- College of Forestry, Gansu Agricultural University, Lanzhou, China
Soil enzymes play a vital role in the functioning of wetland ecosystems, driving energy flow and material cycling processes. Gahai wet meadow, one of the important components of alpine wetlands on the Qinghai-Tibet Plateau, has suffered serious degradation in the last 30 years due to climate change and human activities. We studied the spatial and temporal heterogeneity of soil nitrogen content and nitrogen (N)-cycle enzyme activities (i.e., urease, protease, nitrate reductase and nitrite reductase) in four degraded wet meadows in the Gahai wetlands. Our results suggested that with increasing wet meadow degradation, there was a significant decrease in soil water content, total nitrogen, ammonium nitrogen, microbial biomass nitrogen content, protease activities, and nitrite reductase activities; Conversely, soil temperature, nitrate nitrogen content, urease activities, and nitrate reductase activities increased significantly. Soil urease, protease, and nitrite reductase activities significantly decreased with increasing soil depth;The highest activity levels of the three N-cycle enzymes were observed in July and August. The linear mixed modeling results indicated that there were significant effects of degradation level and soil depth and their interactions on soil nitrate reductase and nitrite reductase activities (p < 0.01), while soil depth had significant effects only on soil urease and protease activities (p < 0.01). Redundancy analyses showed that soil ammonium and nitrate nitrogen were the main drivers of changes in soil N-cycle enzyme activity during the degradation of wet meadows. In summary, our study sheds light on the processes of soil enzyme activity in an alpine wetland ecosystem and provides valuable information for understanding the N cycling in these complex systems.
1 Introduction
Wetlands, as transitional ecosystems between land and water, cover only 6% of the Earth’s surface (Shen et al., 2022b), but have been playing unique ecological functions in maintaining ecosystem stability and regulating climate change (Janse et al., 2019; Wang et al., 2021). Nitrogen (N) is usually one of the major limiting nutrients in wetlands, and wetland soils, as the largest nitrogen reservoir in wetland ecosystems, fulfill essential functions as an N source, sink, and transformer (Wang et al., 2021). The soil nitrogen content and the nitrogen transport and transformation rates significantly affect the changes of N-cycle in wetland ecosystems, and have an essential role in regulating the biogeochemical cycle of wetland ecosystems (Mitsch and Gosselink, 2000).
Wetland vegetation is an essential component of wetland ecosystems and a sensitive indicator of environmental change in the ecosystem (Maneas et al., 2019). Following global warming and intensified human activities, wetlands suffer from serious vegetation degradation or loss worldwide (Xiang et al., 2009; Davidson, 2014; Hu et al., 2017; Fluet-Chouinard et al., 2023), subsequently affects soil cycle enzyme activities in the wetland. As an essential type of wetland, wet meadow degradation has also changed the composition of plant communities from humidogenes and aquatics to mesophytes and xerophytes, and some species may disappear or be replaced by others (Ma et al., 2018). Meanwhile, wetland degradation also results in a transition from pristine swamp soil to degraded meadow soil, or even aeolian sandy soil at severely deteriorated sites, and thus leads to the decomposition of soil organic matter, the loss of humus and peat layers, and the reduction of nutrient content (Gu et al., 2018), which further affects ecological functions (Laurance et al., 2012; Cao et al., 2017).
Soil N-cycle enzymes fulfill an important functionin catalyzing soil mineralization, sequestration, nitrification, and denitrification. It is the key indicator for soil N and nutrient cycling, thus soil health and biogeochemical function of terrestrial ecosystems (Dunn et al., 2014; Wang et al., 2018; Pu et al., 2019). Specifically, soil urease and protease can convert soil organic nitrogen into available nitrogen decomposition (Zantua and Bremner, 1975; Caldwell, 2005). Soil nitrification and denitrification are regulated by nitrate reductase, nitrite reductase, and nitrous oxide reductase (Wang et al., 2021). Many studies have demonstrated that soil enzyme activities are not only influenced by many factors, including soil environmental conditions, microbial structure, and substrate quality (Weintraub et al., 2007; Schimel et al., 2017; Li et al., 2022a), as well as vegetation type, litter degradation, and nutrient return (Sinsabaugh et al., 2008; Dong et al., 2019a). Vegetation degradation in wet meadows changes the vegetation cover, biomass, seed bank quantity, soil microbial community structure, soil carbon and nitrogen stocks, and functional genes (Zhang et al., 2002; Ma et al., 2011; Pan et al., 2020; Li et al., 2022c). Moreover, soil enzyme activity is also closely related to the differences in hydrothermal conditions caused by soil layer thickness, with surface soil temperature and nutrients being superior to those of the subsoil, beneficially promoting soil microbial populations and thus affecting enzyme activity(Chen et al., 2021). Wetland ecosystem degradation caused significant changes in regional vegetation, soil and other factors, which to some extent into affected the related soil enzyme activities. However, changes in soil N-cycle enzyme activities under different vegetation degradation phases remains poorly understood. Therefore, studies of the changes in soil N-cycle enzyme activities in vegetation degradation sites are essential for alpine wetland health evaluation and management.
The Qinghai-Tibet Plateau (QTP), located in western China, has 2.5×106 km2 of wet meadows (about 35% of the total area of the QTP), which is the most dominant wetland type on the QTP (Xue et al., 2018; Li et al., 2019). Wet meadows play pivotal roles in soil and water conservation, climate regulation, and maintenance of biodiversity (Wei et al., 2015). However, the alpine wetlands are more vulnerable to climate change than those in lower elevation regions (Xue et al., 2014). During recent years, there has been a clear warming trend on the QTP, with annual mean temperature increasing by 0.4°C per decade (Masson-Delmotte et al., 2021) and precipitation decreasing by 22 mm per decade (Yang et al., 2014). At the same time, almost all wetlands on the QTP have been used for grazing (Hirota et al., 2005), and long-term overgrazing by livestock has significantly increased vegetation loss (Chen et al., 2013). These changes have resulted in vegetation degradation of wet meadows on the QTP (Ma et al., 2011; Wu et al., 2017). Previous studies showed that vegetation degradation in wetlands significantly reduced soil enzyme activities (Dong et al., 2019b; Li et al., 2021). The occurrence and development of vegetation directly or indirectly mediates soil enzyme activities as the vegetation provides excreta or exogenous enzymes, exudates, and oxygen to the soil (Paolo Nannipieri et al., 2018). Nevertheless, very few studies considered the effects of vegetation degradation on soil N-cycle enzyme activities in wet meadows, especially on the QTP. Therefore, in order to better predict soil nitrogen turnover in wet meadows on the QTP under the background of climate change and anthropogenic disturbances, studies on changes of soil N-cycle enzyme activities along the vegetation degradation in the alpine wetland on the QTP are needed.
To address these concerns, we quatified the soil N-cycle enzyme activities at non-degraded (ND), lightly degraded (LD), moderately degraded (MD), and heavily degraded (HD) wet meadows on the QTP. Our objectives were (1) addressing the question of how soil N-cycle enzyme activities varied as a consequence of increased vegetation degradation; (2) analyzing the relationship between soil N-cycle enzyme activities and soil micro-environment and contents of inorganic N components. We hypothesized that (a) as the level of vegetation degradation increases, soil N-cycle enzyme activities gradually decrease due to lack of input from substrate sources; (b) with increasing soil depth, soil enzyme activities gradually decreases because soil temperature tends to decrease; (c) soil N-cycle enzyme activities will show dynamic changes over time as plant growth rhythms and soil hydrothermal conditions vary under different the degrees of vegetation degradation.
2 Materials and methods
2.1 Study site
The study was conducted at the Gahai-Zecha International Nature Reserve, Gansu province, China (34°16′N, 102°26′E), which is located on the northeastern edge of the QTP (Figure 1). The elevation of the Reserve ranges from 3400 to 4300 m.a.s.l. A cold temperate continental monsoon climate characterizes the region. It has an annual average temperature of 1.2°C, annual precipitation of 782 mm, and annual evaporation of 1150 mm, with the most precipitation occurs during May to September (Figure 2). The soil type on the site is typical meadow soil with a sandy loam texture, which is very representative of the QTP (Ma et al., 2018).
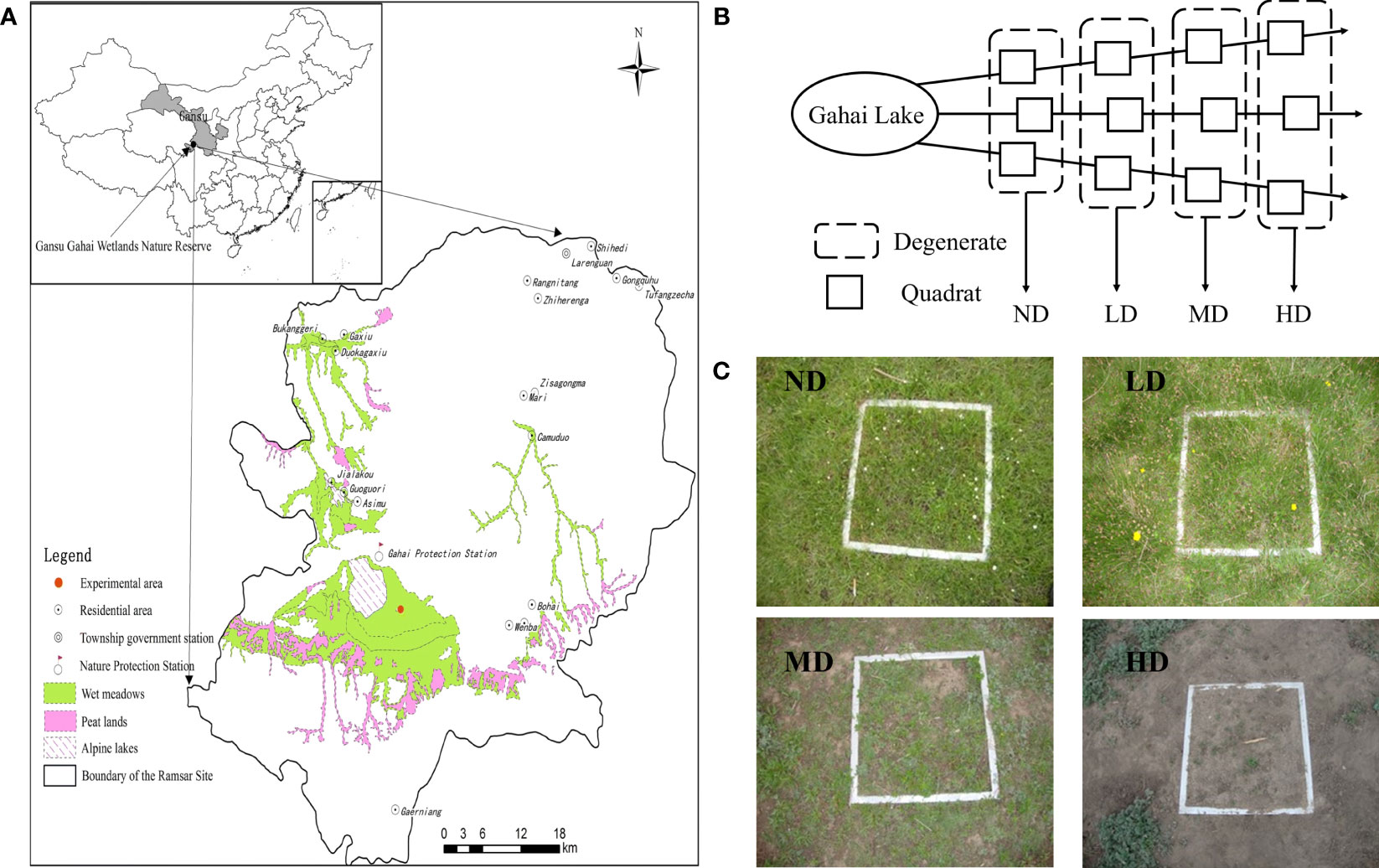
Figure 1 Information of study area and sampling site information. The map of the study area (A); Schematic diagram of sampling lines along vegetation degradation gradient (B). Four degraded wet meadow types (C).
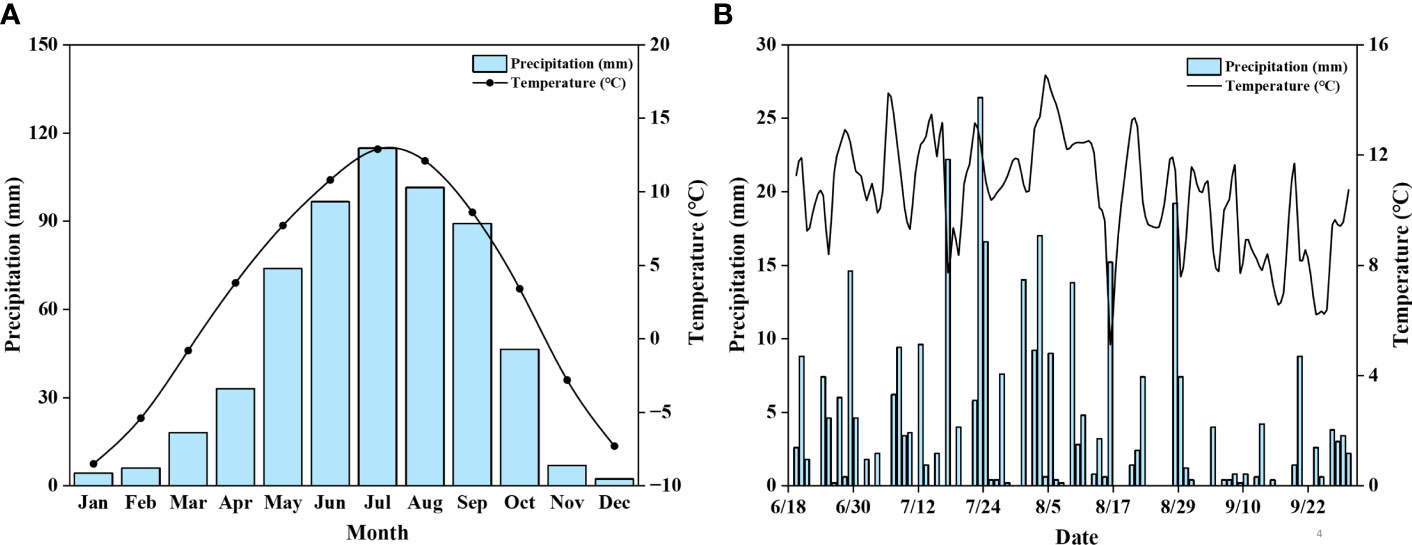
Figure 2 Average monthly precipitation and temperature from 1981 to 2010 (A); Daily precipitation and the average temperature for the study area from June to September 2020 (B). The datum of temperature and precipitation were taken from the local climate station located at the Nature Reserve with a logging interval of every 60 min.
2.2 Experimental design
As our previous work reported (Ma et al., 2018), observations of plant species composition, above-ground biomass, and plant cover in Gahai wetlands were adopted to identify four vegetation degradation treatments [i.e., non-degraded (ND), lightly degraded (LD), moderately degraded (MD), and heavily degraded (HD)]. Specifically the four degradation treatments were all on the same elevation gradient with a maximum difference of less than 10 meters (Wu et al., 2020a). We randomly selected three radial sampling lines starting the moisture gradient from the center of Gahai Lake and established three 10 m × 10 m sampling plots for each degradation type on the same line. A total of 12 plots were established for sampling soils. A buffer zone of at least 5 m was set up between two linear transects to reduce potential edge effects (Figure 1B). Details on the vegetation status of the sample sites were reported in our previous work (Ma et al., 2018). The basic soil physicochemical properties of the plots of the different vegetated degraded wet meadows are shown in Table 1. In early May 2013, we established plots and maintained them until soil sampling. In addition, Tibetan sheep and yaks haves grazed in these areas during October to April.
2.3 Soil sampling
From June to September 2020 (June 18th, July 16th, August 13th, and September 15th), soil samples were collected pattern at seven random points following an “S” pattern at depths of 0-10 cm, 10-20 cm, and 20-40 cm in the plots using a 5 cm diameter soil sampler. For each plot, soil samples from the same soil depths were pooled to form a mixed soil sample. All soil samples were placed in self-sealing bags, stored in bubble boxes with ice packs, and quickly transported back to the laboratory. After removing stones, residual roots, and debris, the sample was divided into two sub-samples. One sub-sample was stored at 4°C to determine soil ammonium nitrogen, nitrate nitrogen, and microbial biomass nitrogen; the other sub-sample was air-dried and passed a 2 mm sieve for the determination of soil N-cycle enzyme activities and other physicochemical properties.
2.4 Soil physicochemical properties and nitrogen component analysis
Soil total nitrogen (TN) was determined using the semi-micro Kjeldahl distillation-titration method (Lu, 2000). Soil ammonium nitrogen and nitrate nitrogen were determined by leaching-distillation of the KCl solution method (Riley et al., 2001). Chloroform fumigation with K2SO4 solution leaching was used for determining microbial biomass nitrogen (MBN) (Brookes et al., 2002). Soil water content (SWC) and temperature (TEM) were recorded at 0-10 cm, 10-20 cm, and 20-40 cm depths using 5 TM and EC-TM sensors, respectively, during May 2019 and October 2022; sensors were connected to a datalogger (Em 50G, Decagon, USA) and data were automatically transferred to the logger every 10 minutes.
2.5 Soil N-cycle enzyme activity analysis
The soil urease and protease activities were analyzed following Guan et al. (1986). For urease activity, air-dried soil (3 g) was incubated with 6 ml urea solution and 12 ml citrate buffer for 24 h at 37°C. At the end of the incubation, 4 ml of sodium phenolate solution and 3 ml of NaClO solution were added, and then the soil suspension was shaken for 30 min. After the solution reached room temperature, it was assayed at 578 nm using a spectrophotometer (UV-2450, Shimadzu, Kyoto, Japan). For measuring protease activities, 2 g of air-dried soil was incubated for 24 h at 37°C with 20 ml 1% casein solution and 0.1 ml toluene. At the end of the incubation, 0.5 ml 0.1 N NaClO solution and 3 ml Na2SO4 solution were added, and the soil suspension was shaken for 30 minutes, then centrifuged for 15 min (6000 r/min), and 1 ml 2% ninhydrin solution was added, and the solution was bathed in boiling water for 10 min, the solution was assayed colorimetrically at 560 nm using a spectrophotometer.
Soil nitrate reductase activity was determined colorimetrically using potassium nitrate as a substrate (Abdelmagid and Tabatabai, 1987). The air-dried soil samples (1 g) were incubated with 1 ml of 0.8 M 2,4-dinitrophenol solution, 1 ml of 0.1 M potassium nitrate solution,1 ml of 0.1 M glucose solution, and 5 ml distilled water at 30°C for 24 h. After incubation, 1 ml Alumina potassium alum saturated solution was added to the soil samples. And shaken for 30 minutes and filtered. Afterward, 1 ml of the filtrate was mixed with 4 ml of a color reagent (α-naphthylamine-sulfanilic acid) and mixed for 15 min. The optical density was then observed in a spectrophotometer against the blank at 520 nm. Soil nitrite reductase activity was determined by a modified colorimetric method using sodium nitrite as a substrate (Schinner et al., 1996). Triplicates of 1 g soil samples were incubated with 2 ml of Sodium nitrite solution (0.25 M), and 5 ml distilled water at 30°C for 24 h. The subsequent assay was performed similarly to the nitrate reductase activity assay.
We measured the four soil N-cycle enzyme activities with both substrate-free (substituted with water) and soil-free controls to illustrate the hydrolysis of non-enzymatic substrates.
2.6 Statistical analyses
Prior to the data analysis, we tested the data for normal distribution and homogeneity of variances using the Shapiro-Wilk test and Levene’s test, respectively. Moreover, performed a natural logarithm transformation on data that did not satisfy the normal distribution. One-way ANOVA was used to determine the effect of vegetation degradation and soil depth on soil properties, followed by Tukey’s HSD post hoc test for multiple comparisons (p < 0.05). We used the lme4 package to perform the linear mixed model (LMM) analysis to test the effects of vegetation degradation levels, soil depth and their interactions on soil N-cycle enzyme activities (Bates et al., 2014). Redundancy analysis (RDA) was performed on environmental indicators and N-cycle enzyme activities using the vegan package (Oksanen et al., 2013), and the Monte Carlo permutation test (n = 999) and forward selection were applied to analyze the effects of environmental factors on soil N-cycle enzyme activities. The contribution of each explanatory variable was decomposed based on hierarchical partitioning theory using the rdacca.hp package (Lai et al., 2022). All the analyses were carried out in R (R Core Team, 2022). Graphic illustrations were generated using Origin 2022 software (Origin Lab Corporation, Northampton, MA, USA).
3 Results
3.1 Variation of soil water content and temperature under different levels of vegetation degradation
As the degradation of wet meadow vegetation increases, soil temperature rised while soil water content decreased (Figure 3). In all soil layers, the soil water content followed a sequence of ND > LD > MD > HD, and the change in water content tended to level off as the soil layer deepens. The average soil water content in the 0–10 cm layer was 33.10 m3·m-3, higher than in the 10–20 cm layer (28.40 m3·m-3) and the 20–40 cm layer (23.60 m3·m-3). Soil water content in ND and LD plots tended to decrease gradually over time, while increased gradually in MD plots. Soil temperature in each soil layer showed a single-peaked curve over time, reached a maximum in the middle of August. The average soil temperature in the 0–10 cm soil layer was 11.90°C, higher than in the 10–20 cm layer (11.52°C) and the 20–40 cm layer (11.25°C).
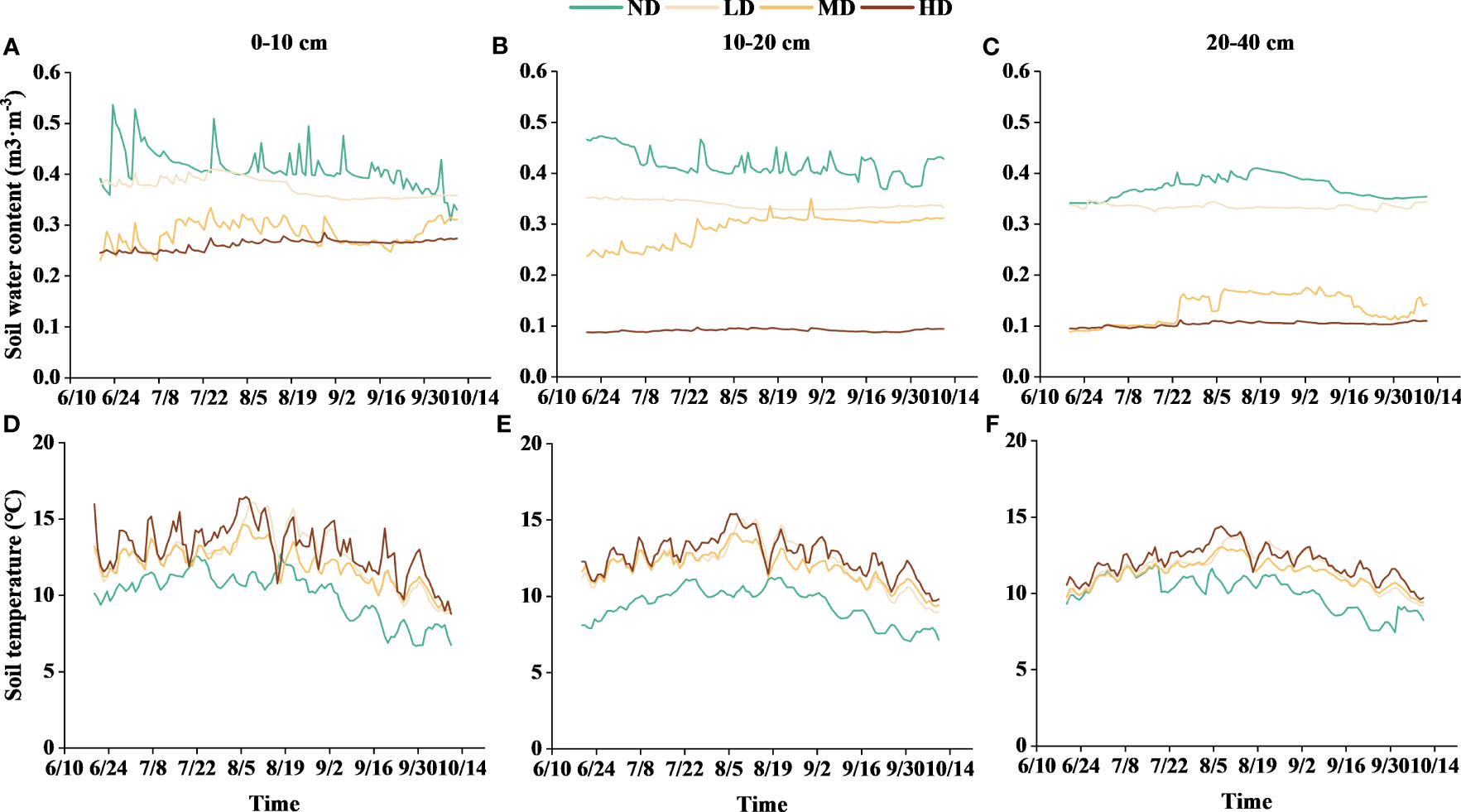
Figure 3 Soil water content and temperature in the 0-40 cm soil layers at different levels of vegetation degradation from June 20th to October 11th. (A), (B) and (C) show the soil water content in the 0-10 cm, 10-20 cm and 20-40 cm soil layers, respectively; (D), (E) and (F) show the soil temperature in the 0-10 cm, 10-20 cm and 20-40 cm soil layers, respectively. ND, non-degraded; LD, lightly degraded; MD, moderately degraded; HD, heavily degraded.
3.2 Variation of soil N fraction content under different levels of vegetation degradation
Soil nitrate-nitrogen contents increased from 4.33 mg·kg-1 at the ND plots to 8.25 mg·kg-1 at the HD, an increase of 90.28%. Soil total nitrogen, ammonium nitrogen, and microbial biomass nitrogen contents decreased during the process of vegetation degradation, varied from 3.27 to 1.96 g·kg-1, 6.37 to 3.65 mg·kg-1, and 48.06 to 27.46 μg·kg-1 respectively (Table 2). Except for ammonium and nitrate nitrogen in the LD, the remaining properties in the LD, MD, and HD were significantly different from those in the ND (p < 0.05). In addition, soil N inorganic contents varied across the vertical soil profile. Soil ammonium nitrogen and microbial biomass nitrogen alone appear to have a general downward trend in the soil depth series at the four degradation levels. In contrast, soil nitrate nitrogen contents were increased. The total nitrogen contents were decreased across soil depths at ND, LD, and MD plots, but the corresponding values were increased at HD.
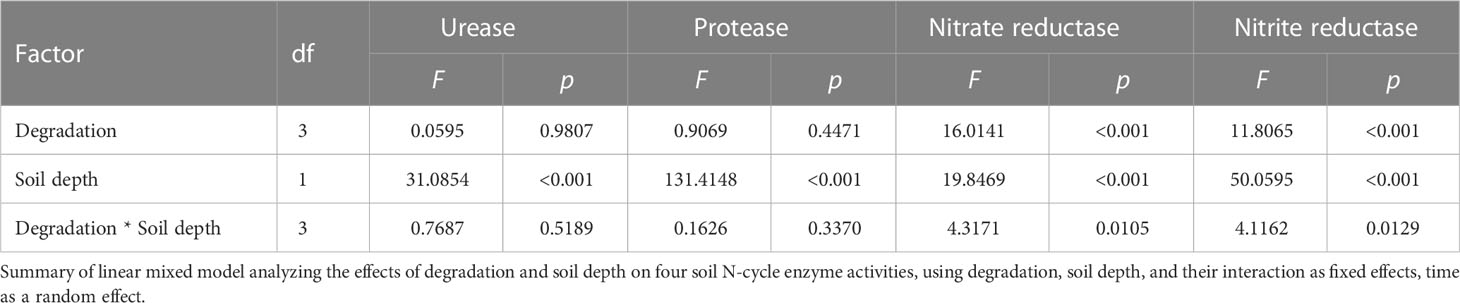
Table 2 Results of linear mixed model analysis testing the effects of degradation and soil depth on soil N-cycle enzyme activities.
3.3 Variation of soil N-cycle enzyme activities in different levels of vegetation degradation
The activities of soil urease, protease, nitrate reductase, and nitrite reductase changed remarkably during the process of vegetation degradation (p < 0.05) (Figure 4). Vegetation degradation decreased the urease and nitrate reductase activities but increased the protease and nitrite reductase activities, with more pronounced effects in the upper soil (0–10 and 10–20 cm) than in the deeper soil (20–40 cm). With the increase of soil depth, soil urease, protease, and nitrite reductase activities decreased. However, soil nitrate reductase activities increased in ND, LD, and MD, while first decreased and then increased in HD. The linear mixed model showed that vegetation degradation and soil depth had significant effects on soil N-cycle enzyme activities (p < 0.001) (Table 3). Significant interaction effects of vegetation degradation and soil depth were observed in the nitrite reductase activity (p < 0.001).
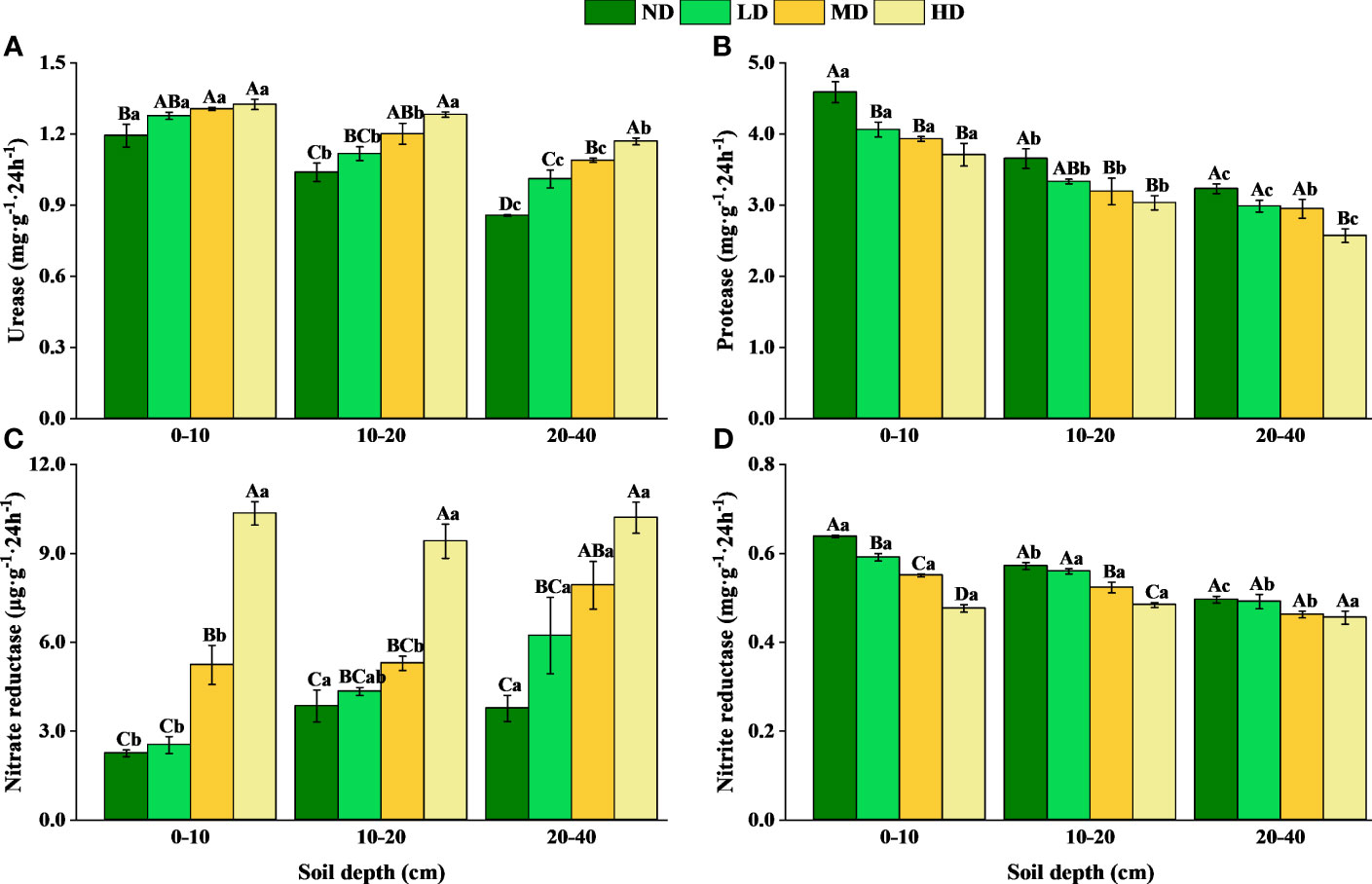
Figure 4 Soil N-cycle enzyme activities in different levels of vegetation degradation (mean ± sd, n = 3). Urease activity (A); Protease activity (B); Nitrate reductase activity (C); Nitrite reductase activity (D). ND, non-degraded; LD, lightly degraded; MD, moderately degraded; HD, heavily degraded. Different capital letters indicate significant differences between degradation levels (p < 0.05), and different lowercase letters indicate significant differences between soil layers (p < 0.05).
3.4 Temporal variation in soil N-cycle enzyme activities in different levels of vegetation degradation
The four soil N-cycle enzyme activities showed notable temporal fluctuations in the 0–40 cm soil layer under the four vegetation degradation levels (Figure 5). In all vegetation degradation levels, soil urease and nitrite reductase activities peaked in August, with urease activities ranged from 1.12 to 1.60 mg·g-1·24h-1 and nitrite reductase activities ranged from 0.52 to 0.63 mg·g-1·24h-1. The urease and nitrite reductase enzyme activities at four degradation levels had significant differences (p < 0.05) throughout the growing season. Soil protease activity was lowest in June and highest in July. Soil nitrate reductase activity fluctuated from June to August and increased significantly in September. The mean values of soil protease and nitrate reductase enzyme activities in LD, MD, and HD were significantly lower than those in ND (p < 0.05).
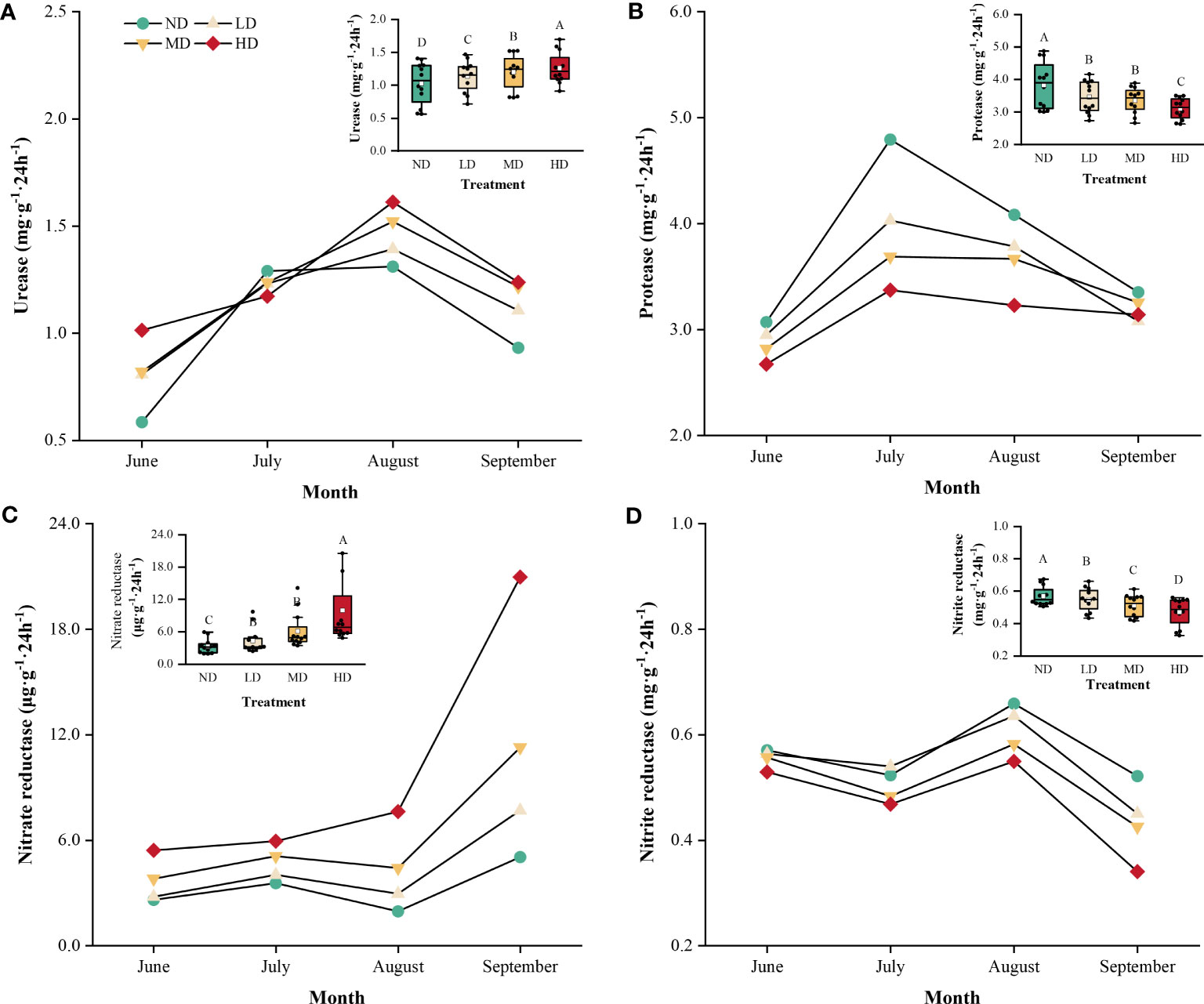
Figure 5 Temporal dynamics of soil N-cycle enzyme activities in wet meadows with different levels of vegetation degradation. Urease activity (A); Protease activity (B); Nitrate reductase activity (C); Nitrite reductase activity (D). The average values of soil N-cycle enzyme activities at each degradation level are shown in the box plots. Capital letters indicate the result of the post hoc Tukey’s HSD multiple range test for variations in soil enzyme activities among wet meadows with different levels of degradation. ND, non-degraded; LD, lightly degraded; MD, moderately degraded; HD, heavily degraded.
3.5 Relationships among soil variables and N-cycle enzyme activities
The four soil N-cycle enzyme activities (urease, protease, nitrate reductase, and nitrite reductase) were positively correlated with TEM (Figure 6). Urease activity was negatively with TN and , but positively correlated with and MBN. Protease activity was significantly and positively correlated with , , MBN, and SWC. A positive correlation between protease activity and TN was observed, although the correlation coefficient was relatively low. Meanwhile, TN, , MBN, and SWC were negatively with nitrate reductase activity while positively correlated with nitrite reductase activity; was positively correlated with nitrate reductase activity, while negatively with nitrite reductase activity.
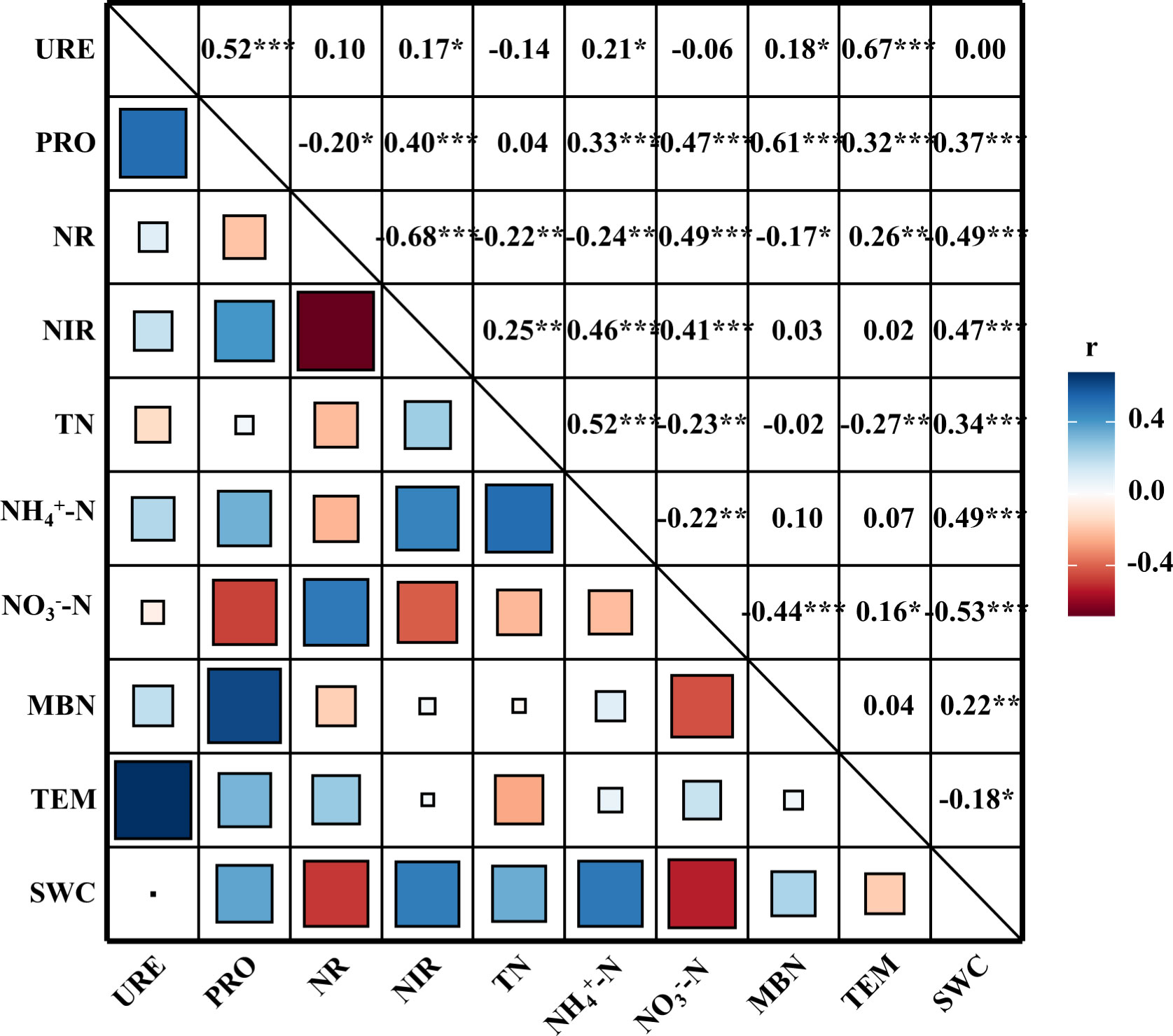
Figure 6 The Pearson correlation coefficient between soil N-cycle enzyme activity and environmental factors. URE, urease; PRO, protease; NR, nitrate reductase; NIR, nitrite reductase; TN, total nitrogen; , ammonium nitrogen; , nitrate nitrogen; MBN, microbial biomass nitrogen; SWC, soil water content; TEM, temperature. *, **, and *** represent p < 0.05, p < 0.01, and p < 0.001, respectively.
RDA showed that the first two axes explained a total of 78.37% (June), 65.25% (July), 81.72% (August), and 56% (September) of the total variation in soil N-cycle enzyme activities (p < 0.001) (Figure 7). and SWC explained 42.43% and 16.37% of soil N-cycle enzyme activities in June, respectively; MBN and explained 33.66% and 22.45% in July, respectively; TN and explained 25.78% and 21.92% in August respectively; MBN and explained 23.63% and 20.17% in September respectively.
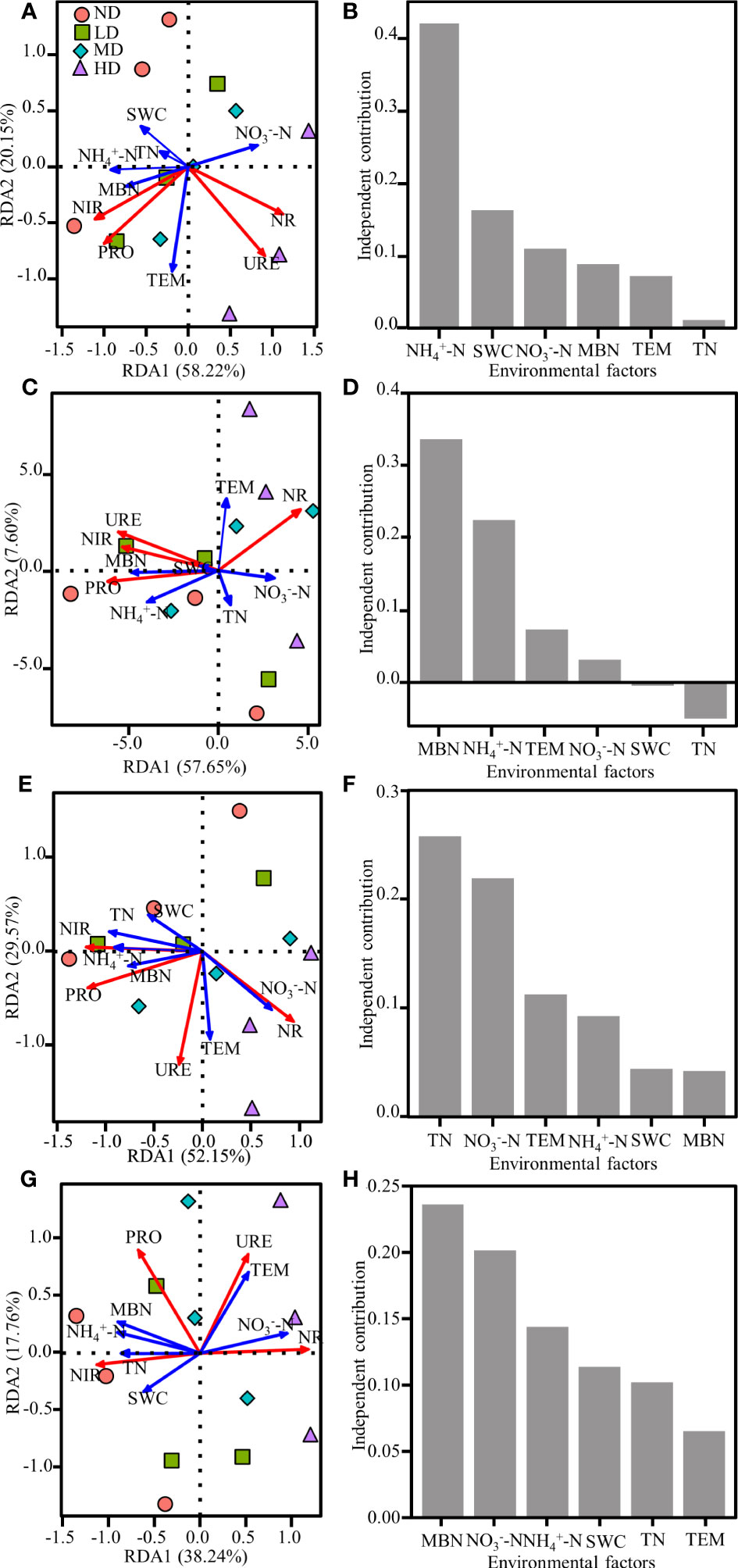
Figure 7 Redundancy analysis (RDA) between soil N-cycle enzyme activities and soil physical and chemical properties. (A), (C), (E), and (G) showed the RDA two-dimensional ranking diagram for June, July, August and September, respectively, the blue arrows represent soil physical and chemical properties, and the red arrows represent soil N-cycle enzyme activities. The length of the arrows represents the degree of influence between soil physicochemical properties and N-cycle enzyme activity. (B), (D), (F), and (H) showed the effects of soil microenvironments and nitrogen content on soil N-cycle enzyme activities in June, July, August and September, respectively. URE, urease; PRO, protease; NR, nitrate reductase; NIR, nitrite reductase; TN, total nitrogen; , ammonium nitrogen; , nitrate nitrogen; MBN, microbial biomass nitrogen; SWC, soil water content; TEM, temperature.
4 Discussion
4.1 Responses of soil N-cycle enzyme activities to vegetation degradation
Soil enzymes, produced by both soil microorganisms and plant roots, serve an essential role in facilitating biogeochemical cycling in terrestrial ecosystems (Sinsabaugh et al., 2009). Soil urease facilitates the hydrolysis of urea to ammonia (Su et al., 2004), while protease promotes the hydrolysis of proteins and peptides to amino acids, which are both involved in the soil N-cycle and are essential indicators of soil nitrogen availability (Wallenstein et al., 2009). Our study found that soil urease activity was significantly higher other degraded sites compared to non-degraded ones (Figure 3). We argue that vegetation degradation may increase soil urease activity in wet meadows through changes in the soil microclimate and nutrient availability. Degradation of wet meadow vegetation has impacted the regional water table and soil temperature, leading to alterations in the type abundance of soil microbes. The lack of vegetation cover can increase surface soil temperatures, and urease activity is known to be more sensitive to temperature changes (Sardans et al., 2008). The increase in temperature will break the regional low-temperature limitation effect, increasing enzyme secretion by soil microorganisms and improving the production of soil urease (Burns et al., 2013). This was confirmed by the strong positive correlation between soil urease activity and soil temperature (Figure 6). In addition, vegetation degradation may increase certain soil nutrient contents (Table 2), thus accelerating organic matter decomposition and nutrient cycling, increasing in microbial activity and urease. However, it must be noted that while increased urease activity may provide short-term benefits, it may also lead to long-term degradation of wetland ecosystems by changing the chemical and physical properties of the soil and reducing its ability to support healthy vegetation.
Contrarily, vegetation degradation led to a significant decrease in soil protease activity (Figure 4). It indicates that temperature was not the primary factor influencing the variability of soil protease activity in wet meadows. Previous research has suggested that soil protease activity is primarily influenced by soil type, vegetation, and plant biomass (Song et al., 2013). On the one hand, during the degradation of wet meadows, with the continuous reduction of soil water, the changing trend of plant succession pattern is from aquatic to wet to moderate to dry. Vegetation degradation significantly reduced vegetation height, cover, species richness, and above-ground biomass (Ma et al., 2018). This may have resulted in a reduced amount of vegetation litter and root exudates, thus a decrease in the substrate source material, leading to a reduction in protease activity (Vranova et al., 2013). There result support our first hypothesis (a). On the other hand, the gradual decrease in topsoil moisture due to vegetation degradation reduces enzymatic reactions (Table 2) (Li et al., 2022d). To some extent, it affects the source of protease substrates and their diffusion and synthesis rates (Sardans et al., 2008; Brockett et al., 2011), leading to a reduction in protease activity. It is consistent with the findings of the reduced protease activity as a result of degradation in the Zoige Alpine wetlands (Huo et al., 2013).
Soil nitrate reductase is able to catalyze to , while nitrite reductase catalyzes to N2O or (Szajdak and Gaca, 2010; Li et al., 2015). Both of them are the dominant enzymes involved in soil denitrification and the allosteric assimilation of to (Coyotzi et al., 2017; Jin et al., 2019). Our study demonstrated that vegetation degradation led to a significant increased in soil nitrate reductase activity but decreased in nitrite reductase activity (Figure 4). There was a close relationship between soil nitrogen content and nitrate reductase and nitrite reductase activities (Figure 7). The main reason for there results was that nitrate nitrogen was involved in soil denitrification as a substrate. The increase in soil nitrate nitrogen content due to vegetation degradation could lead to a rise in the rate of nitrate reductase synthesis (Deiglmayr et al., 2006). As previously reported, soil nitrate reductase was a substrate-inducing enzyme, and its activity was easily induced by nitrate (Wang et al., 2000), hence nitrate reductase activity may be increased with increasing nitrate nitrogen content. Vegetation degradation caused coupling between soil N-cycle enzyme activity and soil nutrient content in wet meadows. Multiple factors acted together in soil nitrogen cycling in wet meadows, and our results corroborate previous studies on wetland ecosystems under vegetation degradation (Song et al., 2014; Fan et al., 2021).
4.2 Response of soil N-cycle enzyme activities to soil depth
Soil N-cycle enzyme activities in wet meadows exhibited a significant difference at varying soil depths (Figure 4). We found that surface enzyme activities were higher compared to those in deeper layers for all vegetation degradation levels, except for nitrate reductase activity. Such difference may be due to the surface soil having more nutrient contents than the deep soil (Peng and Wang, 2016), and these results supported our second hypothesis. In a previous study, we found out that the soils in the region are shallow, the vegetation is dominated by herbaceous plants with a shallow and underdeveloped root system (Ma et al., 2020). The reduction of plant roots often leads to a decreased in soil enzyme activity (Xiao et al., 2015), and a weakening effect of roots on N-cycle enzyme activity in deep soils (Enriquez et al., 2015). In addition, the presence of apoplast and humus in the surface layer increases the content of soil organic matter, which acts as a precursor for enzyme synthesis Song et al., 2014; Ai et al., 2023). Furthermore, the concentration of enzyme substrates is more abundant in the surface soil layer than in deep layers (Niemi et al., 2005), which further reduces soil enzyme activity (Acosta-Martínez et al., 2006). Surface soils also possess a better structure, porosity composition, and agglomerates and are more susceptible to external disturbances than deep soils (Pan et al., 2020). In particular, the metabolic enzymatic capacity of soil microorganisms is inhibited by the weakening of hydrothermal conditions in the soil profile (Xiao et al., 2015). Soil water content not only directly affects the growth of soil microorganisms and nutrient effectiveness but also indirectly changes the magnitude of soil enzyme activity by altering soil oxygen content and leaching (Fan et al., 2021). Finally, soil nutrient effectiveness decreases as soil depth increases, leading to a decline in microbial activity and fecundity (Xiao et al., 2015). These are in line with the changes in soil enzyme activity under vegetation degradation in other studies (Ananbeh et al., 2019; Li et al., 2020).
4.3 Temporal dynamics of soil N-cycle enzyme activities
Our study observed evident temporal dynamics change of N-cycle enzyme activities, and the peak values of soil urease, protease, and nitrite reductase activities were occurred in July and August (Figure 5), which aligns with our third hypothesis. Soil enzyme production and turnover rates may be influenced by changes in hydrothermal conditions (Kivlin and Treseder, 2014). In the QTP region, vegetation starts to turn green in May due to the low temperature and low soil nutrient content of the soil, and the above-ground part of the vegetation grows relatively slowly in June, when the main nutrients are still in the ground (Wu et al., 2020b), and soil organic matter content is high, leading to gradual improvement in soil N-cycle enzyme activity. During July and August, the temperature and precipitation exhibited optimal conditions (Figure 2). These favorable environmental factors promote plant metabolism and concomitant augmentation in root secretions (Finzi et al., 2006), consequently enhanced soil microbial activity. The favorable hydrothermal conditions increase the contact area between soil enzymes and substrates, further increasing the soil N-cycle enzyme activity (Kotroczó et al., 2014). As soil temperature and moisture declined in September, unfavorable hydrothermal conditions inhibited microbial growth, reproductive metabolism, and other activities (Cui et al., 2019), leading to a reduction in soil N-cycle enzyme activities.
In wet meadow vegetation undergoes different growth stages and phenological changes throughout the season (Shen et al., 2023). Plants input organic matter into the soil during growth by exuding root secretions. These organic substances act as substrates for soil enzymes, thus stimulating soil enzyme activity (Li et al., 2022b). On the contrary, when plants are dormant or senescent, the decrease in organic matter content leads to a decrease in the activity of soil enzymes (Burns et al., 2013; Wang et al., 2022). At the same time, differences in plant growth and nutrient requirements with different periods lead to changes in the input of substrate sources (Shen et al., 2022a), and subsequently affect the temporal dynamics of soil enzyme activity.
Furthermore, as an integral part of the wetland hydrologic system, wet meadows are subject to seasonal fluctuations in water levels (Cao et al., 2017). This seasonal variation causes significant differences in the hydrothermal conditions and oxygen exchange rates of the soil (Freeman et al., 1996), which can particularly affect changes in the activity of soil nitrate reductase and nitrite reductase (Li et al., 2012), as they are more sensitive to changes in soil water content (Figure 6). Specifically, soils may be inundated during high water table, leading to reduced oxygen utilization and altered microbial activity. While, during low water table, the soil becomes drier (Engelaar et al., 1995), potentially affecting the enzyme-substrate interactions and ultimately the soil enzyme activity (Pu et al., 2019). In conclusion, through the combined effects of different biotic factors (vegetation growth rhythms) and abiotic factors (soil hydrothermal conditions) (Brockett et al., 2011). Resulting in dynamic changes in soil N-cycle enzyme activity in wet meadows with different vegetation degradation, thus affecting soil nutrient cycling and other biogeochemical processes.
5 Conclusions
In the present study, we analyzed the changes of soil nitrogen contents and related soil N-cycle enzyme activities to different levels of vegetation degradation in the northeastern Qinghai-Tibet Plateau wet meadows. The results showed that the vegetation degradation in wet meadows reduced soil water content, total nitrogen, ammonium nitrogen, and microbial biomass nitrogen content in the 0–40 cm soil layer but increased soil temperature and nitrate nitrogen content. Vegetation degradation also reduced soil protease and nitrite reductase activities while raised soil urease and nitrate reductase activities, which could further aggravate wet meadow degradation. The degree of degradation and soil depth had significant effects on soil N-cycle enzyme activities, with soil urease, protease, and nitrite reductase activities showing significant surface aggregation. Soil ammonium and nitrate nitrogen contents were the most important abiotic drivers of soil N-cycle enzyme activities during the degradation succession. In conclusion, our findings provide useful insights into the soil N-cycle enzyme activities of degraded wet meadows on the QTP, which are essential for assessing the soil N-cycle under alpine wetland degradation.
Data availability statement
The original contributions presented in the study are included in the article/Supplementary Material. Further inquiries can be directed to the corresponding author.
Author contributions
WC: Conceptualization, Methodology, Data curation, Software, Formal analysis, Writing - original draft. WM: Supervision, Project administration, Funding acquisition, Writing - review and editing. LS: Investigation. YT: Methodology. YL: Investigation. GX: Visualization, Writing - review. JY: Investigation, Writing - review. All authors contributed to the article and approved the submitted version.
Funding
This study was supported by the Gansu Agricultural University Youth Mentorship Fund Project (GAU-QDFC-2021-11), the National Natural Science Foundation of China (31860143), the Gansu Provincial Education Department: Young Doctoral Fund Project (2021QB-024), the Gansu Provincial Education Department: Industrial Support Program Project (2021CYZC-15).
Acknowledgments
We would like to thank all the people who were involved in the field and laboratory work. Many thanks to the staff of the Gahai field stations for their assistance with sample collection.
Conflict of interest
The authors declare that the research was conducted in the absence of any commercial or financial relationships that could be construed as a potential conflict of interest.
Publisher’s note
All claims expressed in this article are solely those of the authors and do not necessarily represent those of their affiliated organizations, or those of the publisher, the editors and the reviewers. Any product that may be evaluated in this article, or claim that may be made by its manufacturer, is not guaranteed or endorsed by the publisher.
Supplementary material
The Supplementary Material for this article can be found online at: https://www.frontiersin.org/articles/10.3389/fevo.2023.1210643/full#supplementary-material
References
Abdelmagid H. M., Tabatabai M. A. (1987). Nitrate reductase activity of soils. Soil Biol. Biochem. 19, 421–427. doi: 10.1016/0038-0717(87)90033-2
Acosta-Martínez V., Cruz L., Sotomayor-Ramírez D., Pérez-Alegría L. (2006). Enzyme activities as affected by soil properties and land use in a tropical watershed. Appl. Soil Ecol. 35, 35–45. doi: 10.1016/j.apsoil.2006.05.012
Ai L., Wu F. Z., Fan X. B., Yang Y., Zhang Y., Zheng X. P., et al. (2023). Different effects of litter and root inputs on soil enzyme activities in terrestrial ecosystems. Appl. Soil Ecol. 183, 104764. doi: 10.1016/j.apsoil.2022.104764
Ananbeh H., Stojanović M., Pompeiano A., Voběrková S., Trasar-Cepeda C. (2019). Use of soil enzyme activities to assess the recovery of soil functions in abandoned coppice forest systems. Sci. Total Environ. 694, 133692. doi: 10.1016/j.scitotenv.2019.133692
Bates D., Mächler M., Bolker B., Walker S. (2014). Fitting linear mixed-effects models using lme4. J. Stat. Softw 67, 1–48. doi: 10.18637/jss.v067.i01
Brockett B. F. T., Prescott C. E., Grayston S. J. (2011). Soil moisture is the major factor influencing microbial community structure and enzyme activities across seven biogeoclimatic zones in western Canada. Soil Biol. Biochem. 44, 9–20. doi: 10.1016/j.soilbio.2011.09.003
Brookes P. C., Landman A., Pruden G., Jenkinson D. S. (2002). Chloroform fumigation and the release of soil nitrogen: a rapid direct extraction method to measure microbial biomass nitrogen in soil. Soil Biol. Biochem. 17, 837–842. doi: 10.1016/0038-0717(85)90144-0
Burns R. G., DeForest J. L., Marxsen J., Sinsabaugh R. L., Stromberger M. E., Wallenstein M. D., et al. (2013). Soil enzymes in a changing environment: Current knowledge and future directions. Soil Biol. Biochem. 58, 216–234. doi: 10.1016/j.soilbio.2012.11.009
Caldwell B. A. (2005). Enzyme activities as a component of soil biodiversity: a review. Pedobiologia 49, 637–644. doi: 10.1016/j.pedobi.2005.06.003
Cao R., Wei X., Yang Y. H. S., Xi X. Q., Wu X. W. (2017). The effect of water table decline on plant biomass and species composition in the Zoige peatland: a four-year in situ field experiment. Agr. Ecosyst. Environ. 247, 389–395. doi: 10.1016/j.agee.2017.07.008
Chen X., Feng J. G., Ding Z. J., Tang M., Zhu B. (2021). Changes in soil total, microbial and enzymatic C-N-P contents and stoichiometry with depth and latitude in forest ecosystems. Sci. Total Environ. 816, 151583. doi: 10.1016/j.scitotenv.2021.151583
Chen H., Zhu Q., Peng C. H., Wu N., Wang Y. F., Fang X. Q., et al. (2013). The impacts of climate change and human activities on biogeochemical cycles on the Qinghai-Tibetan Plateau. Glob. Change Biol. 19, 2940–2955. doi: 10.1111/gcb.12277
Core Team R. (2022). R: A Language and Environment for Statistical Computing (Vienna, Austria: R Foundation for Statistical Computing). Available at: https://www.R-project.org/.
Coyotzi S., Doxey A. C., Clark I. D., Lapen D. R., Van Cappellen P., Neufeld J. D. (2017). Agricultural soil denitrifiers possess extensive nitrite reductase gene diversity. Environ. Microbiol. 19, 1189–1208. doi: 10.1111/1462-2920.13643
Cui Y. X., Bing H. J., Fang L. C., Jiang M., Shen G. T., Yu J. L., et al. (2019). Extracellular enzyme stoichiometry reveals the carbon and phosphorus limitations of microbial metabolisms in the rhizosphere and bulk soils in alpine ecosystems. Plant Soil 458, 7–20. doi: 10.1007/s11104-019-04159-x
Davidson N. C. (2014). How much wetland has the world lost? Long-term and recent trends in global wetland area. Mar. Freshw. Res. 65, 934–941. doi: 10.1071/MF14173
Deiglmayr K., Philippot L., Kandeler E. (2006). Functional stability of the nitrate-reducing community in grassland soils towards high nitrate supply. Soil Biol. Biochem. 38, 2980–2984. doi: 10.1016/j.soilbio.2006.04.034
Dong L. L., Sun T., Berg B., Zhang L. L., Zhang Q. Q., Wang Z. W. (2019b). Effects of different forms of N deposition on leaf litter decomposition and extracellular enzyme activities in a temperate grassland. Soil Biol. Biochem. 134, 78–80. doi: 10.1016/j.soilbio.2019.03.016
Dong C. C., Wang W., Liu H. Y., Xu X. T., Zeng H. (2019a). Temperate grassland shifted from nitrogen to phosphorus limitation induced by degradation and nitrogen deposition: Evidence from soil extracellular enzyme stoichiometry. Ecol. Indic. 101, 453–464. doi: 10.1016/j.ecolind.2019.01.046
Dunn C., Jones T. G., Girard A., Freeman C. (2014). Methodologies for extracellular enzyme assays from wetland soils. Wetlands 34, 9–17. doi: 10.1007/s13157-013-0475-0
Engelaar W. M. H. G., Symens J. C., Laanbroek H. J., Blom C. W. P. M. (1995). Preservation of nitrifying capacity and nitrate availability in waterlogged soils by radial oxygen loss from roots of wetland plants. Biol. Fertil. Soils 20, 243–248. doi: 10.1007/BF00336084
Enriquez A. S., Chimner R. A., Cremona M. V., Diehl P., Bonvissuto G. L. (2015). Grazing intensity levels influence C reservoirs of wet and mesic meadows along a precipitation gradient in Northern Patagonia. Wetlands Ecol. Manage. 23, 439–451. doi: 10.1007/s11273-014-9393-z
Fan S. Y., Sun H., Yang J. Y., Qin J. H., Shen D. J., Chen Y. X. (2021). Variations in soil enzyme activities and microbial communities along an altitudinal gradient on the eastern Qinghai–Tibetan Plateau. Forests 12, 681. doi: 10.3390/f12060681
Finzi A. C., Sinsabaugh R. L., Long T. M., Osgood M. P. (2006). Microbial community responses to atmospheric carbon dioxide enrichment in a Warm-Temperate Forest. Ecosystems 9, 215–226. doi: 10.1007/s10021-005-0078-6
Fluet-Chouinard E., Stocker B. D., Zhang Z., Malhotra A., Melton J. R., Poulter B., et al. (2023). Extensive global wetland loss over the past three centuries. Nature 614, 281–286. doi: 10.1038/s41586-022-05572-6
Freeman C., Liska G., Ostle N. J., Lock M. A., Reynolds B., Hudson J. (1996). Microbial activity and enzymic decomposition processes following peatland water table drawdown. Plant Soil 180, 121–127. doi: 10.1007/BF00015418
Gu Y. F., Bai Y., Xiang Q. J., Yu X. M., Zhao K., Zhang X. P., et al. (2018). Degradation shaped bacterial and archaeal communities with predictable taxa and their association patterns in Zoige wetland at Tibet plateau. Sci. Rep. 8, 1–11. doi: 10.1038/s41598-018-21874-0
Guan S. Y., Zhang D., Zhang Z. (1986). Soil Enzyme and Its Research Methods (Beijing: Agricultural Press).
Hirota M., Tang Y. H., Hu Q. W., Kato T., Hirata S., Mo W., et al. (2005). The potential importance of grazing to the fluxes of carbon dioxide and methane in an alpine wetland on the Qinghai-Tibetan Plateau. Atmos. Environ. 39, 5255–5259. doi: 10.1016/j.atmosenv.2005.05.036
Hu S. J., Niu Z. G., Chen Y. F., Li L. F., Zhang H. Y. (2017). Global wetlands: Potential distribution, wetland loss, and status. Sci. Total Environ. 586, 319–327. doi: 10.1016/j.scitotenv.2017.02.001
Huo L. L., Chen Z. K., Zou Y. C., Lu X., Guo J. W., Tang X. G. (2013). Effect of Ruoerge alpine wetland degradation on the density and fractions of soil organic carbon. Ecol. Eng. 51, 287–295. doi: 10.1016/j.ecoleng.2012.12.020
Janse J. H., van Dam A. A., Hes E. M. A., de Klein J. J. M., Finlayson C. M., Janssen A. B. G., et al. (2019). Towards a global model for wetlands ecosystem services. Curr. Opin. Env. Sust. 36, 11–19. doi: 10.1016/j.cosust.2018.09.002
Jin P., Chen Y. Y., Yao R., Zheng Z. W., Du Q. Z. (2019). New insight into the nitrogen metabolism of simultaneous heterotrophic nitrification-aerobic denitrification bacterium in mRNA expression. J. Hazard. Mater. 371, 295–303. doi: 10.1016/j.jhazmat.2019.03.023
Kivlin S. N., Treseder K. K. (2014). Soil extracellular enzyme activities correspond with abiotic factors more than fungal community composition. Biogeochemistry 117, 23–37. doi: 10.1007/s10533-013-9852-2
Kotroczó Z., Veres Z., Fekete I., Krakomperger Z., Tóth J. A., Lajtha K., et al. (2014). Soil enzyme activity in response to long-term organic matter manipulation. Soil Biol. Biochem. 70, 237–243. doi: 10.1016/j.soilbio.2013.12.028
Lai J. S., Zou Y., Zhang J. L., Peres-Neto P. R. (2022). Generalizing hierarchical and variation partitioning in multiple regression and canonical analyses using the rdacca.hp R package. Methods Ecol. Evol. 13, 782–788. doi: 10.1111/2041-210X.13800
Laurance S. G. W., Baider C., Vincent Florens F. B., Ramrekha S., Sevathian J.-C., Hammond D. S. (2012). Drivers of wetland disturbance and biodiversity impacts on a tropical oceanic island. Biol. Conserv. 149, 136–142. doi: 10.1016/j.biocon.2011.12.015
Li Y. Y., Dong S. K., Wen L., Wang X. X., Wu Y. (2012). Soil seed banks in degraded and revegetated grasslands in the alpine region of the Qinghai-Tibetan Plateau. Ecol. Eng. 49, 77–83. doi: 10.1016/j.ecoleng.2012.08.022
Li Z. F., Gao J. X., Wen L. Q., Zou C. X., Feng C. Y., Li D. Q., et al. (2019). Dynamics of soil respiration in alpine wetland meadows exposed to different levels of degradation in the Qinghai-Tibet Plateau, China. Sci. Rep. 9, 1–14. doi: 10.1038/s41598-019-43904-1
Li M., Hao Y. B., Yan Z. Q., Kang E. Z., Wang J. Z., Zhang K. R., et al. (2022c). Long-term degradation from marshes into meadows shifts microbial functional diversity of soil phosphorus cycling in an alpine wetland of the Tibetan Plateau. Land Degrad. Dev. 33, 628–637. doi: 10.1002/ldr.4180
Li Y., Hodak M., Bernholc J. (2015). Enzymatic mechanism of copper-containing nitrite reductase. Biochemistry 54, 1233–1242. doi: 10.1021/bi5007767
Li J. W., Li M., Zhao L. Y., Sun X. Q., Gao M. H., Sheng L. X., et al. (2022a). Characteristics of soil carbon emissions and bacterial community composition in peatlands at different stages of vegetation succession. Sci. Total Environ. 839, 156242. doi: 10.1016/j.scitotenv.2022.156242
Li T., Peng C. H., Bu Z. J., Zhu Q., Song H. X., Guo X. Y., et al. (2021). Woody plants reduce the sensitivity of soil extracellular enzyme activity to nutrient enrichment in wetlands: a meta-analysis. Soil Biol. Biochem. 159, 108280. doi: 10.1016/j.soilbio.2021.108280
Li J. S., Shao X. Q., Huang D., Liu K. S., Shang J. Y., Zhang Q., et al. (2022b). Short-term biochar effect on soil physicochemical and microbiological properties of a degraded alpine grassland. Pedosphere 32, 426–437. doi: 10.1016/S1002-0160(21)60084-X
Li X. F., Zhang Y., Ding C. X., Liu Y., Wu K. X., Jiang F. Z., et al. (2020). Water addition promotes vegetation recovery of degraded alpine meadows by regulating soil enzyme activity and nutrients in the Qinghai–Tibetan Plateau. Ecol. Eng. 158, 106047. doi: 10.1016/j.ecoleng.2020.106047
Li M., Zhang K. R., Yan Z. Q., Liu L., Kang E. Z., Kang X. M. (2022d). Soil water content shapes microbial community along gradients of wetland degradation on the Tibetan Plateau. Front. Microbiol. 13. doi: 10.3389/fmicb.2022.824267
Lu R. K. (2000). Methods of Soil Agricultural Chemical Analysis (Beijing: Chinese Agricultural Science and Technology Press).
Ma W. W., Alhassan A. R. M., Wang Y. S., Li G., Wang H., Zhao J. M. (2018). Greenhouse gas emissions as influenced by wetland vegetation degradation along a moisture gradient on the eastern Qinghai-Tibet Plateau of North-West China. Nutr. Cycling Agroecosyst. 112, 335–354. doi: 10.1007/s10705-018-9950-6
Ma W. W., Li G., Wu J. H., Xu G. R., Wu J. Q. (2020). Response of soil labile organic carbon fractions and carbon-cycle enzyme activities to vegetation degradation in a wet meadow on the Qinghai–Tibet Plateau. Geoderma 377, 114565. doi: 10.1016/j.geoderma.2020.114565
Ma M. J., Zhou X. H., Du G. Z. (2011). Soil seed bank dynamics in alpine wetland succession on the Tibetan Plateau. Plant Soil 346, 19–28. doi: 10.1007/s11104-011-0790-2
Maneas G., Makopoulou E., Bousbouras D., Berg H., Manzoni S. (2019). Anthropogenic changes in a mediterranean coastal wetland during the last century—The case of Gialova Lagoon, Messinia, Greece. Water 11, 350. doi: 10.3390/w11020350
Masson-Delmotte V., Zhai P., Pirani A., Connors S. L., Péan C., Berger S., et al. (2021). Climate change 2021: the physical science basis. Contribution of working group I to the sixth assessment report of the intergovernmental panel on climate change. (Cambridge University Press).
Nannipieri P., Trasar-Cepeda C., Dick R. P. (2018). Soil enzyme activity: a brief history and biochemistry as a basis for appropriate interpretations and meta-analysis. Biol. Fertil. Soils 54, 11–19. doi: 10.1007/s00374-017-1245-6
Niemi R. M., Vepsäläinen M., Wallenius K., Simpanen S., Alakukku L., Pietola L. (2005). Temporal and soil depth-related variation in soil enzyme activities and in root growth of red clover (Trifolium pratense) and timothy (Phleum pratense) in the field. Appl. Soil Ecol. 30, 113–125. doi: 10.1016/j.apsoil.2005.02.003
Oksanen J., Blanchet F. G., Kindt R., Legendre P., Minchin P. R., O’hara R. B., et al. (2013). Vegan: Community ecology package. R package version 2.5-7. Available at: https://cran.r-project.org/web/packages/vegan/index.
Pan T., Hou S., Liu Y. J., Tan Q. H., Liu Y. H., Gao X. F. (2020). Influence of degradation on soil water availability in an alpine swamp meadow on the eastern edge of the Tibetan Plateau. Sci. Total Environ. 722, 137677. doi: 10.1016/j.scitotenv.2020.137677
Peng X. Q., Wang W. (2016). Stoichiometry of soil extracellular enzyme activity along a climatic transect in temperate grasslands of northern China. Soil Biol. Biochem. 98, 74–84. doi: 10.1016/j.soilbio.2016.04.008
Pu Y. L., Zhu B., Dong Z. X., Liu Y., Wang C. Q., Ye C. (2019). Soil N2O and NOx emissions are directly linked with N-cycling enzymatic activities. Appl. Soil Ecol. 139, 15–24. doi: 10.1016/j.apsoil.2019.03.007
Riley W. J., Ortiz-Monasterio I., Matson P. A. (2001). Nitrogen leaching and soil nitrate, nitrite, and ammonium levels under irrigated wheat in Northern Mexico. Nutr. Cycling Agroecosyst. 61, 223–236. doi: 10.1023/A:1013758116346
Sardans J., Peñuelas J., Estiarte M. (2008). Changes in soil enzymes related to C and N cycle and in soil C and N content under prolonged warming and drought in a Mediterranean shrubland. Appl. Soil Ecol. 39, 223–235. doi: 10.1016/j.apsoil.2007.12.011
Schimel J., Becerra C. A., Blankinship J. (2017). Estimating decay dynamics for enzyme activities in soils from different ecosystems. Soil Biol. Biochem. 114, 5–11. doi: 10.1016/j.soilbio.2017.06.023
Schinner F., Ohlinger R., Kandeler E., Margesin R. (1996). Methods in Soil Biology (New York: Springer-Verlag).
Shen X. J., Jiang M., Lu X. G. (2023). Diverse impacts of day and night temperature on spring phenology in freshwater marshes of the Tibetan Plateau. Limnol. Oceanogr. Lett. 8, 323–329. doi: 10.1002/lol2.10285
Shen X. J., Liu Y. W., Zhang J. Q., Wang Y. J., Ma R., Liu B. H., et al. (2022b). Asymmetric impacts of diurnal warming on vegetation carbon sequestration of marshes in the Qinghai Tibet Plateau. Global Biogeochem. Cycles 36, e2022GB007396. doi: 10.1029/2022GB007396
Shen M. G., Wang S. P., Jiang N., Sun J. P., Cao R. Y., Ling X. F., et al. (2022a). Plant phenology changes and drivers on the Qinghai–Tibetan Plateau. Nat. Rev. Earth Environ. 3, 633–651. doi: 10.1038/s43017-022-00317-5
Sinsabaugh R. L., Hill B. H., Follstad Shah J. J. (2009). Ecoenzymatic stoichiometry of microbial organic nutrient acquisition in soil and sediment. Nature 462, 795–798. doi: 10.1038/nature08632
Sinsabaugh R. L., Lauber C. L., Weintraub M. N., Ahmed B., Allison S. D., Crenshaw C., et al. (2008). Stoichiometry of soil enzyme activity at global scale: Stoichiometry of soil enzyme activity. Ecol. Lett. 11, 1252–1264. doi: 10.1111/j.1461-0248.2008.01245.x
Song Y. Y., Song C. C., Li Y. C., Hou C. C., Yang G. S., Zhu X. Y. (2013). Short-term effects of nitrogen addition and vegetation removal on soil chemical and biological properties in a freshwater marsh in Sanjiang Plain, Northeast China. Catena 104, 265–271. doi: 10.1016/j.catena.2012.12.008
Song Y. Y., Song C. C., Tao B. X., Wang J. Y., Zhu X. Y., Wang X. W. (2014). Short-term responses of soil enzyme activities and carbon mineralization to added nitrogen and litter in a freshwater marsh of Northeast China. Eur. J. Soil Biol. 61, 72–79. doi: 10.1016/j.ejsobi.2014.02.001
Su Y. Z., Li Y. L., Cui J. Y., Zhao W. Z. (2004). Influences of continuous grazing and livestock exclusion on soil properties in a degraded sandy grassland, Inner Mongolia, northern China. Catena 59, 267–278. doi: 10.1016/j.catena.2004.09.001
Szajdak L. W., Gaca W. (2010). Nitrate reductase activity in soil under shelterbelt and an adjoining cultivated field. Chem. Ecol. 26, 123–134. doi: 10.1080/02757540.2010.501028
Vranova V., Rejsek K., Formanek P. (2013). Proteolytic activity in soil: A review. Appl. Soil Ecol. 70, 23–32. doi: 10.1016/j.apsoil.2013.04.003
Wallenstein M. D., Mcmahon S. K., Schimel J. P. (2009). Seasonal variation in enzyme activities and temperature sensitivities in Arctic tundra soils. Glob. Change Biol. 15, 1631–1639. doi: 10.1111/j.1365-2486.2008.01819.x
Wang J. L., Fu Z. S., Chen G. F., Zou G. Y., Song X. F., Liu F. X. (2018). Runoff nitrogen (N) losses and related metabolism enzyme activities in paddy field under different nitrogen fertilizer levels. Environ. Sci. pollut. R. 25, 27583–27593. doi: 10.1007/s11356-018-2823-3
Wang R., Guegler K., LaBrie S. T., Crawford N. M. (2000). Genomic analysis of a nutrient response in arabidopsis reveals diverse expression patterns and novel metabolic and potential regulatory genes induced by nitrate. Plant Cell 12, 1491–1509. doi: 10.1105/tpc.12.8.1491
Wang S. Y., Liu Y., Chen L., Yang H. Y., Wang G. G., Wang C. T., et al. (2021). Effects of excessive nitrogen on nitrogen uptake and transformation in the wetland soils of Liaohe estuary, northeast China. Sci. Total Environ. 791, 148228. doi: 10.1016/j.scitotenv.2021.148228
Wang Z. W., Ma S. Q., Hu Y., Chen Y. C., Jiang H. M., Duan B. L., et al. (2022). Links between chemical composition of soil organic matter and soil enzyme activity in alpine grassland ecosystems of the Tibetan Plateau. Catena 218, 106565. doi: 10.1016/j.catena.2022.106565
Wei D., Ri X., Tarchen T., Dai D. X., Wang Y. S., Wang Y. H. (2015). Revisiting the role of CH4 emissions from alpine wetlands on the Tibetan Plateau: Evidence from two in situ measurements at 4758 and 4320 m above sea level. J. Geophys. Res-Biogeo. 120, 1741–1750. doi: 10.1002/2015JG002974
Weintraub M. N., Scott-Denton L. E., Schmidt S. K., Monson R. K. (2007). The effects of tree rhizodeposition on soil exoenzyme activity, dissolved organic carbon, and nutrient availability in a subalpine forest ecosystem. Oecologia 154, 327–338. doi: 10.1007/s00442-007-0804-1
Wu J. Q., Ma W. W., Li G., Alhassan A. R. M., Wang H. Y., Chen G. P. (2020a). Vegetation degradation along water gradient leads to soil active organic carbon loss in Gahai wetland. Ecol. Eng. 145, 105666. doi: 10.1016/j.ecoleng.2019.105666
Wu J. Q., Wang H. Y., Li G., Ma W. W., Wu J. H., Gong Y., et al. (2020b). Vegetation degradation impacts soil nutrients and enzyme activities in wet meadow on the Qinghai-Tibet Plateau. Sci. Rep. 10, 21271. doi: 10.1038/s41598-020-78182-9
Wu P. F., Zhang H. Z., Cui L. W., Wickings K., Fu S. L., Wang C. T. (2017). Impacts of alpine wetland degradation on the composition, diversity and trophic structure of soil nematodes on the Qinghai-Tibetan Plateau. Sci. Rep. 7, 837. doi: 10.1038/s41598-017-00805-5
Xiang S., Guo R. Q., Wu N., Sun S. C. (2009). Current status and future prospects of Zoige Marsh in Eastern Qinghai-Tibet Plateau. Ecol. Eng. 35, 553–562. doi: 10.1016/j.ecoleng.2008.02.016
Xiao Y., Huang Z. G., Lu X. G. (2015). Changes of soil labile organic carbon fractions and their relation to soil microbial characteristics in four typical wetlands of Sanjiang Plain, Northeast China. Ecol. Eng. 82, 381–389. doi: 10.1016/j.ecoleng.2015.05.015
Xue Z. S., Lyu X. G., Chen Z. K., Zhang Z., Jiang M., Zhang K., et al. (2018). Spatial and temporal changes of wetlands on the Qinghai-Tibetan Plateau from the 1970s to 2010s. Chin. Geogr. Sci. 28, 935–945. doi: 10.1007/s11769-018-1003-1
Xue Z. S., Zhang Z. S., Lu X. G., Zou Y. C., Lu Y. L., Jiang M., et al. (2014). Predicted areas of potential distributions of alpine wetlands under different scenarios in the Qinghai-Tibetan Plateau, China. Global Planetary Change 123, 77–85. doi: 10.1016/j.gloplacha.2014.10.012
Yang G., Chen H., Wu N., Tian J. Q., Peng C. H., Zhu Q., et al. (2014). Effects of soil warming, rainfall reduction and water table level on CH4 emissions from the Zoige peatland in China. Soil Biol. Biochem. 78, 83–89. doi: 10.1016/j.soilbio.2014.07.013
Zantua M. I., Bremner J. M. (1975). Preservation of soil samples for assay of urease activity. Soil Biol. Biochem. 7, 297–299. doi: 10.1016/0038-0717(75)90070-X
Keywords: wetland ecosystems, soil enzyme activities, wet meadow, vegetation degradation, nitrogen cycle
Citation: Chang W, Ma W, Song L, Tang Y, Long Y, Xu G and Yuan J (2023) Responses of soil N-cycle enzyme activities to vegetation degradation in a wet meadow on the Qinghai-Tibet Plateau. Front. Ecol. Evol. 11:1210643. doi: 10.3389/fevo.2023.1210643
Received: 23 April 2023; Accepted: 31 July 2023;
Published: 24 August 2023.
Edited by:
Jianghua Wu, Memorial University of Newfoundland, CanadaReviewed by:
Guang Li, Gansu Agricultural University, ChinaBaoming Du, Shanghai Jiao Tong University, China
Zichun Guo, Institute of Soil Science, Chinese Academy of Sciences (CAS), China
Copyright © 2023 Chang, Ma, Song, Tang, Long, Xu and Yuan. This is an open-access article distributed under the terms of the Creative Commons Attribution License (CC BY). The use, distribution or reproduction in other forums is permitted, provided the original author(s) and the copyright owner(s) are credited and that the original publication in this journal is cited, in accordance with accepted academic practice. No use, distribution or reproduction is permitted which does not comply with these terms.
*Correspondence: Weiwei Ma, MjgxMjA1MjM2QHFxLmNvbQ==