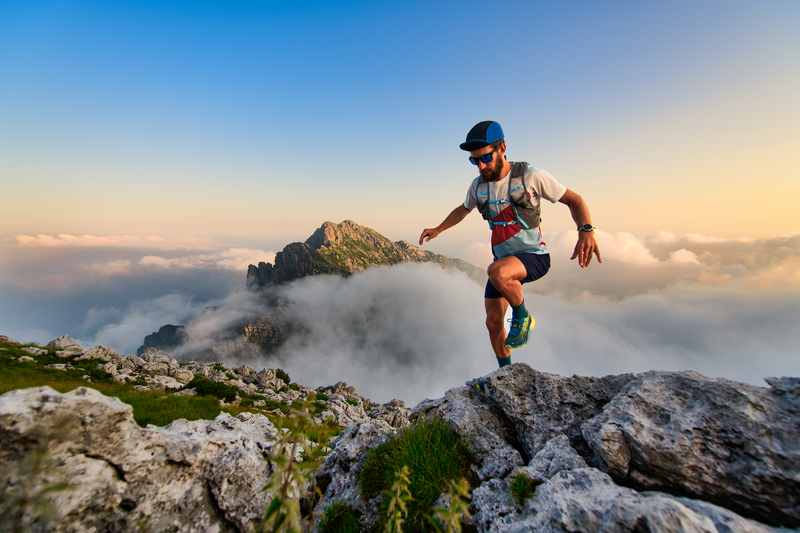
95% of researchers rate our articles as excellent or good
Learn more about the work of our research integrity team to safeguard the quality of each article we publish.
Find out more
ORIGINAL RESEARCH article
Front. Ecol. Evol. , 24 October 2023
Sec. Behavioral and Evolutionary Ecology
Volume 11 - 2023 | https://doi.org/10.3389/fevo.2023.1216463
The ability to respond to environmental changes plays a crucial role for coping with environmental stressors related to climate change. Substantial changes in environmental conditions can overcome developmental homeostasis, exposing cryptic genetic variation. The katydid Neoconocephalus triops is a tropical species that extended its range to the more seasonal environment of North America where it has two reproductive generations per year. The harsher winter conditions required adults to diapause which resulted in substantially different mating calls of the diapausing winter animals compared to the non-overwintering summer animals in northern Florida. The summer call corresponds to that of tropical populations, whereas the winter call represents the alternative call phenotype. We quantified call plasticity in a tropical (Puerto Rico) and a temperate population of N. triops (Florida) that differ in experiencing winter conditions in their geographic regions. We hypothesized that the plastic call traits, i.e., double-pulse rate and call structure, are regulated independently. Further, we hypothesized that phenotypic plasticity of double-pulse rate results in quantitative changes, whereas that of call structure in qualitative changes. We varied the photoperiod and duration of diapause during male juvenile and adult development during rearing and analyzed the double-pulse rate and call structure of the animals. Double-pulse rate changed in a quantitative fashion in both populations and significant changes appeared at different developmental points, i.e., the double-pulse rate slowed down during juvenile development in Florida, whereas during adult diapause in Puerto Rico. In the Florida population, both the number of males producing and the proportion of total call time covered by the alternative call structure (= continuous calls) increased with duration spent in diapause. In the Puerto Rico population, expression of the alternative call structure was extremely rare. Our results suggest that the expression of both pulse rate and call structure was quantitative and not categorical. Our systematic variation of environmental variables demonstrated a wide range of phenotypic variation that can be induced during development. Our study highlights the evolutionary potential of hidden genetic variation and phenotypic plasticity when confronted with rapidly changing environments and their potential role in providing variation necessary for communication systems to evolve.
In the foreseeable future, climate change will expose many organisms to different or more extreme environmental conditions than they have experienced during their recent evolution. Their evolutionary trajectory will depend on their capacity to respond to these changes (Seebacher et al., 2015; McInerny and Etienne et al., 2012) in terms of persistence in the geographic region or colonization of new regions (Agrawal, 2001). Understanding evolutionary responses to the rapidly changing environment will be a major challenge for evolutionary and environmental biology in the coming decades (sensu Bozinovic and Pörtner, 2015).
Most organisms have been selected to maintain their phenotype over the range of environmental conditions that they commonly experience (e.g., physiological and developmental homeostasis; review in Debat and David, 2001). Compensatory mechanisms may accommodate less optimal genetic alternatives under common conditions and thus preserve cryptic genetic variation (West-Eberhard, 2005). However, when organisms experience environmental conditions that exceed the capacity of homeostasis, cryptic genetic variability may be revealed as alternative phenotypic variants (= phenotypic plasticity; West-Eberhardt, 2003; Paaby and Rockman, 2014). If the exposed alternative phenotypes are adaptive, such phenotypic plasticity may lead to rapid evolutionary change and in extreme cases the selected trait may even become dominant in a population within a single generation (Campbell-Staton et al., 2017).
Here, we characterize call plasticity of the reproductive communication system in the tropical katydid Neoconocephalus triops (Orthoptera, Tettigoniidae) that extended its range into the novel environment of temperate North America (Greenfield, 1990). This katydid occurs through most of the American tropics and Caribbean, extending into temperate regions as far North as Ohio and Missouri (Whitesell, 1974; Walker and Greenfield, 1983; Greenfield, 1990). Its life history is typical for tropical katydids with direct egg development (i.e., without egg diapause) and multiple generations per year. In Puerto Rico, N. triops is multivoltine and males call all year long (Greenfield, 1990). In temperate regions, N. triops is bivoltine with one generation reproducing in the fall and their offspring overwintering as adults and reproducing in the following spring (Whitesell, 1974). Each generation of both tropical and temperate N. triops populations are largely the offspring of the immediately preceding generation, i.e., the two generations do not form separate gene pools (Whitesell, 1974).
Calls of tropical males consist of rapidly repeated pulse pairs or double-pulses (~110-120 double-pulses/s at 25°C; Beckers and Schul, 2010) that are grouped into rhythmically repeated chirps of about 1s duration (see Methods for detailed description). The double-pulse rate is the most important temporal call feature used in species recognition in N. triops (Beckers and Schul, 2008) and some tropical populations of N. triops are substantially more attracted to chirped than continuous calls (Beckers, 2008). In temperate regions, calls from the non-overwintering generation (fall) are comparable to those of tropical populations, i.e., the calls have a similar double-pulse rate and are structured in chirps (Whitesell and Walker, 1978; Beckers and Schul, 2008). Calls of overwintering generations, however, differ substantially from those of the non-overwintering generation: The double-pulse rate is about 20% slower at the same ambient temperature and the calls are typically categorized as continuous (Whitesell and Walker, 1978; Beckers and Schul, 2008). This ‘alternative call’ differs so strikingly from the ‘standard call’ of tropical and non-overwintering males that the overwintering generation was initially described as a different species (i.e., N. fuscostriatus; Whitesell, 1969; Walker and Greenfield, 1983). The differences between standard and alternative calls in chirp structure and pulse rate each are equivalent to species defining differences among other Neoconocephalus species (Schul et al., 2013).
The short photoperiod experienced by overwintering adults is a critical factor for the induction of the alternative phenotype in the temperate population (Whitesell and Walker, 1978; Beckers and Schul, 2008). When reared under the conditions that reliably result in the alternative phenotype in temperate N. triops, even tropical (Puerto Rico, Costa Rica) N. triops males expressed similarly reduced pulse rates (Beckers and Schul, 2010). However, neither the environmental conditions controlling call plasticity, nor the possible phenotypic variability have been systematically quantified.
The environmental conditions that N. triops experienced during its range expansion likely selected for traits supporting overwintering (e.g., frost tolerance, adult diapausing stages) while maintaining synchrony between male and female reproductive periods. We previously concluded that these traits necessary for survival likely had pleiotropic effects that resulted in the expression of the alternative call type as a by-product rather than an adaptation (Beckers and Schul, 2010). As a result, female preferences likely evolved to accommodate this male call plasticity for communication to remain intact in both generations in the temperate environment (Beckers and Schul, 2010).
In this study, we quantified phenotypic plasticity in double-pulse rate and call structure in two populations of N. triops. One population expresses call phenotypic plasticity in the field (Florida; Whitesell and Walker, 1978), and the other one does not (Puerto Rico; Walker and Greenfield, 1983; Greenfield, 1990). We chose these two populations because they represent the transition from continuously breeding, multivoltine N. triops tropical populations that do no express call plasticity (Puerto Rico) to bivoltine populations expressing call plasticity in the field (Florida) as the result of newly experienced winter conditions in temperate regions. In our experiments, we systematically varied the rearing conditions during development (i.e., time spent in diapause conditions) and measured their effects on the two call traits in the two populations. Preliminary observations (OMB unpublished data) suggest that some N. triops produce chirped calls with slow pulse-rates after being placed in diapause. Therefore, we hypothesized that phenotypic plasticity of double-pulse rate and chirp structure are two distinct traits, responding independently to changes in photoperiod. Calls of Neoconcephlaus species (SINA, 2023), and the majority of other Orthopterans (e.g., Gerhardt and Huber, 2002), are either structured in chirps or are continuous, indicating that call structure is a qualitative trait. In contrast, pulse rate varies quantitatively among acoustic insects and displays continuous variation in context of temperature changes (e.g., Gerhardt and Huber, 2002; N. triops: Whitesell and Walker, 1978). We hypothesized that plasticity of call structure in N. triops is likely a qualitative trait, with two distinct trait versions (chirped vs continuous call) while plasticity of pulse rate is likely a quantitative trait displaying continuous variation.
Our study contributes to a better understanding of potential origins and evolutionary responses to rapid environmental change that can contribute to phenotypic variation necessary for communication signals to evolve. The reported results will serve as the basis for future studies on the neural and genetic mechanisms underlying this plasticity and call diversification.
Short photoperiods related to winter conditions induce differences in the mating calls of N. triops (Whitesell and Walker, 1978; Beckers and Schul, 2008; Beckers and Schul, 2010). We reared juveniles from Florida and Puerto Rico under winter conditions to adulthood and then transferred them for different periods of time past adult molt (= treatment groups) in diapause for up to 140 days, which corresponds approximately to the diapausing duration in northern Florida (Beckers and Schul, 2008). We recorded and compared pulse rate and call structure across diapause treatments for each population (for details, see below). In addition, we reared and recorded the calls of a short-day control group (without diapause) and a control group reared in long-day to adulthood. We also recorded Wildtype animals from both populations for reference.
We collected about 50-80 non-diapausing males and females each during the summers of 2020 and 2021 around Gainesville, Florida, USA. We collected eggs from these animals to raise their offspring under experimental conditions (i.e., different durations spent in diapause and control conditions) in the laboratory. All experiments with the Florida population were conducted at Murray State University, Murray, Kentucky, USA.
We collected about 50-80 non-diapausing tropical males and females each in the winter of 2016/2017 in North-Eastern Puerto Rico. These animals were used to establish a permanent culture at the University of Missouri (Columbia, Missouri, USA). All experiments with the Puerto Rico population were conducted at the University of Missouri, Columbia, Missouri, USA.
We placed 30-160 adult animals (collected in the field or lab generations derived from culture) in cages for mating and oviposition in potted grass. These cages were in climate-controlled chambers or incubators at a light/dark cycle of 15/9h with day/night temperatures of 26/22°C, respectively. Relative humidity was kept between 40 and 60%. These conditions roughly correspond to summer conditions in northern Florida and Puerto Rico and have been used in previous studies (Beckers and Schul, 2008; Beckers and Schul, 2010).
We replaced the egg-laying grass every two weeks throughout the lifespan of the females. We removed the eggs from the grass and placed them in petri dishes (90 x 15 mm, Fisher-Scientific) lined with filter paper (85 mm, Grade 1, Whatman). We kept the filter paper moist with a saturated Methylparaben (NF grade, Amresco) solution to allow for egg development and prevent fungal growth in the petri dishes (Beckers and Schul, 2008). We placed the dishes inside a closed food storage box (Tupperware) at the same conditions as the breeding animals (see above) and checked daily for the emergence of juveniles.
Hatchlings were transferred to custom-built screen cages (45 x 10 x 25cm, length x width x height, 50-100 juveniles/cage) and kept on wheat seedlings, and water gel (Tasty Worm Nutrition) inside incubators. After 8-10 weeks under short-day conditions (see below), when insects reached 4-5th instars, we separated males and females and moved them to cages at a lower density (about 15-25 per cage). We removed adult males every day (Florida) or every 3 days (Puerto Rico) from the cages and transferred them into diapause conditions (see below). All treatment and Short-day control males experienced as juveniles cycles of 11/13h light/dark at temperatures of 23/17°C, respectively. Previous work (Beckers and Schul, 2008; Beckers and Schul, 2010) and preliminary experiments demonstrated that these short-day conditions paired with diapause conditions result in the expression of the winter or alternative call phenotype. Short-day controls were reared under short-day conditions (i.e., 11/13h light/dark at 23/17°C, respectively) and Long-day controls under long-day conditions (15/9h light/dark at 26/22°C, respectively) from first instars to adulthood. Short-day controls were transferred to long-day conditions and Long-day controls were kept in long-day conditions for males to start calling. The humidity across all rearing conditions ranged between 40 and 60%.
After short-day rearing to adulthood, we transferred animals to diapause conditions. The duration males spent in diapause differed among treatment groups and ranged between 0 to 140 days. The specific treatment groups were: 0, 28, 56, 84, 112, and 140 days in diapause. Preliminary experiments showed that Florida and Puerto Rico animals differed in their adult development. When Puerto Rico animals were kept in short-day conditions, they started to call after 10-14 days, i.e., they had completely matured and were ready to reproduce. Florida animals, however, never started calling under these conditions, indicating that their adult development was arrested, as expected when in reproductive diapause. To account for these differences, we transferred adult Puerto Rico animals after 2-4 days in short-day conditions into diapause conditions in which they did not call, while Florida adult animals stayed for 14 days in short-day conditions before being transferred to diapause conditions following a previously established rearing protocol (Beckers and Schul, 2008). Diapause conditions had cycles of 9.5/14.5h light/dark at 17/12°C, respectively.
After staying in diapause for a given treatment duration, we transferred the males to long-day conditions (light/dark cycle of 15/9h at 26/22°C, respectively) to induce final maturation and calling. While Florida males typically started calling after 3-4 weeks, Puerto Rico males often called within the first few days after being transferred to these long-day conditions.
We conducted diapause experiments with the Puerto Rico population in 2019-20 with approximately the 5th-8th generation in culture. Experiments with the Florida population took place between 2020-22 and used primarily F1 animals (i.e., offspring from wild collected insects) and a small number of F2 and F3 insects.
Males were placed individually in small cylindrical mesh enclosures (10 cm diameter, 15 cm high) housed inside of boxes lined with acoustic foam (HFW-2 or HFW-4, Hush Foam, Silent source). Microphones were placed inside these boxes within 10 cm of the male. The boxes were placed inside temperature-controlled semi anechoic chambers. Echoes were sufficiently suppressed to not influence the measurements of pulse and chirp pattern. The individual boxes strongly reduced the crosstalk between individual male’s recordings. We cannot rule out that males heard each other during recordings. Even though N. triops males do synchronize the beginning of their calling, i.e., they stimulate each other to start calling at the minute-scale, they do not synchronize the timing of their chirps (seconds-scale) or double-pulses (milliseconds-scale; OMB and JS personal observation). Thus, potential acoustic interaction among males would not influence the measurements in this study.
We recorded Florida males with electret tie-clip microphone (ATR3350; Audio-Technica) and digitized the signals through an 8-channel interface (Tascam US-1800) and the software Cubase 5.0SL (Steinberg) at 48kHz sampling rate and 16-bit resolution. We recorded Puerto Rico males using USB voice recorders (VR1.0; DB9PRO) at 48kHz sampling rate and 16-bit resolution. These voice recorders use 1/8” electret microphones with high sensitivity between 1-20 kHz. Recordings were inspected and trimmed for analysis using either Audacity for MacOS (Version 3.21; Audacity team) or WavePad (Audio Editor V. 16.65, NCH Software) software. We recorded all males at 25 ± 1°C ambient temperature.
The basic call of N. triops is shown in Figure 1. Neoconocephalus triops males produce calls with a double-pulse (DP) pattern with each DP consisting of two sets of alternating opening and closing movements of the wings (Walker, 1975), with only the closing movements producing sound pulses. The two loud pulses have different periods (i.e., duration from the beginning of the first pulse to the beginning of the second pulse), resulting in paired sound pulses (Figure 1A). These DP are grouped into rhythmically repeated chirps of roughly 1s duration that are separated by 50-100ms of silence (Figure 1B).
Figure 1 Schematic of N. triops male calls at the millisecond (A) and second (B) scale. (A) High amplitude sound pulses are produced during the closing movements of the forewings. Alternating pulse periods (p1, p2) result in a double-(DP) pulse rhythm. We measured the double-pulse period (DP-P), which is the duration between the beginning of the first pulse of a DP and the beginning of the first pulse of the next DP and converted them to DP rates (1/DP-P). (B) Double-pulses are grouped into chirps of about 1s duration, repeated after silent intervals of about 50ms duration. We measured chirp periods (CHP-P) from the beginning of a chirp to the beginning of the following chirp.
We measured the time from the beginning of each DP to the beginning of the next (= DP-period, Figure 1A) of 1-3s of calling of each male, which corresponded to about 100-300 DP-periods. For the temporal measurements, we extracted the envelope from the signals while reducing the sampling rate to 12 kHz (Florida) or 8 kHz (Puerto Rico), equivalent to a temporal resolution of 0.083ms or 0.125ms, respectively. We used the custom software Song_X developed by JS to measure the DP-periods. For each animal, we calculated the average DP-period. The pulse pattern of insects or frog calls are typically described as pulse rates rather than pulse periods (Gerhardt and Huber, 2002). We therefore converted the DP-period of each individual to the DP-rate (= 1/DP-period) and present the data here as DP-rate (= DP/s; Figure 2).
Figure 2 Double-pulse rate (mean ± SD) of Puerto Rico (A) and Florida (B) N. triops in response of rearing conditions. Wildtype (WT) males were collected in the field, Long-Day controls (LD) had juvenile development and post adult molt maturation in 15:9 light/dark cycle and did not experience diapause conditions. All other treatment groups had juvenile development with 11/13h light/dark cycle and diapause conditions of 9.5/14.5 light/dark for the indicated durations. The 0-day diapause treatment corresponds to the Short-Day control. ‘n.s.’ indicates no significant statistical difference. Different letters between treatments indicate significant differences (p < 0.05).
To measure chirp periods (i.e., the duration between the beginning of a chirp and the beginning of the subsequent chirp, Figure 1B), we selected 6-8 minutes of calling for each animal. These sections typically contained multiple calling bouts of 0.5-5 minutes duration. For temporal measurements, we extracted the envelope with a sample rate of 1 kHz for analysis and used the custom software Song_X to measure the chirp periods.
At the beginning of calling bouts, a few of the chirps often had low amplitude that made it difficult to reliably measure their periods. Such chirps early in the bout were excluded from analysis. The last one or two chirps were substantially shorter than those of the rest of the bout and were excluded from the analysis as well. A calling bout ended if the silent interval between two chirps was longer than 5s. We did not include the silent periods between calling bouts in the analysis. Uninterrupted calling for longer than 5s (or ‘unusually long chirps’) are categorized throughout the manuscript as ‘calling pieces’ to distinguish this type of calling from rhythmically interrupted chirps and calling bouts (see Supplementary Materials). The distribution of chirp durations in the Puerto Rico population is tightly centered around 800-1000ms with a sharp drop off to longer chirps. Less than 20 chirps among the more than 10,000 measured chirps of this population were longer than 2.0s, only one was longer than 5.0s. The distribution of chirp periods in the Florida population was broader extending to 3s. Chirp durations beyond three seconds occurred at a low frequency; about 150 chirps (out of more than 10,000) had durations between 5 and >500s. Few chirps with durations between 3 and 8s occurred. We tried other cut-offs between 3 and 8s, which did not change the outcomes or conclusions of this analysis.
The loss of the chirp structure resulting in continuous calls (alternative structure) in ‘winter males’ (Whitesell and Walker, 1978; Beckers and Schul, 2008) suggests deterioration of the neural chirp pattern generator. To detect effects of this deterioration on the chirp pattern (aside from the complete loss), we used the Coefficient of Variation (or ‘C.V.’ = standard deviation/mean) as a measure for the quality of the chirp pattern, i.e., low C.V. values indicate consistent chirp periods and thus a high quality of the pattern, whereas high C.V. values indicate more variability and thus a lower pattern quality. We expected that adult diapause results in reduced pattern quality (i.e., increased within-male variation and C.V.). We calculated the average chirp period and the C.V. for each individual’s call recording.
We did not collect chirp data from wild katydids from Puerto Rico in 2017 but used recordings collected previously from the same population (Beckers and Schul, 2010). We used these recordings for the comparison of DP-rate with the lab-reared animals. We present the average chirp period of the 2010 data as Wildtype (WT) population. However, these recordings were too short (10-20 chirps per animal) to calculate representative chirp period C.V.
Across all recordings used in this study, chirp durations were overwhelmingly shorter than 3s. For the Florida population 149 chirps were longer than 5s. These 149 chirps covered 18.2% of the total calling time analyzed for the Florida population (17,817 chirps in total), so they represent a significant part of this population’s calling behavior. We excluded these ‘calling pieces’ with durations longer than 5s from the chirp analysis and analyzed them separately, i.e., we present the fraction of males producing such long calling pieces and the fraction of their calling time covered by these long call pieces in separate analyses. In contrast, only one of all recorded Puerto Rico males produced a single calling piece that was longer than 5s (out of 14,052 chirps analyzed).
We reared animals from hatching to adult molt in long-day conditions (Long-day control), short-day conditions (Short-day control), and collected animals from the field in Florida and Puerto Rico (Wildtype or ‘WT’) as references. Note that we did not record animals collected as adults in Puerto Rico for this study and used recordings that were done in the context of another project (Beckers and Schul, 2010). We used these recordings to determine the DP-rate and chirp period but not for the C.V. of the pulse period because we did not have long enough recordings to calculate a C.V. that would truly represent the consistency of the chirp pattern. We compared the double-pulse rate and chirp period in two separate ANOVAs for each population. One ANOVA compared the double-pulse rate across the controls (Long-day, Short-day, WT) and another ANOVA those of the rearing treatments within each population (0 days to 140 days). We used post-hoc Tukey tests to determine significant differences between control groups and treatment groups within each population. We compared the double-pulse rates and chirp periods between Florida and Puerto Rico WT animals using t-tests. We calculated for each recording the C.V. of the chirp period to compare the variability as a measure for chirp pattern quality. We used ANOVAs and post-hoc Tukey tests to compare the C.V.s across treatments within each population.
In contrast to Florida animals, only one of all Puerto Rico animals produced a long call piece (i.e., > 5s). To compare the proportion of males producing calling pieces longer than 5s in the Florida population, we used a Fisher exact test. Because very few Florida animals produced these calling pieces in the three control groups and 28-day treatment (N = 2 - 4), we could not run an ANOVA across the data to test the proportion of calling that consisted of such long calling pieces. To test for a change in this proportion toward longer treatment durations, we pooled the data of the 56- and 84- day treatments and compared those data to that of the pooled 112- and 140-days treatments using a t-test. All data analyzed with parametric tests complied with normality and homogeneity of variance assumptions of these models. We used JMP (version 16.2.0 for Mac) for all statistical analyses.
The DP-rate of wild-collected Florida animals did not differ from that of Puerto Rico animals (T-test, t-ratio = 1.544, p = 0.146). Next, we compared the DP-rates among the three controls (Wildtype, Long-day, Short-day) within each population to test for effects of juvenile development on this call trait. DP-rates for the Puerto Rico population were around 105 DP/s for all three controls (Figure 2A) and the DP-rates did not differ among controls (ANOVA, F-ratio = 1.15, p = 0.33). In the Florida population (Figure 2B), Wildtype controls had an average DP-rate of 104.8 DP/s and those of Long-and Short-day controls were 98.7 and 91.3 DP/s, respectively. These controls differed significantly (ANOVA, F-ratio = 16.70, p < 0.0001) and post-hoc tests revealed significant differences among all three controls (all post-hoc Tukey tests: p < 0.02).
Next, we tested for effects of diapause duration on DP-rate in each population. These treatments had the same juvenile developmental conditions as the Short-day control (= 0-day diapause) and differed in the duration that the animals stayed as adults in diapause conditions. DP-rates of Puerto Rico males differed significantly across diapause durations (ANOVA, F-ratio = 24.92, p < 0.0001), decreasing from about 105 DP/s at short diapause durations to 81.2 DP/s at 138 days (Figure 2A). DP-rates of animals that spent 15 to 52 days in diapause did not differ from the Short-day control (= 0 days; all post-hoc Tukey tests: p ≥ 0.99). Animals that spent more than 100 days in diapause had significantly slower DP-rates than the Short-day control (all post-hoc Tukey tests: p < 0.0001). DP-rates at 123 and 138 days were significantly lower than those of all groups up to 95 days (all post-hoc Tukey tests: p ≤ 0.0004).
In the Florida population, the DP-rates of diapause treatments ranged from 83.5 to 91.3 DP/s (Figure 2B) and even though our analysis detected a significant difference (ANOVA, F-ratio: 3.12, p = 0.014) none of the treatments differed significantly from the Short-day control (= 0-days diapause) or among treatments (all post-hoc Tukey tests: p ≥ 0.051). Notably, even though marginally not significant (0.051 ≥ p ≥ 0.073), the 84- to 140-day diapause treatments resulted in the slowest DP-rates compared to the Short-day control, possibly indicating a further reduction in DP-rate due to diapause.
The characteristic chirp pattern of N. triops was affected during diapause and continuous calls were typically only observed in the ‘winter’ generation (Whitesell and Walker, 1978; Beckers and Schul, 2008). Examples of the time course of chirp periods within calling bouts are shown in Figure 3. Most males from Puerto Rico showed little variation of the chirp period during each calling bout while some males produced shorter chirp periods at the beginning and end of each calling bout (Figure 3A). These patterns appeared in all treatment groups and were not correlated with rearing or diapause conditions.
Figure 3 Examples for the time course of chirp periods during a calling bout of male N. triops. The chirp periods are plotted at the time when they occurred during a calling bout. (A) Five calling bouts of Puerto Rico males without call pieces > 5s. Each calling bout was produced by a different male. (B) Six calling bouts of Florida males without call pieces > 5s. Each calling bout was produced by a different male. (C) Four calling bouts of Florida males with longer call pieces. The gray bars at the top indicate times with continuous calling (i.e., calling pieces longer than 5s) and the diamonds indicate the beginning of such long call pieces. The rightmost calling bout fell just short of reaching the 5s threshold. Each calling bout stems from a different male. (D) The only calling bout of a Puerto Rico male with a call piece >5s (Long-day control male). Note that the timescale in (C, D) are different from (A, B).
Males from Florida showed similar chirp period patterns in all treatment groups, however, chirp periods were typically more variable than those of the Puerto Rico population (Figure 3B and see below). In addition, a distinctly different pattern occurred in all Florida treatment groups, i.e., calling bouts started with a distinct chirp pattern and lengthening chirp periods over 10-90s before switching to periods of ‘continuous calling’ (i.e., call pieces longer than 5s) of variable duration (range 5.5s – 489s) followed by a short stretch of chirps at the end (Figure 3C). Almost all cases of continuous calling followed this temporal chirp pattern in the Florida population. We also observed ‘transitionary’ patterns that never reached call pieces longer than 5s (Figure 3C, right). Only one male of the Puerto Rico population (N = 57) produced a single call piece longer than 5s. The time course of chirp periods during this calling bout was comparable to Florida calls (Figure 3D).
To quantify the effect of juvenile development and diapause on the chirp pattern, we analyzed chirped and continuous call sections separately. Comparing the chirp periods of Wildtype animals between Florida and Puerto Rico populations indicated that the chirp periods of Florida animals were significantly longer than those of the Puerto Rican animals (T-test, T-ratio = -4.83, p = 0.0002). The average chirp periods of Puerto Rico males ranged between 980 and 1032ms for the three control treatments and they did not differ significantly from each other (ANOVA, F-ratio = 0.59, p = 0.56; Figure 4A). Chirp periods differed significantly across diapause treatments (ANOVA, F-ratio = 3.54, p = 0.005). However, the only significant differences were that the 30-day chirp period was shorter than those of the 61-, 123-, and 138-day treatments (all post-hoc Tukey tests: p ≤ 0.026), while none differed from the Short-day control (post-hoc Tukey tests, all p ≥ 0.17).
Figure 4 (A) Chirp period duration (mean ± SD) of Florida (black symbols) and Puerto Rico (grey symbols) N. triops at different rearing treatments. (B) Mean of within-male Coefficient of Variation (C.V.) of the chirp period of the measurements shown in (A). The C.V. is used as a proxy for the “quality” of the chirp rhythm. WT males were collected in the field, Long-day (LD) males with juvenile development and post adult molt maturation in 15/9h light/dark cycle and no diapause. All other males developed as juveniles in a 11/13h light/dark cycle and diapaused in a 9.5/14.5h light/dark cycle. The duration in diapause is indicated on X-axis. The 0-day diapause treatment corresponds to the Short-Day control. ‘n.s.’ indicates no significant statistical difference. Different letters and asterisk indicate significant differences between treatments (all p < 0.05).
Mean chirp periods of Florida males of the three control treatments ranged between 1239.5 and 1356.9ms and did not differ significantly (ANOVA, F-ratio= 1.49, p = 0.239; Figure 4A). Mean chirp periods increased from the Short-day control with increasing diapause duration to 1692ms at 140-days of diapause, however, this increase was not significant (ANOVA, F-ratio = 1.90, p = 0.109; Figure 4A).
We use the coefficient of variation (C.V.) of individual males’ chirp periods to evaluate the within-male consistency of the chirp rhythm (Figure 4B). In the Puerto Rico population, we detected no difference between the Long-day and Short-day control (= 0-day, T-test, t-ratio = -0.83, p = 0.424). In the Florida population, we detected a significant difference across the three controls (ANOVA, F-ratio = 5.59, p = 0.008), with the C.V. of Short-day controls being larger than those of Wildtype animals (post-hoc Tukey test, p = 0.006). In the Puerto Rico population, our analysis detected a significant effect of diapause treatment on the chirp period C.V. (ANOVA, F-ratio = 2.40, p = 0.041), yet post-hoc comparisons did not identify significant differences between any two treatments (all post-hoc Tukey tests, p ≥ 0.11). Similarly, the mean C.V. of the Florida diapause treatments did not differ significantly across diapause durations (ANOVA, F-ratio = 0.77, p = 0.58).
The proportion of Florida males producing long call pieces (> 5s duration) did not differ among the Wildtype, Long-, and Short-day controls (Fisher Exact Test: p = 0.501; Figure 5A). However, the proportion of males producing long calling pieces significantly increased with diapause duration from about 20% at 0 days and 28 days of diapause to 70% at 140 days of diapause (Fisher Exact test: p = 0.049). The proportion of total calling time covered by long (> 5s) calling pieces was highly variable among males (Figure 5B). The proportion of long calling pieces increased significantly between intermediate treatment durations (pooled 56- and 84-days) compared to long treatment durations (pooled 112- and 140-days; T-test, t-ratio = 2.35, p = 0.031).
Figure 5 Continuous calling of the Florida population of N. triops. (A) Proportion of males of each treatment that produced call pieces longer than 5s (N = 8-15 per treatment). (B) Proportion of long calling pieces (>5s chirps) relative to the total calling time. Individual data points and means (grey) for treatments 60 days and longer are shown. The grey trendline connects only the data used in the analysis (see Methods). Note that out of all analyzed animals (N = 8-15 per treatment) only those are shown that produced long calling pieces. Each symbol represents data from one animal. WT males were collected in the field, Long-day (LD) males with juvenile development and post adult molt maturation in 15/9h light/dark cycle and no diapause. All other males developed as juveniles in a 11/13h light/dark cycle and diapaused in a 9.5/14.5h light/dark cycle. The duration in diapause is indicated on X-axis. The 0-day diapause treatment corresponds to the Short-Day control. Asterisks indicate significant differences (p < 0.05).
Phenotypic plasticity of DP-rate in N. triops was a quantitative trait in both tested populations (Figure 2). Induction of the alternate (=low) pulse rate occurred at different developmental stages, i.e., juvenile stage in Florida animals (Figure 2B) and adult diapause in Puerto Rico animals (Figure 2A). Unexpectedly, phenotypic plasticity of chirp structure, which occurred almost exclusively in the Florida population, was also a quantitative trait (Figure 5B). In Florida males, the expression of the alternative continuous call structure became more prominent with longer duration of adult diapause (Figure 5B) and took place after the induction of the alternative double-pulse rate. These results support our prediction that phenotypic plasticity of double-pulse rate and chirp structure are both independently induced and likely independently evolving traits.
When N. triops expanded its range into temperate North America, it experienced environmental conditions during winter that interfered with its tropical life history: Offspring of the summer generation maturing in late fall were exposed to temperatures too cold for reproduction. Thus, this population experienced strong selection for adult diapause, i.e., arresting development after the fall adult molt and delaying reproduction until spring. Observed differences in life history between Florida and Puerto Rico populations seem to support this selection hypothesis for adult diapause: Independent of diapause duration, short-day raised Florida males did not start calling, unless exposed to long-day conditions for more than four weeks, suggesting that significant maturation steps were still required for calling. We have observed this same pattern in previous studies as well (Beckers and Schul, 2008; Beckers and Schul, 2010). In contrast, Puerto Rico Males raised in the same conditions started calling two to three weeks after adult molt, even when remaining in short-day conditions (J.S. personal observation). Puerto Rico males occasionally called while in diapause and, when transferred to long-day conditions after diapause, most males called within one week. Thus, development of Puerto Rico males was not arrested and their inactivity in diapause conditions was likely caused by the low temperatures (12-17°C).
The arrested development induced during juvenile development in the Florida population exceeded the capacity of compensatory (or homeostatic) mechanisms that otherwise maintain the standard call phenotype (i.e., fast pulse rate, chirped call structure) in the more stable environment of the tropics. The resulting alternative call phenotype represents a reversal to an ancestral state in call structure (i.e., continuous calling for Neoconocephalus; Frederick and Schul, 2016), as well as a substantial change in a critical call parameter for female call recognition (i.e., double-pulse rate; Beckers and Schul, 2008). Neither of these call changes seem to be adaptive, in terms of resulting in an increase in attractiveness to females. Rather to the contrary, the alternate, slower double-pulse rate reduces female attraction and affects males’ fitness (Beckers and Schul, 2008). Thus, the increased diversity in mating calls expressed in the ‘winter’ generation of N. triops is likely a byproduct of selection for diapause rather than the result of direct selection on these traits. We discuss below first the evolutionary context of this call plasticity and second its neural basis.
Initially, phenotypic plasticity of N. triops was described as two categorical call phenotypes: A summer (or standard) call and a winter call (or alternative) call (Whitesell, 1974; Whitesell and Walker, 1978). The standard call is rhythmically interrupted into chirps and has a ~20% faster double-pulse rate than the slower and continuous alternate call. However, our results indicate that this categorization needs to be revised. First, the double-pulse rate and the call structure are not categorical but quantitative traits. Second, population differences as well as large scale differences in the timing of induction of either call trait indicate that the call is modular, i.e., the two call traits can be induced independently of each other. Note that in Florida the change in DP-rate was induced during juvenile development, whereas in Puerto Rico it changed during adult diapause. We report here a previously undescribed combination of call structure and pulse rate resulting in a new call phenotype: Puerto Rican males that were kept in diapause for long periods produced a chirped call (standard structure) with the slow DP-rate of the alternate call (Figure 2A: >100d; none of these animals produced call pieces longer than 5s). Similarly, Florida males that we reared in short-day conditions as juveniles until adulthood or additionally kept for 28 days in diapause afterwards produced chirped calls with slow pulse rates (Figures 2B, 5A: 0-28d; slow DP-rate but majority of males called with chirps). The quantitative and modular nature of call plasticity ultimately contributes to even more phenotypic variation in this calling behavior than initially assumed, because intermediate states of both call traits and new combinations can be expressed as well.
When N. triops expanded its range into temperate North America, male call plasticity challenged the match between signals and preferences in the new environment, requiring female preferences to evolve in response to male call changes (Beckers and Schul, 2010). The developmental capacity to express quantitative, smaller incremental changes rather than a few large qualitative changes in the mating calls may have facilitated the necessary co-evolution between calls and female preferences (Beckers and Schul, 2010). Thus, female preference followed rapid phenotypic change of male calls here, making this system another example that challenges the sexual selection paradigm that male signals typically evolve in response to female preferences (Bush and Schul, 2010).
Phenotypic plasticity can be a potent mechanism to provide phenotypic variation in species-specific traits (e.g., pulse rate) necessary to propel the evolution of communication systems that female mate recognition typically keeps rather invariable (Gerhardt and Huber, 2002). Our experimental design allowed us to detect intermediate trait states and modularity of the variable call traits, displaying the potential of novel behavioral phenotypes in N. triops. It is possible that a similar approach in other plastic communication systems may reveal further signal variation than has been described (e.g., Gryllus rubens: Walker, 2000; Beckers et al., 2019; Laupala cerasina: Grace and Shaw, 2004; Allonemobius fasciatus: Olvido and Mousseau, 1995). The trait variation of other behavioral and morphological traits that have been described largely as categorical (e.g., reproductive alternatives: Eberhard and Gutiérrez, 1991; Rowland and Emlen, 2009; feeding alternatives: Collins and Cheek, 1983; Pfennig, 1992; seasonal alternatives: Shapiro, 1976) might merely represent ends of a quantitative trait spectrum and may benefit from being tested, analyzed, and redescribed in a more nuanced way. Considering the complexity of phenotype induction through multiple environmental factors acting at various time points (review in West-Eberhardt, 2003), it would not be surprising if phenotypic plasticity is generally more quantitative in nature with graded phenotypes induced over a range of stimuli rather than two or few categorical phenotypes induced at discreet thresholds.
In the temperate environment, adult diapause in N. triops is crucial for survival through the winter. Thus, selection should favor a lower threshold and/or earlier induction of the physiological changes for diapausing. In contrast, in tropical environments where N. triops breeds all year long (Walker and Greenfield, 1983), induction of diapause would be maladaptive by prolonging generation time even though conditions would allow breeding. Note that daylength, i.e., the environmental factor inducing diapause in Florida, is more constant in Puerto Rico than in Florida, it still varies significantly throughout the year. Selection therefore should favor higher thresholds for diapause induction in this population, leading to divergence of the threshold value between Florida and Puerto Rico populations. The drastically later induction of DP-rate changes (juvenile vs. adult stage) and lack of call structure changes in the Puerto Rico population might indicate such a divergence in threshold value between populations. Similarly, the threshold for minor and major male morphs in the polyphenic dung beetle Onthophagus taurus has evolved among populations, likely as the result of regional differences in inter- and intraspecific competition (Moczek, 2003). These studies highlight how phenotypic plasticity can contribute variation necessary for selection to act and populations to evolve.
Consistent and repeatable environmental conditions can lead to the permanent expression of only one, more suitable phenotype in a previously plastic system (West-Eberhardt, 2003). In this case, selection through genetic accommodation can act to genetically fix this phenotype (genetic canalization; Waddington, 1961). In essence, the threshold evolves to be not responsive to environmental input any longer, resulting in the loss of plasticity (West-Eberhardt, 2003). This evolutionary scenario could play out in N. triops in more seasonal temperate regions further north from Florida where the reproductive season is significantly shortened and does not allow for a second generation to occur in late summer (Whitesell, 1974). These conditions are met at the northern edge of N. triops’ range where the katydid has only one reproductive generation per year and all individuals diapause as adult until the following spring (OMB personal observations; SINA, 2023). We are currently testing the hypothesis that this northern population of N. triops has lost its capacity to express both call phenotypes.
Given that the alternative (= slower) double-pulse rate appears to be a pleitropic effect of adult diapause induction (Beckers and Schul, 2010), canalization of diapause should also genetically fix this alternative call trait. Further, this should select in these univoltine populations for female preference for the lower pulse rate. Canalization of the alternative call phenotype could potentially lead to reproductive isolation between this temperate univoltine population and tropical N. triops, depending on the location of the secondary contact. The bivoltine Florida population expressing both call types currently acts as a bridge for gene flow between the northern and tropical populations.
The two populations of N. triops also differed substantially in plasticity of their call structure, with one population displaying different call structures (Florida), whereas the other population seemingly did not (Puerto Rico). In the Florida population, continuous callers occurred in all treatment groups and their proportion reached ~70% after three months in diapause. In contrast, only a single Puerto Rico male produced a single continuous call section across all treatment groups. Female preferences for call structure shows striking geographic variation (Beckers, 2008). Females from Costa Rica require chirped calls and do not show significant responses to continuous calls. In contrast, females from Florida respond equally well to chirped and continuous calls (Beckers and Schul, 2008). The loss of selectivity for chirped calls in the Florida population may be a consequence of the diapause-related continuous calls in the winter generation, i.e., the loss of chirp preference facilitated successful communication of winter animals. Interestingly, females from Puerto Rico also lack the preference for chirped calls and respond to chirped and continuous calls equally well (Beckers, 2008). This pattern has two possible explanations. First, the loss of preference for chirped calls evolved in Florida as a response to the continuous calls of the winter generation and spread to Puerto Rico. Alternatively, the loss of preference may have occurred in tropical populations and facilitated the spread into temperate Florida by mitigating the effect of the plasticity of the call structure. Existing data does not allow to distinguish between these hypotheses.
Generation of the double-pulse pattern requires some form of neural resonance in the central call pattern generator (Marder, 2000). Such resonances may occur within single neurons, i.e., across cell membranes, or among neurons or networks involving a combination of membrane and synaptic properties (Hutcheon and Yarom, 2000; Izhikevich, 2001). Tuning of resonances relies typically on ratios of currents rather than absolute values. Thus, similar outcomes can be achieved with different combinations of current levels (Schulz et al., 2007; Franklin et al., 2010; Hudson and Prinz, 2010). Changing of the excitation/inhibition balance within the network is another potential mechanism to tune central pattern generator resonances (Buzsáki and Wang, 2012). Importantly, changing neural resonance likely is the outcome of quantitative changes of gene expression and does not require major re-organizations of the neural topography of the central pattern generators.
In Florida N. triops, the alternate double-pulse rate was induced during juvenile development. Here, the environmental conditions affected the tuning of the double-pulse pattern generator before it became functional after adult molt. In the Puerto Rico population this change occurred after the adult molt, i.e., after experiencing short-day conditions and extended periods of diapause conditions. At the time of the change, the double-pulse central pattern generator was likely already functional, which was indicated by calling of some Puerto Rico males in short-day and diapause conditions. Thus, in Puerto Rico the change in double-pulse rate was likely the result of changes in an already existing resonance rather than the development of a slower resonance from the start. Determining which neural changes underlie the changes of double-pulse rate in the two populations would require more detailed neural and transcriptomic studies.
Crickets and katydids have separate CPGs for chirp and pulse pattern which are located in different ganglia in the thoracic/abdominal central nervous system (Schöneich and Hedwig, 2011; Jacob and Hedwig, 2016; Katydids: JS in prep.). Males of the Florida population that produce continuous call pieces also produce chirped calling before and after continuous calling parts (Figure 3), indicating that the chirp generator is functional. The integration of the chirp pattern with the pulse rhythm, however, seemingly breaks down during continuous calling. Thus, time spent in diapause likely interferes with the integration of the two patterns during the post adult maturation of the central nervous system. Similarly, the increased chirp period C.V. of diapausing Florida males (Figure 4B) suggests that induction of diapause also deteriorated the quality of the chirp pattern in addition to the effect on pattern integration.
Climate change, colonizations, and invasions can expose species to novel or more extreme environmental conditions (Hughes, 2000; Ghalambor et al., 2007). In these instances, homeostatic mechanisms that buffer typical environmental variation are challenged (Rutherford, 2000), exposing novel trait variation through phenotypic plasticity (Ghalambor et al., 2007). The exposed hidden genetic variation underlying these novel traits may then become a necessity for species to evolve (Rutherford, 2000) and successfully settle or survive in these new environmental conditions. Thus, hidden genetic variation exposed to selection through plastic phenotypes may represent the basis for some (pre-)adaptations and may determine the ability of individuals to cope with environmental challenges beyond the natural range of variation (Rutherford and Lindquist, 1998; Ghalambor et al., 2007). The value of studying invasive species experiencing novel environmental conditions lies in better understanding and predicting possible evolutionary responses to climate change, which has recently gained attention (reviews in Caplat et al., 2013; Moran and Alexander, 2014). Our study provided important insights into these processes in the context of sexual communication, a suite of traits that aids in species isolation but also speciation (e.g., Arnegard et al., 2010). Further research into the hidden genetic capacity is of importance to our understanding of species existence, extinction, and evolution in face of rapidly changing environments.
The raw data supporting the conclusions of this article will be made available by the authors, without undue reservation.
The manuscript presents research on animals that do not require ethical approval for their study.
OB, TK, and JS contributed to the conception and design of the study. OB, TK, and JS contributed to the writing and revision of the manuscript and approved the submitted version. OB and JS collected and analyzed the data. All authors contributed to the article and approved the submitted version.
The authors would like to thank David Gray, Jeffrey Cole, and Laurel Symes for editing and providing feedback to the manuscript. We thank Kai Murphey, Kate Chaumont, Christopher Hall, Clayton Lindsay, Claudia Ebbecke, and Kayla Murphy for their help with the insect culture. The research was funded by grants from the National Science Foundation awarded to OMB (IOS 1755118), TK (IOS 1755260) and JS (IOS 1755175).
The authors declare that the research was conducted in the absence of any commercial or financial relationships that could be construed as a potential conflict of interest.
All claims expressed in this article are solely those of the authors and do not necessarily represent those of their affiliated organizations, or those of the publisher, the editors and the reviewers. Any product that may be evaluated in this article, or claim that may be made by its manufacturer, is not guaranteed or endorsed by the publisher.
The Supplementary Material for this article can be found online at: https://www.frontiersin.org/articles/10.3389/fevo.2023.1216463/full#supplementary-material
Agrawal A. A. (2001). Phenotypic plasticity in the interactions and evolution of species. Science 294 (5541), 321–326. doi: 10.1126/science.1060701
Arnegard M. E., McIntyre P. B., Harmon L. J., Zelditch M. L., Crampton W. G., Davis J. K., et al. (2010). Sexual signal evolution outpaces ecological divergence during electric fish species radiation. Am. Nat. 176, 335–356. doi: 10.1086/655221
Beckers O. M. (2008). The evolutionary significance of developmental plasticity in the communication system of Neoconocephalus triops (Orthoptera: Tettigoniidae) (Columbia, MO, United States: University of Missouri-Columbia).
Beckers O. M., Murphey K. J., Pease J. R., Norman N. (2019). Parallel plasticity of mating songs and preferences in the field cricket Gryllus rubens. Ethology 125 (7), 476–484. doi: 10.1111/eth.12872
Beckers O. M., Schul J. (2008). Developmental plasticity of mating calls enables acoustic communication in diverse environments. Proc. R. Soc Lond. B 275, 1243–1248. doi: 10.1098/rspb.2007.1765
Beckers O. M., Schul J. (2010). Female adaptation to developmental plasticity in male calling behavior. Behavioral ecology and sociobiology. J. Exp. Biol. 64 (8), 1279–1290. doi: 10.1007/s00265-010-0942-z
Bozinovic F., Pörtner H. O. (2015). Physiological ecology meets climate change. Ecol. Evol. 5 (5), 1025–1030. doi: 10.1002/ece3.1403
Bush S. L., Schul J. (2010). Evolution of novel signal traits in the absence of female preferences in Neoconocephalus katydids (Orthoptera, Tettigoniidae). PloS One 5 (8), e12457. doi: 10.1371/journal.pone.0012457
Buzsáki G., Wang X. J. (2012). Mechanisms of gamma oscillations. Annu. Rev. Neurosci. 35, 203–225. doi: 10.1146/annurev-neuro-062111-15044
Campbell-Staton S. C., Cheviron Z. A., Rochette N., Catchen J., Losos J. B., Edwards S. V. (2017). Winter storms drive rapid phenotypic, regulatory, and genomic shifts in the green anole lizard. Science 357 (6350), 495–498. doi: 10.1126/science.aam5512
Caplat P., Cheptou P. O., Diez J., Guisan A., Larson B. M., Macdougall A. S., et al. (2013). Movement, impacts and management of plant distributions in response to climate change: insights from invasions. Oikos 122 (9), 1265–1274. doi: 10.1111/j.1600-0706.2013.00430.x
Collins J. P., Cheek J. E. (1983). Effect of food and density on development of typical and cannibalistic salamander larvae in Ambystoma tigrinum nebulosum. Am. Zool. 23 (1), 77–84. doi: 10.1093/icb/23.1.77
Debat V., David P. (2001). Mapping phenotypes: canalization, plasticity, and developmental stability. Trends Ecol. Evol. 16 (10), 555–561. doi: 10.1016/S0169-5347(01)02266-2
Eberhard W. G., Gutiérrez E. E. (1991). Male dimorphisms in beetles and earwigs and the question of developmental constraints. Evolution 45 (1), 18–28. doi: 10.1111/j.1558-5646.1991.tb05262.x
Franklin C. C., Ball J. M., Schulz D. J., Nair S. S. (2010). Generation and preservation of the slow underlying membrane potential oscillation in model bursting neurons. J. Neurophysiol. 104, 1589–1602. doi: 10.1152/jn.00444.2010
Frederick K. H., Schul J. (2016). Character State reconstruction of call trait diversity in Neoconocephalus katydids reveals multiple independent origins of derived call traits. PLoS Curr. 8. doi: 10.1371/currents.tol.0c5d76728d73ef9c3dbe8065f70ea4cb
Gerhardt H. C., Huber F. (2002). Acoustic communication in insects and anurans (Chicago: University of Chicago Press).
Ghalambor C. K., McKay J. K., Carroll S. P., Reznick D. N. (2007). Adaptive versus non-adaptive phenotypic plasticity and the potential for contemporary adaptation in new environments. Funct. Ecol. 21 (3), 394–407. doi: 10.1111/j.1365-2435.2007.01283.x
Grace J. L., Shaw K. L. (2004). Effects of developmental environment on signal preference coupling in a Hawaiian cricket. Evolution 58, 1627–1633. doi: 10.1111/j.0014-3820.2004.tb01744.x
Greenfield M. D. (1990). “Evolution of acoustic communication in the genus Neoconocephalus: discontinuous songs, synchrony, and hetereospecific interactions,” in The Tettigoniidae: biology, systematics and evolution. Eds. Bailey W. J., Rentz D. C. F. (Berlin, Germany: Springer-Verlag), 71–97.
Hudson A. E., Prinz A. A. (2010). Conductance ratios and cellular identity. PloS Comput. Biol. 6, e1000838. doi: 10.1371/journal.pcbi.1000838
Hughes L. (2000). Biological consequences of global warming: is the signal already apparent? Trends Ecol. Evol. 15 (2), 56–61. doi: 10.1016/S0169-5347(99)01764-4
Hutcheon B., Yarom Y. (2000). Resonance, oscillation and the intrinsic frequency preference of neurons. Trends Neurosci. 23, 216–222. doi: 10.1016/S0166-2236(00)01547-2
Izhikevich E. M. (2001). Resonate-and-fire neurons. Neural. Netw. 14, 883–894. doi: 10.1016/S0893-6080(01)00078-8
Jacob P. F., Hedwig B. (2016). Acoustic signalling for mate attraction in crickets: Abdominal ganglia control the timing of the calling song pattern. Behav. Brain. Res. 309, 51–66. doi: 10.1016/j.bbr.2016.04.025
Marder E. (2000). Motor pattern generation. Curr. Opin. Neurobiol. 10, 691–698. doi: 10.1016/S0959-4388(00)00157-4
McInerny G. J., Etienne R. S. (2012). Stitch the niche–a practical philosophy and visual schematic for the niche concept. J. Biogeogr. 39 (12), 2103–2111. doi: 10.1111/jbi.12032
Moczek A. P. (2003). The behavioral ecology of threshold evolution in a polyphenic beetle. Behav. Ecol. 14 (6), 841–854. doi: 10.1093/beheco/arg062
Moran E. V., Alexander J. M. (2014). Evolutionary responses to global change: lessons from invasive species. Ecol. Lett. 17 (5), 637–649. doi: 10.1111/ele.12262
Olvido A. E., Mousseau T. A. (1995). Effect of rearing environment on calling song plasticity in the striped ground cricket. Evolution 49 (6), 1271–1277. doi: 10.2307/2410452
Paaby A. B., Rockman M. V. (2014). Cryptic genetic variation: Evolution's hidden substrate. Nat. Rev. Genet. 15 (4), 247–258. doi: 10.1038/nrg3688
Pfennig D. W. (1992). Polyphenism in spadefoot toad tadpoles as a locally adjusted evolutionarily stable strategy. Evolution 46 (5), 1408–1420. doi: 10.1111/j.1558-5646.1992.tb01133.x
Rowland J. M., Emlen D. J. (2009). Two thresholds, three male forms result in facultative male trimorphism in beetles. Science 323 (5915), 773–776. doi: 10.1126/science.1167345
Rutherford S. L. (2000). From genotype to phenotype: buffering mechanisms and the storage of genetic information. Bioessays 22 (12), 1095–1105. doi: 10.1002/1521-1878(200012)22:12<1095::AID-BIES7>3.0.CO;2-A
Rutherford S. L., Lindquist S. (1998). Hsp90 as a capacitor for morphological evolution. Nature 396 (6709), 336–342. doi: 10.1038/24550
Schöneich S., Hedwig B. (2011). Neural basis of singing in crickets: central pattern generation in abdominal ganglia. Naturwissenschaften 98, 1069–1073. doi: 10.1007/s00114-011-0857-1
Schul J., Bush S. L., Frederick-Hudson K. H. (2013). “Evolution of call patterns and pattern recognition mechanisms in Neoconocephalus katydids,” in Animal Signals and Communication - Topics in insect hearing and acoustic communication. Ed. Hedwig G. (New York: Springer Verlag, Heidelberg), 176–183.
Schulz D. J., Goaillard J.-M., Marder E. E. (2007). Quantitative expression profiling of identified neurons reveals cell-specific constraints on highly variable levels of gene expression. Proc. Natl. Acad. Sci. U.S.A. 104, 13187–13191. doi: 10.1073/pnas.0705827104
Seebacher F., White C. R., Franklin C. E. (2015). Physiological plasticity increases resilience of ectothermic animals to climate change. Nat. Clim Chang 5 (1), 61–66. doi: 10.1038/nclimate2457
Shapiro A. M. (1976). Seasonal polyphenism. Evol. Biol. 9, 259–333. doi: 10.1007/978-1-4615-6950-3_6
SINA (2023) Singing Insects of North America. Available at: https://sina.orthsoc.org/index.htm.
Waddington C. H. (1961). Genetic assimilation. Adv. Genet. 10, 257–290. doi: 10.1016/S0065-2660(08)60119-4
Walker T. J. (1975). Stridulatory movements in eight species of Neoconocephalus (Tettigoniidae). J. Insect. Physiol. 21 (3), 595–603. doi: 10.1016/0022-1910(75)90163-8
Walker T. J. (2000). Pulse rates in the songs of trilling field crickets (Orthoptera: Gryllidae: Gryllus). Entom. Soc Am. 93 (3), 565–572. doi: 10.1603/0013-8746(2000)093[0565:PRITSO]2.0.CO;2
Walker T. J., Greenfield M. D. (1983). Songs and systematics of Caribbean neoconocephalus (Orthoptera: tettigoniidae). Trans. Am. Entomol. Soc 109, 357–389.
West-Eberhard M. J. (2005). Developmental plasticity and the origin of species differences. Proc. Natl. Acad. Sci. U.S.A. 102, 6543–6549. doi: 10.1073/pnas.0501844102
West-Eberhardt M. J. (2003). Developmental Plasticity and Evolution (Inc., New York: Oxford University Press).
Whitesell J. J. (1969). Biology of United States coneheaded katydids of the genus Neoconocephalus (Orthoptera: Tettigoniidae) (Gainesville, FL, USA: M.S. Thesis, University of Florida).
Whitesell J. J. (1974). Geographic variation and dimorphisms in song, development, and color in a katydid: field and laboratory studies (Tettigoniidae, Orthoptera) (Gainesville, FL, United States: Ph.D. thesis at the University of Florida).
Keywords: homeostasis, signal evolution, communication, diapause, development
Citation: Beckers OM, Kijimoto T and Schul J (2023) Phenotypic plasticity of male calls in two populations of the katydid Neoconocephalus triops (Insecta: Tettigoniidae). Front. Ecol. Evol. 11:1216463. doi: 10.3389/fevo.2023.1216463
Received: 03 May 2023; Accepted: 20 September 2023;
Published: 24 October 2023.
Edited by:
David Andrew Gray, California State University, Northridge, United StatesReviewed by:
Jeffrey Cole, Pasadena City College, United StatesCopyright © 2023 Beckers, Kijimoto and Schul. This is an open-access article distributed under the terms of the Creative Commons Attribution License (CC BY). The use, distribution or reproduction in other forums is permitted, provided the original author(s) and the copyright owner(s) are credited and that the original publication in this journal is cited, in accordance with accepted academic practice. No use, distribution or reproduction is permitted which does not comply with these terms.
*Correspondence: Oliver M. Beckers, b2JlY2tlcnNAbXVycmF5c3RhdGUuZWR1
Disclaimer: All claims expressed in this article are solely those of the authors and do not necessarily represent those of their affiliated organizations, or those of the publisher, the editors and the reviewers. Any product that may be evaluated in this article or claim that may be made by its manufacturer is not guaranteed or endorsed by the publisher.
Research integrity at Frontiers
Learn more about the work of our research integrity team to safeguard the quality of each article we publish.