- Misaki Marine Biological Station, The University of Tokyo, Miura, Kanagawa, Japan
In some species of termites, a part of nymphs can undergo “regressive molt,” in which imaginal characters such as wing buds and body size are reduced. The function of regressive molt is thought to be a regulatory mechanism controlling caste composition in a colony. However, little is known about the physiological and developmental processes resulting in the regressive molt. In this study, therefore, regressive molt was observed in a damp-wood termite Hodotermopsis sjostedti, under rearing conditions, and histological and morphological observations during regressive molt (especially the reduction of wing buds) were carried out. It was found that nymphs with wing buds were classified into three morphological types. Almost all nymphs with swollen wing buds (Type 1) differentiated into alates, while nymphs with pigmented wing buds (Type 2) or with flat wing buds (Type 3) underwent regressive molts to become pseudergates (workers). Through the regressive molt, a part of nymphs differentiated into presoldiers or neotenic reproductives. Histological observations showed that, in nymphs undergoing regressive molt, epithelial tissue of wing buds was degenerated. Consistently, real-time qPCR analyses revealed that, apoptosis-related factors were up-regulated in the thoracic parts of Type 3 nymphs. Furthermore, expression analyses on developmental and endocrine factors by real-time qPCR were carried out, showing that, the expression pattern of these factors in regressive molt is nearly identical to that in stationary molt. These results suggest that the differentiation fate of nymph is determined during the intermolt period before the differentiation into Type 1 or Type 2/3 nymphs. The regressive molt itself would be almost identical to stationary molt.
Introduction
In colonies of eusocial animals, divisions of labors among morphologically and behaviorally distinct individuals (castes) are essential for social functions, so that, in evolutionary point of view, the acquisition of castes is considered an important step in the evolution of eusociality (Noirot, 1969; Weesner, 1969). In particular, the reproductive division of labor, i.e., the differentiation between fertile and sterile castes, is one of the most important characteristics of eusociality (Wilson, 1971; Lin and Michener, 1972). Among the various castes, non-reproductive workers, which consist of the majority of colony members, are engaged in a variety of colony labors such as foraging and nursing. This working caste is one of the most universal castes found in almost all eusocial animals (Wilson, 1971; Anderson, 1984). In many species of eusocial insects (e.g., honeybees, ants and termites), since workers have reproductive organs but do not reproduce on their own, the regulatory mechanisms of worker number and proportion would be an important issue in sociobiology.
In termites (superfamily Termitoidea, order Blattodea), one of the representative examples of eusocial insects, in general, a colony contains reproductives, soldiers and workers (Noirot, 1969). Reproductives can be categorized into primary and secondary reproductives, based on their developmental pathways. Primary reproductives derive from alates (winged imagos), that found new colonies after nuptial flights (Nutting, 1969), with adult characteristics like well-developed compound eyes and functional wings. In contrast, secondary reproductives, also known as replacement or supplementary reproductives, directly differentiate from workers by developing gonads, when primary reproductives die or become senescent (Thorne, 1996; Korb and Hartfelder, 2008). Soldiers defend their colonies against enemies, by using some weapon characters such as mandibles for biting or frontal projections from which defensive substances are discharged (Deligne et al., 1981). Workers, or pseudergates (false workers with totipotency to become any castes) seen in some basal termite lineages (Archotermopsidae, Stolotermitidae, Kalotermitidae, Serritermitidae, and some species of Rhinotermitidae), perform a variety of colony tasks, including foraging, nest maintenance, and grooming (Rosengaus and Traniello, 1993).
Caste differentiation is known as an example of phenotypic plasticity, or polyphenism, in which multiple phenotypes develop based even on a single genotype (Nijhout, 2003). In the case of caste polyphenism in social insects, primary extrinsic factors are social interactions among colony members (Miura, 2005). Termites are hemimetabolous insects, so that they undergo repeated molts during postembryonic development (Howard and Thorne, 2010). Especially termites exhibit various patterns of molts which can be classified to multiple types, i.e., a growth molt during juvenile stages (progressive molt), molt without growth (stationary or regressive molt), or molts into distinctive castes (soldiers and reproductives; Korb and Hartfelder, 2008). Workers in derived termite species, especially belonging to the family Termitidae, also known as “higher termites,” are differentiated at relatively early stages of postembryonic development. Therefore, they lose the potential to differentiate into alates, so that they are called “true workers” (Noirot and Pasteels, 1987; Korb and Hartfelder, 2008). On the other hand, in relatively basal termite species, all of the colony members follow the same postembryonic pathways, becoming “pseudergates” (Noirot, 1985; Korb and Hartfelder, 2008; Legendre et al., 2013). Pseudergetes, which means “false workers,” still have the totipotency to differentiate into any castes, including reproductives (Grassé and Noirot, 1947; Korb and Hartfelder, 2008). The focal termite species in this study, i.e., Hodotermopsis sjostedti (Archotermopsidae), possess pseudergates (Figure 1), but they are hereafter called as “workers” in this paper for simplicity.
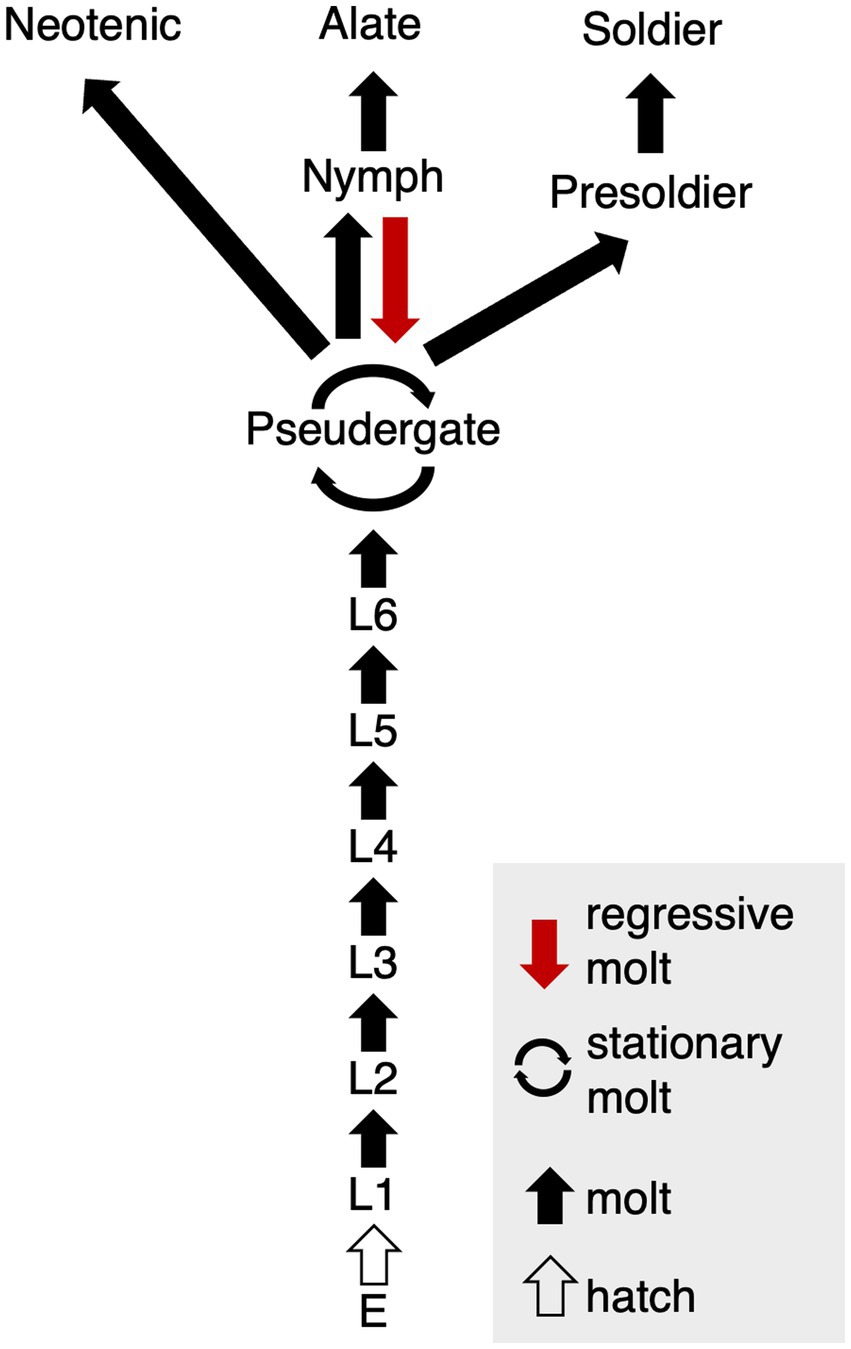
Figure 1. Caste differentiation pathway in H. sjostedti. Abbreviations are: E, egg; L, larva, i.e., immatures without wing buds. Each arrow indicates, respectively, multiple molt types (red arrow, regressive molt; cyclic arrow, stationary molt; black arrow, normal molt; white arrow, hatch) (modified from Miura et al., 2004).
The “stationary molt” from worker to worker without growth and the “regressive molt” from nymph to workers are unique to termites (Grassé and Noirot, 1947; Roisin, 2000; Korb and Hartfelder, 2008, Figure 1). Normally, in the course of differentiation into alates, workers firstly differentiate into nymphs with wing bud, or wing pads. However, some nymphal individuals cannot accomplish the alate differentiation, but instead they revert back to workers, and thus called “regressive” molt. In this molt, imaginal traits such as wing buds that have developed are either regressed or lost (Noirot and Pasteels, 1987; Roisin, 1990, 1994; Korb and Hartfelder, 2008). Regressive molt has been observed in Rhinotermitidae, Kalotermitidae, and Archotermopsidae (Termopsidae) and is known to be induced by interactions among colony members, such as biting of wing buds (Desneux, 1906; Miller, 1942; Noirot and Pasteels, 1987; Myles, 1988; Lenz et al., 1991; Roisin, 1994).
Regressive molt can be a regulatory mechanism that maintain the number of workers in a colony and thus may play an important role in maintaining sociality. Although some recent studies show that juvenile hormones are involved in regressive molt (Korb et al., 2009, 2021), histological and physiological transitions that occur during regressive molt still remain unclear. Also, the difference between regressive and stationary molts has not yet been elucidated. Therefore, in this study, to uncover the underlying mechanism, regressive molt was extensively investigated in a Japanese damp-wood termite, Hodotermopsis sjostedti, with rearing experiments and histological observations. In addition, expression analyses by real-time qPCR on apoptotic factors, hormone-related genes and morphogenetic factors were carried out during regressive molt. Drastic morphological changes occur after the release of gut contents, called “gut-purge,” in all the molt patterns, including caste differentiation and regressive molt (e.g., Cornette et al., 2007; Masuoka et al., 2018; Oguchi et al., 2022). Therefore, histological observations and gene expression analyses were carried out at both before and after gut-purge.
Materials and methods
Termites
Colonies of Hodotermopsis sjostedti Holmgren (family Archotermopsidae) were collected on Yakushima Island, Kagoshima prefecture, Japan, in May 2021, and May 2022 (Supplementary Table S1). Collected colonies were kept at approximately 25°C under constant darkness, as described previously (Sugime et al., 2019). These colonies are hereafter called “stock colonies.” For their food, moistened pine lumber was occasionally supplied. All the collected colonies contained nymphs, and after several weeks of rearing in the laboratory, alate emerged in some colonies. According to the previous research (Miura et al., 2000), age, sex and castes were identified within 1 month after the colony sampling. In this species, nymphs are known to have only single instar (Miura et al., 2004; Nii et al., 2019) and these nymphs were used for the following examinations. In addition, among the collected nymphs, three distinct types were recognized based on the thoracic morphologies including wing buds (see Results for details).
Rearing experiments
To determine the developmental fates of all the nymphal types, rearing experiments were conducted. All rearing experiments were conducted within 1 month after colony sampling. Total of 20 nymphs (10 males and 10 females) were kept in a petri dish under constant darkness at 25°C. Moistened wood shavings were fed as food and rehydrated by misting as needed. The rearing experiments were carried out to compare among 3 different types, i.e., Type 1–3. The number of replicates (number of petri dishes) for each type was Type 1 = 8 (160 individuals), Type 2 = 11 (220 individuals), and Type 3 = 3 (60 individuals). Individuals of Type 1 and Type 2 nymph derived from colony no. 21 (Supplementary Table S1). Type 3 nymphs derived from colonies No. 1 and No. 2 to make the 7 and 4 experimental groups, respectively, (Supplementary Table S1). Caste composition and morphological features (such as color change and the presence or absence of wounds in wing buds) was observed every 7 days after isolation during 30 days. Dead individuals were removed from the petri dishes. During the rearing experiment, nymphs were not added even if individuals in a petri dish died.
Morphological observations on regressively-molted individuals
To elucidate detailed morphological changes during regressive molt, morphologies of the individuals molted from nymphs (including regressively-molted individuals) were compared, with those of workers, presoldiers, neotenic reproductives and alates found in the stock colonies, by using a binocular microscope and a scanning electron microscope (SEM) as previously described (Miura et al., 2004). Stock-colony-derived workers, presoldiers, neotenics and alates are hereafter called just “workers,” “presoldiers,” “neotenics,” and “alates” respectively. In alates, wings were excised to make it easier to visualize the thoracic morphology. Individuals were decapitated and fixed overnight by FAA fixative (formalin: acetic acid: ethanol = 6:1:16), and preserved in 70% ethanol. The samples were then transferred into increasing concentrations of ethanol, followed by t-butanol. After that, the samples were freeze-dried using a Freeze Dryer (ES-2030, Hitachi Global, Tokyo, Japan). Samples were coated with gold using an ion coater (Eiko IB-3; Eiko Engineering, Ibaraki, Japan) and were observed by a scanning electron microscope (JSM-5510LV, JEOL Ltd., Tokyo, Japan).
Histological observations
As previously described (Cornette et al., 2007), paraffin sections were made to observe histological transition during regressive molt, particularly focusing on reproductive organs and wing buds. According to a previous research (Oguchi et al., 2016), the reproductive organs were compared regressively-molted individuals with nymphs, alates, neotenics, and workers. The wing buds were observed before and after gut purging in each nymphal type. Samples were dehydrated in increasing concentrations of ethanol, then transferred into xylene and finally embedded into paraffin. Sagittal serial sections (8 μm thick) were processed and stained with hematoxylin and eosin as previously described (Cornette et al., 2007).
Gene identifications
To investigate gene expression patterns during the regressive molt, orthologs of developmental genes were identified based on the transcriptome data of H. sjostedti (DDBJ Sequence Read Archive: DRA005483; Sugime et al., 2019). In this study, apoptosis-related genes, wing-patterning genes and hormone-related genes were focused.
As for the apoptosis-related genes, 3 genes (apaf-1 related killer [Ark], Drosophila ICE [Drice], death caspase-1 [Dcp-1]) were identified. The protein sequences of those candidate genes in Drosophila melanogaster were used as queries for tBLASTn searches against the datasets of the H. sjostedti. Based on the obtained sequences, primers for real-time qPCR were designed using Primer3Plus. As for wing-patterning genes (Engrailed [En], Ultrabithorax [Ubx], Sex combs reduced [Scr], Apterous [Ap], Vestigial [Vg], Wingless [Wg], Decapentaplegic [Dpp] and Extradenticle [Exd]) and hormone-related genes (Methoprene-tolerant [Met], Kruppel homolog 1 [Kr-h1], Broad-Complex [Brc], Ecdysone receptor [EcR], Ultraspiracle [USP], ecdysone-induced transcription factor [E93], Insulin receptor 1-3 [InR1-3], Protein kinase B [PKB] and Forkhead box O [Foxo]), the primers for real-time qPCR were designed as described in previous studies (Nii et al., 2019; Sugime et al., 2019). Primers used in this study are listed in Supplementary Table S2.
RNA extraction and gene expression analyses by qPCR
Total RNA was extracted from the thoracic part of a male individual including prothorax, mesothorax, and metathorax, excluding guts and legs, using ISOGEN (Nippon Gene, Tokyo, Japan). All individuals of pseudergate, Type 1 and 2 nymphs were collected from colony no. 21 (Supplementary Table S1). Additionally, Type 3 nymphs were collected from colony no. 17 (Supplementary Table S1). The examined time points were before gut purge and gut-purge in the imaginal (Type 1 nymph), regressive (Type 2 and 3 nymph) and stationary molt lines. After extraction, samples were DNase treated and purified with TURBO DNA-free Kit (Thermo Fisher Scientific, Waltham, MA, USA) and RNAClean XP beads (Beckman Coulter Genomics, Danvers, MA, USA). For each sample, total RNA was reverse-transcribed with a High Capacity cDNA Reverse Transcription Kit (Applied Biosystems, Foster City, CA, USA). Quantifications of the relative amounts of target transcripts were then performed using a fast SYBR Green Master Mix and the sequence detection system ABI PRISM 7500 (Applied Biosystems, Foster City, CA, USA). To identify an appropriate endogenous control gene, the suitability of different candidate reference genes (18S rRNA [18S], glyceraldehyde-3-phosphate dehydrogenase [GAPDH], elongation factor 1 alpha [EF1α], and ribosomal protein 49 [RP49]) was evaluated using the software geNorm (Vandesompele et al., 2002) and Normfinder (Andersen et al., 2004). The stability value reflects the variation in expression, and the smaller measure is evaluated as a stable reference gene. In the geNorm, two candidate genes (EF1α and RP49) with high stability are finally selected (Supplementary Table S3). Normfinder selected the single gene with the lowest stability value, and in this case, RP49 was selected as the best candidate (Supplementary Table S3). Therefore, RP49 was found to be the most appropriate reference gene (Supplementary Table S3). Data acquisitions and analyses were handled by ABI Prism 7500 software ver. 2.0.4 (Applied Biosystems, Foster City, CA, USA), using the relative standard curve method. The number of samples was n = 4 for each sample category.
Statistical analyses
First, the Shapilo-Wilk test and Bartlett test were performed to verify the sample distribution and variance of the measurement results, respectively. The results revealed that some groups in the expression analyses of apoptosis-related genes were not normally distributed (Shapilo-Wilk test, p < 0.05), and significant variances among groups were detected (Bartlett test, p < 0.05). For these data, Welch’s t-test with Bonferroni correction was used. On the other hand, since gene expression analyses of endocrine-related genes and wing formation-related genes were assumed to be normally distributed and equivariant, Tukey’s test (p < 0.05) was performed after one-way ANOVA (p < 0.05). All statistical analyses were performed using R version 4.0.3 (R Core Team, 2020). To evaluate the similarities and differences among molt patterns (regressive, stationary, alate molts, etc.), a principal component analysis (PCA) was performed with the gene expression data.
Results
Caste differentiation fates according to nymphal types
Since nymphal individuals were morphologically categorized into 3 types, i.e., Type 1–3 (Figures 2A–F). Wing buds of Type 1 nymph were swollen, in which wing tissue was largely developed (Figures 2A,D). The Type 2 nymphs had darkened wing buds and the edges of the wing buds were collapsed in some of the nymphs (Figures 2B,E). The wing buds of the Type 3 nymph were similar to those of Type 1, but, they were flattened, not as swollen as those of Type 1 (Figures 2C,F). In stock colonies, alate appeared in only 3 of 21 colonies. Of the three colonies, Type 1 nymphs were found in two colonies and only alates were found in the other one (Supplementary Table S1). Some colonies contained nymphs but alates do not emerge from them. All these nymphs were Type 2 and 3 nymphs (Supplementary Table S1).
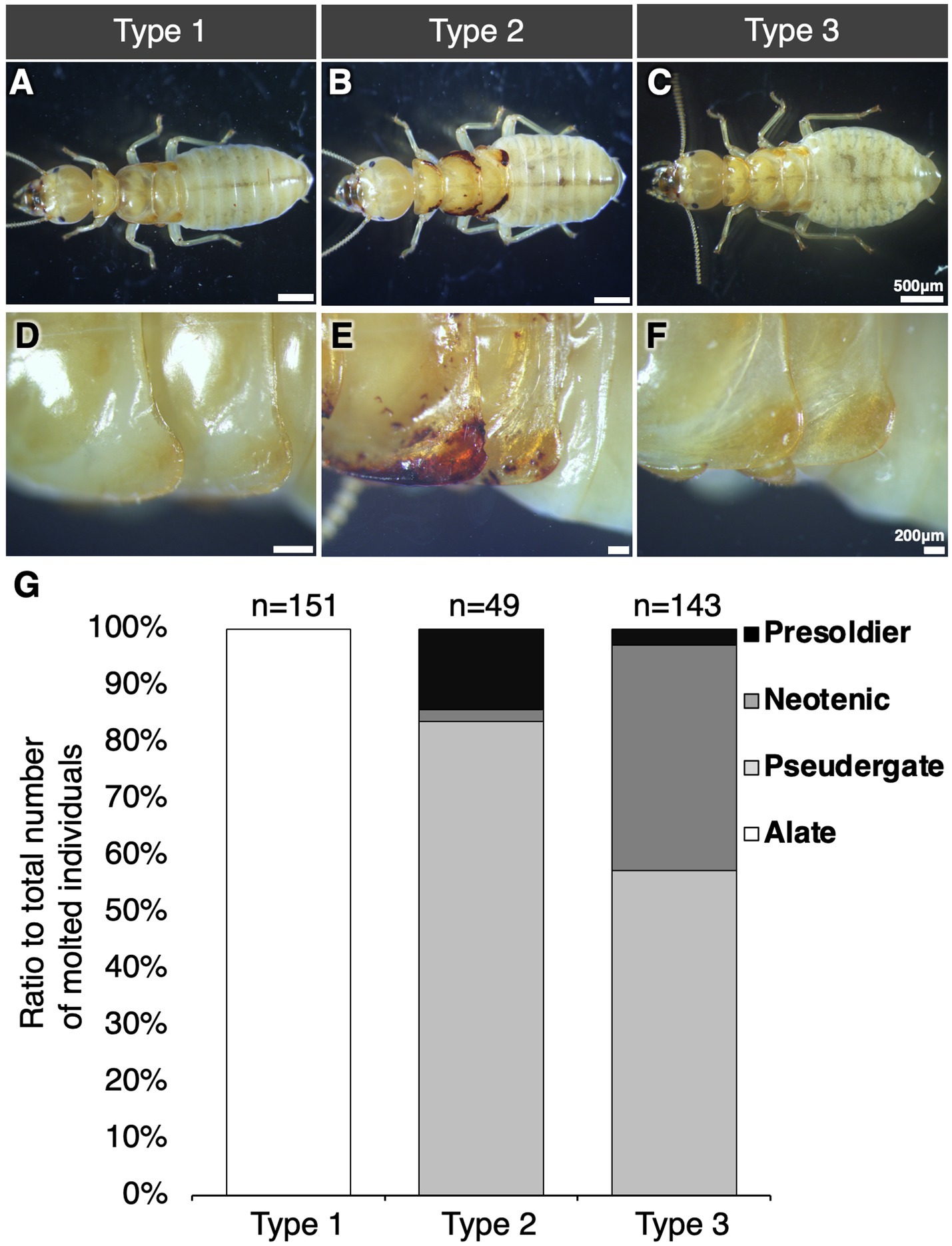
Figure 2. Three nymphal types (A–F) and caste differentiation fates (G). Whole body (A–C) and wing bud morphology (D–F) of three types of nymphs are shown. Bars indicate 500 μm (A–C) and 200 μm (D–F), respectively. (G) Caste-differentiation fates of nymphal types during 30 days of the rearing experiments. The vertical axis represents the differentiated caste ratio to total number of molted individuals. Half of the individuals of all types molted after 30 days of rearing experiment (Supplementary Figure S1).
The differentiation fates in each type of nymphs were examined by rearing experiments (Figure 2G). In all types, at least half of the reared individuals molted during 30 days after the onset of rearing (Supplementary Figure S1). As the result of the rearing experiment, each type showed a different caste fate. All Type 1 nymphs were differentiated into alate. In contrast, in Types 2 and 3, alates did not appear and all molted individuals underwent regressive molt and differentiated into worker, neotenic, or presoldier. Reproduction by regressively-molted neotenic was not observed during the experimental period. After 30 days of rearing, most individuals molted, but some remained nymphs or died (Supplementary Figure S1). The wing buds of Type 3 nymphs did not develop as in Type 1 nymphs and did not turn black as in Type 2 nymphs.
Morphological features in regressively-molted castes
Morphological changes during nymphal development including regressive molt were observed in detail (Figure 3; Supplementary Figures S2, S3). Morphological examinations revealed that regressive molted castes possess the same morphological characteristics as castes that directly molted from workers.
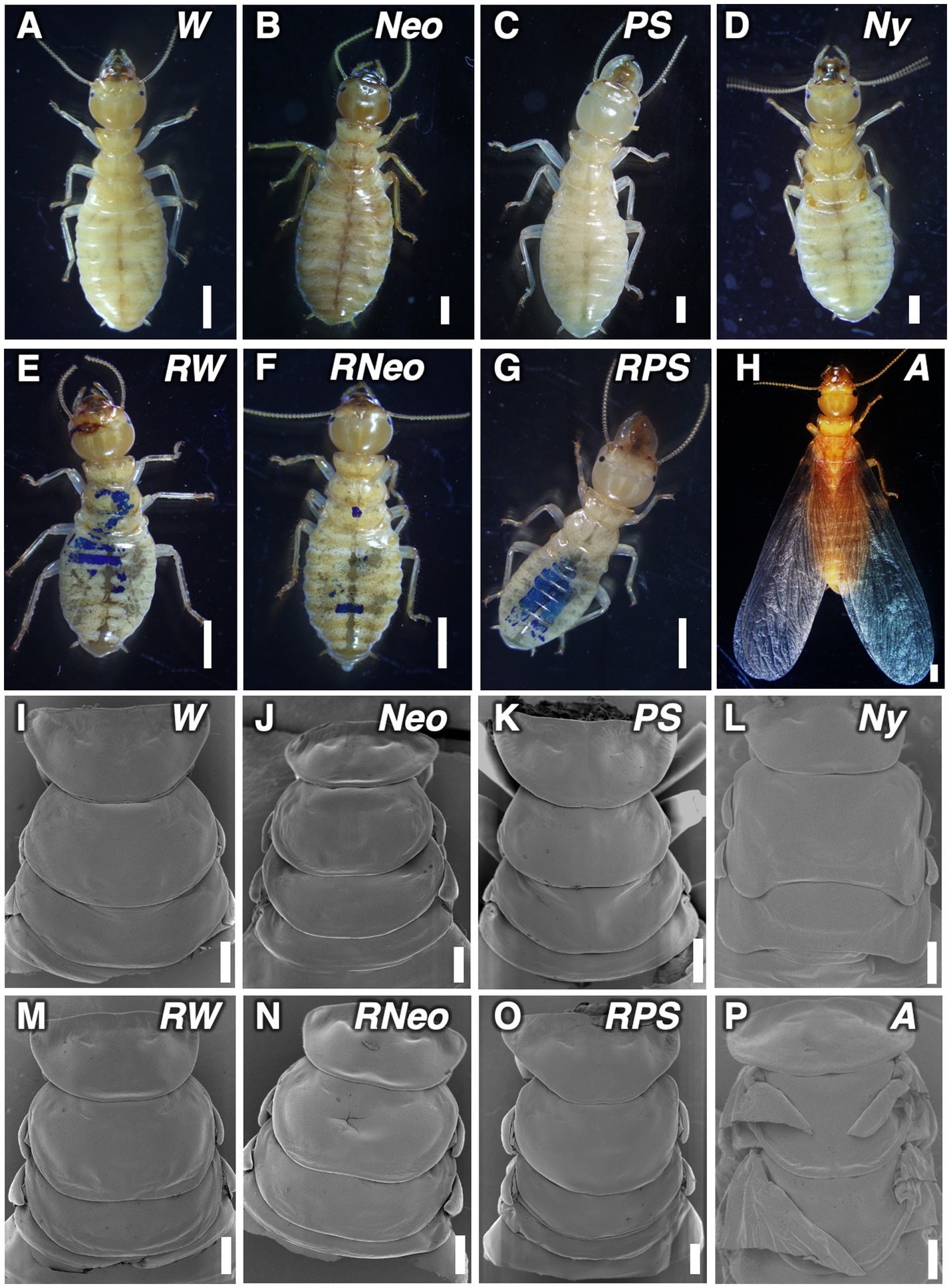
Figure 3. External morphologies of individuals in stock colonies and regressively molted individuals, observed by binocular microscope and SEM. (A,I) worker (W); (B,J) neotenic (Neo); (C,K) presoldier (PS); (D,L) nymph (Ny); (E,M) regressively molted worker (RW); (F,N) regressively molted neotenic (RNeo); (G,O) regressively molted presoldier (RPS); (H,P) P: alate (A). Bars indicate 200 μm (A–H) and 500 μm (I–P), respectively.
Abdominal structure of regressively-molted female workers was similar to that of female workers (Supplementary Figures S2G,J). The abdominal structure of the regressively-molted female neotenic showed the loss of stylus, which is a representative characteristic of reproductive caste (Supplementary Figures S2H,K). Furthermore, these regressively-molted neotenics had relatively well developed reproductive organs compared to workers and nymphs, (Supplementary Figure S3). Especially, vitellogenic oocytes were seen in regressively-molted female neotenics (Supplementary Figure S3F). Moreover, regressively-molted male neotenics possessed developed cysts (Supplementary Figure S3K). The regressively-molted presoldiers had larger mandibles that were comparable to those of normal presoldiers (Figures 3C,G; Supplementary Figures S2C,F).
Regardless of the castes after the regressive molt i.e., workers, presoldiers, or neotenics, all the regressively-molted individuals that lost the wing buds that had developed during the preceding nymphal stage (Figures 3E–G,M). In contrast, alates possessed well-developed functional wings (Figures 3H,P).
Wing-bud degeneration before regressive molt
Histological observations of thorax in nymphs showed that histological changes inside wing buds were different among the different nymphal types (Figure 4). The inner structure of wing buds in Type 1 nymphs showed marked wing development (Figure 4A). Before the gut-purged period, epithelial tissues of the wings were strongly stained with hematoxylin (Figure 4A). In the gut-purged period, the wings were observed to be swollen and folded inward (Figure 4B). In all the Type 2 and Type 3 nymphs, wing development was not observed until the molt (Figures 4C–F). A sclerotized area was observed toward the wing-bud tips in the Type 2 nymphs, and the tissue was not stained by HE (Figures 4C,D). Especially after gut-purged periods, a new cuticle was observed between the sclerotized area and the wing-bud tissue (Figure 4D). In Type 3 nymphs, epithelial tissues were observed all around the wing-bud tips, but there was no strong hematoxylin coloration as in Type 1 nymphs (Figure 4E). During the gut-purged period in Type 3 nymphs, the inner epidermis of wing buds was degenerating (Figure 4F).
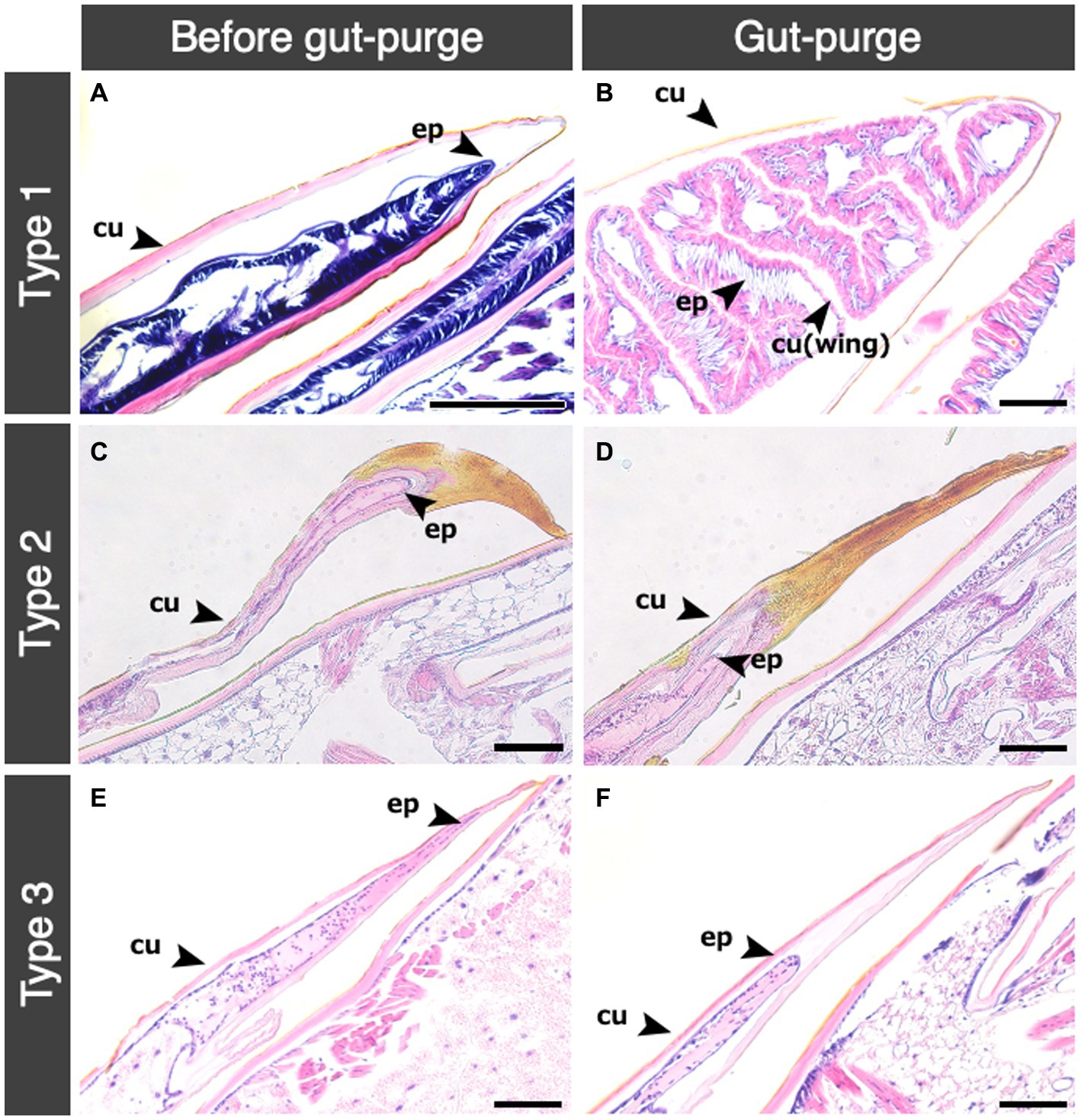
Figure 4. Histological transition of wing bud in the three types of nymphs. Histological transitions in the wing bud of mesothorax among three nymphal types are shown. Abbreviations are: cu, cuticle; ep, epidermis. (A) Type 1 before gut purge period; (B) Type 1 in gut-purge period; (C) Type 2 before gut purge period; (D) Type 2 in gut-purge period; (E) Type 3 before gut purge period; (F) Type 3 in gut purge period. Bars indicate 100 μm.
Since the regression of wing-bud tissue was observed in Type-2 and 3 nymphs (Figures 4C–F), expression analyses of apoptosis-related genes were conducted to verify the involvement of apoptotic cell death (Figure 5). In the before-gut-purge period, almost all genes were highly expressed in Type 3 nymphs. In contrast, at the time of gut purge, Ark was upregulated in Type 2, but not as highly expressed as in the before-gut-purge period. Thus, it was suggested that active apoptosis in the wing buds occurred in Type 2 and 3 nymphs.
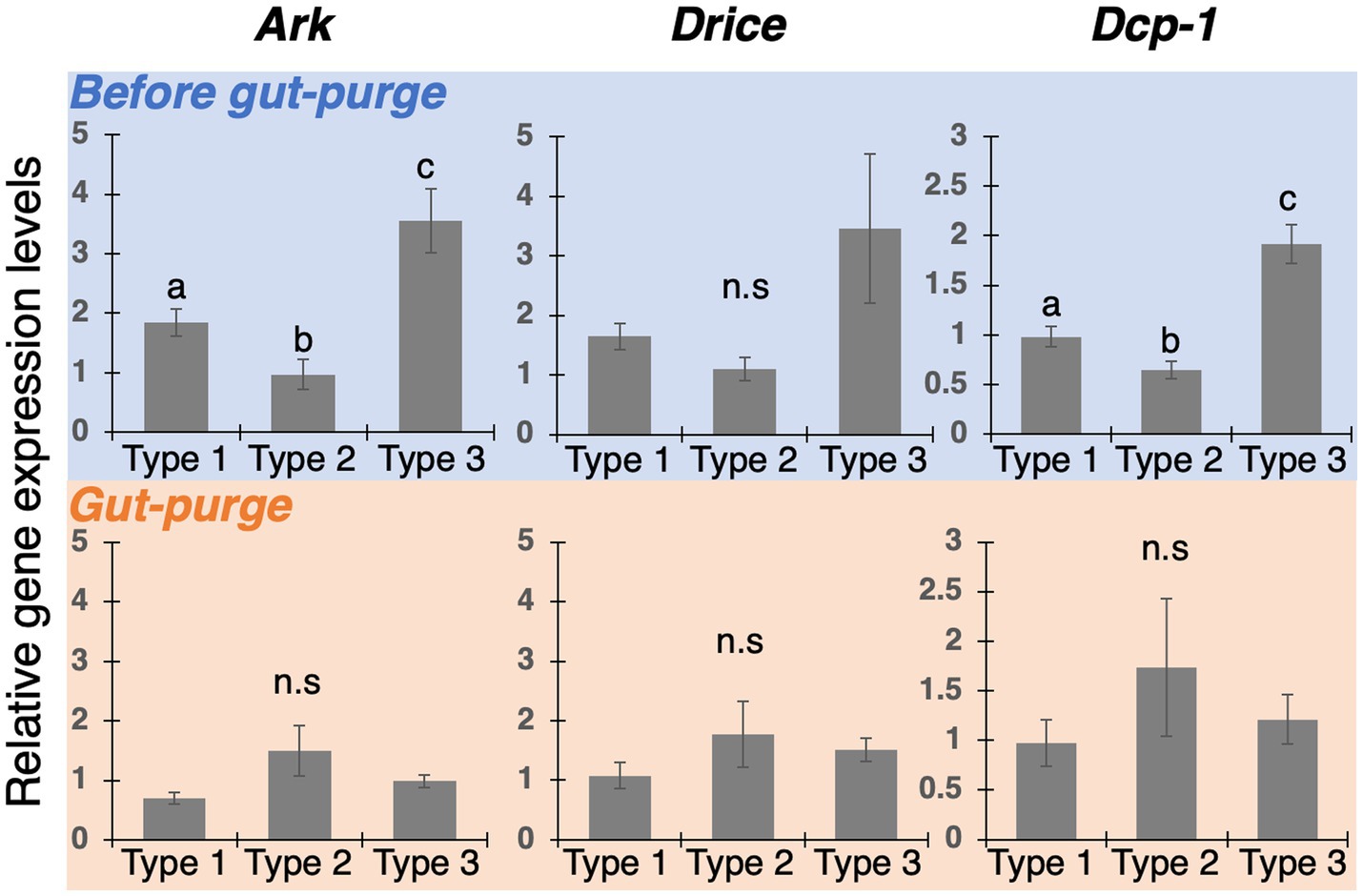
Figure 5. Expression patterns of apoptosis-related genes (Ark, Drice and Dcp-1) in the thoracic parts of each nymphal type. Relative expression levels (data are mean ± s.d., all samples were normalized by before-gut-purge worker) were quantified by realtime qPCR. Different letters on bars indicate significant differences (Welch’s t-test with Bonferroni correction, p < 0.05). The number of samples was n = 4.
Expression of hormone-related and wing-patterning genes
Since it was predicted that the physiological and developmental mechanisms underlying regressive molt are similar to those of stationary molt, gene expressions during molt in each nymphal type and stationary molt were compared using real-time qPCR to examine the hypothesis (Supplementary Figure S4). Based on the obtained data, the gene-expression comparisons were carried out by principal component analysis (PCA; Figure 6A). Only Type 1 nymphs before alate differentiation formed a specific cluster. On the other hand, the distribution of Type 2 and 3 nymphs before regressive molt and worker before stationary molt tended to overlap. On the PC2 axis, these clusters were distributed separately, and Kr-h1, E93, Vg, and Foxo were highly responsible factors for the PC2.
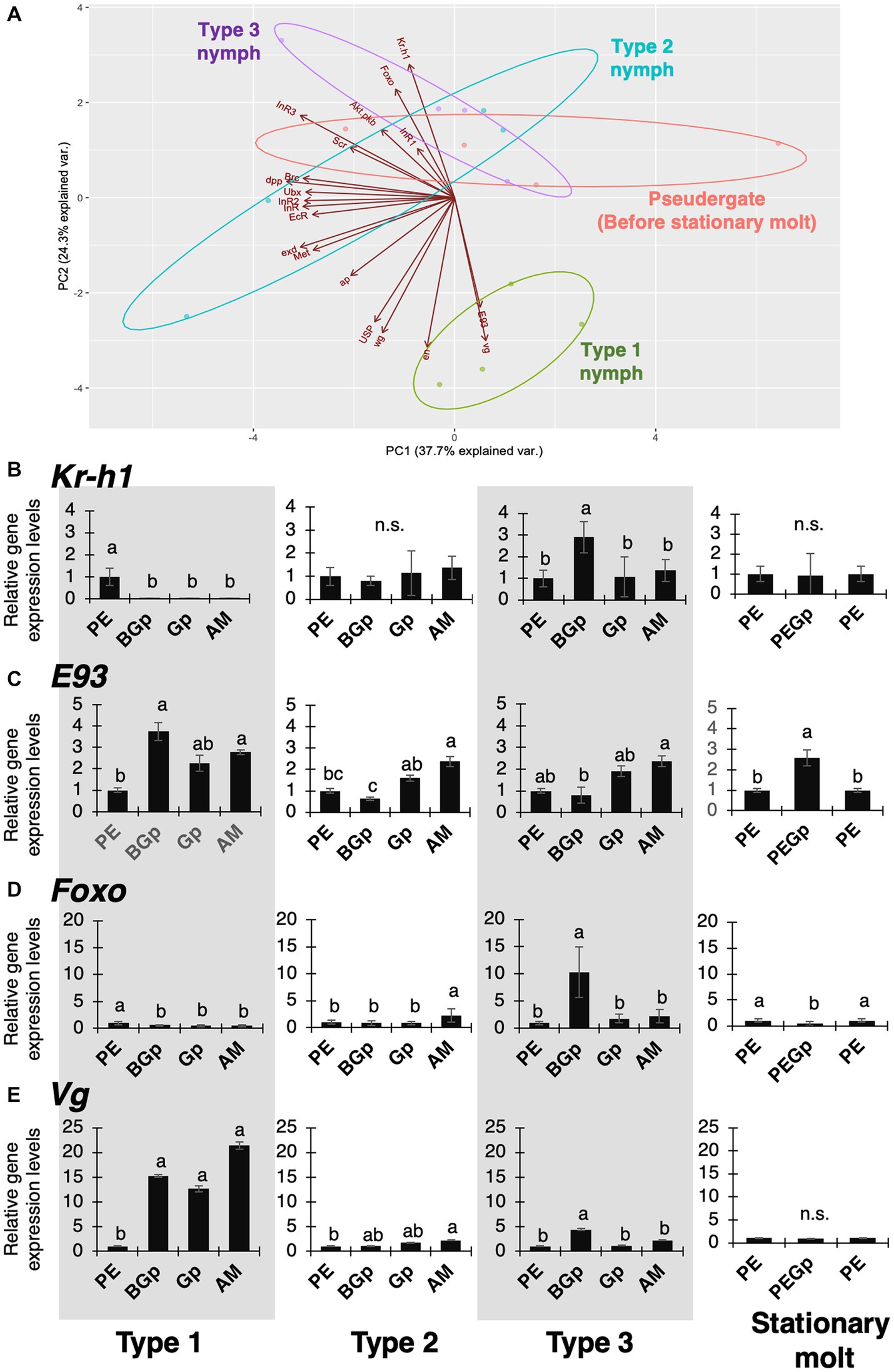
Figure 6. Principal components analysis and expression patterns of hormone-related genes and wing-patterning genes. (A) Biplot of the principal component scores among alate differentiation, regressive molt and stationary molt based on the gene expression data during gut-purge period. Plots from the principal component analysis are explained by the first and second principal component axes. (B–E) Relative expression levels (data are mean ± s.d., all samples were normalized by before-gut-purge worker) of hormone-related and wing-patterning genes in the thoracic part. PE: worker in before-gut-purge period (as control); BGp: before gut-purge; Gp: gut-purge; AM: after molt. Different letters on bars indicate significant differences (Tukey’s test, p < 0.05). The number of samples was n = 4.
Furthermore, detailed expression dynamics of each gene during the intermolt period was investigated. The expression of Kr-h1, an early response transcription factor in the juvenile hormone pathway, was consistently low during the intermolt period in Type 1 nymph, but was consistently high in Type 2 and 3 nymphs and stationary molt (Figure 6B). E93, the ecdysone-related master regulator of was consistently expressed at high level in Type 1 nymphs, but relatively low in Type 2 and 3 nymphs, especially before gut-purge period (Figure 6C). Interestingly, Foxo, a downstream factor in the insulin pathway, was upregulated only before gut purge in Type 3 nymphs (Figure 6D). Vg, the master control gene for wing formation, was constantly highly expressed in Type 1 nymphs, in which active wing formation occurs, but was markedly lower in Type 2 and 3 nymphs, where wing formation does not occur, as in stationary molts (Figure 6E).
Discussion
Differentiation fates and developmental totipotency in nymphs
Based on the morphological characteristics of their wing buds, nymphs in H. sjostedti can be categorized into three distinct types (Figures 2A–F). The three types seemed to be at the same instar, since there is only a single nymphal instar in the focal species. However, the three types seemed to have experienced different physiological changes during the single nymphal instar stage.
It was also shown that, by rearing experiments, each nymphal type underwent a different caste-differentiation fate (Figure 2G). Type 1 nymphs were morphologically conspicuous, since their bodies became fatty and whitish and their wing buds became largely swollen, indicating that they would soon molt to alates. Actually, all individuals of Type 1 differentiated into alates (Figure 2G) and other studies also confirmed that individuals with swollen Type 1 wing buds become alates (Miura et al., 2004; Nii et al., 2019; Oguchi et al., 2022). In contrast, the other types of nymphs (Type 2 and 3) differentiated into workers, neotenics, and presoldiers, indicating that they underwent regressive molt with reducing their wing buds (Figures 3, 4), as observed in a previous study (Koshikawa et al., 2001). These two types were categorized based on their wing-bud morphology; Type 2 nymphs have pigmented sclerotized wing buds, while Type 3 nymph have relatively flattened whitish wing buds. Regressively-molted workers, neotenics, and presoldiers from Type 2 and 3 nymphs were found to have similar histological and morphological characteristics to those found in the stock colonies. For example, regressively-molted presoldiers had enlarged mandibles, while regressively-molted neotenic reproductives possessed well-developed reproductive organs (Figure 3; Supplementary Figures S2, S3).
As indicated by these results, during the intermolt stage of nymph before differentiating into 3 nymphal types, their developmental fates would be determined by some extrinsic and/or intrinsic factors. In some species of lower termites, such as Kalotermes flavicollis, differentiation from nymphs into various castes has been reported (Grassé and Noirot, 1947; Lüscher, 1974). Also, similar developmental flexibility of nymphs in H. sjostedti has also been suggested by some previous studies (Miura et al., 2000; Koshikawa et al., 2001), although the detailed developmental and physiological processes during the regressive molt have not directly been observed. Additionally, in the principal component analysis based on gene expression analyses, Type 2 and 3 nymphs showed markedly wider variations in comparison with Type 1 nymphs (Figure 6A). These variations may reflect heterogeneous physiological states of Type 2 and 3 nymphs, leading to the differentiation into various castes in the rearing experiments (Figures 2A, 6A). The results in this study showed that nymphs can differentiate into various castes through regressive molt (Figure 7A). This suggests that nymphs still have developmental totipotency, that may contribute to the regulatory mechanism of caste ratios in termite colonies.
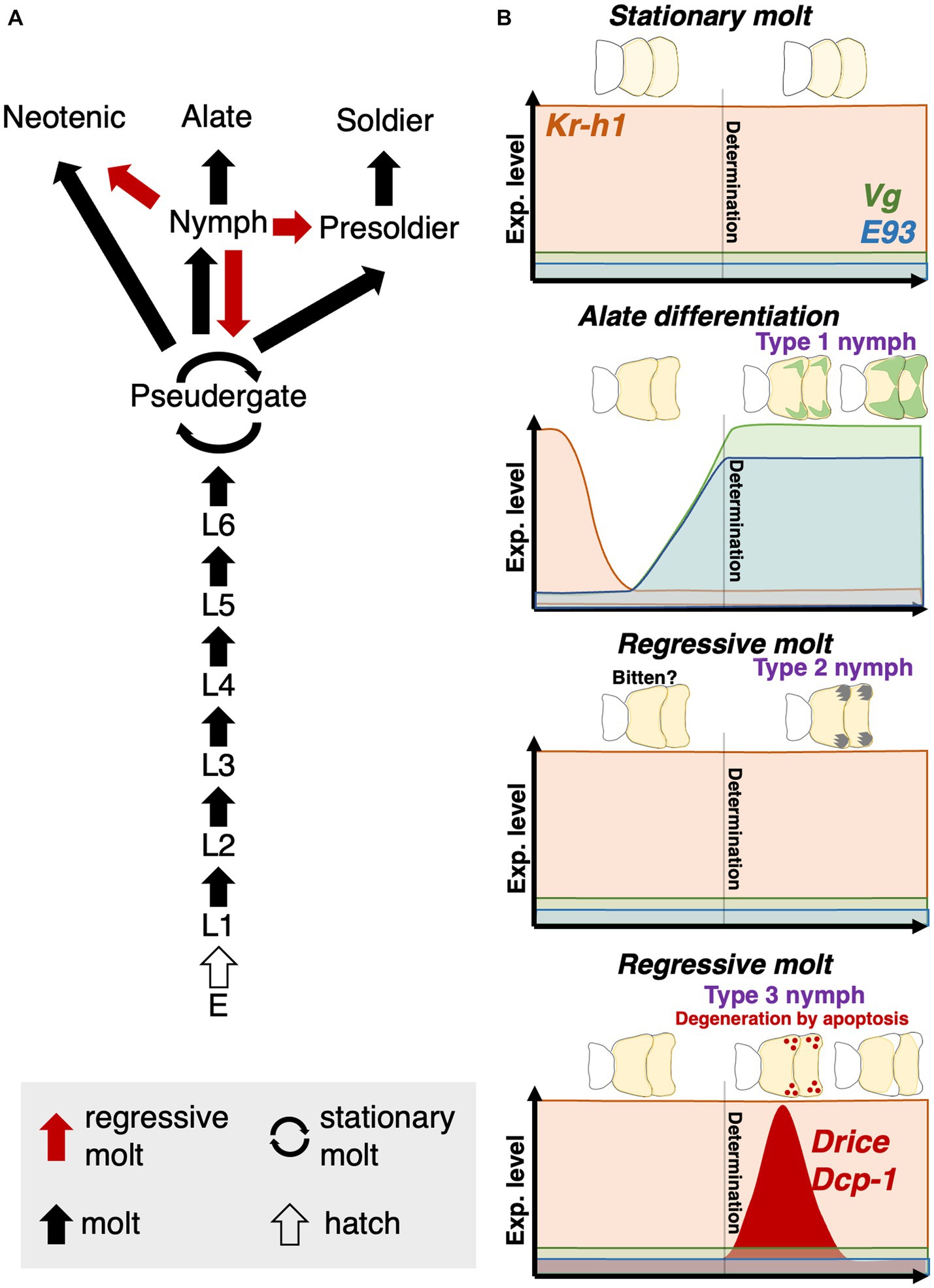
Figure 7. (A) Updated version of the caste differentiation pathway of H. sjostedti. Based on this study, not only pseudergates but also nymphs have developmental totipotency to be able to differentiate into any castes. (B) Schematic diagrams of physiological and developmental factors, controlling stationary mot, alate differentiation and two types of regressive molt. The schematic thoraces show histological changes inside wing buds during nymphal stages before alate differentiation or regressive molt. The schematic graphs show the expression patterns of some factors, which were shown to contribute to the nymphal differentiation into alate or the regressive molt. “Determination” represents a hypothetical point of the caste-fate determination. The nymphal stage in H. sjostedti consists only of a single instar, in which the “determination” of alate differentiation or regressive molt should occur.
Histological observations focusing on wing buds showed that actively-proliferating epithelial tissues, stained well with hematoxylin, were observed inside the wing buds of Type 1 nymphs that would differentiate into alates (Figures 4A,B; Nii et al., 2019), although no such tissues were observed in Type 2 and 3 nymphs that undergo regressive molt (Figures 4C–F). These findings suggest that the differentiation fates of Type 2 and 3 nymphs have already been determined, losing the potential to differentiate into alates.
Generally, in termites, the intermolt period preceding to any caste differentiation can be temporally subdivided into two phases, i.e., the early period during which environmental factors affect the developmental fates and the later period during which morphogenesis happens (Nijhout and Wheeler, 1982). Therefore, in the focal species, in the sensitive period in the nymphal intermolt, the developmental fates to differentiate into alates or to undergo regressive molt would be determined.
Degeneration of wing epithelial tissues before regressive molt
In this study, it was shown that all individuals molted from Type 2 and 3 nymphs lost their wing buds through regressive molt (Figures 3E–G,M,O). Histological observations focusing on the internal-tissue transition during the intermolt period inside the wing buds showed that epithelial tissues of wing buds became degenerated before the regressive molt, before gut purge was observed (Figure 4F). In many cases in social insects, it has been reported that apoptotic degeneration of unnecessary tissues is involved in the degeneration of wing buds and related tissues like flight muscles (Sameshima et al., 2004; Toga et al., 2011).
Also, in this study, gene expression analyses by real-time qPCR revealed that apoptosis-related factors (Ark, Drice, and Dcp-1) were highly expressed in Type 3 individuals before gut purge (intermolt stage of nymphs), in which the wing buds were not pigmented and sclerotized (Figure 5). In Drosophila, Ark is a component of the apoptosome complex and activates the downstream factors (Yu et al., 2006), while Drice and Dcp-1 have functions to degrade proteins, leading to apoptotic degenerations (Xu et al., 2006; Kumar, 2007). Thus, at least in Type 3 nymphs, through the actions of Ark, Drice, and Dcp-1, it is suggested that the apoptotic cell death is involved in the degeneration of epithelial tissues inside wing buds.
In contrast, up-regulations of apoptosis-related factors were not detected in Type 2 nymphs whose wing buds were pigmented and sclerotized (Figure 5, Type 2). In this type, the apical region inside a wing bud is occupied by pigmented cuticle (Figure 4). Between the sclerotized cuticular layer and the epithelial tissue, newly-produced cuticular layers were seen (Figure 4, Type 2). It is suggested that the apoptotic degeneration of wing epithelia was already completed before becoming pigmented.
Some social insects, including termites, are known to regulate caste differentiation by damaging body parts among colony members, such as amputation of thoracic appendage or antenna cropping (e.g., Peeters and Higashi, 1989; Miyaguni et al., 2013). In particular, in the alate differentiation of termites, it was proposed that gnawing on each other’s wing buds due to reproductive conflicts among nymphs leads to regressive molt (Zimmerman, 1983; Myles, 1986, 1988; Roisin, 1994). Biting of wing buds by other colony members have been reported in some species of Kalotermitidae, resulting in regressive molt (Springhetti, 1969; Sewell and Watson, 1981; Zimmerman, 1983; Myles, 1986; Roisin and Pasteels, 1991; Roisin, 1994). Therefore, the pigmentation and sclerotization of wing buds in Type 2 nymphs observed in this study could be caused by biting among individuals. However, during the rearing experiments performed in this study, wing-bud biting among nymphs were not observed, so that there are also possibilities that other factors may induce the pigmentation and sclerotization of wing buds. A previous study in the family Kalotermitidae proposed that the regression from nymphs may be due to poor nutritional conditions (Myles, 1986; Roisin, 1994). Actually, there were no apparent bite scars in Type 3 nymphs in this study that underwent regressive molt, suggesting that such environmental conditions may cause the nymphal regression in H. sjostedti. It is also possible that the wing buds of nymphs may be bitten off by other castes, such as workers, soldiers and reproductives. To verify this possibility, rearing experiments should be conducted with various castes combinations and detailed behavioral observations will be necessary.
Physiological mechanism underlying regressive molt
To understand the physiological and developmental mechanisms controlling regressive molt in termites, gene-expression analyses were carried out focusing on endocrine factors and wing-patterning genes, by comparing regressive molt with alate differentiation and stationary molt (Figure 6). In principal component analysis on the expression data, the different molt types showed distinctive patterns along the PC2 axis, to which 4 factors (Kr-h1, E93, Vg and Foxo) highly contributed (Figure 6A).
The qPCR results of a wing morphogenetic factor Vg, and two endocrine factors (Kr-h1 and E93) showed that the expression patterns in Type 1 nymphs were different from those in other categories, while those in Type 2 and 3 were similar to those in stationary molt (Figures 6B–E), suggesting that the pattern of regressive molt is relatively similar to that of stationary molt, while different from that of alate differentiation. Also, the expressions of 4 genes (Kr-h1, E93, Vg and Foxo) may contribute to the determination of caste fates during the process, as suggested by previous studies showing the involvements of Kr-h1 and E93, i.e., key players in the MEKRE93 pathway, in the caste differentiation in termites (e.g., Belles and Santos, 2014; Korb and Belles, 2017; Oguchi et al., 2022).
Furthermore, Kr-h1, which is an early-response gene to juvenile hormone (JH), was highly expressed in Type 2/3 nymphs and stationary molt, although it was downregulated in Type 1 nymphs (Figure 6B), suggesting that the high JH level might positively regulate regressive molt. This result corresponds to some previous studies on regressive molt in termites (Korb et al., 2009, 2021). This also agrees with the fact that the low JH level during the nymphal intermolt required the alate differentiation (Nijhout and Wheeler, 1982; Cornette et al., 2008; Miura and Scharf, 2010). Furthermore, E93 that promotes imaginal molt and Vg, i.e., a master regulator of wing development, were both highly expressed in Type 1 nymphs before gut purge (Figures 6C,E), indicating that these factors may contribute to the development of wings and/or flight muscles. In contrast, the expressions of E93 and Vg were repressed in Type 2 and 3 (Figures 6C,E), suggesting that the alate development of these types of nymphs was arrested. These results on the physiological factors controlling caste differentiation in termites overall correspond to the general physiological mechanisms known in larval and imaginal molts in solitary insects. This may also indicate that the regressive molt in termites can be accomplished by modifications of the physiological mechanisms widely shared among diverse insect lineages.
This study suggests the similarity between regressive and stationary molts, but this phenomenon is also similar to the supernumerary molts and developmental delay, known in some cockroach lineages (Bell et al., 2007). In some cockroach species, developmental delays, such as an increase in the number of instars (i.e., supernumerary molts) and duration of instars, were observed in nymphs due to poor nutritional status or injury to body parts (Zabinski, 1936; Seamans and Woodruff, 1939; O'farrell and Stock, 1954; Willis et al., 1958; Tanaka et al., 1987). Therefore, some pre-adaptive physiological conditions of developmental plasticity may have contributed to the acquisition of stationary/regressive molts in termites. In some insect species, it was reported that environmental stresses affect the elevation of JH levels (Lin et al., 2004). In termites, conflicts among colony members and/or unfavorable nutritional conditions may trigger the regressive molt (Roisin, 1994), so that such stresses may contribute to the elevation of JH in nymphs, resulting in the induction of regressive molt. Since the developmental events for the adult-character formation including wings and compound eyes abruptly occur at the latest stage of alate development (Sehnal and Svacha, 1996; Roisin and Korb, 2010), nymphs before the latest stages still possess the potential to regressively molt to immature castes. Actually, in H. sjostedti and Z. nevadensis, it was reported that the rapid development of wings happens just prior to the molt into alates (Miura et al., 2004; Nii et al., 2019). Also, as revealed in this study, the developmental fate of nymphs is not determined at the molt to nymphal stage, but determined during the intermolt period, depending on JH level during the intermolt period (Figure 7B). Therefore, even after the molt into nymphs with developing wing buds, it may be possible to cancel the imaginal development, by entering the regressive developmental pathway, with relatively low costs.
Data availability statement
The original contributions presented in the study are included in the article/Supplementary materials, further inquiries can be directed to the corresponding authors.
Author contributions
KK, KO, and TM designed the study and performed field sampling. KK and KO maintenance of the animals and carried out several experiments. All authors contributed to the article and approved the submitted version.
Funding
This work was supported by a Grant-in-Aid for Scientific Research A (No. 25251041) for TM and by Grant-in-Aid for JSPS Fellows for KO (Nos. 21J01321 and 22K20662), from the Ministry of Education, Culture, Sports, Science and Technology of Japan.
Acknowledgments
We would like to express our gratitude to Soma Chiyoda, Sumio Udagawa, Run Minoura, Yoshinobu Hayashi, Shigeyuki Koshikawa, and Hitoshi Aonuma for their assistances in field sampling. We thank reviewers for their valuable comments on the manuscript. Thanks are also due to Takema Fukatsu, Takeo Kubo, Tetsuji Kakutani, and Rei Ueshima for their valuable comments and advices during the study.
Conflict of interest
The authors declare that the research was conducted in the absence of any commercial or financial relationships that could be construed as a potential conflict of interest.
Publisher’s note
All claims expressed in this article are solely those of the authors and do not necessarily represent those of their affiliated organizations, or those of the publisher, the editors and the reviewers. Any product that may be evaluated in this article, or claim that may be made by its manufacturer, is not guaranteed or endorsed by the publisher.
Supplementary material
The Supplementary material for this article can be found online at: https://www.frontiersin.org/articles/10.3389/fevo.2023.1200081/full#supplementary-material
SUPPLEMENTARY FIGURE S1
Molt and mortality ratios after 30 days. Molt ratio and mortality for each type after 30 days of rearing. The vertical axis represents the ratio to total number of rearing individuals.
SUPPLEMENTARY FIGURE S2
External morphologies of regressively molted individuals. (A,G) worker (W); (B,H) neotenic (Neo); (C,I) presoldier (PS); (D,J) regressively molted worker (RW); (E,K) regressively molted neotenic (RNeo); (F,L) regressively molted presoldier (RPS). All abdominal parts are female. Bars indicate 500 μm.
SUPPLEMENTARY FIGURE S3
Histological observation on reproductive organs of individuals in stock colony and regressively molted individuals. (A,G) worker (W); (B,H) neotenic (Neo); (C; Ny), (I; Ny): nymph; (D,J) regressively molted worker (RW); (E,K) regressively molted neotenic (RNeo); (F; A), (L; A): alate. Bars indicate 200 μm (A–F) and 100 μm (G–L), respectively. Allows indicate vitellogenic oocytes with columnar follicle cells.
SUPPLEMENTARY FIGURE S4
Expression pattern of hormone-related genes and wing-patterning genes. The expression patterns during the gut-purge period of all investigated genes are shown. St: stationary molt (worker). See “Results” for details on gene name abbreviations. Different letters on bars indicate significant differences (Tukey’s test, p<0.05). The number of samples was n = 4.
References
Andersen, C. L., Jensen, J. L., and Ørntoft, T. F. (2004). Normalization of real-time quantitative reverse transcription-PCR data: a model-based variance estimation approach to identify genes suited for normalization, applied to bladder and colon cancer data sets. Cancer Res. 64, 5245–5250. doi: 10.1158/0008-5472.CAN-04-0496
Anderson, M. (1984). The evolution of eusociality. Annu. Rev. Ecol. Syst. 15, 165–189. doi: 10.1146/annurev.es.15.110184.001121
Bell, W. J., Roth, L. M., and Nalepa, C. A. (2007). Cockroaches: ecology, behavior, and natural history. Baltimore: JHU Press.
Belles, X., and Santos, C. G. (2014). The MEKRE93 (Methoprene tolerant-Krüppel homolog 1-E93) pathway in the regulation of insect metamorphosis, and the homology of the pupal stage. Insect Biochem. Mol. Biol. 52, 60–68. doi: 10.1016/j.ibmb.2014.06.009
Cornette, R., Gotoh, H., Koshikawa, S., and Miura, T. (2008). Juvenile hormone titers and caste differentiation in the damp-wood termite Hodotermopsis sjostedti (Isoptera, Termopsidae). J. Insect Physiol. 54, 922–930. doi: 10.1016/j.jinsphys.2008.04.017
Cornette, R., Matsumoto, T., and Miura, T. (2007). Histological analysis of fat body development and molting events during soldier differentiation in the damp-wood termite, Hodotermopsis sjostedti (Isoptera, Termopsidae). Zool. Sci. 24, 1066–1074. doi: 10.2108/zsj.24.1066
Deligne, J. E. A. N., Quennedey, A. N. D. R. E., and Blum, M. S. (1981). “The enemies and defense mechanisms of termites” in Social insects. ed. H. Hermann (New York: Academic Press), 1–76.
Desneux, J. (1906). The Kashmir termite, Termopsis wroughtoni. J. Bombay Nat. Hist. Soc. 17, 293–298.
Grassé, P. P., and Noirot, C. (1947). Le polymorphisme social du termite a cou jaune (Kalotermes flavicollis F.) Les faux-ouvriers ou pseudergates et les mues regressive. C. R. Acad. Sci. 214, 219–221.
Howard, K. J., and Thorne, B. L. (2010) in “Eusocial evolution in termites and Hymenoptera” in biology of termites: A modern synthesis. eds. D. E. Bingell, Y. Roisin, and N. Lo (New York: Springer), 97–132.
Korb, J., and Belles, X. (2017). Juvenile hormone and hemimetabolan eusociality: a comparison of cockroaches with termites. Curr. Opin. Insect. Sci. 22, 109–116. doi: 10.1016/j.cois.2017.06.002
Korb, J., Greiner, C., Foget, M., and Geiler, A. (2021). How can termites achieve their unparalleled postembryonic developmental plasticity? A test for the role of intermolt-specific high juvenile hormone titers. Front. Ecol. Evol. 9:619594. doi: 10.3389/fevo.2021.619594
Korb, J., and Hartfelder, K. (2008). Life history and developmental framework for understanding developmental plasticity in lower termites. Biol. Rev. 83, 295–313. doi: 10.1111/j.1469-185X.2008.00044.x
Korb, J., Hoffmann, K., and Hartfelder, K. (2009). Endocrine signatures underlying plasticity in postembryonic development of a lower termite, Cryptotermes secundus (Kalotermitidae). Evol. Dev. 11, 269–277. doi: 10.1111/j.1525-142X.2009.00329.x
Koshikawa, S., Matsumoto, T., and Miura, T. (2001). Regressive molt in the Japanese damp-wood termite Hodotermopsis japonica (Isoptera: Termopsidae). Sociobiology 38, 495–500.
Kumar, S. (2007). Caspase function in programmed cell death. Cell Death Differ. 14, 32–43. doi: 10.1038/sj.cdd.4402060
Legendre, F., Whiting, M. F., and Grandcolas, P. (2013). Phylogenetic analyses of termite post-embryonic sequences illuminate caste and developmental pathway evolution. Evol. Dev. 15, 146–157. doi: 10.1111/ede.12023
Lenz, M., Amburgrey, T. L., Dai, M. J. K., Preston, A. F., Rudolf, D., and Williams, E. R. (1991). Interlaboratory studies on termite-wood decay fungi associations, II, response of termites to Gloeophyllum trabrem grown on different species of wood (Isoptera: Mastotermitidae, Termopsidae, Rhinotermitidae, Termitidae). Sociobiology 18, 203–254.
Lin, H., Dusset, C., and Huang, Z. Y. (2004). Short-term changes in juvenile hormone titers in honey bee workers due to stress. Apidologie 35, 319–327. doi: 10.1051/apido:2004018
Lin, N., and Michener, C. D. (1972). Evolution of sociality in insects. Q. Rev. Biol. 47, 131–159. doi: 10.1086/407216
Lüscher, M. (1974). “Kasten und Kasten-differenzierung bei niederen Termiten” in Sozialpolymorphismus bei Insekten. ed. G. H. Schmidt (Stuttgart: Wissenschaftliche Verlagsgesellschaft), 694–739.
Masuoka, Y., Yaguchi, H., Toga, K., Shigenobu, S., and Maekawa, K. (2018). TGF β signaling related genes are involved in hormonal mediation during termite soldier differentiation. PLoS Genet. 14:e1007338. doi: 10.1371/journal.pgen.1007338
Miller, E. M. (1942). The problem of castes and caste differentiation in Prorhinotermes Simplex (Hagen). Bull. Univ. Miami 15, 3–27.
Miura, T. (2005). Developmental regulation of caste-specific characters in social-insect polyphenism. Evol. Dev. 7, 122–129. doi: 10.1111/j.1525-142X.2005.05014.x
Miura, T., Hirono, Y., Machida, M., Kitade, O., and Matsumoto, T. (2000). Caste developmental system of the Japanese damp-wood termite Hodotermopsis japonica (Isoptera: Termopsidae). Ecol. Res. 15, 83–92. doi: 10.1046/j.1440-1703.2000.00320.x
Miura, T., Koshikawa, S., Machida, M., and Matsumoto, T. (2004). Comparative studies on alate wing formation in two related species of rotten-wood termites: Hodotermopsis sjostedti and Zootermopsis nevadensis (Isoptera: Termopsidae). Insect. Soc. 51, 247–252. doi: 10.1007/s00040-003-0736-2
Miura, T., and Scharf, M. E. (2010) in “Molecular basis underlying caste differentiation in termites” in biology of termites: A modern synthesis. eds. D. E. Bingell, Y. Roisin, and N. Lo (Dordrecht: Springer), 211–253.
Miyaguni, Y., Sugio, K., and Tsuji, K. (2013). Antennal cropping in the Asian dry-wood termite, Neotermes koshunensis. Insect. Soc. 60, 223–229. doi: 10.1007/s00040-013-0286-6
Myles, T. G. (1986). Evidence of parental and/or sibling manipulation in three species of termites from Hawaii. Proc. Hawaiian Entomol. Soc. 27, 129–136.
Myles, T. G. (1988). “Resource inheritance in social evolution from termites to man” in The ecology of social behavior. ed. C. N. Slobodchikoff (San Diego: Academic Press), 379–423.
Nii, R., Oguchi, K., Shinji, J., Koshikawa, S., and Miura, T. (2019). Reduction of a nymphal instar in a dampwood termite: heterochronic shift in the caste differentiation pathways. Evol. Dev. 10, 1–13. doi: 10.1186/s13227-019-0123-8
Nijhout, H. F. (2003). Development and evolution of adaptive polyphenism. Evol. Dev. 5, 9–18. doi: 10.1046/j.1525-142X.2003.03003.x
Nijhout, H. F., and Wheeler, D. E. (1982). Juvenile hormone and the physiological basis of insect polymorphisms. Q. Rev. Biol. 57, 109–133. doi: 10.1086/412671
Noirot, C. (1969). “Formation of castes in the higher termites” in Biology of termites. eds. K. Krishna and F. M. Weesner (New York: Academic Press), 311–350.
Noirot, C. (1985). “Pathways of caste development in the lower termites” in Caste differentiation in social Insects. eds. J. Watson, B. Okot-Kotber, and C. Noirot (Oxford: Pergamon Press), 41–57.
Noirot, C. H., and Pasteels, J. M. (1987). Ontogenetic development and evolution of the worker caste in termites. Experientia 43, 851–860. doi: 10.1007/BF01951642
Nutting, W. L. (1969). “Flight and colony foundation” in Biology of termites. eds. K. Krishna and F. M. Weesner (New York, NY: Academic Press), 233–282.
O'farrell, A. F., and Stock, A. (1954). Regeneration and the Moulting cycle in. Blattella Germanica L. III. Successive regeneration of both Metathoracic legs. Aust. J. Biol. Sci. 7, 525–536. doi: 10.1071/BI9540525
Oguchi, K., Koshikawa, S., and Miura, T. (2022). Hormone-related genes heterochronically and modularly regulate neotenic differentiation in termites. Dev. Biol. 485, 70–79. doi: 10.1016/j.ydbio.2022.02.012
Oguchi, K., Shimoji, H., Hayashi, Y., and Miura, T. (2016). Reproductive organ development along the caste differentiation pathways in the dampwood termite Hodotermopsis sjostedti. Insect. Soc. 63, 519–529. doi: 10.1007/s00040-016-0495-x
Peeters, C., and Higashi, S. (1989). Reproductive dominance controlled by mutilation in the queenless ant Diacamma australe. Naturwissenschaften 76, 177–180. doi: 10.1007/BF00366404
R Core Team (2020). R: A language and environment for statistical computing. R Foundation for Statistical Computing, Vienna, Austria. Available at: https://www.R-project.org/ (Accessed November 18, 2022).
Roisin, Y. (1990). Reversibility of regressìve molts in the termite Neotermes papua. Naturwissenschaften 77, 246–247. doi: 10.1007/BF01138496
Roisin, Y. (1994). Intragroup conflicts and the evolution of sterile castes in termites. Am. Nat. 143, 751–765. doi: 10.1086/285631
Roisin, Y. (2000). “Diversity and evolution of caste patterns” in Diversity and evolution of caste patterns. eds. T. Abe, D. E. Bignell, and M. Higashi (Dordrecht: Kluwer Academic Publishers), 95–119.
Roisin, Y., and Korb, J. (2010). “Social organisation and the status of workers in termites” in Biology of termites: a modern synthesis. eds. D. E. Bingell, Y. Roisin, and N. Lo (Dordrecht: Springer), 133–164.
Roisin, Y., and Pasteels, J. M. (1991). Polymorphism in the giant cocoa termite, Neotermes papua (Desneux). Insect. Soc. 38, 263–272. doi: 10.1007/BF01314912
Rosengaus, R. B., and Traniello, J. F. (1993). Temporal polyethism in incipient colonies of the primitive termite Zootermopsis angusticollis: a single multiage caste. J. Insect Behav. 6, 237–252. doi: 10.1007/BF01051507
Sameshima, S. Y., Miura, T., and Matsumoto, T. (2004). Wing disc development during caste differentiation in the ant Pheidole megacephala (Hymenoptera: Formicidae). Evol. Dev. 6, 336–341. doi: 10.1111/j.1525-142X.2004.04041.x
Seamans, L., and Woodruff, L. C. (1939). Some factors influencing the number of molts of the German roach. J. Kansas Entomol. Soc. 12, 73–76.
Sehnal, F., and Svacha, P., and Zrzavy, JZ. (1996). “Evolution of insect metamorphosis” in Metamorphosis: Postembryonic reprograming of gene expression in amphibian and insect cells, eds. L. I. Gilbert, J. R. Tata, and B. G Atkinson. (CA: Academic Press), 3–58.
Sewell, J. J., and Watson, J. A. L. (1981). Developmental pathways in Australian species of Kalotermes Hagen (Isoptera). Sociobiology 6, 243–323.
Springhetti, A. (1969). I1 controllo sociale della differenziazinoe degli alati in Kalotermes flavicollis Fabr. (Isoptera). Annali dell Universita di Ferrara (Nuova Serie) Sezione III (Biologia Animale) 3, 73–96.
Sugime, Y., Oguchi, K., Gotoh, H., Hayashi, Y., Matsunami, M., Shigenobu, S., et al. (2019). Termite soldier mandibles are elongated by dachshund under hormonal and Hox gene controls. J. Dev. 146:dev171942. doi: 10.1242/dev.171942
Tanaka, A., Ohtake-Hashiguchi, M., and Ogawa, E. (1987). Repeated regeneration of the German cockroach legs. Growth 51, 282–300.
Toga, K., Yoda, S., and Maekawa, K. (2011). The TUNEL assay suggests mandibular regression by programmed cell death during presoldier differentiation in the nasute termite Nasutitermes takasagoensis. Naturwissenschaften 98, 801–806. doi: 10.1007/s00114-011-0825-9
Vandesompele, J., Preter, K. D., Pattyn, F., Poppe, B., Van Roy, N., Paepe, A. D., et al. (2002). Accurate normalization of real-time quantitative RT-PCR data by geometric averaging of multiple internal control genes. Genome Biol. 3, 1–2.
Weesner, F. M. (1969). “External anatomy” in Biology of termites. eds. K. Krishna and F. M. Weesner (New York: Academic Press), 19–47.
Willis, E. R., Riser, G. R., and Roth, L. M. (1958). Observations on reproduction and development in cockroaches. Ann. Entomol. 51, 53–69. doi: 10.1093/aesa/51.1.53
Xu, D., Wang, Y., Willecke, R., Chen, Z., Ding, T., and Bergmann, A. (2006). The effector caspases drICE and dcp-1 have partially overlapping functions in the apoptotic pathway in Drosophila. Cell Death Differ. 13, 1697–1706. doi: 10.1038/sj.cdd.4401920
Yu, X., Wang, L., Acehan, D., Wang, X., and Akey, C. W. (2006). Three-dimensional structure of a double apoptosome formed by the Drosophila Apaf-1 related killer. J. Mol. Biol. 355, 577–589. doi: 10.1016/j.jmb.2005.10.040
Zabinski, J. (1936). Inconstancy of the number of moults during the post-embryonal development of certain Blattidae. Ann. Musei Zool. Polonici Warsaw 11, 237–240.
Keywords: regressive molt, stationary molt, alate differentiation, developmental totipotency, apoptosis, termite
Citation: Kobayashi K, Oguchi K and Miura T (2023) Physiological and developmental mechanism of regressive molt in a damp-wood termite Hodotermopsis sjostedti. Front. Ecol. Evol. 11:1200081. doi: 10.3389/fevo.2023.1200081
Edited by:
Kazuki Tsuji, University of the Ryukyus, JapanReviewed by:
Kiyoto Maekawa, University of Toyama, JapanTomonari Nozaki, Graduate University for Advanced Studies (Sokendai), Japan
Copyright © 2023 Kobayashi, Oguchi and Miura. This is an open-access article distributed under the terms of the Creative Commons Attribution License (CC BY). The use, distribution or reproduction in other forums is permitted, provided the original author(s) and the copyright owner(s) are credited and that the original publication in this journal is cited, in accordance with accepted academic practice. No use, distribution or reproduction is permitted which does not comply with these terms.
*Correspondence: Kohei Oguchi, ay5vaGdyZWVuMjI2QGdtYWlsLmNvbQ==; Toru Miura, bWl1QG1tYnMucy51LXRva3lvLmFjLmpw