- 1Department of Ecology and Evolutionary Biology, Tulane University, New Orleans, LA, United States
- 2Department of Biological Sciences, Dartmouth College, Hanover, NH, United States
Ecological niches are pivotal in addressing questions of species richness gradients like the Latitudinal Diversity Gradient (LDG). The Hutchinsonian niche hypervolume model and derivatives are some of the most proven tools. Accordingly, species occupy mathematically convenient spaces in relation to functional, especially trophic, relationships, as well as the physical environment. In one application, the number of species in a community is a function of average niche sizes, overlaps, and total niche volume. Alternatively, the number of coexisting species derives from invasibility criteria in relation to species-interaction modules. The daunting complexity of tropical communities begs the question of how well these ecologically inspired paradigms accommodate present knowledge of species interactions and functional relationships. Recent studies of hyperdiverse tropical insectivorous bird species suggests reevaluating the applicability of such concepts. Here I review Neotropical, arthropod-feeding bird species interactions needed to explain these species’ trophic relationships, including their diets, feeding substrates, and behavioral and morphological traits relevant to resource acquisition. Important emergent generalizations include extraordinary specializations on both prey resource locations (substrates) and behaviors, rather than on particular resources per se, and a preponderance of adaptations to exploit the anti-predator traits of prey, traits evolved in response to other predators. These specializations and implicit arms races necessitate evolutionary approaches to niches necessary to understand the relevant natural history and ecology, how these species compete interspecifically, and even how these predator species interact with prey via evolutionary enhancements. These findings, compared and contrasted with prevailing concepts and findings, suggest expanding niche concepts to accommodate both the large temporal and regional geographic scales to understand the accumulated species richness of the mainland Neotropics. These trophic specializations also highlight why many of these birds are so sensitive to human disturbances, especially habitat loss, fragmentation, and degradation.
1 Introduction
Ecologists have long recognized diverse patterns of species richness on multiple spatial scales. The best recognized pattern is the Latitudinal Diversity Gradient (LDG), which has inspired many explanations, in part because of its global scale, with dozens of ecological, evolutionary, and hybrid hypotheses (e.g., Mittelbach et al., 2007; Fine, 2015; Pontarp et al., 2019; Fenton et al., 2023). This plethora of hypotheses suggests the challenge of achieving consensus, due partly to a variety of competing or complimentary explanations. Understanding tropical diversity is particularly daunting because of the variety of species and species interactions.
Niche concepts have played a central role in most of these hypotheses for the LDG and other species-richness gradients because the number of coexisting species is generally held to correspond with the variety of niches (e.g., McPeek, 2022). A variety of niche concepts have been described, notably Grinnell’s locating habitat in relation to a variety of environmental variables including climatic; and Elton’s emphasis on species interactions such as competition and predation, and at relatively smaller spatial scales (e.g., Soberón, 2007). Hutchinson’s (1957) n-dimensional niche hypervolume (e.g., Chase and Leibold, 2003; Holt, 2009; Mittelbach and McGill, 2019; McPeek, 2022) incorporates elements of both Grinnellian and Eltonian niche concepts, and has greatly stimulated research. Like other niche concepts, the n-dimensional hypervolume model makes important assumptions and predictions: Interspecific competition is generally viewed as a central, but emphatically not the only relevant ecological process (McPeek, 2022). Resources for which species compete are often assessed along a variety of resource gradients in the hypervolume that circumscribes demographically viable conditions. Ecological approaches to niches often assume their existence independently of the history of species traits. Species are also often assumed to be restricted by competitors to the realized niche, representing a subset of conditions circumscribed by the fundamental niche. Niches compete for space in the hypervolume.
A widespread application of niche concepts has been addressing whether relatively species-rich regions such as the Neotropics result from greater ecological specialization (smaller niches), greater niche total volume, and/or greater niche overlap, which latter might result from disturbances necessitating ecological flexibility. Another more recent niche concept builds on mathematical models of population and species interactions and coexistence criteria. Using a few distinctive modules of largely trophic species interactions, in the tradition of Eltonian niche concepts, these models address coexistence typically under conditions of model equilibria or community invasibility (McPeek, 2017; Mittelbach and McGill, 2019; McPeek, 2022). Modules include interspecific competition, apparent competition (interaction via shared predator), predator-prey interactions (including parasites, parasitoids, herbivores), generalists vs. specialists, keystone species, and non-equilibrial species such as “walking dead” and neutral species. These explicitly mechanistic modules address both direct and indirect species interactions and multiple species per trophic level (e.g., McPeek, 2022) so as to explain species diversity patterns.
Niche concepts have been widely applied to diverse organisms and ecosystems. Overemphasis on interspecific competition as the ecological force shaping the niche, in Hutchinson’s (1957) and many derivatives, led to enough controversy that some ecologists have advocated abandoning niche concepts altogether (Chase and Leibold, 2003). However, ecologists have found niche concepts to remain sufficiently useful to inspire a variety of theoretical constructs and empirical investigations (e.g., Blonder, 2018; McPeek, 2022).
Niche ecology, often devoid of evolution, still drives much thinking and research, and occupies much textbook and primary literature. Considerations of spatial and temporal scale, and the importance of evolutionary phenomena in particular, have advanced understanding of the LDG, moving the discourse beyond ecological niche explanations alone (e.g., Ricklefs and Schluter, 1993; Mittelbach et al., 2007; Mittelbach and McGill, 2019). Evolutionary approaches generally approach relevant phenomena like speciation at biogeographic scales, and ecological processes including competition and predation to more local scales. However, to the extent that species richness depends ultimately on the difference between speciation and extinction rates, phenomena like the LDG are quintessentially evolutionary, requiring evolutionary framing (e.g., Mittelbach et al., 2007; Fenton et al., 2023).
One of the greatest challenges to developing any general theory of species diversity patterns is to integrate evolutionary with ecological processes and methods explicitly, address multiple spatial and temporal scales, incorporate new empirical information, and clarify the roles of different categories of species interactions. This review addresses these challenges so as to point research in a direction that can better synthesize niche concepts. Specifically, this paper (1) briefly reviews methods, findings, and implications of recent research on insectivorous Neotropical bird species and their insect prey (Sherry et al., 2020; Sherry and Kent, 2022); (2) reviews the mechanistic Biotic Challenge Hypothesis (BCH) inferred from this extensive natural history involving bird-insect arms races; (3) enumerates a variety of ways in which the BCH challenges widely held niche and community patterns and processes; and (4) points future research in the direction of unifying principles that can apply widely to daunting tropical species diversity and interactions. Too often contradictory niche concepts are critical to review and resolve, not least of all because of their conservation implications.
2 Lessons from neotropical insectivorous birds
A useful operational niche definition is “the joint description of the environmental conditions that allow a species to satisfy its minimum requirements so that the birth rate of a local population is equal to or greater than its death rate along with the set of per capita effects of that species on these environmental conditions” (Chase and Leibold, 2003, p. 19). Minimum requirements for most species include limiting resources. Food resources have traditionally been assumed to limit populations of vertebrates like birds, and will be the focus here as well; and as will become clear this leads to a variety of novel insights about coexistence in these birds. It must be acknowledged that safe nest sites also potentially limit bird populations, particularly tropical populations (e.g., Visco and Sherry, 2015; Visco et al., 2015); and even though safe nesting sites are not as obvious resources as foods, they must eventually be integrated with food resources into niche concepts—beyond the scope of this paper. This working niche definition also acknowledges reciprocity, i.e., impacts of both environment on species and vice versa, which is important in the present context.
In our review of insectivorous Neotropical birds (Sherry et al., 2020) we took advantage of a comprehensive list of bird species that are Neotropical endemics and primarily insectivorous: Arthropods, mostly insects and spiders, comprised = 70% of diet, based on a database of birds of the world (Şekercioğlu et al., 2004; Şekercioğlu et al, 2019). Henceforth I use “insectivorous/insectivore” for species that feed largely on arthropods, primarily insects and spiders. This list of bird species guided a review of diets and foraging behavior, to the extent of data availability, primarily from family accounts in Handbook of Birds of the World, supplemented by other relevant studies. Diet data are particularly scarce, despite their importance to niche concepts as described below. Simultaneously, insect defenses against predators, again emphasizing the tropics, were reviewed and summarized by Sherry, 2021, Supplemental Material Appendices A and B; https://academic.oup.com/auk/article/137/4/ukaa049/5901431#supplementary-data). This fundamental natural history information revealed patterns relevant to these birds’ tropical niches, which in turn contributed to a novel synthesis, the Biotic Challenge Hypothesis, addressing the origins and coexistence of these hyperdiverse tropical birds and their coevolutionary arms races with their prey.
“Hyperdiverse” refers to extraordinary species richness. The Neotropics contain 3,314 bird species in total, 2,079 (just over 60%) of which are insectivorous (Sherry et al., 2020). Inclusion of seasonal visitors to the tropics, mostly latitudinal migrants, increases these numbers to 2,250 insectivores out of 3,567 total species, a substantial proportion of the ~11,000 bird species globally. This diversity has encompassed about 66 million years of evolutionary history (Sherry et al., 2020), beginning with the Chicxulub asteroid impact that triggered the mass extinction of most dinosaurs and lots of other organisms. The Neotropics contain more bird species (Harvey et al., 2020) as well as more diverse trees, fishes, and other taxa than any other terrestrial region globally (Antonelli et al., 2018).
2.1 Importance of interspecific competition?
The scant dietary data available for Neotropical birds provide an important perspective on the prevalence and nature of interspecific competition. For example, review of the families of Neotropical birds indicated repeated reference to prey taxa shared widely by Neotropical insectivorous birds, including Orthoptera (especially Tettigoniidae = katydids), ants, termites, diverse beetles, and earthworms in the case of a variety of ground-feeding insectivores. Up to 120 species of insectivorous birds coexist locally in the Neotropics (Sherry et al., 2020), and dietary plus foraging behavior implicate substantial dietary overlap. For example, diverse army ant-following birds eat a variety of the same Orthoptera and other insects that other flycatchers, woodcreepers (Furnariidae), and mixed-species flock foragers hunt using different search-and-capture tactics (Sherry et al., 2020). Tropical diet studies, controlling for availability fluctuations in time and space, have reinforced a widespread tendency for coexisting birds to consume substantially the same, locally available, prey (Sherry et al., 2016; Kent and Sherry, 2020; Kent et al., 2022; Sherry and Kent, 2022). Sherry and Kent (2022) inferred strong interspecific competition among coexisting wood warblers (Parulidae), particularly while wintering in Jamaica, based on a method predicated on three basic criteria proposed by Dhondt (2012): (1) two or more coexisting species are resource-limited, (2) they compete intraspecifically for these resources, and (3) they overlap in use of these same resources. Given the general dearth of aggressively overt contest competition (but see Robinson and Terborgh, 1995), We (Sherry et al., 2020; Kent et al., 2022; Sherry and Kent, 2022) inferred that widespread and demonstrable competition is largely diffuse and exploitative, a mechanism potentially overlooked because of a dearth of relevant dietary data and tests. Diffuse interspecific competition is emphasized here because of its spotty recognition, some authors addressing it (e.g., McPeek, 2022) and others not (e.g., Mittelbach and McGill, 2019).
2.2 Foraging specializations
Integrating Neotropical avian insectivore foraging behavior with insect anti-predator adaptations (Sherry et al., 2020) indicated some previously unrecognized patterns with particular relevance to these birds’ ecological/evolutionary niches, and to diffuse interspecific exploitation competition. The most novel and consequential pattern was the overwhelming preponderance of specialized Neotropical avian insectivores adapted to exploit the anti-predator traits of their prey (Table 1). Sherry et al. (2020) interpreted these species interactions as evolutionary “enhancement” (Charnov et al., 1976), a distinctive and poorly appreciated evolutionary phenomenon. Enhancement results because the traits evolved by some prey against particular predators provide an opportunity for exploitation by another set of predators adapted to these defensive traits. The initial defenses likely adapted possessors, whose increased ecological abundance and predictability created a new target for predators that could exploit the defense. A familiar—albeit non insectivore—example of enhancement involves piscivorous marine fish that select for prey to school and swim to the surface, and even (in the case of flying fish) aerially escape the predators below, creating the foraging opportunities for predators such as cormorants, boobies, and frigatebirds hunting from above, and vice versa. Enhancement explicitly recognizes diet overlap, even by species foraging distinctively in terms of morphology, behavior, and substrate. Enhancement is distinctive from ecological facilitation, which provides an immediate and typically unidirectional ecological benefit, exemplified by nitrogen-fixing plants providing the soil nutrient conditions that other plants exploit immediately, e.g., in a terrestrial plant succession. Enhancement can benefit different predator guilds, but is distinctive from mutualism insofar as the participants interact only indirectly. Enhancement evidences multiple sequential (and not necessary contemporary) evolutionary adaptions: Initial predation➔evolution of anti-predator adaptations by prey➔opportunity for another class of predator to exploit with novel foraging traits, following subsequent adaptations favoring such exploitation. Enhancement is probably coevolutionary, particularly of the prey and the specialized predators exploiting the prey anti-predator adaptations, but documenting co-evolution is challenging. This is because enhancements are typically highly diffuse, involving multiple predator species, both the more generalized predators initiating and maintaining the prey anti-predator adaptations and the more evolutionarily derived exploiters of the anti-predator adaptations.
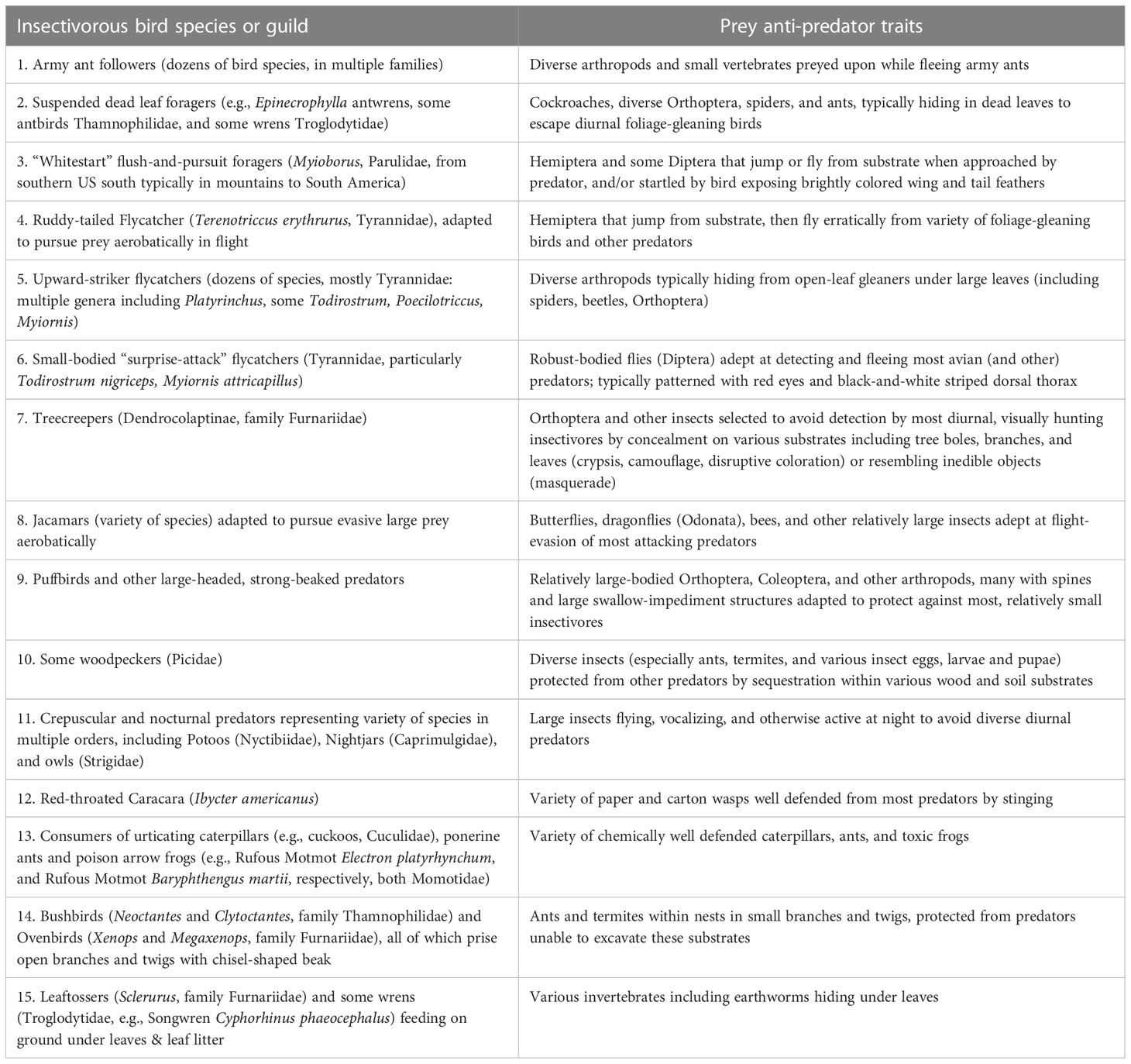
Table 1 Some examples of Neotropical Insectivorous birds and bird guilds adapted to exploit the anti-predator traits of their prey. Sources of information about these birds’ foraging behaviors and some relevant morphological traits given by Supplemental Material Appendix A in Sherry et al. (2020) (https://academic.oup.com/auk/article/137/4/ukaa049/5901431#supplementary-data). Supplementary Material Appendix B (same source) provides an overview of Neotropical insect anti-predator traits.
A large variety of tropical insectivorous birds coexist via enhancement (Table 1), a phenomenon poorly incorporated into most niche concepts. One implication of enhancement for niches is that they are not something waiting to be exploited, intrinsic to habitats, but rather are the product of evolutionary species interactions and histories (Vermeij, 1994). These histories, and the kinds of enhancements, likely differ by continent, latitude, and species-richness within latitude such as islands vs. mainlands—making them contingent on biogeography. Enhancements, and other potentially evolutionary tropical species interactions (predator–prey, mutualistic, parasite–host, competitive) pose challenges to a variety of conventional niche-theoretical ideas. Because enhancements are evolutionary species interactions, they emphasize how “ecological” niches are often actually evolutionary phenomena, particularly conspicuous and maybe relatively frequent in species-rich tropical environments, but undoubtedly general (McPeek, 2017, e.g., Figure 3.13 and associated text). Enhancements, and the evolutionary arms races involved, necessitate expanding niche concepts to be more comprehensive than simply an ecological resource or habitat available for occupation. Enhancements can also be viewed as the evolutionary equivalent or homolog of diverse indirect ecological species interactions emphasized by many of McPeek (2022) modular food webs.
A second pattern that dietary specializations in Neotropical insectivores revealed was adaptations better defined by foraging (and dietary) stereotypy than by narrow or restricted range of resources (Sherry, 1990; Sherry et al., 2020; Sherry and Kent, 2022). Diets can be broad, depending on what prey types occur in the substrates birds exploit, but can be nearly identical among conspecific foraging individuals because of stereotypical foraging adaptations. For example, the upward-strike foraging tactics of species like the Golden-crowned Spadebill (Platyrinchus coronatus) and other Neotropical flycatchers (Table 1) expose it to a consistent variety of insects and arthropods refuging beneath large tropical leaves (Sherry, 1984)—hence the stereotypy in foraging behavior (substrates, attack mode) and diet. The importance of this pattern is that the stereotypical behavior of many tropical foragers is linked to particular foraging situations or substrates, emphasizing the evolutionary nature of foraging niches (Sherry, 1990). Sherry and Kent (2022) illustrate diagrammatically how diffuse competition coupled with optimal foraging can drive substrate specializations despite high dietary overlap.
These insectivorous bird–insect relationships also emphasize the adaptations not for particular insects per se, i.e., not specifically on trophic relationships of these predators and prey in the context of food webs, but rather on insect traits. These prey traits include all the anti-predator behaviors exemplified in Table 1, including fleeing behaviors, stinging, distastefulness, all manner of cryptic and related defenses of disguise, mimicry, and the substrates selected by these prey, such as leaf undersides and suspended dead leaves as places of concealment. Adaptations of the predators for foraging emphasize multiple trophic levels, namely predators and prey, and not just partitioning of resources between/among competitor species within the same (predator or competitor) trophic level. Many of the most distinctive predator traits involve adaptations to exploit a particular substrate. Models of predator–prey trophic interactions and food webs that ignore such prey traits overlook important natural history revealed by these bird–arthropod arms races and evolutionary enhancement.
2.3 Biotic challenge hypothesis
The foregoing phenomena, particularly diffuse competition from hundreds of other species seeking insect prey plus the arms races involving insect predators and their prey, motivated the BCH (Sherry et al., 2020). This hypothesis asserts that the mainland tropics, particularly the Neotropics with its prodigious species richness, constitutes a challenging environment to acquire sufficient food because of both perennial competition for insect prey depressing their abundance and the anti-predator adaptations of the prey exacerbating their elusiveness (Figure 1). This foraging challenge to insectivores has favored evolution of both the foraging specializations allowing these predators to exploit arthropods refuging in particular substrates and other prey traits, and physiologically conservative life-histories to increase metabolic efficiency.
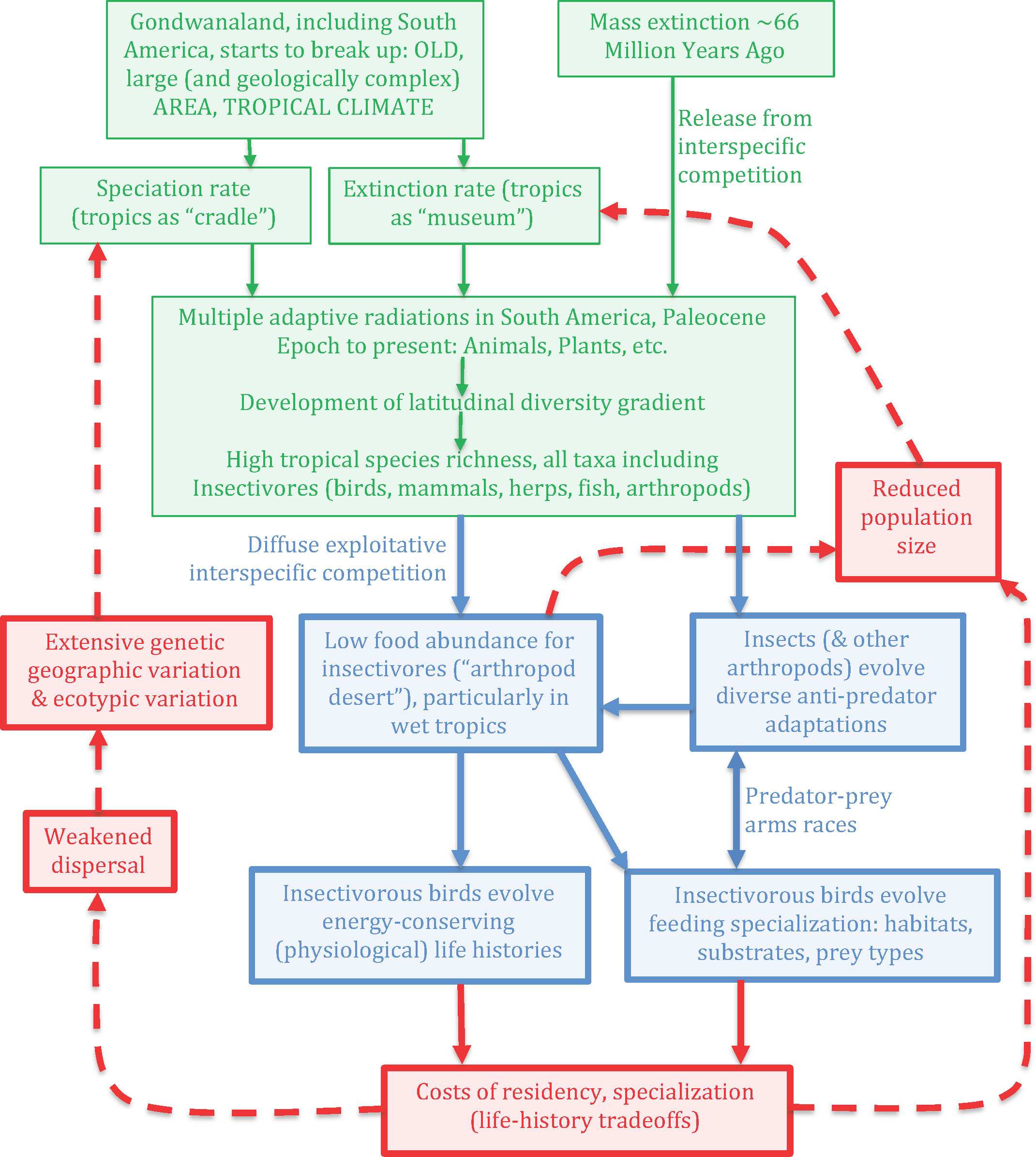
Figure 1 Flow diagram for the evolution of ecological specialization and energy-conservation physiology in species-rich tropical communities via the Biotic Challenge Hypothesis (modified from Sherry et al., 2020, original figure copyright American Ornithological Society and Oxford University Press). All caps in upper left box emphasize conventionally recognized contributions to high tropical species diversity following mass extinction 66 MYA (green boxes and arrows), namely large area, age, and tropical (relatively aseasonal) climate. Heavily outlined boxes represent novel components of the BCH theory itself (blue), life-history consequences (red box at bottom of figure), and positive feedbacks (red, and dashed lines), ultimately on speciation and extinction rates. Although not central to the theory of evolution of community structure via ecological specializations, positive feedback on speciation rate at low latitude (upward oriented dashed arrows, left-hand side of figure) represent consequences of specialization for life histories and reduced dispersal ability. Added to this diagram since Sherry et al. (2020) is another feedback mechanism contributing to species extinctions via reduced population size (heavy red, dashed lines, right side of figure).
A few general aspects of the BCH are important to emphasize before contrasting its predictions with widespread niche concepts below. BCH builds on widely accepted drivers of species richness, especially in the tropics, namely large Neotropical area, long age since major disturbance, and tropical climate with relatively reduced seasonality compared to higher latitudes (e.g., Mittelbach et al., 2007; Fine, 2015, other references in Sherry et al., 2020). The Chicxulub asteroid impact 66 MYA was particularly important by driving most birds and bird-like reptiles extinct (Prum et al., 2015). The BCH adds onto these ideas the multiple adaptive radiations comprising insectivorous Neotropical birds (Sherry et al., 2020), plus a variety of other insectivores extant in the Neotropics today, including mammals such as anteaters and small primates, a variety of reptiles and amphibians, invertebrates including insects and spiders, and even some plants—all contributing to diffuse food competition. The BCH incorporates the fundamental evolutionary driver of species richness patterns, namely speciation rate exceeding extinction rate, where terrestrial species richness peaks in tropical mainland areas like lowland South America.
The BCH mechanism (Figure 1) incorporates the foregoing evolutionary and ecological processes, and adds a second feedback, compared to the Sherry et al. (2020) version, namely connecting evolutionary feeding specialization and energy-conservation physiological adaptations back to reduced population size and extinction rate, which in turn feeds back negatively on species richness. By increasing extinction rate, this feedback counters what would otherwise be endless proliferation of species from the positive feedback loop on left-hand side of figure. Species and lineages have not proliferated endlessly, and in fact divergence appears to slow down at least within lineages, consistent with niche-filling (e.g., Pigot and Tobias, 2013). This negative feedback involves a variety of ecological and evolutionary processes that put species at risk of extinction: Ecologically, opportunities are increasingly exploited as species fill up existing trophic opportunities, i.e., ecological/energy limits. Evolutionarily, species tradeoff increasingly efficient exploitation via foraging specializations with poor dispersal capacity (Salisbury et al., 2012; Sherry et al., 2020) creating vulnerability to environmental fluctuations in trophic opportunities. Also, rarity, which is particularly notable in tropical insectivorous birds (Robinson et al., 2000) and results from the accumulation of species over long time periods due to multiple adaptive radiations, can increase population vulnerability to extinction in a fluctuating environment (e.g., Storch et al., 2017; Curtis et al., 2021). The resulting tropical species richness (largest green box in figure) ultimately results from speciation rate exceeding extinction rate on average compared to higher latitudes and elevations, albeit not indefinitely.
3 Widespread niche concepts versus the BCH
Ten questions are addressed next that compare and contrast widespread niche concepts with the BCH.
3.1 What is a niche?
The most widely applied niche concept is Hutchinson (1957) n-dimensional niche hypervolume model, which represents the window of ecologically favorable physical and biological conditions for coexisting species. An important innovation was the abstract geometric (volumetric) representation of species’ ecological and geographical requirements underlying their competition, and the applicability of powerful mathematical and statistical methods for ease of model testing (Blonder, 2018). This hypervolume and derivatives (e.g., for climate, phylogenies) assume that nearby species in niche space have nearby properties, which allows mapping species relationships mathematically from one set of data to another, and which in turn assumes continuous variables at least for purposes of mathematical analyses. This is problematical with tropical insectivorous birds, because according to the BCH, foraging (trophic) specializations typically involve prey behavioral and substrate adaptations, which are anything but continuous variables, as illustrated by prey flushed by army ants, refuging under large leaves, hiding in suspended dead leaves, and jumping in escape tactics (Table 1).
Another important, yet questionable niche hypervolume assumption is that proxies for actual resources or resource traits map onto species competitive relationships underlying positions within the niche hypervolume. One proxy is “niche partitioning” (others treated in subsequent section). MacArthur (1958 and subsequently) reinforced Hutchinson’s niche hypervolume concept by making it operational around the concept of “resource partitioning”, i.e., divergence in niche space resulting from interspecific competition for population-limiting resources. However, the resources underlying competition are often difficult to quantify, and many studies substitute “niche partitioning”, even using “resources” and “niches” interchangeably (e.g., Owen-Smith et al., 2017). The problem is that the forces shaping niches are often obscured or ignored. Other forces than interspecific competition can cause species to diverge (e.g., apparent competition, driven by shared predators), and interpreting species niche differences, especially using surrogates (Section 3.5), is risky.
A recent study of Amazonian woodcreepers (Dendrocolaptinae; Powell et al., 2022) is illustrative. This study admirably tackles coexistence in a hyperdiverse Neotropical taxon using a Hutchinsonian niche framework, but includes niche traits so disparate and removed from resources as to obscure potential competition. These authors assert (Abstract) that “The behaviors we quantified (sociality, vertical strata, and myrmecophily [following ant swarms]), together with morphology (mass and bill size), separated all 13 [coexisting] species”, but how do these traits “separate” resources? Sociality as indexed by participation in mixed-species flocks are linked to protection from predators and mutualism (e.g., Sridhar et al., 2012), and in any case flock members forage differently for different prey (Sherry et al., 2020). Powell et al. classified bird species as myrmecophilous or not, despite different degrees of myrmecophily; and striking differences in body mass within this guild signified social dominance rather than any prey traits per se. Very little vertical stratification occurred, and when it did it was unelated to any potential prey differences. Bill size differences were not linked to prey size differences (such data being unavailable), and importantly distinct bill shapes were not considered here, such as that of the Curved-billed Scythebill Campyloramphus procurvoides) associated with distinctive foraging substrates (and prey taxa), in this case bamboo stems and substrates concealing prey (Marantz et al., 2003). Powell et al. assume, probably correctly, that these species compete for arthropod prey, but ordination by actual prey categories—were such data available—would almost certainly lead to different species relationships and conclusions.
3.2 Which came first, niches or species?
Niche concepts are widely applied to explain biological diversity, exemplified by the LDG. Building on theoretical ideas of Volterra, Lotka, and Gause, MacArthur (1972) championed a conceptual basis for ecological community structure as a function of niche width, overlap, and volume, while Hutchinson (1959) articulated the goal of addressing species coexistence and diversity (see also Pigot et al., 2016). Community Ecology has built on these ideas in proposing essentially the following chain of causation for species diversity (e.g., Pianka 1966, most explicitly, in his climatic-stability hypothesis), a key step in which is increased specialization and greater niche volume allowed by greater tropical ecological stability.
3.2.1 Ecological stability (e.g., in tropics) ➔ increased specialization ➔ increased niche packing and larger niche volume ➔ more species coexisting via niche (resource) partitioning
The BCH assumes a fundamentally different chain of causation for coexistence and specialization, in which the ultimate driver of species diversity is speciation minus extinction rates (e.g., Mittelbach et al., 2007). Speciation has exceeded extinction longer in the Neotropics than other terrestrial environments, accounting for greater tropical species accumulation. Ecological stability is thus important to species coexistence, but probably via its indirect impacts on speciation and extinction (Figure 1) rather than directly as implied by this bolded ecological scenario.
The idea that the accumulation of species has driven—i.e., necessitated, rather than allowed—specialization and coexistence simplifies as follows:
3.2.2 Population allopatry (via dispersal or vicariance) ➔ accumulation and initial independent differentiation of sister populations (diffuse competition, arms races, etc.) ➔ dispersal and secondary contact ➔ coexistence via foraging specialization, with or without direct competition/character displacement
This contrast between conventional models of competition as a direct driver of species coexistence versus the more explicitly evolutionary BCH scenario emphasizes the greater temporal and spatial scales of the latter (upper left-hand box, Figure 1; references in Sherry et al., 2020). The BCH encompasses the many Neotropical adaptive radiations, most starting post mass extinctions, 66 million years ago (Prum et al., 2015). These adaptive radiations contribute to diffuse competition among diverse insectivorous clades and species.
Both conventional and BCH scenarios incorporate biological interactions, including predator–prey trophic interactions consistent with Eltonian niches, but the BCH incorporates improved understanding of tropical speciation. Ultimately, ecological differentiation is necessary for coexistence (Pigot and Tobias, 2013), but the issue is the sequencing and timing of the coexistence mechanisms. Long time scales and large areas, exemplified by the Neotropics, have created exceptional opportunities for population (Salisbury et al., 2012) and species accumulation. Moreover, mainland tropical speciation requires long time periods (several million years; Salisbury et al., 2012), encompassing some divergence both during the early, allopatric, and subsequent secondary contact phases (Anderson and Weir, 2022). Isolation of allopatric populations precedes, and thus occurs somewhat independently of the latter coexistence phase. Evolution of insectivores in response to prey traits are likely as important in the long term—and could occur throughout speciation processes—as direct niche divergence in response to incipient species that is limited to the latter, sympatric phase.
Thus, niches are not just resource spaces to be occupied, but rather the outcome of direct and indirect species interactions, both within and among trophic levels. This incorporation of evolutionary species interactions parallels ecological niche conceptions that explicitly incorporate other species as niche axes (e.g., McPeek, 2022), indicating that niches beget niches and on evolutionary as well as ecological time scales. Plants at the bottom of food chains require resources like Nitrogen, Phosphorous, light, and CO2, thus exploiting these resources without co-evolution, but tropical insectivorous birds interact with their prey evolutionarily as well as ecologically. Arthropods must have evolved particular anti-predator traits in order for the existence of new niches to be exploited by new predators (Table 1). To take one example, suspended dead leaf foragers consume arthropods, a trophic ecological interaction, but this niche would not exist were it not for the selection by diverse other insectivores for arthropods that hide in this substrate, an evolutionary enhancement.
3.3 Do species “partition” resources in response to competition?
One of the most consequential ideas arising from niche theory and niche hypervolume concepts is that species coexist by partitioning niches so as to reduce competition. The first component—partitioning niches—is a widespread assumption, e.g., Powell et al. (2022) discussed above, and Kricher (2017, p. 300): “Because they have differing body sizes and bill shapes, several species of woodcreepers coexist and feed with little or no apparent competition”. This often implicit assumption that species must occupy unique niches, i.e., compete with each other to take up reduced-overlap space along one or more niche axes intrinsic to the environment, has inspired considerable research. Tropical niches can accordingly explain the LDG by greater species packing, resulting from either more niche overlap or smaller niche size/volume (specialization); and/or availability of greater total niche space, e.g., via predictable year-round ecosystem productivity of distinctively tropical resources like large insects or fruit (e.g., Fine, 2015). Most early applications emphasized greater species richness via greater range of resources available year-round (e.g., MacArthur, 1969; Orians, 1969; Schoener, 1971; Askins, 1983), although Marra and Remsen (1997) provided evidence for greater tropical specialization via smaller foraging niches. Orians’ study illustrates ecological approaches by identifying the relatively wind-free lowland tropical rainforest interior environments with large-leaved plants as a niche supporting a number of species of sit-and-wait predators like puffbirds, jacamars, and some of the upward-striker flycatchers; and Zimmer and Isler (2003) added dead leaf clusters containing arthropods as a Neotropical niche. The BCH invokes evolutionary processes explicitly by recognizing the predator–prey arms races that have driven so many arthropods to refuge under large tropical leaves and in dead leaf clusters in the first place.
Contemporary research advances on these early niche studies in important ways, while persisting in an essentially Hutchinsonian, resource-partitioning niche approach by asking whether tropical species either pack more closely in niche space or occupy larger niche volumes. Most contemporary studies also assume—often implicitly—that niche evolution occurs primarily within a trophic level, e.g., via competition (but see Schleuning et al., 2022). Most of these studies include surrogates for diet, such as morphological traits (e.g., Pigot et al., 2016; Pigot et al., 2020; Hughes et al., 2022) and proportions of dietary categories such as invertebrates, carrion, fruit, nectar, etc. (Jarzyna et al., 2021); although Schumm et al. (2020) incorporated arthropod sizes and abundance to explain the ecological carrying capacity for Himalayan insectivorous bird species, with comparisons to several tropical mountain ranges (see also Pigot et al., 2016). Recently constructed global-scale morphological data sets (Pigot et al., 2016; Pigot et al., 2020; Tobias, 2022), including detailed beak shape (Hughes et al., 2022); facilitate such broad-scale comparative studies, as do phylogenetic distances (Pigot et al., 2020; Schumm et al., 2020; Jarzyna et al., 2021). This research supports important generalizations: (1) the importance of morphological convergence in birds globally (Pigot et al., 2020), (2) a tendency for high species richness to be associated with both greater species packing and larger niche volume (Pigot et al., 2016; Jarzyna et al., 2021; Hughes et al., 2022), and (3) acknowledgement of both non-equilibrium historical (evolutionary) and equilibrium ecological coexistence processes contributing to community structure (e.g., Schumm et al., 2020). The BCH takes advantage of insectivore diet data, coupled with knowledge of foraging behavior and relevant morphology and physiology, to complement these more statistical, and often more global-scale studies. The BCH differs from these studies primarily by suggesting an explicit, non-Hutchinsonian, mechanism for tropical species richness (Figure 1) and by incorporating resources (as opposed to surrogates—see Section 3.5) and bird–arthropod co-evolution into the mechanism. The BCH is more non-equilibrial than equilibrial in the sense of Schumm et al. (2020), although Figure 1 includes a tendency towards an evolutionary equilibrium, or at least asymptotic species richness over long time-periods.
The second component, or assumption of many resource partitioning studies is that niche partitioning reduces interspecific competition, e.g., as envisioned in reduced overlap among coexisting species in some early models of competitor species arrayed along one or more resource axes (e.g., Figures 8–16 in MacArthur, 1972). These models imply reduced competition as niches slide apart from each other in response to interspecific competition, or are kept apart by community assembly constraints. However, if competition is thus reduced, then niche adaptations relevant to competition must be in response to fleetingly intense past competitive episodes whenever ongoing competition is not demonstrable, as is often the case (the ghost of competition past; Connell, 1980).
The BCH suggests, instead, that interspecific competition is ongoing, more or less continually maintaining the foraging specializations, at least as long as the same constellation of species depresses the same resources diffusely (Sherry and Kent, 2022). The BCH argues that species are adapted to specialize on resource-related traits rather than shifting evolutionarily in relation to competitor species per se (see Section 2.2). To the potential argument that this difference is semantic, note that resource traits like foraging substrates are not easily arrayed along any particular niche axes; and species do not diverge in their use of these traits so much as specialize on them adaptively. These specializations are adaptations to different adaptive peaks (resources and resource traits like refugia from many predators), and so divergence may occur, but because species are adapting to different peaks rather than diverging from each other per se.
According to the BCH, ongoing diffuse, exploitative interspecific competition shapes foraging behavior and morphology of tropical insectivorous birds adaptively (Sherry et al., 2020; Sherry and Kent, 2022). Just a few such traits (Table 1): Upward-striker flycatchers are characterized by broad, flat beaks to exploit arthropods hiding under large leaves like palms and aroids and flycatchers that pursue prey aerobatically tend to have relatively large wings and tail (Leisler and Winkler, 1985). Epinecrophylla Antbirds have long legs and bills, for acrobatically reaching and immobilizing insects within suspended dead leaf clusters. Such temporarily stable and ongoing selection pressures on foraging behavior should be exacerbated in species-rich environments where competition is relatively diffuse, with many competitors, sometimes >100 species vying for many of the same resources, continually, year-round and over longer time periods in the most equatorial, lowland, species-rich communities such as Amazonia. An estimated 121 and 53 resident endemic avian insectivore species coexist locally near Manaus, Brazil, and Caribbean lowlands, Costa Rica, respectively (Sherry et al., 2020). Diffuse competition in such species-rich communities often involves distantly related predators, which besides diverse birds includes mammals, frogs, snakes, and arthropods. One example is the birds that eat katydids by day, and that have likely selected for many of these same insects to be active at night, increasing (enhancing) their vulnerability and availability to crepuscular and nocturnal birds—and bats. Considering such insects as partitioned resources misses the important evolutionary consequences of these complex, mutually beneficial predator–prey relationships—i.e., enhancement. These species are not so much competing with each other for resources as they are jointly depressing resources, and competing for substrates, or for other prey-behavior traits to exploit—and outcompete most other species simultaneously—via specialized and thus efficient foraging traits.
Increasing recent attention to the evolution of species resource-exploitation traits reinforces the possibility that species do not always diverge in response to interspecific competition for prey. For example, McPeek et al. (2022) show with models of character displacement and trait evolution that competing species do not always diverge: Communities can comprise nested subsets of species when unidirectional selection is involved, as in predator-prey arms races. Species’ evolutionary divergence may also be impeded by phylogenetic niche conservatism (e.g., Mitelbach and McGill 2019).
3.4 Is there a “limiting similarity” of coexisting species?
Gause’s review of many experimental lab and field studies, in which one species tends to outcompete and drive an ecologically similar species to extinction, led to the generalization that species are less likely to coexist the more similar they are ecologically. Competition thus potentially destabilizes species interactions by disallowing too similar species to coexist. This need for some minimal ecological difference between competing species begged the question of how much difference? This question motivated the idea of a “limiting similarity”, some minimum resource-utilization or niche difference necessary for coexistence (Macarthur and Levins, 1967; MacArthur, 1972; Abrams, 1983). Subsequent modeling (e.g., Slatkin, 1980; Taper and Case, 1985) and abundant empirical studies of such character displacement have failed to find a consistent pattern of displacement, depending for example on whether coevolutionary or community assembly processes prevailed. The limiting similarity concept is largely abandoned now by community ecologists for a variety of reasons (e.g., Terborgh, 2015; Mittelbach and McGill, 2019; but see McPeek, 2017; McPeek, 2019).
The BCH additionally critiques the limiting similarity concept insofar as no single globally applicable limiting similarity exists. This is because regional, e.g., continental species diversity drives species to specialize on different prey traits (see Section 3.2). As more and more species accumulate, exemplified by mainland Neotropical areas, differences between competing species likely decrease. Species are likely to parse prey categories and especially foraging substrates more and more finely, the greater the species richness, e.g., in Neotropics compared to Nearctic latitudes.
The BCH also argues that the limiting similarity idea is untestable because the trophic specializations of species such as Neotropical insectivorous birds do not form continuous variables, whose differences defy any simple quantitative test. For example, what is meant by the ecological distance, or limiting similarity, between suspended dead leaves and large leaf undersides as arthropod hiding places to which different Neotropical insectivores have become adapted? When competition drives such prey trait specializations, and these are essential components of species’ trophic niches, then competition cannot cause partitioning of limiting resources that are used up in the process of exploitation. Foraging substrates are not resources and they are not used up, so it is nonsensical to equate foraging specializations of insectivorous tropical birds with partitionable resources.
3.5 What’s a resource?
Interspecific competition invokes resources that limit the growth of populations, which by any reasonable definition are used up in the consumption process. Food is an obvious resource, and the wherewithal for reproduction such as hosts exploited by parasites or nesting sites that are in short supply relative to demand are also resources. Nest holes, and nest boxes put out by humans, are examples of the latter, and are used up temporarily insofar as individuals can preempt them. Concepts of competition and coexistence warrant careful attention to the nature of resources and how they are measured. Terrestrial birds’ typical resources include animal proteins, such as insects and other invertebrates, live or dead animals eaten by raptors and vultures, and diverse plant products like fruit, nectar, and seeds (Sherry, 2016). Resources for plants include soil nitrogen and phosphorus, water, and sunlight, which latter can be used up by plants shading each other. All these resources are at least potentially used up in proportion to exploitation intensity, making resource exploitation density-dependent. Depletable resources are an important category of limiting factor, and thus figure importantly in models developed to understand ecological communities (McPeek, 2022). Many of McPeek’s models use saturating (resource-exploitation) functional responses, which assume density-dependence.
Morphology and foraging behavior serve frequently as resource surrogates. These traits are often at least partially heritable, providing an arguably superior way to look at resources because of the averaging long-term effect of natural selection in fluctuating environments. Insectivorous birds often feed on insects too small to identify at a distance, tempting one to use surrogates; and too few studies have linked heritable traits to diet (Rosamond et al., 2020; see also Schumm et al., 2020). One problem with such eco-morphological surrogates is that different predator species can eat many of the same prey—and thus potentially compete—using different foraging behaviors, and correspondingly, sometimes distinctive morphological (and other phenotypic) traits. One example: birds that forage for katydids and other orthoptera in tropical forests by hitching up tree trunks as treecreepers (e.g., Powell et al., 2022) or by antbirds (Thamnophilidae) exploiting many of the same prey with different morphological traits adapted to grasp small, vertical stems at the ant swarm front (Zimmer and Isler, 2003). Another example: Epinecrophylla antwrens search for diverse arthropods concealed in suspended dead leaves, while other birds such as Myiobius flycatchers follow these antwren mixed-species flocks and pursue many of the same arthropods fleeing the antwrens (Sherry, 1984). This situation of divergent foraging behaviors exploiting similar prey, is widespread in nature—the “many-to-one” phenomenon in biomechanics (Wainwright et al., 2005)—and weakens one-to-one correspondence between the actual resources and the morphological surrogate. A related problem with surrogates involves different predators adapted to feed on the same prey species—and depress its abundance—using distinctive phenotypic traits for different prey life-history stages, such as predators on adult butterflies or moths versus eggs, larvae (caterpillars), or pupae.
The single biggest challenge with foraging behavior as a surrogate for resources is that it often, and maybe generally, fails to measure, or correspond with, actual resources. Foraging behavior often underestimates actual resource overlap. Almost no studies have measured foraging overlap (i.e., similarity) among coexisting species and diet overlap simultaneously, but a few studies that have measured both reinforce this discrepancy. Kent et al. (2022) found dietary overlaps to be surprisingly and consistently high despite evidence for interspecific competition; and Kent and Sherry (2020) tested the relationship of foraging and diet directly with five warbler species wintering in Jamaican wet limestone forests—the island’s rainforest equivalent—and found the greatest difference among the five species, almost zero overlap, in their foraging substrates. Diet overlap, by contrast, was generally high, due to all five species eating the same three species of ants, the same beetle species, and bark lice (insect order Psocoptera). In the case of the ants consumed, birds feeding on different substrates probably ate the same ant species that moved around among different substrates. In a study more appropriate to the species-rich mainland Neotropics, Rosenberg (1997) documented high dietary overlap among coexisting dead-leaf foraging species despite distinctive differences in foraging height, size and type of leaves searched, and prey size, among other traits. If the only data available were foraging substrates—as has been the case in so many niche studies going back to MacArthur’s (1958) classic study of spruce woods warblers—one could conclude erroneously that coexisting species partitioned “resources”. Again, the problem with this interpretation is that foraging substrates are not resources, and thus not the target of competition, as described below.
Another problem with foraging behavior as a surrogate for resources is that the former entails many different component behaviors, with no clear way to combine them into one estimate of resources overall. This complexity of foraging behaviors arose in our study of coexisting warblers in Jamaica (Kent and Sherry, 2020), in which we simultaneously quantified the substrates where the birds attacked (or captured) prey, substrates from which birds launched an attack such as gleaning from a leaf or twig while perched versus pursuing prey in the airspace, height above ground. MacArthur (1958) quantified rate of movement through the environment as a measure of searching strategy or intensity, and how individuals moved through the substrates such as vertically, horizontally, or tangentially inside or outside the vegetation. However, he did not try to combine them. On the other hand, Cody (1974) devised multiple ways to combine foraging behaviors—none entirely satisfactory. Use of foraging behavior becomes challenging without compelling ways to weight different foraging components and link them to resources.
The BCH addresses this confusion surrounding the nature of resources by explicitly distinguishing the things that are consumed—the actual resources like insects—from where and how these are procured using foraging and morphological adaptations to exploit substrates and prey traits (Table 1). A large number of avian studies have integrated foraging behavior, morphology, and diet (e.g., Sherry, 2016), but very few tropical insectivorous bird studies have included dietary data necessary to interpret foraging and morphological data, let alone to assess dietary stereotypy as a measure of dietary specialization relevant to the BCH. Insectivore diets are particularly challenging, although new methods are increasing feasibility (next paragraph). Additionally, over evolutionary time, particularly in the tropics, the resources themselves have evolved and coevolved in response to consumption. Over the tens of millions of years permitted by Neotropical avian adaptive radiations, co-evolution of predators with prey become paramount to understanding resources as something more than hypothetical hypervolume niche axes (Figure 1). Coevolution of herbivores with plants may provide similarly important arms races, but are beyond the scope of this paper.
In the process of formulating the BCH, Sherry et al. (2020) reviewed scant information about diets of Neotropical insectivorous birds, information that proved pivotal. New technologies increasingly augment diet data non-invasively, e.g., by identifying the source of gut DNA (e.g., Kartzinel et al., 2015; De Sousa et al., 2019; Hoenig et al., 2021). A variety of animal studies has used these and other methods to document widespread similarity, i.e., overlap in diets, often linked to opportunism (e.g., De León et al, 2014; Owen-Smith et al., 2017; Golcher-Benavides and Wagner, 2019; Gordon et al., 2019; Kent et al., 2022; but see Kartzinel et al., 2015). Despite the growing accessibility and application of these methods, they have not been applied widely—where they would prove invaluable—in hyperdiverse tropical communities, such as the Neotropical insectivores emphasized here.
Reframing resource surrogates such as morphological or foraging niches meaningfully is challenging. Simply using morphological and/or foraging traits in a Principal Components Analysis, or other multivariate statistical procedure designed to identify species similarities or differences, without knowledge of how these traits impact actual resource exploitation, is more descriptive than insightful about mechanisms. It would be better to consider the niche axes of tropical insectivorous birds as species traits related to anti-predator adaptations rather than as species abundances, insofar as predators’ diets often diverge based on prey anti-predator traits and behaviors. Niche studies thus need to identify the traits making the prey susceptible to different predator taxa: Different traits (such as prey size, resting substrates, evasive behaviors—Table 1) make prey more-or-less vulnerable to different predators. Understanding how predator traits filter particular prey is critical for any trophic-dynamic (food web) modeling, which requires knowledge of relevant prey resources, predatory behaviors and traits, and prey resource intake rates. This is because of trait-mediated indirect effects on demography (e.g., McPeek, 2022).
3.6 Bottom-up or top-down?
Trophic dynamic considerations are important to niche concepts both higher and lower in trophic level than the particular species of concern, such as tropical insectivorous birds here. Bottom-up ecological control of populations and communities, disproportionately important to classical niche concepts such as Eltonian, focuses on resources and competition for resources, emphasizing food available to consumers (and nutrients to plants) as primary determinants of population demography. Top-down control by predators and parasites is increasingly considered a critical limiting factor. Both are important in most communities, although relative strengths likely vary.
Terborgh (2015) critiqued niche theories restricted to bottom-up processes, and provided a compelling case for the importance of top-down ecological forces to community structure, particularly in the tropics (Feeley and Terborgh, 2008). Accordingly, most populations in nature are predator-regulated, generally restricting densities and weakening interspecific competition. Terborgh (2015) also emphasized the role of natural enemies maintaining plant coexistence and diversity via the Janzen-Connell phenomenon (e.g., Lebrija-Trejos et al., 2023). This effect involves specialized natural enemies of plants, including fungi and herbivores on seeds and leaves, preventing plants from growing too close to each other, thus opening space for different plant species (with different natural enemies) to coexist. Terborgh (2015) recommended a model including predation and competition interacting to regulate species richness, promoting the greatest richness at intermediate levels of predation, a model with the virtue of synthesizing multiple ecological theories. The greatest shortcomings of Terborgh’s (2015) proposal are minimization of the role of bottom-up ecological processes and omission of evolutionary explanations of the LDG (“The role of evolution in producing diversity is not under consideration here,” p. 11,415). Terborgh’s approach thus overlooks evolutionary arms races informing the BCH, ignores the evolution of specializations in natural enemies necessary for the Janzen-Connell effect, and also overlooks diffuse competition acting evolutionarily to contribute to high alpha diversity given enough evolutionary time.
Both ecological and evolutionary predator–prey interactions (and natural enemies generally) cannot be emphasized enough, which indicates a weakness of the BCH, namely insufficient attention to effects of indirect predation impacts in both higher and lower trophic levels than the insectivorous birds that provide the focus of the theory. Predation on adult tropical birds may be relatively rare, and likely poorly documented, but predation on birds’ nest contents and fledglings is omnipresent, and critical demographically (e.g., Visco and Sherry, 2015; Visco et al., 2015). Predation involving avian nests and immature life-history stages remains poorly integrated into tropical avian coexistence mechanisms. Avian arms races with their arthropod prey also provides opportunities for the diversification of these arthropods: For example, the very arthropod refugia that birds exploit (Table 1) provide opportunities for the arthropods to themselves become more specialized evolutionarily, which likely contributes to their speciation and coexistence—an important topic beyond the scope of this paper. McPeek (2022) explicitly models these indirect coexistence mechanisms ecologically, and discusses their importance to community structure generally: Diversity at one trophic level begets diversity at another.
3.7 Do species interact pair-wise?
Niche theory often specifies that species compete, or more generally interact ecologically (including predator–prey, mutualism, and other interaction types), pair-wise, implicit in interaction coefficients, such as α1,2 = the competition coefficient in the Lotka-Volterra interspecific competition model, in this case species 2 competing against 1. This assumption is also explicit in the theory of the community matrix, which is a matrix of every possible interaction between pairs of species plus interactions of individuals within a species as diagonal elements of the matrix. This dyadic assumption may have been reinforced by an idea going back at least to Darwin’s On the Origin of Species, namely that speciation tends to be a bifurcating process, resulting in two derivative species; and that competition is likely strongest between the most closely related, and thus most recently derived species. Competition may or may not be strongest between the most closely related species, but this does not preclude the potentially powerful force of all the other competitors vying for the same limited resources—i.e., diffuse competition.
This dyadic assumption is so commonplace and subtle as arguably to go largely unrecognized as such. When considered explicitly the context is generally testable quantitative predictions. Its mathematical convenience is to allow tracking all potential trophic impacts of individual species on each other. One can consider the impact of every species on the population growth rate of every other species, using appropriate coefficients of interaction, e.g., negative for competition, positive and negative for predator–prey interactions, and positive for mutualism. Such interactions can also vary in strength from zero (for species that do not interact at all) to strong, as strong as or stronger than the competitive interactions involving individuals of the same species. One can then analyze the outcomes and stability of the networks of species interactions, including predicting impacts of removing or adding species to the mix (e.g., McPeek, 2017; McPeek, 2022). This approach extrapolates theoretically to high species richness, such as many tropical communities, but such extrapolation needs testing empirically (McPeek, 2022). Although mathematically tractable, extrapolation to communities such as mainland Neotropical forests remains challenging because many, and in some taxa most, species are not even described yet, let alone studied enough to calculate species-interaction coefficients and other parameters necessary for meaningful models.
Another facet of this dyadic assumption is the conceptualization of species arrayed along one or more niche resource axes, in which species interact primarily with adjacent species along these axes—again, pair-wise. This has also proven mathematically convenient, but dyadic species interactions obscure an important aspect of diffuse competition involving many simultaneous competitors. Dyadic species interactions emphasize those within a trophic level, especially interspecific competition, whereas diffuse competition emphasizes aggregate depression of resources necessitating specializations such as seen frequently in tropical insectivorous birds (Figure 1). The BCH builds on diffuse species interactions, which are more likely the more species that coexist. Coexisting tropical species appear not to interact with each other individually, in part because they rarely coexist with close relatives (Sherry et al., 2020), but rather interact indirectly with many other species simultaneously and anonymously. This in turn recognizes evolutionary interactions with prey and their traits, i.e., species at a lower trophic level, exemplified by enhancement (Table 1). Tropical insectivorous species thus appear not to “recognize” many other species as competitors (but see Robinson and Terborgh, 1995).
3.8 Is dispersal the cause or result of community structure?
Dispersal profoundly impacts niches. Limitations to dispersal explain Provincial (regional) biotae and limited species pools contributing to local diversity—i.e., biogeographical realms. Additionally, all species disperse, for example to colonize new sites, escape competition from a parent, or avoid inbreeding. Dispersal is critical to understand population dynamics via immigration and emigration, and life-histories. Invasion, the ultimate source of new species, is a kind of dispersal critical to community ecological concepts of coexistence. Repeated colonizations can establish a species outside its previous geographic (or ecological) range—a kind of supply-side ecology.
Dispersal also contributes new species indirectly via speciation, exemplified by island archipelagos providing the opportunities for isolating dispersing populations like the Darwin’s finches in the Galapagos Islands and the Hawaiian Honeycreepers, although speciation can also occur without dispersal, e.g., via vicariance as illustrated by impacts of the Andes Mountains uplift. The Dynamic Theory of Island Biogeography (MacArthur and Wilson, 1967) elevates dispersal to one of two iconic processes—the other being extirpation—determining equilibrial species richness whenever these two processes are equal. However, equilibration of immigration and extinction on islands may take thousands of years, pushing islands out of equilibrium (Ricklefs and Birmingham, 2001), e.g., in the “land bridge” islands of Trinidad and Tobago that inherited South American species prior to their isolation by post-Pleistocene sea level rise. Williamson (1981) formalized this idea of some islands being out of dynamic equilibrium ecologically by distinguishing oceanic islands, in which speciation and adaptive radiation and local adaptation are relatively more prominent processes introducing and adapting species, from “continental islands”, dominated by the dispersal and extirpation (ecological scale) processes emphasized by MacArthur and Wilson. This acknowledgement of evolutionary scale phenomena, on islands in this context, reinforces this paper’s argument for greater attention to evolutionary scale niche properties and phenomena.
Hubbell (2001) widely cited, if controversial, Unified Neutral Theory of Biodiversity and Biogeography (UNT) builds on the Dynamic Theory of Island Biogeography to explain the extraordinary diversity of tropical tree species. Hubbell’s theory is notable by incorporating evolutionary scale processes (speciation, and colonization of local communities from regional species pools) and generating models that replicate widespread empirical patterns. Hubbell’s theory also assumes identical demographic parameters for species—hence neutrality and stochastic colonization and extirpation subject to regulation of total tree density—thereby obviating the need for niche differences. Besides relaxing the need for niche differences for coexistence, the UNT relaxes the need for life-history tradeoffs (e.g., between dispersal and other traits), unlike most life-history theories (Mittelbach and McGill, 2019). It is thus incompatible with well known natural history described in the context of the BCH. The BCH also assumes a tradeoff between competitive and dispersal adaptations, the former helping species compete in hyperdiverse tropical communities. See Chase and Leibold (2003), Kricher (2011), and Mittelbach and McGill (2019) for other critiques of UNT.
Many tropical insectivorous birds have evolutionarily increased foraging specialization at the expense of dispersal compared to many other birds (Salisbury et al., 2012; Sherry et al., 2020), linked to relatively rounded and inefficient wing morphology (Claramunt et al., 2022; Naka et al., 2022); and thus their entry into new communities via dispersal is probably relatively slow. However, dispersal certainly occurs in tropical birds, however slowly; is probably maintained or increased with climate changes in the tropics, including alternating wet and dry periods that have probably also stimulated major movements of birds within the tropics; and likely varies with tropical ecological circumstances including habitat stability, territoriality, and diet (e.g., Sheard et al., 2020; Sherry et al., 2020; Miller et al., 2021; Johnson et al., 2023). The BCH thus emphasizes that dispersal ability itself evolves, at least partly as a function of diffuse competition selecting for exploitation of particular substrates or prey traits (Figure 1; Sherry et al., 2020). Accordingly, efficiency in some aspects of specialized flight performance, such as prey-attack speed and maneuverability, has been favored at the expense of sustained flight capacity needed for dispersal; and dispersal capacity has been sacrificed evolutionarily to conserve energy in competitor-rich environments with well defended prey. Dispersal is thus both the product and determinant of coexistence, species richness (and interactions), and niche traits—meriting far more study in this context.
Approaches to community structure increasingly add speciation and adaptive radiation as an alternative to dispersal to augment species diversity in communities (e.g., Pigot and Tobias, 2013; Hughes et al., 2022). Speciation automatically adds new species to geographic areas such as South America, although avian sister species do not initially coexist locally because of predominantly allopatric speciation. The BCH acknowledges speciation rate exceeding extinction rate as a sufficient condition adding new species to the environment, but not for local coexistence. Coexistence necessitates sister species persisting, then expanding via dispersal to overlap, and diverging sufficiently for coexistence either before (Pigot and Tobias, 2013) or after secondary contact/sympatry (Tobias et al., 2014; Anderson and Weir, 2022). Thus, the invasibility criterion for species to enrich local communities holds even with adaptive radiation, but the time scale is far greater from an evolutionary perspective.
3.9 How do both ecological and evolutionary processes contribute to tropical diversity?
Ecological/equilibrium processes and evolutionary/non-equilibrium processes structuring communities are sometimes treated as alternatives (e.g., Schumm et al., 2020), but both are important and likely interact, blurring the distinction (Pigot et al., 2018). The question remains: How do species interact with each other and the environment over a range of time-scales to assemble communities of diverse sizes and characteristics. I have emphasized evolutionary species interactions here because they are sometimes overlooked or taken for granted by ecologists—who tend to focus, pragmatically, on short time-scale phenomena, interpretations, and methods most amenable to experimentation. Predator–prey, parasite–host, and mutualistic species interactions are studied evolutionarily, more often than is interspecific competition, at least partly for historical reasons (Chase and Leibold, 2003).
As recognized explicitly above, the accumulation of species in the tropics compared to higher latitudes is associated with evolutionary feeding specializations, and these specializations involve traits relevant to exploit specific prey behaviors and substrates (Salisbury et al., 2012; Pigot et al., 2016; Hughes et al., 2022; Johnson et al., 2023). The BCH explains these traits mechanistically as the outcome of both ecological species interactions (interspecific competition, predator–prey interactions) and evolutionary processes including the adaptations implicit in coevolution and arms races, the processes (speciation and extinction) causing the accumulation of species, and evolutionary enhancements in which species evolve to exploit the anti-predator behaviors of prey. The extraordinary array of body shape adaptations for foraging—involving wings, tails, beaks, tarsi, facial bristles in birds—must have necessitated major genetic changes, and not just single gene substitutions. Recognizing the magnitude of these genetic changes necessitates recognizing long evolutionary periods for predators and prey to evolve in response to each other, i.e., for arms races to play out. Moreover, diffuse competition such as appears to be particularly important in the Neotropics, involving up to hundreds of species of tropical insectivores vying for many of the same prey, is the result of the accumulation of multiple adaptive radiations.
Early field studies of interspecific competition emphasized experimental tests (e.g., Connell, 1980). Wiens (1977, p. 596) asserted that “ingeniously designed manipulative experiments … should be central to studies of competition”. This once widespread approach ignores genetic effects and consequences, thus constituting an ecological rather than evolutionary approach. Resource partitioning is also sometimes described in behavioral contexts, independently of potential genetic constraints. A strictly ecological approach to competition takes resources as a given, whereas the evolutionary approach advocated here recognizes both predators and prey evolving in response to each other.
Some ecologists will object to characterizing most approaches to competition as short-term and local scale, by claiming, correctly, that character displacement is a quintessentially evolutionary process resolving interspecific competition. Character displacement is indeed well documented as a mechanism for phenotypic traits of competing species to diverge directly from each other in response to competition between them. Typically, it has been documented in pairs of closely related species, although multiple-species extensions (Dayan and Simberloff, 2005; Roth-Monzon et al., 2020; Anderson and Weir, 2021) are also provided as evidence of competition in more species-rich communities. Character displacement has also been invoked to understand multiple-species, mainland tropical insectivore communities (e.g., Tobias, 2022). However, character displacement may fail to extrapolate to species-rich communities including the mainland Neotropics, for two reasons. First, a preponderance of local species interactions in the tropics (competitive, predator–prey, parasite–host, and even mutualistic) do not involve close relatives such as are most likely to undergo character displacement. Any simple phenotypic shift, such as beak size in Darwin’s finches or body size/shape in Anolis lizards (Losos 2009), will likely be resisted in the most species-rich mainland communities due to the complexity of how each species interacts there with myriad prey, predators, etc.—an idea referred to as evolutionary gridlock, first proposed by Vermeij (1994). Second, much of the competition shaping the ways species coexist in species-rich communities is diffuse, as argued above, not directly in relation to any other one (or few) closely related species, and thus highly indirect. The BCH invokes ways of species interacting evolutionarily, above and beyond character displacement, which is thus not the only way tropical species respond to competition.
3.10 Does tropical diversity depend on community invasibility?
An important theoretical approach replacing many of the niche concepts discussed above is community invasibility, i.e., assembly (reviewed by McPeek, 2017; Mittelbach and McGill, 2019; McPeek, 2022). Accordingly, the critical question relevant to species coexistence is what kinds and number of species can invade an existing community. McPeek addresses this question using simple ecological modules, and similarly tested empirically in simple communities, that ask how different species must be to coexist. McPeek (2022) defines a module as a network configuration of pair-wise species interactions, and builds mathematical model communities of food webs primarily using coupled differential equations, and starting with the simplest possible community of a species consuming a resource such as a nutrient in the case of photosynthesizers. He then systematically adds additional resources, consumers, predators (and later in the book neutral species, parasites, etc.) so as to model processes of consumption, feeding saturation, predation, intraguild predation, competition, apparent competition, mutualism and facilitation, pathogenesis, keystone predation, and omnivory vs. specialization. He also addresses environmental heterogeneity—both temporal and spatial variation—dispersal such as in metacommunities, neutral species, migrants (which can coinhabit if not coexist). McPeek clearly intends to explain global diversity of natural communities insofar as he motivates most chapters with some of the most complex natural communities, including tropical assemblages. This body of theory provides a compelling, even parsimonious explanation for the diversity we see in nature.
A variety of similar predictions from this body of community ecology theory and the BCH are fascinating. For example, both approaches include a prominent, and certainly not exclusive, role for interspecific competition. McPeek (2022) explicitly considers diffuse competition by adding a variety of species within a particular trophic level, much as the BCH emphasizes diffuse competition in the species-rich tropical communities considered. Both McPeek’s ecological approach and the BCH explicitly consider a variety of indirect species interactions as crucial to understand coexistence, the idea that diversity begets diversity. Species traits, and not just species abundances, figure in species coexistence in both the ecological and evolutionary approaches: Ecologically, predator feeding rates are impacted by different prey via saturating functional responses, and evolutionarily both predators and prey select for traits in the other trophic level that influence where and how species feed or hide/defend themselves.
Despite these parallels, community ecology based on mathematical models of invasibility cannot explain the diversity and adaptations seen empirically in tropical communities, let alone the geographically and geologically distinctive Neotropics. The BCH, along with many approaches today explicitly invoke evolution: Whereas invasibility theory addresses how species may coexist generally, evolutionary approaches address adaptations necessary for coexistence in increasingly diverse (e.g., tropical) communities. McPeek (2022) sees ecological interactions driving species richness whereas the BCH envisions species richness contributing to driving species interactions, as considered above. Whereas invasibility theory is based on trophic interactions necessary to model species population growth when rare, arms races implicit in evolutionary approaches (and in the BCH) emphasize the traits predators use to forage for food (and reproduce via nesting traits in birds, etc.) and the traits prey use to defend themselves such as the substrate hiding places. Whereas community ecology can explain coexistence in local communities, the number and types of species in regional communities—from which local communities draw—are the result of speciation and extinction processes (e.g., Figure 1), as widely recognized. As a variety of ecologists and evolutionary biologists have emphasized, mechanisms of species proliferation and coexistence vary with spatial and temporal scale, and both are necessary. However, evolutionary mechanisms and processes are necessary to understand communities above and beyond what community ecologists like McPeek (2022) have modeled mathematically.
4 Synthesis: latitudinal gradient of niche concepts
With distance from equatorial lowlands, both latitudinally and elevationally, the physical environment, especially temperature and water availability, becomes more limiting to plant productivity, which in turn determines energy available for all other organisms in local ecosystems. Conversely, organisms’ ability to interact becomes increasingly significant in the most species-rich, largely aseasonal, equatorial environments. The BCH simply highlights and illustrates the importance of these latter constraints for insectivorous birds, especially interspecific competition for food resources and predator–prey arms races. This well known pattern in which biological species interactions intensify towards the equator (Dobzhansky, 1950; MacArthur, 1969; Schemske et al., 2009) has more to teach us about niches.
One inference from the BCH is that the “Latitudinal Diversity Gradient” is far more than simply a gradient of species richness, or even of intensification of ecological species interactions: It entails vast differences in the ages, impact of evolutionary history and geography, and complexity and intricacy of biotic species interactions such as the arms races involving tropical insectivorous birds and their prey. Dispersal capacity is markedly reduced in a variety of resident tropical birds (references in Section 3.8), and the BCH proposes a mechanism, namely the tradeoff between foraging specializations and flight capacity (Figure 1). Increasing application of phylogenetic tools is revealing the ages of some specialized tropical taxa and traits (e.g., Tobias et al., 2014; Hughes et al., 2021; Jarzyna et al., 2021). The LDG is not even strictly latitudinal, insofar as geography has impacted tropical speciation and extinction rates differentially among continents, latitudes, and elevations. Empirical documentation of ecological specializations for feeding illustrated in Neotropical insectivorous birds (Table 1) emphasizes the importance of environmental stability that has allowed speciation rate to exceed extinction rate for varying periods of time (Fine, 2015), and thus selected for these specializations to arise and persist. Thus, global species richness gradients such as latitudinal and elevational are both a cause and consequence of niche evolution.
Recognizing this reciprocity, that species diversity shapes niches and vice versa, has important implications for spatial and temporal niche scales. For example, the BCH accepts that Neotropical diversity requires understanding the impacts of time since the mass extinction of most dinosaurs 66 million years ago, the geography of the Neotropics that has contributed to speciation across mountain ranges and large rivers, and relative environmental stability of the Neotropics, all contributing to its species accumulation (Mittelbach et al., 2007; Fine, 2015). Simultaneously, local community composition and coexistence result disproportionately from contemporary ecological interactions (e.g., Ricklefs and Schluter, 1993) and community assembly (McPeek, 2022). Relatively reduced dispersal capacity of many tropical birds (Section 3.8) also emphasizes the local spatial scales important to tropical community assembly, species coexistence, and extinction.
Scale also informs how we study niches. At the largest scales, global patterns such as convergence identify global evolutionary constraints (e.g., Pigot et al., 2020), but a disadvantage is relying on abstract niche surrogates like a handful of morphological traits with ambiguous trait interpretation (Section 3.5). The BCH by contrast restricts its avian subset to predators on arthropods, leading to mechanistic inferences arising from integrating physiological, morphological, foraging (including substrates), and dietary data. Sacrificing breadth of study scope for more detailed investigations of the intricacies of tropical species interactions, i.e., moving down the ladder of abstraction, promises future niche insights.
Niche concepts need to better integrate evolution, which may seem obvious and certainly not new here. Organisms have to be adapted to local conditions to persist, both to a harsh physical environment at high latitudes, elevations, and deserts, on the one hand; and to diverse species and their interactions in the tropics. However, some niche concepts do not explicitly recognize important consequences of this idea, even some relatively recent proposals (e.g., Peñuelas et al., 2019) that fail to address such issues as the intricate arms races particularly prominent in the tropics. Predator–prey and other potentially indirect (and evolutionary) species interactions better explain Hutchinson’s paradox of the plankton than simple resource variability (McPeek, 2022).
Species interactions at high elevations (at least in the Andes Mts.; Hughes et al., 2022) are biased towards closer relatives phylogenetically, and the same may apply at high latitudes, if only because of a smaller species pool to colonize newly available habitats and Pleistocene events contributing directly to recent speciation by episodically fragmenting geographic ranges. Dispersal ability over long distances is also at a premium for high-latitude and migratory species (Sheard et al., 2020), facilitating opportunistic colonization of recently available, and fluctuating environments. Studies of species richness gradients, such as the LDG, will benefit from more integration of these diverse evolutionary processes and correlates, contrasting adaptations to the physical versus biological environment, and phylogenetic perspectives.
Tropical niches are not just about more species, or even statistically more species interactions, which increases as a function of number of species squared: N × (N − 1)/2. Tropical niches resulting from co-evolutionary arms races, such as illustrated by Neotropical birds and arthropods, are much more than this: They are the result of intense competition among predators, selection for increasingly effective ways to forage among the predators, increasingly effective defenses among the prey, and feedbacks on both speciation and extinction rates (Figure 1). These feedbacks, involving evolutionary species interactions, are not easily captured in traditional niche models. Interspecific competition is as much an evolutionary phenomenon, in the variety of manifestations and impacts, as predator–prey, parasite–host, plant–herbivore, and mutualistic interactions, and needs increasingly to be tested with evolutionary tools. The BCH is limited to date by illustrating these aspects of tropical niches with birds and insects. Testing the BCH is itself limited by the availability of relevant data, especially dietary data and functional linkages to morphology, behavior, and physiology. The ten questions in Section 3 are largely unanswerable presently without more such data on the natural history of diets, an important grand challenge for ornithologists and others (Robinson, 2022).
5 Conservation implications
According to the BCH, tropical resources are effectively scarce and hard to acquire by birds, due to both intense diffuse exploitation competition and defenses evolved by the prey involved in deep-time arms races. Scarce and well defended resources select for specialized foraging, especially in relation to particular substrates or prey-escape behaviors; and also select for conservative metabolic rates as a way to eke out a living. Specializations by predators on arthropod prey taxa, prey behaviors, and prey substrates all make these predators relatively sensitive to any changes in their prey abundances and traits, changes that humans are bringing about everywhere, but particularly acute in the tropics (e.g., Şekercioğlu et al., 2002; Stouffer et al., 2020; Curtis et al., 2021; Sherry, 2021). Arms races are certainly not limited to the tropics, nor are human global change impacts, but specializations of tropical organisms make them particularly sensitive to changing environments.
The effective scarcity of tropical resources from insectivorous birds’ perspective is hypothesized, according to the BCH, to have contributed to a positive feedback loop on speciation rates, contributing to the Latitudinal Diversity Gradient observed today (Figure 1). This positive feedback loop is mediated through relatively poor dispersal capacity in these birds, the cost (tradeoff) of the low metabolic rate plus wings and tails specialized for foraging efficiency at the cost of flight efficiency. Poor dispersal capacity in these tropical birds is a severe handicap in human-fragmented tropical landscapes, contributing to widespread declines in tropical insectivorous birds (Sherry, 2021; Claramunt et al., 2022). The rampant habitat loss of tropical forests inevitably fragments them; and agricultural pesticides and other human activities further degrade what habitats remain (Visco et al., 2015). These birds are not just vulnerable to declining prey abundance and an inability to move among fragments, but also to changes in feeding opportunities and substrates to which these birds are adapted to feed, such as declining army ant abundance that flush what prey remain, degradation of tropical mixed-species flocks (e.g., Rutt et al., 2020) that provide feeding opportunities and safety, and direct (physiological) and indirect impacts of warming and drying climate (e.g., Curtis et al., 2021).
One last deduction from the BCH is important to understand threats to tropical insectivorous birds. Specialization on particular arthropod prey defenses often has the consequence of small population sizes of these tropical predators. More species, everything else the same, similarly leaves smaller average population densities per unit area, and thinly spread populations—notorious in tropical rainforests (e.g., Robinson et al., 2000)—providing another risk factor for these birds, contributing to the positive feedback on extirpation and extinction rates (Figure 1). It is no wonder tropical insectivorous birds are declining precipitously (Sherry, 2021).
Author contributions
The author confirms being the sole contributor of this work and has approved it for publication.
Acknowledgments
I thank the editors of this Research Topic for the invitation to participate, and both formal reviewers for their abundantly invaluable and constructive feedback. Mark McPeek made substantial constructive criticisms and suggestions on an earlier draft of the manuscript. Any errors of omission or commission are strictly those of the author. Tulane University (Department of Ecology and Evolutionary Biology) and Dartmouth College provided support of the research and writing of this manuscript. Many ideas for this manuscript arose while the author held a Charles Bullard Fellowship supporting his research at the Harvard Forest. An NSF OPUS grant (award number 2147043) provided most financial support while writing this manuscript.
Conflict of interest
The author declares that the research was conducted in the absence of any commercial or financial relationships that could be construed as a potential conflict of interest.
Publisher’s note
All claims expressed in this article are solely those of the authors and do not necessarily represent those of their affiliated organizations, or those of the publisher, the editors and the reviewers. Any product that may be evaluated in this article, or claim that may be made by its manufacturer, is not guaranteed or endorsed by the publisher.
References
Abrams P. (1983). The theory of limiting similarity. Ann. Rev. Ecol. Syst. 14, 359–376. doi: 10.1146/annurev.es.14.110183.002043
Anderson S. A. S., Weir J. T. (2021). Character displacement drives trait divergence in a continental fauna. Proc. Natl. Acad. Sci. USA 118, 1–8. doi: 10.1073/pnas.2021209118
Anderson S. A. S., Weir J. T. (2022). The role of divergent ecological adaptation during allopatric speciation in vertebrates. Science 378, 1214–1218. doi: 10.1126/science.abo7719
Antonelli A., Zizka A., Carvalho F. A., Scharn R., Bacon C. D., Silvestro D., et al. (2018). Amazonia is the primary source of Neotropical biodiversity. Proc. Natl. Acad. Sci. USA 115, 6034–6039. doi: 10.1073/pnas.1713819115
Askins R. A. (1983). Foraging ecology of temperate zone and tropical woodpeckers. Ecology 64, 945–956. doi: 10.2307/1937215
Blonder B. (2018). Review and synthesis: hypervolume concepts in niche- and trait-based ecology. Ecography 41, 1441–1455. doi: 10.1111/ecog.03187
Charnov E., Orians G. H., Hyatt K. (1976). Ecological implications of resource depression. Am. Nat. 110, 247–259. doi: 10.1086/283062
Chase J. M., Leibold M. A. (2003). Ecological niches: linking classical and contemporary approaches (Chicago, IL: University of Chicago Press).
Claramunt S., Hong M., Bravo A. (2022). The effect of flight efficiency on gap-crossing ability in Amazonian forest birds. BioTropica 54, 860–868. doi: 10.1111/btp.13109
Cody M. L. (1974). Competition and the structure of bird communities (Princeton NJ: Princeton University Press).
Connell J. H. (1980). Diversity and the coevolution of competitors, or the ghost of competition past. Oikos 35, 131–138. doi: 10.2307/3544421
Curtis J. R., Robinson W. D., Rompré G., Moore R. P., McCune B. (2021). Erosion of tropical bird diversity over a century is influenced by abundance, diet and subtle climatic tolerances. Sci. Rep. 21, 1–13. doi: 10.1038/s41598-021-89496-7
Dayan T., Simberloff D. (2005). Ecological and community-wide character displacement: the next generation. Ecol. Letts. 8, 875–894. doi: 10.1111/j.1461-0248.2005.00791.x
De León L. F., Podos J., Gardezi T., Herrel A., Hendry A. P. (2014). Darwin’s finches and their diet niches: sympatric coexistence of imperfect generalists. J. Evol. Biol. 27, 1093–1104. doi: 10.1111/jeb.12383
De Sousa L. L., Silva S. M., Xavier R. (2019). DNA metabarcoding in diet studies: unveiling ecological aspects in aquatic and terrestrial ecosystems. Envir. DNA 1, 199–214. doi: 10.1002/edn3.27
Feeley K. J., Terborgh J. W. (2008). Direct vs. indirect effects of habitat reduction on the loss of avian species from tropical forest fragments. Anim. Conserv. 11, 353–360. doi: 10.1111/j.1469-1795.2008.00182.x
Fenton I. S., Aze T., Farnsworth A., Valdes P., Saupe E. E. (2023). Origination of the modern-style diversity gradient 15 million years ago. Nature 614, 708–712. doi: 10.1038/s41586-023-05712-6
Fine P. V. (2015). Ecological and evolutionary drivers of geographic variation in species diversity. Ann. Rev. Ecol. Evol. Syst. 46, 369–392. doi: 10.1146/annurev-ecolsys-112414-054102
Golcher-Benavides J., Wagner C. E. (2019). Playing out Liem’s paradox: opportunistic piscivory across Lake Tanganyikan cichlids. Am. Nat. 194, 260–267. doi: 10.1086/704169
Gordon R., Ivens S., Ammerman L. K., Fenton M. B., Littlefair J. E., Ratcliffe J. M., et al. (2019). Molecular diet analysis finds an insectivorous bat community dominated by resource sharing despite diverse echolocation and foraging strategies. Ecol. Evol. 93117, 3129. doi: 10.1002/ece3.4896
Harvey M. G., Bravo G. A., Claramunt S., Cuervo A. M., Derryberry G. E., Battilana J., et al. (2020). The evolution of a tropical biodiversity hotspot. Science 370, 1343–1348. doi: 10.1126/science.aaz6970
Hoenig B. D., Snider A. M., Forsman A. M., Hobson K. A., Latta S. C., Miller E. T., et al. (2021). Current methods and future directions in avian diet analysis. Ornithology 139, 1–28. doi: 10.1093/ornithology/ukab077
Holt R. D. (2009). Bringing the Hutchinsonian niche into the 21st century: ecological and evolutionary perspectives. Proc. Natl. Acad. Sci. USA 106, 19659–19665. doi: 10.1073/pnas.0905137106
Hubbell S. P. (2001). The unified neutral theory of biodiversity and biogeography (Princeton, NJ: Princeton University Press).
Hughes E. C., Edwards D. P., Bright J. A., Capp E. J. R., Cooney C. R., Varley Z. K., et al. (2022). Global biogeographic patterns of avian morphological diversity. Ecol. Letts. 25, 598–610. doi: 10.1111/ele.13905
Hutchinson G. E. (1957). Concluding remarks. Cold Spring Harbor Symp. 22, 415–427. doi: 10.1101/SQB.1957.022.01.039
Hutchinson G. E. (1959). Homage to Santa Rosalia or why are there so many kinds of animals? Am. Nat. 93, 145–159. doi: 10.1086/282070
Jarzyna M. A., Quintero I., Jetz W. (2021). Global functional and phylogenetic structure of avian assemblages across elevation and latitude. Ecol. Letts. 24, 196–207. doi: 10.1111/ele.13631
Johnson O., Ribas C. C., Aleixo A., Naka L. N., Harvey M. G., Brumfield R. (2023). Amazonian Birds in more dynamic habitats have less population genetic structure and higher gene flow. T. Molec. Ecol. 32, 2186–2205. doi: 10.1111/mec.16886
Kartzinel T. R., Chen P. A., Coverdale T. C., Erickson D. L., Kress W. J., Kuzmina M. L., et al. (2015). DNA metabarcoding illuminates dietary niche partitioning by African large herbivores. Proc. Natl. Acad. Sci. USA 112, 8019–8024. doi: 10.1073/pnas.1503283112
Kent C., Huh K. M., Hunter S. C., Judson K., Powell L., Sherry T. W. (2022). High resource overlap and small dietary differences are widespread in food-limited warbler communities. Ibis 164, 44–59. doi: 10.1111/ibi.13006
Kent C. M., Sherry T. W. (2020). Behavioral niche partitioning reexamined: do behavioral differences predict dietary differences in warblers? Ecology 101–110, e03077. doi: 10.1002/ecy.3077
Lebrija-Trejos E., Hernández A., Wright S. J. (2023). Effects of moisture and density-dependent interactions on tropical tree diversity. Nature 615, 100–104. doi: 10.1038/s41586-023-05717-1
Leisler B., Winkler H. (1985). Ecomorphology. Curr. Ornithol. 2, 155–186. doi: 10.1007/978-1-4613-2385-3_5
Losos J. (2009). Lizards in an evolutionary tree: ecology and adaptive radiation of anoles (Oxford, UK: Oxford University Press).
MacArthur R. (1958). Population ecology of some warblers of northeastern coniferous forests. Ecology 39, 599–619. doi: 10.2307/1931600
MacArthur R. H. (1969). Patterns of communities in the tropics. Biol. J. Linn. Soc 1, 19–30. doi: 10.1111/j.1095-8312.1969.tb01809.x
MacArthur R. H. (1972). Geographical ecology: patterns in the distribution of species (New York, NY: Harper & Row).
MacArthur R. H., Levins R. (1967). The limiting similarity, convergence, and divergence of coexisting species. Amer. Nat. 101, 377–385. doi: 10.1086/282505
MacArthur R. H., Wilson. E. O. (1967). The theory of island biogeography. Princeton Monogr. Biol. (Princeton, NJ: Princeton University Press).
Marantz C. A., Aleixo A., Bevier L. R., Patten M. A. (2003). “Family dendrocolaptidae (Woodcreepers),” in Handbook of the birds of the world, volume 8: broadbills to tapaculos. Eds. del Hoyo J. A., Elliott A., Christie D. A. (Barcelona: Lynx Edicions).
Marra P. P., Remsen J. V. (1997). Insights into the maintenance of high species diversity in the Neotropics: habitat selection and foraging behavior in understory birds of tropical and temperate forests. Ornithol. Monogr. 48, 445–483. doi: 10.2307/40157547
McPeek M. A. (2017). Evolutionary community ecology (Monographs in Population Biology 58). (Princeton, NJ: Princeton University Press).
McPeek M. A. (2019). Limiting similarity? The ecological dynamics of natural selection among resources and consumers caused by both apparent and resource competition. Am. Nat. 193, E92–E115. doi: 10.1086/701629
McPeek M. A. (2022). Coexistence in ecology: a mechanistic perspective (Monographs in Population Biology 66). (Princeton, NJ: Princetion University Press).
McPeek M. A., McPeek S. J., Fu F. (2022). Character displacement when natural selection pushes in only one direction. Ecol. Monogr. 92, 1–20. doi: 10.1002/ecm.1547
Miller M. J., Bermingham E., Turner B. L., Touchon J. C., Johnson A. B., Winker K. (2021). Demographic consequences of foraging ecology and genetic diversification in Neotropical bird species. Ecol. Letts. 24, 563–571. doi: 10.1111/ele.13674
Mittelbach G. G., Schemske D. W., Cornell H. V., Allen A. P., Brown J. M., Bush M. B., et al. (2007). Evolution and the latitudinal diversity gradient: speciation, extinction and biogeography. Ecol. Lett. 10, 315–331. doi: 10.1111/j.1461-0248.2007.01020.x
Naka L. N., da Silva Costa B. M., Rodrigues Lima G., Claramunt S. (2022). Riverine barriers as obstacles to dispersal in Amazonian birds. Front. Ecol. Evol. 10. doi: 10.3389/fevo.2022.846975
Orians G. H. (1969). The number of bird species in some tropical forests. Ecology 50, 783–801. doi: 10.2307/1933692
Owen-Smith N., Cromsigt J. P. G. M., Arsenault R. (2017). “Megaherbivores, competition and coexistence within the large herbivore guild,” in Conserving Africa’s mega-diversity in the anthropocene. Eds. Cromsigt J. P. G. M., Archibald S., N. Owen-Smith (Cambridge: Camrbidge University Press), 111–134.
Peñuelas J., Fernández-Martínez M., Ciais P., Jou D., Piao S., Obersteiner M., et al. (2019). The bioelements, the elementome, and the biogeochemical niche. Ecology 100, doi.org/10.1002/ecy.2652. doi: 10.1002/ecy.2652
Pigot A. L., Sheard C., Miller E. T., Bregman T. P., Freeman B. G., Roll U., et al. (2020). Macroevolultionary convergence connects morphological form to ecological function in birds. Nat. Ecol. Evol. 4, 230–239. doi: 10.1038/s41559-019-1070-4
Pigot A. L., Jetz W., Sheard C., Tobias J. A. (2018). The macroecological dynamics of species coexistence in birds. Nat. Ecol. Evol. 2, 1112–1119. doi: 10.1038/s41559-018-0572-9
Pigot A. L., Tobias J. A. (2013). Species interactions constrain geographic range expansion over evolutionary time. Ecol. Letts. 16, 330–338. doi: 10.1111/ele.12043
Pigot A. L., Trisos C. H., Tobias J. A. (2016). Functional traits reveal the expansion and packing of ecological niche space underlying an elevational diversity gradient in passerine birds. Proc. R. Soc. B. 283, 20152013. doi: 10.1098/rspb.2015.2013
Pontarp M., Bunnefeld L., Cabral J. S., Etienne R. S., Fritz S. A., Gillespie R., et al. (2019). The latitudinal diversity gradient: novel understanding through mechanistic eco-evolutionary models. Trends Ecol. Evol. 34, 211–223. doi: 10.1016/j.tree.2018.11.009
Powell L. L., Rutt C. L., Mokross K., Wolfe J. D., Johnson E. I., Rodrigues P. F., et al. (2022). Sociality and morphology differentiate niches of 13 sympatric Amazonian woodcreepers (Dendrocolaptinae). Ornithology 139, 1–15. doi: 10.1093/ornithology/ukac002
Prum R. O., Berv J. S., Dornburg A., Field D. J., Townsed J. P., Lemmon E. M., et al. (2015). A comprehensive phylogeny of birds (Aves) using targeted next-generation DNA sequencing. Nature 526, 569–573. doi: 10.1038/nature15697
Ricklefs R. E., Bermingham E. (2001). Nonequilibrium diversity dynamics of the lesser antillean avifauna. Science 294, 1522–1524. doi: 10.1126/science.1065005
Ricklefs R. E., Schluter D. (1993). “Species diversity: regional and historical influences,” in Species diversity in ecological communities: historical and geographical perspectives. Eds. Ricklefs R. E., Schluter D. (Chicago, IL: University of Chicago Press), 350–363.
Robinson W. D. (2022). Grand challenges at the frontiers of bird science. Front. Bird Sci. 1. doi: 10.3389/fbirs.2022.994063
Robinson W. D., Brawn J. D., Robinson S. K. (2000). Forest bird community structure in central Panama: influence of spatial scale and biogeography. Ecol. Monogr. 70, 209–235. doi: 10.1890/0012-9615(2000)070[0209:FBCSIC]2.0.CO;2
Robinson S. K., Terborgh J. (1995). Interspecific aggression and habitat selection by Amazonian birds. J. Anim. Ecol. 64, 1–11. doi: 10.2307/5822
Rosamond K. M., Sherry T. W., Hunter S. C., Kent C. M. (2020). Morphological traits influence prey selection by coexisting species of new world warblers (Parulidae). J. Field Ornithol. 91, 393–408. doi: 10.1111/jofo.12352
Rosenberg K. V. (1997). Ecology of dead-leaf foraging specialists and their contribution to Amazonian bird diversity. Ornithol. Monogr. 48, 673–700. doi: 10.2307/40157560
Roth-Monzon A. J., Belk M. C., Zéñuga-Vega J. J., Johnson J. B. (2020). Beyond pairwise interactions: multispecies character displacement in Mexican freshwater fish communities. Amer. Nat. 195, 983–996. doi: 10.1086/708513
Rutt C. L., Mokross K., Kaller M. D., Stouffer P. C. (2020). Experimental forest fragmentation alters Amazonian mixed-species flocks. Biol. Conserv. 242, https://doi.org/10.1016/j.biocon.2020.108415. doi: 10.1016/j.biocon.2020.108415
Schumm M., White A. E., Supriya K., Price T. D. (2020). Ecological limits as the driver of bird species richness patterns along the east Himalayan elevational gradient. Amer. Nat. 195, 802–817. doi: 10.1086/707665
Şekercioğlu C. H., Daily G. C., Ehrlich P. R. (2004). Ecosystem consequences of bird declines. Proc. Natl. Acad. Sci. USA 101, 18042–18047. doi: 10.1073/pnas.0408049101
Şekercioğlu Ç.H., Mendenhall C. D., Oviedo-Brenes F., Horns J. J., Ehrlich P. R., Daily G. (2019). Longterm declines in bird populations in tropical agricultural countryside. Proc. Natl. Acad. Sci. USA 116, 9903–9912. doi: 10.1073/pnas.1802732116
Şekercioğlu Ç.H., Ehrlich P. R., Daily G. C., Aygen D., Goehring D., Sandi R. F. (2002). Disappearance of insectivorous birds from tropical forest fragments. Proc. Natl. Acad. Sci. USA 99, 263–267. doi: 10.1073/pnas.012616199
Salisbury C. L., Seddon N., Cooney C. R., Tobias J. A. (2012). The latitudinal gradient in dispersal constraints: specialization drives diversification in tropical birds. Ecol. Letts. 15, 847–855. doi: 10.1111/j.1461-0248.2012.01806.x
Schemske D. W., Mittelbach G. G., Cornell H. V., Sobel J. M., Roy K. (2009). Is there a latitudinal gradient in the importance of biotic interactions? Ann. Rev. Ecol. Evol. Syst. 40, 245–269. doi: 10.1146/annurev.ecolsys.39.110707.173430
Schleuning M., García D., Tobias J. A. (2022). Animal functional traits: towards a trait-based ecology for whole ecosystems. Funct. Ecol. 37, 4–12. doi: 10.1111/1365-2435.14246
Schoener T. (1971). Large-billed insectivorous birds: a precipitous diversity gradient. Condor 73, 154–161. doi: 10.2307/1365836
Sheard C., Neate-Clegg M. H. C., Alioravainen N., Jones S. E. I., Vincent C., MacGregor H. E. A., et al. (2020). Ecological drivers of global gradients in avian dispersal inferred from wing morphology. Nat. Comms. 2463, 1–9. doi: 10.1038/s41467-020-16313-6
Sherry T. W. (1984). Comparative dietary ecology of sympatric, insectivorous Neotropical flycatchers (Tyrannidae). Ecol. Monogr. 54, 313–338. doi: 10.2307/1942500
Sherry T. W. (1990). When are birds dietarily specialized? distinguishing ecological from evolutionary approaches. Stud. Avian Biol. 13, 337–352.
Sherry T. W. (2016). “Chapter 8. avian food and foraging,” in The Cornell Lab of Ornithology handbook of bird biology, 3rd Edition. Eds. Lovette I. J., Fitzpatrick J. W. (John Wiley & Sons: West Sussex, UK), pp. 264–310.
Sherry T. W. (2021). Sensitivity of tropical insectivorous birds to the anthropocene: a review of multiple mechanisms and conservation implications. Front. Ecol. Evol. 9. doi: 10.3389/fevo.2021.662873
Sherry T. W., Johnson M. D., Williams K., Kaban J., McAvoy C., Medori A., et al. (2016). Dietary opportunism, resource partitioning, and consumption of coffee-berry borers by five migratory wood warblers (Parulidae) wintering in Jamaican shade coffee plantations. J. Field Ornithol. 87, 273–292. doi: 10.1111/jofo.12160
Sherry T. W., Kent C. M. (2022). Extensions and limitations of MacArthur: a review of ecological and evolutionary approaches to competition and diet in the New World wood warblers (Parulidae). Ornithology 139, 1–17. doi: 10.1093/ornithology/ukac010
Sherry T. W., Kent C. M., Sánchez N. V., Şekercioğlu Ç.H. (2020). Insectivorous birds in the Neotropics: ecological radiations, specialization, and coexistence in species-rich communities. Auk: Ornithol. Adv. 137, 1–27. doi: 10.1093/auk/ukaa049
Soberón J. (2007). Grinnellian and eltonian niches and geographic distributions of species. Ecol. Lett. 10, 1115–1123. doi: 10.1111/j.1461-0248.2007.-1107.x
Sridhar H., Srinivasan U., Askins R. A., Canales-Delgadillo J. C., Chen C. C., Ewert D. N., et al. (2012). Positive relationships between association strength and phenotypic similarity characterize the assembly of mixed-species bird flocks worldwide. Am. Nat. 180, 777–790. doi: 10.1086/668012
Storch D., Bohdalková E., Okie J. (2017). The more-individuals hypothesis revisited: the role of community abundance in species richness regulation and the productivity-diversity relationship. Ecol. Letts. 21, 1–18. doi: 10.1111/ele.12941
Stouffer P. C., Jirinec V., Rutt C. L., Bierregaard R. O. Jr., Hernández-Palma A., Johnson E. I., et al. (2020). Long-term change in the avifauna of undisturbed Amazonian rainforest: ground-foraging birds disappear and the baseline shifts. Ecol. Letts. 24, 186–195. doi: 10.1111/ele.13628
Taper M. L., Case T. J. (1985). Quantitative genetic models for the coevolution of character displacement. Ecology 66, 355–371. doi: 10.2307/1940385
Terborgh J. W. (2015). Toward a trophic theory of species diversity. Proc. Nat. Acad. Sci. 112, 11415–11422. doi: 10.1073/pnas.1501070112
Tobias J. A. (2022). A bird in the hand: global-scale morphological trait datasets open new frontiers of ecology, evolution and ecosystem science. Ecol. Letts. 25, 573–580. doi: 10.1111/ele.13960
Tobias J. A., Cornwallis C., Derryberry E., Claramunt S., Brumfield R., Seddon N. (2014). Species coexistence and the dynamics of phenotypic evolution in adaptive radiation. Nature 506, 359–363. doi: 10.1038/nature12874
Vermeij G. J. (1994). The evolutionary interaction among species: selection, escalation, and coevolution. Ann. Rev. Ecol. Syst. 2, 219–236. doi: 10.1146/annurev.es.25.110194.001251
Visco D. M., Michel N. L., Boyle A. W., Sigel B. J., Woltmann S., Sherry T. (2015). Patterns and causes of understory bird declines from human-disturbed tropical forest landscapes: a case study from central America. W.Biol. Conserv. 191, 117–129. doi: 10.1016/j.biocon.2015.05.018
Visco D. M., Sherry T. W. (2015). Increased abundance, but reduced nest predation in the chestnut-backed antbird in Costa Rican rainforest fragments: surprising impacts of a pervasive snake species. special issue article: tropical insectivores. Biol. Conser. 188, 22–31. doi: 10.1016/j.biocon.2015.01.015
Wainwright P. C., Alfaro M. E., Bolnick D. I., Hulsey C. D. (2005). Many-to-one mapping of form to function: a general principle in organismal design? Integr. Comp. Biol. 45, 256–262. doi: 10.1093/icb/45.2.256
Wiens J. A. (1977). On competition and variable environments. Amer. Sci. 65, 590–597. Available at: https://www.jstor.org/stable/27848086
Keywords: arms race, enhancement, foraging, insectivore, latitude, niche, specialization, tropics
Citation: Sherry TW (2023) Niche concept scale in space and time: evolutionary perspectives from tropical insectivorous birds. Front. Ecol. Evol. 11:1197920. doi: 10.3389/fevo.2023.1197920
Received: 31 March 2023; Accepted: 13 June 2023;
Published: 03 July 2023.
Edited by:
Britta Uhl, Goethe University Frankfurt, GermanyReviewed by:
Monte Neate-Clegg, University of California, Los Angeles, United StatesW. Douglas Robinson, Oregon State University, United States
Copyright © 2023 Sherry. This is an open-access article distributed under the terms of the Creative Commons Attribution License (CC BY). The use, distribution or reproduction in other forums is permitted, provided the original author(s) and the copyright owner(s) are credited and that the original publication in this journal is cited, in accordance with accepted academic practice. No use, distribution or reproduction is permitted which does not comply with these terms.
*Correspondence: Thomas W. Sherry, dHNoZXJyeUB0dWxhbmUuZWR1