- 1Univ. Littoral Côte d’Opale, CNRS, Univ. Lille, IRD, UMR 8187, LOG, Laboratoire d’Océanologie et de Géosciences, Wimereux, France
- 2Centre d’Innovation et de Recherche des Matériaux et Polymères (CIRMAP), Service des Matériaux Polymères et Composites (SMPC), Université de Mons, Mons, Belgium
- 3Center for Marine Science and Technology, Amity Institute of Biotechnology, Amity University, Noida, Uttar Pradesh, India
The accumulation of plastic debris around the world, especially in marine environments, has been well documented during the past decades. Recent studies have found that inorganic surfaces of microplastics (MPs) can be used by microorganisms as living substrates and form an ecosystem named “plastisphere.” Some microorganisms present in MPs are capable of producing polymer-degrading enzymes. In addition, MPs can also serve as vectors and carry microorganisms (including potential pathogens) into higher trophic levels through their ingestion by animals. In this study, impacts on copepod microbiota during chronic exposure to MPs were investigated by exposing copepods to a classic single-use polymer (low-density polyethylene (LDPE)) and a biodegradable polymer (polybutylene adipate terephthalate (PBAT)). Copepods were exposed to “virgin” and “weathered” MPs during four generations at an environmentally relevant concentration of 300 µg/L, followed by one “detoxification” generation without MP exposition. Impacts of MP exposure on copepod microbiota were investigated using 16S rRNA gene high-throughput sequencing. The result of nonmetric multidimensional scaling (NMDS) analysis showed that copepods (with or without MP exposure) carried distinguishable microbiota as compared with the microbiota of water and microalgae used for maintaining copepods. According to the results of permutational analysis of variance (PERMANOVA), the microbiota of MP-exposed (both PBAT and LDPE) copepods was significantly different from the microbiota of unexposed copepods during generations one to four. After “detoxification,” however, no significant difference in microbiota composition was observed among all generation five copepods. Altogether, impacts on copepod microbiota of MP exposure for multiple generations were observed, despite plastic origin (biodegradable or not) and aging conditions. Furthermore, copepod microbiota seemed to return to their original structure as soon as the MP exposure stopped.
Introduction
Due to their numerous usages in modern society for packaging, building industry, automotive, and more, world petroleum-based plastic production increased every year before the coronavirus disease (COVID) crisis in 2020 (Plastics, 2021). Even if recycling facilities and energy recovery are progressing and decreasing plastic landfills, 6.9 million tons of plastics remained in landfills in 2020 (Plastics Europe, 2021) in member states and candidate countries of the European Union (EU27 + 3). Moreover, Geyer et al. (2017) estimated that 60% of all time-produced plastics were accumulating in landfills and oceans. During the past decades, researchers demonstrated the ecological impact of this pollutant that killed high numbers of marine organisms situated at higher trophic levels, such as fishes (Romeo et al., 2015), seabirds (Tanaka et al., 2013), or marine mammals (Laist, 1997; Lusher, 2015). At present, the main solution proposed and applied to avoid this worldwide pollution is the replacement of some single-use nonbiodegradable plastics with biodegradable ones such as polybutylene adipate terephthalate (PBAT) or polylactic acid (PLA), whose durability in the environment is considered limited. However, the global assessment of the ecotoxicological impacts of these emerging bioplastics on marine ecosystems was not deeply documented (Ali et al., 2023).
When entering the seas, plastic debris is subject to physical and mechanical degradation, including photo-degradation, oxidation, abrasion (Andrady, 2011), and biological fouling in less than 24 h (Sheavly and Register, 2007). These phenomena lead to the fragmentation of plastic debris into microplastics and nanoplastics (Peng et al., 2020). Microplastics (MPs) (<5 mm) represent the hidden part and majority of plastic pollution (Lindeque et al., 2020). Apart from the degradation of larger plastic debris, sources of MPs also include synthetic textile fibers unleashed from our laundry (Napper and Thompson, 2016) and cosmetic products such as microbeads in facial cleansers (Napper et al., 2015). Approximately 6% to 12% of MPs are not captured in wastewater treatment plants and are released into the natural aquatic environments (Iyare et al., 2020), with the sea as the final destination. Because of their properties (e.g., small size, abundance, variety of density, etc.), MPs may affect a broad range of organisms from lower trophic levels such as phytoplankton (Li S. et al., 2020) and copepods (Cole et al., 2015; Thery et al., 2022) to higher trophic levels such as blue mussel Mytilus edulis (Von Moos et al., 2012; Li L.-L. et al., 2020) or even humans through seafood consumed (Wright et al., 2013). In copepods, studies have demonstrated that ingestion of MPs can induce oxidative stress (Jeong et al., 2017) or modify proteome (Zhang et al., 2019), leading to lower survival and fecundity rate (Lee et al., 2013; Cole et al., 2015). When ingested and then excreted by copepods, MPs can modify the solidity, volume, and density of fecal pellets (Cole et al., 2016; Coppock et al., 2019). The fate of fecal pellets in the natural environment is driven by sedimentation speed, generally leading them to the bottom of the water column. Changes in properties in fecal pellets may affect this natural mechanism and further have an influence on carbon and nutrient cycles (Cole et al., 2016).
Previous studies have shown that MPs can be colonized by their first bacteria community only a few hours after entering the marine environment (Sheavly and Register, 2007; Dang et al., 2008; Lobelle and Cunliffe, 2011; Jacquin et al., 2019), and the community forms into a biofilm format (Oberbeckmann et al., 2015) that was named as “plastisphere” (Zettler et al., 2013). MPs offer support and protection for microorganisms in the plastisphere (Muthukumar et al., 2011), including potential pathogenic species such as Vibrio sp. (Zettler et al., 2013). Therefore, ingestion of such “plastisphere-carrying” MPs may introduce foreign microbial communities into animals and have impacts on their microbiota. Microbiota play a key role in maintaining the health of hosts (Man et al., 2017), and disequilibrated microbiota can lead to a higher susceptibility to diseases (de Steenhuijsen Piters et al., 2022). Recently, researchers started to investigate the effects of MPs on the microbiota of animals’ guts and feces. Studies showed that MP exposure significantly contributed to the decrease of bacterial families involved in nitrogen cycling and organic matter decomposition in oligochaete gut (Zhu et al., 2018) and induced dysbiosis in zebrafish intestine (Qiao et al., 2019). MP exposure also affected bacterial richness and diversity in mice gut and feces microbiota (Lu et al., 2018) and altered microbiota in blue mussels, which may lead to an increasing abundance of potential human pathogens (Li L.-L. et al., 2020). Complementary to these findings, our study differs by considering both biodegradable and nonbiodegradable MPs and their impacts on copepod microbiota throughout a multigenerational experiment. Altogether, we can hypothesize that MP ingestion, especially MPs with plastispheres, may induce dysbiosis in the microbiota of copepods that could further affect the life traits of individuals.
Copepods are considered a model organism in ecotoxicology (Kwok et al., 2015) as they represent a widely distributed group in all aquatic ecosystems (Turner, 2004), and some species can be cultured in the laboratory. Previous studies have demonstrated acute and sublethal toxicity in copepods with a short-term exposure of a few days at high concentrations of MPs (Lee et al., 2013; Cole et al., 2015). Considering the relatively short life cycle of copepods, the effects of long-term MP exposures at environmentally realistic concentrations should be investigated using a multigenerational approach. For example, Zhang et al. (2019) have studied the multigenerational effects of polystyrene beads on the marine copepod Tigriopus japonicus proteome plasticity for three generations (two exposed to MPs and one recovered). The copepod species of interest in this paper, Eurytemora affinis, has been previously studied for multigenerational effects of natural factors such as temperature and salinity (Souissi et al., 2021) and effects of pollutants such as trace metals and contaminated sediments (Das et al., 2020; Das et al., 2022). However, studies regarding the microbiota of copepods are relatively rare. Most existing studies were focused on the specific relationship between copepods and Vibrio sp. (Heidelberg et al., 2002; Huq et al., 2005).
In this study, we monitored the effects on Eurytemora affinis microbiota for several generations during chronic MP exposure at an environmentally realistic concentration. Two types of MPs, biodegradable plastics (PBAT) and nonbiodegradable plastics (LDPE), were selected for the exposure experiment. In addition, both untreated “virgin” and pretreated “weathered” forms of MPs were used for investigating potential impacts on copepod microbiota not only by MP itself but also by microorganisms (may include potential pathogens) that were attached to MPs during the “weathering”. High-throughput sequencing of the small subunit of ribosome gene (16S rDNA) was employed to analyze the impacts of MP exposure on copepod microbial diversity. To our knowledge, this is the first study investigating the effects of MP exposure on copepod microbiota in several generations.
Materials and methods
Biological material
Cultures of the model organism E. affinis used for this study originated from a sampling of wild copepods in September 2014 from the oligohaline zone of the Seine Estuary (Michalec et al., 2017; Das et al., 2020). A specific protocol with controlled conditions (salinity at 15 PSU, constant temperature at 19°C, and 12:12 photoperiod) was developed (Souissi et al., 2016) to maintain the mass culture and provide standardized individuals for ecotoxicological studies (Das et al., 2020). For this experiment, a specific 300 L copepod culture, generously fed with Rhodomonas sp., was started 1 month before the start of the experiment to ensure the acclimation of copepods.
Microplastics
For this study, PBAT was selected to represent biodegradable plastics and LDPE for nonbiodegradable plastics. Pellets of LDPE (product code: BM50, EXXONMOBIL™, Dallas, USA) and PBAT for (product code: Ecoflex F blend C 1200, distributed by B-Plast 2000, BASF, Ludwigshafen, Germany) were cryogenically ground (CRYOMILL, RETSCH®, Hann, Germany) into MPs in a size range of 1–10 µm because copepods were previously shown to ingest MPs < 10 µm (Thery et al., 2022). The size range of each MP sample was confirmed by Dynamic Light Scattering (Mastersizer 2000, Malvern Panalytical, Malvern, UK). MPs were prepared individually for each exposure replicates in sterilized 10 mL pill jars (540 µg MPs per jar). To prepare weathered (biologically fouled) MPs, 540 µg of MPs were mixed with 10 mL of 15 PSU water (25 µm filtered seawater diluted with deionized water to 15 PSU) in pill jars and incubated at 19°C with a 12:12 photoperiod on a rotary shaker at 200 rpm for 45 days. Each jar was covered with a drilled top to allow air exchange and prevent contamination/evaporation.
Experimental approach
During four successive generations, copepods were exposed to five conditions: control (no MPs), virgin PBAT, weathered PBAT, virgin LDPE, and weathered LDPE (each in triplicate), followed by the fifth generation without MP exposure for detoxification. For each replicate, copepods were maintained in a 2-L beaker filled with 1.8 L of 15 PSU water with constant airflow through a glass pipette. During the five generations, copepods were fed every 2 days with 10 mL of Rhodomonas sp. microalgae (Dayras et al., 2021). Previously established methods (Souissi et al., 2010; Souissi et al., 2016) were followed for preparing microalgae cultures and feeding copepods.
In order to have copepods in synchronized lifecycle stages, a specific protocol was followed (Das et al., 2020). To start each generation, every beaker was filled with a laying nest and 1.8 L of freshly prepared 15 PSU water, followed by 30 ovigerous females from the mass culture. After 2–3 days, eggs that have been released by females will have passed through the 200-µm mesh of the laying nest. At this moment, non-ovigerous females were removed with laying nests, and then MP samples were added according to respective conditions (final concentration 300 µg/L). This final concentration would be considered environmentally realistic according to a previous review (Paul-Pont et al., 2018) on the presence and concentration of MPs in different seawater surveys. After hatching and the development of larval stages into adults (10–15 days), copepods of each replicate were harvested with a 33-µm mesh. To start the next generation, 30 ovigerous females were selected from each harvest and added into their respective beakers after 15 PSU water renewal. The remaining copepods were briefly fixed with 70% ethanol and abundantly rinsed with sterilized MilliQ water.
DNA extraction, 16S rDNA library preparation, sequencing, and sequence processing
A total of 50 adult individuals (including males, females, and ovigerous females) were collected randomly and stored in a 1.5-mL microtube at −20°C until microbiota DNA extraction. At the end of each generation, after copepods were harvested, water samples of each beaker were filtered through 0.22 µm Sterivex filter units (Millipore, Burlington, MA, USA), and filters were stored at −20°C. In addition, the microalgae cultures for copepod feeding were also sampled during the multigenerational exposure (200 µL per filter, one time per generation from generations one to four) using Sterivex filters and then stored at −20°C. Before DNA extraction, copepods were first crushed and homogenized using sterile single-use micropestles. For water and microalgae samples, filters were cut out of Sterivex units and placed into 1.5 mL microtubes. For all samples, total DNA was extracted using the QIAamp PowerFecal Pro DNA Kit (Qiagen, Hilden, Germany), following the manufacturer’s instructions. The 16S amplicon library was prepared by amplification of the V3–V4 region of the bacterial 16S ribosomal RNA gene using primers 341F (5′-CCTACGGGNGGCWGCAG-3′) and 805R (5′-GACTACHVGGGTATCTAATCC-3′). Phusion™ High-Fidelity DNA Polymerase (Thermo Fisher Scientific, Waltham, MA, USA) was used for polymerase chain reaction (PCR) following the manufacturer’s instructions. PCR products were examined on 1% agarose gel electrophoresis, and the purification protocol was achieved with the Nucleospin® Gel and PCR Clean-up (MACHEREY-NAGEL GmbH & Co. KG, Düren, Germany). The quantification of extracted DNA was performed using the Qubit™ dsDNA HS Assay Kit (Thermo Fisher Scientific, Waltham, MA, USA) and the Qubit® 2.0 Fluorometer (Thermo Fisher Scientific, Waltham, MA, USA). Second PCR and sequencing were achieved by Eurofins Genomics (Germany GmbH). Finally, sequence processing was completed following methods used in a previous publication of the group (Li L.-L. et al., 2020). Briefly, rDNA sequences were analyzed following the standard procedure (https://mothur.org/wiki/miseq_sop/) (Schloss et al., 2011) of the MOTHUR program v1.47.0 (Kozich et al., 2013). First, sequences were extracted and sorted based on their index tag, and then processed for quality filtering (e.g., discarding suspected chimeras, single singletons, and erroneous sequences). The remaining high-quality reads were clustered into operational taxonomic units (OTUs) at a 97% similarity threshold (Behnke et al., 2011). After normalization of the dataset, OTUs were searched against the SILVA database (release 138, http://www.arb-silva.de/) (Quast et al., 2012) using BLASTN (Altschul et al., 1990) for taxonomic affiliation. Sequencing data have been submitted to the NCBI sequence read archive database (SRA accession: PRJNA980077).
Statistical analyses and graphical representations
Dissimilarity indices of microbial assemblages were computed using the Bray–Curtis method as a dissimilarity index with square root OTU read abundance normalization using R studio with the “vegan” package (Dixon, 2003). Hierarchical cluster analysis was performed with the Ward method using the “Cluster” package (Maechler et al., 2013). Results were then plotted in a dendrogram using “factoextra” (Kassambara and Mundt, 2017) and “RcolorBrewer” (Neuwirth and Neuwirth, 2011) packages. Nonmetric multidimensional scaling (NMDS) and permutational analysis of variance (PERMANOVA-Adonis) were performed using the vegan package (Dixon, 2003). Post-hoc Adonis tests were achieved using the “pairwiseAdonis” package (Teng et al., 2018). Alpha-diversity estimators (diversity—Simpson and Shannon; richness—Chao 1; and dominance—Equitability) were calculated using the Past 4.03 software (Hammer et al., 2001) for each replicate of each treatment. Values were averaged for each treatment and distribution represented in boxplots using the “ggplot2” package. Possible significant differences in alpha-diversity estimators between conditions of each generation were evaluated with one-way ANOVA or Kruskal–Wallis one-way analysis of variance, depending on results of normality and homoscedasticity of variances with R software. A p-value under 0.05 was considered statistically significant.
Results
16S rRNA sequences analysis
Before quality filtering and normalization across samples, a total of 4,053,284 reads were obtained. After normalization and exclusion of singletons, a total of 264178 high-quality reads (10,567 ± 3,938 reads per sample) corresponding to 7,540 OTUs were identified for the microbiota of copepods during the multigeneration exposition. In water samples collected at the end of each generation, 2,532 OTUs (710 ± 171 OTUs per sample) were found, while only 395 OTUs (176 ± 34 OTUs per sample) were detected in the copepod feeding media composed of microalgae Rhodomonas sp. Across the five generations, the microbiota of copepods in the control condition was composed of 994 ± 203 OTUs (Figure 1). Respectively, the microbiota of copepods shared 113 ± 32 OTUs with the microbiota of water samples (representing 6.7% of copepod microbiota) and 47 ± 21 OTUs with the microbiota of microalgae feedings (2.85%) (Figure 1). Throughout the five generations, the lists of OTUs shared among the three matrices stay similar.
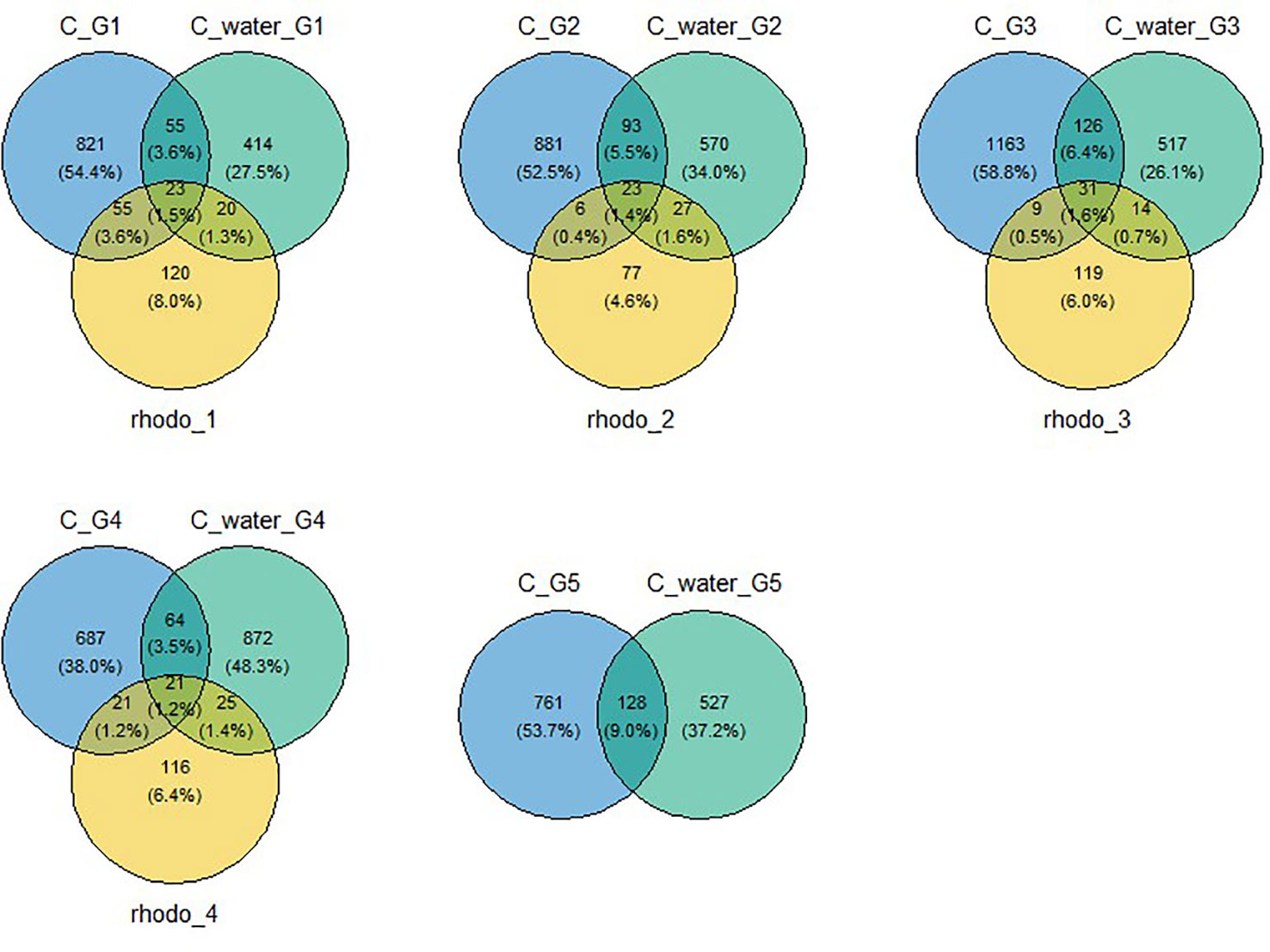
Figure 1 Venn diagram of OTUs shared among the microbiota of copepods in control, water, and algae used to feed copepods during the five-generation experiment. Algae was not sampled during the last generation. C, control; C_water, water used to start generation; rhodo, Rhodomonas sp. Algae used to feed copepods; G1, generation one; G2, generation two; G3, generation three; G4, generation four; G5, generation five (depuration).
Effects of microplastics on copepod microbiota alpha-diversity
The alpha-diversity estimator showed no significant differences in bacterial communities among treatments across the five generations for Simpson, Shannon, and Equitability indices (Figure 2). Two significant differences (p = 0.037) for Chao 1 indices were detected during generation two (G2) between control (T) and weathered PBAT (PBATw) (p = 0.05) and between virgin LDPE (PE) and weathered PBAT (PBATw) (p = 0.05).
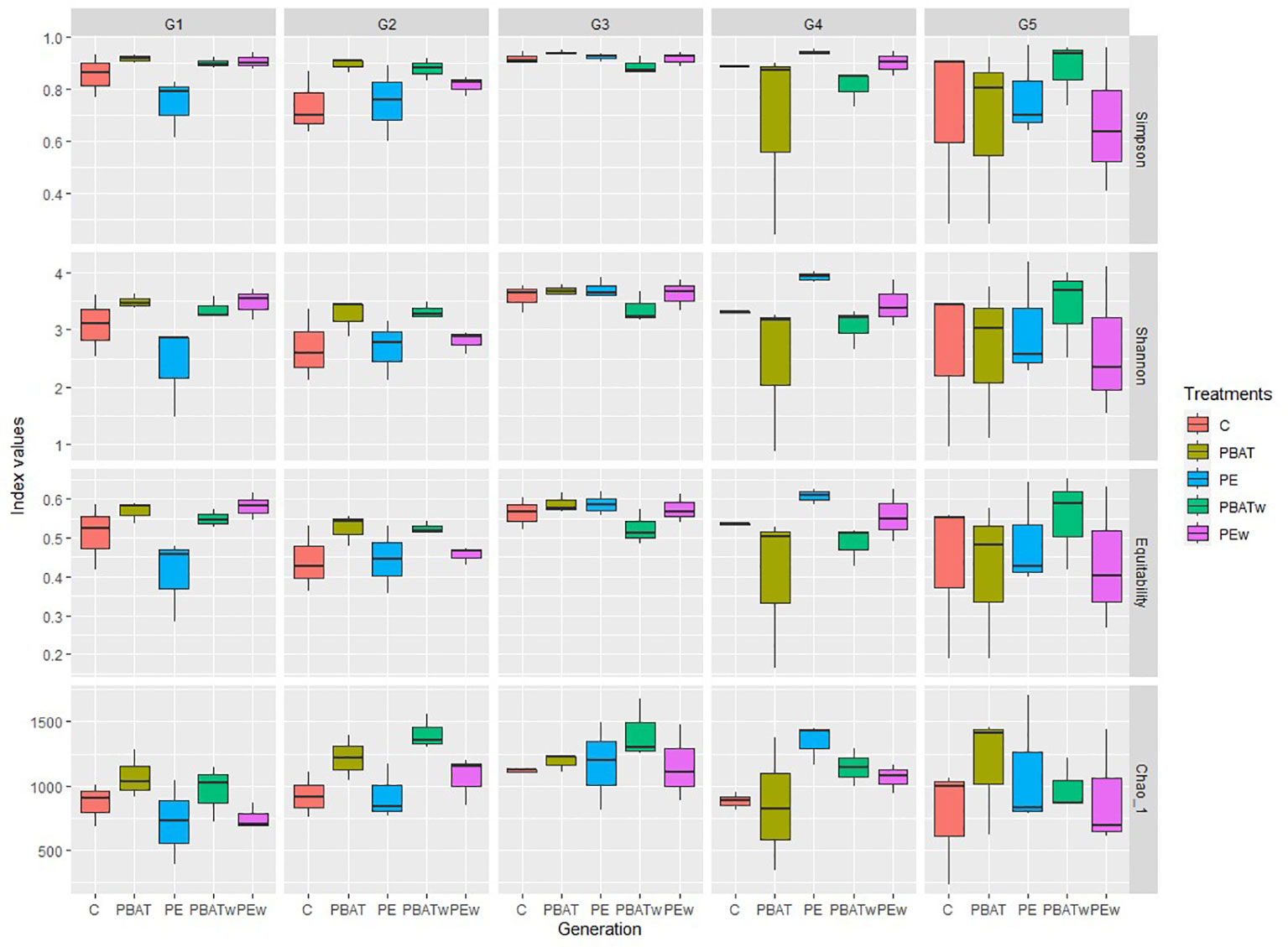
Figure 2 Indices for alpha-diversity of taxa. Boxplots show mean and standard deviation values of the diversity (Simpson and Shannon), richness (Chao 1), and dominance (Equitability) estimators for copepod microbiota among treatments and along the four MP exposure generations (G1–G4) and depuration generation (G5). Each box corresponds to a treatment with control (C), virgin MPs of PBAT (PBAT), virgin MPs of LDPE (PE), weathered MPs of PBAT (PBATw), and weathered MPs of LDPE (PEw).
Bacterial assemblage composition along copepod generations
Taxonomic classification of the 16S rRNA amplicon sequences identified 55 orders of bacteria in the copepod microbiota during the five generations of the experiment. Rhodobacterales, Pseudomonadales, Enterobacterales, and Rhizobiales were the top four most abundant orders present in all conditions during all experiments and represented respectively 29.6%, 15%, 11.1%, and 8%. Flavobacteriales were abundant during the first generation for all treatments but decreased in the control” and “PE” treatments during the second generation. They also decreased in the third generation in the “PBAT” and “PBATv” treatments but stayed abundant in the “PEv” treatment (Figure 3). During generations four and five, the abundance of this order was low in all samples except for the PBAT treatment in generation four, which had an increase. In the class of Bacilli, results showed an increase in thermoactinomycetales during the second and third generations and then a decrease during the two last generations. Bacillales were also relatively abundant during the third generation (Figure 3).
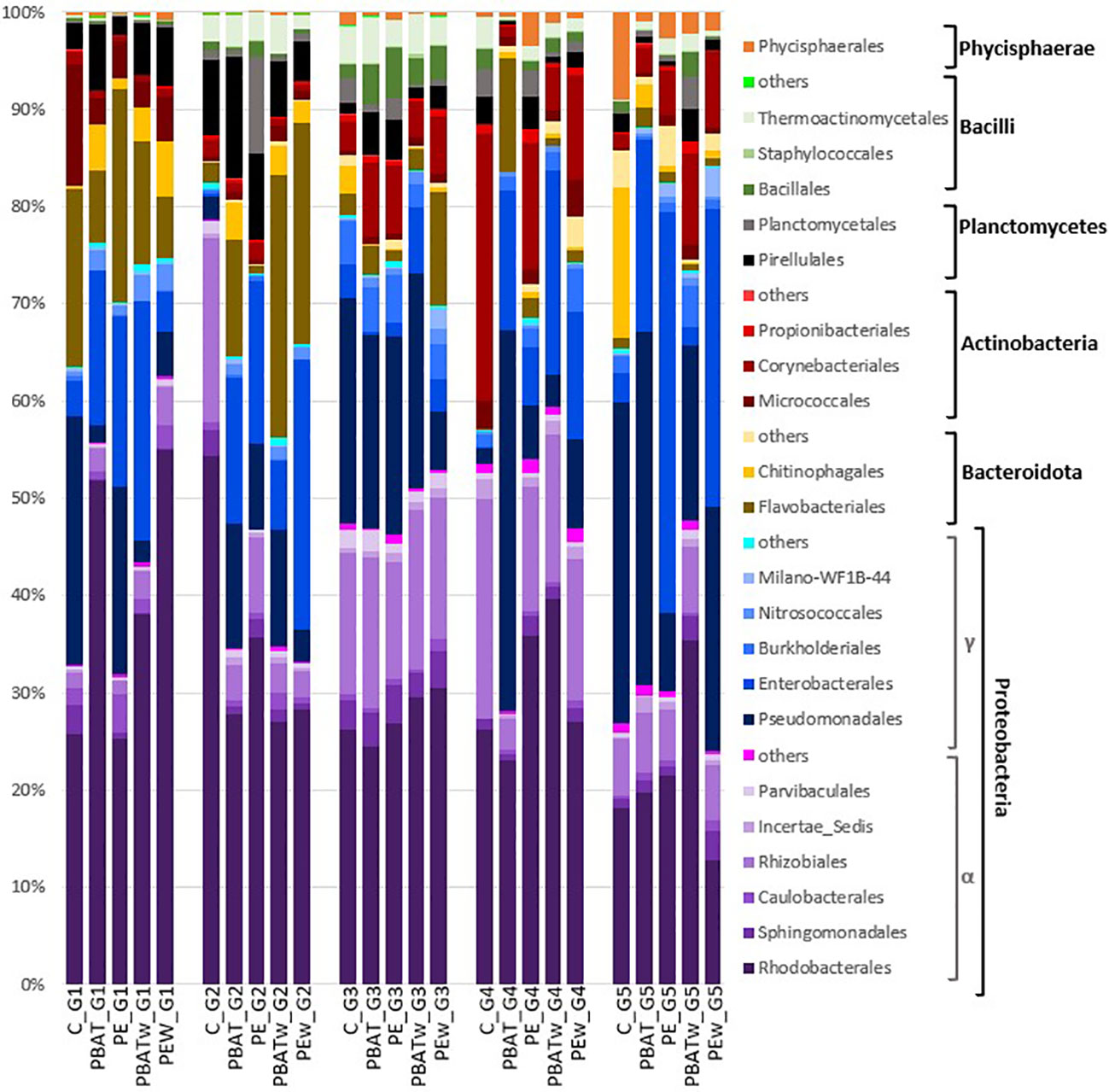
Figure 3 Microbial community composition of copepod microbiota at the order level. Taxa were grouped by class and then by phylum. Proteobacteria was presented in shades of purple for Alphaproteobacteria class and in shades of blue for Gammaproteobacteria; Bacteroidota in shades of brown; Actinobacteria in shades of red; Planctomycetes in black and grey; Bacilli in shades of green; and Phycisphareae in orange. Only classes for which abundance was superior to 1% of total OTU reads were represented (total recovery of 97.6%). C, control; PBAT, virgin PBAT; PE, virgin LDPE; PBATw, weathered PBAT; PEw, weathered LDPE; G1, generation one; G2, generation two; G3, generation three; G4, generation four; G5, generation five (depuration).
In the cultured water that was used to start each generation of copepods, bacterial communities showed differences at the order level during the five generations. Pseudomonadales, Flavobacteriales, Rhodobacterales, and Burkhoderiales were the main order present in the first generation. During the second generation, the abundance of the abovementioned orders globally decreased and the abundance of Enterobacterales highly increased. During the third generation, Enterobacterales decreased while Pseudomonadales, Milano-WF1B-44, and Rhodobacterales increased. Some orders such as Verrucomicrobiales, Cytophagales, and Balneolales appeared at higher abundance (Supplementary Material 1) compared to the first two generations. During generations four and five, mainly Kordiimonadales and Milano-WF1B-44 increased and were the two most abundant orders in generation five. On the other hand, Balneolales and Rhodobacterales decreased. Bacterial communities present in the copepod feeding media (Rhodomonas sp.) were relatively stable, and mainly composed of orders of Pseudomonadales, Rhodobacterales, Flavobacteriales, and Chitinophagales (Supplementary Material 1).
Results of the hierarchical cluster analysis (based on the Bray–Curtis distances) have shown that copepod microbiota were generally clustered according to their generations (Figure 4). On the dendrogram, treatments of all five generations could be divided into three groups: generations one and two, generations three and four, and depuration generation five (Figure 4). The bacterial communities of the two first generations had a closer Bray–Curtis distance with the depuration generation (generation five) compared to generations three and four with generation five (Figure 4). Within treatments of the same MP types (PBAT or LDPE), virgin and weathered samples generally had closer Bray–Curtis distances for the same generation (Figure 4). In addition, we noticed that the virgin PBAT-treated copepods in the depuration generation were placed in the first group with most of the generation one and two samples.
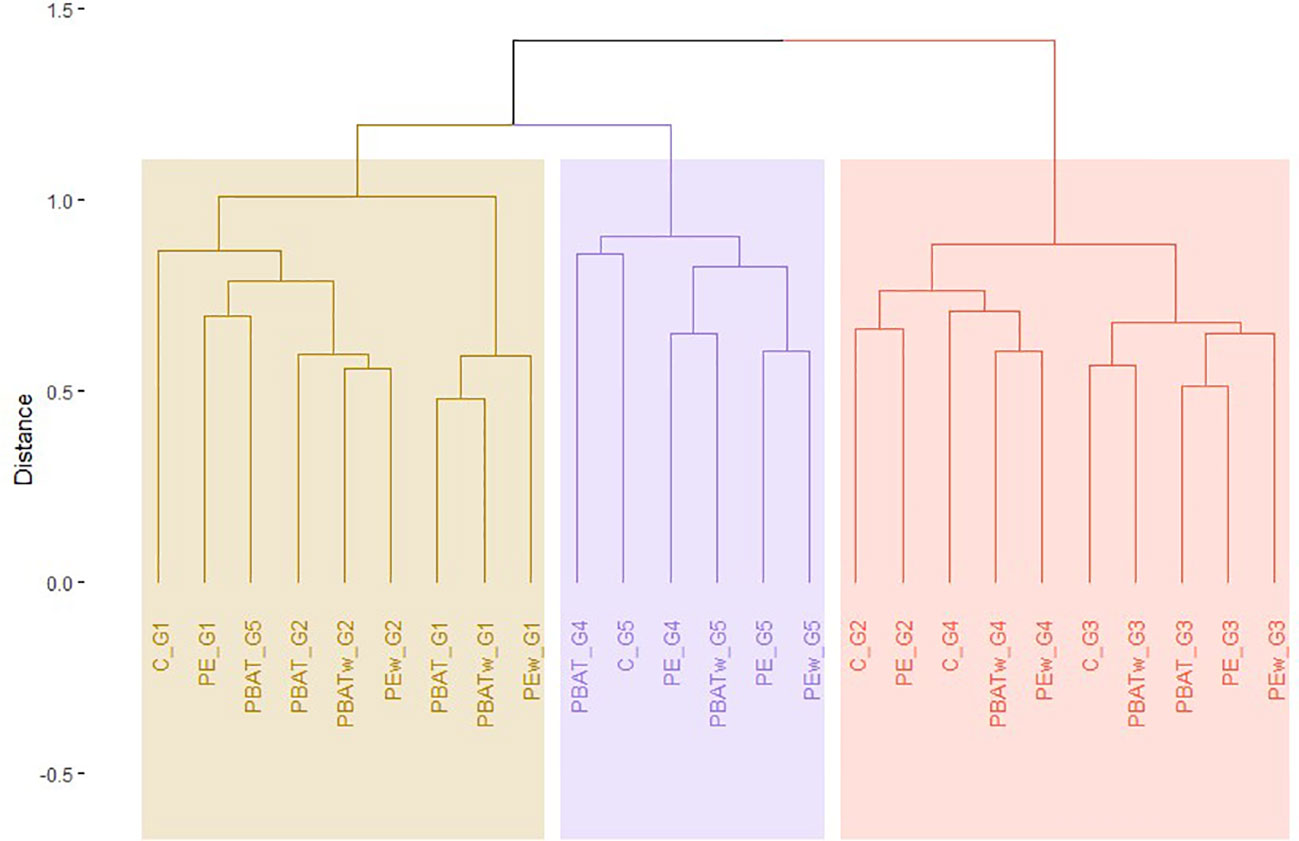
Figure 4 Hierarchical clustering of different treatments along the five generations of experiment (1–4, contaminated generations; 5, depuration generation) of copepod microbiota based on the Bray–Curtis dissimilarities calculated on square root transformed number of OTU reads. The colored rectangles indicate arbitrary cluster separation. T, control; PBAT, virgin PBAT; PE, virgin LDPE; PBATv, weathered PBAT; PEv, weathered LDPE; G1, generation one; G2, generation two; G3, generation three; G4, generation four; G5, generation five (depuration).
In order to better understand the dispersion of different groups based on bacterial communities, an nMDS plot was carried out to analyze microbiota data from copepods, water used for copepods, and microalgae used as food (Figure 5). Three groups appeared on this plot: the first group includes water samples used to start each generation (orange circle); the second group includes the four Rhodomonas sp. samples for feeding (black circle), and the third group includes samples corresponding to copepod microbiota with slight differences according to generations (Figure 5). These results agreed with the Venn diagram result (Figure 1) and suggested copepods carrying their specific microbiota, as copepod microbiota only shared a mean value of 6.7% and 2.9% OTUs with the water samples and the Rhodomonas sp. Feeds, respectively.
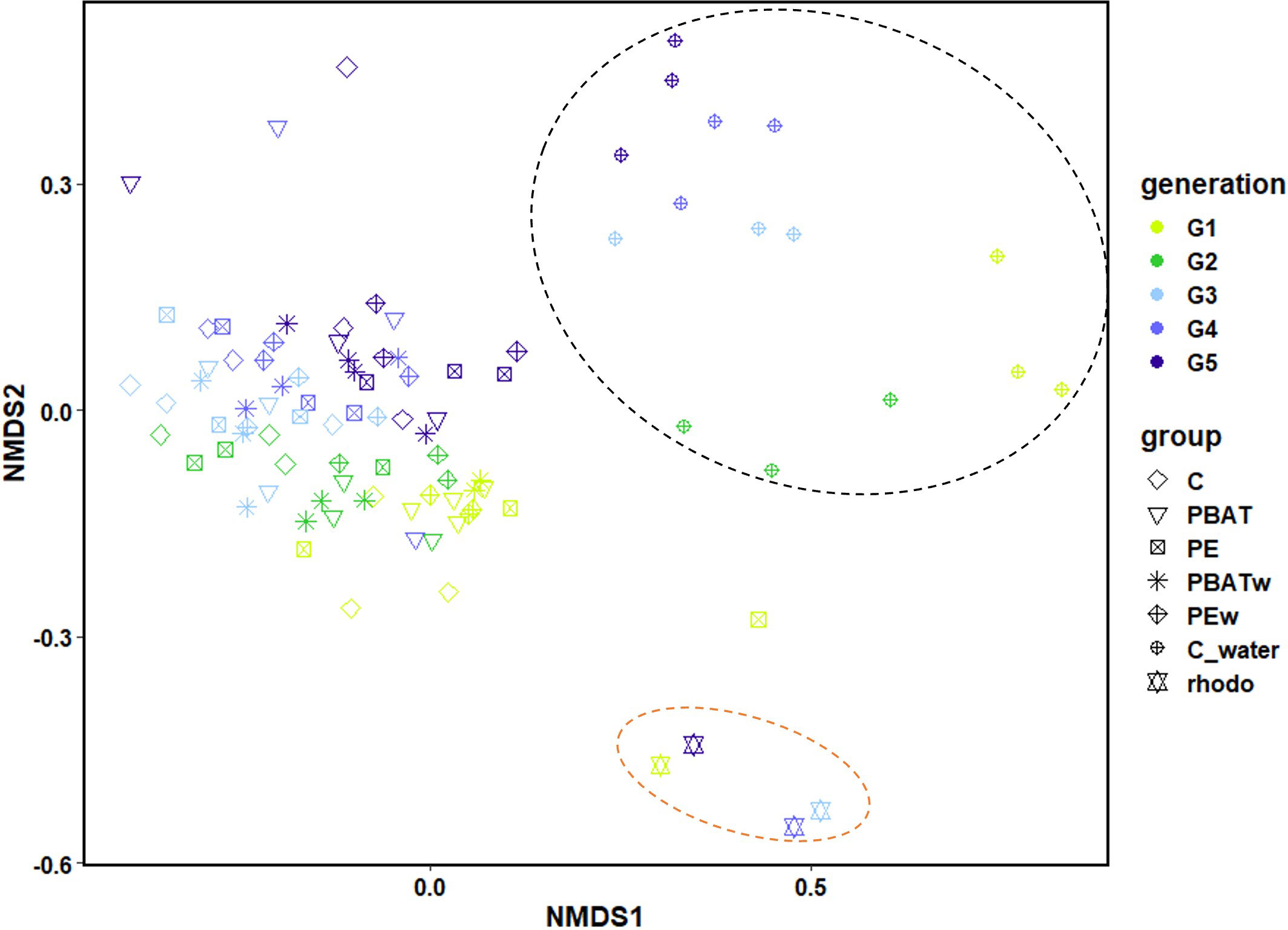
Figure 5 Nonmetric multidimensional scaling (NMDS) on the copepod microbiota communities, water used for the experiment for each generation of copepods, and algae used for feeding copepods. The analysis was performed during the four-generation MP exposure and the last (fifth) generation of depuration. The colors of the symbols correspond to the different generations of copepods, and the shapes of the symbols correspond to the different treatments. Colored circles were arbitrarily placed to represent the different groups: the black circle represent samples of water used to start treatments of the different generations, and the orange circle is the group of Rhodomonas sp. samples. G1, generation one; G2, generation two; G3, generation three; G4, generation four; G5, generation five (depuration); C_water, control of water without copepods; C, control with copepods; PBAT, contaminated with virgin PBAT MPs; PE, contaminated with virgin LDPE MPs; PBATw, contaminated with weathered PBAT MPs; LDPEw, contaminated with weathered LDPE MPs.
Effects of MPs on copepod microbiota beta-diversity
Results of nMDS analyses coupled with PERMANOVA analysis for the four generations exposed to MPs (G1–G4) revealed that generation (p = 0.001), type of MPs (p < 0.01), and effects of generation × type of MPs (p < 0.05) significantly influenced copepod microbiota (Table 1). In order to further investigate how factors significantly influence the bacterial communities of the different treatments, a post-hoc test (pairwise ADONIS) was performed. Side-by-side comparison between “control” and PBAT showed that generation (p = 0.0001), type of MPs (p < 0.002), and effects of generation × type of MP (p < 0.001) significantly affected copepod microbiota (Table 2). Regarding control and “LDPE,” only generation (p = 0.0001) and the type of MPs (p < 0.05) had a statistically significant impact on copepod microbiota (Table 2). Between LDPE and PBAT, only generation (p = 0.0001) had a significant influence on copepod microbiota. PERMANOVA was also performed on data of the depuration generation (G5), and no significant differences were found among treatments (Table 3).
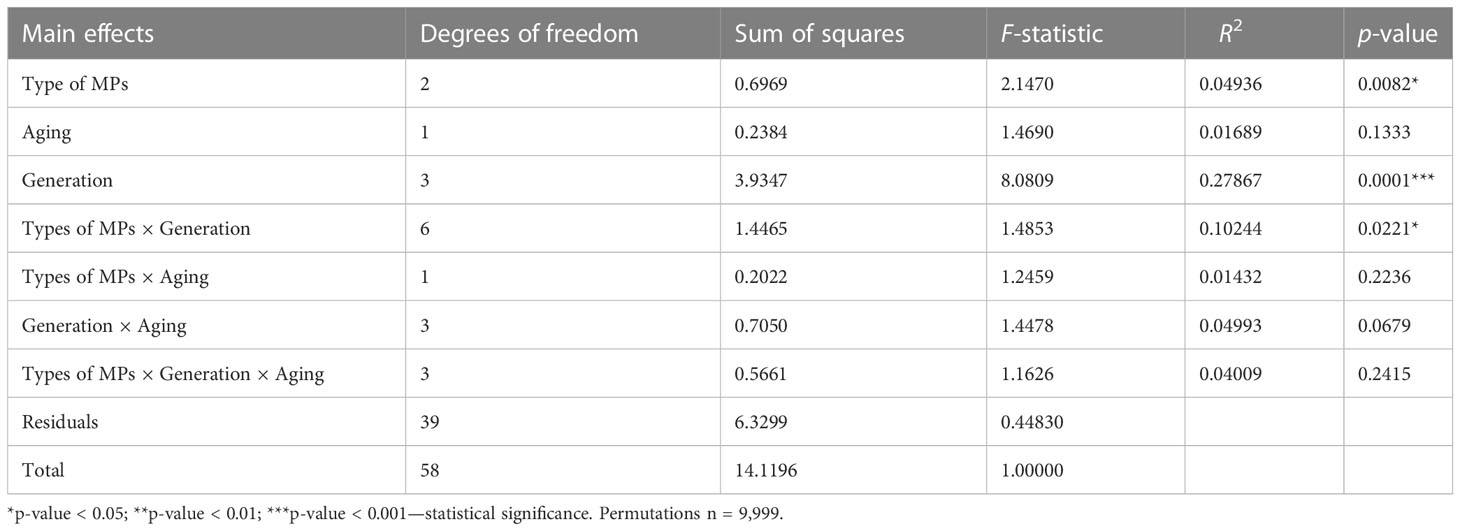
Table 1 PERMANOVA (ADONIS) results for copepod microbiota (OTUs) communities’ structures during the four generations exposed to MPs (G1–G4), calculated on the Bray–Curtis distance (significant effects of factors (p < 0.05) are indicated with asterisks).
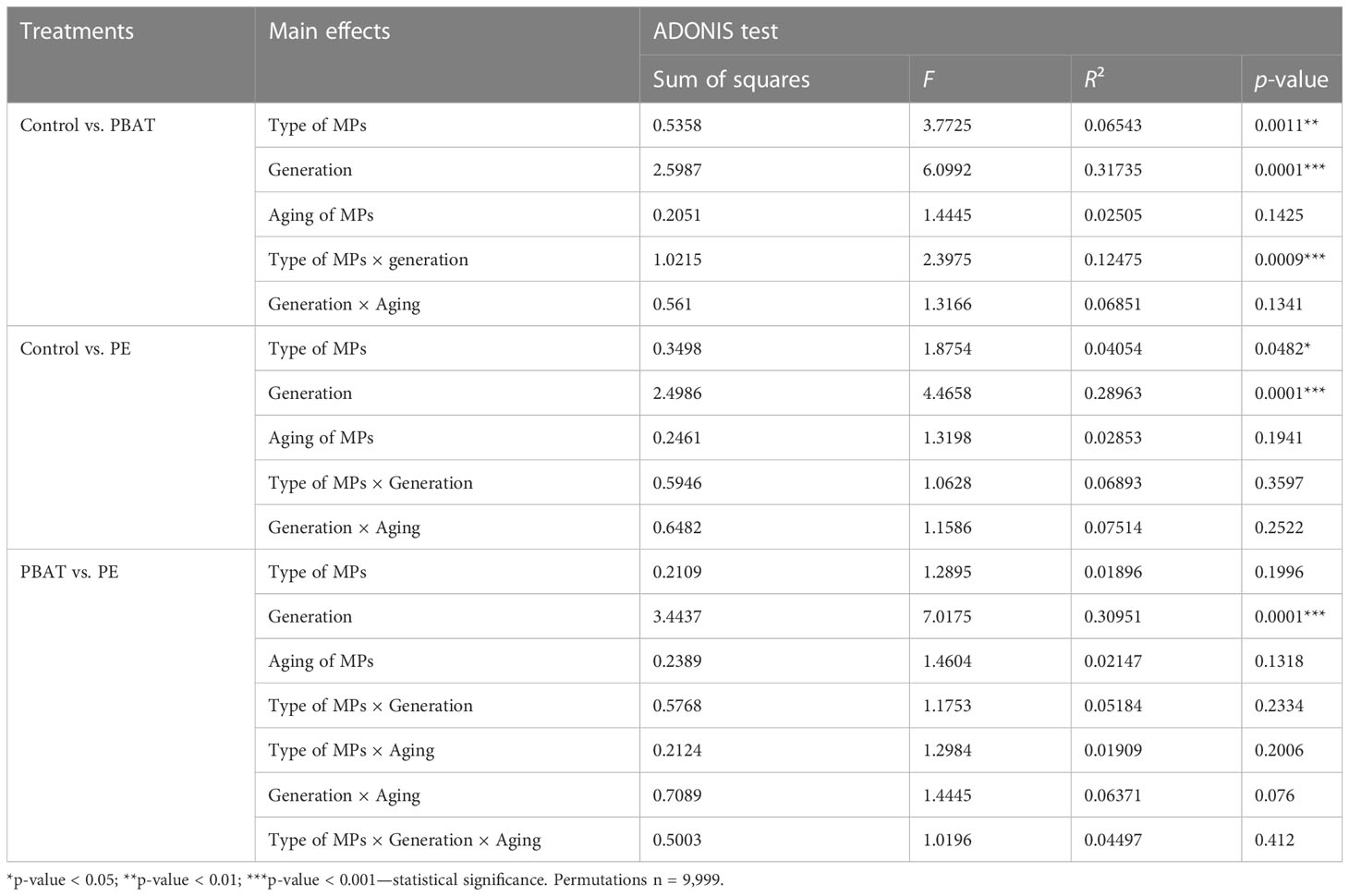
Table 2 Statistical analyses of pairwise ADONIS differences in copepod microbiota profiles between treatments during the four generations exposed to MPs (G1–G4), calculated on the Bray–Curtis dissimilarity measures (significant effects of factors (p < 0.05) are indicated with asterisks).

Table 3 PERMANOVA (ADONIS) results for copepod microbiota (OTUs) communities’ structures during the generation of depuration (G5), based on the Bray–Curtis distance (significant effects of factors (p < 0.05) are indicated with asterisks).
For each generation, sequence read count differences between the control and the different treatments were analyzed using a nonparametric Wilcoxon test. In total, 34 OTUs have shown significant differences in abundance (increase/decrease) after being exposed to MPs as compared with controls (Supplementary Material 2). At the class level, OTUs came from Alphaproteobacteria (47.1%), Gammaproteobacteria (23.5%), Actinobacteria (14.7%), Bacteroidota (8.8%), and Planctomycetes (5.9%). Alphaproteobacteria was composed of Rhodobacterales (10 OTUs), Rhizobiales (five OTUs), and Caulobacterales (one OTU). Gammaproteobacteria by Enterobacterales (five OTUs) and Legionellales, Nitrosococcales, and Pseudomonadales has one OTU for each order. Actinobacteria was composed of Micrococcales (three OTUs) and Corynebacteriales (two OTUs). Bacteroidota was composed of Flavobacteriales (two OTUs) and Cytophagales (one OTU). Pirellulales (two OTUs) was the only family found for Planctomycetes. Respectively, 12, 11, 8, 0, and 4 OTUs changed in abundance during generations one, two, three, and four, and depuration generation has more than 82% of OTUs for which abundance increased in MP treatment compared to control one (Supplementary Material 2). Only one OTU (OTU 941, “Microbacterium”) had a significant read number difference for the two treatments (weathered PBAT and weathered LDPE) in generation one. Notably, OTUs affected by MP treatments, as detected by the Wilcoxon test, were mostly present in low read counts (≤ 3). Treatment with weathered PBAT in generation one was shown to have the highest effect at the OTU level, with eight OTUs that differed in read counts (seven OTUs increased and one decreased) after MP exposure. Overall, weathered MPs (i.e., weathered PBAT and LDPE) treatment seems to have the highest effect on OTU read abundance compared to control. Finally, the Wilcoxon test identified one OTU (OTU 2602 – Labrenzia alba) that was present in the control condition but absent in virgin PBAT and weathered LDPE treatments.
Discussions
The impact of MPs on copepods has been previously documented from the molecular scale (Heindler et al., 2017; Choi et al., 2020) to life trait alterations (Cole et al., 2015; Cole et al., 2016). However, to our knowledge, potential impacts of MPs on copepod microbiota have not been reported. Copepods represent a high proportion of zooplankton carbon biomass and are keystone between primary producers and secondary consumers in marine food webs (Turner, 2004). In addition, evidence has shown that microbiota plays an essential role in maintaining animal health (Zhang et al., 2010) and supporting the immune system (Nicholson et al., 2012). Furthermore, MPs offer support and protection for microorganisms in the “plastisphere” (Muthukumar et al., 2011), including potential pathogenic species such as Vibrio sp. (Zettler et al., 2013). Therefore, it is essential to investigate the impacts of MP exposure on copepods in all aspects, including copepod microbiota.
Previous studies have shown that MP exposure affected planarian microbiota richness with a significant increase in Simpson and Shannon indexes (Han et al., 2022) and marine medaka microbiota with a decrease in Shannon and Chao 1 index (Zhang et al., 2023). In our study, however, effects in E. affinis microbiota after MP exposure seem to be more subtle, with only a significant difference in the Chao 1 index between the control and the treatment of weathered PBAT during the second generation observed. Possible explanations could come from the fact that studies by Han et al. (2022) and Zhang et al. (2023) focused specifically on gut microbiota, which is considered more sensitive to pollutants as compared with external microbiota (Fackelmann and Sommer, 2019). Indeed, in our study, due to the small size of E. affinis and the lack of protocols for gut isolation, total DNA was extracted from each sample. Therefore, microbiota could come from both the internal (gut) and external (exoskeleton) parts of copepods, including MPs that were ingested by copepods and adhered to the external part of copepods. This hypothesis is supported by a previous study confirming the ingestion of E. affinis for MP of the same size range (Thery et al., 2022). In addition, the environmentally realistic concentration of MPs used in this study might well contribute to the observation of subtle changes. Indeed, contrasting with other studies exposing their model organisms to high MP concentrations, the environmentally realistic concentration of MPs used to expose copepods was not enough to affect their microbiota alpha-diversity estimators. Similar results were reported on the blue mussel Mytilus edulis exposed to comparable concentrations of MPs (200 µg/L), leading to no significant differences in the alpha-diversity estimator of their gut microbial diversity (Li L.-L. et al., 2020). Similar to the previous report for the blue mussel Mytilus edulis exposed to an environmentally realistic concentration of MPs, no significant differences were observed in the alpha-diversity estimator of gut microbial diversity (Li L.-L. et al., 2020).
Results of microbial community structure analyses at the OTU level suggested that copepods carried their own specific microbiota that was different from cultured water and Rhodomonas algal food supplies. However, we noticed that the community structure was shifting throughout the experiment at each copepod generation. This observation could be due to the input of “new” bacterial communities at the beginning of each generation by changing water. Indeed, our results showed water community structure evolved during the five generations with high increases/decreases in the main orders of bacteria. Moreover, even if community structures were quite stable during the experiment, few changes at the order level were observed. Regarding our experimental design, the generation factor is clearly represented by the water used for each generation and the algae media. This hypothesis is a trivial explanation of why generation was the most significant factor in the copepod microbiota community, as shown by PERMANOVA and pairwise couple comparison with the post-hoc test.
According to the results of PERMANOVA, the other factor that significantly influences copepod microbiota is exposure to MPs. This finding agreed with a previous report by Li L.-L. et al., 2020 that also identified “MP exposure” as a significant factor influencing the gut microbiota of blue mussels using PERMANOVA analysis. Our results also agreed with existing studies on mice, zebrafish, and planarians that suggested MP exposure could induce significant impacts on gut microbiota (Lu et al., 2018; Qiao et al., 2019; Han et al., 2022). In our study, results of post-hoc analysis suggested that the effects of MPs on copepod microbiota compared to a control were independent of MP types (PBAT or LDPE), as a pairwise comparison between these two treatments showed a nonsignificant difference. Interestingly, the difference between the control and the PBAT-exposed samples was greater than the difference between the control and the LDPE-exposed samples, as the p-value of “control vs. PBAT” was approximately 10 times lower. As a result, the interaction between the factor “generation” and PBAT was also identified to have a significant effect on microbial community structure.
To investigate possible reasons for the greater effect on copepod microbiota of PBAT exposure than of LDPE exposure, we analyzed the biofilm communities attached to PBAT and LDPE. The preliminary (observational) results suggested no differences in bacterial communities between the two MP types that were weathered up to one hundred twenty days (data not shown). A similar observation was reported by Delacuvellerie et al. (2021) that showed no differences in bacterial communities between PBAT and LDPE after 82 days of immersion in the Mediterranean Sea. This result may be further explored and the untreated data can provide future perspectives of this study. Possible reasons for observing a greater effect of PBAT exposure might involve the interaction between copepods and PBAT. For example, as biodegradable plastics, we cannot rule out the possibility that PBAT MPs could be degraded (even partially) by the digestive enzymes of copepods after ingestion. Products of digested/degraded PBAT may further influence the gut microbiota of copepods. Altogether, we can suggest that both PBAT and LDPE MPs could potentially impact copepod microbiota in a natural environment with possible effects on life traits, even though PBAT is generally considered as more “eco-friendly.” On the opposite, a recent study on marine medaka (Oryzias melastigma) suggested that polylactic acid (PLA), a compostable polymer, was less hazardous compared to “classic” plastics (i.e., polyethylene terephthalate and polystyrene) regarding effects on alpha-diversity indexes (Shannon and Chao 1) and complexity of networks of gut microbiota (Zhang et al., 2023).
According to the results of PERMANOVA, no significant difference was observed in the microbiota structures of copepods between virgin and weathered MP exposure. This is surprising since the result of diversity analysis suggested a significant difference between the bacterial community on virgin MPs compared to 45 days weathered MPs (data not shown). This result is in contrast with a previous report by Li L.-L. et al., 2020 that showed significantly greater impacts by weathered MPs on blue mussel gut microbiota than by virgin MPs. A possible explanation for such a difference in observation could be in the experimental design. For Li L.-L. et al., 2020, culture tanks for mussels were cleaned every other day, refilled with fresh seawater, and supplied with fresh virgin or weathered MPs. For copepod cultures in this study, water and MPs were refreshed at the beginning of each generation. Therefore, during each generation (10–15 days), virgin MPs could have biofilms develop and become weathered MPs. Finally, the PERMANOVA result of the depurated generation suggested no statistical differences between the control and previously MP-exposed samples. This result implied a relatively quick recovery of copepod microbiota even after several generations of exposure to MPs.
According to our results of taxonomy analysis, abundant bacterial phyla found in copepod microbiota (Proteobacteria, Bacteroidota, and Actinobacteria) were similar to those found in other copepods living in natural environments of the North Sea and the Atlantic Ocean (Brandt et al., 2010; Cregeen, 2016). Considering this taxonomic level, we were not able to find differences between exposed and control copepod microbiota. At a higher taxonomic resolution, the results of the Wilcoxon test suggested 34 OTUs were significantly (p < 0.05) impacted by MP exposure. Generally, the number of impacted OTUs seemed to be slowly decreasing from the first to the third generation and was close to zero for the two last generations. Among these 34 OTUs, OTU 941 (“Microbacterium”) was identified both in weathered PBAT and weathered LDPE during the first generation. It should be noted that OTU 941, as well as all others within these 34 OTUs, were represented by low read counts (≤ 3 read counts). Similarly, we found in generation three that OTU 2602 (Labrenzia alba) was absent in virgin PBAT and weathered LDPE compared to control. Although this OTU was only present in low read numbers, it is possible that MP impaired the symbiotic relationship between copepods and Labrenzia alba, as it was shown that the genome of this species possesses symbiosis factors between microbes and marine invertebrate hosts (Couceiro et al., 2021). However, even if the Wilcoxon test revealed several significant OTUs impacted by MP exposure, since these OTUs were only supported by low read numbers, we suggest considering these findings with caution.
It is worth mentioning that two Vibrio species (Vibrio splendidus and Vibrio tasmaniensis) were detected in one replica of virgin PBAT-exposed copepods for the second, third, and fourth generations. Both Vibrio species are known mollusc pathogens: Vibrio tasmaniensis has been reported to correlate to mass mortality events in cultured oysters (Roux et al., 2002; Lopez-Joven et al., 2018), while Vibrio splendidus was detected in cleaner fish used for removing sea lice from salmon in salmon farms (Gulla et al., 2015). Special relationships between chitinous exoskeleton zooplankton such as copepods and the Vibrio genus were previously described as well (Tamplin et al., 1990; Heidelberg et al., 2002; Brandt et al., 2010). Nevertheless, the two Vibrio species were detected in only one replica in generations two to four but were not detected in the following depurated generation or water samples used to start each generation.
Conclusion
Altogether, our results suggest that MPs impact copepod microbiota rapidly (from the first generation) and continuously (until the last exposed generation) despite plastic origin, types, and aging conditions. Thus, the biodegradable polymer PBAT might not be an ideal alternative for replacing nonbiodegradable plastics considering similar impacts on copepod microbiota (and possibly on microbiota of other organisms). It would be interesting to compare other MPs, both biodegradable and nonbiodegradable, for their impacts on the microbiota of copepod and other organisms. Our study also showed that copepod microbiota returned promptly to their original composition as soon as MP exposition stopped. This result, which needs to be confirmed by further studies on a wider range of organisms, brings some hope for the resilience of organisms and restoring the ecosystem by preventing plastics from entering the environment.
Data availability statement
The datasets presented in this study can be found in online repositories. The names of the repository/repositories and accession number(s) can be found below: NCBI Trace Sequence Archive NCBI under BioProject accession number PRJNA980077.
Author contributions
JT and L-LL wrote the manuscript. JT conceived and performed the experiments. L-LL and SM helped with molecular biology protocols and analysis. DD contributed to the microplastic exposure experiment. SM, L-LL, SS, SB, and J-MR helped in drafting the manuscript and approved the current version. All authors contributed to the article and approved the submitted version.
Funding
This study is a part of the Ph.D. thesis of JT from the University of Mons and the University of Littoral Côte d’Opale, funded in equal parts by the two universities. This work has been financially supported by the French State and the French Region Hauts-de-France, in the framework of the project CPER IDEAL 2021-2029. Samira Benali acknowledge supports by the European Community (FEDER) for general support in the frame of LCFM-BIOMAT. Jean-Marie Raquez is a FRS-FNRS Research Associate.
Acknowledgments
This is a short text to acknowledge the contributions of specific colleagues, institutions, or agencies that aided the efforts of the authors.
Conflict of interest
The authors declare that the research was conducted in the absence of any commercial or financial relationships that could be construed as a potential conflict of interest.
Publisher’s note
All claims expressed in this article are solely those of the authors and do not necessarily represent those of their affiliated organizations, or those of the publisher, the editors and the reviewers. Any product that may be evaluated in this article, or claim that may be made by its manufacturer, is not guaranteed or endorsed by the publisher.
Supplementary material
The Supplementary Material for this article can be found online at: https://www.frontiersin.org/articles/10.3389/fevo.2023.1231346/full#supplementary-material
References
Ali W., Ali H., Gillani S., Zinck P., Souissi S. (2023). Polylactic acid synthesis, biodegradability, conversion to microplastics and toxicity: a review. Environ. Chem. Lett. 21, 1761–1786. doi: 10.1007/s10311-023-01564-8
Altschul S. F., Gish W., Miller W., Myers E. W., Lipman D. J. (1990). Basic local alignment search tool. J. Mol. Biol. 215, 403–410. doi: 10.1016/S0022-2836(05)80360-2
Andrady A. L. (2011). Microplastics in the marine environment. Mar. Pollut. Bull. 62, 1596–1605. doi: 10.1016/j.marpolbul.2011.05.030
Behnke A., Engel M., Christen R., Nebel M., Klein R. R., Stoeck T. (2011). Depicting more accurate pictures of protistan community complexity using pyrosequencing of hypervariable SSU rRNA gene regions: Protistan community complexity. Environ. Microbiol. 13, 340–349. doi: 10.1111/j.1462-2920.2010.02332.x
Brandt P., Gerdts G., Boersma M., Wiltshire K. H., Wichels A. (2010). Comparison of different DNA-extraction techniques to investigate the bacterial community of marine copepods. Helgol. Mar. Res. 64, 331–342. doi: 10.1007/s10152-010-0188-1
Choi J. S., Hong S. H., Park J.-W. (2020). Evaluation of microplastic toxicity in accordance with different sizes and exposure times in the marine copepod Tigriopus japonicus. Mar. Environ. Res. 153, 104838. doi: 10.1016/j.marenvres.2019.104838
Cole M., Lindeque P. K., Fileman E., Clark J., Lewis C., Halsband C., et al. (2016). Microplastics alter the properties and sinking rates of zooplankton faecal pellets. Environ. Sci. Technol. 50, 3239–3246. doi: 10.1021/acs.est.5b05905
Cole M., Lindeque P., Fileman E., Halsband C., Galloway T. S. (2015). The impact of polystyrene microplastics on feeding, function and fecundity in the marine copepod calanus helgolandicus. Environ. Sci. Technol. 49, 1130–1137. doi: 10.1021/es504525u
Coppock R. L., Galloway T. S., Cole M., Fileman E. S., Queirós A. M., Lindeque P. K. (2019). Microplastics alter feeding selectivity and faecal density in the copepod, Calanus helgolandicus. Sci. Total Environ. 687, 780–789. doi: 10.1016/j.scitotenv.2019.06.009
Couceiro J. F., Keller-Costa T., Marques M., Kyrpides N. C., Woyke T., Whitman W. B., et al. (2021). The Roseibium album (Labrenzia alba) Genome Possesses Multiple Symbiosis Factors Possibly Underpinning Host-Microbe Relationships in the Marine Benthos. Microbiol. Resour. Announc. 10, e00320–e00321. doi: 10.1128/MRA.00320-21
Cregeen S. J. J. (2016) Microbiota of dominant Atlantic copepods: Pleuromamma sp. as a host to a betaproteobacterial symbiont. Available at: https://eprints.soton.ac.uk/403351/.
Dang H., Li T., Chen M., Huang G. (2008). Cross-ocean distribution of rhodobacterales bacteria as primary surface colonizers in temperate coastal marine waters. Appl. Environ. Microbiol. 74, 52–60. doi: 10.1128/AEM.01400-07
Das S., Ouddane B., Hwang J.-S., Souissi S. (2020). Intergenerational effects of resuspended sediment and trace metal mixtures on life cycle traits of a pelagic copepod. Environ. pollut. 267, 115460. doi: 10.1016/j.envpol.2020.115460
Das S., Ouddane B., Souissi S. (2022). Responses of the copepod Eurytemora affinis to trace metal exposure: A candidate for sentinel to marine sediment resuspension effects. Mar. pollut. Bull. 181, 113854. doi: 10.1016/j.marpolbul.2022.113854
Dayras P., Bialais C., Sadovskaya I., Lee M.-C., Lee J.-S., Souissi S. (2021). Microalgal diet influences the nutritive quality and reproductive investment of the cyclopoid copepod Paracyclopina nana. Front. Mar. Sci. 8. doi: 10.3389/fmars.2021.697561
Delacuvellerie A., Benali S., Cyriaque V., Moins S., Raquez J.-M., Gobert S., et al. (2021). Microbial biofilm composition and polymer degradation of compostable and non-compostable plastics immersed in the marine environment. J. Hazard. Mater. 419, 126526. doi: 10.1016/j.jhazmat.2021.126526
de Steenhuijsen Piters W. A. A., Watson R. L., de Koff E. M., Hasrat R., Arp K., Chu M. L. J. N., et al. (2022). Early-life viral infections are associated with disadvantageous immune and microbiota profiles and recurrent respiratory infections. Nat. Microbiol. 7, 224–237. doi: 10.1038/s41564-021-01043-2
Dixon P. (2003). VEGAN, a package of R functions for community ecology. J. Vegetation Sci. 14, 927–930. doi: 10.1111/j.1654-1103.2003.tb02228.x
Fackelmann G., Sommer S. (2019). Microplastics and the gut microbiome: How chronically exposed species may suffer from gut dysbiosis. Mar. pollut. Bull. 143, 193–203. doi: 10.1016/j.marpolbul.2019.04.030
Geyer R., Jambeck J. R., Law K. L. (2017). Production, use, and fate of all plastics ever made. Sci. Adv. 3, 1700782. doi: 10.1126/sciadv.1700782
Gulla S., Sørum H., Vågnes Ø., Colquhoun D. J. (2015). Phylogenetic analysis and serotyping of Vibrio splendidus-related bacteria isolated from salmon farm cleaner fish. Dis. Aquat. Org, 117, 121–131. doi: 10.3354/dao02938
Hammer Ø., Harper D. A., Ryan P. D. (2001). PAST: Paleontological statistics software package for education and data analysis. Palaeontol. Electronica 4 (1), 9.
Han Y., Zhang X., Liu P., Xu S., Chen D., Liu J. N., et al. (2022). Microplastics exposure causes oxidative stress and microbiota dysbiosis in planarian Dugesia japonica. Environ. Sci. pollut. Res. 29, 28973–28983. doi: 10.1007/s11356-022-18547-x
Heidelberg J. F., Heidelberg K. B., Colwell R. R. (2002). Bacteria of the γ-subclass proteobacteria associated with zooplankton in Chesapeake bay. Appl. Environ. Microbiol. 68, 5498–5507. doi: 10.1128/AEM.68.11.5498-5507.2002
Heindler F. M., Alajmi F., Huerlimann R., Zeng C., Newman S. J., Vamvounis G., et al. (2017). Toxic effects of polyethylene terephthalate microparticles and Di(2-ethylhexyl)phthalate on the calanoid copepod, Parvocalanus crassirostris. Ecotoxicol. Environ. Saf. 141, 298–305. doi: 10.1016/j.ecoenv.2017.03.029
Huq A., Sack R. B., Nizam A., Longini I. M., Nair G. B., Ali A., et al. (2005). Critical factors influencing the occurrence of vibrio cholerae in the environment of Bangladesh. Appl. Environ. Microbiol. 71, 4645–4654. doi: 10.1128/AEM.71.8.4645-4654.2005
Iyare U., P. K., Ouki S., Bond T. (2020). Microplastics removal in wastewater treatment plants: a critical review. Environ. Sci.: Water Res. Technol. 6, 2664–2675. doi: 10.1039/D0EW00397B
Jacquin J., Cheng J., Odobel C., Pandin C., Conan P., Pujo-Pay M., et al. (2019). Microbial ecotoxicology of marine plastic debris: A review on colonization and biodegradation by the “Plastisphere”. Front. Microbiol. 10. doi: 10.3389/fmicb.2019.00865
Jeong C.-B., Kang H.-M., Lee M.-C., Kim D.-H., Han J., Hwang D.-S., et al. (2017). Adverse effects of microplastics and oxidative stress-induced MAPK/Nrf2 pathway-mediated defense mechanisms in the marine copepod Paracyclopina nana. Sci. Rep. 7, 41323. doi: 10.1038/srep41323
Kassambara A., Mundt F. (2017). Package ‘factoextra’. Extract visualize results multivariate Data analyses 76 (2), 26–27.
Kozich J. J., Westcott S. L., Baxter N. T., Highlander S. K., Schloss P. D. (2013). Development of a dual-index sequencing strategy and curation pipeline for analyzing amplicon sequence data on the MiSeq illumina sequencing platform. Appl. Environ. Microbiol. 79, 5112–5120. doi: 10.1128/AEM.01043-13
Kwok K. W. H., Souissi S., Dur G., Won E.-J., Lee J.-S. (2015). ““Chapter 12 - copepods as references species in estuarine and marine waters,”,” in Aquatic Ecotoxicology. Eds. Amiard-Triquet C., Amiard J.-C., Mouneyrac C. (London: Academic Press), 281–308. doi: 10.1016/B978-0-12-800949-9.00012-7
Laist D. W. (1997). “Impacts of marine debris: entanglement of marine life in marine debris including a comprehensive list of species with entanglement and ingestion records,” in Marine Debris: Sources, Impacts, and Solutions Springer Series on Environmental Management. Eds. Coe J. M., Rogers D. B. (New York: NY: Springer), 99–139. doi: 10.1007/978-1-4613-8486-1_10
Lee K.-W., Shim W. J., Kwon O. Y., Kang J.-H. (2013). Size-dependent effects of micro polystyrene particles in the marine copepod Tigriopus japonicus. Environ. Sci. Technol. 47, 11278–11283. doi: 10.1021/es401932b
Li L.-L., Amara R., Souissi S., Dehaut A., Duflos G., Monchy S. (2020). Impacts of microplastics exposure on mussel (Mytilus edulis) gut microbiota. Sci. Total Environ. 745, 141018. doi: 10.1016/j.scitotenv.2020.141018
Li S., Wang P., Zhang C., Zhou X., Yin Z., Hu T., et al. (2020). Influence of polystyrene microplastics on the growth, photosynthetic efficiency and aggregation of freshwater microalgae Chlamydomonas reinhardtii. Sci. Total Environ. 714, 136767. doi: 10.1016/j.scitotenv.2020.136767
Lindeque P. K., Cole M., Coppock R. L., Lewis C. N., Miller R. Z., Watts A. J. R., et al. (2020). Are we underestimating microplastic abundance in the marine environment? A comparison of microplastic capture with nets of different mesh-size. Environ. pollut. 265, 114721. doi: 10.1016/j.envpol.2020.114721
Lobelle D., Cunliffe M. (2011). Early microbial biofilm formation on marine plastic debris. Mar. pollut. Bull. 62, 197–200. doi: 10.1016/j.marpolbul.2010.10.013
Lopez-Joven C., Rolland J.-L., Haffner P., Caro A., Roques C., Carré C., et al. (2018). Oyster farming, temperature, and plankton influence the dynamics of pathogenic vibrios in the Thau Lagoon. Front. Microbiol. 9. doi: 10.3389/fmicb.2018.02530
Lu L., Wan Z., Luo T., Fu Z., Jin Y. (2018). Polystyrene microplastics induce gut microbiota dysbiosis and hepatic lipid metabolism disorder in mice. Sci. Total Environ. 631–632, 449–458. doi: 10.1016/j.scitotenv.2018.03.051
Lusher A. (2015). Microplastics in the marine environment: distribution, interactions and effects. Mar. anthropogenic Litter, 245–307. doi: 10.1007/978-3-319-16510-3_10
Maechler M., Rousseeuw P., Struyf A., Hubert M., Hornik K., Studer M., et al. (2013). Package ‘cluster’ (Dosegljivo na).
Man W. H., de Steenhuijsen Piters W. A. A., Bogaert D. (2017). The microbiota of the respiratory tract: gatekeeper to respiratory health. Nat. Rev. Microbiol. 15, 259–270. doi: 10.1038/nrmicro.2017.14
Michalec F.-G., Holzner M., Barras A., Lacoste A.-S., Brunet L., Lee J.-S., et al. (2017). Short-term exposure to gold nanoparticle suspension impairs swimming behavior in a widespread calanoid copepod. Environ. pollut. 228, 102–110. doi: 10.1016/j.envpol.2017.04.084
Muthukumar T., Aravinthan A., Lakshmi K., Venkatesan R., Vedaprakash L., Doble M. (2011). Fouling and stability of polymers and composites in marine environment. Int. Biodeterior. Biodegrad. 65, 276–284. doi: 10.1016/j.ibiod.2010.11.012
Napper I. E., Bakir A., Rowland S. J., Thompson R. C. (2015). Characterisation, quantity and sorptive properties of microplastics extracted from cosmetics. Mar. pollut. Bull. 99, 178–185. doi: 10.1016/j.marpolbul.2015.07.029
Napper I. E., Thompson R. C. (2016). Release of synthetic microplastic plastic fibres from domestic washing machines: Effects of fabric type and washing conditions. Mar. pollut. Bull. 112, 39–45. doi: 10.1016/j.marpolbul.2016.09.025
Neuwirth E., Neuwirth M. E. (2011). Package ‘RColorBrewer’. Phys. Rev. D—Part. Fields Gravit. Cosmol. 84, 1–5.
Nicholson J. K., Holmes E., Kinross J., Burcelin R., Gibson G., Jia W., et al. (2012). Host-gut microbiota metabolic interactions. Science 336, 1262–1267. doi: 10.1126/science.1223813
Oberbeckmann S., Löder M. G. J., Labrenz M., Oberbeckmann S., Löder M. G. J., Labrenz M. (2015). Marine microplastic-associated biofilms – a review. Environ. Chem. 12, 551–562. doi: 10.1071/EN15069
Paul-Pont I., Tallec K., Gonzalez-Fernandez C., Lambert C., Vincent D., Mazurais D., et al. (2018). Constraints and priorities for conducting experimental exposures of marine organisms to microplastics. Front. Mar. Sci. 5. doi: 10.3389/fmars.2018.00252
Peng L., Fu D., Qi H., Lan C. Q., Yu H., Ge C. (2020). Micro- and nano-plastics in marine environment: Source, distribution and threats — A review. Sci. Total Environ. 698, 134254. doi: 10.1016/j.scitotenv.2019.134254
Qiao R., Deng Y., Zhang S., Wolosker M. B., Zhu Q., Ren H., et al. (2019). Accumulation of different shapes of microplastics initiates intestinal injury and gut microbiota dysbiosis in the gut of zebrafish. Chemosphere 236, 124334. doi: 10.1016/j.chemosphere.2019.07.065
Quast C., Pruesse E., Yilmaz P., Gerken J., Schweer T., Yarza P., et al. (2012). The SILVA ribosomal RNA gene database project: improved data processing and web-based tools. Nucleic Acids Res. 41, D590–D596. doi: 10.1093/nar/gks1219
Romeo T., Pietro B., Pedà C., Consoli P., Andaloro F., Fossi M. C. (2015). First evidence of presence of plastic debris in stomach of large pelagic fish in the Mediterranean Sea. Mar. Pollut. Bull. 95, 358–361. doi: 10.1016/j.marpolbul.2015.04.048
Roux F. L., Gay M., Lambert C., Waechter M., Poubalanne S., Chollet B., et al. (2002). Comparative analysis of Vibrio splendidus-related strains isolated during Crassostrea gigas mortality events. Aquat. Living Resour. 15, 251–258. doi: 10.1016/S0990-7440(02)01176-2
Schloss P. D., Gevers D., Westcott S. L. (2011). Reducing the effects of PCR amplification and sequencing artifacts on 16S rRNA-based studies. PloS One 6, e27310. doi: 10.1371/journal.pone.0027310
Sheavly S. B., Register K. M. (2007). Marine debris & Plastics: environmental concerns, sources, impacts and solutions. J. Polym. Environ. 15, 301–305. doi: 10.1007/s10924-007-0074-3
Souissi A., Hwang J.-S., Souissi S. (2021). Reproductive trade-offs of the estuarine copepod Eurytemora affinis under different thermal and haline regimes. Sci. Rep. 11, 20139. doi: 10.1038/s41598-021-99703-0
Souissi A., Souissi S., Devreker D., Hwang J.-S. (2010). Occurence of intersexuality in a laboratory culture of the copepod Eurytemora affinis from the Seine estuary (France). Mar. Biol. 157, 851–861. doi: 10.1007/s00227-009-1368-x
Souissi A., Souissi S., Hwang J.-S. (2016). Evaluation of the copepod Eurytemora affinis life history response to temperature and salinity increases. Zool. Stud. 55, e4. doi: 10.6620/ZS.2016.55-04
Tamplin M. L., Gauzens A. L., Huq A., Sack D. A., Colwell R. R. (1990). Attachment of Vibrio cholerae serogroup O1 to zooplankton and phytoplankton of Bangladesh waters. Appl. Environ. Microbiol. 56, 1977–1980. doi: 10.1128/aem.56.6.1977-1980.1990
Tanaka K., Takada H., Yamashita R., Mizukawa K., Fukuwaka M., Watanuki Y. (2013). Accumulation of plastic-derived chemicals in tissues of seabirds ingesting marine plastics. Mar. Pollut. Bull. 69, 219–222. doi: 10.1016/j.marpolbul.2012.12.010
Teng F., Darveekaran Nair S. S., Zhu P., Li S., Huang S., Li X., et al. (2018). Impact of DNA extraction method and targeted 16S-rRNA hypervariable region on oral microbiota profiling. Sci. Rep. 8, 16321. doi: 10.1038/s41598-018-34294-x
Thery J., Bialais C., Kazour M., Moreau M., Dufour D., Benali S., et al. (2022). A new method for microplastics identification in copepods. Front. Environ. Chem. 3. doi: 10.3389/fenvc.2022.905303
Turner J. T. (2004). The importance of small planktonic copepods and their roles in pelagic marine food webs. Zool. Stud. 43 (2), 255–266.
Von Moos N., Burkhardt-Holm P., Köhler A. (2012). Uptake and Effects of Microplastics on Cells and Tissue of the Blue Mussel Mytilus edulis L. after an Experimental Exposure. Environ. Sci. Technol. 46, 11327–11335. doi: 10.1021/es302332w
Wright S. L., Thompson R. C., Galloway T. S. (2013). The physical impacts of microplastics on marine organisms: A review. Environ. pollut. 178, 483–492. doi: 10.1016/j.envpol.2013.02.031
Zettler E. R., Mincer T. J., Amaral-Zettler L. A. (2013). Life in the “Plastisphere”: microbial communities on plastic marine debris. Environ. Sci. Technol. 47, 7137–7146. doi: 10.1021/es401288x
Zhang C., Jeong C.-B., Lee J.-S., Wang D., Wang M. (2019). Transgenerational proteome plasticity in resilience of a marine copepod in response to environmentally relevant concentrations of microplastics. Environ. Sci. Technol. 53, 8426–8436. doi: 10.1021/acs.est.9b02525
Zhang C., Li F., Liu X., Xie L., Zhang Y. T., Mu J. (2023). Polylactic acid (PLA), polyethylene terephthalate (PET), and polystyrene (PS) microplastics differently affect the gut microbiota of marine medaka (Oryzias melastigma) after individual and combined exposure with sulfamethazine. Aquat. Toxicol. 259, 106522. doi: 10.1016/j.aquatox.2023.106522
Zhang C., Zhang M., Wang S., Han R., Cao Y., Hua W., et al. (2010). Interactions between gut microbiota, host genetics and diet relevant to development of metabolic syndromes in mice. ISME J. 4, 232–241. doi: 10.1038/ismej.2009.112
Keywords: microplastics, microbiota, copepod, Eurytemora affinis, high-throughput sequencing, LDPE, PBAT
Citation: Thery J, Li L-L, Das S, Dufour D, Benali S, Raquez J-M, Souissi S and Monchy S (2023) Multigenerational exposure of microplastics on the microbiota of E. affinis (copepod): a comparative study between biodegradable and nonbiodegradable microplastics. Front. Ecol. Evol. 11:1231346. doi: 10.3389/fevo.2023.1231346
Received: 30 May 2023; Accepted: 18 July 2023;
Published: 08 August 2023.
Edited by:
Ram Kumar, Central University of Bihar, IndiaReviewed by:
P. Sathish Kumar, National Institute of Ocean Technology, IndiaSofiene Tlili, Conservatoire National des Arts et Métiers (CNAM), France
Isabella Buttino, Istituto Superiore per la Protezione e la Ricerca Ambientale (ISPRA), Italy
Copyright © 2023 Thery, Li, Das, Dufour, Benali, Raquez, Souissi and Monchy. This is an open-access article distributed under the terms of the Creative Commons Attribution License (CC BY). The use, distribution or reproduction in other forums is permitted, provided the original author(s) and the copyright owner(s) are credited and that the original publication in this journal is cited, in accordance with accepted academic practice. No use, distribution or reproduction is permitted which does not comply with these terms.
*Correspondence: Jérémy Thery, amVyZW15LnRoZXJ5MTlAZ21haWwuY29t