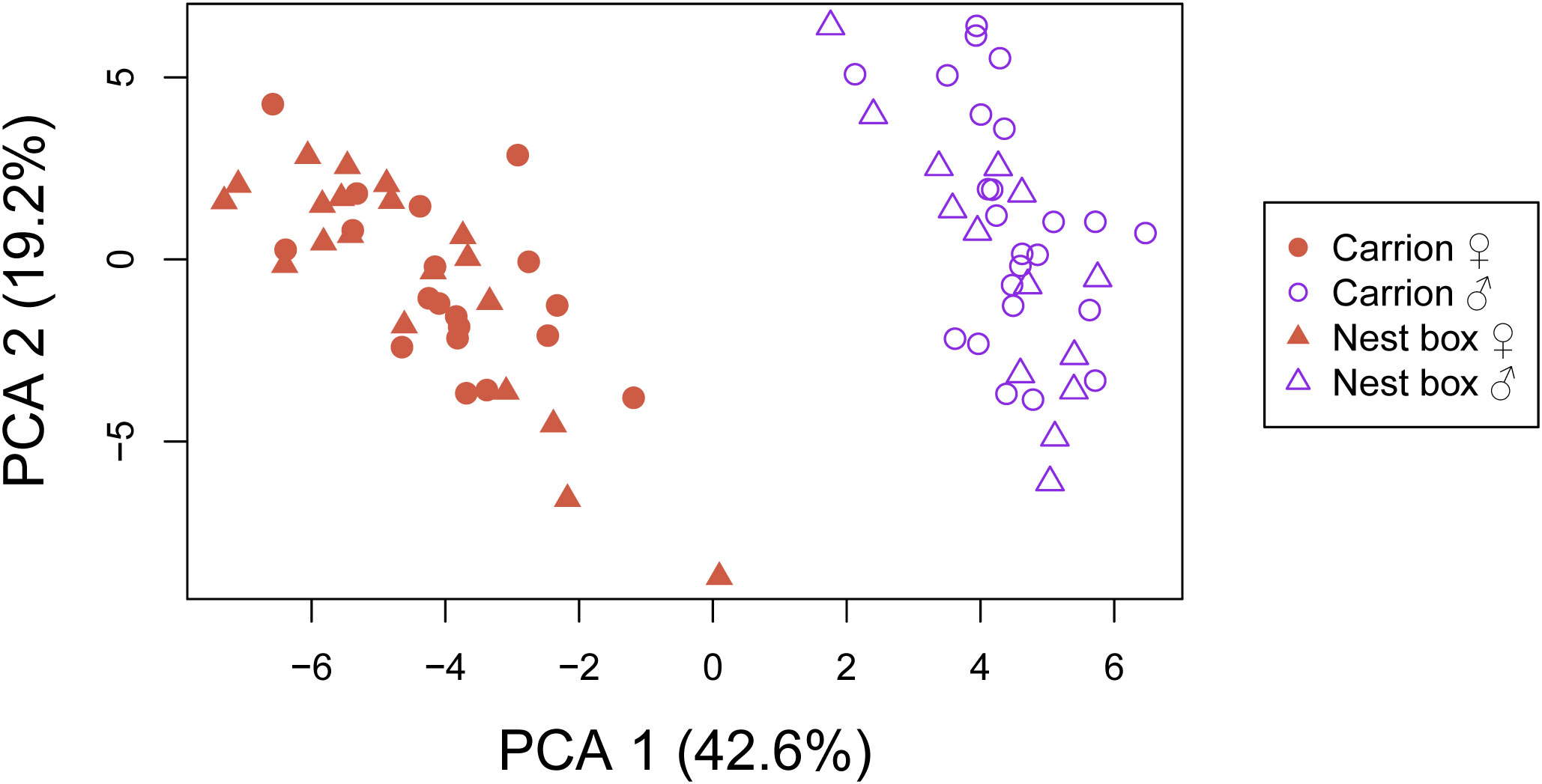
Figure 2 Principal component analysis (PCA) based on cuticular hydrocarbon extracts from 77 individual Nasonia vitripennis wasps of both sexes, representative of the carrion and bird nest box ecotypes. Carrion ecotype: 19 females from 4 isofemale lines, 24 males from five isofemale lines. Bird nest box ecotype: 20 females from four isofemale lines, 14 males from three isofemale lines. The first two principal components contributed 42.6% and 19.2% to the total separation of the samples, respectively.
3.3 No genetic differentiation between N. vitripennis wasps from distinct habitats
Microsatellite profiles were generated for 27 foundresses of the isofemale lines derived from carrion (n=9) and nest boxes (n=18). Two females from nest boxes were excluded from further analysis, because seven and four of the microsatellite loci failed to amplify in these individuals, respectively, resulting in a robust dataset of 25 females in total that were genotyped for all 14 established microsatellite loci. Of the 14 microsatellite loci, only two were in Hardy-Weinberg equilibrium (HWE), Nv114 and Nv303 (Supplementary Table S1). The other 12 loci showed significant deviation from HWE, consistent with the generally high inbreeding rates observed in this species (Grillenberger et al., 2008). No significant linkage disequilibrium was detected between the microsatellite loci. Based on these analyses, we decided to include all 14 loci in our subsequent dataset. Wasps from both microhabitats showed a mean heterozygosity Ho=0.537 (SD=0.014), and a mean GIS=0.309 (SD=0.038) (Table 1), the latter indicating a heterozygote deficiency, which is likely due to inbreeding. A G’st analysis showed no genetic differentiation between N. vitripennis females collected from nest boxes versus females collected from carrion (G’st=0.003 (SD=0.007), P=0.347). The Bayesian assignment using InStruct found support for K=9 genetic clusters (Supplementary Table S3), but these did not diverge according to microhabitat (Figure 3). However, the proportions of ancestry estimated by Instruct (q-values) are very similar among the individual wasps, consistent with absence of genetic structure within the Hoge Veluwe population. This is confirmed by the results of the K-means clustering, which indicate K=1, or a single genetic group for the N. vitripennis population at the Hoge Veluwe without separate substructures (Supplementary Table S4). This is further reflected in a Principal component analysis that showed no distinct genetic clusters according to host habitat i.e., postulated ecotype (Figure 4).

Table 1 Number of alleles, fixation index and heterozygosity of the analyzed Nasonia vitripennis population from the Hoge Veluwe National Park, Netherlands.
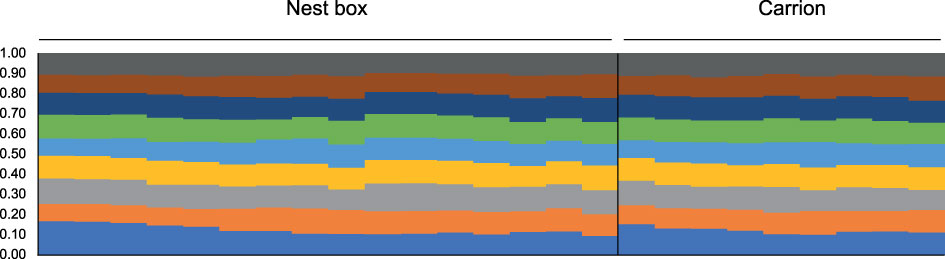
Figure 3 Population structure of Nasonia vitripennis in the Hoge Veluwe National Park in the Netherlands as calculated with InStruct for optimal number (K=9) of genetic clusters. Each individual wasp is represented by a vertical bar and colored according to assignment to one of nine ancestry clusters. Samples are ordered according to cluster assignment and microhabitat.
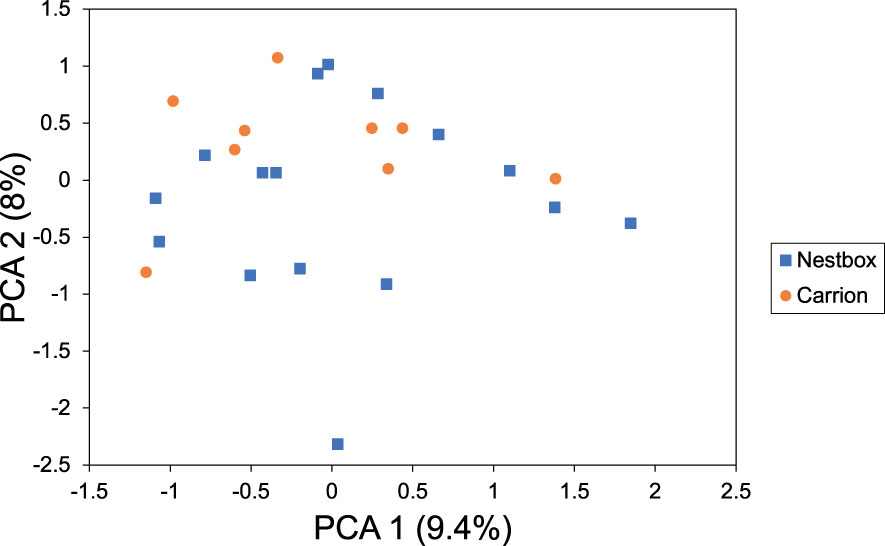
Figure 4 Principal component analysis (PCA) of the divergence of 14 microsatellite loci analyzed from 25 foundresses collected from carrion (n=9) and bird nest boxes (n=16). Principal component 1 (PC 1) accounted for 9.4% of the total separation, PC 2 for 8%.
4 Discussion
Based on our chemical profile analysis as well as our population genetic assessment, we could not detect any degree of separation into ecotypes in the studied Nasonia vitripennis population from the Netherlands. Individual isofemale lines derived from host pupae either occurring on bird next boxes or carrion could not be unambiguously differentiated as either ecotype based on distinct chemical profiles or their genetic divergence.
Concerning cuticular hydrocarbon (CHC) divergence, it has been shown that CHC profiles have the potential to locally adapt to divergent ecological conditions within a few generations (Menzel et al., 2017; Menzel et al., 2018; Hartke et al., 2019). CHC differences might also occur due to assortative mating and have been found to be correlated with speciation events in other insects (Schwander et al., 2013; Chung and Carroll, 2015). Moreover, CHC profiles have frequently been found as the first and most characteristic phenotypic traits to diverge between populations and evolutionarily young species pairs (Espelie et al., 1990; Raychoudhury et al., 2010; Sprenger et al., 2021). Therefore, it is surprising that we could not detect any congruent CHC divergence separating the two postulated N. vitripennis ecotypes. Interestingly, a study focusing solely on bird nest boxes on a wide European latitudinal gradient ranging from Corsica to Finland also did not find any discernible CHC patterns correlating with local population clines (Buellesbach et al., 2022a). This strongly suggests that CHCs constitute genetically fixed traits with comparably little plasticity for local adaptation in this species (Niehuis et al., 2011; Buellesbach et al., 2022b).
Due to the long-standing postulation of the discernibility between the bird and carrion ecotypes of N. vitripennis (Grassberger and Frank, 2004; Peters and Abraham, 2010; Malec et al., 2021), our findings that clearly do not support their unambiguous differentiation on a phenotypic and genetic level were unexpected. An important aspect largely neglected in earlier studies conducted on populations of this species is the estimation of how frequent habitat switches can occur in nature. As inseminated female N. vitripennis wasps can disperse over distances as far as 2 km (Grillenberger et al., 2009), steady gene flow between bird nest and carrion populations within these distances, as would be the case in our studied ecosystem in the Netherlands, appears very likely. Another potentially important aspect is the difference in phenology between the two postulated ecotypes: As the occurrence of carrion can be expected to be year-round, the nest ecotype would presumably be restricted to the respective birds’ breeding season. When this season has passed by the end of spring for most birds, N. vitripennis wasps are unlikely to already enter diapause, which usually only occurs later in the year (Saunders, 1965; Paolucci et al., 2013). It is thus far unknown whether wasps parasitizing hosts in bird nests during early summer can simply switch to carrion hosts once bird breeding season ends. However, wasps collected from bird nest boxes do readily parasitize carrion-breeding Calliphora hosts (van de Zande et al., 2014; Kalyanaraman et al., 2021). It is thus unlikely that gene flow between microhabitats is interrupted over long enough periods of time to allow for the establishment of stable and distinct ecotypes in European N. vitripennis populations.
Interestingly, Malec et al. recently reported a reduction in F1 offspring between different N. vitripennis strains collected in microhabitats corresponding to the postulated ecotypes within a German population, suggesting incipient reproductive barriers (Malec et al., 2021). However, they did not verify the infection status caused by the naturally occurring Wolbachia bacteria in their investigated N. vitripennis strains. The strains used in Malec et al. (2021) had been cultured in the laboratory for over 20 generations. However, maternal Wolbachia transmission is not 100% (Hoffmann et al., 1990; Turelli et al., 1992) and prolonged laboratory rearing has been reported to result in loss of the Wolbachia infection in N. vitripennis strains (Pannebakker et al., 2020). This is crucial, as Wolbachia-infected males are incompatible with Wolbachia-uninfected females, leading to different degrees of offspring reduction (Breeuwer and Werren, 1990; Bordenstein and Werren, 1998; Bordenstein et al., 2001). Therefore, without accounting for the persistence of Wolbachia infections, interpretations of the cause of offspring reduction between strains derived from different ecotypes have to remain very cautious. However, previous population genetic studies indicated gene flow can become limited over larger distances (300 km or further) in separated N. vitripennis populations (Grillenberger et al., 2009; Paolucci et al., 2013), which could theoretically allow for different degrees of local adaptation to occur. Nevertheless, in the aforementioned study on European N. vitripennis clines over a large latitudinal gradient (> 3,500 km), no population-specific mate preference or assortative mating behavior that would have hinted at incipient prezygotic reproductive isolation could be detected (Buellesbach et al., 2022a). This also suggested that female sexual attractiveness as encoded in the CHC profiles remains constantly detectable, potentially constraining any larger profile divergences at least for the females in N. vitripennis (Sun et al., 2023).
Our results therefore challenge the long-standing assumption of clearly distinguishable ecotypes based on host microhabitats in N. vitripennis and invite further, more careful investigations of within-population variation in this cosmopolitan parasitoid species. Future studies could potentially investigate several different populations from more extreme environments, and also take into account the postulated ancestral state of host preference, likely constituting carrion since sampled bird nest boxes are almost always primarily human-made (e.g. Darling and Werren, 1990; Peters and Abraham, 2010; Buellesbach et al., 2022a). Furthermore, natural dispersal rates should be monitored and considered more cautiously to obtain a more robust estimate on the actual degree of gene flow occurring between different host patches.
In conclusion, we could not confirm the differentiation into the nest and carrion ecotype in our sampled N. vitripennis population from the Netherlands, neither on the population genetic nor on the phenotypic level assessed through the wasps’ chemical profiles. Our findings invite a more cautious approach to studying ecotype formation in this model organism for parasitoid wasps and strongly hint at persisting gene flow despite the ecological preference for different host microhabitat patches.
Data availability statement
The datasets presented in this study can be found in online repositories. The names of the repository/repositories and accession number(s) can be found in the article/Supplementary Material.
Ethics statement
The manuscript presents research on animals that do not require ethical approval for their study.
Author contributions
Conceptualization: BP, ML. Methodology: BP, JB, ML. Validation: BP, JV, JB. Formal analysis: BP, JV, JB. Investigation: BP, JV, ML, JB. Resources: BP, JB. Data curation: BP, JB. Writing – original draft preparation: BP, JB, ML. Writing – review and editing: BP, JB, ML. Visualization, BP, JB, ML. Supervision: BP, JB. Project administration: BP, JB. Funding acquisition: BP, JB. All authors contributed to the article and approved the submitted version.
Funding
This research was partially supported by a research grant by the Deutsche Forschungsgemeinschaft (DFG, German Research Foundation) – 427879779 to JB (BU3439/1-1).
Acknowledgments
We thank Marcel Visser and Kees van Oers (KNAW-NIOO), Gerard Driessen, Annika Liefferink and Liset Eendebak for help with the collection of the wasps at Hoge Veluwe National Park, and the Hoge Veluwe management for providing access and permission to collect and deer carrion. We also thank Gabriella Bukovinszkine-Kiss for help with strain maintenance. Frederik Post and Kristina Emke provided invaluable help with chemical data acquisition and analysis. Marc Maas drew the life cycle of N. vitripennis in Figure 1.
Conflict of interest
The authors declare that the research was conducted in the absence of any commercial or financial relationships that could be construed as a potential conflict of interest.
Publisher's note
All claims expressed in this article are solely those of the authors and do not necessarily represent those of their affiliated organizations, or those of the publisher, the editors and the reviewers. Any product that may be evaluated in this article, or claim that may be made by its manufacturer, is not guaranteed or endorsed by the publisher.
Supplementary material
The Supplementary Material for this article can be found online at: https://www.frontiersin.org/articles/10.3389/fevo.2023.1232639/full#supplementary-material
References
Bakovic V., Schuler H., Schebeck M., Feder J. L., Stauffer C., Ragland G. J. (2019). Host plant-related genomic differentiation in the European cherry fruit fly, Rhagoletis cerasi. Mol. Ecol. 28, 4648–4666. doi: 10.1111/mec.15239
Bell K., Bordenstein S. R. (2022). A Margulian view of symbiosis and speciation: The Nasonia wasp system. Symbiosis 87, 3–10. doi: 10.1007/s13199-022-00843-2
Beukeboom L. W., Niehuis O., Pannebakker B. A., Koevoets T., Gibson J. D., Shuker D. M., et al. (2010). A comparison of recombination frequencies in intraspecific versus interspecific mapping populations of Nasonia. Heredity 104, 302–309. doi: 10.1038/hdy.2009.185
Bordenstein S. R., O’Hara F. P., Werren J. H. (2001). Wolbachia induced incompatibility precedes other hybrid incompatibilities in Nasonia. Nature 409, 707–710. doi: 10.1038/35055543
Bordenstein S. R., Werren J. H. (1998). Effects of A and B Wolbachia and host genotype on interspecies cytoplasmic incompatibility in Nasonia. Genetics 148, 1833–1844. doi: 10.1093/genetics/148.4.1833
Breeuwer J. A. J., Werren J. H. (1990). Microorganisms associated with chromosome destruction and reproductive isolation between two insect species. Nature 346, 558–560. doi: 10.1038/346558a0
Buellesbach J., Diao W., Schmitt T., Beukeboom L. W. (2022a). Micro-climate correlations and conserved sexual dimorphism of cuticular hydrocarbons in European populations of the jewel wasp Nasonia vitripennis. Ecol. Entomology 47, 38–51. doi: 10.1111/een.13089
Buellesbach J., Gadau J., Beukeboom L. W., Echinger F., Raychoudhury R., Werren J. H., et al. (2013). Cuticular hydrocarbon divergence in the jewel wasp Nasonia: Evolutionary shifts in chemical communication channels? J. Evol. Biol. 26, 2467–2478. doi: 10.1111/jeb.12242
Buellesbach J., Holze H., Schrader L., Liebig J., Schmitt T., Gadau J., et al. (2022b). Genetic and genomic architecture of species-specific cuticular hydrocarbon variation in parasitoid wasps. Proc. R. Soc Lond. Ser. B: Biol. Sci. 289, 20220336. doi: 10.1098/rspb.2022.0336
Butlin R. K. (1987). Speciation by reinforcement. Trends Ecol. Evol. 2, 8–13. doi: 10.1016/0169-5347(87)90193-5
Chung H., Carroll S. B. (2015). Wax, sex and the origin of species: Dual roles of insect cuticular hydrocarbons in adaptation and mating. Bioessays 37, 822–830. doi: 10.1002/bies.201500014
Crespo-Pérez V., Kazakou E., Roubik D. W., Cárdenas R. E. (2020). The importance of insects on land and in water: a tropical view. Curr. Opin. Insect Sci. 40, 31–38. doi: 10.1016/j.cois.2020.05.016
Darling D. C., Werren J. H. (1990). Biosystematics of Nasonia (Hymenoptera: Pteromalidae): Two new species reared from birds’ nests in North America. Ann. Entomological Soc. America 83, 352–370. doi: 10.1093/aesa/83.3.352
Espelie K. E., Berisford C. W., Dahlsten D. L. (1990). Cuticular hydrocarbons of geographically isolated populations of Rhopalicus pulchripennis (Hymenoptera, Pteromalidae) - Evidence for 2 species. Comp. Biochem. Physiology Part B: Biochem. Mol. Biol. 96, 305–308. doi: 10.1016/0305-0491(90)90378-7
Everaerts C., Maekawa K., Farine J. P., Shimada K., Luykx P., Brossut R., et al. (2008). The Cryptocercus punctulatus species complex (Dictyoptera: Cryptocercidae) in the eastern United States: Comparison of cuticular hydrocarbons, chromosome number, and DNA sequences. Mol. Phylogen. Evol. 47, 950–959. doi: 10.1016/j.ympev.2008.03.011
Excoffier L., Smouse P. E., Quattro J. M. (1992). Analysis of molecular variance inferred from metric distances among DNA haplotypes: application to human mitochondrial DNA restriction data. Genetics 131, 479–491. doi: 10.1093/genetics/131.2.479
Finck J., Berdan E. L., Mayer F., Ronacher B., Geiselhardt S. (2016). Divergence of cuticular hydrocarbons in two sympatric grasshopper species and the evolution of fatty acid synthases and elongases across insects. Sci. Rep. 6, 33695. doi: 10.1038/srep33695
Gao H., Bryc K., Bustamante C. D. (2011). On identifying the optimal number of population clusters via the deviance information criterion. PloS One 6, e21014. doi: 10.1371/journal.pone.0021014
Gao H., Williamson S., Bustamante C. D. (2007). A Markov chain Monte Carlo approach for joint inference of population structure and inbreeding rates from multilocus genotype data. Genetics 176, 1635–1651. doi: 10.1534/genetics.107.072371
Giesbers M. C. W. G., Gerritsma S., Buellesbach J., Diao W., Pannebakker B. A., van de Zande L., et al. (2013). “Prezygotic isolation in the parasitoid wasp genus Nasonia,” in Speciation: natural processes, genetics and biodiversity (Hauppauge, NY: Nova Science Publishers), 165–192.
Grassberger M., Frank C. (2004). Initial study of arthropod succession on pig carrion in a central European urban habitat. J. Med. Entomol. 41, 511–523. doi: 10.1603/0022-2585-41.3.511
Grillenberger B. K., Gadau J., Bijlsma R., Van De Zande L., Beukeboom L. W. (2009). Female dispersal and isolation-by-distance of Nasonia vitripennis populations in a local mate competition context. Entomologia Experimentalis Applicata 132, 147–154. doi: 10.1111/j.1570-7458.2009.00881.x
Grillenberger B. K., Koevoets T., Burton-Chellew M. N., Sykes E. M., Shuker D. M., Van de Zande L., et al. (2008). Genetic structure of natural Nasonia vitripennis populations: validating assumptions of sex-ratio theory. Mol. Ecol. 17, 2854–2864. doi: 10.1111/j.1365-294X.2008.03800.x
Hartke J., Sprenger P. P., Sahm J., Winterberg H., Orivel J., Baur H., et al. (2019). Cuticular hydrocarbons as potential mediators of cryptic species divergence in a mutualistic ant association. Ecol. Evol. 9, 9160–9176. doi: 10.1002/ece3.5464
Higgie M., Chenoweth S., Blows M. W. (2000). Natural selection and the reinforcement of mate recognition. Science 290, 519–521. doi: 10.1126/science.290.5491.519
Hoffmann A. A., Turelli M., Harshman L. G. (1990). Factors affecting the distribution of cytoplasmic incompatibility in Drosophila simulans. Genetics 126, 933–948. doi: 10.1093/genetics/126.4.933
Holt R. D. (2009). Bringing the Hutchinsonian niche into the 21st century: ecological and evolutionary perspectives. Proc. Natl. Acad. Sci. 106, 19659–19665. doi: 10.1073/pnas.0905137106
Hutchinson G. E. (1957). Concluding remarks. Cold Spring Harbor Symp. Quant. Biol. 22, 415–427. doi: 10.1101/SQB.1957.022.01.039
Kalyanaraman D., Gadau J., Lammers M. (2021). The generalist parasitoid Nasonia vitripennis shows more behavioural plasticity in host preference than its three specialist sister species. Ethology 127, 964–978. doi: 10.1111/eth.13217
Koevoets T., Niehuis O., Van De Zande L., Beukeboom L. (2012). Hybrid incompatibilities in the parasitic wasp genus Nasonia: negative effects of hemizygosity and the identification of transmission ratio distortion loci. Heredity 108, 302–311. doi: 10.1038/hdy.2011.75
Koppik M., Thiel A., Hoffmeister T. S. (2014). Adaptive decision making or differential mortality: what causes offspring emergence in a gregarious parasitoid? Entomologia Experimentalis Et Applicata 150, 208–216. doi: 10.1111/eea.12154
Le Moan A., Gagnaire P. A., Bonhomme F. (2016). Parallel genetic divergence among coastal–marine ecotype pairs of European anchovy explained by differential introgression after secondary contact. Mol. Ecol. 25, 3187–3202. doi: 10.1111/mec.13627
Lin Y., Hyyppä J. (2019). Characterizing ecosystem phenological diversity and its macroecology with snow cover phenology. Sci. Rep. 9, 15074. doi: 10.1038/s41598-019-51602-1
Luna M. G., Hawkins B. A. (2004). Effects of inbreeding versus outbreeding in Nasonia vitripennis (Hymenoptera: pteromalidae). Environ. Entomol. 33, 765–775. doi: 10.1603/0046-225X-33.3.765
Mair M. M., Kmezic V., Huber S., Pannebakker B. A., Ruther J. (2017). The chemical basis of mate recognition in two parasitoid wasp species of the genus Nasonia. Entomol. Exp. Appl. 164, 1–15. doi: 10.1111/eea.12589
Malec P., Weber J., Böhmer R., Fiebig M., Meinert D., Rein C., et al. (2021). The emergence of ecotypes in a parasitoid wasp: a case of incipient sympatric speciation in Hymenoptera? BMC Ecol. Evol. 21, 204. doi: 10.1186/s12862-021-01938-y
Maniatis T., Fritsch E. F., Sambrook J. (1982). Molecular cloning: A laboratory manual (Cold Spring Harbor, N. Y: Cold Spring Harbor Laboratory).
Meirmans P. G. (2012). AMOVA-based clustering of population genetic data. J. Hered. 103, 744–750. doi: 10.1093/jhered/ess047
Meirmans P. G. (2020). Genodive version 3.0: Easy-to-use software for the analysis of genetic data of diploids and polyploids. Mol. Ecol. Resour. 20, 1126–1131. doi: 10.1111/1755-0998.13145
Menz M. H., Phillips R. D., Anthony J. M., Bohman B., Dixon K. W., Peakall R. (2015). Ecological and genetic evidence for cryptic ecotypes in a rare sexually deceptive orchid, Drakaea elastica. Bot. J. Linn. Soc 177, 124–140. doi: 10.1111/boj.12230
Menzel F., Blaimer B. B., Schmitt T. (2017). How do cuticular hydrocarbons evolve? Physiological constraints and climatic and biotic selection pressures act on a complex functional trait. Proc. R. Soc. London Ser. B: Biol. Sci. 284, 20161727. doi: 10.1098/rspb.2016.1727
Menzel F., Zumbusch M., Feldmeyer B. (2018). How ants acclimate: Impact of climatic conditions on the cuticular hydrocarbon profile. Funct. Ecol. 32, 657–666. doi: 10.1111/1365-2435.13008
Niehuis O., Buellesbach J., Judson A. K., Schmitt T., Gadau J. (2011). Genetics of cuticular hydrocarbon differences between males of the parasitoid wasps Nasonia giraulti and Nasonia vitripennis. Heredity 107, 61–70. doi: 10.1038/hdy.2010.157
Ortiz-Barrientos D., Grealy A., Nosil P. (2009). “The genetics and ecology of reinforcement implications for the evolution of prezygotic isolation in sympatry and beyond,” in Year in evolutionary biology (Oxford: Blackwell Publishing), 156–182.
Pannebakker B. A., Cook N., van den Heuvel J., van de Zande L., Shuker D. M. (2020). Genomics of sex allocation in the parasitoid wasp Nasonia vitripennis. BMC Genomics 21, 499. doi: 10.1186/s12864-020-06904-4
Pannebakker B. A., Niehuis O., Hedley A., Gadau J., Shuker D. (2010). The distribution of microsatellites in the Nasonia parasitoid wasp genome. Insect Mol. Biol. 19, 91–98. doi: 10.1111/j.1365-2583.2009.00915.x
Paolucci S., van de Zande L., Beukeboom. L. W. (2013). Adaptive latitudinal cline of photoperiodic diapause induction in the parasitoid Nasonia vitripennis in Europe. J. Evolutionary Biol. 26, 705–718. doi: 10.1111/jeb.12113
Peters R., Abraham R. (2010). The food web of parasitoid wasps and their non-phytophagous fly hosts in birds’ nests (Hymenoptera: Chalcidoidea, and Diptera: Cyclorrhapha). J. Nat. Hist. 44, 625–638. doi: 10.1080/00222930903437317
Pigliucci M. (2005). Evolution of phenotypic plasticity: where are we going now? Trends Ecol. Evol. 20, 481–486. doi: 10.1016/j.tree.2005.06.001
Pritchard J. K., Stephens M., Donnelly P. (2000). Inference of population structure using multilocus genotype data. Genetics 155, 945–959. doi: 10.1093/genetics/155.2.945
Raven P. H., Wagner D. L. (2021). Agricultural intensification and climate change are rapidly decreasing insect biodiversity. Proc. Natl. Acad. Sci. 118, e2002548117. doi: 10.1073/pnas.2002548117
Raychoudhury R., Desjardins C. A., Buellesbach J., Loehlin D. W., Grillenberger B. K., Beukeboom L. W., et al. (2010). Behavioral and genetic characteristics of a new species of Nasonia. Heredity 104, 278–288. doi: 10.1038/hdy.2009.147
Raymond M., Rousset F. (1995). GENEPOP (Version 1.2): population genetics software for exact tests and ecumenicism. J. Hered. 86, 248–249. doi: 10.1093/oxfordjournals.jhered.a111573
R Core Team (2020). R: A language and environment for statistical computing (Vienna, Austria: R foundation for statistical computing). Available at: http://www.R-project.org.
Rousset F. (2008). genepop’007: a complete re-implementation of the genepop software for Windows and Linux. Mol. Ecol. Resour. 8, 103–106. doi: 10.1111/j.1471-8286.2007.01931.x
Saunders D. S. (1965). Larval diapause of maternal origin: Induction of diapause in Nasonia vitripennis (Walk.) (Hymenoptera: Pteromalidae). J. Exp. Biol. 42, 495–508. doi: 10.1242/jeb.42.3.495
Schröder H. (2000). Crossing experiments and molecularbiological researches at two ecotypes of Nasonia vitripennis (Hymenoptera, Pteromalidae). Mitt. der Deutschen Gesellschaft für allgemeine und angewandte Entomologie 12, 165–168.
Schwander T., Arbuthnott D., Gries R., Gries G., Nosil P., Crespi B. J. (2013). Hydrocarbon divergence and reproductive isolation in Timema stick insects. BMC Evol. Biol. 13, 151. doi: 10.1186/1471-2148-13-151
Scudder G. G. E. (2017). “The importance of insects,” in Insect biodiversity: science and society. Eds. Foottit R. G., Adler P. H. (Hoboken, NJ: Wiley-Blackwell), 9–43.
Shuker D. M. (2023). “The curious incident of the wasp in the fig fruit: sex allocation and the extended evolutionary synthesis,” in Evolutionary biology: contemporary and historical reflections upon core theory. Eds. Dickins T. E., Dickins B. J. A. (Cham: Springer International Publishing), 473–504.
Sprenger P. P., Hartke J., Schmitt T., Menzel F., Feldmeyer B. (2021). Candidate genes involved in cuticular hydrocarbon differentiation between cryptic, parabiotic ant species. G3 (Bethesda Md.) 11, jkab078. doi: 10.1093/g3journal/jkab078
Steiner S., Hermann N., Ruther J. (2006). Characterization of a female-produced courtship pheromone in the parasitoid Nasonia vitripennis. J. Chem. Ecol. 32, 1687–1702. doi: 10.1007/s10886-006-9102-3
Stronen A. V., Norman A. J., Vander Wal E., Paquet P. C. (2022). The relevance of genetic structure in ecotype designation and conservation management. Evolutionary Appl. 15, 185–202. doi: 10.1111/eva.13339
Sun W., Lange M. I., Gadau J., Buellesbach J. (2023). Decoding the genetic and chemical basis of sexual attractiveness in parasitic wasps. eLife 12, e86182. doi: 10.7554/eLife.86182.sa2
Sunamura E., Hoshizaki S., Sakamoto H., Fujii T., Nishisue K., Suzuki S., et al. (2011). Workers select mates for queens: A possible mechanism of gene flow restriction between supercolonies of the invasive Argentine ant. Naturwissenschaften 98, 361–368. doi: 10.1007/s00114-011-0778-z
Takahashi A., Tsaur S. C., Coyne J. A., Wu C. I. (2001). The nucleotide changes governing cuticular hydrocarbon variation and their evolution in Drosophila melanogaster. Proc. Natl. Acad. Sci. U.S.A. 98, 3920–3925. doi: 10.1073/pnas.061465098
Taylor R. S., Manseau M., Horn R. L., Keobouasone S., Golding G. B., Wilson P. J. (2020). The role of introgression and ecotypic parallelism in delineating intraspecific conservation units. Mol. Ecol. 29, 2793–2809. doi: 10.1111/mec.15522
Turelli M., Hoffmann A. A., McKechnie S. W. (1992). Dynamics of cytoplasmic incompatibility and mtDNA variation in natural Drosophila simulans populations. Genetics 132, 713–723. doi: 10.1093/genetics/132.3.713
Turesson G. (1922). The species and the variety as ecological units. Hereditas 3, 100–113. doi: 10.1111/j.1601-5223.1922.tb02727.x
van den Assem J., Gijswijt M. J., Nubel B. K. (1980). Observations on courtship and mating strategies in a few species of parasitic wasps (Chalcidoidea). Netherlands J. Zoology 30, 208–227. doi: 10.1163/002829679X00386
van de Zande L., Ferber S., de Haan A., Beukeboom L. W., van Heerwaarden J., Pannebakker B. A. (2014). Development of a Nasonia vitripennis outbred laboratory population for genetic analysis. Mol. Ecol. Resour. 14, 578–587. doi: 10.1111/1755-0998.12201
Werren J. H., Richards S., Desjardins C. A., Niehuis O., Gadau J., Colbourne J. K., et al. (2010). Functional and evolutionary insights from the genomes of three parasitoid Nasonia species. Science 327, 343–348. doi: 10.1126/science.1178028
Yannic G., Ortego J., Pellissier L., Lecomte N., Bernatchez L., Côté S. D. (2018). Linking genetic and ecological differentiation in an ungulate with a circumpolar distribution. Ecography 41, 922–937. doi: 10.1111/ecog.02995
Keywords: cuticular hydrocarbons, chemical ecology, microsatellites, population structure, speciation, niche differentiation, parasitic Hymenoptera
Citation: Buellesbach J, Lammers M, van de Belt J and Pannebakker BA (2023) Chemical and population genetic analysis show no evidence of ecotype formation in a European population of the parasitoid wasp Nasonia vitripennis. Front. Ecol. Evol. 11:1232639. doi: 10.3389/fevo.2023.1232639
Received: 31 May 2023; Accepted: 04 September 2023;
Published: 26 September 2023.
Edited by:
Johannes Stökl, University of Bayreuth, GermanyReviewed by:
Susan Gershman, The Ohio State University, United StatesAudrey Bras, University of Helsinki, Finland
Copyright © 2023 Buellesbach, Lammers, van de Belt and Pannebakker. This is an open-access article distributed under the terms of the Creative Commons Attribution License (CC BY). The use, distribution or reproduction in other forums is permitted, provided the original author(s) and the copyright owner(s) are credited and that the original publication in this journal is cited, in accordance with accepted academic practice. No use, distribution or reproduction is permitted which does not comply with these terms.
*Correspondence: Jan Buellesbach, YnVlbGxlc2JAdW5pLW11ZW5zdGVyLmRl; Bart A. Pannebakker, YmFydC5wYW5uZWJha2tlckB3dXIubmw=
†These authors have contributed equally to this work