- Department of Biological Sciences, Simon Fraser University, Burnaby, BC, Canada
The human skin microbiome reportedly contributes to the attraction of mosquitoes to human hosts. We tested the hypothesis that bovine skin microbes affect the attraction of blood-feeding stable flies, Stomoxys calcitrans, to their bovine hosts. Microbes were collected from a calf and adult cow, and subsequently isolated and identified by mass spectrometry and genetic sequencing. Separate groups of (i) four Staphylococcus congeners (S. chromogenes, S. sciuri, S. simulans, S. succinus) and (ii) three bacterial heterogeners (Glutamicibacter protophormiae, Corynebacterium stationis, Wautersiella sp.) grown on agar, each attracted flies in still-air olfactometers, as did each Staphylococcus congener singly. The four Staphylococcus microbes also attracted flies in room bioassays. In greenhouse bioassays with paired black barrels as visual (surrogate host) stimuli, the treatment barrel baited with S. sciuri on agar induced significantly more fly alighting responses than the control barrel with sterile agar. This treatment effect could not be demonstrated on a cattle farm, possibly because of chemically and visually complex surroundings. Ammonia emitted by Staphylococcus microbes attracted flies, and a synthetic blend of microbe odorants enhanced the attractiveness of ammonia. Optimal attraction of stable flies to bovine microbes likely requires the integration of multimodal host cues.
1 Introduction
To locate vertebrate hosts, hematophagous insects exploit multiple host cues (Marzal et al., 2022), including carbon dioxide (CO2) (Takken, 1991; Anderson et al., 2009; Milne et al., 2009; Indacochea et al., 2017), breath volatiles (Warnes and Finlayson, 1985), body-derived odor (Ortiz and Molina, 2010), moisture and heat (Cribellier et al., 2020), infrared (IR) radiation (Schmitz et al., 2000), as well as visual cues such polarized light reflections from dark-coloured fur (Horvath et al., 2017; Meglic et al., 2019). The relative importance of host cues depends on the insect taxon and the spatial scale. For stable flies, Stomoxys calcitrans, visual host cues seem particularly important (Murchie et al., 2018; Onju et al., 2020; Sharif et al., 2020; Blake et al., 2023), and are likely sensed over a long range. For mosquitoes, host cues such as body heat, skin odor and moisture are most important at close to intermediate ranges (Marzal et al., 2022). Female mosquitoes respond to host chemical and physical cues in sequential and interactive processes. Exhaled in the breath of a potential host, CO2 context-specifically elicits host-seeking behavior (Gillies, 1980), induces upwind flight toward the CO2 source (Healy and Copland, 1995), and enhances attraction to warmth (Liu and Vosshall, 2019). In addition to exhaled CO2 and breath volatiles, odorants emanating from bacteria on human skin guide host-foraging mosquitoes (Showering et al., 2022).
The common human skin bacteria Staphylococcus epidermidis, Corynebacterium minutissimum, and Bacillus subtilis emit odorants that attract Anopheles gambiae mosquitoes (Verhulst et al., 2010). Skin microbiota differ among humans and thus affect their relative attractiveness to mosquitoes (Showering et al., 2022). Humans most attractive to A. gambiae have high densities of skin microbes and great abundance of Staphylococcus spp., suggesting that Staphylococcus spp. contribute to the attractiveness of humans to mosquitoes (Verhulst et al., 2011). As microbes produce species- or strain-specific odor blends (Green et al., 2014; Peach et al., 2021), it follows that the species composition of skin microbiomes also affects its odor profile and thus the attractiveness of humans to host-seeking insects. With the human skin microbiome known to affect mosquito attraction and host recognition (Verhulst et al., 2011), it is conceivable that the skin microbiomes of other vertebrates, such as cattle (Zinicola et al., 2015a), may also affect their attractiveness to blood-feeding insects including stable flies, which are major pests of cattle in livestock production industries. Repeated biting by flies reduces weight gain and milk production (Bruce and Decker, 1958; Campbell et al., 1977; Campbell et al., 2001), causing billions of dollars in economic losses per year (Taylor et al., 2012).
Skin microbiota of cattle have been extensively investigated (Winther et al., 2022), particularly within the context of bacterial infections that cause diseases such as mastitis (Andrews et al., 2019; De Buck et al., 2021) and bovine digital dermatitis (Zinicola et al., 2015a; Zinicola et al., 2015b; Nielsen et al., 2016; Espiritu et al., 2020; Caddey and De Buck, 2021; Caddey et al., 2021). Bacteria and their volatile odorant and gas emissions have also been shown to attract stable flies to oviposition sites and to induce oviposition (Romero et al., 2006; Albuquerque and Zurek, 2014; Scully et al., 2017). However, whether cattle skin microbes attract stables flies to their cattle hosts has not yet been investigated.
Here, we tested the hypothesis that skin-dwelling microbes of cattle contribute to the attraction of stable flies to cattle hosts. To this end, we (1) identified skin-dwelling microbes of cattle, (2) tested select microbes for their attractiveness to flies, and (3) investigated mechanisms underlying the attraction of flies to bioactive microbes.
2 Material and methods
2.1 Rearing of experimental flies
Flies were housed in a hyperbolic growth chamber (BioChambers Inc., Winnipeg, MB, CA) on the Burnaby campus of Simon Fraser University. They were fed citrated bovine blood twice a day and provided cloth oviposition sites at least 3 times per week. Eggs were transferred to a larval rearing medium, containing wood chips (200 g; Hyon Bedding, Prince George, BC, CA), wheat bran (500 g; Rogers Foods, Armstrong, BC, CA), staple fish food (115 g; Nutrafin, Montreal, QC, CA), and a solution of ammonium bicarbonate (50 g) dissolved in 1600–2000 mL of water (Friesen et al., 2018). Reared flies were separated by sex based on morphological and sex-specific characteristics, with males having a visually larger dark spot at the base of their abdomen than females, and females protruding their ovipositor when abdomens are gently squeezed with a pair of forceps. Only female flies, 7–11 days old, were tested in laboratory bioassays because females – proportionally – responded better than males in pre-screening bioassays.
2.2 Identification of microbes on cattle skin
2.2.1 Collection and isolation of microbes
Microbes were collected from a live cow calf (with consent and in the presence of the animal’s owner), and from the hide of a recently slaughtered adult cow. Samples were obtained from a front leg, back leg, and the back of both the live calf and the cow hide, because these areas are most frequented by stable flies. For microbe collections, cotton swabs (Puritan, Guilford, ME, USA) were dipped in sterile distilled water and then were firmly rubbed for 30 s against an approximately 6.5-cm2 patch of cow skin/hide before being streaked for 30 s over the entire surface of Mueller Hinton, Yeast Extract, or Potato Dextrose agars in separate Petri dishes (d = 8.5 cm). These different agar types were meant to enable growth of as many bacterial species as possible. One sample was obtained from each body region per agar type, yielding nine samples each from the live calf and the cow hide. Morphologically distinct microbes growing on agar were isolated by continuous re-streaking. All microbial work was done in a biosafety cabinet (BSC; NUAIRE Biological Safety Cabinets, Class II type A2) using aseptic techniques. Microbe stock-samples were kept at −80°C in a solution of glycerol, distilled water, and liquid microbe culture (1:1:2).
2.2.2 Identification of microbes
Isolated microbes were identified using either Matrix Assisted Laser Desorption/Ionization Time of Flight Mass Spectrometry (MALDI-TOF MS) or genetic sequencing.
MALDI-TOF MS (Bruker Corp., Billerica, MA, USA) (Jimenez et al., 2017) was conducted using an extended Direct Transfer method. For each bacterial strain, two preparations were processed. Briefly, after growing unknown bacteria on agar overnight, single colonies were transferred to a well on a MALDI plate via a sterile toothpick, producing a heavy smear on the well. The same toothpick was then used to produce a lighter smear on the next well. Subsequently, 1 µL of 70% formic acid was applied to all microbe-treated wells and allowed to evaporate. Finally, each well received 1 µL of 2-cyano-3-(4-hydroxyphenyl) acrylic acid (HCCA matrix). After wells had dried, MALDI Biotyper measurements were taken. We used a Bruker bacterial test standard (Bruker Corp.) for calibration in accordance with manufacturer instructions. We analyzed all spectra using Biotyper software (Bruker Corp.). This Biotyper software calculates an arbitrary score for each sample between 0 and 3 by comparing sample mass spectra to reference mass spectra; we accepted species assignments at scores of >2.0 in accordance with the manufacturer’s recommended protocol.
In preparation for genetic sequencing of distinct bacterial colonies, Kodaq PCR Master Mix (Applied Biological Materials, Richmond, BC, CA) was used to amplify the V3-V4 loop of the 16S rRNA gene with the Universal Forward Primer (UniF) – 5’-CCTACGGGRBGCASCAG-3’ and the Universal Reverse Primer (UniR) – 5’-GGACTACNNGGGTATCTAAT-3’ (Takai and Horikoshi, 2000) by Polymerase Chain Reactions (PCR). Briefly, for a single colony of any unknown bacterium, a PCR mix (25 µL) was prepared using (i) 12.5 µL of Kodaq PCR Master Mix, (ii) 1 µL of UniF, (iii) 1 µL of UniR, and (iv) 10.5 µL of molecular grade water. The mix was then deposited in a PCR strip tube and a single colony of the unknown bacterium was added. A PTC-200 Peltier Thermal Cycler (MJ Research, Saint-Bruno-de-Montarville, QC, CA) was deployed to run PCR cycles on all samples. The program was set to 94°C for 2 min, followed by thirty 30-s cycles each at 94°C, 55°C, and 72°C, and a final extension step at 72°C for 5 min.
The presence of the expected band size at 466 base pairs (bps) and the success of the PCR amplification was checked on a 0.7% agarose gel with a 1 kb Plus Opti DNA Marker (ladder) (Applied Biological Materials). PCR amplicons were pooled and concentrated using the QIAquick Gel Extraction Kit and the QIA PCR & Gel Cleanup Kit (Qiagen, Venlo, NL). Amplicons were sequenced (Genewiz, South Plainfield, NJ, USA) and the Basic Local Alignment Search Tool (BLAST) (Altschul et al., 1990) was used to compare the sequenced region of individual isolates with known sequences. A species or genus was determined to be a match, if there was at least 95% coverage and 99% identity between a known sequence and the sequenced isolate.
2.3 Testing of select microbes for their attractiveness to flies
2.3.1 Still-air olfactometer bioassays – general experimental design
Bioassays were run in still-air olfactometers, each with a central and two lateral chambers (Figure 1A), where treatment and control stimuli were placed. For each experimental replicate, 20 female flies that had been blood- and water-deprived for 24 h were released into the central chamber from which they could enter, but not exit, lateral chambers through mesh funnels. Experimental replicates were terminated and scored after 24 h by placing olfactometers in a freezer (−15°C) and counting the cold-euthanized flies in each chamber.
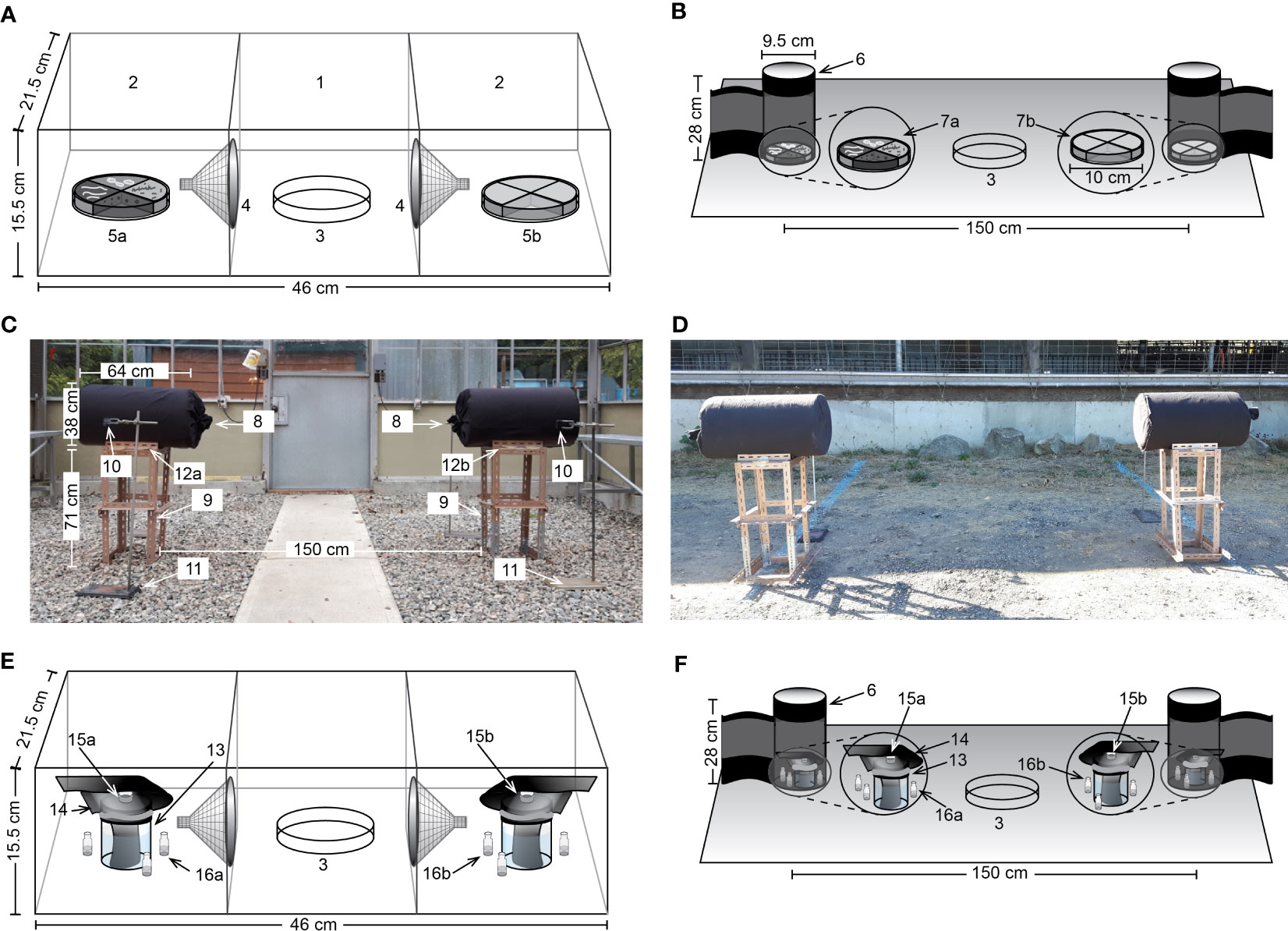
Figure 1 Graphic and photographic illustrations of experimental designs. (A) Three-chamber still-air olfactometer with one central (1) and two lateral chambers (2). Flies were released from a petri dish (3) and entered lateral chambers via mesh funnels (4) in response to treatment or control stimuli (5a, 5b). Treatment stimuli consisted of agar slices inoculated, or not (control), with various bacteria (Exps. 1–12; Table 2). (B) Trap design and placement in a bioassay room (225 × 230 × 230 cm). Flies were released from a petri dish (3) and captured on cardstock cylinders with an adhesive-coated inner surface (6). The treatment stimulus (7a) consisted of four agar slices each inoculated with Staphylococcus chromogenes, S. sciuri, S. simulans or S. succinus, whereas the control stimulus (7b) consisted of corresponding sterile agar slices (Exp. 13). (C) Experimental design employed in a greenhouse compartment (600 × 600 × 360 cm). Alightings of flies on paired black barrels (8) residing on metal stands (9) were recorded by four cameras (10; two shown) mounted on laboratory stands (11). Barrels were baited with three agar plates inoculated, or not (control), with S. sciuri (12a, 12b) (Exp. 14), with two and one plate, respectively, secured at the barrels’ curved front and back sides. (D) Experimental design in a field setting. Treatment stimuli consisted of agar plates (12, 4, 2 or 1) inoculated, or not (control), with S. sciuri (Exps. 15–18). (E) Three-chambered still-air olfactometer, with test stimuli consisting of a jar filled three-quarters with water (13) in which a black cloth (14) was submerged, secured with a rubber band, and wrapped around the jar top carrying an inverted bottle cap (15a, 15b) filled with a treatment or a control stimulus (Exps. 19–21; Table 2). In experiments 22-24, both bottle caps (15a, 15b) contained the same stimulus (see Table 2), and both lateral chambers were fitted with three vials containing mineral oil, with treatment vials (16a), but not control vials (16b), releasing a synthetic Staphylococcus volatile blend (Table S1). (F) Trap design and placement as in subpanel (B) with test stimuli similar to those in subpanel (E) except that a synthetic S. sciuri volatile blend was tested (Table 2, Exp. 23; Table S1).
2.3.2 Still-air olfactometer bioassays – specific experiments (Exps. 1–12)
Of the 38 microbes collected from cattle skin and identified to genus and/or species (see Results; Table 1), those previously reported on vertebrate skin were tested for their attractiveness to stable flies. These microbes included four Staphylococcus congeners [S. sciuri, S. succinus, S. simulans, S. chromogenes (Group 1)] and three heterogeners [Glutamicibacter protophormiae, Corynebacterium stationis, Wautersiella sp. (Group 2)]. For Experiment 1 (n = 12) (Table 2), each of the four Group-1 Staphylococcus species was grown overnight on agar in a petri dish (d = 8.5 cm), and then one quarter slice of the agar from each species was placed in a new sterile petri dish serving as the treatment stimulus, whereas four slices of sterile agar served as the control stimulus. Agar was sliced, and slices were transferred, using sterile (autoclaved) popsicle sticks. Test stimuli involving Risk Group 2 microbes (Government of Canada) were prepared either near a flame or in a biosafety cabinet. For Experiment 2 (n = 12), the same procedure was applied with the three Group-2 microbes except that only three quarter slices were used for the treatment stimulus and three corresponding sterile slices for the control stimulus.
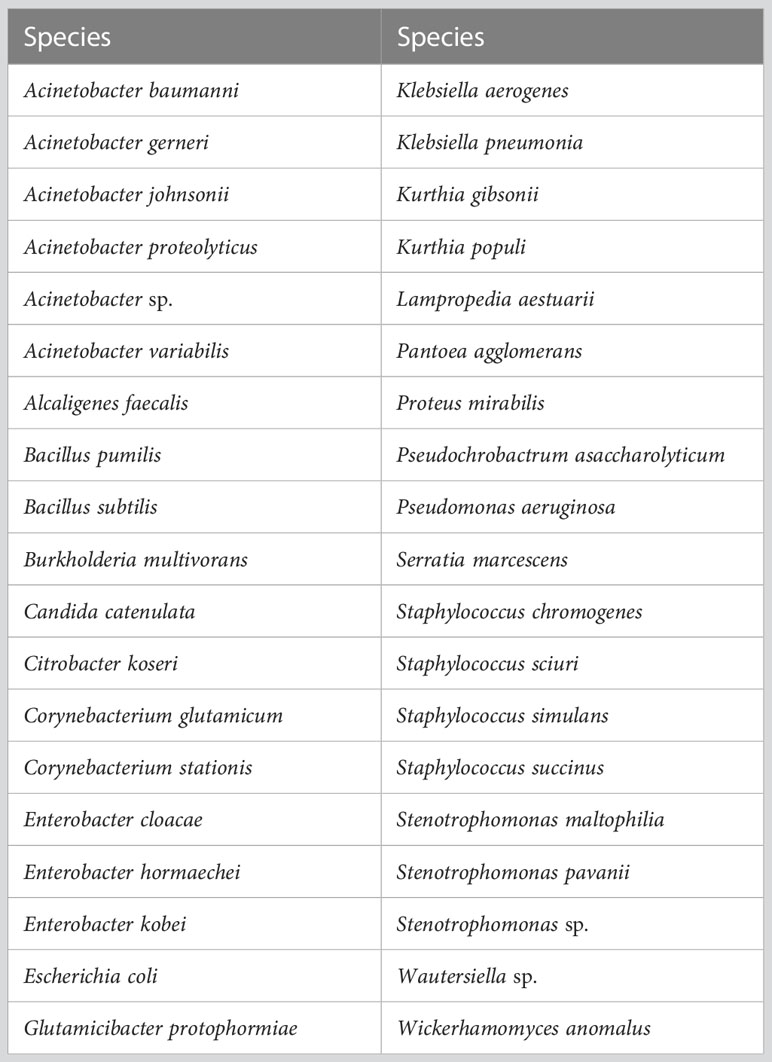
Table 1 List of microbes isolated from the skin of a live calf and/or the hide of a recently slaughtered adult cow and identified to the genus and/or species level.
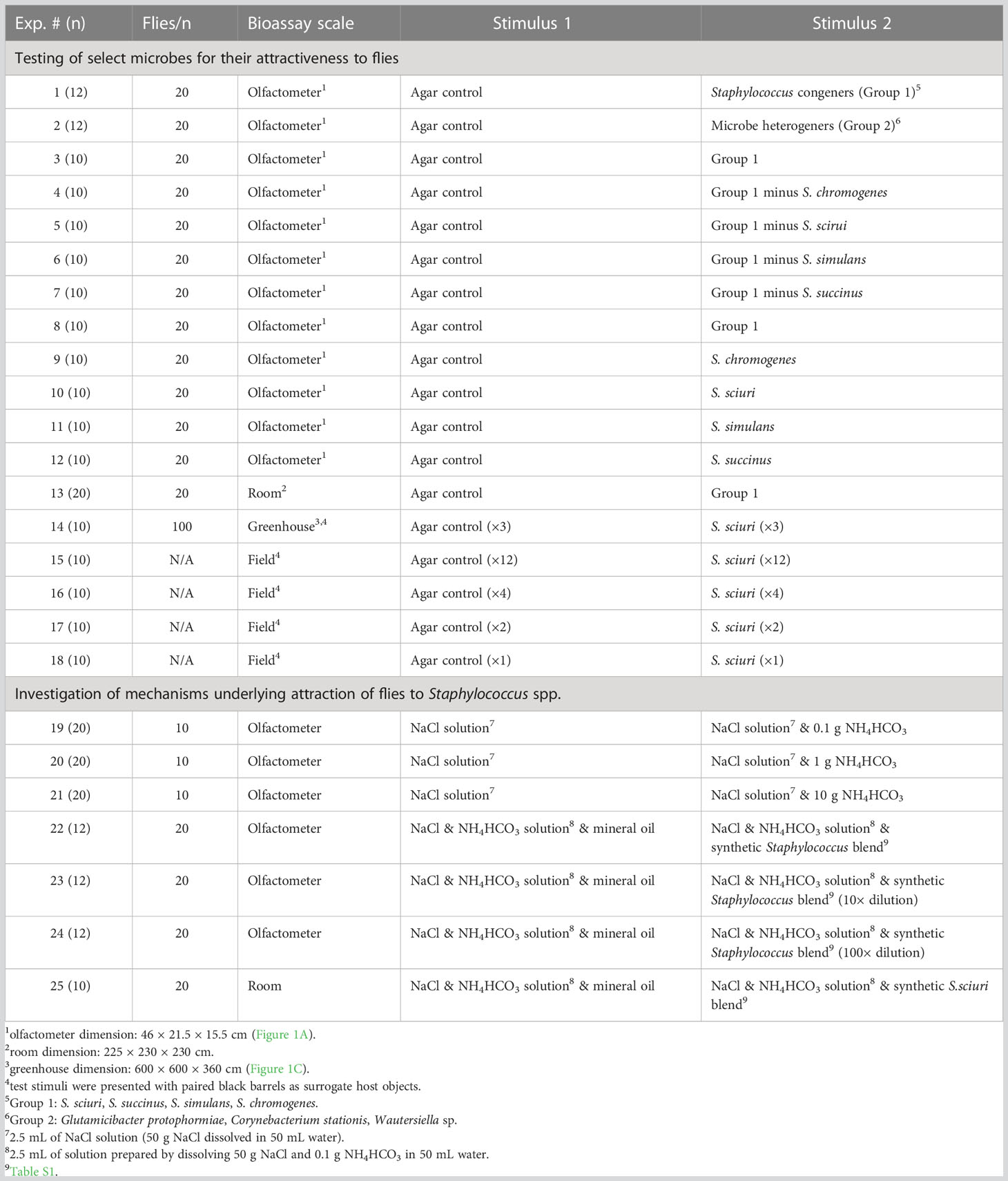
Table 2 Summary of experiments (Exp.) and number of replicates (n) run, numbers of flies tested per replicate (flies/n), the bioassay scale (still-air 2-choice olfactometer, room, greenhouse, field; see Figure 1), and the stimuli tested.
Drawing on results that treatment stimuli in both experiments 1 and 2 attracted significantly more flies than corresponding control stimuli (see Results; 3.2.1), but that Group-1 microbes seemed more attractive (mean treatment to control response ratios: Group 1: 9.5 to 1; Group 2: 4.4 to 1), follow-up experiments were designed to determine the key microbe(s) in Group 1 that mediated attraction of flies. To this end, parallel experiments 3–7 (n = 10 each) tested all four Group-1 microbes (Exp. 3; positive control), and Group-1 microbes without S. chromogenes (Exp. 4), S. sciuri (Exp. 5), S. simulans (Exp. 6), or S. succinus (Exp. 7), all versus sterile agar controls. With evidence that the deletion of any one Staphylococcus species from Group 1 did not reduce the Group’s attractiveness (see Results; 3.2.1), experiments 8–12 (n = 10 each) then tested the four Group-1 Staphylococcus microbes in combination (Exp. 8; positive control) and singly (Exps. 9–12), again all versus sterile agar controls.
2.3.3 Room bioassay (Exp. 13)
To determine whether Group-1 Staphylococcus microbes attract stable flies not only in small scale olfactometers but also over a long range, experiment 13 (n = 20) was set up in a laboratory room (230 × 230 × 225 cm high), with the treatment stimulus (one quarter agar slice of each of the four Staphylococcus microbes) and the control stimulus (sterile agar) prepared as described for experiment 1 (see 2.3.2). The room was kept at a temperature of approximately 26°C and lit by a combination of plant illumination lights (Standard Products Inc., Saint-Laurent, QC, CA) and day lights (Philips, Amsterdam, NL), set to maintain a photoperiod of 15 h L: 9 h D. The treatment and control plates were then placed on the room floor and surrounded by vertical black cardstock cylinders (9.5 × 28 cm high) (Figure 1B), which were coated on the inside with adhesive Tanglefoot® (Tanglefoot, Marysville, USA). For each experimental replicate, 20 flies were released into the room, and fly captures in the cardboard cylinder traps were recorded 24 h later.
2.3.4 Greenhouse bioassay (Exp. 14)
To test whether a Group-1 microbe affects the responses of flies not only in a room setting (see Results; 3.2.2) but also on an even larger scale, experiment 14 (n = 10) was set up in a greenhouse compartment (600 × 600 × 360 cm high) on the Burnaby campus of Simon Fraser University. Staphylococcus sciuri was selected for greenhouse bioassays, and subsequent field bioassays (see below), because it was as attractive as either one, and all four, of the Group-1 microbes (see Results; 3.2.1), and because it was the safest microbe (Risk Group 1 microbe; Government of Canada) for deployment in large-scale settings, being deemed unlikely to cause human or animal diseases.
In the greenhouse bioassay, the paired test stimuli consisted of barrels (38 × 64 cm high) covered in black cloth and placed on metal platforms 71 cm above ground, and 200 cm apart from each other (Figure 1C). In each replicate, three agar plates were secured with double-sided tape to each platform, with two plates at the front and one plate at the back of the barrels’ curved surface. Treatment and control plates were covered in S. sciuri (grown overnight) and kept sterile, respectively. To record alighting by flies on barrels and agar plates, video cameras (Akaso, Frederick, MD, USA) were mounted on stands 83 cm above ground and 100 cm away from both the front and the back of each barrel. To initiate a bioassay replicate, the cameras were turned on, and 100 blood- and water-deprived female flies were released into the compartment, 300 cm away from treatment and control stimuli. Ten minutes later, video recordings were stopped and flies were sweep-netted and released outside. Videos were subsequently examined to determine the number of times flies landed on each of the two barrels over the bioassay period.
2.3.5 Field bioassays (Exps. 15–18)
With evidence that S. sciuri affected alighting responses by flies in the greenhouse compartment (see Results; 3.2.3), experiments 15–18 (n = 10 each) then tested the effect of S. sciuri on fly attraction at a livestock farm (Eagle Acres Dairy; Langley, BC, CA). A near-identical experimental design (Figure 1D) as in the greenhouse experiment was used, except that the platform of each paired barrel was fitted with 12 plates (Exp. 15), 4 plates (Exp. 16), 2 plates (Exp. 17), and 1 plate (Exp. 18). Barrels were placed approximately 300 cm away from the barn in all experiments. Experimental replicates were terminated after 5 min, and positions of treatment and control barrels were alternated between replicates.
2.4 Investigation of mechanisms underlying attraction of flies to Staphylococcus spp.
2.4.1 Evidence for ammonia emission from Staphylococcus microbes
Having shown that ammonia serves as an oviposition resource cue to stable flies (Nayani et al., unpubl.), here we tested whether ammonia also functions as a host-foraging cue for flies. To this end, we tested whether Staphylococcus microbes collected from cattle skin emit ammonia, and whether ammonia attracts host-foraging flies in bioassays. To test for ammonia emission, we grew microbes on agar overnight and measured ammonia emission, using a MultiRAE Wireless Portable Six-Gas Monitor (Honeywell, Charlotte, NC, USA). Three plates of each microbe were grown for measurement of gas emissions. Measurements were taken by placing the probe of the gas meter 0.5–1.0 cm above an agar plate, waiting 1 min for readings to stabilize, and then recording the ppm of ammonia. Between replicates, the gas meter was kept in regular laboratory air for 1 min, thus allowing readings to return to baseline ammonia levels in the atmosphere.
2.4.2 Ammonia bioassays in still-air olfactometers (Exps. 19–21)
With evidence that microbes attractive to flies emit ammonia (see Results; Table 3), parallel still-air olfactometer experiments 19–21 (n = 20 each) then tested whether ammonia on its own is attractive to host-foraging flies. For both the treatment and the control stimulus in these experiments, sodium chloride solutions (50 g NaCl in 50 mL water) were prepared, with treatment solutions also containing ammonium bicarbonate (NH4HCO3) at 0.1 g (Exp. 19), 1 g (Exp. 20), or 10 g (Exp. 21). Aliquots (3 mL) of treatment solutions were pipetted into inverted bottle caps (2.5 × 1 cm; total volume: 4.9 mL), and ammonia ppm for each NH4HCO3 dose (0.1 g, 1.0 g or 10 g in 50 mL water) was measured 0.5 cm above the liquid surface. The mean ammonia ppm measured at 0.1 g, 1.0 g, and 10 g in seven replicates each was 0.42 ppm, 5.6 ppm, and >25 ppm (sensor overload), respectively, well within the ppm range of Staphylococcus microbes growing on agar (Table 3). Carbon dioxide ppm was not measured.
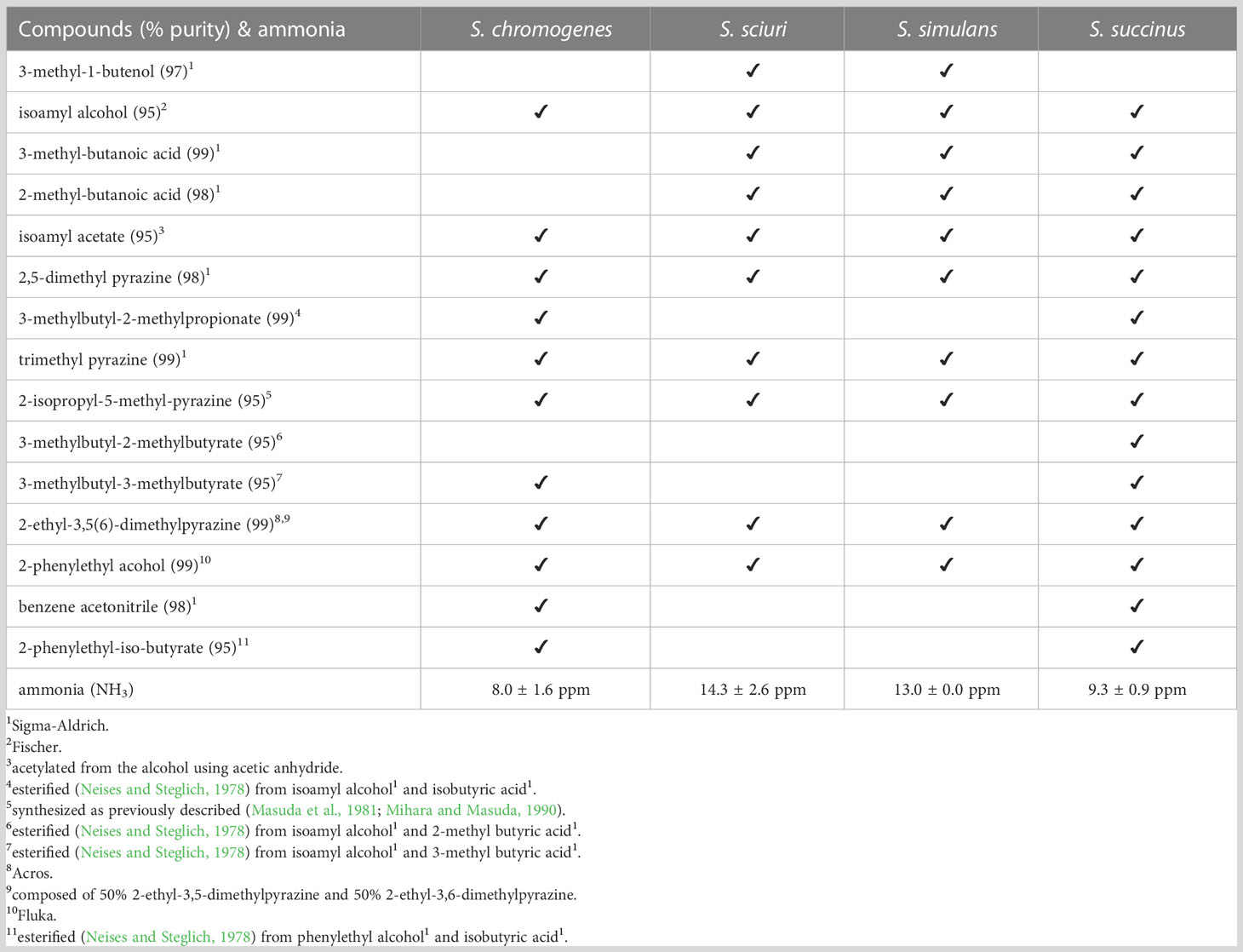
Table 3 List of volatiles identified, and ppm ammonia measured, in the headspace of four Staphylococcus microbes attractive to stable flies.
Aliquots (2.5 mL) of treatment and control solutions were transferred to inverted bottle caps (see above), placed on jars (5.5 × 7 cm; total volume = 166 mL) filled two-thirds with water, and covered with a piece of wet black cloth (Figure 1E) (Nayani et al., unpubl.; Friesen et al., 2018). After treatment and control jars were randomly assigned to the lateral chambers of olfactometers, experimental replicates were initiated by releasing 20 blood- and water-deprived female flies into the central chamber of olfactometers, allowing them to enter, but not to exit, lateral chambers through mesh funnels. Replicates were terminated 24 h later by counting the number of flies in lateral treatment and control chambers.
2.4.3 Collection of microbe-derived headspace volatiles
Headspace volatiles were collected from the four strains of Staphylococcus bacteria that elicited significant behavioral responses from flies in olfactometer experiments. To this end, 10 agar plates were plated with a microbe of interest and incubated overnight. These plates, with open lids, were then placed into a glass chamber (diameter = 19 cm, height = 29.5 cm) connected to a vacuum pump (Neptune Dyna-pump). Charcoal-filtered air was drawn at a flow rate of 1 L · min−1 for 24 h through the chamber and subsequently through a glass column (6 mm outer diameter × 150 mm) containing 200 mg of manufacturer-preconditioned Porapak-Q™ adsorbent (50–80 mesh; Waters Associates, Milford, MA, USA). Volatiles were desorbed from Porapak-Q with one rinse of pentane and ether (1:1; 2 mL), and volatile extracts were concentrated to 0.5 mL and kept at 4°C prior to analyses. All glassware was cleaned with Sparkleen (Thermo Fisher Scientific, MA, U.S.A), rinsed with distilled water, and oven-dried at 130°C prior to starting a new aeration.
2.4.4 Analyses of microbe headspace volatiles by GC-MS
Aliquots of Porapak-Q headspace volatile extracts were analyzed by gas chromatography-mass spectroscopy (GC-MS), using an Agilent 5977 Series 96MDS coupled to an Agilent 7890B GC (Agilent Technologies Inc., Santa Clara, CA, USA). The instrument was operated in full-scan electron ionization mode and fitted with a DB-5 GC-MS column (30 m × 0.25 mm ID, film thickness 0.25 µm; Agilent Technologies). The injector port, MS source, and MS quadrupole were set to 250, 230, and 150°C, respectively. Helium was used as a carrier gas (35 cm s−1; 5:1 split ratio), with the following temperature program: 40°C (held 5 min), 10°C · min−1 to 280°C (held 10 min). Compounds were identified by comparing their mass spectra and retention indices [relative to aliphatic alkanes (Van Den Dool and Kratz, 1963)] with those of authentic standards that were purchased or synthesized in our laboratory (Table 3). Each compound was quantified by comparing its area count with that of an external standard run at 1, 10 and 100 ng/µL.
2.4.5 Behavioral experiments with synthetic microbe headspace volatiles (Exps. 22–25)
With evidence that microbe-derived ammonia attracts flies (see Results; 3.3.2), and that microbes also emit complex volatile blends (see Results, Table 3), experiments 22–25 were designed to test whether blends of synthetic microbial volatiles enhance the attractiveness of ammonia. Blend 1 consisted of all constituents common in the headspace of the four bioactive Staphylococcus species (Table 3, Table S1), whereas blend 2 consisted of all volatiles emitted by S. sciuri (Table 3, Table S1). Blends 1 and 2 were formulated separately in mineral oil to achieve sustained release of volatiles during the 24-h experimental period. Sustained release was tracked through capture and analyses of headspace volatiles from mineral oil formulations (as described in 2.4.4). Formulations were adjusted until their headspace blends matched those produced by bacteria. Both treatment and control stimuli consisted of ammonium bicarbonate solutions prepared and presented as in experiment 19 described above (see 2.4.2), whereas treatment stimuli also presented blend 1 or 2 in mineral oil (Table 3, Table S1), with plain mineral oil being the corresponding control stimulus.
To address potential effects of 3-dimensional scale on responses of flies (Nayani et al., unpubl.), experiments 22–24 (n = 12 each) were run in still-air olfactometers (Figure 1E), and experiment 25 (n = 10) was run in bioassay rooms (230 cm × 230 cm × 225 cm high) (Figure 1F), using experimental designs and protocols described in sections 2.3.1 and 2.3.3. As the bioactivity of synthetic volatile blends can be dose-dependent (Nyasembe et al., 2012; Wondwosen et al., 2021), we tested the synthetic “Staphylococcus blend” (Table S1) formulated in mineral oil at three doses in parallel experiments 22–24: the dose described in Table S1 (Exp. 22), diluted 10× (Exp. 23), and diluted 100× (Exp. 24). By the time we tested the synthetic “S. sciuri blend” (Table S1) in experiment 25, we did not know the results of experiments 22–24 (see Results 3.3.4), which would have prompted us to test the “S. sciuri blend” at a lower dose. However, re-running the S. sciuri blend at a lower dose was not possible due to logistic constraints.
2.5 Statistical analyses
Data of all experiments were analyzed with binomial generalized linear models (BGLMs), using quasibinomial errors to account for overdispersion (RStudio v4.1.1) (Crawley, 2007; R Studio Team, 2023; Nayani et al., unpubl.). These analyses compared an intercept-only model to a null model with a likelihood ratio test to determine whether the proportions of flies responding to treatment stimuli differed from a hypothetical 0.5 proportion. To test for differences in proportions among experiments sharing a common stimulus (Experiments 3–7, 8–12, 19–21 and 22–24), similar generalized linear models with data from multiple experiments were created. Models with an individual intercept for each experiment were compared to a model with a single intercept, again with a likelihood ratio test. When a significant difference between experiments was observed, the data of these experiments were compared using a post-hoc Tukey test for honestly significant differences in proportions of flies attracted to various treatment stimuli (Hothorn et al., 2008; Nayani et al., 2023). A p-value of < 0.05 was considered significant in all experiments. Details of all statistical analyses are reported in “Summary of statistics.xlsx” (Nayani et al., 2023).
3 Results
3.1 Identification of microbes collected from cattle skin/hide
Thirty-eight microbial species in 22 genera were isolated and identified (Table 1). These genera included Acinetobacter, Alcaligenes, Bacillus, Burkholderia, Candida, Citrobacter, Corynebacterium, Enterobacter, Escherichia, Glutamicibacter, Klebsiella, Kurthia, Lampropedia, Pantoea, Proteus, Pseudochrobactrum, Pseudomonas, Serratia, Staphylococcus, Stenotrophomonas, Wautersiella, and Wickerhamomyces.
3.2 Attractiveness of select microbes to flies
3.2.1 Still-air olfactometer bioassays (Exps. 1–12)
Proportionally, more stable flies were attracted to Staphylococcus Group-1 bacteria and to heterogeneric Group-2 bacteria than to corresponding sterile agar controls (Figure 2, Exps. 1, 2; p < 0.05 each). As Group-1 microbes seemed more attractive to flies than Group-2 microbes (mean treatment to control response ratio of flies: Group 1: 9.5 to 1; Group 2: 4.4 to 1), all follow-up experiments were designed to determine the key microbe(s) in Group 1 that mediated fly attraction.
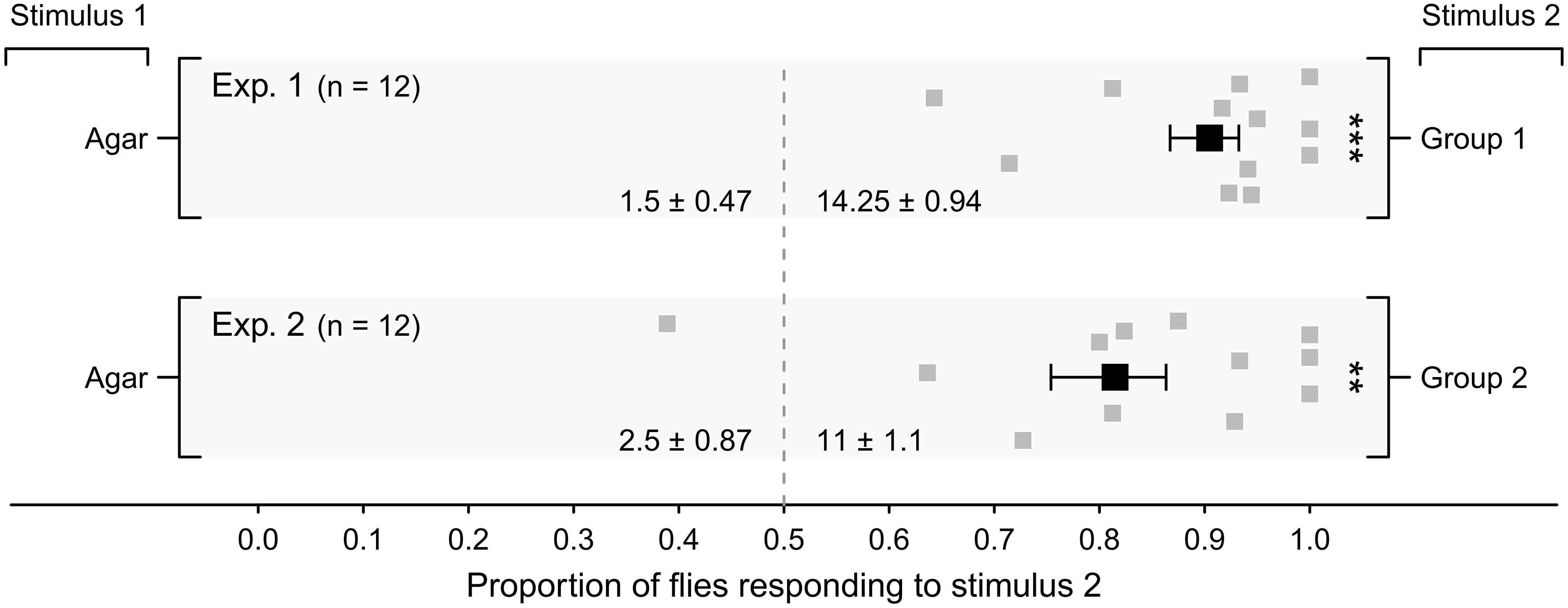
Figure 2 Mean (± SE) proportions of female stable flies captured in lateral chambers of still-air olfactometers (Figure 1A). Control chambers were baited with a plate of four (Exp. 1) or three (Exp. 2) sterile agar slices (Stimulus 1), whereas treatment chambers were baited with a plate of four (Exp. 1) or three (Exp. 2) agar slices, each slice growing (i) Staphylococcus chromogenes, S. sciuri, S. simulans or S. succinus (Stimulus 2; Group 1; Exp. 1), or (ii) Corynebacterium stationis, Glutamicibacter protophormiae or Wautersiella sp. (Stimulus 2; Group 2; Exp. 2). For each experimental replicate, 20 blood- and water-deprived female flies were released into the central chamber of the olfactometer and given 24 h to enter lateral chambers. Grey symbols show the proportion of flies captured in individual replicates in response to stimulus 2, whereas the black symbol shows the mean. Mean numbers of flies captured in response to test stimuli in experiments 1 and 2 are listed at the bottom of each graph; **P < 0.01, ***P < 0.001, as determined by a likelihood ratio test.
In parallel experiments 3–7 (Figure 3), the four Group-1 microbes in combination (positive control) attracted, proportionally, more flies than sterile agar controls (Exp. 3; p < 0.05), as did Group-1 microbes without S. chromogenes (Exp. 4; p < 0.05), S. sciuri (Exp. 5; p < 0.05), S. simulans (Exp. 6; p < 0.05), or S. succinus (Exp. 7; p < 0.05). In parallel experiments 8–12 (Figure 4), the four Group-1 microbes in combination attracted, proportionally, more flies than sterile agar controls (Exp. 8; p < 0.05), as did each of the four microbes singly (Exps. 9–12; p < 0.05 each).
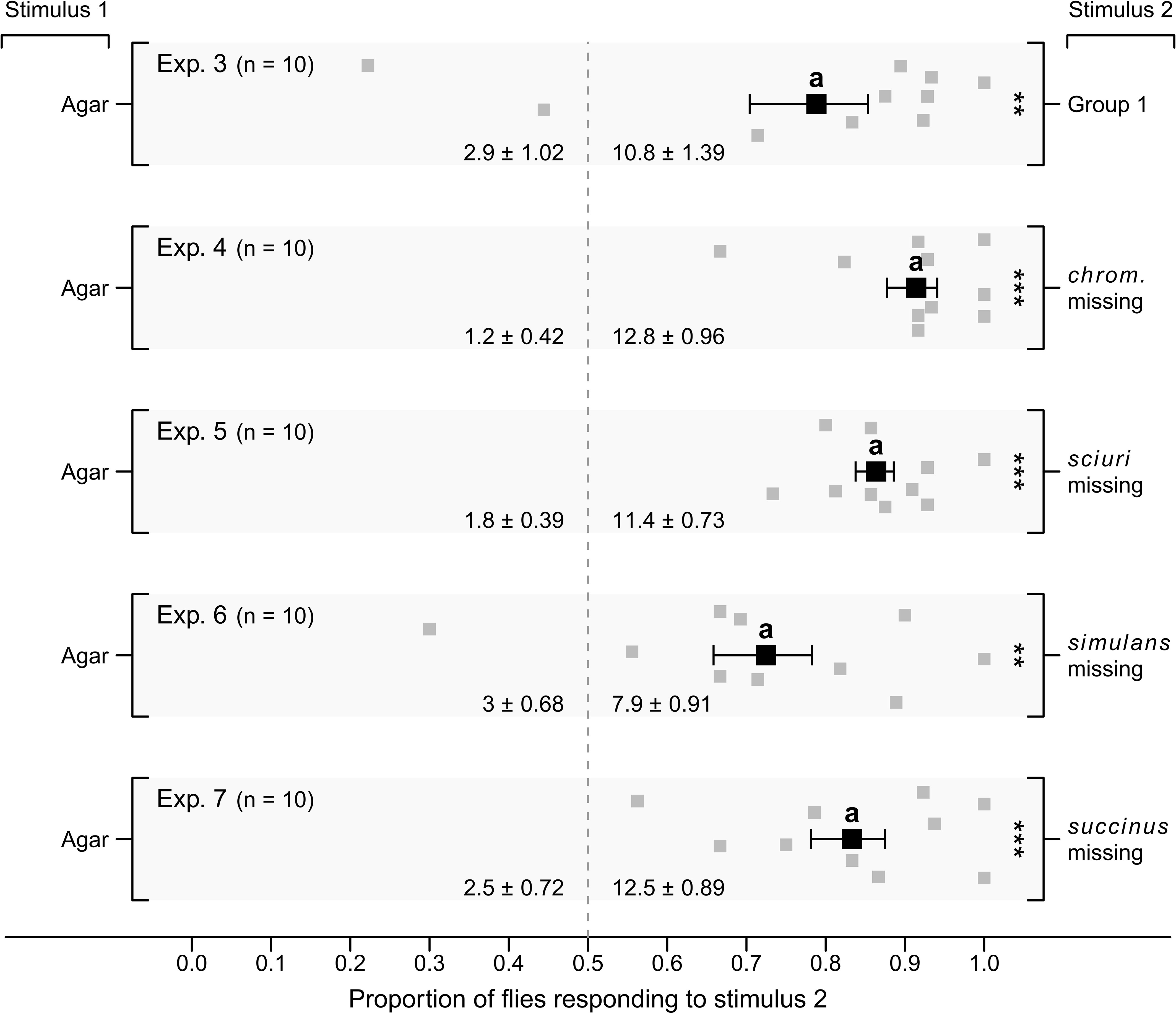
Figure 3 Mean (± SE) proportions of female stable flies captured in lateral chambers of still-air olfactometers (Figure 1A). Control chambers were fitted with a plate of four sterile agar slices (Stimulus 1, Exps. 3–7), whereas treatment chambers were baited with a plate of four agar slices, each slice growing (i) one of four Staphylococcus congeners (S. chromogenes, S. sciuri, S. simulans, or S. succinus) (Stimulus 2; Group 1; Exp. 3), or (ii) one of three Staphylococcus congeners, with one congener missing from Group 1 and one slice of sterile agar added (Stimulus 2; Exps. 4–7). For each experimental replicate, 20 blood- and water-deprived female flies were released into the central chamber of the olfactometer and given 24 h to enter lateral chambers. Grey symbols show the proportion of flies captured in stimulus-2 chambers in each replicate, whereas black symbols show the mean. Mean numbers of flies captured in response to test stimuli in each experiment are listed at the bottom of each graph; **P < 0.01, ***P < 0.001, as determined by a likelihood ratio test; mean proportions in different experiments labelled with the same letter do not differ statistically, post-hoc Tukey tests, P > 0.05.
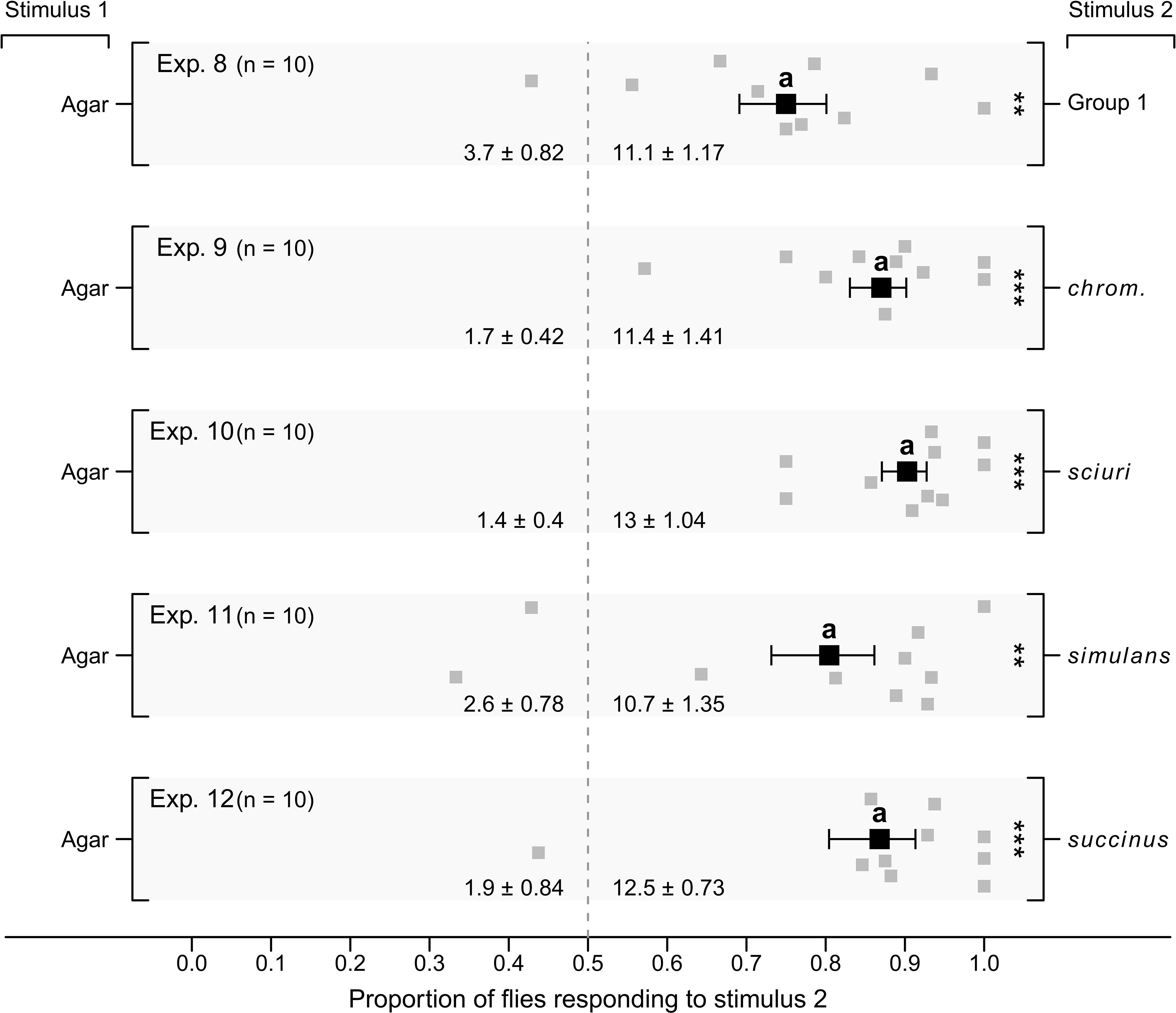
Figure 4 Mean (± SE) proportions of female stable flies captured in lateral chambers of still-air olfactometers (Figure 1A). Control chambers were fitted with a plate of four sterile agar slices (Stimulus 1, Exps. 8–12), whereas treatment chambers were baited with a plate of four agar slices, each slice growing (i) one of four Staphylococcus congeners (S. chromogenes, S. sciuri, S. simulans, or S. succinus) (Stimulus 2; Group 1; Exp. 8), or (ii) one Staphylococcus congener, with three slices of sterile agar added (Stimulus 2; Exps. 9–12). For each experimental replicate, 20 blood- and water-deprived female flies were released into the central chamber of the olfactometer and given 24 h to enter lateral chambers. Grey symbols show the proportion of flies captured in stimulus-2 chambers in each replicate, whereas black symbols show the mean (± SE). Mean numbers of flies captured in response to test stimuli in each experiment are listed at the bottom of each graph; **P < 0.01, ***P < 0.001, as determined by a likelihood ratio test; mean proportions in different experiments labelled with the same letter do not differ statistically, post-hoc Tukey tests, P > 0.05.
All data combined indicate that single Staphylococcus species attract stable flies as effectively as all four Staphylococcus species in combination.
3.2.2 Room bioassay (Exp. 13)
In a large laboratory bioassay room, adhesive-coated cardboard cylinder traps baited with the four Group-1 Staphylococcus microbes on agar captured, proportionally, more flies than cylinder traps baited with sterile agar controls (Figure 5, Exp. 13; p < 0.05), indicating that microbes affected the responses of flies also in a large bioassay room setting.
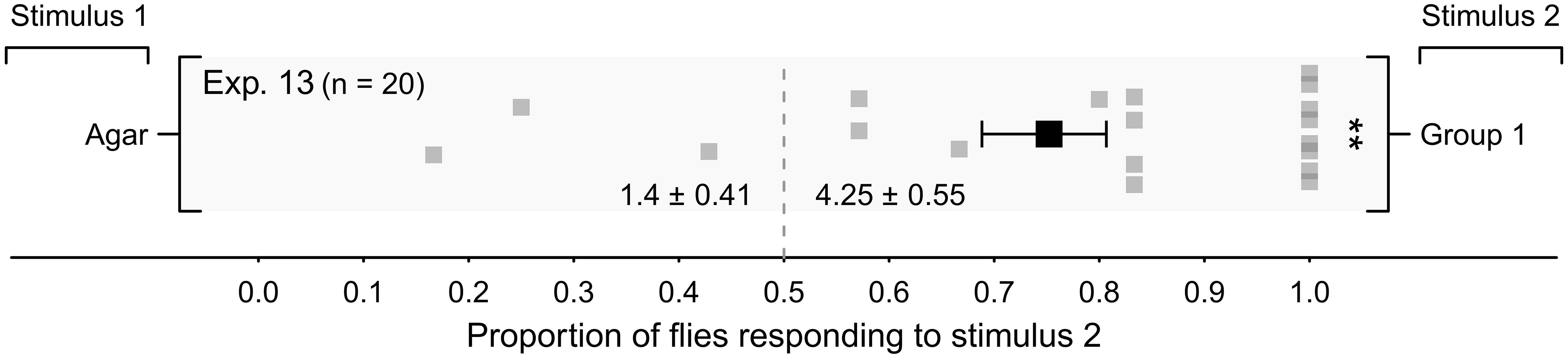
Figure 5 Mean (± SE) proportion of female stable flies captured on paired adhesive-coated cylindrical traps (Figure 1B) in a bioassay room. Control traps were fitted with a plate of four sterile agar slices (Stimulus 1), whereas treatment traps were baited with a plate of four agar slices, each slice growing separately one of four Staphylococcus congeners (S. chromogenes, S. sciuri, S. simulans, or S. succinus) (Group 1; Stimulus 2). For each experimental replicate, 20 blood- and water-deprived female flies were released into the room and given 24 h to respond. Grey symbols show the proportion of flies captured in individual replicates on stimulus-2 traps, whereas the black symbol shows the mean (± SE). Mean numbers of flies captured are listed at the bottom of the graph; **P < 0.01, as determined by a likelihood ratio test.
3.2.3 Greenhouse bioassay (Exp. 14)
In a large greenhouse compartment, black barrels baited with S. sciuri on three agar plates prompted, proportionally, more alighting responses by flies than the black barrels baited with three sterile agar control plates (Figure 6, Exp. 14; p < 0.05), indicating that S. sciuri as a single microbe species modulated the responses of flies in a large-scale setting.
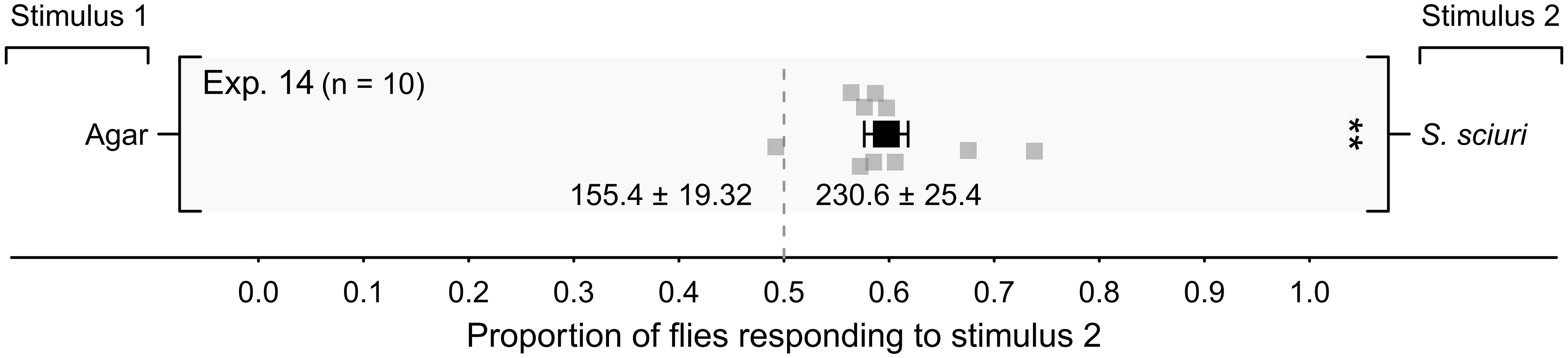
Figure 6 Mean (± SE) proportion of female stable flies alighting on black barrels inside a greenhouse compartment (Figure 1C). Treatment barrels were baited with 3 agar plates growing Staphylococcus sciuri (Stimulus 2), whereas control barrels were fitted with 3 sterile agar plates (Stimulus 1). For each experimental replicate, 100 blood- and water-deprived female flies were released into the greenhouse compartment and given 10 min to respond. Grey symbols show the proportion of flies in each replicate alighting on stimulus-2 barrels, whereas the black symbol shows the mean (± SE). Mean numbers of alightings in response to test stimuli are listed at the bottom of the graph; **P < 0.01, as determined by a likelihood ratio test.
3.2.4 Field bioassays (Exps. 15–18)
On a cattle farm, black barrels baited with S. sciuri on twelve agar plates (Exp. 15), four plates (Exp. 16), two plates (Exp. 17), and on one plate (Exp. 18), all did not prompt proportionally more alighting responses by flies than black barrels baited with the corresponding number of sterile agar control plates (Figure 7; p > 0.05 for all experiments).
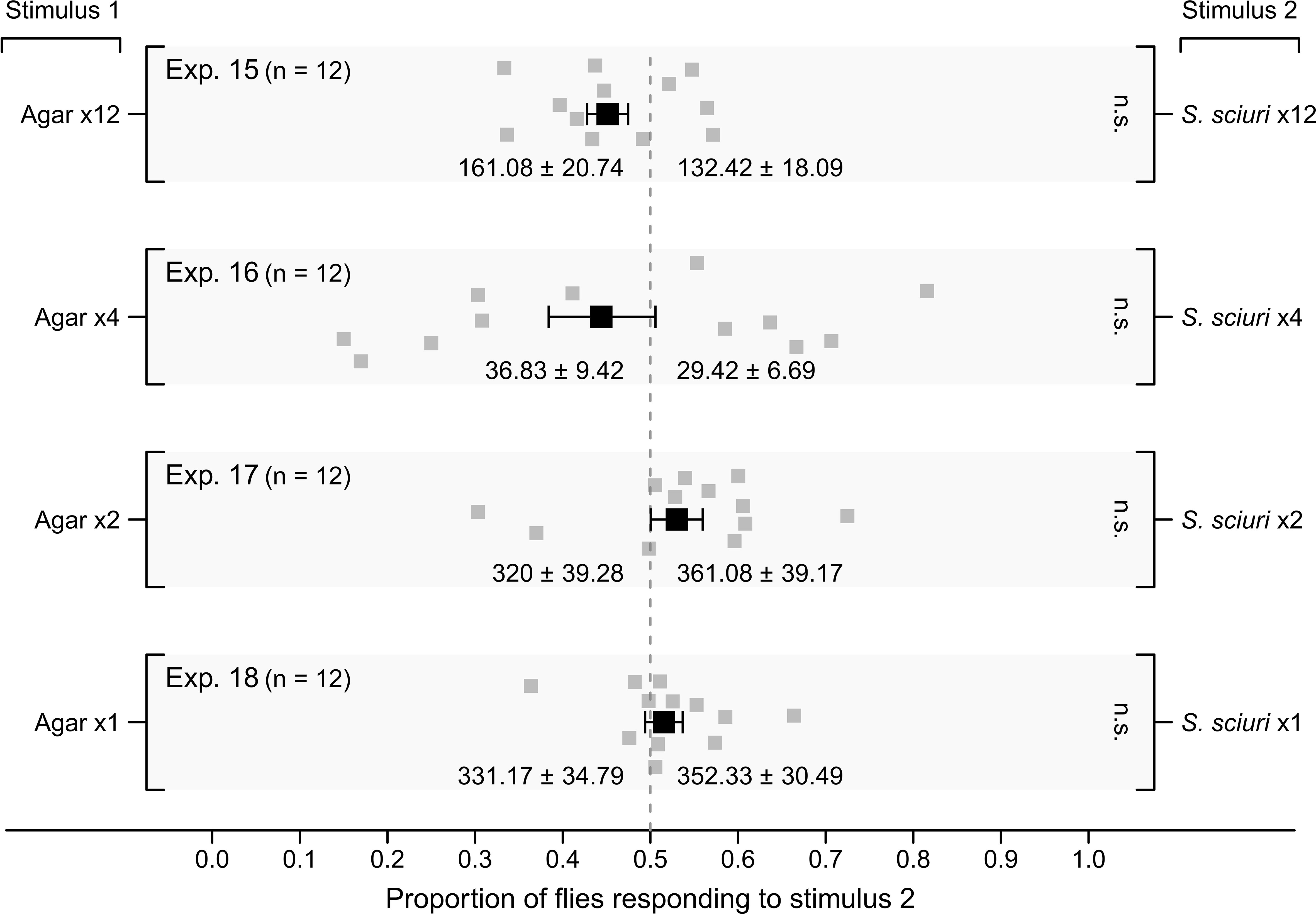
Figure 7 Mean (± SE) proportional alighting responses by wild stable flies on black barrels set up on a cattle farm (Figure 1D). Treatment barrels (Stimulus 2) were baited with 12 agar plates (Exp. 15), 4 plates (Exp. 16), 2 plates (Exp. 17) or 1 plate (Exp. 18) all inoculated with Staphylococcus sciuri, whereas control barrels (Stimulus 1) were fitted with corresponding numbers of sterile agar plates. For each experimental replicate, alighting responses by flies were video recorded for 5 min. Grey symbols show the proportion of alighting responses in each replicate and black symbols show the mean (± SE). Mean numbers of alightings in response to test stimuli are listed at the bottom of each graph. There was no preference for Stimulus 2 in any experiment; n.s., not significant.
3.3 Mechanisms underlying fly attraction to Staphylococcus spp.
3.3.1 Ammonia emission from Staphylococcus microbes
Each of the four Group-1 Staphylococcus species emitted ammonia, as follows: S. chromogenes: 8.0 ± 1.6 ppm; S. sciuri: 14.3 ± 2.6 ppm; S. simulans: 13.0 ± 0.0 ppm; S. succinus: 9.3 ± 0.9 ppm (Table 3).
3.3.2 Effect of ammonia on fly attraction (Exps. 19–21)
In still-air olfactometers (Figure 1E), all test stimuli containing ammonium bicarbonate, and thus emitting ammonia, attracted proportionately more flies than control stimuli lacking ammonium bicarbonate (Figure 8; Exps. 19–21; p < 0.05 each). The amount of ammonium bicarbonate in test stimuli did not affect the proportional response level of flies (p > 0.05), suggesting that the emission of ammonia, rather than its concentration, affected behavioral responses of flies, at least in this experimental context.
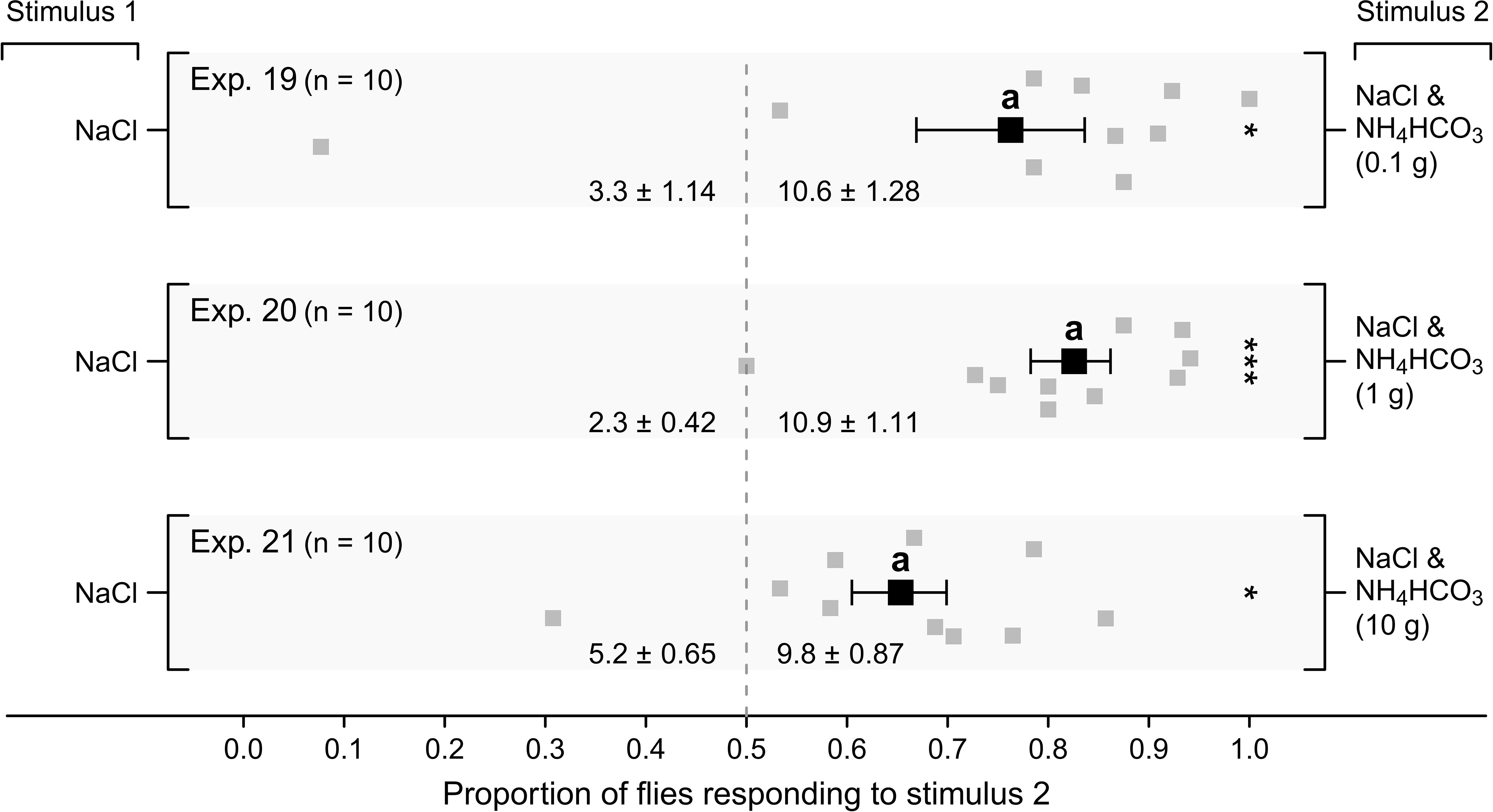
Figure 8 Mean (± SE) proportions of female stable flies captured in lateral chambers of still-air olfactometers (Figure 1E) baited with a sodium chloride solution (50 g NaCl in 50 mL water) (Stimulus 1; Exps. 19–21), or a sodium chloride solution also containing ammonium bicarbonate (NH4HCO3) at 0.1 g (Exp. 19, low dose), 1 g (Exp. 20, medium dose) or 10 g (Exp. 21, high dose), all emitting ammonia (NH3). For each experimental replicate, 20 blood- and water-deprived female flies were released into the central chamber of the olfactometer and given 24 h to approach stimuli in lateral chambers. Grey symbols show the proportion of flies captured in individual replicates in response to stimulus 2, whereas the black symbols show the mean (± SE). Mean numbers of flies captured in response to test stimuli in each experiment are listed at the bottom of each graph; *P < 0.05, ***P < 0.001, as determined by a likelihood ratio test. Mean proportions in different experiments labelled with the same letter do not differ statistically; post-hoc Tukey tests; P > 0.05.
3.3.3 Analyses of microbe headspace volatiles by GC-MS
Each of the four Group-1 Staphylococcus species emitted a complex volatile blend (Table 3). Volatiles shared by all four species included two alcohols (isoamyl alcohol, phenylethyl alcohol), four pyrazines (2,5-dimethyl pyrazine, trimethyl pyrazine, 2-isopropyl-5-methyl-pyrazine, 2-ethyl-3,5(6)-dimethylpyrazine [composed of 50% 2-ethyl-3,5-dimethylpyrazine and 50% 2-ethyl-3,6-dimethylpyrazine]), and isoamyl acetate (Table 3).
3.3.4 Effect of synthetic microbe headspace volatiles on fly attraction (Exps. 22–25)
The “synthetic Staphylococcus blend” (Table S1) – at a 100× dilution – enhanced attraction of flies to ammonia (Figure 9; Exp. 24, p < 0.05), but the blend was not effective at a 10× dilution or without dilution (Exps. 22–23, p > 0.05 each), with either of these higher-dose blends being less attractive than the 100× diluted synthetic Staphylococcus blend (Figure 9).
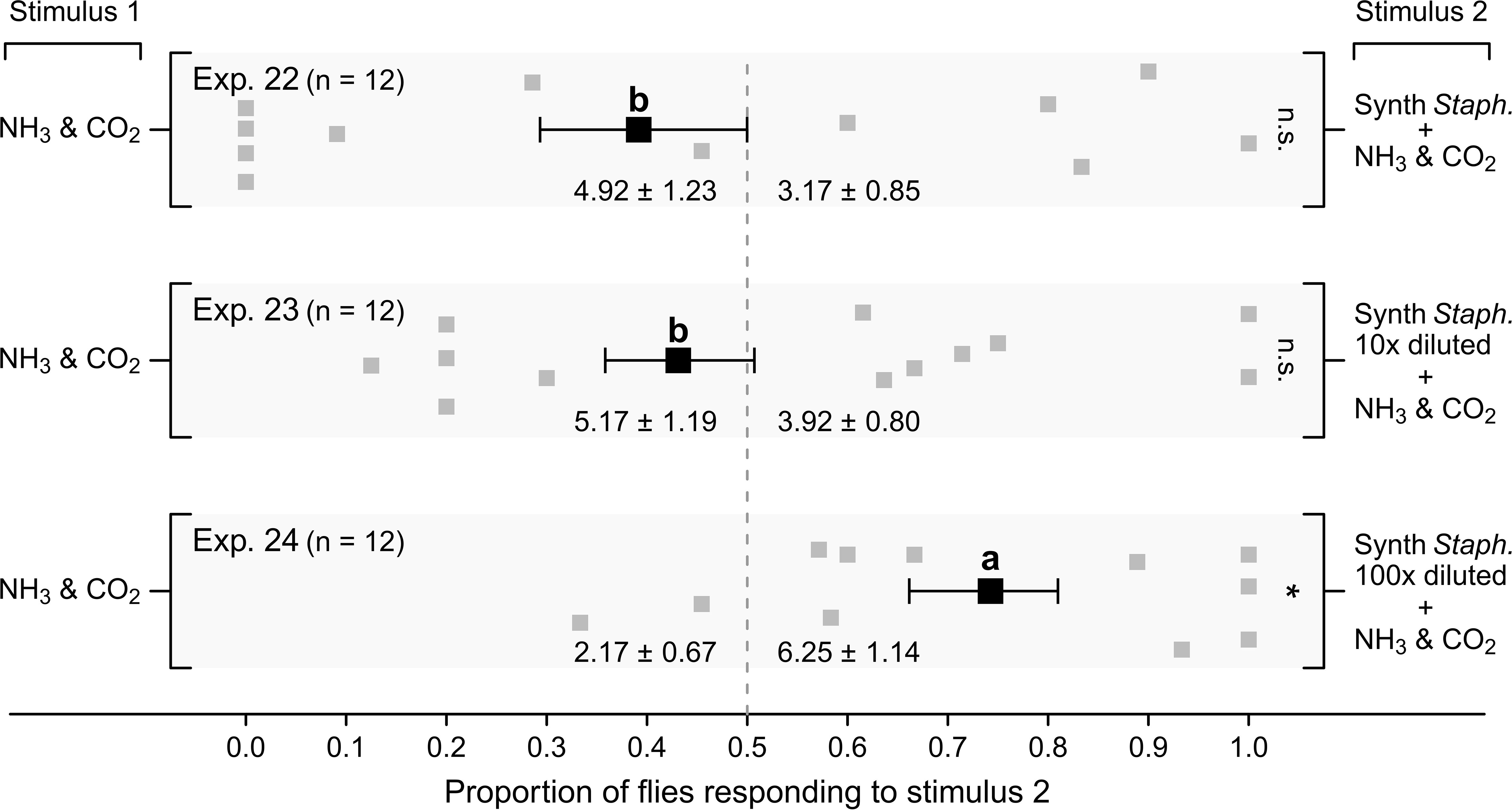
Figure 9 Mean (± SE) proportions of female stable flies captured in lateral chambers of still-air olfactometers (Figure 1E) baited with (i) the synthetic Staphylococcus blend (“Synth. Staph.”) (Table S1) (Exp. 22), (ii) the blend 10× diluted (“Synth Staph. 10× diluted”) (Exp. 23), or (iii) the blend 100× diluted (“Synth. Staph. 100× diluted”) (Exp. 24). All three Stimulus 2 blends were formulated in mineral oil, whereas plain mineral oil served as the corresponding control stimulus. Present in both treatment and control chambers of all experiments was a sodium chloride (NaCl) and ammonium bicarbonate (NH4HCO3) solution (50 g NaCl and 0.1 g NH4HCO3 in 50 mL water) emitting ammonia (NH3) and carbon dioxide (CO2). For each experimental replicate, 20 blood- and water-deprived female flies were released into the central chamber of the olfactometer and given 24 h to approach stimuli in lateral chambers. Grey symbols show the proportion of flies captured in individual replicates in response to stimulus 2, whereas the black symbols show the mean (± SE). Mean numbers of flies captured in response to test stimuli in each experiment are listed at the bottom of each graph; *P < 0.05, n.s., not significant, as determined by a likelihood ratio test. Mean proportions in different experiments labelled with the same letter do not differ statistically; post-hoc Tukey tests; P > 0.05.
The “synthetic S. sciuri blend” (Table S1) did not enhance attraction of flies to ammonia (mean ± SE number of flies responding to treatment and control stimuli: 1.6 ± 0.56 vs 1.0 ± 0.49; Exp. 25, p > 0.05), but in retrospect should also have been tested at lower doses (see above).
4 Discussion
Our data support three conclusions: (1) the cattle skin microbiome is diverse; (2) Staphylococcus spp. as members of the cattle skin microbiome are attractive to stable flies; and (3) attraction of stable flies to Staphylococcus microbes is mediated by microbe-derived gases and odorants.
To test the hypothesis that skin-dwelling microbes of cattle contribute to the attraction of stable flies to cattle hosts, we could – logistically – bioassay only some of the 38 microbes that we had isolated from cattle skin and identified to the genus and/or species level (Table 1). To narrow down the list of the most promising candidate microbes for testing, we focused on those four microbe genera that had previously been reported to be present on animal skin: Corynebacterium, Glutamicibacter, Wautersiella, and Staphylococcus. As evident from the literature, there are many skin-dwelling or skin commensal microbes in the genera Corynebacterium (Corynebacteriaceae) (Cogen et al., 2008; Kong and Segre, 2012; Oh et al., 2012; Belkaid and Segre, 2014; Ross et al., 2017; Byrd et al., 2018), Glutamicibacter (Micrococcaceae) (Noble, 1969; Holland et al., 1977; Holland et al., 1979; Rennie et al., 1991; Bernadsky and Rosenberg, 1992; Ashbee et al., 1993; Bojar et al., 1995; Harvey and Lloyd, 1995; Messiaen et al., 2019), Wautersiella (Weeksellaceae) (Ross et al., 2019; Boxberger et al., 2020; Ma et al., 2021; Wang et al., 2021), and Staphylococcus (Staphylococcaceae) (Verhulst et al., 2011; Oh et al., 2014; Ahle et al., 2020). To further streamline behavioral procedures, we established two microbe bioassay groups. We assigned the four identified Staphylococcus congeners (S. sciuri, S. succinus, S. simulans, S. chromogenes) to Group 1, and the three identified heterogeners (Glutamicibacter protophormiae, Corynebacterium stationis, and Wautersiella sp.) to Group 2. As expected, each group was attractive to stable flies (Figure 2), but Group-1 Staphylococcus microbes seemed comparatively more attractive (mean treatment to control response ratio by flies: Group 1: 9.5 to 1; Group 2: 4.4 to 1), prompting us to focus on Group-1 microbes in follow-up experiments.
To determine the key microbe(s) in Group 1 that mediated attraction of flies, we tested the Group-1 microbes in their quaternary and all possible ternary combinations, all versus sterile agar controls. As the deletion of any one Staphylococcus species from Group 1 did not reduce the group’s attractiveness (Figure 3), we proceeded to test each of the four Group-1 Staphylococcus microbes singly. Our findings that each of S. chromogenes, S. sciuri, S. simulans, and S. succinus, on their own attracted stable flies as effectively as all four species combined (Figure 4), suggested significant overlap in their headspace volatile blends and gas emissions. Volatile and gas analyses then indeed revealed that isoamyl alcohol, isoamyl acetate, 2,5-dimethyl pyrazine, trimethyl pyrazine, 2-isopropyl-5-methyl-pyrazine, 2-ethyl-3,5(6)-dimethylpyrazine, and phenylethyl alcohol were all common volatiles in the headspace of these Staphylococcus congeners, and that each of the four species emitted considerable amounts of ammonia (Table 3).
Staphylococcus bacteria are already known to be attractive to dipterans. Humans with a skin flora rich in Staphylococcus bacteria are more attractive to African malaria mosquitoes, Anopheles gambiae, than humans with a skin flora poor in Staphylococcus bacteria or with a greater skin bacterial diversity (Verhulst et al., 2011). Interestingly, the attractiveness of bacteria to mosquitoes is dependent upon the bacterial growth phase. Staphylococcus epidermidis in its exponential growth phase (when the rate of increase in bacterial cell numbers is greater than the death rate) was not attractive to A. gambiae but became attractive in its stationary growth phase (when the growth rate is equal to the death rate) (Verhulst et al., 2010), suggesting that the concentration or relative composition of bacterial odor and gas profiles affects foraging decisions by host-seeking mosquitoes. In combination, the data indicate that Staphylococcus bacteria contribute to the attractiveness of vertebrate hosts to blood-feeding mosquitoes. A Staphylococcus species has also been shown to attract Mexican fruit flies, Anastrepha ludens, as do several chemicals in the headspace of S. aureus cultures (Robacker et al., 1991; Robacker et al., 1993; Robacker and Flath, 1995). Finally, Staphylococcus bacteria, particularly S. aureus, have been implicated in causing bovine mastitis (Taponen and Pyörälä, 2009). It would be of interest to investigate whether S. aureus attracts stable flies and whether stable flies play a role in vectoring S. aureus between bovine hosts.
Staphylococcus microbes were attractive to stable flies at three separate scales: a small-scale 3-chamber olfactometer (46 × 21.5 × 15.5 cm) (Figure 2; Exp. 1, Figure 3; Exp. 3; Figure 4; Exp. 8), a medium-scale bioassay room (225 × 230 × 230 cm) (Figure 5; Exp. 13), and a large-scale greenhouse compartment (600 × 600 × 360 cm) (Figure 6; Exp. 14). Combined, these data suggest that host-foraging stable flies may follow a concentration gradient of microbe-emitted volatile odorants and gases. Similarly, gravid female stable flies responded to volatile odorants and gases (ammonia and carbon dioxide) emanating from prospective oviposition sites, with odorants and gases in combination being most attractive to gravid female flies (Nayani et al., unpubl.). In light of all these positive bioassay data, it was perplexing that S. sciuri, as a representative of the Staphylococcus group, failed to enhance attraction of stable flies to visual targets in field experiments (Figure 7). Irrespective of the S. sciuri dose (1, 2, 4 or 12 microbe-inoculated agar plates) that was tested, the visual target baited with S. sciuri was no more attractive to flies than the paired unbaited control target (Figure 7). There are multiple potential explanations for the failure of S. sciuri to attract flies in the chemically and visually “noisy” field setting, as follows: (1) any of the microbe doses tested may still have been suboptimal for fly attraction; (2) growing on agar, S. sciuri may have produced an odor and gas profile different from what it typically produces on cattle skin; (3) the odor and gas profile of S. sciuri as a single microbe species may have inadequately represented the odor and gas profile of the entire cattle microbiome; (4) in the presence of complex foraging cues originating from nearby live cattle, a more complex odor profile may have been needed, possibly including odorants and gases emanating not only from the microbiome of cattle but also from their exhale and anus; and (5) for S. sciuri to be competitively attractive to flies, further integration of multimodal host foraging cues may be necessary, including visual (Schofield, 1998; Cilek, 2002; Zhu et al., 2016; Murchie et al., 2018), semiochemical (Jeanbourquin and Guerin, 2007a; Jeanbourquin and Guerin, 2007b; Baleba et al., 2019), thermal, infrared, and aural host foraging cues.
The mechanisms underling attraction of stable flies to Staphylococcus microbes involves microbe-produced gases and odorants. All four Staphylococcus species identified in our study emitted ammonia (Table 3), and ammonia and carbon dioxide emanating from a watery dilution of ammonium bicarbonate attracted stable flies irrespective of the dose tested (Figure 8), indicating that ammonia and/or carbon dioxide contribute to the attraction of flies. We predicted that synthetic blends of microbe-derived odorants would also attract stable flies, or would enhance the attractiveness of microbe-produced gases. This prediction was inspired by reports that synthetic odorants attracted tsetse flies (Saini, 1990; Vale, 1991), horse flies (Mihok and Lange, 2012; Baldacchino et al., 2014), house flies (Cosse and Baker, 1996), fruit flies (Robacker et al., 2000; Hanssen et al., 2019), blow flies (Chaudhury et al., 2015; Brodie et al., 2016), and stable flies (Cilek, 1999; Jeanbourquin and Guerin, 2007a; Jeanbourquin and Guerin, 2007b; Mihok et al., 2007; Tangtrakulwanich et al., 2015; Serra et al., 2017). In our study, a synthetic blend of odorants shared between the four Staphylococcus species (Table 3) enhanced the attractiveness of ammonia and carbon dioxide, revealing an interaction between microbe-produced gases and odorants for fly attraction. Similarly, CO2 and odorants from deer-associated microbes synergistically attracted Western black-legged ticks, Ixodes pacificus (Long et al., 2023). It is remarkable, however, that the bioactivity of odorant blends on attraction of dipterans is contingent upon blend dose (Nyasembe et al., 2012; Wondwosen et al., 2018; this study). In our study, only the 100× dilution of the “synthetic Staphylococcus blend” (Table S1) was attractive to stable flies, and synthetic plant volatile blends at low doses were most attractive to Anopheles mosquitoes (Nyasembe et al., 2012; Wondwosen et al., 2018). Based on these results there is incentive to re-test the “synthetic S. sciuri blend” (Table S1) at a dose lower than previously tested (Figure 9).
In conclusion, Staphylococcus microbes in the cattle skin microbiome attract stable flies in a manner similar to Staphylococcus microbes in the human skin microbiome attracting Malaria mosquitoes. The mechanisms underlying stable fly attraction to cattle skin Staphylococcus microbes entail both microbe-derived odorants and gases such as ammonia and/or carbon dioxide. The effect of microbes on fly attraction may be augmented when presented with other cues of the cattle host “Gestalt”.
Data availability statement
The original contributions presented in the study are publicly available. This data can be found here: https://data.mendeley.com/datasets/rwdt4dbpzm/1.
Author contributions
SN, SM, RG, GG conceptualization. SN, SM, EM, RG, GG methodology. SN experimental data visualization and analysis. SN, SM, EM molecular data analysis. SN, SM, EM, RG, EK data curation. RG, AD chemical synthesis. SN, GG original draft preparation. SN, SM, EM, RG, EK, AD, GG review and editing of submitted manuscript. GG resources, funding acquisition and supervision. All authors contributed to the article and approved the submitted version.
Funding
SN was supported by the Thelma Finlayson Graduate Entrance Scholarship, the Mutual Fire Insurance Company of British Columbia Graduate Scholarship, the Dr. John Yorston Memorial Graduate Scholarship in Biological Sciences and by a Simon Fraser University Graduate Fellowship. Further funding for this project was provided by a Natural Sciences and Engineering Council of Canada (NSERC) – Industrial Research Chair to GG, with BASF Canada Inc., and Scotts Canada Ltd. as the industrial sponsors.
Acknowledgments
We thank Stephen Takács for graphical illustrations in Figure 1, Emmanuel Hung for help with the design of surrogate host objects in greenhouse and field experiments, and Adam Blake for help with statistical analyses. We also thank Erin and Brian Anderson as well as their family for allowing us to perform field studies at their farm Eagle Acres Dairy.
Conflict of interest
The authors declare that the research was conducted in the absence of any commercial or financial relationships that could be construed as a potential conflict of interest.
Publisher’s note
All claims expressed in this article are solely those of the authors and do not necessarily represent those of their affiliated organizations, or those of the publisher, the editors and the reviewers. Any product that may be evaluated in this article, or claim that may be made by its manufacturer, is not guaranteed or endorsed by the publisher.
Supplementary material
The Supplementary Material for this article can be found online at: https://www.frontiersin.org/articles/10.3389/fevo.2023.1212222/full#supplementary-material
References
Ahle C. M., Stødkilde K., Afshar M., Poehlein A., Ogilvie L. A., Soderquist B., et al. (2020). Staphylococcus saccharolyticus: an overlooked human skin colonizer. Microorganisms 8. doi: 10.3390/microorganisms8081105
Albuquerque T. A., Zurek L. (2014). Temporal changes in the bacterial community of animal feces and their correlation with stable fly oviposition, larval development, and adult fitness. Front. Microbiol. 5. doi: 10.3389/fmicb.2014.00590
Altschul S. F., Gish W., Miller W., Myers E. W., Lipman D. J. (1990). Basic local alignment search tool. J. Mol. Biol. 215, 403–410. doi: 10.1016/S0022-2836(05)80360-2
Anderson J. F., Ferrandino F. J., McKnight S., Nolen J., Miller J. (2009). A carbon dioxide, heat and chemical lure trap for the bedbug, Cimex lectularius. Med. Vet. Entomol. 23, 99–105. doi: 10.1111/j.1365-2915.2008.00790.x
Andrews T., Neher D. A., Weicht T. R., Barlow J. W. (2019). Mammary microbiome of lactating organic dairy cows varies by time, tissue site, and infection status. PloS One 14. doi: 10.1371/journal.pone.0225001
Ashbee H. R., Ingham E., Holland K. T., Cunliffe W. J. (1993). The carriage of Malassezia furfur serovars a, b and c in patients with pityriasis-versicolor, seborrheic dermatitis and controls. Br. J. Dermatol. 129, 533–540. doi: 10.1111/j.1365-2133.1993.tb00480.x
Baldacchino F., Manon S., Puech L., Buatois B., Dormont L., Jay-Robert P. (2014). Olfactory and behavioural responses of tabanid horseflies to octenol, phenols and aged horse urine. Med. Vet. Entomol. 28, 201–209. doi: 10.1111/mve.12038
Baleba S. B. S., Torto B., Masiga D., Weldon C. W., Getahun M. N. (2019). Egg-laying decisions based on olfactory cues enhance offspring fitness in Stomoxys calcitrans l. (Diptera: Muscidae). Sci. Rep. 9. doi: 10.1038/s41598-019-40479-9
Belkaid Y., Segre J. A. (2014). Dialogue between skin microbiota and immunity. Science 346, 954–959. doi: 10.1126/science.1260144
Bernadsky G., Rosenberg E. (1992). Drag-reducing properties of bacteria from the skin mucus of the cornetfish (Fistularia commersonii). Microb. Ecol. 24, 63–76. doi: 10.1007/BF00171971
Blake A. J., Hung E., To S., Ng G., Qian J., Gries G. (2023). Stable flies sense and behaviorally respond to the polarization of light. J. Comp. Physiol. doi: 10.1007/s00359-023-01624-y
Bojar R. A., Cunliffe W. J., Holland K. T. (1995). The short-term treatment of acne-vulgaris with benzoyl peroxide - effects on the surface and follicular cutaneous microflora. Br. J. Dermatol. 132, 204–208. doi: 10.1111/j.1365-2133.1995.tb05014.x
Boxberger M., Khedher M. B., Magnien S., Cassir N., La Scola B. (2020). Draft genome and description of Chryseobacterium manosquense strain marseille-Q2069(T) sp. nov., a new bacterium isolated from human healthy skin. New Microbes New Infect. 38. doi: 10.1016/j.nmni.2020.100805
Brodie B. S., Babcock T., Gries R., Benn A., Gries G. (2016). Acquired smell? mature females of the common green bottle fly shift semiochemical preferences from feces feeding sites to carrion oviposition sites. J. Chem. Ecol. 42, 40–50. doi: 10.1007/s10886-015-0658-7
Bruce W. N., Decker G. C. (1958). The relationship of stable fly abundance to milk production in dairy cattle. J. Econ. Entomol. 51, 269–274. doi: 10.1093/jee/51.3.269
Byrd A. L., Belkaid Y., Segre J. A. (2018). The human skin microbiome. Nat. Rev. Microbiol. 16, 143–155. doi: 10.1038/nrmicro.2017.157
Caddey B., De Buck J. (2021). Meta-analysis of bovine digital dermatitis microbiota reveals distinct microbial community structures associated with lesions. Front. Cell. Infect. Microbiol. 11. doi: 10.3389/fcimb.2021.685861
Caddey B., Orsel K., Naushad S., Derakhshani H., De Buck J. (2021). Identification and quantification of bovine digital dermatitis-associated microbiota across lesion stages in feedlot beef cattle. MSystems 6. doi: 10.1128/mSystems.00708-21
Campbell J. B., Skoda S. R., Berkebile D. R., Boxler D. J., Thomas G. D., Adams D. C., et al. (2001). Effects of stable flies (Diptera: Muscidae) on weight gains of grazing yearling cattle. J. Econ. Entomol. 94, 780–783. doi: 10.1603/0022-0493-94.3.780
Campbell J. B., White R. G., Wright J. E., Crookshank R., Clanton D. C. (1977). Effects of stable flies (Diptera: Muscidae) on weight gains and feed-efficiency of calves on growing or finishing rations. J. Econ. Entomol. 70, 592–594. doi: 10.1093/jee/70.5.592
Chaudhury M. F., Zhu J. J., Skoda S. R. (2015). Response of Lucilia sericata (Diptera: Calliphoridae) to screwworm oviposition attractant. J. Med. Entomol. 52, 527–531. doi: 10.1093/jme/tjv054
Cilek J. E. (1999). Evaluation of various substances to increase adult Stomoxys calcitrans (Diptera: Muscidae) collections on alsynite cylinder traps in north Florida. J. Med. Entomol. 36, 605–609. doi: 10.1093/jmedent/36.5.605
Cilek J. E. (2002). Attractiveness of beach ball decoys to adult Stomoxys calcitrans (Diptera: Muscidae). J. Med. Entomol. 39, 127–129. doi: 10.1603/0022-2585-39.1.127
Cogen A. L., Nizet V., Gallo R. L. (2008). Skin microbiota: a source of disease or defence? Br. J. Dermatol. 158, 442–455. doi: 10.1111/j.1365-2133.2008.08437.x
Cosse A. A., Baker T. C. (1996). House flies and pig manure volatiles: wind tunnel behavioral studies and electrophysiological evaluations. J. Agric. Entomol. 13, 301–317.
Cribellier A., Spitzen J., Fairbairn H., van de Geer C., van Leeuwen J. L., Muijres F. T. (2020). Lure, retain, and catch malaria mosquitoes. How heat and humidity improve odour-baited trap performance. Malar. J. 19. doi: 10.1186/s12936-020-03403-5
De Buck J., Ha V., Naushad S., Nobrega D. B., Luby C., Middleton J. R., et al. (2021). Non-aureus staphylococci and bovine udder health: current understanding and knowledge gaps. Front. Vet. Sci. 8. doi: 10.3389/fvets.2021.658031
Espiritu H. M., Mamuad L. L., Kim S., Jin S., Lee S., Kwon S., et al. (2020). Microbiome shift, diversity, and overabundance of opportunistic pathogens in bovine digital dermatitis revealed by 16S rRNA amplicon sequencing. Animals 10. doi: 10.3390/ani10101798
Friesen K., Berkebile D. R., Zhu J. J., Taylor D. B. (2018). Laboratory rearing of stable flies and other muscoid Diptera. JOVE-Journal Vis. Exp. doi: 10.3791/57341
Gillies M. T. (1980). The role of carbon-dioxide in host-finding by mosquitos (Diptera: Culicidae) - a review. Bull. Entomol. Res. 70, 525–532. doi: 10.1017/S0007485300007811
Green G. C., Chan A. D. C., Lin M. (2014). Robust identification of bacteria based on repeated odor measurements from individual bacteria colonies. Sensors actuators B-Chem. 190, 16–24. doi: 10.1016/j.snb.2013.08.001
Hanssen B. L., Park S. J., Royer J. E., Jamie J. F., Taylor P. W., Jamie I. M. (2019). Systematic modification of zingerone reveals structural requirements for attraction of Jarvis’s fruit fly. Sci. Rep. 9. doi: 10.1038/s41598-019-55778-4
Harvey R. G., Lloyd D. H. (1995). The distribution of bacteria (other than staphylococci and Propionibacterium acnes) on the hair, at the skin surface and within the hair-follicles of dogs. Vet. Dermatol. 6, 79–84. doi: 10.1111/j.1365-3164.1995.tb00047.x
Healy T. P., Copland M. J. W. (1995). Activation of Anopheles gambiae mosquitos by carbon dioxide and human breath. Med. Vet. Entomol. 9, 331–336. doi: 10.1111/j.1365-2915.1995.tb00143.x
Holland K. T., Cunliffe W. J., Eady E. A. (1979). Intergeneric and intrageneric inhibition between strains of Propionibacterium acnes and micrococcaceae, particularly Staphylococcus epidermidis, isolated from normal skin and acne lesions. J. Med. Microbiol. 12, 71. doi: 10.1099/00222615-12-1-71
Holland K. T., Cunliffe W. J., Roberts C. D. (1977). Acne vulgaris - an investigation into number of anaerobic diphtheroids and members of micrococcaceae in normal and acne skin. Br. J. Dermatol. 96, 623–626. doi: 10.1111/j.1365-2133.1977.tb05206.x
Horvath G., Szorenyi T., Pereszlenyi A., Gerics B., Hegedus R., Barta A., et al. (2017). Why do horseflies need polarization vision for host detection? polarization helps tabanid flies to select sunlit dark host animals from the dark patches of the visual environment. R. Soc Open Sci. 4. doi: 10.1098/rsos.170735
Hothorn T., Bretz F., Westfall P. (2008). Simultaneous inference in general parametric models. Biometrical J. 50, 346–363. doi: 10.1002/bimj.200810425
Indacochea A., Gard C. C., Hansen I. A., Pierce J., Romero A. (2017). Short-range responses of the kissing bug Triatoma rubida (Hemiptera: Reduviidae) to carbon dioxide, moisture, and artificial light. Insects 8. doi: 10.3390/insects8030090
Jeanbourquin P., Guerin P. M. (2007a). Chemostimuli implicated in selection of oviposition substrates by the stable fly Stomoxys calcitrans. Med. Vet. Entomol. 21, 209–216. doi: 10.1111/j.1365-2915.2007.00685.x
Jeanbourquin P., Guerin P. M. (2007b). Sensory and behavioural responses of the stable fly stomoxys calcitrans to rumen volatiles. Med. Vet. Entomol. 21, 217–224. doi: 10.1111/j.1365-2915.2007.00686.x
Jimenez S. I., Carroll C., Babcock T., Derstine N., Hadwin A., Moore M., et al. (2017). Yeasts harbored by vespine wasps in the pacific Northwest. Environ. Entomol. 46, 217–225. doi: 10.1093/ee/nvw173
Kong H. H., Segre J. A. (2012). Skin microbiome: looking back to move forward. J. Invest. Dermatol. 132, 933–939. doi: 10.1038/jid.2011.417
Liu M. Z., Vosshall L. B. (2019). General visual and contingent thermal cues interact to elicit attraction in female Aedes aegypti mosquitoes. Curr. Biol. 29, 2250–2257. doi: 10.1016/j.cub.2019.06.001
Long J., Maskell K., Gries R., Nayani S. A., Gooding C. E., Gries G. (2023). Synergistic attraction of Western black-legged ticks, Ixodes pacificus, to CO2 and odorant emissions from deer associated microbes. R. Soc Open Sci.. doi: 10.1098/rsos.230084
Ma X., Li G., Yang C., He M., Wang C., Gu Y., et al. (2021). Skin microbiota of the captive giant panda (Ailuropoda melanoleuca) and the distribution of opportunistic skin disease-associated bacteria in different seasons. Front. Vet. Sci. 8. doi: 10.3389/fvets.2021.666486
Marzal A., Magallanes S., Garcia-Longoria L. (2022). Stimuli followed by avian malaria vectors in host-seeking behaviour. Biology-Basel 11. doi: 10.3390/biology11050726
Masuda H., Yoshida M., Shibamoto T. (1981). Synthesis of new pyrazines for flavor use. J. Agric. Food Chem. 29, 944–947. doi: 10.1021/jf00107a014
Meglic A., Ilic M., Pirih P., Skorjanc A., Wehling M. F., Kreft M., et al. (2019). Horsefly object-directed polarotaxis is mediated by a stochastically distributed ommatidial subtype in the ventral retina. Proc. Natl. Acad. Sci. U. S. A. 116, 21843–21853. doi: 10.1073/pnas.1910807116
Messiaen Y., MacLellan J. D., Pelsue D. H. (2019). Evaluation of the number of colony forming units on the skin of dogs after clipping the hair with two sizes of clipper blades. Am. J. Vet. Res. 80, 862–867. doi: 10.2460/ajvr.80.9.862
Mihara S., Masuda H. (1990). Structure elucidation of the product prepared from the reaction of 3-methyl-5,6-dihydro-2(1H)-pyrazinone and ketones or aldehydes. J. Agric. Food Chem. 38, 1032–1034. doi: 10.1021/jf00094a025
Mihok S., Carlson D. A., Ndegwa P. N. (2007). Tsetse and other biting fly responses to nzi traps baited with octenol, phenols and acetone. Med. Vet. Entomol. 21, 70–84. doi: 10.1111/j.1365-2915.2007.00665.x
Mihok S., Lange K. (2012). Synergism between ammonia and phenols for hybomitra tabanids in northern and temperate Canada. Med. Vet. Entomol. 26, 282–290. doi: 10.1111/j.1365-2915.2011.00999.x
Milne M. A., Ross E. J., Sonenshine D. E., Kirsch P. (2009). Attraction of Triatoma dimidiata and Rhodnius prolixus (Hemiptera: Reduviidae) to combinations of host cues tested at two distances. J. Med. Entomol. 46, 1062–1073. doi: 10.1603/033.046.0513
Murchie A. K., Hall C. E., Gordon A. W., Clawson S. (2018). Black border increases Stomoxys calcitrans catch on white sticky traps. Insects 9. doi: 10.3390/insects9010013
Nayani S., Meraj S., Mohr E., Gries R., Kovacs E., Devireddy A., et al (2023). Data and analysis for: Staphylococcus microbes in the bovine skin microbiome attract blood-feeding stable flies. Mendeley Data, V1. doi: 10.17632/rwdt4dbpzm.1
Nielsen M. W., Strube M. L., Isbrand A., Al-Medrasi W. D. H. M., Boye M., Jensen T. K., et al. (2016). Potential bacterial core species associated with digital dermatitis in cattle herds identified by molecular profiling of interdigital skin samples. Vet. Microbiol. 186, 139–149. doi: 10.1016/j.vetmic.2016.03.003
Neises B., Steglich W. (1978). Simple method for esterification of carboxylic-acids. Angew. Chem., Int. Ed. Engl. 17, 522–524. doi: 10.1002/anie.197805221
Noble W. C. (1969). Skin carriage of Micrococcaceae. J. Clin. Pathol. 22, 249. doi: 10.1136/jcp.22.3.249
Nyasembe V. O., Teal P. E. A., Mukabana W. R., Tumlinson J. H., Torto B. (2012). Behavioural response of the malaria vector Anopheles gambiae to host plant volatiles and synthetic blends. Parasites Vectors 5. doi: 10.1186/1756-3305-5-234
Oh J., Byrd A. L., Deming C., Conlan S., Kong H. H., Segre J. A., et al. (2014). Biogeography and individuality shape function in the human skin metagenome. Nature 514, 59–64. doi: 10.1038/nature13786
Oh J., Conlan S., Polley E. C., Segre J. A., Kong H. H. (2012). Shifts in human skin and nares microbiota of healthy children and adults. Genome Med. 4. doi: 10.1186/gm378
Onju S., Thaisungnoen K., Masmeatathip R., Duvallet G., Desquesnes M. (2020). Comparison of blue cotton and blue polyester fabrics to attract hematophagous flies in cattle farms in Thailand. J. Vector Ecol. 45, 262–268. doi: 10.1111/jvec.12397
Ortiz I. M., Molina J. (2010). Preliminary evidence of Rhodnius prolixus (Hemiptera: Triatominae) attraction to human skin odour extracts. Acta Trop. 113, 174–179. doi: 10.1016/j.actatropica.2009.10.014
Peach D. A. H., Carroll C., Meraj S., Gomes S., Galloway E., Balcita A., et al. (2021). Nectar-dwelling microbes of common tansy are attractive to its mosquito pollinator, Culex pipiens l. BMC Ecol. Evol. 21. doi: 10.1186/s12862-021-01761-5
Rennie P. J., Gower D. B., Holland K. T. (1991). In-vitro and in-vivo studies of human axillary odor and the cutaneous microflora. Br. J. Dermatol. 124, 596–602. doi: 10.1111/j.1365-2133.1991.tb04958.x
Robacker D. C., Flath R. A. (1995). Attractants from Staphylococcus aureus cultures for Mexican fruit fly, Anastrepha ludens. J. Chem. Ecol. 21, 1861–1874. doi: 10.1007/BF02033682
Robacker D. C., Garcia J. A., Bartelt R. J. (2000). Volatiles from duck feces attractive to Mexican fruit fly. J. Chem. Ecol. 26, 1849–1867. doi: 10.1023/A:1005544723521
Robacker D. C., Garcia J. A., Martinez A. J., Kaufman M. G. (1991). Strain of Staphylococcus attractive to laboratory strain Anastrepha ludens (Diptera: Tephritidae). Ann. Entomol. Soc Am. 84, 555–559. doi: 10.1093/aesa/84.5.555
Robacker D. C., Warfield W. C., Albach R. F. (1993). Partial characterization and HPLC isolation of bacteria-produced attractants for the Mexican fruit-fly, Anastrepha ludens (Diptera: Tephritidae). J. Chem. Ecol. 19, 543–557. doi: 10.1007/BF00994324
Romero A., Broce A., Zurek L. (2006). Role of bacteria in the oviposition behaviour and larval development of stable flies. Med. Vet. Entomol. 20, 115–121. doi: 10.1111/j.1365-2915.2006.00602.x
Ross A. A., Doxey A. C., Neufeld J. D. (2017). The skin microbiome of cohabiting couples. MSystems 2. doi: 10.1128/mSystems.00043-17
Ross V D., Suzina N. E., Gafarov A. B., Machulin V A., Esikova T. Z., Shorokhova A. P., et al. (2019). Characterization of ultrasmall Chryseobacterium strains FM1 and FM2 isolated from Xenopus laevis skin. Microbiology 88, 172–182. doi: 10.1134/S0026261719020103
R Studio Team (2023) RStudio: integrated development environment for r. Available at: http://www.rstudio.com/.
Saini R. K. (1990). Responses of tsetse, Glossina spp (Diptera: Glossinidae) to phenolic kairomones in a wind-tunnel. Insect Sci. Its Appl. 11, 369–375. doi: 10.1017/S1742758400012790
Schmitz H., Trenner S., Hofmann M. H., Bleckmann H. (2000). The ability of Rhodnius prolixus (Hemiptera: Reduviidae) to approach a thermal source solely by its infrared radiation. J. Insect Physiol. 46, 745–751. doi: 10.1016/S0022-1910(99)00163-8
Schofield S. (1998). Responses to electrified targets and daily activity of Stomoxys spp. (Diptera: Muscidae) in Zimbabwe. Bull. Entomol. Res. 88, 627–632. doi: 10.1017/S0007485300054298
Scully E., Friesen K., Wienhold B., Durso L. M. (2017). Microbial communities associated with stable fly (Diptera: Muscidae) larvae and their developmental substrates. Ann. Entomol. Soc Am. 110, 61–72. doi: 10.1093/aesa/saw087
Serra N. S. J., Goulart H. F., Triana M. F., Dos Santos Tavares S., Almeida C. I. M., Da Costa J. G., et al. (2017). Identification of stable fly attractant compounds in vinasse, a byproduct of sugarcane-ethanol distillation. Med. Vet. Entomol. 31, 381–391. doi: 10.1111/mve.12246
Sharif S., Lienard E., Duvallet G., Etienne L., Mongellaz C., Grisez C., et al. (2020). Attractiveness and specificity of different polyethylene blue screens on Stomoxys calcitrans (Diptera: Muscidae). Insects 11. doi: 10.3390/insects11090575
Showering A., Martinez J., Benavente E. D., Gezan S. A., Jones R. T., Oke C., et al. (2022). Skin microbiome alters attractiveness to Anopheles mosquitoes. BMC Microbiol. 22. doi: 10.1186/s12866-022-02502-4
Takai K., Horikoshi K. (2000). Rapid detection and quantification of members of the archaeal community by quantitative PCR using fluorogenic probes. Appl. Environ. Microbiol. 66, 5066–5072. doi: 10.1128/AEM.66.11.5066-5072.2000
Takken W. (1991). The role of olfaction in host-seeking of mosquitos - a review. Insect Sci. Its Appl. 12, 287–295. doi: 10.1017/S1742758400020816
Tangtrakulwanich K., Albuquerque T. A., Brewer G. J., Baxendale F. P., Zurek L., Miller D. N., et al. (2015). Behavioural responses of stable flies to cattle manure slurry associated odourants. Med. Vet. Entomol. 29, 82–87. doi: 10.1111/mve.12103
Taponen S., Pyörälä S. (2009). Coagulase-negative staphylococci as cause of bovine mastitis-Not so different from Staphylococcus aureus? Vet. Microbiol. 134, 29–36. doi: 10.1016/j.vetmic.2008.09.011
Taylor D. B., Moon R. D., Mark D. R. (2012). Economic impact of stable flies (Diptera: Muscidae) on dairy and beef cattle production. J. Med. Entomol. 49, 198–209. doi: 10.1603/ME10050
Vale G. A. (1991). Responses of tsetse-flies (Diptera: Glossinidae) to odor-baited trees. Bull. Entomol. Res. 81, 323–331. doi: 10.1017/S0007485300033605
Van Den Dool H., Dec. Kratz P. (1963). A generalization of the retention index system including linear temperature programmed gas–liquid partition chromatography. J. Chromatogr. A 11, 463–471. doi: 10.1016/S0021-9673(01)80947-X
Verhulst N. O., Andriessen R., Groenhagen U., Bukovinszkiné Kiss G., Schulz S., Takken W., et al. (2010). Differential attraction of malaria mosquitoes to volatile blends produced by human skin bacteria. PloS One 5, e15829. doi: 10.1371/journal.pone.0015829
Verhulst N. O., Qiu Y. T., Beijleveld H., Maliepaard C., Knights D., Schulz S., et al. (2011). Composition of human skin microbiota affects attractiveness to malaria mosquitoes. PloS One 6. doi: 10.1371/journal.pone.0028991
Wang Y., Yu Q., Zhou R., Feng T., Hilal M. G., Li H. (2021). Nationality and body location alter human skin microbiome. Appl. Microbiol. Biotechnol. 105, 5241–5256. doi: 10.1007/s00253-021-11387-8
Warnes M. L., Finlayson L. H. (1985). Responses of the stable fly, Stomoxys calcitrans (L) (Diptera: Muscidae), to carbon-dioxide and host odors. 1. activation. Bull. Entomol. Res. 75, 519–527. doi: 10.1017/S0007485300014619
Winther A. R., Narvhus J. A., Smistad M., Duarte V., da S., Bombelli A., et al. (2022). Longitudinal dynamics of the bovine udder microbiota. Anim. Microbiome 4. doi: 10.1186/s42523-022-00177-w
Wondwosen B., Dawit M., Debebe Y., Tekie H., Hill S. R., Ignell R. (2021). Development of a chimeric odour blend for attracting gravid malaria vectors. Malar J. 20. doi: 10.1186/s12936-021-03797-w
Wondwosen B., Birgersson G., Tekie H., Torto B., Ignell R., Hill S. R. (2018). Sweet attraction: sugarcane pollen-associated volatiles attract gravid Anopheles arabiensis. Malar J. 17. doi: 10.1186/s12936-018-2245-1
Zhu J. J., Zhang Q., Taylor D. B., Friesen K. A. (2016). Visual and olfactory enhancement of stable fly trapping. Pest Manage. Sci. 72, 1765–1771. doi: 10.1002/ps.4207
Zinicola M., Higgins H., Lima S., Machado V., Guard C., Bicalho R. (2015a). Shotgun metagenomic sequencing reveals functional genes and microbiome associated with bovine digital dermatitis. PloS One 10. doi: 10.1371/journal.pone.0133674
Keywords: microbe, Staphylococcus, Stomoxys calcitrans, attraction, semiochemical, ammonia
Citation: Nayani SA, Meraj S, Mohr E, Gries R, Kovacs E, Devireddy A and Gries G (2023) Staphylococcus microbes in the bovine skin microbiome attract blood-feeding stable flies. Front. Ecol. Evol. 11:1212222. doi: 10.3389/fevo.2023.1212222
Received: 25 April 2023; Accepted: 12 June 2023;
Published: 10 July 2023.
Edited by:
Lukasz Lech Stelinski, University of Florida, United StatesReviewed by:
Niels Verhulst, University of Zurich, SwitzerlandRob Morrison, Agricultural Research Service (USDA), United States
Copyright © 2023 Nayani, Meraj, Mohr, Gries, Kovacs, Devireddy and Gries. This is an open-access article distributed under the terms of the Creative Commons Attribution License (CC BY). The use, distribution or reproduction in other forums is permitted, provided the original author(s) and the copyright owner(s) are credited and that the original publication in this journal is cited, in accordance with accepted academic practice. No use, distribution or reproduction is permitted which does not comply with these terms.
*Correspondence: Saif A. Nayani, c25heWFuaUBzZnUuY2E=
†ORCID: Sanam Meraj, orcid.org/0000-0001-6054-5553
Saif A. Nayani, orcid.org/0000-0002-2536-6637
Gerhard J. Gries, orcid.org/0000-0003-3115-8989
Emma Kovacs, orcid.org/0009-0008-5898-1418